- 1Department of Psychology, The Graduate Center, City University of New York, New York, NY, United States
- 2Fundación Internacional para la Naturaleza y la Sostenibilidad, Chetumal, Mexico
- 3Lab of Integrative Neuroscience, The Rockefeller University, New York, NY, United States
- 4Department of Psychology, Hunter College, City University of New York, New York, NY, United States
Unmanned aerial systems (UASs) are powerful tools for research and monitoring of wildlife. However, the effects of these systems on most marine mammals are largely unknown, preventing the establishment of guidelines that will minimize animal disturbance. In this study, we evaluated the behavioral responses of coastal bottlenose dolphins (Tursiops truncatus) and Antillean manatees (Trichechus manatus manatus) to small multi-rotor UAS flight. From 2015 to 2017, we piloted 211 flights using DJI quadcopters (Phantom II Vision +, 3 Professional and 4) to approach and follow animals over shallow-water habitats in Belize. The quadcopters were equipped with high-resolution cameras to observe dolphins during 138 of these flights, and manatees during 73 flights. Aerial video observations of animal behavior were coded and paired with flight data to determine whether animal activity and/or the UAS's flight patterns caused behavioral changes in exposed animals. Dolphins responded to UAS flight at altitudes of 11–30 m and responded primarily when they were alone or in small groups. Single dolphins and one pair responded to the UAS by orienting upward and turning toward the aircraft to observe it, before quickly returning to their pre-response activity. A higher number of manatees responded to the UAS, exhibiting strong disturbance in response to the aircraft from 6 to 104 m. Manatees changed their behavior by fleeing the area and sometimes this elicited the same response in nearby animals. If pursued post-response, manatees repeatedly responded to overhead flight by evading the aircraft's path. These findings suggest that the invasiveness of UAS varies across individuals, species, and taxa. We conclude that careful exploratory research is needed to determine the impact of multi-rotor UAS flight on diverse species, and to develop best practices aimed at reducing the disturbance to wildlife that may result from their use.
Introduction
Unmanned aerial systems (UASs) are cost-effective and powerful remote-sensing tools used by scientists and wildlife managers to aerially monitor animals and their habitats (Anderson and Gaston, 2013; Linchant et al., 2015). UAS enable similar surveys as manned flight to detect and identify species in remote and inaccessible locations, but at a reduced risk to researchers and improved capacity to collect data for detailed analyses (see Linchant et al., 2015 for review). Aircrafts are often equipped with multiple sensor packages, which enables the simultaneous acquisition of multimodal data such as high-resolution imagery and geospatial data.
UAS have been successfully used to collect biological data on many marine megafauna species (e.g., Kiszka et al., 2016; Johnston et al., 2017). Oftentimes the collection of these data was previously only possible with the use of manned aircraft, close watercraft approaches, and/or invasive sampling methods (e.g., Koski et al., 2009, 2015; Christiansen et al., 2016a). Successful uses of UAS in marine mammal research have included aerial surveys for animal detection, abundance estimation of pinnipeds in breeding colonies, photo-identification of whales, and photogrammetric assessments of body condition and population health (e.g., Koski et al., 2009, 2015; Christiansen et al., 2016a; Adame et al., 2017; Krause et al., 2017). Fixed-winged UAS are most often used to detect and count animals during high-altitude long-distance surveys over large areas for estimates of population abundances and distributions (e.g., Hodgson et al., 2013; Adame et al., 2017). Conversely, low-altitude flights and stable hovering with multi-rotor aircrafts enable close approaches directly to animals, for example, to collect exhaled breath condensate (blow) for health assessments of large whales (e.g., Acevedo-Whitehouse et al., 2010; Apprill et al., 2017; Pirotta et al., 2018). Several studies suggest that UAS result in reduced disturbance to marine mammals when compared with traditional research methods (Acevedo-Whitehouse et al., 2010; Moreland et al., 2015; Arona et al., 2018). Therefore, it is important for researchers to develop effective strategies to safely apply UAS to monitor wildlife species to minimize the risk of negative impacts (Chabot and Bird, 2015; Vas et al., 2015; Smith et al., 2016).
A wide range of species has been documented to exhibit disturbance behaviors to UAS operations in response to UAS (e.g., seabirds, crocodiles, sea turtles, terrestrial and marine mammals; Rümmler et al., 2016; Brisson-Curadeau et al., 2017; Mulero-Pázmány et al., 2017; Bevan et al., 2018). Among marine mammals, pinnipeds exhibited rapid group dispersal following multi-rotor UAS approaches (Pomeroy et al., 2015; Sweeney et al., 2015), but were largely unaffected by fixed-wing UAS flying at high altitudes (Arona et al., 2018), where aircrafts may be relatively undetectable by most wildlife. Multi-rotor UAS operated at altitudes of 9–200 m did not elicit observable behavioral responses in studies of toothed whales (e.g., sperm whales Physeter macrocephalus: Acevedo-Whitehouse et al., 2010; killer whales Orcinus orca: Durban et al., 2015) and baleen whales (e.g., blue whales Balaena mysticetes; gray whales Eschrichtus robustus: Acevedo-Whitehouse et al., 2010; humpback whales Megaptera novaeangliae: Christiansen et al., 2016a). UAS flight had no detectable effects on blue whale respiration or diving behavior during blow collection, but one whale appeared to briefly look up at the UAS (Domínguez-Sánchez et al., 2018). Humpback whales and southern right whales (Eubalaena australis) rarely reacted when approached with multi-rotor UAS at altitudes of ~4 m during blow collection, but they sometimes exhibited a “bucking” response or a turn of the body toward the aircraft (Kerr et al., 2016). In a different study these two species were not observed to respond to close approaches (<10 m) of a similar aircraft (Christiansen et al., 2016a). Differences in species responsivity can be related to a variety of factors. The type of aircraft in use (e.g., fixed-wing vs. multi-rotor), the flight patterns of the UAS (e.g., hovering or active-search), the proximity of the aircraft to animals, and the directness of its approach may all affect study subjects differently (e.g., Pomeroy et al., 2015; McEvoy et al., 2016; Mulero-Pázmány et al., 2017). This provides incentive to develop species-specific “best practices” for the use of UAS. This includes characterizing the short-term effects of these systems on wildlife to establish criteria for avoiding disturbance (Hodgson and Koh, 2016; Smith et al., 2016).
To date, little work has been done to determine the effects of UAS on delphinid and sirenian species or to evaluate their efficacy for conducting behavioral research on them. Smith et al. (2016) reported an observation of bottlenose dolphins (Tursiops truncatus) chasing the shadow of a multi-rotor UAS flying at ~20 m altitude. Nowacek et al. (2001) reported similar responses in one bottlenose dolphin in which it briefly avoided (<10 s) the shadow of a helium-filled aerostat balloon as it passed overhead during behavioral follows. Hodgson (2007) reported that dugongs (Dugong dugon) fled from the shadow of a similar aerostat balloon. Studies using fixed-wing UAS flown at high altitudes (100–300 m) did not detect responses in Florida manatees (Trichechus manatus latirostris; Jones et al., 2006) or dugongs (Hodgson et al., 2013). Information on how to best apply these systems for use on dolphins and sirenians can be beneficial to species monitoring, however it is necessary to first evaluate in the field, how animals respond to its different uses.
During ongoing studies in Belize, we investigated the behavioral responses of bottlenose dolphins and Antillean manatees (T. m. manatus) to small multi-rotor UAS flights in shallow coastal habitats. Dolphins and manatees were located using several different flight strategies and were approached and followed at varying altitudes to determine whether these factors influenced animals' responses to the aircraft.
Methodology
Study Populations and Data Collection
UAS flights to track and observe bottlenose dolphins and Antillean manatees were conducted in Belize from 2015 to 2017. Data were gathered throughout the year in both the wet and dry seasons. Dolphins were found in several shallow marine ecosystems (mean water depth = 3.6 m, range = 0.5 to 20 m) in both coastal and offshore regions of Belize (Figures 1A–C), mostly at Turneffe Atoll Marine Reserve (Figure 1C). Turneffe is an offshore marine atoll 36 km east of the mainland coast where a year-round, small population of resident and non-resident dolphins inhabit shallow seagrass lagoons enclosed by mangrove cayes and a fringing coral reef system (Campbell et al., 2002; Dick and Hines, 2011; Ramos et al., 2016, 2018). Flights with manatees were primarily conducted on the leeward side of St. George's Caye (Figure 1B), located 9.5 km east of mainland Belize near the Belize Barrier Reef. The clear shallow waters of our study area are sheltered by islands and predominantly seagrass habitats which allowed us visibility to the seafloor (up to 5 m) and to observe animals continuously for the duration of most flights.
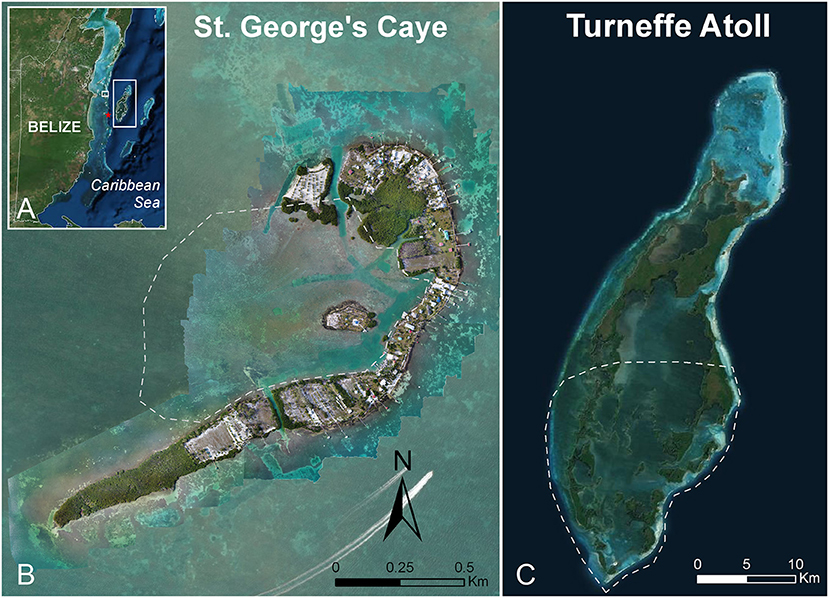
Figure 1. (A) Map of Belize. (B) St. George's Caye, located east of mainland Belize near the Belize Barrier Reef, with overlaid orthomosaic maps. (C) Turneffe Atoll Marine Reserve is located in the offshore waters of Belize. Gray dashed lines outline study areas. The red star denotes flights conducted outside of the main study. Map Source: Esri, DigitalGlobe, GeoEye, Earthstar Geographics, CNES/Airbus DS, USDA, USGS, AeroGRID, IGN, and the GIS User Community.
Three small (1,240–1,400 g) commercial quadcopter UAS were used: the DJI Phantom 2 Vision + (P2), Phantom 3 Professional (P3), and Phantom 4 (P4). All models were white and had very similar structure and appearance. Each model was equipped with a 4K camera mounted on a gimbal with 3-axis stabilization. High-definition video was recorded to an onboard microSD card in MP4 format. To reduce surface glare that restricts visibility into the water, a linear polarizer was placed on the camera lens of each aircraft, and the camera was angled downward at 45–90° toward focal animals. The aircraft was piloted by ER with a remote-control joystick. Monitoring of the live video feed, as well as relevant flight metrics (e.g., battery levels, distance of the aircraft from the pilot), was performed using the DJI GO application on a tablet (Samsung Galaxy 8 or iPad 9) mounted to the remote control.
The aircrafts were launched from shore or from a small boat with the help of a ground station operator who held the aircraft (P2, P3, or P4) overhead prior to launching it. The pilot remotely controlled the aircraft to distances of < 2 km. Flights were between 5 and 22 min in duration and were performed in non-rainy conditions at wind speeds from 0 to 35 kt. Flight movements were categorized as: (i) direct approaches: vertical descents toward animals at speeds of 0.3–1.0 m/s; (ii) horizontal follows: altitude-stable horizontal flight in an effort to closely match animal movement, at flight speeds of 0–5 m/s; or (iii) hovering: stable hovering in place over animals.
Bottlenose Dolphin Flights
Dolphin responses were recorded opportunistically during aerial focal and group follows conducted from small boats and from shore (n = 3). Boat surveys to locate dolphins were conducted from several small vessels (6–15 m long) equipped with one or two outboard engines (85–250 HP). When dolphins were sighted, the vessel approached the group's position slowly (to within 30 m) to collect images for photo-identification using a SLR camera with a telephoto lens (75–400 m). Groups were defined as all dolphins <100 m of each other (Shane, 1990). One dolphin or dolphin group was approached and followed continuously for the entire flight. Group size was measured initially from the boat and verified by counting dolphins in aerial video. Dolphins were classified as adults, juveniles/sub-adults, or calves by relative size and based on previous identifications. Sex was determined by viewing the genital region or by repeated observations of an adult with a calf (Mann et al., 2000). After photo-ID was completed, the UAS was launched to an altitude of 20–50 m and navigated over animals. Once over the animals, the aircraft maintained its initial hovering altitude (50, 40, or 30 m) for 30–180 s, then descended vertically (at 5–10 m intervals) in a direct approach until reaching a stable altitude of either 20, 15, or 5 m. Up to six flights were conducted with each group. There was a maximum of 4 vertical descents per flight. To minimize the behavioral effects of the research vessel, during the majority of flights, the boat remained at a distance of 100–1,500 m away from the focal dolphin(s).
Antillean Manatee Flights
Manatees were sighted during aerial transects using the P3 and P4 models deployed and remotely controlled from shore as a part of ongoing studies and monitoring of local animals (Ramos et al., 2017). Once sighted, animals were randomly selected for UAS exposure. Groups were defined as all manatees <10 m of each other. Multiple different individual manatees and manatee groups were sometimes sighted during a single flight. The aircraft was initially flown to an altitude of 100 m to locate manatees then descended to 60, 50, 40, or 30 m before hovering over manatees for 30–120 s. The UAS descended again and remained at 20, 15, 10, or 5 m during horizontal follows for a maximum of 5 descents per flight. There was evidence from preliminary data collected in 2016 that manatees changed their direction in response to the aircraft's flight path. Overhead flights were conducted to test the hypothesis that UAS flight causes manatees to evade the aircraft. The aircraft was flown in a straight line over the manatees and perpendicular to their swim direction.
Automated UAS imaging flights were conducted on 7 days in 2017 at St. George's Caye using the DJI GS Pro application to gather high-resolution photos that were stitched together to build georectified orthomosaic maps of manatee habitats (Figure 1B). The aircraft was flown to its starting point at an altitude of 150 m and flew autonomously in a saw-toothed transect pattern for distances of 3–7 km capturing one 12 MP (4.1 cm/pixel) image at preselected waypoints. Maps were compiled in OpenDroneMap (www.opendronemap.org) and WebODM (www.webodm.org).
Data Analysis
Information was extracted from the flight data logs to determine flight effort and examine behavioral responses. The onboard GPS logged a waypoint every 100 ms and stored associated information on altitude (m), speed (m/s), and distances traveled (m). The actual altitude of flight was adjusted according to the height of deployment at 2 m above sea level in boat-based deployments. The website www.airdata.com was used to generate flight reports. We suspected that high wind speeds might raises the chances of animals responding to the UAS, because high windspeeds increase the noise of the rotors (Christiansen et al., 2016b). To test this hypothesis, mean wind speed and maximum gust speed (m/s) per flight were examined to identify if high wind speeds increased the likelihood of animals responding to the UAS.
Flight tracks were examined in Google Earth Pro to ground-truth measurements of manatees' positioning and travel distances (m). Habitats at sighting locations were categorized as follows: seagrass bed (dense or patchy), lagoon, channel, channel edge, cove, reef, or resting holes (holes in the substrate where manatees frequently rest; Bacchus et al., 2009).
Aerial videos of dolphins and manatees were initially reviewed using QuickTime 10.4 Media Player (Apple Inc.,). Animal activity data (including location, behavior, and position in the water column) was coded using GriffinVC Behavioral Coding Software (www.github.com/svirs/griffinVC). Response events (RE) were defined as events during which, in the apparent absence of other stimuli, one or more animals appeared to change their behavior following apparent detection of the aircraft. RE began when dolphins visibly changed their behavior in response to the aircraft, and ended when they either returned to pre-response behavior or began a different activity (Table 1), and sometimes included repeated UAS-orienting events. Disturbance was defined as RE in which animals exhibited potentially negative responses, such as increased vigilance, flight responses, and changes in short-term movement patterns (Gill, 2007).
Dolphins were identified according to standard photo-ID techniques (Defran et al., 1990) by matching dorsal fin photos to a catalog of known dolphins in Belize (Campbell et al., 2002; Ramos et al., 2018). We determined the minimum number of different dolphins exposed to the UAS throughout the study instead of the exact number because it was sometimes not possible to identify all dolphins when they were in large groups. To assess dolphins' initial response to the UAS, the first flight over each group (n = 48) was considered separately from subsequent flights.
Aerial videos of dolphins were analyzed to assess if bottlenose dolphins changed their behavior in response to the UAS. We identified RE when dolphins exhibited response behaviors such as orienting and turning toward the aircraft in a fashion uncharacteristic to the behavioral state they were in prior to approach. Table 1 is an ethogram—a catalog of relevant behaviors—lists the non-response and response behaviors exhibited by dolphins. Response behaviors typically involved upward rostrum- and eye-directed movements to the aircraft and repeated changes in position and body orientation. Dolphin observations were first analyzed to identify possible responses to the aircraft using ad libitum sampling of behavior (Altmann, 1974). Behavior states pre-and post-response were identified using continuous sampling. The behavior of individual responding dolphins was coded using all-event sampling to identify each occurrence of seven UAS-oriented behaviors (Table 1): “side-roll,” “full-roll,” “belly-up,” “rostrum-up,” “circular swim,” “spin-and-orient,” and “breach.” The “side-roll,” “circular swim” and “breach” were further characterized using modifiers that indicated whether the dolphin performed the activity with an open mouth, while swimming upside down, or while twisting along the body's longitudinal axis, as these behavioral modifications were occasionally observed.
Dolphin swim direction was scored as left or right in cases that the dolphin executed a turn as part of a response to the UAS. Bodily rotation along the longitudinal axis was scored as clockwise or counterclockwise. Visible increases or decreases in dolphins' swim speed following their initial detection were noted. Direct responses to the boat and instances of social behavior were especially likely to be misidentified as UAS-directed behavior because dolphins at this site typically interact with vessels at first approach. We took special care in noting these behaviors and excluded animals' behavior within the first 5 min of sighting a dolphin group, a period when dolphins are frequently observed interacting with the boat (Ramos, personal observation).
High-resolution images of manatees were used to photo-ID individuals with identifying features (primarily scarring) across their bodies and classify age and sex where possible. Images were taken from screenshots of aerial video (taken at altitudes of 6–60 m) in which most of the manatee's body was visible and exposed or just beneath the water's surface (Figure 2). Multiple images taken at different angles during a single sighting and over the course of the study were examined for individually-distinctive features located anywhere on the body including: trunk, head, left and right dorsal, and ventral surfaces, and tail (e.g., Flamm et al., 2000; Langtimm et al., 2004). Manatees were classified as adults, juveniles/sub-adults, or calves based on their relative size. Females were identified by the presence of a closely-associated calf (e.g., O'Shea and Langtimm, 1995; Langtimm et al., 2004). Identified individuals were compiled into a photo-ID catalog for the study, which was used to determine the total number of manatees exposed to the UAS and re-identify animals. Manatees that could not be identified because of insufficient markings or poor visibility were excluded from analysis for response behaviors.
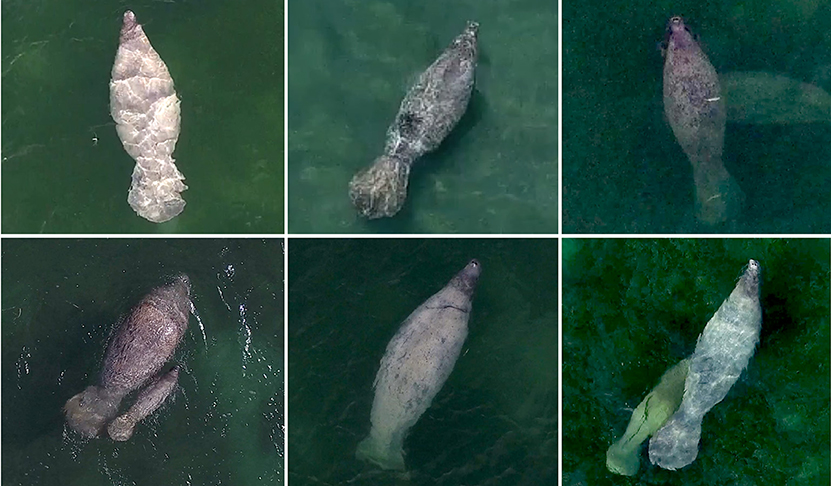
Figure 2. Aerial images of Antillean manatees illustrating identifying features used for photo-identification.
Manatee behavior was sampled continuously from the beginning of observations until animals were out of sight. To identify if and how manatees changed their behavior after UAS detection, their activity was coded using the states and events described in Table 2. RE were identified when a manatee exhibited rapid shifts in behavior that differed from its pre-exposure activity state, including flight responses. We sampled their behavior continuously for up to 20 min after the initial sighting to accurately document the duration of their responses and detect if they responded multiple times to the aircraft. Changes in behavior and/or swim direction immediately following aircraft movements were considered responses.
Each manatee's swim distance (m) was measured from the location of their initial RE to their location at 1-min post-RE. To perform this measurement, the GPS track for each was overlaid in Google Earth Pro onto the high-resolution map we generated, and the distance between these two location points was measured using the path tool. The exact locations of manatees were ground-truthed by visual review of animal position and static habitat features (e.g., sand patches, the edges of seagrass patches) in videos and the map. We used this method to identify manatee swim direction before aircraft movements, then documented any changes in their swim direction during or directly after these overhead movements relative to the aircraft's direction of flight.
Statistical Analyses
Statistics were conducted in GraphPad Prism version 7.00 for Windows (GraphPad Software, La Jolla California USA, www.graphpad.com) and Microsoft Excel 2016. The duration of time that individuals spent responding to the UAS, and the proportion of this response time relative to overall observation time per flight, were calculated for each response event for both species. The proportions of all flights in which dolphins or manatees responded or did not respond to the UAS were compared using a Chi-Square test at p < 0.05. The proportions of individual dolphins responding vs. not responding to the UAS were compared using a Chi-Square test at p < 0.05. Only the first UAS flight for each dolphin group was considered in this comparison). The same test was performed for manatees. The duration of dolphins and manatee responses in their first confirmed response to the UAS (to account for the observation of specific individuals responding on several occasions) were compared between species with a nonparametric Mann–Whitney U-test at p < 0.01. Mean wind speeds and maximum gust speeds were compared across non-response and response flights within species using independent-samples t-tests to determine whether higher wind speeds were associated influence animals' responsiveness to the UAS as p < 0.05.
Results
Bottlenose Dolphin Responses
We included 56 sightings of dolphins in our analysis. These flights took place on 48 days in 2015 (n flights = 53), 2016 (n = 71), and 2017 (n = 14). Flights were on average 14.7 min in length (SD = 2.6 min), ranging from 3.0 to 17.4 min, for a total of 33.3 h of flight time. Of this total flight time, we directly observed dolphins for a total of 31.4 h. In most sightings, multiple flights were conducted with each group. The groups we observed contained a mean of 5.14 dolphins (SD = 3.8) and ranged from 1 to 17 animals. Flight altitudes across all flights ranged from 5 to 100 m, with a mean of 20.74 m.
Dolphins responded briefly to the UAS in 8 RE. Characteristics of dolphin RE are described in Tables 3, 4. Responses were of short duration and largely consisted of turning and upward-directed orienting behaviors (shown in Figures 3A–F and Supplementary Video 1). We observed possible responses to the UAS during 10 other flights; however, these behaviors occurred simultaneously with social interactions, and potential response behaviors were difficult to distinguish from social behavior directed at conspecifics. Dolphins briefly changed their pre-response behavior state then quickly returned to pre-response or a different behavior state. These sometimes involved vessel interactions directly before responses and less so after in which animals actively approached vessel. Dolphins were more likely to respond in the first flight with each dolphin group. Responses were detected in 12.5% of the first flights with each dolphin group, and 85% (n = 6) of all responses occurred during these initial flights.
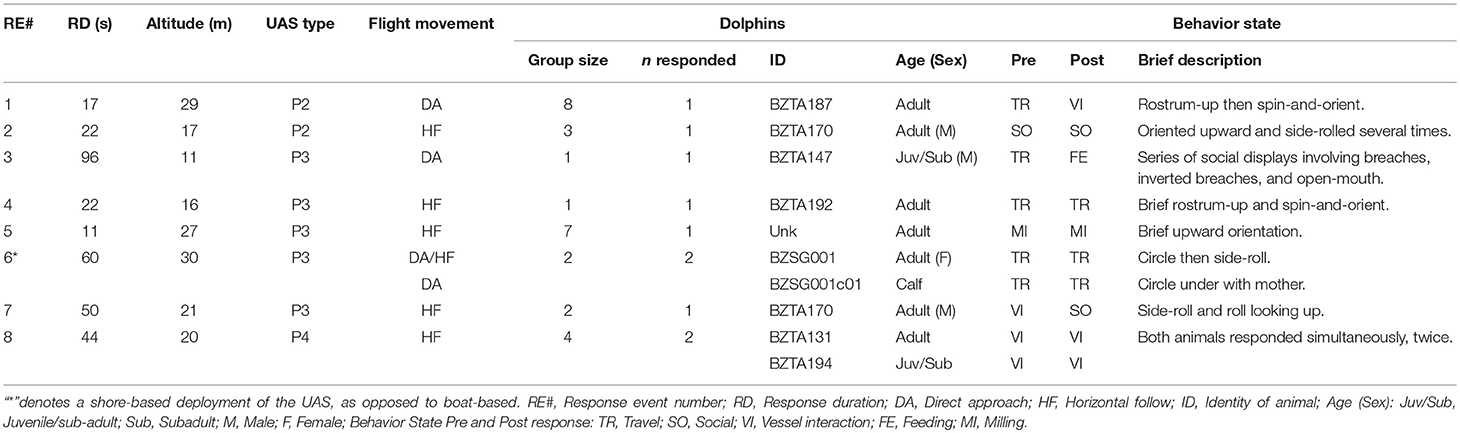
Table 3. Characteristics of response events of bottlenose dolphins to small unmanned aerial systems.

Table 4. Characteristics of bottlenose dolphins and Antillean manatee responses to small unmanned aerial systems.
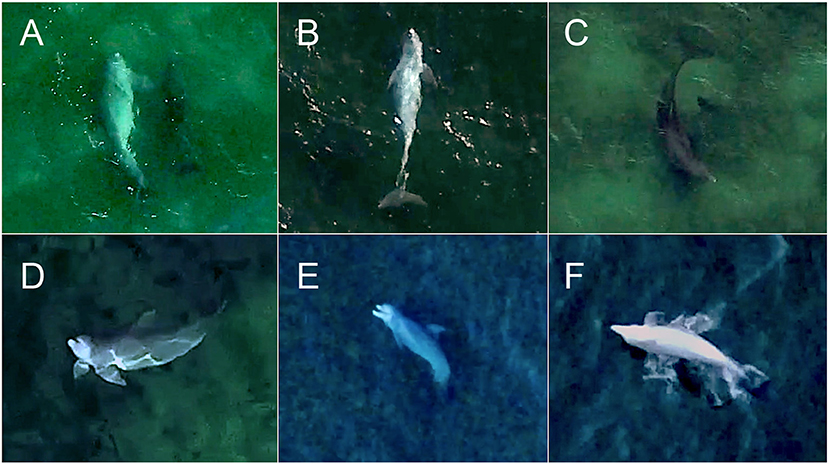
Figure 3. Examples of bottlenose dolphin responses to a small multi-rotor unmanned aerial system. Response behaviors included (A) side-roll, (B) belly-up, (C) circular swim (left), (D) spin-and-orient, (E) side-roll (open-mouth), and (F) breach (inverted). Descriptions of behaviors are in Table 1.
We identified, at minimum, 68 different dolphins across these sightings. Responses to the UAS were detected in 9 of these dolphins, 13.2% of the animals we identified (Table 4). Most responding animals were adults, but two subadults and one calf also showed reactions to the UAS (Table 3). The responding groups ranged in size from 1 to 8 dolphins, but most responses only involved a single dolphin or pairs. Dolphins slightly decreased their swim speed during most RE (n = 5), increased in one RE, and maintained their swim speed in two RE. The most frequently observed behaviors were upward-orienting behaviors like the side-roll, the belly-up, and the rostrum-up (Figure 4). Across response behaviors that involved rotations (n = 34), dolphins turned left in 71% of behaviors and turned right in 29% of behaviors. Circular swims involved clockwise turns in one RE (11%) and counterclockwise turns in 8 RE (88.9%). Only one dolphin exhibited possibly agonistic display behavior: an open mouth and repeated breaches (RE# 3).
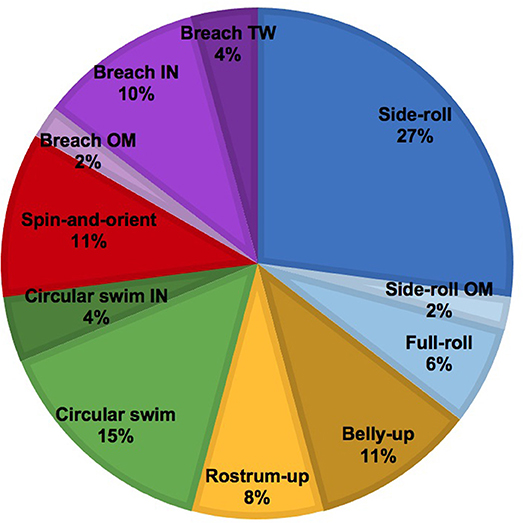
Figure 4. Pie chart illustrating the relative proportion of each response behavior of bottlenose dolphins. Modifiers: OM, Open-mouth; IN, Inverted; TW, Twist.
Dolphins responded to UAS flown at a mean altitude of 19.65 m (Table 4). The stacked histogram that shows the number of dolphin and manatee responses in Figure 5 illustrates our finding that most dolphin responses occurred in a narrow range of low altitudes, while manatee responses occurred at a broader range. Most dolphin responses occurred during horizontal follows by the UAS (n = 9), one occurred during vertical descent, and one during ascent. All dolphins began visibly responding when their bodies were fully underwater, and no parts exposed to the surface. The average latency of dolphins' response, from the time of initial UAS exposure to the start of dolphin RE, was 166 s (SD = 2.75 s), and varied across animals from 3 to 455 s. The majority of RE in dolphins occurred in response to the P3; however, this may be due to the fact that the P3 was flown more than the P2 or P4, rather than being due to differences between the aircraft (Table 3).
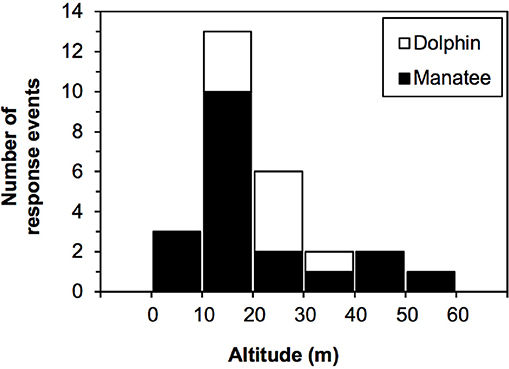
Figure 5. Stacked histogram of the number of response events to the aircraft in bottlenose dolphins and Antillean manatees.
Mean wind speed (mean across all flights = 5.26 m/s, SD = 2.96 m/s) and maximum gust speed (mean = 6.65 m/s, SD = 3.88 m/s) during dolphin response flights were slightly lower than for non-response flights (wind speed: mean = 5.58 m/s, SD = 2.63 m/s; max gust: mean = 6.92 m/s, SD = 3.27 m/s). However, these differences were not significant at p < 0.05.
Antillean Manatee Responses
We included 83 sightings of manatees in our analysis. In contrast to dolphin flights, multiple distinct solitary manatees and small groups were followed in most flights. These flights took place on 16 days in 2016 (n flights = 20) and 2017 (n = 53). Flights were a mean of 17.1 min in length (SD = 1.8 min) and ranged from 14.3 to 20.3 min, for a total of 24.3 h of flight time. Of this total flight time, we directly observed manatees for a total of 22.6 h. Flight altitudes across all flights ranged from 5 to 120 m, with a mean of 43.79 m.
A total of 83 different individual and groups of manatees were exposed to the UAS. Thirty-three of these sightings involved single manatees, and 50 involved groups of ≥2 manatees. We sighted 146 manatees, with 84 adults (mean per group = 1.01, SD = 1.3, range = 1–4), 36 juveniles/sub-adults (mean = 0.43, SD = 0.31, range = 0–2), and 26 calves (mean = 0.31, SD = 0.49, range = 0–2). Manatee groups contained a mean of 1.77 manatees (SD = 1.3), ranging from 1 to 6 animals. Photo-ID analysis revealed these 146 manatees consisted of a minimum of 66 and a maximum of 71 distinct individuals accounting for repeated sightings. Some manatees could not be identified because of a lack of scarring or other definable features (n = 5) indicating some repeated flyovers were undetected. We randomly selected individual manatees for exposure to the UAS, but several manatees (n = 17) in addition to these animals were inadvertently exposed to the aircraft because the individual manatees could not be identified at the time of UAS flyover in the lower resolution tablet view. Individual manatees were exposed to the UAS a mean of 2.1 times (SD = 3.2; range = 1–17 times).
Manatee responses were detected in 24% of all exposures (n = 20), and the characteristics of these responses are described in Table 4. Manatees responded by quickly changing their behavior; often a manatee would execute a powerful tail-kick (a movement which raised a large plume of silt), and swim quickly away from the area. Many animals continued to respond for several minutes (see Figures 6A–D and Supplementary Video 2). A total of 29 identified manatees responded to the UAS, in a total of 36 detected manatee responses, that included repeated responses of some animals. Twenty-three of these manatees appeared to respond directly to the UAS. In other cases, the responses of these directly-responding manatees appeared to cause behavior change in one to four nearby manatees. More adult manatees responded to the UAS (n = 14) than did juveniles/sub-adults (n = 8) or calves (n = 7), but adults were exposed at higher rates. Calves likely responded because of their mothers. Responses between individuals and groups was highly variable; one mother/calf pair was exposed 17 times but only responded in the first UAS approach, while one juvenile/subadult manatee that was exposed 14 times responded with 11 RE.
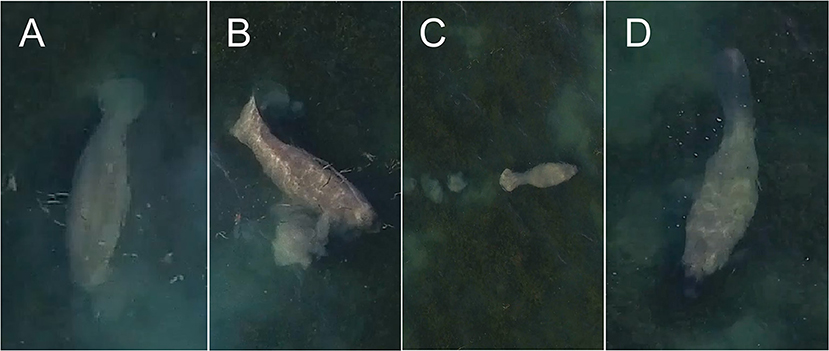
Figure 6. Sequence of response behaviors of an Antillean manatee exposed to a small unmanned aerial system. (A) The feeding manatee was stationary pre-response. (B) Upon detecting the aircraft during direct vertical approach, the manatee began to swim away rapidly (B) leaving a large silt plume and (C) fleeing the area. In some flights, (D) aircraft movements caused manatees to directionally evade the aircraft's flight path.
The UAS caused strong disturbance responses in manatees. In short, the animals that responded fled the aircraft, and they directionally evaded the UAS when pursued. Every manatee that directly responded to the UAS (n = 23) changed their behavior to fleeing. Of these animals, 13 (44.8% of all responding individuals) responded to direct UAS approach upon their first exposure to the aircraft. These animals, which we presume had no previous UAS experience, all changed their behavior following detection of the aircraft from either feeding (n = 11) or milling (n = 2) to fleeing. They included adults (n = 7), juveniles/sub-adults (n = 5), and a single calf. Most of the manatees that appeared to react because of a directly-responding manatee also changed their behavior to fleeing. All manatees responded close to the onset of initial UAS exposure, with an average response latency of 33.4 s (SD = 33.2 s) which ranged from 0 to 120 s. The proportion of manatees (N = 36) that were moving at the onset of the exposure (52%), as opposed to remaining in one place, increased to 69% immediately after exposure. Responding manatees spent an average of 80% of the total flight observation time fleeing the aircraft (SD = 0.18, range = 13–97%). By 1 min after the initial response, manatees that were still responding (n measured = 19) fled a mean distance of 258 m (SD = 163.6 m, range = 2.2–582.0 m). Fleeing manatees typically swam across shallow seagrass flats; 10 manatees fled into the deeper waters of a nearby channel in 4 different RE; and 4 manatees fled into nearby resting holes in 3 different RE.
Animal-directed aircraft movements were more likely to cause responses than either stable hovering or horizontal follows. Manatees responded to direct aircraft approaches (vertical descents) at altitudes of 6–52 m (Figure 5). In flights with no responses (n = 63), manatees were tracked for a total of 14.72 h of horizontal follows, with no signs of UAS-induced behavioral changes. Responses to the P4 (n = 12) were more frequent than to the P3 (n = 8), but manatees were exposed primarily to the P4 (n = 63). More responses occurred during direct approaches (70%; n = 14 RE) than during stationary hovering (30%; n = 6 RE). Most responding manatees (95%) were full underwater when they first responded.
UAS flight over manatees after their initial responses consistently elicited changes in swim direction. Changes in manatee swim direction were identified in 77.8% of RE (n = 14). After their first response in 18 RE, manatees responded to 96.2% of overhead flight movements by changing their swim direction by 45–90° relative to the trajectory of the aircraft. Manatees typically responded < 5 s of the aircraft flying overhead at an altitude range of 6–104 m and continued to respond to each overhead pass with directional evasion. Manatees directionally evaded the aircraft despite aircraft descents of 10 m and ascents of up to 98 m (Figure 7).
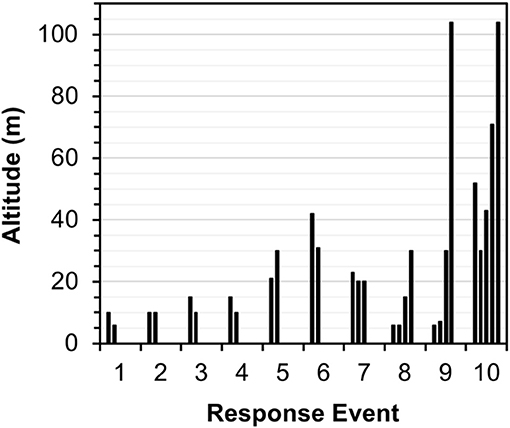
Figure 7. Altitude of Antillean manatee response events to directional overhead UAS flight. Manatees directionally evaded the aircraft following multiple overhead movements despite ascents to 104 m. The number of responses is listed from the first (initial) to the fifth individual response.
Mean wind speed (mean across all flights = 6.56 m/s, SD = 3.3 m/s) and maximum gust speed (mean = 9.5 m/s, SD = 5.01 m/s) were higher in manatee response flights compared to non-response flights (wind speed: mean = 5.02 m/s, SD = 3.26 m/s; max gust: mean = 7.35 m/s, SD = 4.74 m/s). However, these differences were not significant at p < 0.05.
Comparison of Dolphin and Manatee Responses
Manatees were more likely to respond to the UAS than dolphins, and they displayed stronger responses. The difference in frequency of response between the two species was significant, with dolphins responding during 0.05% of UAS flights, vs. manatees which responded in 26% of their flights (p < 0.01, X2 = 15.7196, df = 1). A greater number of individual manatees responded to the UAS than did individual dolphins (Table 4). At minimum, excluding repeated exposures, 19.2% of all manatees observed responded to the UAS, and 10.3% of dolphins responded. However, this difference was not found to be statistically significant (p = 0.1669, X2 = 1.9109, df = 1).
The duration of responses (both on average, and in total) was longer for manatees than for dolphins, both in individual RE and across all events (Table 4). When controlled for individual identity and repeated responses, manatees responded for significantly longer durations than did dolphins within the first UAS flight per group (Mann–Whitney U-test, U = 1, p = 0.00006).
Dolphin RE occurred at lower altitudes, both on average and in comparison of altitude range, than for manatees (Figure 5), but a Mann–Whitney U-test revealed these differences were not significant (p = 0.1521).
Discussion
Our findings document the behavioral responses of coastal bottlenose dolphins and Antillean manatees to small multi-rotor UAS. Aircraft activity caused different behavioral responses in dolphins and manatees that depended on both flight- and animal-related factors. Both species reacted to UAS flights at a broader range of altitudes than previously reported for marine mammals (e.g., Pomeroy et al., 2015). Only a small subset of the dolphins that we tested responded to the aircraft, and that tended to be when they were directly approached and followed. Dolphins who did react to the UAS changed their behavior briefly, orienting toward the aircraft before returning to their pre-response activity. Only one dolphin responded in a way that appeared potentially negative, indicating a possible disturbance. In contrast, many Antillean manatees exhibited strong disturbance behaviors in response to our aircraft. Those that reacted rapidly fled the area. Manatees responded for significantly longer durations than dolphins, in a higher proportion of flights, and with more severe disturbance responses indicating they were more sensitive to UAS and their effects. Most disturbed manatees continually evaded the pursuing UAS until the end of is flight, changing direction repeatedly as the aircraft flew over them at high altitudes. Flying the aircraft directly over disturbed manatees during the post-response period consistently provoked the animals to change direction, indicating heightened vigilance and avoidance. These findings suggest that multi-rotor UAS, in Belize and possibly elsewhere, are a more disruptive stimulus for Antillean manatees than for bottlenose dolphins.
Responses of Bottlenose Dolphins and Antillean Manatees
Dolphins exhibited low overall responsiveness throughout all UAS flights. Animals only reacted in a small proportion of observations. When they did appear to notice and respond to the aircraft, the duration of their responses was short and animals seemed minimally impacted. Dolphins' responses involved investigation of the aircraft (e.g., side-roll, spin-and-orient). These behaviors were similar to reports of whales rolling to one side to view UAS (e.g., Kerr et al., 2016; Domínguez-Sánchez et al., 2018), as well as the “alert” and “head-up” behaviors described in seals during aircraft approach (Pomeroy et al., 2015). Dolphins exhibited open-mouth behaviors during side-rolls, which is similar to reports of a sperm whale rolling on its side with mouth agape in response to a fixed-wing manned aircraft (Smultea et al., 2008). This suggests that measuring the incidence of side-turning behaviors may be a useful diagnostic criterion for detecting cetacean responses to UAS. The single dolphin in our study that engaged in excited responses and possible social displays, and potentially agonistic responses may have been trying to evade the aircraft, but it was unclear if this was the case. A lack of responses to the aircraft when it was flown above 30 m suggests that if a small UAS is responsibly piloted, with minimal animal-directed movements at sufficiently high altitudes, dolphins are unlikely to be significantly impacted.
The evasion we observed in responding manatees appeared similar to typical disturbance responses of marine mammals to close vessel approaches (e.g., Williams et al., 2002; Senigaglia et al., 2016). Manatees exhibited a strong sensitivity to multi-rotor aircraft movements, fleeing from aircraft at altitudes ranging from 6 to 52 m, and repeatedly evading the UAS at altitudes as high as 104 m. The manatee reactions reported here are similar to the bucking behaviors observed in humpback whales and southern right whales during blow collection (e.g., Kerr et al., 2016), both characterized rapid tail movements and apparent evasion following UAS detection. Manatees fled from aircrafts for long durations across seagrass flats, and occasionally into deeper water if it was available. This behavior is similar to the reactions of Florida manatees following boat disturbance (Nowacek et al., 2004), and also resembles observations of seals fleeing haul-out sites into the water following UAS disturbance (Pomeroy et al., 2015). Interestingly, manatees have no regular natural predators but were intensively hunted in Belize from pre-Columbian times (McKillop, 1985) till the late nineteenth century (Bonde and Potter, 1995), pressures that can sometimes cause manatees to shift their activity to avoid human detection (Jiménez, 2002). Manatees in our study area may respond strongly as a result of a combination of historical hunting behavior and daily exposure to boat traffic in the region leading them to evade approaching objects.
Our findings indicate that manatees can be negatively affected by UAS in various ways, including loss of feeding and resting opportunities and possible area avoidance (including avoidance of critical habitats). For example, responding manatees sometimes fled into nearby deep-water channels, where they were at increased risk of encountering boat traffic. These responses might have especially negative results for vulnerable animals; for example, if manatee flight responses cause the separation of mother and calf, there is an increased risk of calf orphaning or calf death (Parente et al., 2004). Repeated evasion by manatees, and persistent and repeated responses by multiple dolphins, suggests that the increases in animal vigilance following disturbance by a UAS can result in short-term changes in natural behavior patterns. Birds and marine mammals on land have shown similar responses to UAS (e.g., Chabot and Bird, 2015; Pomeroy et al., 2015). Improper use of UAS targeting marine megafauna could be especially harmful to animals with restricted home ranges, as they may be repeatedly driven from core habitats. The ability to continuously follow animals, while greatly beneficial in tracking cryptic species like manatees, could be problematic for animals that cannot evade an aircraft. The strong disturbance responses we observed in manatees suggests regulations should require UAS pilots to exercise extra caution when using these systems near sirenians.
Individual differences in the personality and experiences of animals within each population likely drove differences in their responses to UAS flight. For example, the two repeated responses of a dolphin (18 months apart) and numerous repeated responses of a manatee (repeated in the same week and again 12 months later) suggests some animals may be more susceptible to disturbance than others. Risk may be higher if they are resident to areas with frequent UAS operations (as with these two animals) or in areas where individuals cannot be identified or distinguished. It is unknown whether repeated aircraft exposures caused behavioral habituation or sensitization in dolphins and manatees, and distinguishing these processes from naturally variable levels of tolerance in their populations will require further study (Bejder et al., 2009). For example, a mother/calf pair repeatedly exposed to the aircraft on only visibly responded in the first flight. On the other hand, the manatee previously mentioned responded in its first flight and continued to respond in many flights. Dolphins were most likely to respond to UAS toward the beginning of the first flight to which they were exposed, and tended not to respond again in up to 5 repeated flights in the same sighting. Manatees that responded tended not to be present for repeated flights, as the response flight typically caused them to flee the immediate area. Previous experiences with watercraft may affect the likelihood of animals to respond to UAS, and specific individuals or age/sex classes may vary in their susceptibility to disturbance (e.g., Lusseau, 2006). Numerous manatees were identified using scars acquired during close interactions and collisions with watercraft. Such events could sensitize animals to the noise of nearby motorized engines, such as those of our quadcopters.
How Did Dolphins and Manatees Detect the UAS?
Both species showed evidence of using multiple sensory modalities during initial detection of the UAS and throughout their responses. Which of these modalities initially alerted animals to the aircraft, however, is still unclear. Dolphin responses involved clear visual orientation toward and investigation of the aircraft, but these orienting behaviors began after having already detected the aircraft above them. It is possible that the dolphins initially heard the sound of the quadcopters' rotors. Because manatees rapidly fled the area, there is insufficient evidence to speculate as to whether they detected the aircraft visually or acoustically. Bottlenose dolphin visual acuity is equally as good in air as it is in water in regular daylight (Herman et al., 1975), while manatees have poor visual acuity both in air and underwater (Bauer et al., 2003). An approaching aircraft presents animals with an increasingly intense and novel stimulus, both acoustically and visually, and each model used here was equipped with four downward-facing lights. These alternated between a red, blinking light, and a green, constant light. Animals may have been able to see these lights (Kerr et al., 2016). The shadow of the aircraft was not visible on the water's surface in most of our videos; furthermore, the position of the UAS relative to the sun made it unlikely that the animals would detect a shadow.
Across all responses, dolphins and manatees reacted primarily when the aircraft was directly or nearly overhead. In this position, the noise of the four active aircraft motors is greatest. The likelihood of detection of this noise, once it penetrates the water's surface, may be increased by reflection and refraction of the rotor noise off the seabed and surface (Erbe et al., 2017). Recent acoustic experiments with UAS (e.g., Christiansen et al., 2016b; Erbe et al., 2017), coupled with the established hearing capabilities of the West Indian manatee (Gerstein et al., 1999; Gaspard et al., 2012) and bottlenose dolphin (Johnson, 1967), indicate that the three aircraft used in this study produce both in-air and underwater sounds that are audible to both species. However, tests of different multi-rotor UAS models suggest that aircraft noise is unlikely to affect most marine mammals when they are underwater, both because the noise is masked by in-air ambient noise and because most of the sound energy fails to penetrate the water's surface (Christiansen et al., 2016b; Erbe et al., 2017). It is still unclear how manatees detected the UAS at altitudes of up to 104 m, or how they detected the aircraft's flight path well enough to directionally evade it. A combination of a change in altitude of the UAS with a subsequent change in its appearance, and a change in the direction of the noise from the UAS may together facilitate the animal's detection of the aircraft. Future research with animals in captivity will be useful for establishing clear behavioral and sensory thresholds for the use of UAS in studying these species.
Best Practices for UAS Flight Dolphins and Manatees
Mitigating the negative effects of UAS use requires taxa- or species-specific impact assessments. Flight protocols must be designed according to both data collection needs and local regulations of UAS. Our findings support the need for published best-practices guides to UAS-use (e.g., Hodgson and Koh, 2016; Smith et al., 2016). In addition, here we will propose several guidelines for multi-rotor UAS operations with marine mammals. Each of these guidelines must be further validated in future studies.
(1) Develop in situ metrics for detecting animal disturbance in response to the UAS. Responses to the UAS varied between species and individuals. In addition to this, animal responses to the aircraft were not always easily detectable, resulting in unintentional disturbance. Some animals that were exposed to the aircraft on multiple occasions responded to it several times. Individual animals, as well as animals in different age/sex classes, responded differently to the UAS. Metrics to gauge potentially negative effects of UAS on study animals must be designed to take these variations into account.
(2) Fly UAS at the highest altitudes feasible for acquiring sufficient-quality data. The increased animal disturbance we observed in flights lower than 60 m suggests that minimum flight altitude limits need to be established. These limits may differ between species. Systems with improved camera resolution should be prioritized to reduce the need for low-altitude flights.
(3) Minimize aircraft movements and avoid direct approaches to animals. Dolphins and manatees were more likely to respond to vertically-descending direct approaches than to either horizontal follows or stable hovering. In this way they are similar to other reported species (e.g., McEvoy et al., 2016; Smith et al., 2016). This was true despite the relatively short duration of direct approaches; horizontal follows and hovering were prolonged, and yet provoked fewer responses.
(4) Camouflage the aircraft to reduce its visibility and audibility (i.e., noise output). UAS may be painted to reduce their visibility (Kerr et al., 2016). The use of low-noise rotors and propellers could also significantly reduce the probability of detection and disturbance. Additionally, sufficient pilot skill and maintenance of equipment are integral to careful and controlled flight, and in preventing crashes, which are potentially dangerous for both animals and operators.
The findings of this study will inform protocols for scientific, management, and recreational use of UAS with marine mammals, both in Belize and across the range of bottlenose dolphins and Antillean manatees. Increasing unregulated recreational UAS use and a lack of resources for effective enforcement may create a problem for these species, especially at coastal destinations where tourism brings high numbers of boaters and swimmers into critical habitat for marine species. To legally fly UAS near wildlife in Belize, permits from several government offices and managing authorities are required. The Wildlife Protection Act of 1982 makes it illegal to harass marine mammals, with harassment including any disturbance that causes changes in behavioral patterns. These restrictions are similar to those imposed in the USA by the Endangered Species Act and the Marine Mammal Protection Act of 1972, which are enforced by the US Fish and Wildlife Service and the NOAA's National Marine Fisheries Service (Smith et al., 2016). Recommended flight protocols can be integrated into international regulations. For example, in the state of Florida a lack of published data on the effects of UAS exposure on manatees has resulted in restrictions on UAS use. Further study in Belize may be used to inform these permitting guidelines in Florida, despite national boundaries. In general, further study to evaluate exposure thresholds will improve the development of protocols for UAS flight with dolphins and manatees.
Findings from this study illustrate the strength of UAS to gather high-resolution observations of animal behavior. Such information can be critical for effective management of marine fauna. We demonstrated that the use of UAS follows can be effective in tracking specific fine-scale behavioral responses, even among individual animals. Orthomosaic maps produced from UAS images enabled us to precisely verify animals' location, to track individuals, and to measure detailed habitat characteristics. In addition, we developed a method for photo-ID of Antillean manatees through UAS-based imagery of their bodies (see Figure 2). This strategy was most useful in distinguishing individual manatees in groups and over short time scales (e.g., several weeks, 1 yr), but it is still unclear whether individual animals will be successfully re-sighted using this method over a span of multiple years. There are several limitations to this method of manatee identification that will be examined in detail in an article in preparation (Landeo-Yauri et al., in preparation). First, it was heavily dependent on the clear and calm waters of our study site. This water clarity allowed for reliable detection and tracking of manatees over sustained periods of time, and allowed us to examine individuals' scars and visible marks across their entire body. These conditions may be unavailable in many turbid manatee habitats. Secondly, the use of this method is less effective in identifying manatees with insufficient or impermanent scarring. Thirdly, the need to fly to at low altitude to obtain high-quality imagery may cause manatees to flee. Finally, the behavior of the manatees during flight sometimes made identification challenging or impossible. For example, resting manatees rarely expose more than the tip of their snout above the water, making them more difficult to see. Future studies using UAS for manatee photo-ID should carefully examine detectability of animals across field sites and conditions, prioritizing methods that minimize disturbance to target animals.
Our study was subject to several limitations. Different methodologies were employed to detect and approach dolphins and manatees based on species-specific constraints (e.g., movement patterns and group sizes). This restricted us from using identical approaches to compare the responses of dolphins and manatees to UAS. We were also limited in our acquisition of control data with no UAS present, as it was the UAS itself that enabled us to gather high-resolution overhead video of the animals. These factors may limit the generalizability of our results to other populations. Dolphins were observed primarily during boat-based deployments; these required close vessel approaches for photo-ID, and rapid aircraft launches to maintain sight of dolphin groups. Dolphins regularly interacted with the research vessel during observations, and before and after several RE. These factors may have affected the animals' behavior, changing the likelihood that they would respond to UAS (e.g., Lemon et al., 2006). Our ability to discern response differences between aircraft models was limited by logistics requiring the use of available models. The turning and movement behaviors that we used to identify UAS responses are similar to many dolphin social behaviors, and this may have caused us to overestimate UAS response levels in some cases. Unlike manatees, near-constant dolphin movement made detection of specific flight responses or movements away from the UAS infeasible in most videos. Finally, the measures of behavioral change we employed were restricted to visible behaviors and detectable changes in movement patterns. It is possible that focal dolphins and manatees exhibited changes in acoustic activity or physiological state, which we were unable to detect. Animals sometimes respond to disturbance stimuli with increased levels of stress-related hormones and chronic stress if they are unable to avoid harmful stimuli (e.g., Rolland et al., 2012). For example, American black bears (Ursus americanus) and king penguin (Aptenodytes patagonicus) chicks equipped with cardiac biologgers responded to UAS flights with elevated heart rates, at times with no observable behavioral responses (Ditmer et al., 2015; Weimerskirch et al., 2017). Tests using blow samples collected from whales, or measurement of stress hormones and blood cortisol levels in captive marine mammals, will be valuable in evaluating these “invisible” physiological effects of UAS response. Future studies examining species-specific responses to UAS may prioritize shore-based operations to reduce the bias introduced by a nearby research boat. Such studies may also make use of multi-sensor tags to track study animals, rather than relying on visual evidence alone.
Ethics Statement
This study was carried out in accordance with the recommendations of the Belize Fisheries and Forestry Departments [Permit Ref. No. WL/1/1/16(28)] of the Government of Belize. This study was carried out in accordance with the guidelines of the IACUC committee at Hunter College.
Author Contributions
ER, BM, MM, and DR conceived and designed the study. ER and BM collected and analyzed the data. ER, BM, MM, and DR wrote the paper.
Funding
This research was supported in part by grants from the National Science Foundation (grant number PHY-1530544) and The Eric and Wendy Schmidt Fund for Strategic Innovation. ER was partially funded by the Office of Educational Opportunity & Diversity at The Graduate Center, CUNY.
Conflict of Interest Statement
The authors declare that the research was conducted in the absence of any commercial or financial relationships that could be construed as a potential conflict of interest.
Acknowledgments
We thank the countless students, volunteers, and personnel who participated in the field research for this study, including groups with Ecology Project International, National Geographic Student Expeditions, University of Belize, the Environmental Research Institute on Calabash Caye, SEEtheWild, SEE Turtles, and Smithsonian Student Expeditions. Thanks to Sarah Landeo-Yauri for working on photo-ID. Thanks to HelloOcean for support with catamaran research, and The Moorings for their financial and logistical support in donating vessel time to our cause. Special thanks to A. Jeffords for captaining the boat and catching the UAS. Many thanks to L. and J. Searle at ECOMAR for logistical support at St. George's Caye. Thanks to the Oceanic Society for providing financial and logistical support, including housing for ER and other members of our research team. All UAS were owned by The Rockefeller University and ER, and were piloted by ER. This study and all data collection protocols were conducted under research permits granted to ER by several governmental institutions in Belize. Thanks to the Forest Department and Fisheries Department of Belize for permits for marine mammal research, to the Belize Department of Civil Aviation for permission to fly the UAS, and to the Public Utilities Commission for import permits for the crafts. Finally, thank you to Megan S. McGrath and the three reviewers whose comments and recommendations vastly improved this manuscript.
Supplementary Material
The Supplementary Material for this article can be found online at: https://www.frontiersin.org/articles/10.3389/fmars.2018.00316/full#supplementary-material
References
Acevedo-Whitehouse, K., Rocha-Gosselin, A., and Gendron, D. (2010). A novel non-invasive tool for disease surveillance of free-ranging whales and its relevance to conservation programs. Anim. Conserv. 13, 217–225. doi: 10.1111/j.1469-1795.2009.00326.x
Adame, K., Pardo, M. A., Salvadeo, C., Beier, E., and Elorriaga-Verplancken, F. R. (2017). Detectability and categorization of California sea lions using an unmanned aerial vehicle. Mar. Mammal Sci. 33, 913–925. doi: 10.1111/mms.12403
Anderson, K., and Gaston, K. J. (2013). Lightweight unmanned aerial vehicles will revolutionize spatial ecology. Front. Ecol. Environ. 11, 138–146. doi: 10.1890/120150
Apprill, A., Miller, C. A., Moore, M. J., Durban, J. W., Fearnbach, H., and Barrett-Lennard, L. G. (2017). Extensive core microbiome in drone-captured whale blow supports a framework for health monitoring. MSystems 2:e00119-17. doi: 10.1128/mSystems.00119-17
Arona, L., Dale, J., Heaslip, S. G., Hammill, M. O., and Johnston, D. W. (2018). Assessing the disturbance potential of small unoccupied aircraft systems (UAS) on gray seals (Halichoerus grypus) at breeding colonies in Nova Scotia, Canada. PeerJ 6:e4467. doi: 10.7717/peerj.4467
Bacchus, M. L. C., Dunbar, S. G., and Self-Sullivan, C. (2009). Characterization of resting holes and their use by the Antillean manatee (Trichechus manatus manatus) in the Drowned Cayes, Belize. Aquat. Mamm. 35, 62–71. doi: 10.1578/AM.35.1.2009.62
Bauer, G. B., Colbert, D. E., Gaspard, II, J. C., Littlefield, B., and Fellner, W. (2003). Underwater visual acuity of Florida manatees (Trichechus manatus latirostris). Int. J. Comp. Psychol. 16, 130–142.
Bejder, L., Samuels, A., Whitehead, H., Finn, H., and Allen, S. (2009). Impact assessment research: use and misuse of habituation, sensitisation and tolerance in describing wildlife responses to anthropogenic stimuli. Mar. Ecol. Prog. Ser. 395, 177–185. doi: 10.3354/meps07979
Bevan, E., Whiting, S., Tucker, T., Guinea, M., Raith, A., and Douglas, R. (2018). Measuring behavioral responses of sea turtles, saltwater crocodiles, and crested terns to drone disturbance to define ethical operating thresholds. PLoS ONE 13:e0194460. doi: 10.1371/journal.pone.0194460
Bonde, R. K., and Potter, C. W. (1995). “Manatee butchering sites in Port Honduras,” in Sirenews: Newsletter of the International Union for Conservation of Nature and Natural Resources/Species Survival Commission, Sirenia Specialist Group, 24, 7.
Brisson-Curadeau, É., Bird, D., Burke, C., Fifield, D. A., Pace, P., Sherley, R. B., et al. (2017). Seabird species vary in behavioural response to drone census. Sci. Rep. 7:17884. doi: 10.1038/s41598-017-18202-3
Campbell, G. S., Bilgre, B. A., and Defran, R. (2002). Bottlenose dolphins (Tursiops truncatus) in Turneffe Atoll, Belize: occurrence, site fidelity, group size, and abundance. Aquat. Mamm. 28, 170–180.
Chabot, D., and Bird, D. M. (2015). Wildlife research and management methods in the 21st century: Where do unmanned aircraft fit in? J. Unmanned Veh. Syst. 3, 137–155. doi: 10.1139/juvs-2015-0021
Christiansen, F., Dujon, A. M., Sprogis, K. R., Arnould, J. P., and Bejder, L. (2016a). Noninvasive unmanned aerial vehicle provides estimates of the energetic cost of reproduction in humpback whales. Ecosphere 7:e01468. doi: 10.1002/ecs2.1468
Christiansen, F., Rojano-Doñate, L., Madsen, P. T., and Bejder, L. (2016b). Noise levels of multi-rotor unmanned aerial vehicles with implications for potential underwater impacts on marine mammals. Front. Mar. Sci. 3:277. doi: 10.3389/fmars.2016.00277
Defran, R., Shultz, G. M., and Weller, D. W. (1990). A technique for the photographic identification and cataloging of dorsal fins of the bottlenose dolphin (Tursiops truncatus). Individual recognition of cetaceans: use of photo-identification and other techniques to estimate population parameters. Rep. Int. Whal. Commn. 53–55.
Dick, D. M., and Hines, E. M. (2011). Using distance sampling techniques to estimate bottlenose dolphin (Tursiops truncatus) abundance at Turneffe Atoll, Belize. Mar. Mammal Sci. 27, 606–621. doi: 10.1111/j.1748-7692.2010.00435.x
Ditmer, M. A., Vincent, J. B., Werden, L. K., Tanner, J. C., Laske, T. G., Iaizzo, P. A., et al. (2015). Bears show a physiological but limited behavioral response to unmanned aerial vehicles. Curr. Biol. 25, 2278–2273. doi: 10.1016/j.cub.2015.07.024
Domínguez-Sánchez, C. A., Acevedo-Whitehouse, K. A., and Gendron, D. (2018). Effect of drone-based blow sampling on blue whale (Balaenoptera musculus) behavior. Mar. Mammal Sci. 34, 841–850. doi: 10.1111/mms.12482
Durban, J. W., Fearnbach, H., Barrett-Lennard, L. G., Perryman, W. L., and Leroi, D. J. (2015). Photogrammetry of killer whales using a small hexacopter launched at sea. J. Unmanned Veh. Syst. 3, 131–135. doi: 10.1139/juvs-2015-0020
Erbe, C., Parsons, M., Duncan, A. J., Osterrieder, S., and Allen, K. (2017). Aerial and underwater sound of unmanned aerial vehicles (UAV, drones). J. Unmanned Veh. Syst. 5, 92–101. doi: 10.1139/juvs-2016-0018
Flamm, R. O., Owen, E. C., Owen, C. F., Wells, R. S., and Nowacek, D. (2000). Aerial videogrammetry from a tethered airship to assess manatee life-stage structure. Mar. Mammal Sci. 16, 617–630. doi: 10.1111/j.1748-7692.2000.tb00955.x
Gaspard, J. C., Bauer, G. B., Reep, R. L., Dziuk, K., Cardwell, A., Read, L., et al. (2012). Audiogram and auditory critical ratios of two Florida manatees (Trichechus manatus latirostris). J. Exp. Biol. 215, 1442–1447. doi: 10.1242/jeb.065649
Gerstein, E. R., Gerstein, L., Forsythe, S. E., and Blue, J. E. (1999). The underwater audiogram of the West Indian manatee (Trichechus manatus). J. Acoust. Soc. Am. 105, 3575–3583. doi: 10.1121/1.424681
Gill, J. A. (2007). Approaches to measuring the effects of human disturbance on birds. IBIS 149, 9–14. doi: 10.1111/j.1474-919X.2007.00642.x
Herman, L. M., Peacock, M. F., Yunker, M. P., and Madsen, C. J. (1975). Bottlenosed dolphin: double-slit pupil yields equivalent aerial and underwater diurnal acuity. Science 189, 650–652.
Hodgson, A. (2007). “BLIMP-CAM”: Aerial video observations of marine animals. Mar. Tech. Soc. J. 41, 39–43.
Hodgson, A., Kelly, N., and Peel, D. (2013). Unmanned aerial vehicles (UAVs) for surveying marine fauna: a dugong case study. PLoS ONE 8:e79556. doi: 10.1371/journal.pone.0079556
Hodgson, J. C., and Koh, L. P. (2016). Best practice for minimising unmanned aerial vehicle disturbance to wildlife in biological field research. Curr. Biol. 26, R404–R405. doi: 10.1016/j.cub.2016.04.001
Jiménez, I. (2002). Heavy poaching in prime habitat: the conservation status of the West Indian manatee in Nicaragua. Oryx 36, 272–278. doi: 10.1017/S0030605302000492
Johnson, C. S. (1967). “Sound detection thresholds in marine mammals,” in Marine Bioacoustics, ed W. N. Tavolga (Oxford:Pergamon Press), 247–260.
Johnston, D. W., Dale, J., Murray, K. T., Josephson, E., Newton, E., and Wood, S. (2017). Comparing occupied and unoccupied aircraft surveys of wildlife populations: assessing the gray seal (Halichoerus grypus) breeding colony on Muskeget Island, USA. J. Unmanned Veh. Sys. 5, 178–191. doi: 10.1139/juvs-2017-0012
Jones, G. P., Pearlstine, L. G., and Percival, H. F. (2006). An assessment of small unmanned aerial vehicles for wildlife research. Wild Soc. Bull. 34, 750–758. doi: 10.2193/0091-7648(2006)34[750:AAOSUA]2.0.CO;2
Kerr, I., Rogan, A., Graham, J., and Miller, C. (2016). Report to National Marine Fisheries on Observed Reactions of Large Cetaceans to Small Unmanned Aerial Systems (sUAS). Ocean Alliance, Institutional Report, 1–5.
Kiszka, J. J., Mourier, J., Gastrich, K., and Heithaus, M. R. (2016). Using unmanned aerial vehicles (UAVs) to investigate shark and ray densities in a shallow coral lagoon. Mar. Ecol. Prog. Ser. 560, 237–242. doi: 10.3354/meps11945
Koski, W. R., Allen, T., Ireland, D., Buck, G., Smith, P. R., Macrander, A. M., et al. (2009). Evaluation of an unmanned airborne system for monitoring marine mammals. Aquat. Mamm. 35, 347–357. doi: 10.1578/AM.35.3.2009.347
Koski, W. R., Gamage, G., Davis, A. R., Mathews, T., Leblanc, B., and Ferguson, S. H. (2015). Evaluation of UAS for photographic re-identification of bowhead whales, Balaena mysticetus. J. Unmanned Veh. Syst. 3, 22–29. doi: 10.1139/juvs-2014-0014
Krause, D. J., Hinke, J. T., Perryman, W. L., Goebel, M. E., and Leroi, D. J. (2017). An accurate and adaptable photogrammetric approach for estimating the mass and body condition of pinnipeds using an unmanned aerial system. PLoS ONE 12:e0187465. doi: 10.1371/journal.pone.0187465
Langtimm, C. A., Beck, C. A., Edwards, H. H., Fick-Child, K. J., Ackerman, B. B., Barton, S. L., et al. (2004). Survival estimates for Florida manatees from the photo-identification of individuals. Mar. Mammal Sci. 20, 438–463. doi: 10.1111/j.1748-7692.2004.tb01171.x
Lemon, M., Lynch, T. P., Cato, D. H., and Harcourt, R. G. (2006). Response of travelling bottlenose dolphins (Tursiops aduncus) to experimental approaches by a powerboat in Jervis Bay, New South Wales, Australia. Biol. Conserv. 127, 363–372. doi: 10.1016/j.biocon.2005.08.016
Linchant, J., Lisein, J., Semeki, J., Lejeune, P., and Vermeulen, C. (2015). Are unmanned aircraft systems (UASs) the future of wildlife monitoring? A review of accomplishments and challenges. Mammal Rev. 45, 239–252. doi: 10.3390/s16010097
Lusseau, D. (2006). The short-term behavioral reactions of bottlenose dolphins to interactions with boats in Doubtful Sound, New Zealand. Mar. Mammal Sci. 22, 802–818. doi: 10.1111/j.1748-7692.2006.00052.x
Mann, J., Connor, R. C., Barre, L. M., and Heithaus, M. R. (2000). Female reproductive success in bottlenose dolphins (Tursiops sp.): life history, habitat, provisioning, and group-size effects. Behav. Ecol. 11, 210–219. doi: 10.1093/beheco/11.2.210
McEvoy, J. F., Hall, G. P., and McDonald, P. G. (2016). Evaluation of unmanned aerial vehicle shape, flight path and camera type for waterfowl surveys: disturbance effects and species recognition. PeerJ 4:e1831. doi: 10.7717/peerj.1831
McKillop, H. I. (1985). Prehistoric exploitation of the manatee in the Maya and circum-Caribbean areas. World Archaeol. 16, 337–353. doi: 10.1080/00438243.1985.9979939
Moreland, E. E., Cameron, M. F., Angliss, R. P., and Boveng, P. L. (2015). Evaluation of a ship-based unoccupied aircraft system (UAS) for surveys of spotted and ribbon seals in the Bering Sea pack ice. J. Unmanned Veh. Syst. 3, 114–122. doi: 10.1139/juvs-2015-0012
Mulero-Pázmány, M., Jenni-Eiermann, S., Strebel, N., Sattler, T., Negro, J. J., and Tablado, Z. (2017). Unmanned aircraft systems as a new source of disturbance for wildlife: a systematic review. PLoS ONE 12:e0178448. doi: 10.1371/journal.pone.0178448
Nowacek, D. P., Wells, R. S., and Tyack, P. (2001). A platform for continuous behavioral and acoustic observation of free-ranging marine mammals: overhead video combined with underwater video. Mar. Mammal Sci. 17, 191–199. doi: 10.1111/j.1748-7692.2001.tb00992.x
Nowacek, S. M., Wells, R. S., Owen, E. C., Speakman, T. R., Flamm, R. O., and Nowacek, D. P. (2004). Florida manatees, Trichechus manatus latirostris, respond to approaching vessels. Biol. Conserv. 119, 517–523. doi: 10.1016/j.biocon.2003.11.020
O'Shea, T. J., and Langtimm, C. A. (1995). Estimation of survival of adult Florida manatees in the Crystal River, at Blue Spring, and on the Atlantic coast. Natl. Biol. Serv. 1, 194–222.
Parente, C. L., Vergara-Parente, J. E., and Lima, R. P. (2004). Strandings of Antillean manatees, Trichechus manatus manatus, in northeastern Brazil. LAJAM 3, 69–75. doi: 10.5597/lajam00050
Pirotta, V., Smith, A., Ostrowski, M., Russell, D., Jonsen, I. D., Grech, A., et al. (2018). An economical custom-built drone for assessing whale health. Front. Mar. Sci. 4:425. doi: 10.3389/fmars.2017.00425
Pomeroy, P., O'Connor, L., and Davies, P. (2015). Assessing use of and reaction to unmanned aerial systems in gray and harbor seals during breeding and molt in the UK 1. J. Unmanned Veh. Syst. 3, 102–113. doi: 10.1139/juvs-2015-0013
Ramos, E. A., Castelblanco-Martínez, D. N., Garcia, J., Rojas-Arias, J., Foley, J., Audley, K., et al. (2018). Lobomycosis-like disease in bottlenose dolphins in Belize and Mexico: bridging the gap between the Americas. Dis. Aquat. Org. 128, 1–12. doi: 10.3354/dao03206
Ramos, E. A., Castelblanco-Martínez, D. N., Niño-Torres, C. A., Jenko, K., and Auil Gomez, N. (2016). A review of the aquatic mammals of Belize. Aquat. Mamm. 42, 476–493. doi: 10.1578/AM.42.4.2016.476
Ramos, E. A., Castelblanco-Martínez, N., Nino-Torres, C., Landeo, S., Magnasco, M. O., and Reiss, D. (2017). “Small drones: a tool to study, monitor, and manage free-ranging Antillean manatees in Belize and Mexico,” in Sirenews (International Union for Conservation of Nature and Natural Resources/Species Survival Commission, Sirenia Specialist Group), 13–16.
Rolland, R. M., Parks, S. E., Hunt, K. E., Castellote, M., Corkeron, P. J., Nowacek, D. P., et al. (2012). Evidence that ship noise increases stress in right whales. Proc. R. Soc. Lond. B. Biol. Sci. 279, 2363–2368. doi: 10.1098/rspb.2011.2429
Rümmler, M. C., Mustafa, O., Maercker, J., Peter, H. U., and Esefeld, J. (2016). Measuring the influence of unmanned aerial vehicles on Adélie penguins. Polar Biol. 39, 1329–1334. doi: 10.1007/s00300-015-1838-1
Senigaglia, V., Christiansen, F., Bejder, L., Gendron, D., Lundquist, D., Noren, D., et al. (2016). Meta-analyses of whale-watching impact studies: comparisons of cetacean responses to disturbance. Mar. Ecol. Prog. Ser. 542, 251–263. doi: 10.3354/meps11497
Shane, S. H. (1990). “Comparison of bottlenose dolphin behavior in Texas and Florida, with a critique of methods for studying dolphin behavior,” in The Bottlenose Dolphin, eds S. Leatherwood and R. R. Reeves (San Diego, CA; Academic Press), 541–558.
Smith, C. E., Sykora-Bodie, S. T., Bloodworth, B., Pack, S. M., Spradlin, T. R., and Leboeuf, N. R. (2016). Assessment of known impacts of unmanned aerial systems (UAS) on marine mammals: data gaps and recommendations for researchers in the United States. J. Unmanned Veh. Syst. 4, 1–14. doi: 10.1139/juvs-2015-0017
Smultea, M. A., Mobley, J. R. Jr., Fertl, D., and Fulling, G. L. (2008). An unusual reaction and other observations of sperm whales near fixed-wing aircraft. Gulf Caribb. Res. 20, 75–80. doi: 10.18785/gcr.2001.10
Sweeney, K. L., Helker, V. T., Perryman, W. L., Leroi, D. J., Fritz, L. W., Gelatt, T. S., et al. (2015). Flying beneath the clouds at the edge of the world: using a hexacopter to supplement abundance surveys of Steller sea lions (Eumetopias jubatus) in Alaska. J. Unmanned Veh. Syst. 4, 70–81. doi: 10.1139/juvs-2015-0010
Vas, E., Lescroël, A., Duriez, O., Boguszewski, G., and Grémillet, D. (2015). Approaching birds with drones: first experiments and ethical guidelines. Biol. Lett. 11:20140754. doi: 10.1098/rsbl.2014.0754
Weimerskirch, H., Prudor, A., and Schull, Q. (2017). Flights of drones over sub-Antarctic seabirds show species-and status-specific behavioural and physiological responses. Polar Biol. 41, 259–266. doi: 10.1007/s00300-017-2187-z
Keywords: unmanned aerial systems, drone, dolphin, manatee, marine mammal, disturbance, wildlife, monitoring
Citation: Ramos EA, Maloney B, Magnasco MO and Reiss D (2018) Bottlenose Dolphins and Antillean Manatees Respond to Small Multi-Rotor Unmanned Aerial Systems. Front. Mar. Sci. 5:316. doi: 10.3389/fmars.2018.00316
Received: 21 December 2017; Accepted: 16 August 2018;
Published: 12 September 2018.
Edited by:
Peter H. Dutton, National Oceanic and Atmospheric Administration (NOAA), United StatesReviewed by:
Holly Edwards, Florida Fish and Wildlife Conservation Commission, United StatesVanessa Pirotta, Macquarie University, Australia
Alana Grech, ARC Centre of Excellence for Coral Reef Studies, Australia
Copyright © 2018 Ramos, Maloney, Magnasco and Reiss. This is an open-access article distributed under the terms of the Creative Commons Attribution License (CC BY). The use, distribution or reproduction in other forums is permitted, provided the original author(s) and the copyright owner(s) are credited and that the original publication in this journal is cited, in accordance with accepted academic practice. No use, distribution or reproduction is permitted which does not comply with these terms.
*Correspondence: Eric A. Ramos, ZXJpYy5hbmdlbC5yYW1vc0BnbWFpbC5jb20=