- 1Department of Bioscience, Aarhus University, Silkeborg, Denmark
- 2Arctic Research Centre, Aarhus University, Aarhus, Denmark
- 3Department of Bioscience, Aarhus University, Aarhus, Denmark
- 4Global Change Research Group, IMEDEA (CSIC-UIB), Institut Mediterrani d'Estudis Avançats, Esporles, Spain
- 5Danish Centre for Wild Salmon, Randers, Denmark
- 6Greenland Climate Research Centre, Greenland Institute of Natural Resources, Nuuk, Greenland
Ice can be an important structuring factor physically removing intertidal flora and fauna. At high latitudes in particular, the removal of canopy-forming algae by ice scour may be important as their canopy may serve to modify the extreme environment for marine organisms at low tide. We simulated the effect of ice scouring by manipulating the biomass of the canopy-forming algae Ascophyllum nodosum in a sub-Arctic fjord [“Full canopy,” “Reduced canopy,” “Bare (start),” “Bare (annual)”]. Over a three-year period, we quantified key physical parameters and the recolonization of flora and fauna to test the hypothesis that A. nodosum and rock rugosity facilitate recolonization of sub-Arctic intertidal fauna and that potential facilitation could rely on an ability of A. nodosum canopy to modify air temperature and ice scour. Finally, we estimated the recovery period of A. nodosum canopy height to pre-disturbance levels based on estimated early growth rates. We found that A. nodosum canopy facilitated higher species richness and recolonization of dominating faunal species (Littorina saxatilis, Littorina obtusata, Mytilus edulis, and Semibalanus balanoides), and also significantly reduced the high temperatures in summer and raised the low temperatures in winter. The abundance of M. edulis and A. nodosum recolonization increased significantly with rock rugosity and the recovery of A. nodosum canopy height was estimated to a minimum of 15 years. We conclude that algal canopy and rock rugosity play key roles in structuring sub-Arctic intertidal communities, likely by modifying environmental stress such as extreme temperature, desiccation, and by increasing the settling surface and the habitat complexity. As the distribution of canopy-forming algae is expected to shift northward, they may act as a key habitat facilitating a northward colonization of intertidal fauna in the Arctic. We highlight the importance of considering scales relevant to biological communities when predicting impacts of climate change on distributional patterns and community structure in the Arctic intertidal.
Introduction
In intertidal ecosystems, air temperature, exerting a major control on biological processes, can be modified by a number of factors acting at scales that are relevant to biological communities (Helmuth, 1998; Helmuth et al., 2010). For example, sea ice modifies air temperature directly (Scrosati and Eckersley, 2007) and ice scouring may indirectly influence air temperature through the removal of canopy-forming algae (Gutt, 2001; Petzold et al., 2014). Algal canopies may also insulate organisms from extreme temperatures in the high intertidal as typically seen in temperate regions (Beermann et al., 2013; Watt and Scrosati, 2013a) and, thereby, influence community structure locally (Crowe et al., 2013). Ice, either in the form of sea ice or glacial ice, is a characteristic feature of high latitude coastal systems such as those found in Greenland, where export of glacial ice into the coastal ocean is increasing (Howat et al., 2007). In the Godthåbsfjord, West Greenland, for example, the loss rate of glacial ice has doubled within a decade, likely increasing the output of icebergs and thereby the risk of ice scouring in benthic communities (Motyka et al., 2017).
Here, we aim to understand the interplay of biotic and abiotic factors in structuring sub-Arctic rocky intertidal communities that can also improve predictions for climate change-induced range shifts (Gilman et al., 2010; HilleRisLambers et al., 2013). Several studies have shown the impact of canopy-forming algae on the understory community and patterns of recolonization as they alter the physical environment (Dayton, 1971; Hawkins, 1983; Jenkins et al., 1999a, 2004; Cervin et al., 2004). However, these studies are mostly restricted to the temperate intertidal as we found only one example from the sub-Arctic intertidal, mainly focusing on biotic factors (Ingólfsson and Hawkins, 2008).
The literature reports differential responses of intertidal organisms to canopy cover, also depending on the environmental stress level (McCook and Chapman, 1991; Bertness et al., 1999; Broitman et al., 2009; Crowe et al., 2013; Watt and Scrosati, 2013b). For instance, algal canopy cover enhances the survival of newly-settled barnacles only in the high intertidal zone (Dayton, 1971; Hawkins, 1983; Jenkins et al., 1999b). Moreover, species richness and diversity increase with algal canopy cover in the high and mid intertidal zone, again underlining the importance of the bioengineering effects of a canopy mainly in stressful environments (Watt and Scrosati, 2013a,b). Most likely canopies create an interplay of negative and positive interspecific interactions (Jenkins et al., 1999b; Beermann et al., 2013). As an example, barnacle recruitment may be negatively affected by whiplashes from algal fronds, but positively affected by lowered water loss and buffering of temperature, together resulting in a neutral effect of algal canopy cover on barnacles in the mid- and high intertidal (Beermann et al., 2013).
In a highly stressful environment, such as the sub-Arctic intertidal zone, the positive effects of algal canopy likely exceed the negative as suggested by the stress gradient hypothesis (Bertness and Callaway, 1994). However, we lack field studies from the sub-Arctic intertidal to support this hypothesis. In particular, the ability of algal canopies to buffer extreme air temperatures may be important in shaping high latitude intertidal communities. Variation in air temperature is a key stressor for intertidal organisms, impacting a range of biochemical and physiological processes (Helmuth, 1998; Denny and Harley, 2006). Water loss and thereby the risk of desiccation is also affected by air temperature (Helmuth, 1998), and even a few degrees temperature change can markedly impact mortality rates in the intertidal, especially for newly-settled organisms (Foster, 1971b). Ice scouring is another key stressor for intertidal organisms, and crevices in the rocky shore may, like canopy-forming algae, offer microhabitats, that shield organisms from destruction by ice scouring as well as other physical stressors (Foster, 1971b; McCook and Chapman, 1991; Walters and Wethey, 1996; Helmuth et al., 2010).
Sub-Arctic communities are considered to be shaped by large-scale climate variables and physical exposure, but clearly there is a potential for small scale variation induced by canopy-forming algae and rock roughness that may greatly affect the local physical regime, supporting community recovery after a disturbing event such as ice scouring. Therefore, the ability and speed of recovery of algal canopies may greatly affect the recovery process of the intertidal faunal community after ice scouring and potentially limit their northern distribution range.
Kobbefjord is a sheltered Greenlandic fjord in the sub-Arctic region, i.e., immediately south of the Arctic Circle. However, according to the AMAP definition, Kobbefjord is considered to be in the Arctic. We chose this study area as parts of this rocky intertidal are characterized by high biomass of the long-lived fucoid canopy-forming alga Ascophyllum nodosum (Olsen et al., 2010), and the level of mechanical stress from sea ice is considered low. Yet, patches of the community may be in a recovering state after mechanical stress caused by scouring sea ice that form seasonally in the area. Ascophyllum nodosum has a wide geographical distribution extending to 69.7°N on the coast of Greenland (Lüning, 1990) and the growth rate of the Greenland populations respond positively to a warming climate (Marbà et al., 2017).
Here, we present a first attempt at disentangling the multiple factors that influence small-scale variation in physical regimes experienced by sub-Arctic intertidal organisms. First, we test the hypothesis that A. nodosum canopy facilitates the recolonization of sub-Arctic intertidal fauna. We do so by quantifying faunal recolonization rates at different manipulated levels of canopy cover over a 3-year period. Secondly, we measure the temperature and ice scouring intensity experienced by the intertidal organisms at different levels of algal canopy cover. Thirdly, we consider the physical properties of the rock as a settlement surface and microhabitat during recolonization. Finally, we quantify the early growth rates of A. nodosum recruits, and attempt to estimate the recovery period to pre-disturbance canopy height after dislodgement by mechanical disturbance, such as ice scouring. The recovery period of A. nodosum canopy height is expected to be rather slow due to the colder climate as growth rates are lowered at low temperatures (Steen and Rueness, 2004; Keser et al., 2005).
Materials and Methods
Study Area
The study was conducted in the sub-Arctic Kobbefjord, a branch of the Godthåbsfjord system in south-west Greenland (64°08N, 51°23W) (Figure 1A). The shoreline is largely dominated by bedrock, and the mountains surrounding Godthåbsfjord and Kobbefjord are dominated by granites and granitoid gneiss (Mosbech et al., 2000; Nutman and Friend, 2009). The fjord is 17 km long and 0.8–2 km wide with a maximum depth of 150 m. It is influenced by daily tidal amplitudes of 1–5 m (Richter et al., 2011) and sea surface temperatures ranging from −1 to 9°C (Versteegh et al., 2012). Air temperature ranges from a minimum of −25°C in winter to a maximum of 20°C in summer, measured in Nuuk (Blicher et al., 2013). From April to October, the fjord receives freshwater run-off from several rivers in the innermost part of the fjord, resulting in a salinity gradient in the surface water. From December to May, sea ice usually covers the inner part of the fjord (Mikkelsen et al., 2008). This results in a system characterized by large seasonal variation in key physical parameters such as light, temperature, salinity, and mechanical stress (ice scouring).
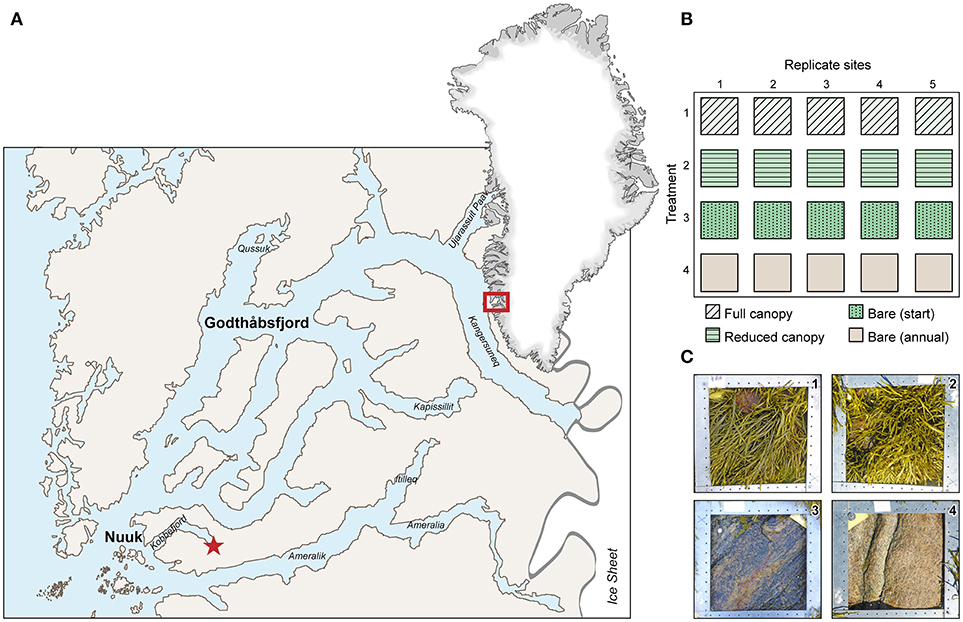
Figure 1. Study area and experimental setup. (A) Godthåbsfjord system, SW Greenland with indication of the study site in inner Kobbefjord ( 64°08N, 51°23W). (B) Experimental setup applied in inner Kobbefjord mid intertidal, with the four treatments applied at each of the five replicate sites. (C) Examples of experimental quadrats from each treatment by August 2014 (the end of experimental period) [1: Full canopy, 2: Reduced canopy, 3: Bare (start), 4: Bare (annual) (Table 1)].
Field Experiment
The experiment was conducted in the inner part of the fjord at a rocky intertidal area covered by canopy-forming fucoid algae (predominantly A. nodosum with occasional presence of Fucus vesiculosus) and spanned a 3-year period from August 2011 to August 2014. Additional quantification of algal recolonization was conducted in August 2016. We used an experimental design with five replicate sites located along 200 m of the shoreline having similar overall vertical rock slope, similar compass direction (all S-SW facing) and evenly developed A. nodosum canopy. At each of the five replicate sites, four experimental treatments were established in 25 × 25 cm quadrats (Table 1, Figures 1B,C) [“Full canopy,” “Reduced canopy,” “Bare (start)” and “Bare (annual)”] and the horizontal sequence of the four treatments was fully randomized within each replicate site. All quadrats were laid out just below the mean tidal level (determined during a full tidal cycle). The slope of the rock within the resulting 20 quadrats varied between 5 and 30°. In “Full canopy,” macroalgae were left untouched whereas fauna was removed from both canopy and rock face at the initiation of the experiment (August 2011). In “Reduced canopy,” macroalgae were cut to a height of 15 cm to imitate a moderate impact of mechanical disturbance from ice scouring still allowing macroalgae to recover (Gendron et al., 2017), and fauna was removed from both the remaining canopy and the rock face at the initiation of the experiment (August 2011). In “Bare (start),” the entire quadrat was cleared at the initiation of the experiment (August 2011) for all macroalgae including their holdfasts and all fauna to imitate maximum ice scouring impact. In “Bare (annual),” the quadrat was cleared annually in a similar way (i.e., August 2011, 2012, and 2013) in order to estimate the variation in annual settling and hence the recolonization potential. We used a metal brush for the clearing of rock surfaces and ensured that all depressions and crevices were thoroughly cleared for organisms. Macroalgae were cleared by hand for macroscopic invertebrate fauna, by working through the canopy, algal individual by individual. In a buffer zone of approximately 10 cm surrounding each quadrat, macroalgae were scraped from the rock and the canopies of algae further away were cut up to levels that prevent them from overlaying the quadrats. In order to quantify and analyze the algal and faunal community at the start of the experiment (August, 2011), referred to as the “Pre-experimental” community, all the organisms cleared from “Bare (start)” quadrats were collected and subsequently counted according to species or taxa, weighed (drained wet weight after being kept in wire mesh sieves) and measured at their maximum dimension (e.g., shell length of mussels, carapace diameter of barnacles, and height of macroalgae). The average minimum age of A. nodosum “Pre-experimental” canopy was evaluated for all individuals longer than 10 cm by counting the number of air bladders (vesicles) on the longest axis, assuming one bladder is formed annually (Åberg, 1996). This method renders a minimum age since it does not account for the age of the shoot before production the first bladder and also does not account for possible breakage of shoots. All values for minimum age, weights and lengths are given as mean (x ± SE).
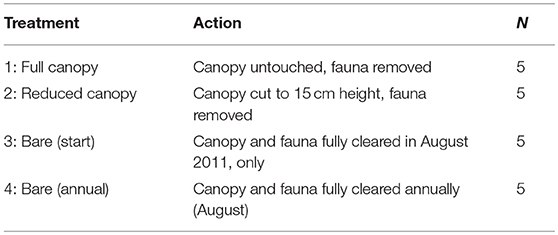
Table 1. The four treatments applied at each of five replicate sites in the inner Kobbefjord mid intertidal, August 2011.
In August 2012, 2013, and 2014 all fauna and macroalgae in “Bare (start)” and “Bare (annual)” were counted and measured at their maximum dimension (e.g., shell length of mussels, carapace diameter of barnacles, and height of macroalgae) to account for inter-annual settling and to estimate the early growth rate of A. nodosum recruits. At the termination of the experiment in August 2014, all quadrats were harvested for both macroalgae and fauna using the same method as in “Bare (start)” August 2011. Retrieved organisms were identified by taxa, counted, weighed, and their maximum dimensions were measured. Macroalgae without bladders were counted as recruits. The vast majority of recruits was below 10 cm length, and as bladders (used to age adult shoots) typically occurred only in individuals >10 cm length, this length limit coarsely separated individuals, here defined as recruits and adults. For each quadrat we quantified the faunal species richness (S) as the number of species and/or taxa present. Finally, in August 2016, 2 years after the final harvest, we quantified the number and length of A. nodosum individuals recruited into all 20 quadrats, adding to the estimate of early growth rates after maximum disturbance.
Between August 2011 and 2014, we quantified a range of physical variables in selected quadrats to characterize the habitat and potential differences between “Bare (start)” and “Full canopy” treatments. The temperature was logged every 1.5 h by sensors (Thermochron iButtons®) placed at site 4. The sensors were placed inside spherical brass housing, to protect them from ice scour and attached to a rock surface cleared of macroalgae and below A. nodosum canopy, respectively. To verify extreme temperatures measured in the intertidal, we compared with air temperature data from a nearby climate station, obtained from the Greenland Ecosystem Monitoring database (GEM). The overall extent of sea ice during the three winters of 2011–2014 was evaluated from photos taken automatically by a camera mounted on the mountain above the experimental area. The photos were taken daily in the period from January to May each year as part of the GEM monitoring program. Additionally, the ice scouring intensity was quantified at each of the five replicate sites by the degree of bending of steel screws inserted into the rock. The screws were standard commercial stainless steel screws having a height of 45 mm, a head diameter of 8 mm and a shaft diameter just below the head of 4 mm. Two screws protruding 2 cm from the rock surface were placed above each quadrat during the winters 2011–12, and 2013–14 (i.e., a total of 80 screws), and the maximum angle of bending (0–90°) of the two screws from each quadrat was used as a proxy for ice scouring intensity. To measure the roughness of the rock surface, i.e., rugosity, within the quadrats when cleared for algae and fauna, we used a profile gauge tool that captured the surface profile of the rock, which was then photographed for later image analysis using the “measure” tool in ImageJ. The ratio of the true surface profile to the linear surface profile gave an estimate of substrate rugosity (Luckhurst and Luckhurst, 1978; Zawada et al., 2010). The unit of analysis was the mean rugosity across the two diagonal profiles in each sample quadrat.
Statistical Analysis
Status of Main Treatments at the End of the Study Period
We compared the macroalgal biomass in each of the main treatments [“Full canopy,” “Reduced canopy,” “Bare (start)”] at the end of the study period (August 2014) with the “Pre-experimental” biomass using Two-sample t-tests. Similarly, macroalgal adult (> 10 cm) and recruit (< 10 cm) densities were compared to the “Pre-experimental” densities.
Algal Canopy and Faunal Recolonization
To assess the effect of the main treatments [three level factor; “Full canopy,” “Reduced canopy,” “Bare (start)”] on biomass of each species and faunal species richness at the end of the study period (2014), we performed one-way ANOVAs with biomass or faunal species richness as response variable and treatment as dependent variable. Biomass data was log-transformed (adding 1 before the transformation) to improve normality and homogeneity of variance assessed visually by Q-Q plots and box plots. To assess the effect of treatment on the density of each species, we performed one-way ANOVAs (GLM) assuming density to follow a Poisson distribution. Before performing the ANOVAs, we confirmed that there was no significant interaction between replicate sites and treatment. Least square means post hoc analyses were performed to test the pairwise difference between treatments. Finally, in order to account for probable inter-annual variation in faunal recolonization, a one-way ANOVA was conducted to compare faunal density between years in the “Bare (annual)” treatment.
The Effect of Canopy-Forming Algae on Temperature and Ice Scouring
For the comparison of extreme temperatures measured in “Full canopy” and “Bare (start),” expected to reflect air temperatures during low tide, the 5th and 95th percentiles of temperature measurements were calculated for each month, year and the entire 3-year period. Means of the percentiles in each month, each year and the entire 3-year period between the “Full canopy” and “Bare (start)” were compared using a two-sample t-test.
To assess the variation in ice scouring intensity across replicate sites between years, the relationship between the maximum degree of the bending of screws from 2011 to 2012 and 2013 to 2014 was examined by linear regression. Then, to assess the effect of the main treatments on ice scouring, we performed a one-way ANOVA with the maximum degree of the bending of screws (2013–14) as the response variable. Similarly, a one-way ANOVA was performed to assess whether rock rugosity differed between the main treatments. Assumptions of normality and homogeneity of variance were assessed visually by Q-Q plots and box plots.
Rock Rugosity and Recolonization
In the following analysis, we treated the 15 quadrats in the main treatments from 2014 as independent data points since rock rugosity did not differ between treatments (Table 4C). Relationships between faunal recolonization (densities or biomasses) and rock rugosity were assessed with linear regression and multiple linear regression (MLR). Similarly, the relationship between A. nodosum recruitment density and rock rugosity was assessed by linear regression. All above analyses were performed using SAS statistical software 9.4 (SAS Institute Inc. Cary, NC, USA).
Recovery Period and Early Growth Rate of Ascophyllum Nodosum
The early growth rate of A. nodosum recruited during the study period was quantified based on yearly length measurements of recruits in “Bare (annual)” and “Bare (start).” In total, 30 quadrat measurements (10 quadrat measures × 3 years) were used for the cohort analysis. Since we expected recruit lengths in “Bare (start)” to reflect multiple age groups of 2–3 years after experiment start, we applied Hartigan's diptest (Hartigan, 1985), testing the presence of multiple age groups with the R (R Core Team, 2017) package diptest (Maechler, 2016). By comparing the maximum difference between the observed distribution and a unimodal distribution, which minimizes this difference, Hartigan's diptest statistically tests the null hypothesis of unimodality. If p < 0.05, the alternative hypothesis of bi- or multimodality is accepted, i.e., the presence of multiple age groups. Subsequently, the Expectation-Maximization (EM) algorithm (Redner and Walker, 1984) was used to estimate the mean length of recruits within each age group with the R package mixtools (Benaglia et al., 2009). This algorithm uses the maximum likelihood method to find the value of each peak in a multimodal distribution. Here, we looked for three peaks, because we expected new recruits each year in “Bare (start),” i.e., three age groups.
Finally, the growth rate of A. nodosum was calculated with linear regression based on the estimated mean lengths within each age group of individuals recruited in the period August 2011 - August 2014. Additional quantification of algal recruit lengths was conducted in August 2016, i.e., 2 years after the final harvest of all quadrats, providing 20 additional estimates similar to the treatment “Bare (start).” This almost doubled the sample area (30 quadrat measurements + 20 quadrat measurements = 50 quadrat measurements), strengthening the overall estimate of early growth rates of algal recruits in inner Kobbefjord. We used the estimated early growth rates for A. nodosum recruits to evaluate the time it takes from initial settling to the recovery of the “Pre-experimental” canopy mean length in Kobbefjord. This was based on the growth pattern for A. nodosum found by Viana et al. (2014), showing linear growth during the first 2 years and, thereafter, exponential growth until a certain length set by abrasion. We calculated the length of A. nodosum after 2 years of linear growth with our early growth rate, and inserted this (as b) in a natural exponential function, which we solved for x (years):
where y is the mean length of “Pre-experimental” canopy, a is the adult relative growth rate of A. nodosum from inner Kobbefjord, calculated as the mean linear growth rate (4.92 cm yr−1, Marbà et al., 2017) relative to the intermediate shoot length after starting exponential growth (i.e., length after 2 years + mature length)/2. Finally, we added the first 2 years to achieve the estimate of years it takes to reach the mean length of “Pre-experimental” canopy.
Results
Canopy-Forming Algae in Inner Kobbefjord
Based on the pre-experimental monitoring in August 2011 [“Bare (start)”], the mid intertidal zone of the inner part of Kobbefjord was dominated by the canopy-forming fucoid, A. nodosum, with the occasional presence of F. vesiculosus. The average length of the A. nodosum canopy (> 10 cm) was 46.9 ± 6.0 cm and the minimum age was 5.9 ± 1.3 years for the “Pre-experimental” community (n = 5).
Figure 2 shows the combined biomass and densities of A. nodosum and F. vesiculosus in each treatment, noting that A. nodosum represented over 99% of the total observed biomass and adult density, and over 80% of the observed recruit density. The macroalgal biomass in the “Pre-experimental” community was on average 31 kg m−2, which was not significantly different from the macroalgal biomass found in either “Full canopy” and “Reduced canopy” in August 2014, three years after experiment start (Table 2, Figure 2). In contrast, the “Bare (start)” treatment, which was fully cleared at the beginning of the experiment, revealed minimal recovery in macroalgal biomass compared to “Pre-experimental,” supporting only 0.11 kg m−2 by August 2014 (Table 2, Figure 2). In addition, the density of macroalgal adults (>10 cm) in “Bare (start)” showed minimal recovery by August 2014 compared to “Pre-experimental,” while “Full canopy,” “Reduced canopy” and “Pre-experimental” displayed a similar level of adult density (Table 2, Figure 2). However, as the adult density in “Pre-experimental” was slightly higher than in “Full canopy” (Table 2, Figure 2), this was not reflected in the biomass. The density of recruits (<10 cm) at the end of the experiment tended to be higher in all treatments than in “Pre-experimental” (Figure 2), yet differences were not significant (Table 2).
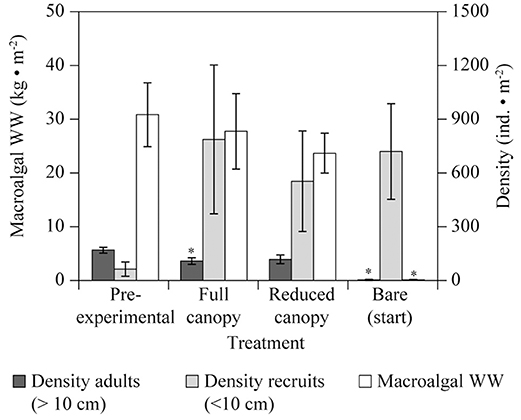
Figure 2. Mid intertidal macroalgal biomass (wet weight, WW) and density of algal recruits and adults, i.e., for A. nodosum and F. vesiculosus combined, in the main treatments “Full canopy,” “Reduced canopy,” and “Bare (start)” in Kobbefjord at the end of the experiment (August 2014). “Pre-experimental” was a baseline measurement based on the material cleared from “Bare (start)” at the start of the experiment (August 2011). *Annotates a significant difference of p < 0.05 for each treatment compared to “Pre-experimental” by a two-sample t-test. N = 5 for all mean values. Error bars are SE.
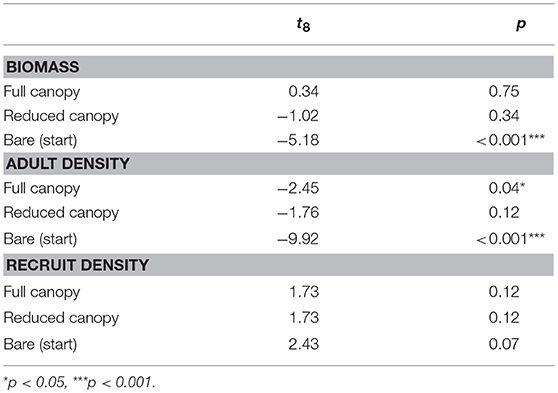
Table 2. Summary of two-sample t-tests comparing “Pre-experimental” macroalgal biomass, adult and recruit density to each treatment.
Algal Canopy and Faunal Recolonization
In total, we observed 11 faunal taxa in the study area (Table 3). Four species, S. balanoides, M. edulis, L. obtusata and L. saxatilis, contributed with 98.5% of the faunal biomass (wet weight). Three years after removal, the recovery of total faunal biomass relative to “Pre-experimental” was 26.1% for “Full canopy,” 28.5% for “Reduced canopy” and 1.4% for “Bare (start).” The recovery of faunal density was considerably faster than biomass for the dominant taxa, being 45.5% for “Full canopy,” 53.8% for “Reduced canopy” and 4.4% for “Bare (start).” Total faunal biomass nor density differed significantly from the “Pre-experimental” total faunal biomass or density.
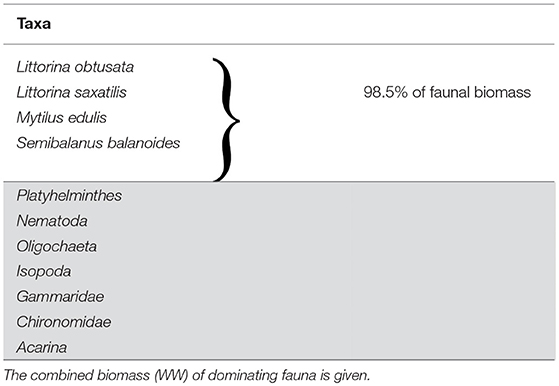
Table 3. Faunal taxa of “Pre-experimental” community in the mid intertidal zone of inner Kobbefjord, SW Greenland, August 2011.
In terms of biomass, L. obtusata, L. saxatilis and M. edulis were affected by canopy cover (Figure 3A, Table 4A), with L. obtusata showing a significantly higher biomass in both “Full canopy” and “Reduced canopy” compared to “Bare” (post-hoc LS test, Bare-Full canopy: p = 0.007, Bare-Reduced canopy: p = 0.004) and L. saxatilis showing a significantly higher biomass in “Reduced canopy” compared to both “Bare” and “Full canopy” (post-hoc LS test, Reduced canopy-Bare: p = 0.001, Reduced canopy-Full canopy: p = 0.042). Mytilus edulis displayed a significantly higher biomass in both “Full canopy” and “Reduced canopy” compared to “Bare” (post-hoc LS test, Bare-Full canopy: p < 0.001, Bare-Reduced canopy: p < 0.001). The density of all four species were affected by canopy cover (Figure 3B, Table 4A). Littorina obtusata displayed a significantly higher density in both “Full canopy” and “Reduced canopy” compared to “Bare” (post hoc LS test, Bare-Full canopy: p = 0.007, Bare-Reduced canopy: p = 0.004) and L. saxatilis showed significantly higher density in “Reduced canopy” compared to “Bare” and “Full canopy” (post-hoc LS test, Reduced canopy-Bare: p < 0.001, Reduced canopy-Full canopy: p = 0.003) (Figure 3B). Mytilus edulis displayed a higher density in both “Full canopy” and “Reduced canopy” compared to “Bare” (post-hoc LS test, Bare-Full canopy: p < 0.001, Bare-Reduced canopy: p < 0.001) (Figure 3B) and S. balanoides displayed a higher density in “Reduced canopy” compared to “Bare” (post-hoc LS test, Reduced canopy-Bare: p = 0.004) (Figure 3B). Moreover, faunal species richness was significantly affected by canopy cover (Table 4B), displaying a higher species richness in “Full canopy” and “Reduced canopy” compared to “Bare” (post-hoc LS test, Bare-Full canopy: p = 0.008, Bare-Reduced canopy: p = 0.026). The inter-annual recolonization of M. edulis density, based on measurements in “Bare (annual),” was not significantly different between years [ANOVA, F(2, 12) = 0.45, p = 0.65]. However, S. balanoides density was twice as high in 2012 as in the following 2 years [ANOVA, F(2, 12) = 5.39, p = 0.021].
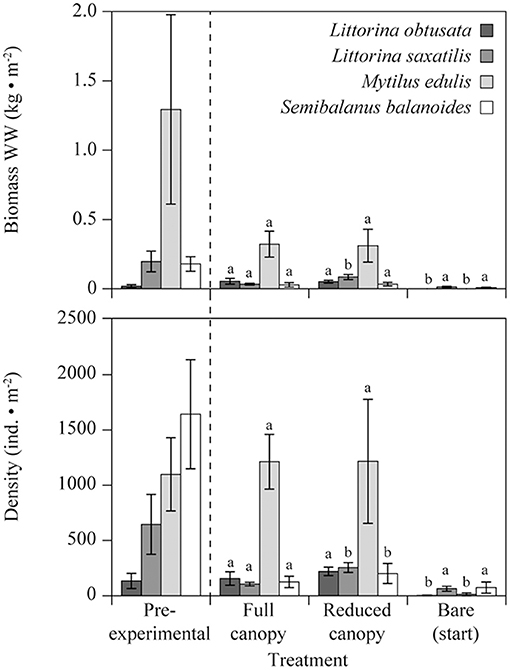
Figure 3. (A) Biomass and (B) density of dominating intertidal faunal species in Kobbefjord across the treatments “Full canopy.” “Reduced canopy” and “Bare (start)” measured in August 2014 and the “Pre-experimental” community measured in August 2011. Letters annotate significant differences of p < 0.05 by a post-hoc test between main treatments for each species, i.e., excluding “Pre-experimental.” N = 5 for all mean values. Error bars are SE.
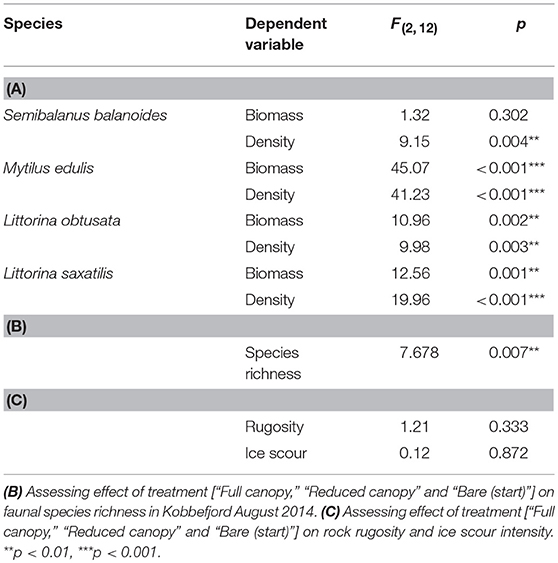
Table 4. Summary statistics of one-way ANOVAs (A) assessing the effect of treatment [“Full canopy,” “Reduced canopy” and “Bare (start)”] on biomass and density of dominating intertidal faunal species in Kobbefjord August 2014.
Modifying Properties of the Canopy-Forming Algae
Sea ice presence varied between years, with just 2 months of sea ice cover in the inner fjord in 2013, compared to 4–5 months of sea ice cover in 2012 and 2014 (Figure 4). We were unable to quantify the ice scouring intensity at site 1 and 2 in 2011–2012 due to loss of screws. However, the maximum value of the two screws in each quadrat, showed a similar pattern across sites in 2011-2012 and 2013-2014 [LR, R2 = 0.30, F(1, 13) = 5.556, p = 0.035], indicating that the variation in ice scouring intensity was consistent across sites between years. Therefore, we used the more complete dataset from 2013–2014 as a proxy for ice scouring intensity in the inner Kobbefjord. The maximum ice scouring intensity in terms of screw bending ranged between 0 and 75° across all quadrats, and maximum ice scouring intensity (2013–2014) did not differ significantly between treatments (Table 4C).
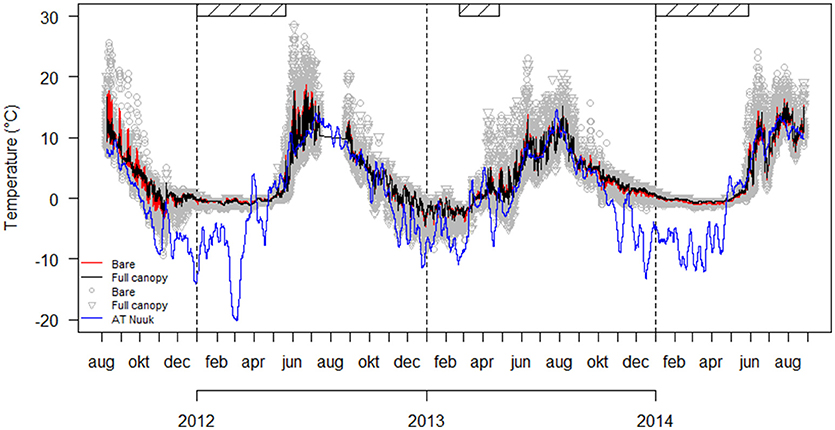
Figure 4. Temperature with running mean (10 observations) recorded in mid intertidal at inner Kobbefjord for the two treatments “Full canopy” and “Bare (start)” from August 2011 through August 2014 (data not available for July 14th–Aug 24th 2012). Air temperature (AT) with running mean (336 observations) recorded in Nuuk by the Greenland Ecosystem Monitoring program in the same period. Hatched bars represent periods of sea ice cover in inner Kobbefjord based on automatized photographing (data only available for January through May).
The running mean temperature measured in “Full canopy” and “Bare (start),” along with the running mean air temperature (AT) measured in Nuuk city from August 2011 to August 2014 are illustrated in Figure 4. Here, we show a slight difference between the mean temperatures in “Full canopy” compared to “Bare (start).” However, in order to uncover and compare the extreme temperatures measured in “Full canopy” and “Bare (start),” we extracted the 5th and 95th percentiles (Table 5, see Supplementary Table 1 for monthly comparisons). We expect these to reflect air temperatures during low tide since the 5th percentiles in winter and the 95th percentiles in summer are below and above the typical range of sea surface temperatures in this area (Versteegh et al., 2012). The means of the percentiles across the 3-year period differed significantly between “Full canopy” and “Bare (start),” suggesting that algal cover buffers the temperature variations (Table 5). Testing the percentiles of years separately also showed that the extreme temperatures of 2011–2012 and 2013–2014 differed significantly between the two treatments, while this was not the case for 2012–2013 (Table 5). Testing the percentiles of each month separately showed that mainly summer and winter months differed between treatments, especially so for colder months (see Supplementary Table 1). In some summer months, canopy cover reduced the 5% highest temperatures by a maximum of 5.5°C and during winter months the 5% lowest temperatures were raised by a maximum of 0.5°C, again with canopy-forming algae reducing temperature extremes.
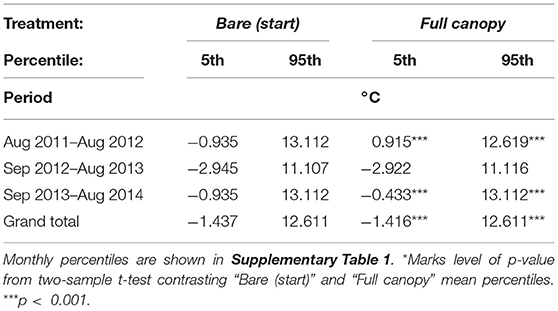
Table 5. 5th (extreme low) and 95th (extreme high) percentiles of yearly temperatures (°C) in treatment “Full canopy” and “Bare (start)” in inner Kobbefjord mid intertidal from August 2011 to August 2014.
Rock Rugosity and Recolonization
Rock rugosity did not differ significantly between the three main treatments (Table 4C). Therefore, we chose to combine all 15 quadrats in a linear regression to test the relation between recruitment and rugosity. As opposed to all other invertebrate taxa, the total density of M. edulis showed a positive linear relationship with rock rugosity [LR, R2 = 0.551, F(1, 13) = 18.21, p < 0.001] [see Supplementary Table 2 in Supplementary Material for full (M)LR details]. With a multiple linear regression, we show that both canopy biomass and rock rugosity influenced the total M. edulis density positively [MLR, R2 = 0.726, F(2, 12) = 19.53, p < 0.001]. When corrected statistically for canopy biomass, rock rugosity explained 62% of the variation in mussel density (R2 = 0.62, p < 0.001) and when corrected statistically for rock rugosity, canopy biomass explained 44% (R2 = 0.44, p = 0.010). In addition, A. nodosum recruit density showed a positive linear relationship with rock rugosity [LR, R2 = 0.419, F(1, 13) = 11.11, p = 0.005].
Recovery Period of Ascophyllum Nodosum
We estimated the early growth rate of A. nodosum recruits in the period August 2011–August 2014 to be 1.4 cm yr−1. This was based on Hartigan's diptest (HD) that showed the distribution of recruit lengths to be multimodal, representing three age groups (HD, n = 76, D = 0.06, p = 0.042), for which we estimated mean lengths with the Expectation-Maximization algorithm. The addition of the algal recruit length data from 20 quadrats, cleared in August 2014 and quantified in August 2016, increased the sample size 9 times. This strengthened analysis (HD, n = 673, D = 0.04, p < 0.001) identified a similar early growth rate of 2.0 cm yr−1. Based on the strengthened estimate of early growth rate, resulting in 4 cm long shoots after 2 years of linear growth, and assuming an exponential growth pattern thereafter with an estimated growth rate of 0.19 % yr−1 [4.92 cm yr−1/(4 + 46.9 cm)/2], we estimated that it would take ca 15 years (including the first 2 years of linear growth) for A. nodosum recruits to reach the mean length of the “Pre-experimental” canopy in inner Kobbefjord.
Discussion
Macroalgal Canopy Cover Facilitates Faunal Recolonization
The recolonization of M. edulis, S. balanoides and the two snails L. obtusata and L. saxatilis displayed a positive response to macroalgal canopy cover (predominately A. nodosum) at the mid intertidal level in inner Kobbefjord. These findings support the hypothesis that algal canopy has an overall facilitating effect on faunal recolonization in a sub-Arctic intertidal. This is opposed to findings from a temperate intertidal, where an overall neutral response in barnacle recruitment to A. nodosum canopy cover was observed at both mid- and high intertidal levels (Beermann et al., 2013). Other studies find positive biotic responses to algal canopy cover confined to the high intertidal (Dayton, 1971; Hawkins, 1983; Jenkins et al., 1999b). In relation to the stress gradient hypothesis, we have already suggested that in a stressful environment like the sub-Arctic, the positive effects of canopy cover in modifying the environment are likely to overrule the negative effects such as space competition and whiplashes from algal fronds. To further verify this hypothesis, we explored the effect of algal canopy in modifying the key stressors in the sub-Arctic intertidal, i.e., extreme air temperature and sea ice scouring.
Macroalgal Canopy Cover Modifies Extreme Temperatures
While earlier studies from Greenland have reported marked diurnal and seasonal temperature variation in the sub-Arctic intertidal (Høgslund et al., 2014), our results take a step further and document that the A. nodosum canopy created significant small-scale spatial variation in temperature by acting as an insulating cover. The macroalgal cover in inner Kobbefjord reduced the highest 5% temperatures in summer by up to 5.5°C and raised the lowest 5% in winter by up to 0.5°C (see Supplementary Table 1). Thus, A. nodosum canopy buffers extreme air temperatures experienced by organisms in a sub-Arctic rocky intertidal. This pattern is consistent with studies from the temperate intertidal where the daily maximum temperature, measured over a month, was lowered by the canopy cover in the mid- and high intertidal zone (Beermann et al., 2013; Watt and Scrosati, 2013a). Therefore, we suggest that the ability of algal canopy to buffer extreme temperatures, likely contributes to the overall positive biotic response to canopy cover found at this study site. In contrast, the canopy-forming algae had no effect on ice scouring intensity, indicating that the canopy did not markedly protect the understory community from the mechanical disturbance of sea ice scouring, which is consistent with previous studies describing A. nodosum's low capacity to withstand ice scouring (Åberg, 1992).
The extreme temperatures measured in this study were within the mean lower and upper thermal limits of the dominating intertidal faunal species, L. saxatilis, L. obtusata, S. balanoides, and M. edulis, documented in Scotland (−16.4–35°C, Davenport and Davenport, 2005). However, the current measures of thermal lethal limits in southern populations may not be the same as for sub-Arctic populations. As an example, M. edulis populations in Canada (Bourget, 1983) displayed lower, mean lower lethal limits than the populations in Scotland (Davenport and Davenport, 2005). Moreover, mortality rates at increasing temperatures may differ depending on life stage (Foster, 1971a; Bourget, 1983) and most studies include only adult life stages.
In inner Kobbefjord mid intertidal, the minimum 5th percentile temperature was −6.0°C and the maximum 95th percentile was 22°C (see Supplementary Table 1 in Supplementary Material). These temperatures are expected to increase in the future, based on a warming trend in mean annual air temperatures in west Greenland (Thyrring et al., 2017). In a nearby climate station, the air temperatures have increased by 0.13 ± 0.02°C per year over the last three decades together with an increase in the duration of annual ice-free cover along the Greenland west coast (Marbà et al., 2017). Thus, in future scenarios, the ability of algal canopy to buffer temperature extremes may become more important in preventing increased mortality in understory faunal communities, both with regard to warmer air temperatures in summer and colder air temperatures in winter, as the annual sea ice cover decreases. For example, it was evident that sea ice was buffering cold air temperatures during winter at the studied site, as we observed a marked difference between air temperatures (AT) and temperatures measured in the intertidal during winter compared to the other seasons. During the winter 2013, with only 2 months of sea ice cover in Kobbefjord, the temperatures from the intertidal also showed greater variation than in the other two winters with 5 months of sea ice cover (2012, 2014, Figure 4).
Multiple other physico-chemical stress factors, not included in our study, such as desiccation, water flow, wave action, salinity and pH, may also be altered by canopy-forming algae, contributing to the overall positive biotic response to canopy cover (Helmuth, 1998; Jenkins et al., 1999a; Beermann et al., 2013; Wahl et al., 2018).
Rock Rugosity Facilitates Recolonization
The coupling between rock roughness and the colonization of intertidal species has been noted before (Guidetti et al., 2004; Skinner and Coutinho, 2005; Chase et al., 2016). In the studied area, M. edulis is, in general, confined to cracks and crevices in the intertidal zone (Blicher et al., 2013) and here, we show that the total recolonization of M. edulis across the main treatments was positively related to both rock rugosity and macroalgal biomass. Moreover, A. nodosum canopy may contribute to an additional level of rugosity, in that holdfasts attached to the rock, as well as the canopies above, form multiple crevices for settlement. This type of rugosity, habitat complexity and increased surface area for colonization may contribute to the overall positive effect that the A. nodosum canopy has on faunal recolonization. In addition, we found that recolonization of A. nodosum was positively related to rock rugosity, suggesting that rock roughness is important for the settlement and early development of germlings, likely explained by an increase in settling surface and protection from ice scouring and other physical stressors in the small crevices (Heaven and Scrosati, 2008; Gerwing et al., 2015; Musetta-Lambert et al., 2015).
Recovery Time of the Intertidal Community
In a sub-Arctic intertidal, where mechanical disturbance such as ice scouring may dislodge the entire community, we show that, for both fauna and macroalgae, the subsequent recovery in density is faster than in biomass. Thus, while the organisms recolonize relatively fast after maximum disturbance, it takes longer to recover the biomass of the intertidal community. On the other hand, when A. nodosum individuals prevail and the canopy is simply sliced by ice scouring, the algal biomass fully recovers after 3 years, and the recolonization rates resemble those found in an untouched canopy.
The early growth rate of newly-settled A. nodosum in the studied intertidal community averaged 1.4–2 cm yr−1. Comparably, the growth rate of adult A. nodosum measured in the same macroalgal community is 4.92 cm yr−1 (Marbà et al., 2017). Early growth rates of A. nodosum recruits (≤ 2 years old) from the Isle of Man and south west Spain are reported at 7.2 cm yr−1 and 9.6 cm yr−1, respectively (Cervin et al., 2005; Viana et al., 2014). However, in Maine, USA, Keser and Larson (1984) reported an early growth rate of 1.6 cm yr−1, which is similar to what we found. Based on existing growth patterns for this species (Viana et al., 2014), but modified for the local conditions, we estimated that A. nodosum in inner Kobbefjord would reach its full length, averaging 46.9 cm, ca 15 years after removal by mechanical disturbance. Correspondingly, we found the macroalgal biomass to be very low, even after 3 years of recovery. The estimated recovery period, in height, is higher than the minimum mean age (6 years) of unbroken branches of A. nodosum found in this study, bearing in mind that holdfasts of A. nodosum have been suggested to be 50–70 years old, and the community has been referred to as “marine trees” (Olsen et al., 2010), displaying similar patterns of slow population growth as terrestrial trees (Capdevila et al., 2016). However, it is close to the minimum age of the longest individuals, assessed as the presence of 13 full years of consecutive growth, of A. nodosum individuals also observed in Kobbefjord (Figure 3 in Marbà et al., 2017). A long recovery period of A. nodosum height corresponds to findings in north temperate regions (Printz, 1956; Cervin et al., 2005; Ingólfsson and Hawkins, 2008). However, they largely assign a delay in A. nodosum biomass recovery to the competition from other fucoid species. In inner Kobbefjord, algal recruits on bare rock were dominated by A. nodosum, with a few F. vesiculosus, suggesting that recovery of A. nodosum in inner Kobbefjord was not delayed by interspecific competition. Rather, the slow recovery of A. nodosum canopy height may be largely due to the constraints of the abiotic environment. Whereas our study assessed the recovery time of canopy height, a complete study of recovery of canopy biomass should also include quantification of population dynamics in terms of recruitment and mortality. Recruitment and mortality rates in the studied A. nodosum population, assessed through the Greenland Ecosystem Monitoring program, showed that the vast majority of the biomass was quite persistent with loss rates of individuals of only 1.6–6.2% per year, whereas the pool of new shoots add markedly to the population dynamics, resulting in overall recruitment rates of 6–111% and mortality rates of 1.6–60% for the period 2012–2015. However, the overall population growth ensured a biomass increase of the population of 1–50% yr−1 during the assessment period 2012–15, which, by far, outweighed the biomass of lost individuals (Juul-Pedersen et al., 2015).
Ascophyllum nodosum mainly dominates in wave-sheltered areas (Scrosati and Heaven, 2008), while other fucoid species, such as F. vesiculosus and F. distichus/F. evanescens takes over at more exposed sites as well as above the northern distributional range of A. nodosum. Like A. nodosum, we expect these other fucoid species to display a similar buffering effect on extreme temperatures and facilitate the colonization of intertidal species. However, buffering capacity most likely depends on the level of canopy biomass, which was high in the studied area.
Based on future projections on climate change, the distributional ranges of both A. nodosum and F. vesiculosus are expected to shift northward (Jueterbock et al., 2013). A northward shift in other high latitude intertidal macroalgal communities have already been documented (Weslawski et al., 2010), and a recent study found that warming tends to enhance the growth of A. nodosum at its northern distribution limit (Marbà et al., 2017). In comparison, the southern distribution limits of dominant intertidal fauna, M. edulis and S. balanoides, are also moving northward (Jones et al., 2010, 2012), and based on our results, algal canopies may be a key habitat in facilitating the northward colonization and abundance of these intertidal species at their northern distribution limit (Gilman et al., 2010).
Conclusion
This study emphasizes the role of A. nodosum canopy and rocky rugosity in structuring high latitude intertidal communities. We show that intertidal canopy-forming macroalgae have an overall facilitating effect on faunal species richness and recolonization, likely by reducing temperature variations and increasing settling surfaces and habitat complexity. We show that the canopy modifies the small-scale variation in temperature experienced by intertidal fauna, but fails to reduce ice scouring at the rock surface, underlining the complexity in how a physical stressor can vary depending on scale. The facilitating properties of algal canopies are important to consider when predicting future distributional patterns of high latitude intertidal fauna. To fully comprehend the community-structuring role of algal canopies, we need to study whether and how they modify other key stressors, such as salinity, pH, water loss and flow, including different intertidal levels. Finally, we estimated that the full establishment and recovery period of the intertidal community may take at least 15 years while biomass and height of A. nodosum canopy builds up, which is important to consider when predicting latitudinal range shifts in the sub-Arctic and Arctic intertidal community.
Data Availability Statement
The raw data supporting the conclusions of this manuscript will be made available by the authors, without undue reservation, to any qualified researcher. The air temperature data from Nuuk was obtained from Greenland Ecosystem Monitoring program (GEM). Requests to access these datasets should be directed to http://data.g-e-m.dk/. The long time series of photos documenting the sea ice in Kobbefjord was obtained from the Andreas Westergaard-Nielsen and request to access these photos should be directed to Andreas Westergaard-Nielsen, (awn@ign.ku.dk).
Author Contributions
All authors have approved the submission version. MS, DK-J, KM, and BO conceived and planned the experiment. DK-J, KM, BO, NM, SØ, MB, and ML conducted the field work. Data analysis was led by SØ with input from DK-J, MS, KM, and BO. SØ produced all figures and wrote the first draft with contributions and comments from all the authors.
Conflict of Interest Statement
ML is employed by company Danish Center for Wild Salmon.
The remaining authors declare that the research was conducted in the absence of any commercial or financial relationships that could be construed as a potential conflict of interest.
Acknowledgments
This project was funded by the Danish Environmental Protection Agency's Arctic initiative (DANCEA) and by the Arctic Research Centre at Aarhus University. We are thankful to Anissa Merzouk for helping with the fieldwork sampling, and we are thankful to Jakob Thyrring and Søren Larsen for support on data analysis. We thank Andreas Westergaard-Nielsen for providing photos of the sea ice cover in Kobbefjord, and we thank the Greenland Ecosystem Monitoring program (www.G-E-M.dk) for providing weather data from Nuuk city. We are also grateful to the Greenland Institute of Natural Resources (GINR), Nuuk, Greenland, and their staff for providing excellent working conditions.
Supplementary Material
The Supplementary Material for this article can be found online at: https://www.frontiersin.org/articles/10.3389/fmars.2018.00332/full#supplementary-material
References
Åberg, P. (1992). A demographic study of two populations of the seaweed Ascophyllum nodosum. Ecology 73, 1473–1487. doi: 10.2307/1940691
Åberg, P. (1996). Patterns of reproductive effort in the brown alga Ascophyllum nodosum. Mar. Ecol. Prog. Ser. 138, 199–207. doi: 10.3354/meps138199
Beermann, A. J., Ellrich, J. A., Molis, M., and Scrosati, R. A. (2013). Effects of seaweed canopies and adult barnacles on barnacle recruitment: the interplay of positive and negative influences. J. Exp. Mar. Biol. Ecol. 448, 162–170. doi: 10.1016/j.jembe.2013.07.001
Benaglia, T., Chauveau, D., Hunter, D. R., and Young, D. (2009). Mixtools: an R package for analyzing finite mixture models. J. Stat. Softw. 32, 1–29. doi: 10.18637/jss.v032.i06
Bertness, M. D., and Callaway, R. (1994). Positive interactions in communities. Trends Ecol. Evol. 9, 187–191. doi: 10.1016/0169-5347(94)90087-6
Bertness, M. D., Leonard, G. H., Levine, J. M., Schmidt, P. R., and Ingraham, A. O. (1999). Testing the relative contribution of positive and negative interactions in rocky intertidal communities. Ecology 80, 2711–2726. doi: 10.2307/177252
Blicher, M. E., Sejr, M. K., and Hogslund, S. (2013). Population structure of Mytilus edulis in the intertidal zone in a sub-Arctic fjord, SW Greenland. Mar. Ecol. Prog. Ser. 487, 89–100. doi: 10.3354/Meps10317
Bourget, E. (1983). Seasonal variations of cold tolerance in intertidal mollusks and relation to environmental conditions in the St. Lawrence Estuary. Can. J. Zool. 61, 1193–1201. doi: 10.1139/z83-162
Broitman, B. R., Szathmary, P. L., Mislan, K. A. S., Blanchette, C. A., and Helmuth, B. (2009). Predator-prey interactions under climate change: the importance of habitat vs. body temperature. Oikos 118, 219–224. doi: 10.1111/j.1600-0706.2008.17075.x
Capdevila, P., Hereu, B., Llu, J., and Linares, C. (2016). Unravelling the natural dynamics and resilience patterns of underwater Mediterranean forests: insights from the demography of the brown alga Cystoseira zosteroides. J. Ecol. 104, 1799–1808. doi: 10.1111/1365-2745.12625
Cervin, G., Åberg, P., and Jenkins, S. R. (2005). Small-scale disturbance in a stable canopy dominated community: implications for macroalgal recruitment and growth. Mar. Ecol. Prog. Ser. 305, 31–40. doi: 10.3354/meps305031
Cervin, G., Lindegarth, M., Viejo, R. M., and Åberg, P. (2004). Effects of small-scale disturbances of canopy and grazing on intertidal assemblages on the Swedish west coast. J. Exp. Mar. Biol. Ecol. 302, 35–49. doi: 10.1016/j.jembe.2003.09.022
Chase, A. L., Dijkstra, J. A., and Harris, L. G. (2016). The influence of substrate material on ascidian larval settlement. Mar. Pollut. Bull. 106, 35–42. doi: 10.1016/j.marpolbul.2016.03.049
Crowe, T. P., Cusson, M., Bulleri, F., Davoult, D., Arenas, F., Aspden, R., et al. (2013). Large-scale variation in combined impacts of canopy loss and disturbance on community structure and ecosystem functioning. PLoS ONE 8:e6623. doi: 10.1371/journal.pone.0066238
Davenport, J., and Davenport, J. L. (2005). Effects of shore height, wave exposure and geographical distance on thermal niche width of intertidal fauna. Mar. Ecol. Prog. Ser. 292, 41–50. doi: 10.3354/meps292041
Dayton, P. K. (1971). Competition, disturbance, and community organization: the provision and subsequent utilization of space in a rocky intertidal community. Ecol. Monogr. 41, 351–389. doi: 10.2307/1948498
Denny, M. W., and Harley, C. D. G. (2006). Hot limpets: predicting body temperature in a conductance-mediated thermal system. J. Exp. Biol. 209, 2409–2419. doi: 10.1242/jeb.02257
Foster, B. A. (1971a). Desiccation as a factor in the intertidal zonation of barnacles. Mar. Biol. 8, 12–29. doi: 10.1007/BF00349341
Foster, B. A. (1971b). On the determinants of the upper limit of intertidal distribution of barnacles (Crustacea: Cirripedia). J. Anim. Ecol. 40, 33–48. doi: 10.2307/3328
Gendron, L., Merzouk, A., Bergeron, P., and Johnson, L. E. (2017). Managing disturbance: the response of a dominant intertidal seaweed Ascophyllum nodosum (L.) Le Jolis to different frequencies and intensities of harvesting. J. Appl. Phycol. 30, 1–16. doi: 10.1007/s10811-017-1346-5
Gerwing, T. G., Drolet, D., Barbeau, M. A., Hamilton, D. J., and Allen Gerwing, A. M. (2015). Resilience of an intertidal infaunal community to winter stressors. J. Sea Res. 97, 40–49. doi: 10.1016/j.seares.2015.01.001
Gilman, S. E., Urban, M. C., Tewksbury, J., Gilchrist, G. W., and Holt, R. D. (2010). A framework for community interactions under climate change. Trends Ecol. Evol. 25, 325–331. doi: 10.1016/j.tree.2010.03.002
Guidetti, P., Bianchi, C. N., Chiantore, M., Schiaparelli, S., Morri, C., and Cattaneo-Vietti, R. (2004). Living on the rocks: substrate mineralogy and the structure of subtidal rocky substrate communities in the Mediterranean Sea. Mar. Ecol. Prog. Ser. 274, 57–68. doi: 10.3354/meps274057
Gutt, J. (2001). On the direct impact of ice on marine benthic communities, a review. Polar Biol. 24, 553–564. doi: 10.1007/s003000100262
Hartigan, P. M. (1985). The dip test of unimodality. Ann. Stat. 13, 70–84. doi: 10.1214/aos/1176346577
Hawkins, S. J. (1983). Interactions of Patella and macroalgae with settling Semibalanus balanoides (L.). J. Exp. Mar. Bio. Ecol. 71, 55–72. doi: 10.1016/0022-0981(83)90104-1
Heaven, C. S., and Scrosati, R. A. (2008). Benthic community composition across gradients of intertidal elevation, wave exposure, and ice scour in Atlantic Canada. Mar. Ecol. Prog. Ser. 369, 13–23. doi: 10.3354/meps07655
Helmuth, B. (1998). Intertidal mussle microclimates: predicting the body temperature of a sessile invertebrate. Ecol. Monogr. 68, 51–74. doi: 10.2307/2657143
Helmuth, B., Broitman, B. R., Yamane, L., Gilman, S. E., Mach, K., Mislan, K. A. S., et al. (2010). Organismal climatology: analyzing environmental variability at scales relevant to physiological stress. J. Exp. Biol. 213, 995–1003. doi: 10.1242/jeb.038463
HilleRisLambers, J., Harsch, M. A., Ettinger, A. K., Ford, K. R., and Theobald, E. J. (2013). How will biotic interactions influence climate change-induced range shifts? Ann. N. Y. Acad. Sci. 1297, 112–125. doi: 10.1111/nyas.12182
Høgslund, S., Sejr, M. K., Wiktor, J., Blicher, M. E., and Wegeberg, S. (2014). Intertidal community composition along rocky shores in South-west Greenland: a quantitative approach. Polar Biol. 37, 1549–1561. doi: 10.1007/s00300-014-1541-7
Howat, I. M., Joughin, I., and Scambos, T. A. (2007). Rapid changes in ice discharge from Greenland outlet glaciers. Science 315, 1559–1561. doi: 10.1126/science.1138478
Ingólfsson, A., and Hawkins, S. J. (2008). Slow recovery from disturbance: a 20 year study of Ascophyllum canopy clearances. J. Mar. Biol. Assoc. 88, 689–691. doi: 10.1017/S0025315408001161
Jenkins, S. R., Hawkins, S. J., and Norton, T. A. (1999a). Direct and indirect effects of a macroalgal canopy and limpet grazing in structuring a sheltered inter-tidal community. Mar. Ecol. Prog. Ser. 188, 81–92. doi: 10.3354/meps188081
Jenkins, S. R., Norton, T. A., and Hawkins, S. J. (1999b). Settlement and post-settlement interactions between Semibalanus balanoides (L.) (Crustacea: Cirripedia) and three species of fucoid canopy algae. J. Exp. Mar. Bio. Ecol. 236, 49–67. doi: 10.1016/S0022-0981(98)00189-0
Jenkins, S. R., Norton, T. A., and Hawkins, S. J. (2004). Long term effects of Ascophyllum nodosum canopy removal on mid shore community structure. J. Mar. Biol. Assoc. 84, 327–329. doi: 10.1017/S0025315404009221h
Jones, S. J., Lima, F. P., and Wethey, D. S. (2010). Rising environmental temperatures and biogeography: poleward range contraction of the blue mussel, Mytilus edulis L., in the western Atlantic. J. Biogeogr. 37, 2243–2259. doi: 10.1111/j.1365-2699.2010.02386.x
Jones, S. J., Southward, A. J., and Wethey, D. S. (2012). Climate change and historical biogeography of the barnacle Semibalanus balanoides. Glob. Ecol. Biogeogr. 21, 716–724. doi: 10.1111/j.1466-8238.2011.00721.x
Jueterbock, A., Tyberghein, L., Verbruggen, H., Coyer, J. A., Olsen, J. L., and Hoarau, G. (2013). Climate change impact on seaweed meadow distribution in the North Atlantic rocky intertidal. Ecol. Evol. 3, 1356–1373. doi: 10.1002/ece3.541
Juul-Pedersen, T., Arendt, K., Mortensen, J., Krawczyk, D., Retzel, A., Nygaard, R., et al. (2015). “Nuuk basic - the marinebasic programme,” in Nuuk Ecological Research Operations 9th Annual Report, ed.T. R. Topp-Jørgensen, E. Hansen, and J. Christensen. Aarhus University, DCE – Danish Centre for Environment and Energy, 46–66.
Keser, M., and Larson, B. R. (1984). Colonization and growth of Ascophyllum nodosum in Maine. J. Phycol. 20, 83–87. doi: 10.1111/j.0022-3646.1984.00083.x
Keser, M., Swenarton, J. T., and Foertch, J. F. (2005). Effects of thermal input and climate change on growth of Ascophyllum nodosum (Fucales, Phaeophyceae) in eastern Long Island Sound (USA). J. Sea Res. 54, 211–220. doi: 10.1016/j.seares.2005.05.001
Luckhurst, B. E., and Luckhurst, K. (1978). Analysis of the influence of substrate variables on coral reef fish communities. Mar. Biol. 49, 317–323. doi: 10.1007/BF00455026
Lüning, K. (1990). Seaweeds: Their Environment, Biogeography, and Ecophysiology. New York, NY: John Wiley and Sons, Inc.
Maechler, M. (2016). Diptest: Hartigan's Dip Test Statistic for Unimodality - Corrected. Available online at: https://cran.r-project.org/package=diptest
Marbà, N., Krause-Jensen, D., Olesen, B., Christensen, P. B., Merzouk, A., Rodrigues, J., et al. (2017). Climate change stimulates the growth of the intertidal macroalgae Ascophyllum nodosum near the northern distribution limit. Ambio 46, 119–131. doi: 10.1007/s13280-016-0873-7
McCook, L. J., and Chapman, A. R. O. (1991). Community succession following massive ice-scour on an exposed rocky shore: effects of Fucus canopy algae and of mussels during late succession. J. Exp. Mar. Biol. Ecol. 154, 137–169. doi: 10.1016/0022-0981(91)90161-O
Mikkelsen, D. M., Rysgaard, S., and Glud, R. N. (2008). Microalgal composition and primary production in Arctic sea ice: a seasonal study from Kobbefjord (Kangerluarsunnguaq), West Greenland. Mar. Ecol. Prog. Ser. 368, 65–74. doi: 10.3354/meps07627
Mosbech, A., Anthonsen, K. L., Blyth, A., Boertmann, D., Buch, E., Cake, D., et al. (2000). Environmental Oil Spill Sensitivity Atlas for the West Greenland Coastal Zone. Available online at: http://www.ens.dk
Motyka, R. J., Cassotto, R., Truffer, M., Kjeldsen, K., Van As, D., Korsgaard, N. J., et al. (2017). Asynchronous behavior of outlet glaciers feeding Godthåbsfjord (Nuup Kangerlua) and the triggering of Narsap Sermia's retreat in SW Greenland. J. Glaciol. 63, 1–21. doi: 10.1017/jog.2016.138
Musetta-Lambert, J. L., Scrosati, R. A., Keppel, E. A., Barbeau, M. A., Skinner, M. A., and Courtenay, S. C. (2015). Intertidal communities differ between breakwaters and natural rocky areas on ice- scoured Northwest Atlantic coasts Intertidal communities differ between breakwaters and natural rocky areas on ice-scoured Northwest Atlantic coasts. Mar. Ecol. Prog. Ser. 539, 19–31. doi: 10.3354/meps11484
Nutman, A. P., and Friend, C. R. L. (2009). New 1:20,000 scale geological maps, synthesis and history of investigation of the Isua supracrustal belt and adjacent orthogneisses, southern West Greenland: a glimpse of Eoarchaean crust formation and orogeny. Precambrian Res. 172, 189–211. doi: 10.1016/j.precamres.2009.03.017
Olsen, J. L., Zechman, F. W., Hoarau, G., Coyer, J. A., Stam, W. T., Valero, M., et al. (2010). The phylogeographic architecture of the fucoid seaweed Ascophyllum nodosum: an intertidal “marine tree” and survivor of more than one glacial-interglacial cycle. J. Biogeogr. 37, 842–856. doi: 10.1111/j.1365-2699.2009.02262.x
Petzold, W., Willers, M. T., and Scrosati, R. A. (2014). Visual record of intertidal disturbance caused by sea ice in the spring on the Atlantic coast of Nova Scotia. F1000Research 3:112. doi: 10.12688/f1000research.4439
Printz, H. (1956). Recuperation and recolonisation in Ascophyllum nodosum. Proc. Int. Seaweed Symp. 2, 194–197.
R Core Team (2017). A Language and Environment for Statistical Computing. Available online at: https://www.r-project.org/
Redner, R. A., and Walker, H. F. (1984). Mixture densities, maximum likelihood and the EM algorithm. Soc. Ind. Appl. Math. 26, 195–239. doi: 10.1137/1026034
Richter, A., Rysgaard, S., Dietrich, R., Mortensen, J., and Petersen, D. (2011). Coastal tides in West Greenland derived from tide gauge records. Ocean Dyn. 61, 39–49. doi: 10.1007/s10236-010-0341-z
Scrosati, R., and Eckersley, L. K. (2007). Thermal insulation of the intertidal zone by the ice foot. J. Sea Res. 58, 331–334. doi: 10.1016/j.seares.2007.08.003
Scrosati, R., and Heaven, C. (2008). Trends in abundance of rocky intertidal seaweeds and filter feeders across gradients of elevation, wave exposure, and ice scour in eastern Canada. Hydrobiologia 603, 1–14. doi: 10.1007/s10750-007-9160-8
Skinner, L. F., and Coutinho, R. (2005). Effect of microhabitat distribution and substrate roughness on barnacle Tetraclita stalactifera (Lamarck, 1818) settlement. Brazilian Arch. Biol. Technol. 48, 109–113. doi: 10.1590/S1516-89132005000100014
Steen, H., and Rueness, J. (2004). Comparison of survival and growth in germlings of six fucoid species (Fucales, Phaeophyceae) at two different temperature and nutrient levels. Sarsia 89, 175–183. doi: 10.1080/00364820410005818
Thyrring, J., Blicher, M. E., Sørensen, J. G., Wegeberg, S., and Sejr, M. K. (2017). Rising air temperatures will increase intertidal mussel abundance in the Arctic. Mar. Ecol. Prog. Ser. 584, 91–104. doi: 10.3354/meps12369
Versteegh, E. A. A., Blicher, M. E., Mortensen, J., Rysgaard, S., Als, T. D., and Wanamaker, A. D. (2012). Oxygen isotope ratios in the shell of Mytilus edulis: Archives of glacier meltwater in Greenland? Biogeosciences 9, 5231–5241. doi: 10.5194/bg-9-5231-2012
Viana, I. G., Bode, A., and Fernández, C. (2014). Growth and production of new recruits and adult individuals of Ascophyllum nodosum in a non-harvested population at its southern limit (Galicia, NW Spain). Mar. Biol. 161, 2885–2895. doi: 10.1007/s00227-014-2553-0
Wahl, M., Schneider Covach,ã, S., Saderne, V., Hiebenthal, C., Müller, J., Pansch, C., et al. (2018). Macroalgae may mitigate ocean acidification effects on mussel calcification by increasing pH and its fluctuations. Limnol. Oceanogr. 63, 3–21. doi: 10.1002/lno.10608
Walters, L. J., and Wethey, D. S. (1996). Settlement and early post-settlement survival of sessile marine invertebrates on topographically complex surfaces: the importance of refuge dimensions and adult morphology. Mar. Ecol. Prog. Ser. 137, 161–171. doi: 10.3354/meps137161
Watt, C. A., and Scrosati, R. A. (2013a). Bioengineer effects on understory species richness, diversity, and composition change along an environmental stress gradient: experimental and mensurative evidence. Estuar. Coast. Shelf Sci. 123, 10–18. doi: 10.1016/j.ecss.2013.02.006
Watt, C. A., and Scrosati, R. A. (2013b). Regional consistency of intertidal elevation as a mediator of seaweed canopy effects on benthic species richness, diversity, and composition. Mar. Ecol. Prog. Ser. 491, 91–99. doi: 10.3354/meps10521
Weslawski, J. M., Wiktor, J., and Kotwicki, L. (2010). Increase in biodiversity in the arctic rocky littoral, Sorkappland, Svalbard, after 20 years of climate warming. Mar. Biodivers. 40, 123–130. doi: 10.1007/s12526-010-0038-z
Keywords: biotic interactions, physical disturbance, rocky intertidal, community recovery, recruitment, Greenland
Citation: Ørberg SB, Krause-Jensen D, Mouritsen KN, Olesen B, Marbà N, Larsen MH, Blicher ME and Sejr MK (2018) Canopy-Forming Macroalgae Facilitate Recolonization of Sub-Arctic Intertidal Fauna and Reduce Temperature Extremes. Front. Mar. Sci. 5:332. doi: 10.3389/fmars.2018.00332
Received: 25 June 2018; Accepted: 28 August 2018;
Published: 19 September 2018.
Edited by:
Matthew H. Long, Woods Hole Oceanographic Institution, United StatesReviewed by:
Stein Fredriksen, University of Oslo, NorwayJan Marcin Weslawski, Institute of Oceanology (PAN), Poland
Copyright © 2018 Ørberg, Krause-Jensen, Mouritsen, Olesen, Marbà, Larsen, Blicher and Sejr. This is an open-access article distributed under the terms of the Creative Commons Attribution License (CC BY). The use, distribution or reproduction in other forums is permitted, provided the original author(s) and the copyright owner(s) are credited and that the original publication in this journal is cited, in accordance with accepted academic practice. No use, distribution or reproduction is permitted which does not comply with these terms.
*Correspondence: Sarah B. Ørberg, c2FvQGJpb3MuYXUuZGs=