- 1Instituto Mediterráneo de Estudios Avanzados, (CSIC-UIB), Esporles, Spain
- 2Red Sea Research Center, King Abdullah University of Science and Technology, Thuwal, Saudi Arabia
- 3Laboratori d'Investigacions Marines i Aqüicultura (Balearic Government), Illes Balears, Spain
Short-term hypoxia that lasts just a few days or even hours is a major threat for the marine ecosystems. The single effect of the human-induced levels of hypoxia and other anthropogenic impacts such as elevated pCO2 can reduce the ability of preys to detect their predators across taxa. Moreover, both processes, hypoxia and elevated pCO2, are expected to co-occur in certain habitats, but the synergic consequences of both processes and the ability of fish to recover remain unknown. To provide empirical evidence to this synergy, we experimentally evaluated the risk-taking behavior in juveniles of the European seabass (Dicentrachus labrax), an important commercial fisheries species after recovering from short-term hypoxia and different pH scenarios. The behavior of seabass juveniles was monitored in an experimental arena before and after the exposure to a simulated predator and contrasted to control fish (BACI design) (current levels of hypoxia and elevated pCO2) using a mechanistic function-valued modeling trait approach. Results revealed that fish recovering from elevated pCO2, alone or combined with hypoxia, presented less avoidance behavior in failing to seek refuge when a simulated predator was present in the arena compared to those exposed to control pCO2 levels. Our results show that recovery from short-term exposure to acidification and hypoxia was not synergistic and suggest that recovery from acidification takes longer than from short-term hypoxia treatment through a potential effect on the sensorial and hence behavioral capacities of fish.
Introduction
Worldwide marine costal ecosystems are decreasing their dissolved oxygen (DO) by 5.5 ± 0.2% year−1 (Vaquer-Sunyer and Duarte, 2008) and expected to decrease even faster in the future (Conley et al., 2009). Especially in a 30 km band along the coastline the decline in oxygen concentrations is more prevalent and acute (Gilbert et al., 2009). Furthermore shallow and enclosed bays with soft substrate, receiving riverine inputs, and showing high stratification are most likely to experience hypoxia (Stachowitsch and Avcin, 1988). In coastal ecosystems, short-term hypoxia can only last a few days or even hours and has been observed in various sites (Breitburg, 1990; Levin et al., 2009; Rabalais et al., 2010; Vaquer-Sunyer et al., 2012; Benito et al., 2015). In Chesapeake Bay, for example, 40% of the days DO values are below 2 mg L−1 and 10% below 1 mg L−1 (Breitburg, 1990; also see review Steckbauer et al., 2011), and in the Mediterranean Sea DO can reach up to 14.8 mg L−1 in March but decline below 2 mg L−1 during summer months and is directly correlated with high water temperatures (Benito et al., 2015). Ecosystems with sea grasses or macroalgae might experience hypoxia on a daily basis as a result of the DO fluctuations due to production during the day and respiration during the night (Tyler and Targett, 2007; Rabalais et al., 2010; Hendriks et al., 2014), which increases in the summer months due to higher temperatures (Vaquer-Sunyer et al., 2012; Melzner et al., 2013).
Although mobile species are more likely to escape hypoxic areas, it is known that they can be trapped or affected by it, as hypoxia leads to habitat loss, increased energetic costs, altered ecological performances, or even worse, result in direct mortality (Craig et al., 2001). Many examples across taxa demonstrate how increased severity of hypoxia induces behavioral changes in preys that make them more vulnerable to predators (Taylor and Eggleston, 2000; Mistri, 2004; Riedel et al., 2007). Fish are particularly vulnerable to coastal hypoxia, which often results in fish kills and “dead-zones” to fisheries (Vaquer-Sunyer and Duarte, 2008), with sublethal levels of hypoxia affecting predator-prey interactions through behavioral changes in prey that make them more vulnerable to predators (Taylor and Eggleston, 2000; Mistri, 2004; Riedel et al., 2007). Several fish species have shown a reduced swimming performance under hypoxia caused either directly or as a result of saving oxygen consumption for other activities such as digestion (Chabot and Claireaux, 2008), also affecting their vulnerability to predators (Domenici et al., 2007). Moreover, an increased severity of hypoxia not only affects individual organisms but the social structure of fish schools, making them more vulnerable to disruption and consequently decreasing their anti-predator abilities (Whoriskey et al., 1985; Domenici et al., 2007). Diel-cycling hypoxia might be less effectively avoided by fish and could provide chronic exposure that affects reproduction (Levin et al., 2009) and lowers the avoidance threshold for further hypoxic events (Brady and Targett, 2013). Some fish species have been shown to quickly recover their swimming ability and ventilating capacities after hypoxia (Farrell et al., 1998), and this rapid recovery can even confer them competitive benefits (Wu, 2001). Although anti-predator response has previously been evaluated under treatment conditions, no data exists of effects after short-term exposure.
Increasing anthropogenic CO2 emissions and decreasing seawater pH, a process termed Ocean Acidification (OA) in the open ocean (Orr et al., 2005), represents an emerging pressure in the ocean ecosystem (Doney et al., 2009; Kroeker et al., 2013). In eutrophied coastal areas, the trend toward increased CO2 is amplified by respiratory CO2 release associated with a decline in O2 concentrations in hypoxic areas (Cai et al., 2011; Duarte et al., 2013), which are proliferating worldwide (Diaz and Rosenberg, 2008; Vaquer-Sunyer and Duarte, 2008). Hence, increasing CO2 and declining O2 concentrations are often co-occurring stressors (Brewer and Peltzer, 2009; Mayol et al., 2012), which may amplify the responses of organisms to each individual stressor (Burgents et al., 2005; Kim et al., 2013; Rosa et al., 2013; Gobler et al., 2014). Yet, whereas the experimental assessment of the responses of marine organisms to elevated pCO2 has increased rapidly (Hendriks et al., 2010; Kroeker et al., 2013), assessments of the responses to the combined levels of increasing CO2 and declining O2 concentrations are scarce (Burgents et al., 2005; Kim et al., 2013; Rosa et al., 2013; Gobler et al., 2014). In parallel, evidence that elevated pCO2 induces changes in the behavior of prey affecting its interactions with the predators has been reported (Dixson et al., 2010). Those authors observed altered behavior of larval fish at 700 ppm CO2, with many individuals becoming attracted to the smell of predators and completely impaired ability to sense predators at 850 ppm CO2. Fish are especially vulnerable to elevated pCO2 because it reduces their ability to detect their predators with consequences for recruitment and settlement processes, population dynamics, and ecosystem (Munday et al., 2010; Cripps et al., 2011; Allan et al., 2013). Therefore, there is growing evidence for disrupted predator-prey interactions induced by elevated pCO2.
The prevalence of the co-occurrence of these two stressors is predicted to increase as hypoxia continues to spread across the coastal ocean and pCO2 increases due to eutrophication and respiration (Mayol et al., 2012; Melzner et al., 2013). Available evidence suggests that synergies between hypoxia and elevated pCO2 affect the physiology, condition, metabolism and life history of invertebrates, such as marine bivalves, sipunculid worms, and shrimps (Reipschläger et al., 1997; Burgents et al., 2005; Pörtner et al., 2005; Kim et al., 2013; Gobler et al., 2014). Moreover, a recent study (DePasquale et al., 2015) showed the negative effects of the combined conditions of hypoxia and elevated pCO2 on hatching and survival of three estuarine fish species Menidia beryllina, Menidia menidia, and Cyprinodon variegatus. Anti-predator behavior in fish has been shown to be influenced by both hypoxia (Kramer, 1987; Poulin et al., 1987; Domenici et al., 2007) and elevated pCO2 (Ferrari et al., 2012b; Allan et al., 2013), but the possible synergistic effect on anti-predator behavior of fish has not been tested to date.
Here we test the predator-avoidance behavior of fish after recovering from a short-term event of hypoxia and elevated pCO2, either in isolation or in combination. Specifically, we predict that hypoxia and elevated pCO2 together should exert synergistic effects disrupting the behavior of juvenile fish to a greater extent than observed under the individual stressors.
Materials and Methods
The experiment was conducted during end of September and beginning of October 2012 at the regional government aquaculture laboratory (LIMIA), Mallorca, Spain. The experiment was performed with naïve 5-month old juvenile European seabass (9.0 ± 0.63 (SD) cm total length, n = 24), reared from wild parents of Mediterranean origin by a commercial aquaculture producer (CULMAREX; Mallorca, Spain). In the aquaculture station, the juvenile production cycle is set at 20°C, oxygen levels over 8 mg L−1 and pH = 7.9 (CULMAREX pers. comm.). Prior to the experiment, individuals were acclimated for 48 h in a 100-liter tank, aerated and with open water circulation. Fish were fed ad libitum twice daily with food pellets (CV4 Skretting pellets). A random subsample of fish (n = 6 per treatment) from this tank was moved to each one of four treatment tanks (25 l) and exposed to one of the four experimental treatments.
The four treatments involved two different levels of CO2 (and pH) and oxygen, as follows: (a) Control—involving pCO2 corresponding to atmospheric equilibrium (380 ppm) and saturated oxygen (6–8 mg L−1); (b) Hypoxia alone- pCO2 corresponding to atmospheric equilibrium (380 ppm) and low oxygen (2.0–3.5 mg L−1, after Vaquer-Sunyer and Duarte, 2008); (c) Acidification alone—a treatment with elevated pCO2, corresponding to atmospheric levels expected by the end of the century (1000 ppm, Orr et al., 2005) and saturated oxygen; and (d) Combined Hypoxia and Acidification together—treatment with low O2 (2.0–3.5 mg L−1) and elevated pCO2 (1000 ppm) (Table 1). The four treatment tanks were filled with 20 μm-filtered water, which was prepared following the protocol explained above, and placed in temperature-controlled chambers at 24°C. Dissolved oxygen (DO) levels between 2.0 and 3.5 mg L−1, were chosen to represent sublethal O2 concentrations (Vaquer-Sunyer and Duarte, 2008). For further details of gas mixing and measurements of water conditions see Supporting Material 1.
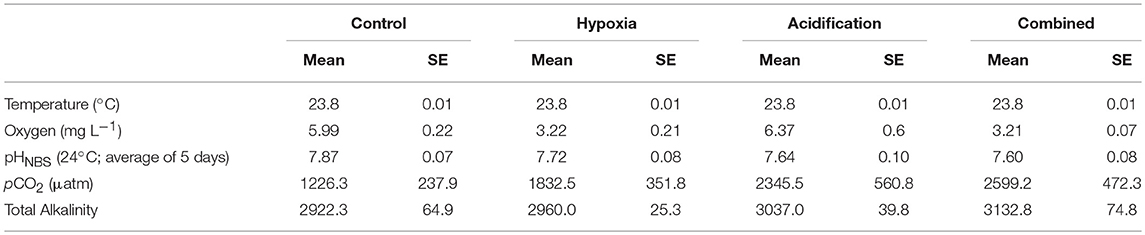
Table 1. Measured conditions (mean ± SE) of the seawater of each treatment: Control (ambient pCO2 and ambient O2), Hypoxia (ambient pCO2 and reduced O2), Acidification (elevated pCO2 and ambient O2) and Combined (reduced O2 and elevated pCO2).
After remaining for short time exposure (4 days) in the treatment tanks, 3 fish per treatment (12 individuals, repeated next day to a total of 24 individuals) were transferred for acclimation into individual rectangular observing arenas (6 L; 25 × 14 × 17 cm) under control conditions, the night (12 h) before the behavioral assay. Individual arenas were visually isolated from each other to avoid disturbance from the other fish and contained a refuge (a piece of artificial seagrass) on one side of the arena. We based our behavioral assay in the replicated-BACI design, which was adapted from (Ferrari et al., 2012a) and followed three observational periods based on video-recording: I) a 5-min recording before-stimulus (simulated predator) period, II) a 1-min stimulus period, and III) a 5-min after-stimulus period. The stimulus consisted of the introduction of a dead individual of the predator species Scorpaena porcus inside a watertight (to avoid odor cues) clear plastic bag filled in with water. The bag was placed at the opposite side of the refuge and contained a thin layer of gravel to ensure it would sink and settle properly on the bottom of the tank. The bag occupied less than 5% of the bottom surface of the arena. To stimulate prey fish activity, 4–5 food pellets were added on the opposite side of the shelter before the three periods. The experiment was monitored through video recording using a video camera (Go Pro Model Hero2, © Woodman Labs, Inc.) located above the experimental arenas. Videos were reduced to one frame per second sequences (300 frames per period, i.e., 600 frames for each fish trial). These frame sequences were obtained and analyzed using ImageJ (Schneider et al., 2012). The position of the fish was followed using a tracking plug-in (MTrack) within the same software (Meijering et al., 2012), which allowed recording the coordinates of the fish for each frame. In addition, the coordinates of the borders of the aquarium, the refuge and the predator were obtained. The order of fish from the different treatments was randomized and the video observer was blind with respect to the CO2 and O2 treatment groups. The behavioral assays were simultaneously performed in three fish per treatment (as in Rosa et al., 2013) distributed randomly in 12 arenas. After the 5 min of the above detailed protocol, the behavioral assay finished and arenas were cleaned and prepared for the next day assay.
Data obtained from each of the 24 fish was composed of a number of coordinates, which were spatially and temporally correlated over time named as discrete-time Markov chain. Therefore, we adopted a function-valued approach where the parameters of a behavioral movement model were compared, rather than comparing direct observations (e.g., distances covered and speeds of fish in the arena) to correct for temporal and spatial autocorrelation (Stinchcombe and Kirkpatrick, 2012). We modeled the movement of the fish as a Biased Random Walk model (BRW), being representative of the risk-taking behavior adopted by a prey-fish in the field. This approach models fish movement within the arena as a random process, but with a tendency (see parameter k below) to remain close to a specific point (the refuge). The tendency to remain close to the refuge is expected to increase in the presence of a predator, and the model tests if the treatments affect their tendency to remain close to the refuge in the presence of those predators. This modeling approach has been widely used to describe the home range behavior of marine coastal fish, where the spatial coverage used by the fish reaches an asymptote because the random movement has an extra pattern that links the fish to a specific point or center of the home range (Börger et al., 2008; Palmer et al., 2009; Alós et al., 2012).
The model describes the trajectory of a given fish in the behavioral arena as
where and denote the position of the center of the activity area at the instant n and n+1, respectively, is the position of the refuge, k min−1 describes the tendency to move to the refuge and describes the stochastic component of the movement process, through a normally distributed term with zero mean and standard deviation (τ) approximated by:
where ε defines the scale of the random walk and k defines the tendency to remain near the refuge. For a given activity area size, k increases with the tendency to move to and remain close to the refuge. As k asymptotically approaches zero, the movement pattern approaches a standard (i.e., unbiased) random walk. When k is negative, fish tend to avoid the shelter. The movement parameter k of the i fish was hierarchically estimated from the two time-series (before and after stimulus) for each treatment where fish were considered a random level using a Bayesian approach. The difference kafter,i. and kbefore,i (or δki) gives an estimate of how much the movement characteristics of the fish i changed between the “before” and “after” it experienced the stimulus. The model was hierarchical in the sense that the data of all fish were analyzed together and all δki of the fish submitted to the same treatment are assumed to be normally distributed around δktreatment. Note that the top level of the model was a crossed two (fixed) factors design (Control, Hypoxia, Acidification, Combination). To provide a measure of significance of a change in the δktreatment we assumed the effect to be relevant when the Bayesian Credibility Intervals (BCI) of the posterior distribution of the δktreatment did not involve zero (pure absence of change in the behavior).
The Bayesian approach has several advantages for deriving inferences in such complex hierarchical models with temporal and spatial autocorrelation (Lunn et al., 2000), being thus suitable to test our hypothesis. Inter-treatment comparisons were conducted by comparing the Bayesian treatment means of each parameter using intervals (2.5 and 97.5%). Unlike the p-value, the Bayesian posterior distributions are interpreted correctly as a belief that there is a given probability that the parameter of interest lies within the interval (Ellison, 2004). The model was implemented and run using the R2jags library (Horne et al., 2007; available at: http://cran.r-project.org/web/packages/R2jags/index.html) of the R package (at http://www.r-project.org/), which uses Just another Gibbs sampler (JAGS http://mcmc-jags.sourceforge.net/) to perform Markov Chain Monte Carlo (MCMC) sampling of probability distribution of models parameters. We assumed that the priors for δki were normally distributed with mean δktreatment and the same tolerance (1/variance) for all four groups of fish. A uniform uninformative flat prior was assumed for this common tolerance. Similarly, uninformative priors were assumed for between-fish variability (within treatment) and for SDi (Equation 2). Finally, normal uninformative priors were assumed for δktreatment. Three MCMC were run using randomly chosen initial values for all the estimated parameters (within reasonable intervals). The chains were iterated until convergence was reached. Then 1,000 valid iterations were obtained for estimating posteriors. Intuitive interpretation of the movement parameters is not straightforward. Therefore, just for graphically interpretation the estimated values of ktreatment and SDi were used to simulate 1,000 consecutive fish positions that were visualized by 2D kernel density plots completed using adehabitatHR package in R (Calenge, 2006).
All procedures were carried out in strict accordance with the recommendations from Directive 2010/175 63/UE, adhering to Spanish law (RD53/ 2013, BOE n. 34 February 8th 2013).
Results
The treatment conditions of temperature and oxygen matched the target concentrations and were held to a stable level. Unfortunately, the pCO2 in the water was higher than target values (380 and 1000 ppm) due to the respiration of the fish and thus the pH lower than intended (Table 1). Temperature was 23.8°C (±0.01 SE) throughout the experiment. Oxygen was held to a value of 5.99 ± 0.022 mg L−1 and 6.37 ± 0.60 mg L−1 for the Control and Acidification treatment, and 3.22 ± 0.21 mg L−1 and 3.21 ± 0.07 mg L−1 for the Hypoxia and Combined treatment, respectively. pHNBS was 7.87 ± 0.07 SE and 7.72 ± 0.08 for the Control and Hypoxia treatment, and 7.64 ± 0.10 and 7.60 ± 0.08 for the Acidification and Combined treatment, respectively. No significant difference in size between treatments was observed (p = 0.89, one-way ANOVA).
There was a significant change (larger k, i.e., greater tendency to swim near the refuge after the presence of the predator) for the fish in the Control (δktreatment = 0.1238 ± 0.0575 min−1) and Hypoxia (δktreatment = 0.2222 ± 0.1019 min−1) treatments (Table 2). Conversely, fish submitted to Acidification (δktreatment = 0.0298 ± 0.0647 min−1) and the Combined treatments (δktreatment = 0.0031 ± 0.0616 min−1) did not change their behavior in the presence of the predator (Figure 1). The absence of a change in trend toward remaining near the refuge in the presence of the predator was evident when the fish were concurrently exposed to elevated pCO2 with or without the combination of reduced O2 values (Figure 2; Table 2).
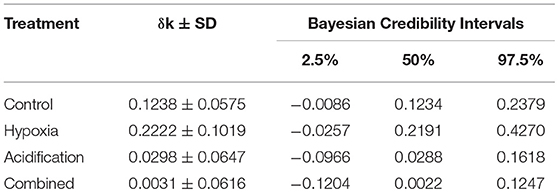
Table 2. Bayesian Confidence Intervals indicating the tendency of the fish to remain close to their refuge after introduction of a predator: a δk > 0 indicates the tendency to move to and remain close to the refuge; a δk around 0 means the movement pattern approaches a (i.e., unbiased) random walk, and a negative δk shows that the fish tend to avoid the shelter.
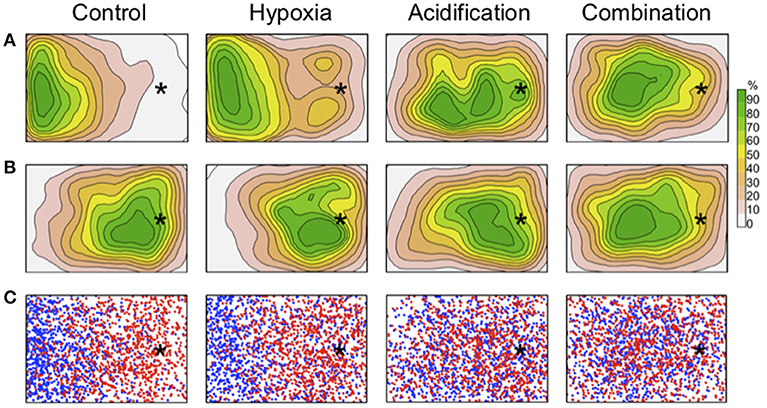
Figure 1. Behavioral response shown as 2-dimensional kernel density plot. The model shows behavioral response to the 4 treatments before (A) and after introduction of the simulated predator (B) Lower graphs (C) show a simulated distribution before (blue) and after (red) the predator was introduced to the arenas (after the model developed by Palmer et al. (2009). The stars mark the position of the shelter. Simulated predator, food, and aeration were positioned at the left side of the arenas (not visible in the square).
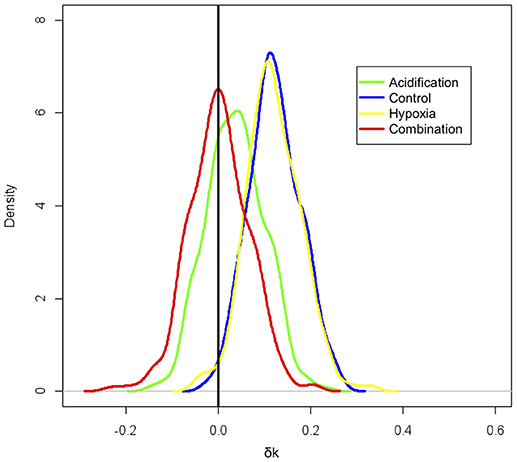
Figure 2. Tendency of the fish to remain close to the refuge: increase in k indicates the tendency to move to and remain close to the refuge; a decrease toward zero means the movement pattern approaches a (i.e., unbiased) random walk, and a negative k shows that the fish tend to avoid the shelter.
As shown in the 2-dimensional kernel density plot (Figure 1), fish in the Control and Hypoxia treatments were attracted by the food (released to the left of each tank, in Figure 1) and left the refuge, but as soon as the stimulus (simulated predator) was added to the experimental arena, fish enhanced their tendency to move around the refuge. Fish that were exposed to elevated pCO2 only and to the combined treatment moved randomly in the middle of the arena and showed no attraction toward the refuge in the absence or in the presence of the virtual predator (Figure 1). Fish in the Acidification treatment showed a slightly stronger, although non-significant, average effect to be attracted by the shelter than fish in the Combined treatment (Figure 2).
Discussion
Whereas hypoxia and elevated pCO2 are concurrent stressors that are increasing in coastal areas, experimental assessments of the responses of organisms have focused on individual stressors, with only a few experiments (e.g., Burgents et al., 2005; Kim et al., 2013; Gobler et al., 2014; DePasquale et al., 2015; Steckbauer et al., 2015; Klein et al., 2017) examining the potential synergy between these concurrent stressors. Although little is known about the concurrent effects of hypoxia and elevated pCO2 on teleost fish, a recent study showed the negative effects of the combined stressors on hatching and survival on the three estuarine fish species Menidia beryllina, Menidia menidia, and Cyprinodon variegates (DePasquale et al., 2015). The present results support previous results (Ferrari et al., 2012b), in that elevated pCO2, even after a time of recovery (and despite our differences in pH were not very high) leads to a disruption of the capacity of the fish to avoid predation risk, and shows that this shift in behavior is, however, not associated with hypoxia. Therefore, the hypothesis of significant synergy between these two stressors was rejected. Nevertheless, as physiological recovery from hypoxia is often a matter of hours (Farrell et al., 1998), the results might have been different had the animals been tested immediately, without the over-night recovery or if tests had been conducted under treatment conditions.
Hypoxia can constrain fish movement, which is energy demanding, affecting the capacity of fish to move toward refuge in the presence of a predator (Kramer, 1987). Although, fish are able to escape hypoxic areas (Tyler and Targett, 2007), previous diel-cycle hypoxia lowers the avoidance threshold from < 2.8 mg O2 L−1 (in saturation-acclimated fish) to ~ 1.4 mg O2 L−1 (in diel-cycling hypoxia acclimated fish) in the juvenile weakfish Cynoscion regalis (Brady and Targett, 2013). Moreover, fish can be trapped when a flooding tide appears, but are able to recover from short-term hypoxia very quickly (Rabalais et al., 2001). After recovering for 45 min the swimming performance and ventilation rate in the wild sockeye salmon (Oncorhynchus nerka) returned to those of the control values (Farrell et al., 1998). However, as shown on the juvenile summer flounder Paralichthys dentatus (Brady and Targett, 2010), diel-cycling hypoxia-acclimated individual did not recover as well from low DO exposure as did saturation-acclimated fish. This indicates that the oxygen value of the water prior to a hypoxic event influences the capability of species to cope with their escaping capabilities and recovery after the event.
Predator-prey interactions play a major role in structuring food webs and are a main driver of behavioral responses, including avoidance responses of fish to predators (Turner and Mittelbach, 1990). In general, prey fish randomly forage in their home range, with a general tendency to remain near refuges, particularly so in the presence of predators (Krause et al., 1998). We examined this behavior using a function-valued approach, where we compared the parameters of the behavioral model rather than inferring statistics on the fish positions per se, avoiding the problems derived from spatial and temporal autocorrelation of the observations on position. Using this approximation, we obtained more accurate information of how the two stressors tested (Hypoxia and Acidification) affect each individuals capacity to hide from predators. The novel approach used here provides a pathway for further studies testing similar hypothesis across taxa under laboratory conditions.
As the results of this study correlated to elevated pCO2 and not hypoxia, the question remains, why there is a bigger impact of that stressor. The elevated pCO2 values are leading to a lower pH in the blood (hypercapnia), and thereby are a substantial threat to some species as elevated pCO2 lowers the pH of animal tissues, affecting its performance (Pörtner et al., 2004). It is known that elevated pCO2 values readily cross biological membranes, enter the blood and intracellular spaces. Active animals with locomotory muscles, such as epipelagic fish, use passive buffering of short-term pH changes in the body associated with elevated pCO2 in ambient waters. They have high activities of anaerobic metabolic enzymes and have high capacity for buffering pH changes (Castellini and Somero, 1981; Seibel et al., 1997), whereas organisms with low buffering capacity will experience greater fluctuations in intracellular pH during hypercapnia. Although shallow-water fish are capable to compensate for acid–base disturbances within several days when exposed to mild hypercapnia (Michaelidis et al., 2007; Ishimatsu et al., 2008), other experiments show that a similar increase in seawater pCO2 lowers the intracellular pH of a sluggish benthic fish by 0.2, whereas in an active epipelagic fish such as tuna, it only causes a 0.02 pH unit drop (Seibel and Walsh, 2003; Fabry et al., 2008). As we did not measure pH or PCO2 in the blood of the test species, it is uncertain how the blood pH was affected by the different treatments and to what extent they suffered from hypercapnia and how far they normalized after recovering overnight.
The lack of predator avoidance under elevated pCO2 is most likely related to interference with neurotransmitter function (Nilsson et al., 2012). Those authors reversed abnormal olfactory preferences and loss of behavioral lateralization by treating the fish with an antagonist of the GABA-A receptor, indicating that elevated pCO2 interferes with neurotransmitter function. Elevated pCO2 decreases the cognitive abilities in juvenile coral reef fish to detect predator odor (Ferrari et al., 2012a). As a consequence of a much-reduced ability to assess predation risk, even when recovering for 12 h, they will have a much lower survival. Elevated pCO2 levels also have a negative impact on the ability of larval clownfish to detect olfactory cues that help them locate reef habitat and suitable settlement sites (Munday et al., 2010). Moreover, elevated pCO2 might also cause a shift in predator behavior as shown for the common coral reef meso-predator, the brown dottyback (Pseudochromis fuscus), by shifting from preference to avoidance of the smell of injured prey (Cripps et al., 2011). Although the different oxygen and CO2 levels might be influencing both, prey and predator, it is yet unclear if the altered behavior of predators is sufficient to fully compensate for the effects of ocean acidification on prey mortality.
Despite a clear interpretation of some of the results can be made, there are some limitations to this study. The total number of fish per treatment, six, was not large, but uncertainty was dealt with optimally through a probabilistic approach. This, however, suggests that small non-significant differences observed could change if sample size was enlarged. Also, it is clear that known physiological explanations match our behavioral results, but both plastic and genetic adaptations may be more important at population level in future scenarios. Furthermore, although the arenas were bubbled with 380 ppm CO2 (Control and Hypoxia) and 1000 ppm CO2 (Acidification and Combined), the calculated pCO2 of the water was higher than expected due to the respiration of the individuals. This however, still enabled the observation of the typical negative effects of elevated pCO2 on behavior, especially as elevated pCO2 in coastal ecosystems nowadays can already be as high or higher than the predictions for the open ocean for the end of the century.
Conclusions
With this study we show that fish recovering from single or combined effects of CO2 and hypoxia only show behavioral alterations due to prior elevated pCO2 history. This effect is evident even after one night of recovery. The lack of effect of hypoxia, combined or not with CO2, should be further tested using shorter recovery times, or during the treatment. The consequences of the present findings must be understood in a frame of slow but needed progress toward the combined effects of changing levels of these stressors in the frame of warmer and more acidic oceans.
Author Contributions
AS, JA, IC, and CD designed the research. AS, JA, and IC executed the research. AS, CD-G, JA, and IC analyzed the data. All authors contributed to writing and improving the manuscript.
Conflict of Interest Statement
The authors declare that the research was conducted in the absence of any commercial or financial relationships that could be construed as a potential conflict of interest.
Acknowledgments
This is a contribution to the projects MedSeA of the FP7 of the EU (contract no. FP7-ENV-2010-265103) and ESTRESX (ref. CTM2012-32603), funded by the Spanish ministry of economy and competitivity. AS was funded by a Ph.D. fellowship from the Government of the Balearic Islands (Department on Education, Culture, and Universities) and the EU (European Social Fund) as well as King Abdullah University of Science and Technology. CD-G was funded by a fellowship from the National Institute for Agricultural and Food Research and Technology (INIA). JA was supported by a JdC post-doc grant funded by the Spanish Ministry of Economy, Industry and Competitiveness (ref. IJCI-2016-27681). IC thanks the funding of CERES H2020 project (BG-02-2015, 678193). We thank I.E. Hendriks and Miquel Palmer for advice, and Culmarex Group for providing European Sea Bass specimens.
Supplementary Material
The Supplementary Material for this article can be found online at: https://www.frontiersin.org/articles/10.3389/fmars.2018.00350/full#supplementary-material
References
Allan, B. J. M., Domenici, P., Mccormick, M. I., Watson, S., and Munday, P. L. (2013). Elevated CO2 affects predator-prey interactions through altered performance. PLoS ONE 8:e58520. doi: 10.1371/journal.pone.0058520
Alós, J., Palmer, M., and Arlinghaus, R. (2012). Consistent selection towards low activity phenotypes when catchability depends on encounters among human predators and fish. PLoS ONE 7:e48030. doi: 10.1371/journal.pone.0048030
Benito, J., Benejam, L., Zamora, L., and García-Berthou, E. (2015). Diel cycle and effects of water flow on activity and use of depth by common carp. Trans. Am. Fish. Soc. 144, 491–501. doi: 10.1080/00028487.2015.1017656
Börger, L., Dalziel, B. D., and Fryxell, J. M. (2008). Are there general mechanisms of animal home range behaviour? A review and prospects for future research. Ecol. lett. 11, 637–650. doi: 10.1111/j.1461-0248.2008.01182.x
Brady, D., and Targett, T. (2013). Movement of juvenile weakfish Cynoscion regalis and spot Leiostomus xanthurus in relation to diel-cycling hypoxia in an estuarine tidal tributary. Mar. Ecol. Prog. Ser. 491, 199–219. doi: 10.3354/meps10466
Brady, D. C., and Targett, T. E. (2010). Characterizing the escape response of juvenile summer flounder Paralichthys dentatus to diel-cycling hypoxia. J. Fish Biol. 77, 137–152. doi: 10.1111/j.1095-8649.2010.02663.x
Breitburg, D. L. (1990). Near-shore hypoxia in the chesapeake bay - patterns and relationships among physical factors. Estuar. Coast. Shelf Sci. 30, 593–609. doi: 10.1016/0272-7714(90)90095-9
Brewer, P. G., and Peltzer, E. T. (2009). Limits to marine life. Science 324, 347–348. doi: 10.1126/science.1170756
Burgents, J. E., Burnett, K. G., and Burnett, L. E. (2005). Effects of hypoxia and hypercapnic hypoxia on the localization and the elimination of Vibrio campbellii in Litopenaeus vannamei, the Pacific white shrimp. Biol. Bull. 208, 159–168. doi: 10.2307/3593148
Cai, W. J., Hu, X., Huang, W. J., Murrell, M. C., Lehrter, J. C., Lohrenz, S. E., et al. (2011). Acidification of subsurface coastal waters enhanced by eutrophication. Nat. Geosci. 4, 766–770. doi: 10.1038/ngeo1297
Calenge, C. (2006). The package “adehabitat” for the R software: a tool for the analysis of space and habitat use by animals. Ecol. Modell. 197, 516–519. doi: 10.1016/j.ecolmodel.2006.03.017
Castellini, M., and Somero, G. (1981). Buffering capacity of vertebrate muscle: correlations with potentials for anaerobic function. J. Comp. Physiol. 143, 191–198.
Chabot, D., and Claireaux, G. (2008). Environmental hypoxia as a metabolic constraint on fish: the case of Atlantic cod, Gadus morhua. Mar. Pollut. Bull. 57, 287–294. doi: 10.1016/j.marpolbul.2008.04.001
Conley, D. J., Carstensen, J., Vaquer-Sunyer, R., and Duarte, C. M. (2009). Ecosystem thresholds with hypoxia. Hydrobiologia 629, 21–29. doi: 10.1007/s10750-009-9764-2
Craig, J. K., Crowder, L. B., Gray, C. D., McDaniel, C. J., Kenwood, T. A., and Hanifen, J. G. (2001). “Ecological effects of hypoxia on fish, sea turtles, and marine mammals in the northwestern Gulf of Mexico,” in Coastal Hypoxia: Consequences for Living Resources: Ecosystems, eds N. N. Rabalais and R. E. Turner (Washington, DC: American Geophysical Union), 269–291.
Cripps, I. L., Munday, P. L., and McCormick, M. I. (2011). Ocean acidification affects prey detection by a predatory reef fish. PLoS ONE 6:e22736. doi: 10.1371/journal.pone.0022736
DePasquale, E., Baumann, H., and Gobler, C. (2015). Vulnerability of early life stage Northwest Atlantic forage fish to ocean acidification and low oxygen. Mar. Ecol. Prog. Ser. 523, 145–156. doi: 10.3354/meps11142
Diaz, R. J., and Rosenberg, R. (2008). Spreading dead zones and consequences for marine ecosystems. Science 321, 926–929. doi: 10.1126/science.1156401
Dixson, D. L., Munday, P. L., and Jones, G. P. (2010). Ocean acidification disrupts the innate ability of fish to detect predator olfactory cues. Ecol. Lett. 13, 68–75. doi: 10.1111/j.1461-0248.2009.01400.x
Domenici, P., Lefrançois, C., and Shingles, A. (2007). Hypoxia and the antipredator behaviours of fishes. Philos. Transac. R. Soc. Lond. Ser. B Biol. Sci. 362, 2105–2121. doi: 10.1098/rstb.2007.2103
Doney, S. C., Fabry, V. J., Feely, R. A., and Kleypas, J. A. (2009). Ocean acidification: the other CO2 problem. Ann. Rev. Mar. Sci. 1, 169–192. doi: 10.1146/annurev.marine.010908.163834
Duarte, C. M., Hendriks, I. E., Moore, T. S., Olsen, Y. S., Steckbauer, A., Ramajo, L., et al. (2013). Is ocean acidification an open-ocean syndrome? understanding anthropogenic impacts on seawater pH. Estuar. Coast. 36, 221–236. doi: 10.1007/s12237-013-9594-3
Ellison, A. M. (2004). Bayesian inference in ecology. Ecol. Lett. 7, 509–520. doi: 10.1111/j.1461-0248.2004.00603.x
Fabry, V. J., Seibel, B. A., Feely, R. A., and Orr, J. C. (2008). Impacts of ocean acidification on marine fauna and ecosystem processes. J. Mar. Sci. 65, 414–432. doi: 10.1093/icesjms/fsn048
Farrell, A. P., Gamperl, A. K., and Birtwell, I. K. (1998). Prolonged swimming, recovery and repeat swimming performance of mature sockeye salmon Oncorhynchus nerka exposed to moderate hypoxia and pentachlorophenol. J. Exp. Biol. 201, 2183–2193.
Ferrari, M. C. O., Manassa, R. P., Dixson, D. L., Munday, P. L., McCormick, M. I., Meekan, M. G., et al. (2012a). Effects of ocean acidification on learning in coral reef fishes. PLoS ONE 7:e31478. doi: 10.1371/journal.pone.0031478
Ferrari, M. C. O., McCormick, M. I., Munday, P. L., Meekan, M. G., Dixson, D. L., Lönnstedt, O., et al. (2012b). Effects of ocean acidification on visual risk assessment in coral reef fishes. Funct. Ecol. 26, 553–558. doi: 10.1111/j.1365-2435.2011.01951.x
Gilbert, D., Rabalais, N. N., Diaz, R. J., Zhang, J., Version, P., Discussion, I., et al. (2009). Evidence for greater oxygen decline rates in the coastal ocean than in the open ocean. Biogeosci. Discuss. 6, 9127–9160. doi: 10.5194/bgd-6-9127-2009
Gobler, C. J., DePasquale, E. L., Griffith, A. W., and Baumann, H. (2014). Hypoxia and acidification have additive and synergistic negative effects on the growth, survival, and metamorphosis of early life stage bivalves. PLoS ONE 9:e83648. doi: 10.1371/journal.pone.0083648
Hendriks, I. E., Duarte, C. M., and Álvarez, M. (2010). Vulnerability of marine biodiversity to ocean acidification: a meta-analysis. Estuar. Coast. Shelf Sci. 86, 157–164. doi: 10.1016/j.ecss.2009.11.022
Hendriks, I. E., Olsen, Y. S., Ramajo, L., Basso, L., Steckbauer, A., Moore, T. S., et al. (2014). Photosynthetic activity buffers ocean acidification in seagrass meadows. Biogeosciences 11, 333–346. doi: 10.5194/bg-11-333-2014
Horne, J., Garton, E., Krone, S., and Lewis, J. (2007). Analyzing animal movements using Brownian bridges. Ecology 88, 2354–2363. doi: 10.1890/06-0957.1
Ishimatsu, A., Hayashi, M., and Kikkawa, T. (2008). Fishes in high-CO2, acidified oceans. Mar. Ecol. Prog. Ser. 373, 295–302. doi: 10.3354/meps07823
Kim, T. W., Barry, J. P., and Micheli, F. (2013). The effects of intermittent exposure to low pH and oxygen conditions on survival and growth of juvenile red abalone. Biogeosciences 10, 7255–7262. doi: 10.5194/bg-10-7255-2013
Klein, S. G., Pitt, K. A., Nitschke, M. R., Goyen, S., Welsh, D. T., Suggett, D. J., et al. (2017). Symbiodinium mitigate the combined effects of hypoxia and acidification on a non-calcifying cnidarian. Glob. Chang. Biol. 23, 3690–3703. doi: 10.1111/gcb.13718
Kramer, D. L. (1987). Dissolved oxygen and fish behavior. Environ. Biol. Fishes 18, 81–92. doi: 10.1007/BF00002597
Krause, J., Loader, S. P., McDermott, J., and Ruxton, G. D. (1998). Refuge use by fish as a function of body length-related metabolic expenditure and predation risks. Proc. R. Soc. Lond. B 265, 2373–2379. doi: 10.1098/rspb.1998.0586
Kroeker, K. J., Kordas, R. L., Crim, R., Hendriks, I. E., Ramajo, L., Singh, G. S., et al. (2013). Impacts of ocean acidification on marine organisms: quantifying sensitivities and interaction with warming. Glob. Chang. Biol. 19, 1884–1896. doi: 10.1111/gcb.12179
Levin, L. A., Ekau, W., Gooday, A. J., Jorissen, F., Middelburg, J. J., Naqvi, S. W. A., et al. (2009). Effects of natural and human-induced hypoxia on coastal benthos. Biogeosciences 6, 2063–2098. doi: 10.5194/bg-6-2063-2009
Lunn, D. J., Thomas, A., Best, N., and Spiegelhalter, D. (2000). WinBUGS–a Bayesian modelling framework: concepts, structure, and extensibility. Stat. Comput. 10, 325–337. doi: 10.1023/A:1008929526011
Mayol, E., Ruiz-Halpern, S., Duarte, C. M., Castilla, J. C., and Pelegri, J. L. (2012). Coupled CO2 and O2-driven compromises to marine life in summer along the Chilean sector of the Humboldt current system. Biogeosciences 9, 1183–1194. doi: 10.5194/bg-9-1183-2012
Meijering, E., Dzyubachyk, O., and Smal, I. (2012). Methods for cell and particle tracking. Meth. Enzymol. 504, 183–200. doi: 10.1016/B978-0-12-391857-4.00009-4
Melzner, F., Thomsen, J., Koeve, W., Oschlies, A., Gutowska, M. A., Bange, H. W., et al. (2013). Future ocean acidification will be amplified by hypoxia in coastal habitats. Mar. Biol. 160, 1875–1888. doi: 10.1007/s00227-012-1954-1
Michaelidis, B., Spring, A., and Pörtner, H. O. (2007). Effects of long-term acclimation to environmental hypercapnia on extracellular acid–base status and metabolic capacity in Mediterranean fish Sparus aurata. Mar. Biol. 150, 1417–1429. doi: 10.1007/s00227-006-0436-8
Mistri, M. (2004). Effects of hypoxia on predator-prey interactions between juvenile Carcinus aestuarii and Musculista senhousia. MEPS 275, 211–217. doi: 10.3354/meps275211
Munday, P. L., Dixson, D. L., Mccormick, M. I., Meekan, M., Ferrari, M. C. O., and Chivers, D. P. (2010). Replenishment of fish populations is threatened by ocean acidification. Proc. Natl. Acad. Sci. U.S.A. 107, 12930–12934. doi: 10.1073/pnas.1004519107
Nilsson, G. E., Dixson, D. L., Domenici, P., Mccormick, M. I., Sørensen, C., Watson, S., et al. (2012). Near-future carbon dioxide levels alter fish behaviour by interfering with neurotransmitter function. Nat. Climate Change 2, 201–204. doi: 10.1038/nclimate1352
Orr, J. C., Fabry, V. J., Aumont, O., Bopp, L., Doney, S. C., Feely, R. A., et al. (2005). Anthropogenic ocean acidification over the twenty-first century and its impact on calcifying organisms. Nature 437, 681–686. doi: 10.1038/nature04095
Palmer, H., Beutel, M., and Gebremariam, S. (2009). High rates of ammonia removal in experimental oxygen-activated nitrification wetland mesocosms. J. Environ. Eng. ASCE 135, 972–979. doi: 10.1061/(ASCE)EE.1943-7870.0000053
Pörtner, H. O., Langenbuch, M., and Michaelidis, B. (2005). Synergistic effects of temperature extremes, hypoxia, and increases in CO2 on marine animals: from Earth history to global change. J. Geophys. Res. 110:C09S10. doi: 10.1029/2004JC002561
Pörtner, H. O., Langenbuch, M., and Reipschläger, A. (2004). Biological impact of elevated ocean CO2 concentrations : lessons from animal physiology and earth history. J. Oceanogr. 60, 705–718. doi: 10.1007/s10872-004-5763-0
Poulin, R., Wolp, N. G., and Kramer, D. L. (1987). The effect of hypoxia on the vulnerability of guppies (Poecilia reticulata, Poeciliidae) to an aquatic predator (Astronotus ocektus, Cichlidae). Environ. Biol. Fishes 20, 285–292.
Rabalais, N. N., Diaz, R. J., Levin, L. A., Turner, R. E., Gilbert, D., and Zhang, J. (2010). Dynamics and distribution of natural and human-caused hypoxia. Biogeosciences 7, 585–619. doi: 10.5194/bg-7-585-2010
Rabalais, N. N., Turner, R. E., and Wiseman, W. J. J. (2001). Hypoxia in the Gulf of Mexico. JEQ 30, 320–329. doi: 10.2134/jeq2001.302320x
Reipschläger, A., Nilsson, G. E., and Pörtner, H. O. (1997). A role for adenosine in metabolic depressionin themarine invertebrate Sipunculus nudus. Am. J. Physiol. 272, R350–R356.
Riedel, B., Stachowitsch, M., and Zuschin, M. (2007). Sea anemones and brittle stars: unexpected predatory interactions during induced in situ oxygen crises. Mar. Biol. 153, 1075–1085. doi: 10.1007/s00227-007-0880-0
Rosa, R., Trübenbach, K., Repolho, T., Pimentel, M., Faleiro, F., Boavida-Portugal, J., et al. (2013). Lower hypoxia thresholds of cuttlefish early life stages living in a warm acidified ocean. Proc. R. Soc. B. 280:20131695. doi: 10.1098/rspb.2013.1695
Schneider, C. A., Rasband, W. S., and Eliceiri, Z. (2012). NIH Image to ImageJ: 25 years of image analysis. Nat. Methods 9, 671–675. doi: 10.1038/nmeth.2089
Seibel, A., Thuesen, E. V., Childress, J. J., and Gorodezky, L. A. (1997). Decline in pelagic Cephalopod metabolism habitat depth reflects differences in locomotory efficiency. Biol. Bull. 192, 262–278. doi: 10.2307/1542720
Seibel, B. A., and Walsh, P. J. (2003). Biological impacts of deep-sea carbon dioxide injection inferred from indices of physiological performance. J. Exp. Biol. 206, 641–650. doi: 10.1242/jeb.00141
Stachowitsch, M., and Avcin, A. (1988). Eutrophication-Induced Modifications of Benthic Communities. Eutrophication in the Mediterranean Sea: Receiving Capacity and Monitoring of Long-Term Effects. Regione Emilia Romagna and University of Bologna, Bologna. 49, 67–80.
Steckbauer, A., Duarte, C. M., Carstensen, J., Vaquer-Sunyer, R., and Conley, D. J. (2011). Ecosystem impacts of hypoxia: thresholds of hypoxia and pathways to recovery. Environ. Res. Lett. 6. doi: 10.1088/1748-9326/6/2/025003
Steckbauer, A., Ramajo, L., Hendriks, I. E., Fernandez, M., Lagos, N. A., Prado, L., et al. (2015). Synergistic effects of hypoxia and increasing CO2 on benthic invertebrates of the central Chilean coast. Front. Mar. Sci. 2:49. doi: 10.3389/fmars.2015.00049
Stinchcombe, J. R., and Kirkpatrick, M. (2012). Genetics and evolution of function-valued traits: understanding environmentally responsive phenotypes. Trends Ecol. Evol. 27, 637–647. doi: 10.1016/j.tree.2012.07.002
Taylor, D. L., and Eggleston, D. B. (2000). Effects of hypoxia on an estuarine predator-prey interaction: foraging behavior and mutual interference in the blue crab Callinectes sapidus and the infaunal clam prey Mya arenaria. Mar. Ecol. Prog. Ser. 196, 221–237. doi: 10.3354/meps196221
Turner, A. M., and Mittelbach, G. G. (1990). Predator avoidance and community structure: interactions among piscivores, planktivores, and plankton. Ecology 71, 2214–2254. doi: 10.2307/1938636
Tyler, R. M., and Targett, T. E. (2007). Juvenile weakfish Cynoscion regalis distribution in relation to diel-cycling dissolved oxygen in an estuarine tributary. Mar. Ecol. Prog. Ser. 333, 257–269. doi: 10.3354/meps333257
Vaquer-Sunyer, R., and Duarte, C. M. (2008). Thresholds of hypoxia for marine biodiversity. Proc. Natl. Acad. Sci. U.S.A. 105, 15452–15457. doi: 10.1073/pnas.0803833105
Vaquer-Sunyer, R., Duarte, C. M., Jordà, G., and Ruiz-Halpern, S. (2012). Temperature dependence of oxygen dynamics and community metabolism in a shallow mediterranean macroalgal meadow (Caulerpa prolifera). Estuar. Coast. 35, 1182–1192. doi: 10.1007/s12237-012-9514-y
Keywords: recovery, behavior, hypoxia, elevated pCO2, acidification, biased random walk, predator-prey interactions
Citation: Steckbauer A, Díaz-Gil C, Alós J, Catalán IA and Duarte CM (2018) Predator Avoidance in the European Seabass After Recovery From Short-Term Hypoxia and Different CO2 Conditions. Front. Mar. Sci. 5:350. doi: 10.3389/fmars.2018.00350
Received: 29 March 2018; Accepted: 11 September 2018;
Published: 09 October 2018.
Edited by:
Aurélien Paulmier, UMR5566 Laboratoire d'études en Géophysique et Océanographie Spatiales (LEGOS), FranceReviewed by:
Shiguo Li, Research Center for Eco-Environmental Sciences (CAS), ChinaYuanyuan Feng, Tianjin University of Science and Technology, China
Copyright © 2018 Steckbauer, Díaz-Gil, Alós, Catalán and Duarte. This is an open-access article distributed under the terms of the Creative Commons Attribution License (CC BY). The use, distribution or reproduction in other forums is permitted, provided the original author(s) and the copyright owner(s) are credited and that the original publication in this journal is cited, in accordance with accepted academic practice. No use, distribution or reproduction is permitted which does not comply with these terms.
*Correspondence: Alexandra Steckbauer, YWxleGFuZHJhLnN0ZWNrYmF1ZXJAa2F1c3QuZWR1LnNh; c3RlY2tiYXVlci5vY2VhbkBnbWFpbC5jb20=