- 1Department of Marine Sciences, Skidaway Institute of Oceanography, University of Georgia, Savannah, GA, United States
- 2Georgetown College, Georgetown, KY, United States
- 3Proteomics and Mass Spectrometry Core Facility, University of Georgia, Athens, GA, United States
- 4School of Earth and Atmospheric Sciences, Georgia Institute of Technology, Atlanta, GA, United States
- 5Division of Biology and Paleo Environment, Lamont-Doherty Earth Observatory, Palisades, NY, United States
The nutritionally available pool of dissolved organic phosphorus (DOP) supports marine primary productivity in a range of ocean ecosystems but remains poorly resolved. Here, the relative lability of model phosphorus (P) compounds representing the major P(V) bond classes of marine DOP – phosphomonoesters (P-O-C) and phosphoanhydrides (P-O-P) – was assessed in diatom cultures of the genus Thalassiosira, as well as coastal field sites of the western North Atlantic. In diatom samples, maximum enzymatic hydrolysis rates revealed that the P-anhydride bonds of inorganic tripolyphosphate (3poly-P), followed by the P-anhydride bonds of adenosine 5′-triphosphate (ATP), were preferentially degraded relative to the P-monoesters adenosine 5′-monophosphate (AMP) and 4-methylumbelliferone phosphate (MUF-P). Consistent with these rate measurements, targeted proteomics analysis demonstrated that the underlying phosphatase diversity present in diatom samples was dominated by P-anhydride degrading enzymes (inorganic pyrophosphatases and nucleoside triphosphatases). Furthermore, biomass-normalized rates of ATP degradation were always suppressed under P-replete conditions in diatom cultures, but the effect of overall P availability on 3poly-P degradation was inconsistent among diatom strains, suggesting that inorganic polyphosphate (poly-P) degradation may persist irrespective of prevailing P levels in the marine environment. Indeed, the majority of field sites examined in the P-replete coastal western North Atlantic exhibited significantly higher maximum rates of inorganic poly-P hydrolysis relative to P-monoester hydrolysis, which was largely driven by phytoplankton dynamics. Based on these results, the possibility that P-anhydride utilization may contribute comparably or even more substantially than P-esters to community-level P demand, phytoplankton growth, and primary productivity should be considered.
Introduction
Marine primary productivity is fundamentally constrained by the availability of nutrients such as phosphorus (P). P is incorporated into vital biomolecules that play essential roles in cellular structure (phospholipids), the storage and transmission of genetic information (DNA and RNA), energy transduction (adenosine 5′-triphosphate; ATP), metabolic signaling (inositol trisphosphate, (de)phosphorylated proteins and metabolites), as well as stress response and homeostasis (inorganic polyphosphate; poly-P) (Kornberg et al., 1999; Gray and Jakob, 2015). Although marine primary production is principally limited by either nitrogen or iron over most of the global surface ocean (Moore et al., 2013), P can be present at low or limiting concentrations in many marine systems, from the vast oligotrophic gyres (Rivkin and Swift, 1979; Wu et al., 2000; Moore et al., 2008; Moore et al., 2013) to coastal seas (Berland et al., 1972; Fahmy, 2003; Thingstad et al., 2005; Hardison et al., 2013). Rising anthropogenic inputs of nitrogen have also led to an increasing prevalence of P limitation in many ocean environments, especially in near-shore zones (Harrison et al., 1990; Fisher et al., 1992, 1999; Huang et al., 2003; Kemp et al., 2005; Zhang et al., 2007; Xu et al., 2008; Fu et al., 2012; Laurent et al., 2012; Turner and Rabalais, 2013; Kim et al., 2014). Yet even when P is not limiting, it can be sufficiently scarce to shape microbial physiology and community composition (Moore et al., 2008).
Marine plankton communities can access a variety of P sources to satisfy nutritional P demands. Orthophosphate is considered the most biologically preferred P source, but in the open ocean where orthophosphate is scarce, i.e., <1–10% of total dissolved P (Wu et al., 2000; Lomas et al., 2010), the recycling of dissolved organic P (DOP) supports approximately 90% of gross primary productivity (Karl, 2014). Even in coastal systems that are replete with orthophosphate, a highly labile fraction of the DOP pool is rapidly recycled to support primary production (Benitez-Nelson and Buesseler, 1999). The cycling and biological utilization of DOP is therefore fundamentally tied to the productivity and function of marine ecosystems on a global scale (Letscher and Moore, 2015) across extremes in trophic status.
Natural marine DOP is an operationally defined assemblage of organic, inorganic, and polymeric P-containing molecules. Despite a high degree of heterogeneity at the molecular level, marine DOP contains consistent proportions of three major P-bond classes: P-esters, P-anhydrides, and phosphonates (Young and Ingall, 2010). P-esters contain P in its most common + V oxidation state, typically in the form of the P-O-C (monoester) or C-O-P-O-C (diester) bonds. Representative P-monoesters include nucleotides and phosphosugars, while P-diesters include phospholipids and nucleic acids. Overall, P-esters are the most abundant DOP compound class, representing ∼80% of total marine DOP (Young and Ingall, 2010). Another P(V) bond class is the P-anhydrides, which are characterized by P-O-P bonds like those present in nucleoside di- and triphosphates, inorganic pyrophosphate, and poly-P. P-anhydrides account for ∼10% of total marine DOP and are the most recently recognized DOP bond class, based on advances in techniques to recover the low molecular weight fraction of dissolved organic matter (Diaz et al., 2008; Young and Ingall, 2010). Finally, phosphonates make up ∼10% of DOP (Young and Ingall, 2010). In phosphonates, P is present in its + III oxidation state bound directly to carbon. The identities of natural marine phosphonates are not fully known but may include phosphonolipids and low molecular weight metabolites (Repeta et al., 2016).
The composition of DOP helps determine its bioavailability. For example, while phosphonate utilization may be restricted to certain prokaryotic taxa (Dyhrman et al., 2006; Ilikchyan et al., 2009; Beversdorf et al., 2010; Martinez et al., 2010), P-esters are generally recognized as the major nutritional source of DOP in the ocean (Lin et al., 2016). The widespread importance of P-esters is based on the quantitative dominance of this pool (Young and Ingall, 2010), as well as the environmental prevalence of alkaline phosphatase (AP) (Luo et al., 2009, 2011; Sebastian and Ammerman, 2009). APs are well-known P-esterases, which are active throughout the ocean (Hoppe and Ullrich, 1999; Hoppe, 2003; Mahaffey et al., 2014) and play a vital role in phytoplankton P nutritional physiology, especially in P-deplete environments (Dyhrman and Ruttenberg, 2006; Lin et al., 2016). P-anhydrides may also be a critical, yet under-characterized source of P. For instance, nucleoside triphosphates such as ATP are highly labile P sources (Moore et al., 2005; Alonso-Saez and Gasol, 2007; Michelou et al., 2011; Mazard et al., 2012; Duhamel et al., 2014) in which two-thirds of the available P is contained in P-anhydride bonds. At Station ALOHA in the oligotrophic North Pacific Subtropical Gyre (NPSG), the turnover of dissolved ATP exceeds that of the bulk DOP pool, indicating that it may be preferentially utilized over other DOP sources (Björkman and Karl, 2005). Similarly, depth profiles of inorganic poly-P in the Sargasso Sea suggest that this P-anhydride is preferentially utilized over other forms of P (Martin et al., 2014). Indeed, inorganic poly-P is bioavailable to a diversity of microorganisms, including Prochlorococcus, Synechococcus (Moore et al., 2005), eukaryotic marine phytoplankton (Diaz et al., 2016), and natural phytoplankton communities (Björkman and Karl, 1994).
Despite the widespread importance of DOP and the strong compositional control over its utilization, the relative contribution of specific DOP pools to microbial P demand and primary productivity remains unclear at the community level. Therefore, an improved understanding of compound-specific DOP dynamics is necessary to advance a mechanistic view of overall marine ecosystem productivity and functioning. In particular, a growing appreciation of inorganic poly-P cycling (Diaz et al., 2008; Martin et al., 2014; Saad et al., 2016) brings into question the importance of this P-anhydride source relative to more traditionally recognized P-esters. Therefore, in this study, the relative lability of model P-esters and P-anhydrides was assessed in representative diatom cultures of the genus Thalassiosira, as well as natural samples from the coastal western North Atlantic. To identify underlying mechanisms involved in the compound-specific utilization of DOP, targeted proteomic analysis of diatom culture samples was also conducted.
Materials and Methods
Model Diatoms, Growth Conditions, and Culture Sampling
Thalassiosira oceanica CCMP1005, Thalassiosira pseudonana CCMP1335, and Thalassiosira pseudonana CCMP1014 were obtained from the National Center for Marine Algae and Microbiota (NCMA), Bigelow Laboratories, East Boothbay, Maine. Diatoms were grown in batch cultures on autoclaved (121°C, 20 min) f/2 media (Guillard and Ryther, 1962) prepared using filtered (0.2 μm) natural seawater from the South Atlantic Bight. To examine the effect of overall P availability on the degradation of model DOP sources, diatoms were grown under P-replete (+P) and P-deplete (-P) conditions. In +P media, phosphate was provided at an initial concentration of 36 μM. In -P cultures, no phosphorus source was added. Molar N:P ratios were ∼24 and >882 in +P and -P media, respectively. T. oceanica and T. pseudonana were cultivated at 23°C and 18°C, respectively, on a 14 h:10 h light:dark cycle (340 μmol photons m-2 s-1). Phytoplankton growth was monitored daily by measuring in vivo chlorophyll fluorescence with an AquaFluor handheld fluorometer (Turner Designs). Cell counts were conducted using a hemocytometer counting chamber (Karlson et al., 2010).
P hydrolysis rates from a variety of model DOP substrates (see below) were investigated in diatom cultures with two types of experiments. In the first experiment type, the degradation of P sources was monitored in +P and -P cultures of each diatom strain by subsampling the cultures every 3 days across 30 days of cultivation. On a given sampling day, each subsample (6 mL) was directly filtered (0.2 μm, 33 mm) to produce the cell-free filtrate, which was then incubated with a variety of P sources for up to 24 h to determine substrate-specific P hydrolysis rates for that day, as detailed below. In the second type of P hydrolysis experiment, +P cultures of each diatom strain were sampled after 30 days of cultivation, and P hydrolysis rates for each substrate were determined, as detailed below, in whole cultures and cell-free filtrates.
To generate samples for exoproteome analysis, ∼140 mL of cell-free filtrate was sampled from +P cultures after 30 days of growth. These filtrate samples were concentrated and exchanged twice into 20 mM Tris (pH = 8.0) using a 10 kDa Centricon® Plus-70 centrifugal filter device (Millipore), to a final volume 0.5 mL.
Field Sampling
Surface seawater (5–35 m) was collected in September 2016 during two sampling campaigns in the coastal western North Atlantic (Supplementary Table S1). Three sites were sampled aboard the R/V Endeavor using a Niskin rosette sampler and incubated immediately in order to determine rates of P hydrolysis. Two sites accessible by small boat in Woods Hole Harbor and Buzzard’s Bay, MA, were sampled utilizing a peristaltic pump. These samples were transported on ice packs and analyzed for P hydrolysis rates within 5–6 h of collection. Additional samples were preserved and analyzed for chlorophyll, bacteria and phytoplankton abundance, and soluble reactive P (SRP), as detailed below.
Chlorophyll
In the dark, 250 mL of seawater was filtered onto 25 mm GF/F filters. Samples were stored in the dark at -20°C until analyzed according to protocols adapted from Strickland and Parsons (1972). Briefly, samples were extracted in 90% acetone in the dark (4°C, 9 h) and measured using a 10AU fluorometer (Turner). Sample signals were calibrated using a chlorophyll-a standard (Sigma) and were corrected for pheopigments by accounting for the fluorescence of extracts before and after acidification to 0.003 M HCl.
Abundance of Bacteria and Phytoplankton
Seawater samples were preserved for flow cytometry with 0.5% glutaraldehyde (final concentration), flash frozen in liquid nitrogen and stored at -80°C until analysis. Bacteria and group-specific phytoplankton counts were conducted on a Guava EasyCyte HT flow cytometer (Millipore). Instrument-specific beads were used to calibrate the cytometer. Samples were analyzed at a low flow rate (0.24 μL s-1) for 3 min. To enumerate bacteria, samples were diluted (1:100) with filtered seawater (0.01 μm). Samples and filtered seawater blanks were stained with SYBR Green I (Invitrogen) according to the manufacturer’s instructions and incubated in a 96-well plate in the dark at room temperature for 1 h. Bacterial cells were counted based on diagnostic forward scatter vs. green fluorescence signals. Major phytoplankton groups were distinguished based on plots of forward scatter vs. orange fluorescence (phycoerythrin-containing Synechococcus sp.), and forward scatter vs. red fluorescence (eukaryotes). Size classes of eukaryotic phytoplankton were further distinguished based on forward scatter (pico-, nano- and large eukaryotes).
Soluble Reactive P
Seawater samples were collected into acid cleaned, high density polyethylene bottles. Samples used for determining in situ SRP concentrations were frozen and stored upright at -20°C until analysis. Field samples and diatom filtrates were both analyzed for SRP using a standard colorimetric method (Hansen and Koroleff, 1999). To determine in situ SRP concentrations in field samples, SRP analysis was conducted using a 4 cm glass spectrophotometry cell on triplicate subsamples, and the detection limit, defined as three times the standard deviation of replicate blank measurements, was 115 nmol L-1 SRP. For incubations to determine P hydrolysis rates (see below), replicate samples were analyzed in clear 96-well plates on a multimode plate reader (Molecular Devices) with a detection limit of 800 nmol L-1 P.
P-hydrolysis of Model DOP Substrates
Field and culture samples were incubated with a variety of model DOP substrates to determine P hydrolysis rates. Model P substrates were selected to represent the major P(V) bond classes present in natural marine DOP (Figure 1). The fluorogenic probe 4-methylumbeliferone phosphate (MUF-P) and the nucleotide adenosine 5′-monophosphate (AMP) were utilized as model P-esters. ATP was used as both a representative P-ester and an organic P-anhydride. Finally, two inorganic polyphosphate compounds with an average chain length of 3 or 45 P atoms (3poly-P and 45poly-P, respectively), were utilized as representative inorganic P-anhydrides. All P compounds were obtained from Millipore Sigma.
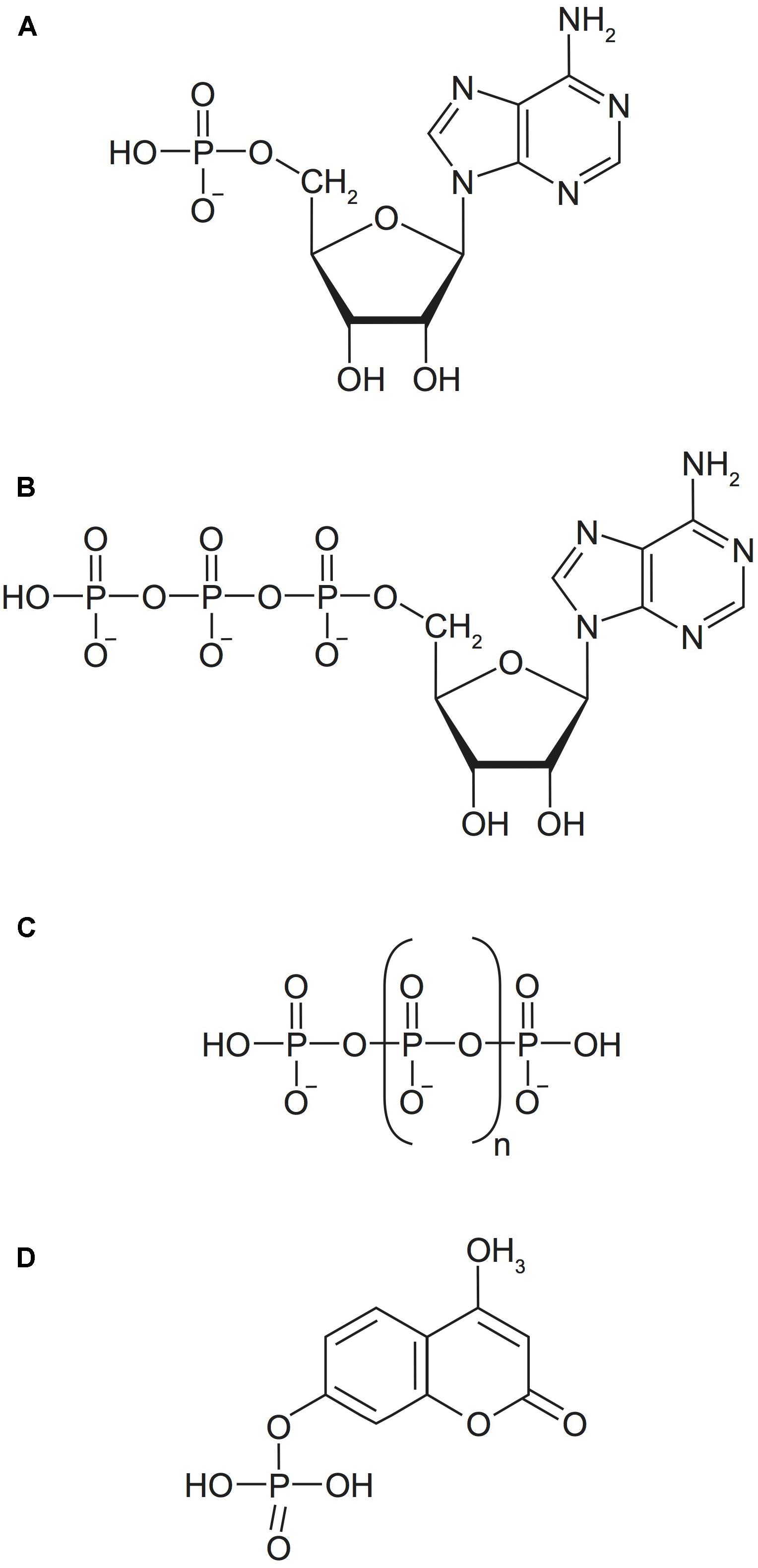
FIGURE 1. Model P substrates utilized in this study represent the major P(V) bond classes of natural marine DOP. (A) Adenosine monophosphate (AMP), a P-monoester. (B) Adenosine triphosphate (ATP), a P-monoester and P-anhydride. (C) Inorganic polyphosphate (poly-P), a P-anhydride, n ≥ 1. (D) 4-methylumbelliferyl phosphate (MUF-P), a fluorogenically labeled P-monoester.
P hydrolysis rates were determined in whole cultures (MUF-P only), cell-free culture filtrates (MUF-P, AMP, ATP, and 3poly-P), and whole seawater samples (MUF-P, 3poly-P, and 45poly-P). Samples were amended with each substrate at a final concentration of 20 μM P. This concentration was assumed to be rate-saturating based on preliminary experiments with natural samples. Thus, the rates of P hydrolysis reported herein represent maximum hydrolysis rates, consistent with many previous investigations of P-monoester degradation (or alkaline phosphatase activity, APA) in the marine environment [see Mahaffey et al. (2014) and references therein]. P hydrolysis rates were determined through one of the following two methods. In the first method, which was applied to all P substrates, the production of SRP was monitored over time using the colorimetric protocol outlined above. In the second method, the time-dependent hydrolysis of the fluorogenic probe MUF-P was monitored using a standard fluorescence technique (Duhamel et al., 2011). Briefly, hydrolysis of MUF-P to 4-methylumbellierone (MUF) was measured (excitation: 359 nm, emission: 449 nm) and calibrated with a multi-point standard curve of MUF (10–500 nmol L-1). In both methods, samples were corrected for substrate autohydrolysis by accounting for negative controls, which were filtered (0.2 μm) and boiled (99°C, 15 min) prior to P amendment in order to eliminate enzyme activity.
In culture and field experiments, replicate samples and controls were incubated at room temperature (∼25°C) in a transparent (SRP method) or black (fluorescence method) 96-well plate. To ensure linearity of the hydrolysis rates, samples and controls were measured at multiple time points during the incubation period on a multimode plate reader (Molecular Devices). Overall incubation times were optimized depending on the sample type, P substrate, and the detection method employed. The SRP and fluorescence techniques were characterized by substantial differences in sensitivity. For example, the lower limit of detection, defined as three times the standard deviation of replicate blank measurements, was 800 nmol P L-1 for the SRP method and 2 nmol P L-1 for the fluorescence method. The upper limits of detection for the fluorescence method, defined as the P concentration above which the calibration curves begin to lose linearity, was 500 nmol L-1 P. Thus, samples exhibiting MUF-P hydrolysis rates within the limits of detection of the fluorescence method had to be incubated at least 1.6 times and up to 400 times longer before reaching detectable levels of SRP. The actual incubation times for the various sample types and analytical methods were as follows: whole seawater samples (fluorescence method: 12–15 h, SRP method: 24–65 h), cell-free culture filtrates (fluorescence method: 1–2 h, SRP method: 30 min to 24 h), whole culture samples (fluorescence method: 5 min to 1 h).
The SRP method was not used with whole culture samples due to interferences associated with the uptake/adsorption of enzymatically released phosphate by the cultures, which was problematic at high cell densities. To verify the lack of such interference in whole seawater samples, SRP calibration curves (2–20 μM P) were prepared in each sample and analyzed at multiple time points throughout the incubation period.
Genome Searches
Putative APs were identified in the translated genomes of T. pseudonana CCMP1335 (taxid: 296543) and T. oceanica CCMP1005 (taxid: 159749) with NCBI’s BLASTP search tool. The following protein sequences were used as queries: PhoA from Escherichia coli (GenBank: AAA83893), PhoD from Bacillus subtilis (UniProt: P42251), and PhoX from Pseudomonas fluorescens (Protein Data Bank: 4A9V). Only hits with an E-value < 1 × 10-4 are reported.
Proteomics
Concentrated exoproteome samples were digested using an in-solution tryptic digestion kit (ThermoScientific), according to the manufacturer’s instructions. Peptide samples were analyzed at the Proteomics and Mass Spectrometry (PAMS) facility at the University of Georgia on a Thermo-Fisher LTQ Orbitrap Elite mass spectrometer coupled with a Proxeon Easy NanoLC system (Waltham, MA, United States).
The enzymatic peptides were loaded into a reversed-phase column (self-packed column/emitter with 200 Å 5 μM Bruker MagicAQ C18 resin), then directly eluted into the mass spectrometer. Briefly, the two-buffer gradient elution (0.1% formic acid as buffer A and 99.9% acetonitrile with 0.1% formic acid as buffer B) starts with 5% B, holds at 5% B for 2 min, then increases to 25% B in 60 min, to 40% B in 10 min, and to 95% B in 10 min.
The data-dependent acquisition method was used to acquire MS data. A survey MS scan was acquired first, and then the top 5 ions in the MS scan were selected following collision-induced dissociation (CID) and higher-energy collisional dissociation (HCD) MS/MS analysis. Both MS and MS/MS scans were acquired by the Orbitrap mass spectrometer at resolutions of 120,000 and 30,000, respectively. Data were acquired using Xcalibur software (version 2.2, Thermo Fisher Scientific).
Protein identification was performed using Thermo Proteome Discoverer (version 1.4) with Mascot (Matrix Science). The reference database for T. oceanica CCMP1005 consisted of the translated whole genome (NCBI Bioproject PRJNA36595) (Lommer et al., 2012) amended with a list of common contaminants, such as human keratin. The T. pseudonana CCMP1335 database was compiled from the translated nuclear (Bioproject PRJNA191), chloroplast (Bioproject PRJNA20561), and mitochondrial (Bioproject PRJNA15818) genomes (Armbrust et al., 2004; Oudot-Le Secq et al., 2007), and was also amended with common contaminant sequences. Databases included a reversed ‘decoy’ version for false discovery rate (FDR) analysis. The FDR of identified peptides was ∼2%. Protein matches were also filtered to exclude hits with fewer than two unique peptide identifications. Gene ontology (GO) annotations were integrated into results using the ProteinCenter Annotation node.
Statistical Analysis
P hydrolysis rates in diatom cell-free filtrates were measured throughout the growth curve of each species cultivated in replete (+P) or P-deficient (-P) media. Differences in hydrolysis rates among the three P sources under each growth condition were assessed using repeated measures analysis of variance (ANOVA) and post hoc testing with Tukey’s honest significant difference (HSD) method. Average ratios of ATP and 3polyP hydrolysis were compared using a one-sample T-test. To test whether the degradation of each P source was P-regulated, cell-normalized rates of P hydrolysis were compared in +P and -P cultures using repeated measured ANOVA. Average P hydrolysis rates measured in whole cultures and in cell-free filtrates with different quantification methods were compared using Tukey’s HSD test. MUF-P hydrolysis rates determined by the SRP vs. fluorescence methods were assessed using simple linear regression. Field-based rates of polyphosphate and MUF-P hydrolysis were compared at each site using an independent two-sample T-test assuming equal variance. Potential relationships between P hydrolysis rates and in situ chlorophyll concentrations were explored with simple linear regression. All statistical analyses were performed in Microsoft Excel® or JMP® Pro (V13.0).
Results
Degradation of Model P Sources by Diatom Cultures
Relative Lability of P Sources in Cell-Free Filtrates
In order to explore the DOP nutritional preferences of Thalassiosira spp., the labilities of model P sources representing the major P(V) DOP bond classes (Figure 1) were tested in whole cultures and cell-free filtrates grown in P-replete (+P) and P-deficient (-P) media. Because P hydrolysis rates were quantified based on the production of SRP, cell-free filtrates were primarily utilized instead of whole cultures in order to avoid technical challenges associated with the analysis of SRP at high cell densities (see Discussion). All model P sources were degraded in diatom filtrates (Figures 2–4) and showed negligible hydrolysis (below detection) in boiled controls, consistent with enzymatic P-hydrolysis. In -P cultures, P hydrolysis rates were substantial within 1 week (T. oceanica and T. pseudonana CCMP1014) (Figures 2B,F) or after 2 weeks (T. pseudonana CCMP1335) (Figure 2D). In +P cultures, DOP degradation increased dramatically after about ∼2 weeks (Figure 2). Hydrolysis rates increased in all cultures over time, consistent with the depletion of SRP and the eventual onset of stationary phase (Figures 2A,C,E). Degradation rates among the three P sources were significantly different in each diatom culture and media condition throughout the growth curves (Figure 2). In every case, regardless of the diatom strain and the initial level of P availability, the rate of inorganic 3poly-P hydrolysis was significantly highest, followed by ATP, and finally AMP (Figure 2). Over the entire growth curve, ATP and AMP hydrolysis rates were statistically similar for both strains of T. pseudonana (Figures 2C–E), except for T. pseudonana CCMP1014 cultivated in -P media (Figure 2F). On the other hand, T. oceanica always hydrolyzed ATP significantly more than AMP, regardless of prevailing P availability (Figures 2A,B).
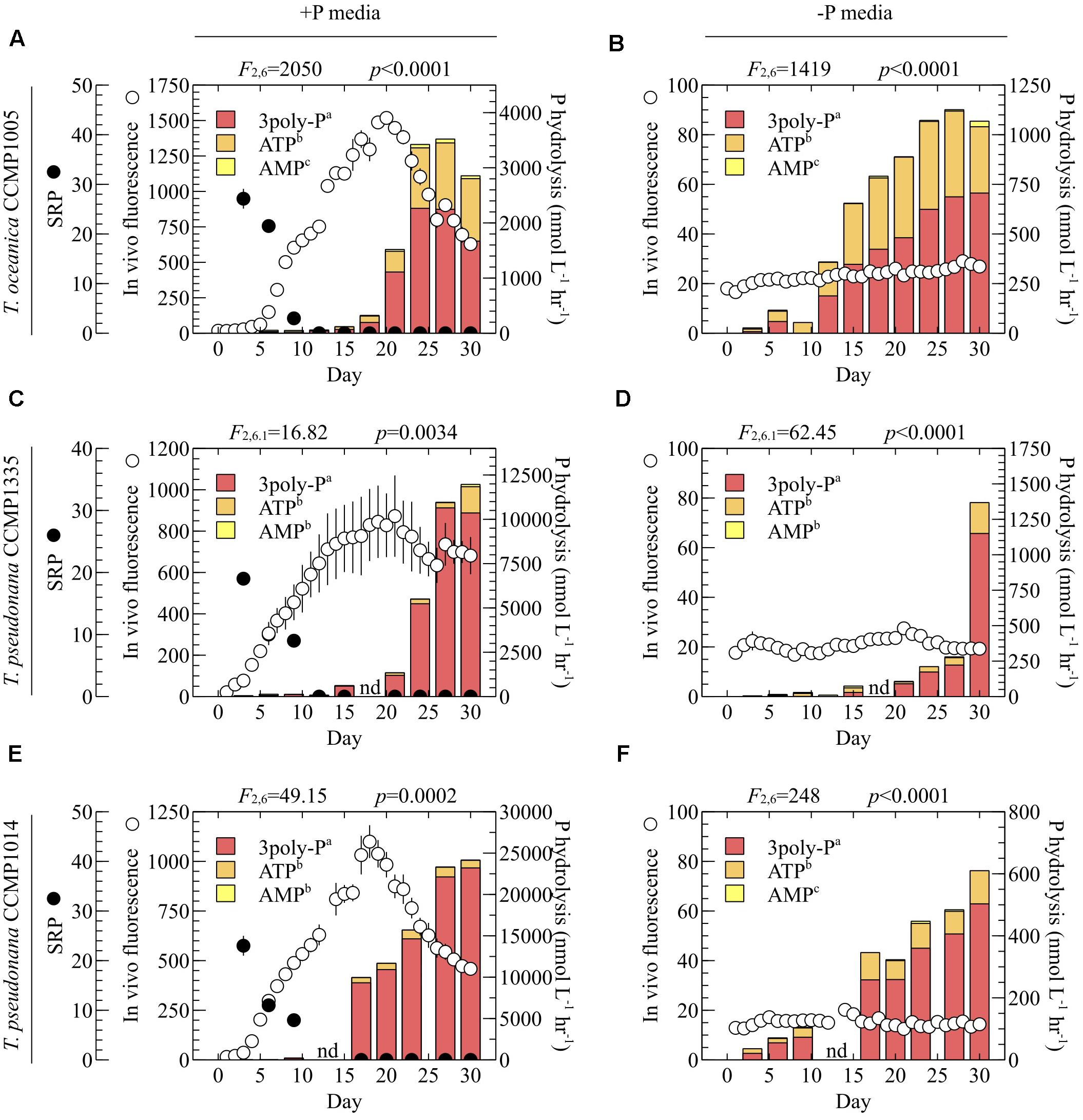
FIGURE 2. P hydrolysis rates of model DOP compounds in diatom cell-free filtrates. (A,B) T. oceanica CCMP1005, (C,D) T. pseudonana CCMP1335, and (E,F) T. pseudonana CCMP1014. (A,C,E) P-replete media, and (B,D,F) P-deficient media. Soluble reactive phosphorus (SRP) concentrations were below detection (800 nmol L-1) in –P cultures and are not depicted. Error bars indicate one standard deviation (SD) of the mean of three biological replicates. Statistical results from repeated measures ANOVA are provided above each plot and indicate the overall effect of P sources on hydrolysis rates. Results from the pairwise post hoc comparison of each P source via Tukey’s honest significant difference test are provided next to the legend entries. P sources lacking a shared letter are significantly different (p < 0.05). nd, no data.
A maximum of three orthophosphate molecules can be released from ATP and 3poly-P. However, the complete degradation of phosphoanhydride bonds would produce three orthophosphate groups from 3poly-P but only two from ATP, since the final orthophosphate is bound to the adenosine moiety by a P-monoester linkage (Figure 1). Therefore, if the P-anhydride bonds of ATP are degraded, and the P-monoester bond remains intact, the ratio of ATP:3polyP hydrolysis should be 2/3 (or ∼66.7%). However, ratios of ATP:3poly-P hydrolysis over the last 1–2 weeks of growth were significantly less than 2/3 (Table 1 and Figures 2A,C–F), except in the case of T. oceanica (-P) (Table 1 and Figure 2B), in which ATP degradation was statistically similar to 66.7% of 3poly-P degradation.
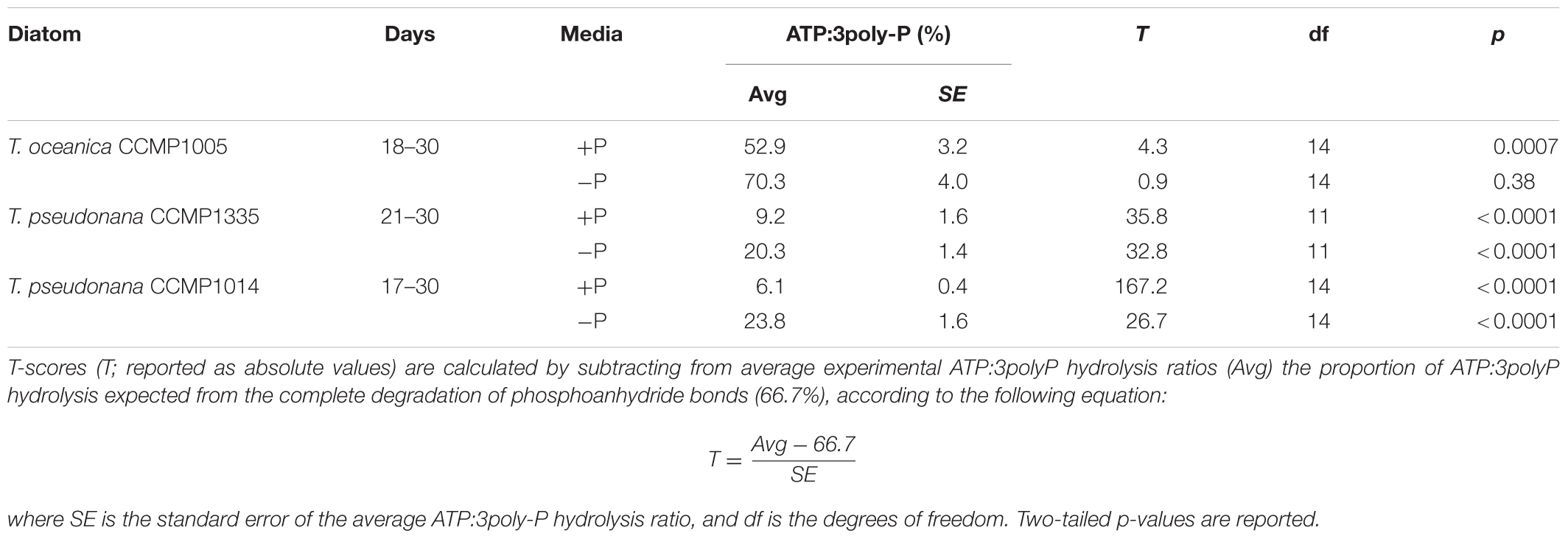
TABLE 1. Comparison of ATP and 3poly-P hydrolysis rates in diatom cell-free filtrates from Figure 2.
The Effect of Overall P Availability on Substrate-Specific DOP Hydrolysis in Cell-Free Filtrates
The lability of 3poly-P, ATP, and AMP was assessed in the cell-free filtrates of diatoms cultivated in P-replete and P-deficient media. To account for differences in biomass, P hydrolysis rates were normalized to total cell counts in cultures at the respective sampling time. ATP hydrolysis was significantly upregulated under -P conditions in all diatom cultures (Figures 3G–I). On the other hand, P availability did not significantly affect AMP hydrolysis in any culture (Figures 3J–L). P-deficient conditions induced significant upregulation of 3poly-P hydrolysis by T. oceanica CCMP1005, significant downregulation by T. pseudonana CCMP1014, and no change by T. pseudonana CCMP1335 (Figures 3D–F).
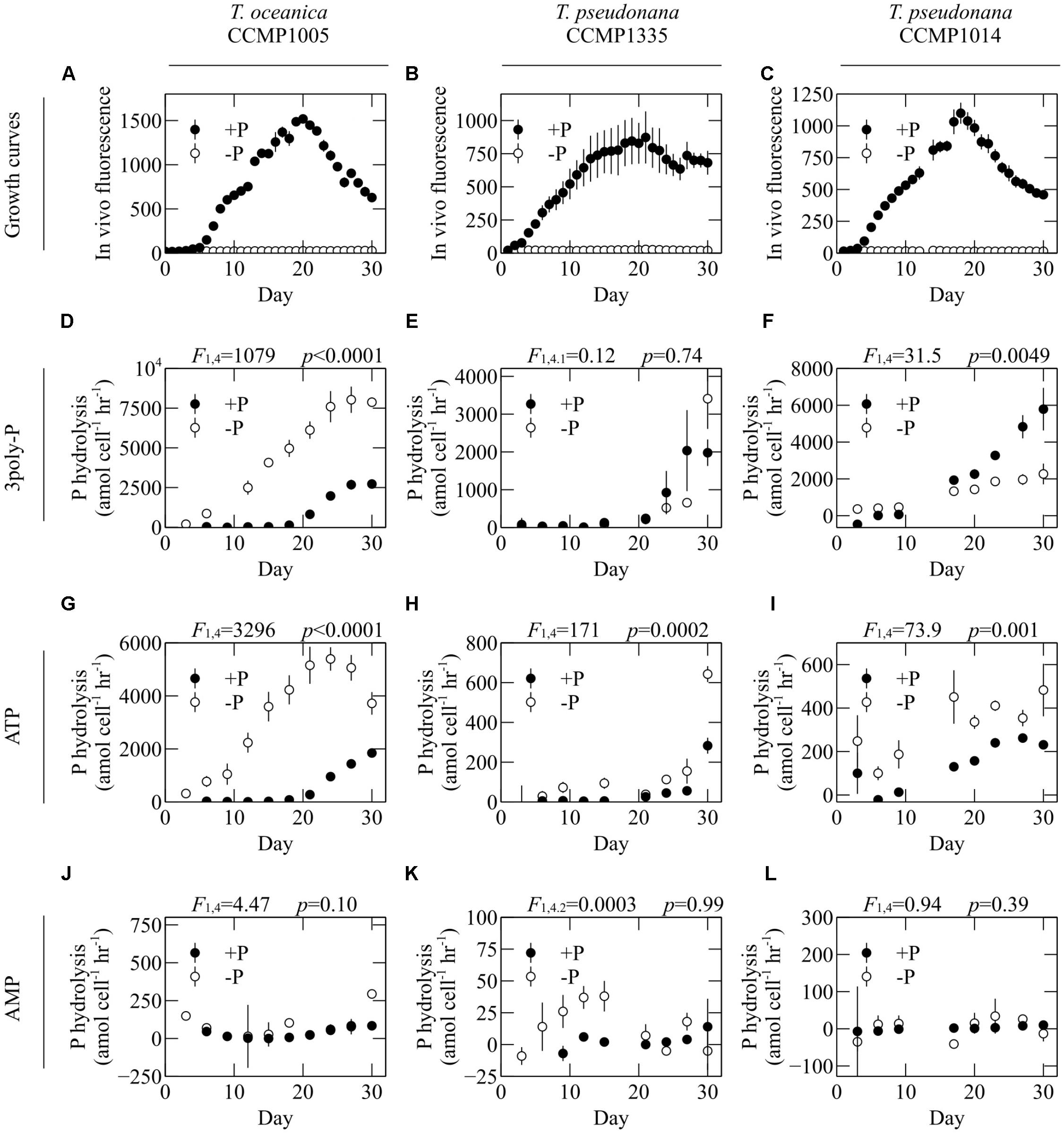
FIGURE 3. The effect of overall P availability on DOP hydrolysis rates. (A–C) Growth curves and hydrolysis rates of (D–F) 3poly-P, (G–I) ATP, and (J–L) AMP in the cell-free filtrates of T. oceanica CCMP1005 (A,D,G,J), T. pseudonana CCMP1335 (B,E,H,K), and T. pseudonana CCMP1014 (C,F,I,L) cultivated under P-replete (+P) and P-deficient (–P) conditions. Error bars represent one standard deviation of the mean of three biological replicates. Statistical results from repeated measures ANOVA are provided above each plot and indicate the overall effect of P availability on hydrolysis rates.
Comparison to Fluorescence-Based MUF-P Hydrolysis Measurements
For all diatoms, 3poly-P hydrolysis in cell-free filtrates was significantly higher than MUF-P hydrolysis in whole cultures (Figure 4). ATP degradation by T. oceanica CCMP1005 cell-free filtrates was also significantly higher than whole culture MUF-P hydrolysis (Figure 4A). Rates of P-ester hydrolysis in the cell-free filtrates of all diatoms reflected strong methodological agreement. For example, AMP and MUF-P (fluorescence and SRP methods) were degraded at similar rates (Figure 4). In fact, SRP-based estimates of MUF-P hydrolysis systematically underestimated fluorescence-based measurements of MUF-P degradation by about ∼17% (p = 0.0008) in culture filtrates (Figure 5).
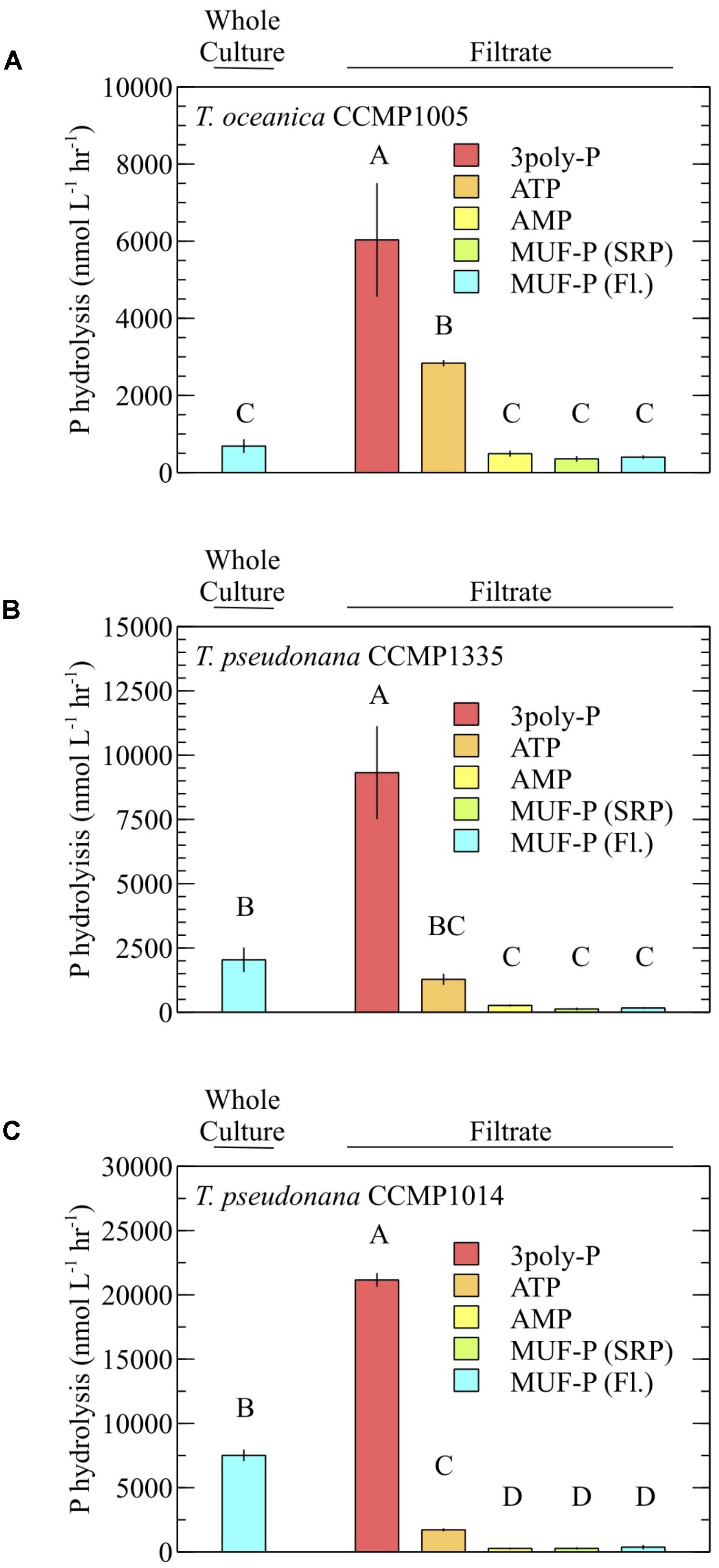
FIGURE 4. P hydrolysis rates of model DOP compounds in the presence (whole culture) and absence (filtrate) of cells and using different detection methods. (A) T. oceanica CCMP1005, (B) T. pseudonana CCMP1335, and (C) T. pseudonana CCMP1014. All rates were quantified by measuring the accumulation of phosphate over time (SRP method), except where MUF-P degradation was quantified via the standard fluorometric method (Fl.). Cultures were analyzed after 30 days of growth. Error bars represent one standard deviation of the mean of three (A,B) or two (C) biological replicates. P hydrolysis rates lacking a shared letter are significantly different (p < 0.05; Tukey’s honest significant difference).
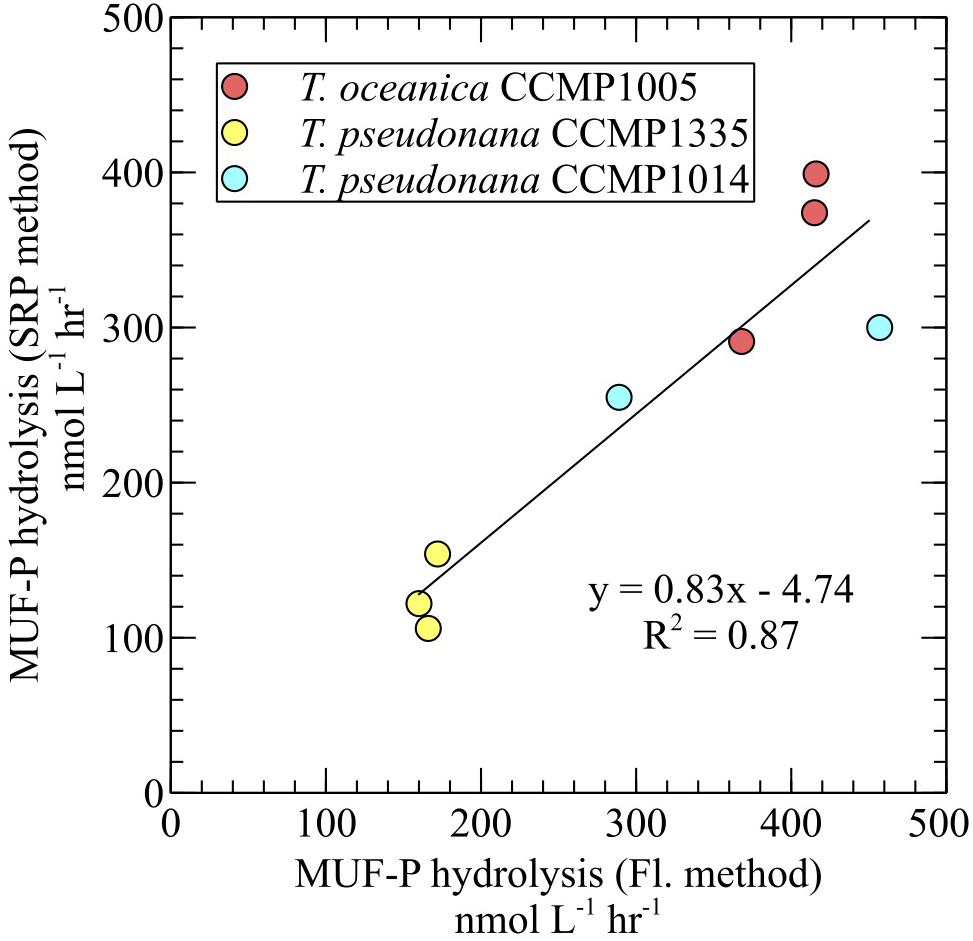
FIGURE 5. Comparison of MUF-P hydrolysis rates obtained by the SRP quantification method and the fluorometric method in cell-free culture filtrates.
Substrate-Specific P Hydrolysis Rates in the Coastal Western North Atlantic
Maximum hydrolysis rates of inorganic poly-P were measured in whole seawater samples from the coastal North Atlantic (Figure 6A) using the SRP method with two substrates: 3poly-P and 45poly-P. Poly-P chain length had no effect on P-hydrolysis rates, and the results from both poly-P sources were therefore combined into single average rates of inorganic poly-P hydrolysis. These rates were compared to maximum MUF-P hydrolysis rates determined via fluorescent detection. Average volume-normalized P hydrolysis rates ranged from 3.8–19.3 nmol P L-1 hr-1 and 1.4–50.6 nmol P L-1 hr-1 for inorganic poly-P and MUF-P, respectively (Figure 6B). Chlorophyll-normalized rates ranged from 5.6–7.7 nmol P μg chl-1 hr-1 and 2.5–20.1 nmol P μg chl-1 hr-1 for inorganic poly-P and MUF-P, respectively (Supplementary Table S1). SRP levels were above ∼700 nmol L-1, except in a phytoplankton bloom along the New Jersey Coast, where SRP was 150 ± 15 nmol L-1 (avg ± SD, n = 3; Supplementary Table S1).
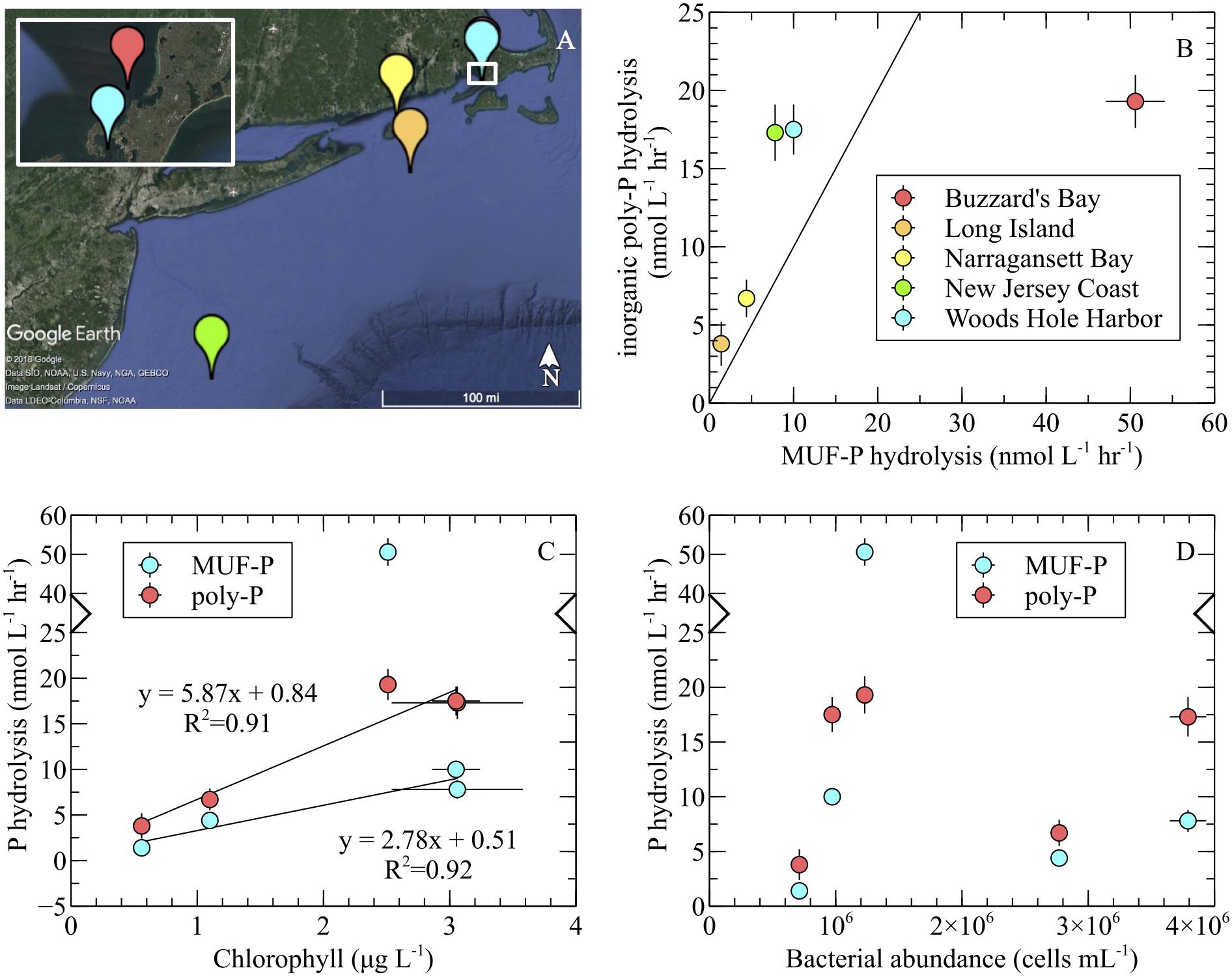
FIGURE 6. Inorganic poly-P hydrolysis (SRP method) and MUF-P hydrolysis (fluorometric method) in the coastal western North Atlantic. (A) Samples were collected from the New Jersey coast (green; 39°24′43.50′′N, 73°14′56.64′′W), offshore Long Island (orange; 40°53′43.98′′N, 71°22′49.92′′W), Narragansett Bay (yellow; 41°17′12.12′′N, 71°30′11.64′′W), Woods Hole harbor (blue; 41°31′24.60′′N, 70°40′40.80′′W), and Buzzard’s Bay (red; 41°32′38.28′′N, 70°40′8.46′′W). (B) Substrate-specific P hydrolysis comparison. The diagonal line depicts the 1:1 ratio of P hydrolysis rates. (C) Relationship of P hydrolysis rates to chlorophyll levels. For inorganic poly-P hydrolysis, the regression analysis was performed on the entire dataset (note the two overlapping data points at ∼3 μg L-1). For MUF-P hydrolysis, the Buzzard’s Bay outlier (note broken axis) was excluded from the regression analysis. Chlorophyll correlated significantly with inorganic poly-P degradation (p = 0.01) and MUF-P hydrolysis (p = 0.04). (D) P hydrolysis rates and bacterial abundance. In all panels, error bars represent the standard error of the mean of replicate samples (MUF-P hydrolysis: n = 3; inorganic poly-P hydrolysis: n = 8; chlorophyll: n = 3; bacterial abundance: n = 3).
Inorganic poly-P hydrolysis rates were significantly higher than rates of MUF-P hydrolysis (p < 0.05) in four out of the five field sites sampled, by a factor of ∼1.5 to ∼2.7 (Figure 6B and Table 2). However, in one field site (Buzzard’s Bay), MUF-P hydrolysis was ∼2.5-fold higher than inorganic poly-P hydrolysis (p < 0.05; Figure 6B and Table 2). Inorganic poly-P hydrolysis rates exhibited a significant linear correlation with chlorophyll levels (p = 0.01; Figure 6C), which was also evident for rates of MUF-P hydrolysis when the Buzzard’s Bay outlier was excluded (p = 0.04; Figure 6C). However, P hydrolysis rates did not exhibit a significant relationship with bacterial abundance counts (Figure 6D). Bacteria cell counts (cells mL-1) were ∼125 times higher than phytoplankton counts at the Buzzard’s Bay site, yet only ∼17 to ∼45 times higher at all other locations (Supplementary Table S1).
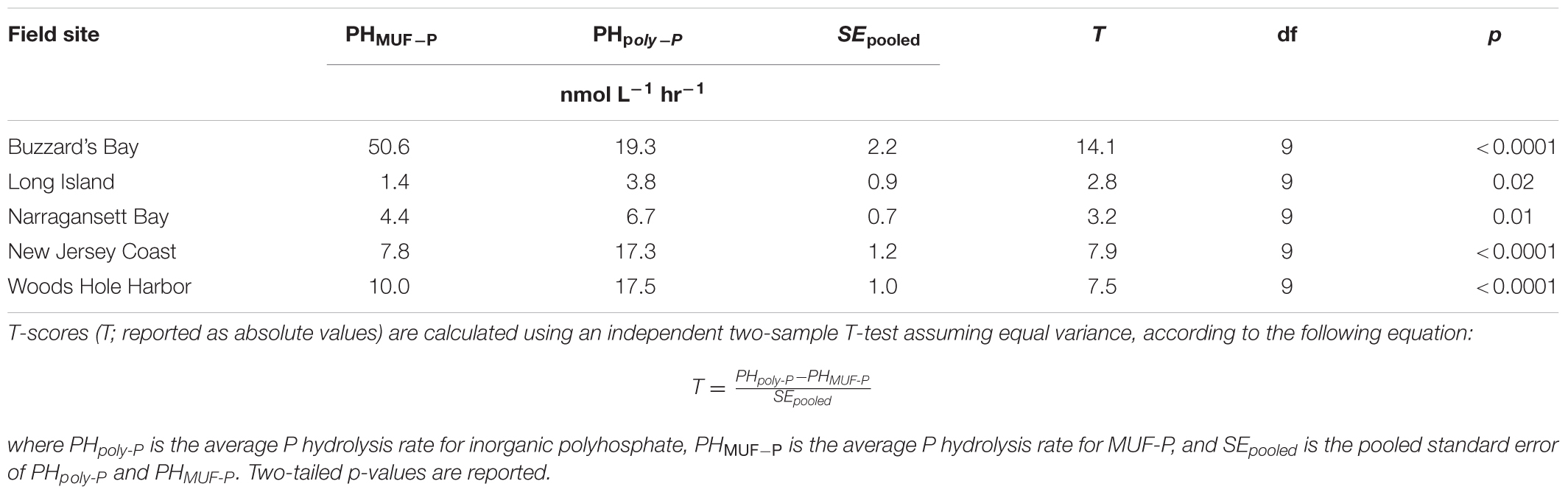
TABLE 2. Comparison of inorganic poly-P and MUF-P hydrolysis rates in field samples from Figure 6.
SRP uptake/adsorption by whole seawater samples can potentially lead to the underestimation of P hydrolysis rates made by the SRP method. The degree of SRP uptake/adsorption was therefore assessed with standard additions of Na2HPO4 (P = 2–20 μM). Phosphate levels in these amended samples did not change over the course of the 24–65 h incubations.
Phosphatase Diversity in Diatom Cultures
The full genome data of two model diatom strains utilized in this study, T. oceanica CCMP1005 and T. pseudonana CCMP1335, are publicly available, enabling the analysis of phosphatase diversity in these two species. First, the genomes were searched for putative homologs of three bacterial alkaline phosphatase isoforms (PhoA, PhoD, and PhoX) using BLASTP analysis. In T. oceanica CCMP1005, results revealed the presence of two putative PhoD homologs, one putative PhoX homolog, but no homologous PhoA sequences (Table 3). On the other hand, T. pseudonana CCMP1335 possesses one putative homolog each of PhoA and PhoD, but no homologous sequences to PhoX (Table 3).
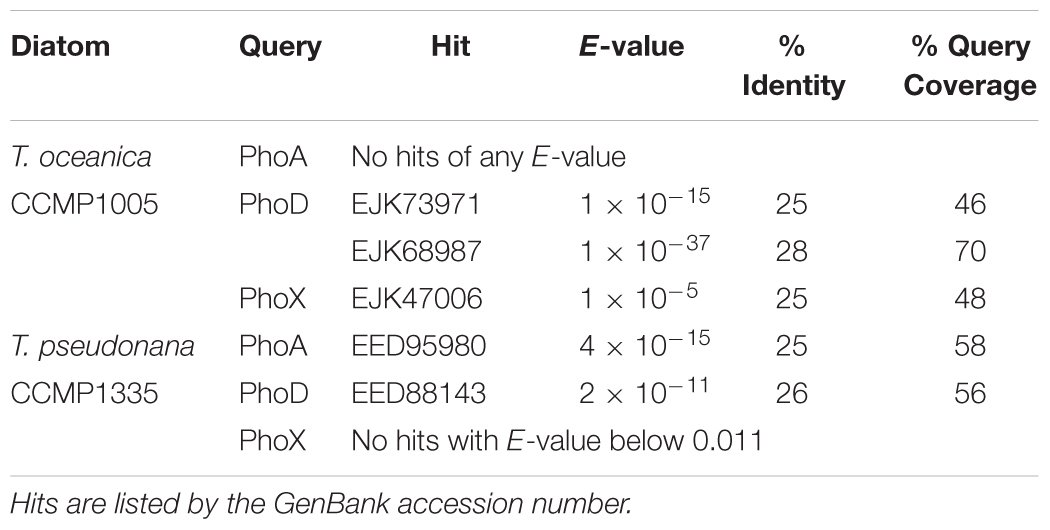
TABLE 3. Putative homologs of bacterial alkaline phosphatase in the genomes of T. oceanica CCMP1005 and T. pseudonana CCMP1335.
Since cell-free filtrates were the primary focus of substrate-specific P hydrolysis measurements, the diversity of phosphatases was investigated in cell-free filtrates of T. oceanica CCMP1005 and T. pseudonana CCMP1335 (under +P conditions for each culture only). Excluding contaminant sequences (i.e., keratin), a total of 309 and 133 proteins were identified in extracellular proteomes of T. oceanica CCMP1005 and T. pseudonana CCMP1335, respectively (Supplementary Tables S2, S3). Among these hits, 24 from T. oceanica CCMP1005 and 9 from T. pseudonana CCMP1335 possess conservative domains consistent with phosphatase activity. The majority of these hits were shared among biological triplicates of each diatom (Figure 7). None of the putative bacterial alkaline phosphatase homologs identified through genome searches (Table 3) were detected in the cell-free culture filtrates, however. Rather, results indicated a diversity of other putative phosphatases, including P-anhydride- and P-ester-degrading enzymes. In fact, P-anhydride-degrading enzymes dominated the phosphatase diversity in the cell-free filtrates of both microorganisms, including inorganic pyrophosphatase and nucleoside triphosphates (Figure 7).
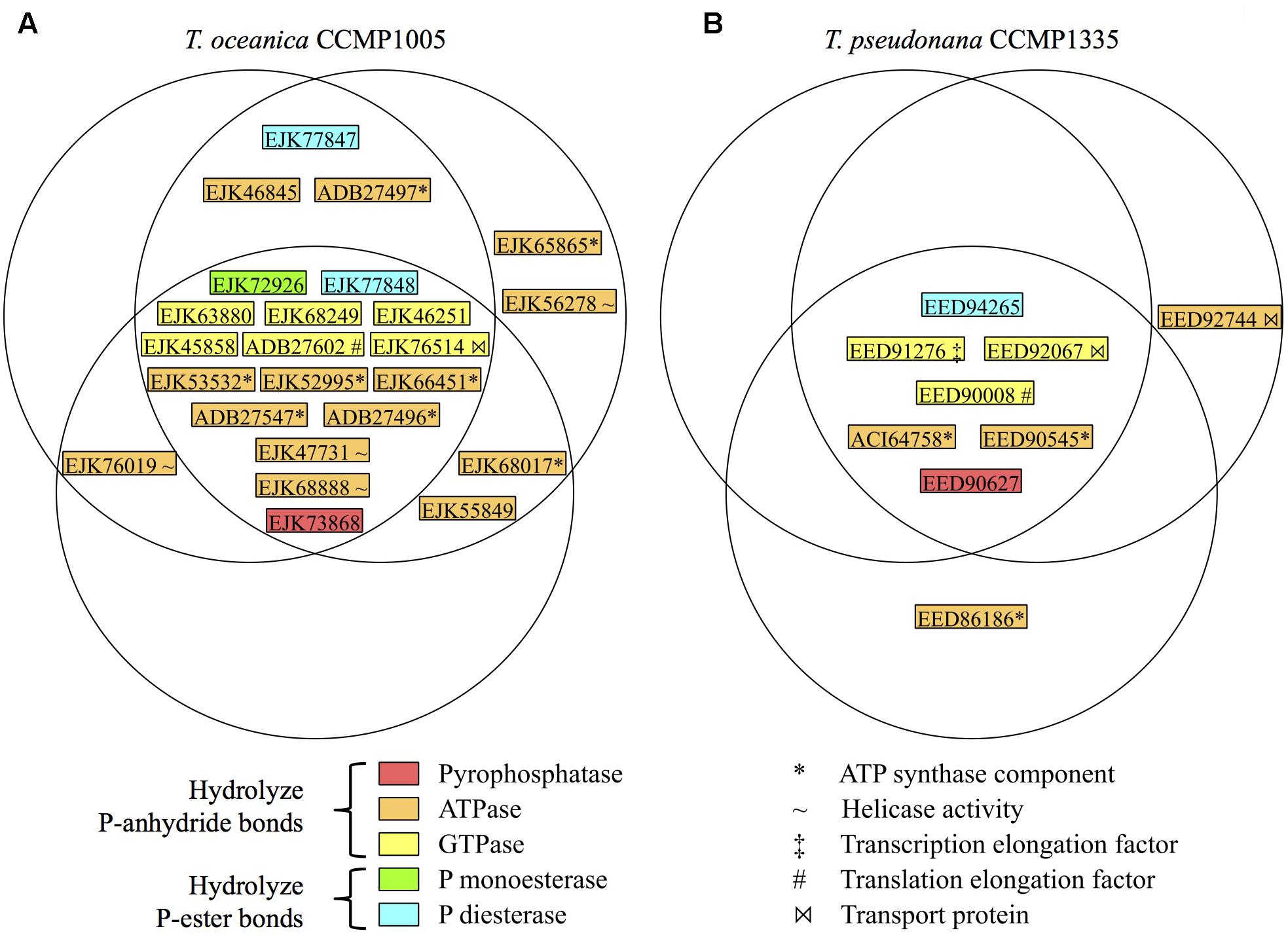
FIGURE 7. Diversity and distribution of phosphatases in replicate cell-free filtrates of (A) T. oceanica CCMP1005 and (B) T. pseudonana CCMP1335. Each circle represents one biological replicate. GenBank accession numbers are provided. For ease of comparison to a previous study by Dyhrman et al. (2012), the JGI PID’s for the T. pseudonana CCMP1335 phosphatases identified in this study are as follows: Genbank EED91276 (JGI PID 269148); ACI64758 (41256); EED90008 (41829); EED90627 (269348); EED90545 (269322); EED92067 (22792); EED92744 (262083); EED86186 (jgi| Thaps3_bd| 1287| e_gw1.26x41.22.1).
Discussion
The goal of this study was to assess the relative lability of model P sources that represent the major marine DOP bond classes. The focus here was on the P(V) DOP bond classes – P-esters and P-anhydrides – because of the potential role that these compound classes have in P utilization by the bulk phytoplankton community. Maximum potential hydrolysis rates of model P-esters and P-anhydrides were assessed in the cell-free filtrates of representative diatom cultures and coastal field sites, while phosphatase diversity was also investigated in model diatom filtrates. Overall, results revealed that an innate capacity for the preferential utilization of P-anhydrides was prevalent across all of these sample types.
Degradation of Model P Sources by Diatom Cultures
In model diatoms, maximum rates of P hydrolysis were examined in cell-free filtrates that were prepared via syringe filtration of culture samples. Cell-surface and intracellular phosphatases may have been adventitiously released through the potential disruption and lysis of some cells during this process. Thus, P hydrolysis rates in these cell-free filtrates may overestimate truly dissolved rates of P hydrolysis. However, the aim here was not to quantify cell-free P hydrolysis rates per se, but to avoid technical barriers associated with analyzing the degradation of unlabeled P sources in the presence of cells. In particular, tracking P hydrolysis through the production of SRP becomes difficult at high cell densities because microorganisms have a strong ability to adsorb (Sanudo-Wilhelmy et al., 2004) and assimilate dissolved P.
Cell-free filtrates can only provide a glimpse into the overall DOP preferences of a culture because most DOP degradation takes place in the presence of cells. Thus, MUF-P hydrolysis in whole cultures was measured with a standard fluorometric protocol in order to help contextualize the P dynamics observed in cell-free filtrates. Surprisingly, inorganic 3poly-P degradation in the filtrates vastly exceeded MUF-P hydrolysis levels in whole cultures (p < 0.05), by at least ∼3-fold (T. pseudonana CCMP1014) and up to ∼9-fold (T. oceanica CCMP1005; Figure 4). This finding is probably not a result of artificially elevated 3poly-P hydrolysis due to a filtration artifact, because most extracellular DOP-degrading enzymes localize on the cell surface and thus, most of the DOP degradation capacity is removed by filtering the cells out. Indeed, MUF-P hydrolysis was significantly lower in cell-free filtrates than in whole cultures (p < 0.05; Figure 4). Nor are the large differences between whole-culture MUF-P hydrolysis and filtrate-based 3poly-P degradation rates a consequence of the different detection methods used. In fact, the SRP method is the more conservative approach (Figure 5) yet still shows remarkable correspondence with the MUF-P fluorescence method (Figure 4), especially given the large difference in sensitivity between these two techniques.
Relatively low levels of P hydrolysis were detected in the cell-free filtrates of actively growing and late log-phase cultures (Figure 2A, days 6–18; Figure 2C, days 6–15; Figure 2E, days 9, 17; Figures 3D–I). However, P hydrolysis rates were highest during the later stages of cultivation, including stationary phase and crash (Figures 2, 3). In fact, the hydrolysis of 3poly-P and ATP increased as the cultures matured, even after accounting for increases in biomass through the growth curve (Figures 3D–I). These results may be a consequence of differences in the release, stability, or activity of cell-free P hydrolases over the life of the culture. For example, one interpretation is that active P-hydrolyzing enzymes can be released from healthy, growing cells, but these enzymes may be more extensively released from aging and senescent cells. This release could be actively driven by the diatoms themselves and/or mediated by physical mechanisms such as turbulence (e.g., daily culture mixing) or filtering. Like natural seawater, the cell-free filtrates examined in this study likely contain a mixture of actively and passively released proteins. If cell-free P hydrolysis is indeed linked to cell aging and senescence, as our data suggest, then the dissolved P hydrolase activity of seawater may be highest under certain environmental conditions, such as the collapse of phytoplankton blooms.
All P substrates examined (MUF-P, AMP, ATP, and 3poly-P) were actively degraded in diatom filtrates, but inorganic 3-polyP, followed by ATP, were the most rapidly transformed, regardless of overall P availability (Figures 2, 3). Rates of ATP hydrolysis were lower (most cases) or approximately equal (one case) to that expected from the complete degradation of P-anhydride bonds (Table 1). Thus, P-ester hydrolysis may not have played a role in ATP degradation, even though P-ester hydrolysis lead to a relatively small level of AMP degradation in the same samples. Assuming that only terminal phosphate groups are enzymatically labile (Huang et al., 2018), we speculate that P-anhydrides may block P-esterases from accessing the P-O-C bond of ATP. Overall, these findings suggest that Thalassiosira spp. may preferentially hydrolyze inorganic poly-P compared to other DOP sources under a wide range of prevailing P availability. A variety of potential mechanisms may underlie the preferential degradation of P-anhydrides in diatom cell-free filtrates, including differences in the amount, substrate versatility, stability, and diversity of P-hydrolyzing enzymes.
Thalassiosira oceanica exhibited the most versatile response to P amendments, consistent with a diverse nutrient utilization profile expected in this isolate from the P-limited Sargasso Sea. For example, T. oceanica had the broadest potential to utilize both 3poly-P and ATP, regardless of overall P status (Figure 2). Furthermore, every culture upregulated the degradation of ATP under P-deplete conditions, but T. oceanica was the only one that also increased its capacity to degrade 3poly-P in response to P scarcity (Figure 3). These results suggest that while enhanced ATP utilization may be a widespread adaptation to low P availability among Thalassiosira spp., elevated 3poly-P utilization may not be. Rather, in T. pseudonana, active P-anhydride degradation may be constitutive. Overall, the P utilization potential of T. pseudonana CCMP1014, an oceanic isolate from the NPSG, exhibited more similarity toward T. pseudonana CCMP1335 than T. oceanica. This finding may indicate that species- rather than habitat-level factors may be a more prevalent control over DOP cycling by these diatoms, although each of these strains has been in culture for over 40 years and some genetic drift has likely occurred.
P Hydrolysis of Model DOP Sources in the Coastal Western North Atlantic
Although some SRP uptake probably occurred in our field incubations, the results suggest that it was too slow to decrease SRP concentrations by more than the detection limit of 800 nmol L-1. This outcome is consistent with SRP uptake rates observed in other coastal systems with similar chlorophyll concentrations as our field sites (Harrison et al., 1977). SRP uptake therefore did not affect measured rates of inorganic poly-P hydrolysis.
Degradation rates of inorganic 3poly-P and 45poly-P were similar in field samples, which is consistent with the chain length-independent utilization of poly-P by some eukaryotic phytoplankton observed in a previous study (Diaz et al., 2016). Maximum rates of inorganic poly-P hydrolysis determined by the SRP method were significantly higher than fluorescence-based measurements of MUF-P hydrolysis in four out of five field sites examined in the coastal North Atlantic (Figure 6B and Table 2). These differences may be even more extreme, based on the finding that the SRP method for P hydrolysis is systematically conservative relative to the fluorescence-based approach (Figure 5).
The majority of studies on marine DOP hydrolysis have focused on P-monoester degradation in oligotrophic waters, where DOP hydrolysis is thought to function primarily as a phosphate-inhibitable phytoplankton response to P depletion (Duhamel et al., 2010; Lin et al., 2016). Above the threshold SRP level of ∼30 nmol L-1, P-monoester hydrolysis is typically less than ∼1 nmol L-1 hr-1 (∼20 nmol μg chl-1 hr-1) in these systems (Mahaffey et al., 2014). Consistent with this finding, chlorophyll-normalized rates of MUF-P and inorganic poly-P hydrolysis are less than ∼20 nmol μg chl-1 hr-1 in the relatively high-P coastal sites examined here (SRP > ∼150 nmol L-1). However, because total chlorophyll levels are much higher in the waters examined in this study, the volume-normalized rates are much higher (∼4- to ∼20-fold) than in the oligotrophic ocean. Similar rates of volume-normalized P-ester hydrolysis have been previously reported from coastal systems and deep waters (Li et al., 1998; Dyhrman and Ruttenberg, 2006; Nicholson et al., 2006; Davis et al., 2014).
The strong correlation of P hydrolysis rates with chlorophyll levels (Figure 6C), and the lack of correlation with bacterial abundance (Figure 6D), suggests that the observed P dynamics were driven by phytoplankton. MUF-P hydrolysis (but not inorganic poly-P degradation) at the Buzzard’s Bay site was inconsistent with the overall relationship to chlorophyll, which suggests additional, non-phytoplankton associated mechanisms of P-monoester degradation, such as contributions from heterotrophic bacteria. Heterotrophic bacteria utilize phosphatases to remineralize and acquire the carbon and nitrogen contained in DOP (Wilkins, 1972; Wanner and McSharry, 1982; Hoppe and Ullrich, 1999). Because the degradation of inorganic poly-P yields no carbon or nitrogen, heterotrophic bacteria may not contribute extensively to the degradation of inorganic poly-P sources. Thus, in a system dominated by bacterially driven DOP hydrolysis, the inorganic poly-P and P-ester dynamics may be decoupled, such that P-ester hydrolysis rates are substantially higher than inorganic poly-P degradation, consistent with results from the Buzzard’s Bay site. In agreement with this hypothesis, the ratio of bacteria to phytoplankton cells was ∼3-fold higher in Buzzard’s Bay than at any other location (Supplementary Table S1). Furthermore, White et al. (2012) found that 3poly-P was less extensively degraded than P-esters like AMP and glucose-6-phosphate in seawater incubations that had been pre-conditioned to favor bacterial growth.
Phosphatase Diversity in Diatom Cultures
Enzymes modulating the P-hydrolysis reactions observed in diatom cultures and coastal Atlantic field samples may include phosphatases such as AP, inorganic pyrophosphatase, and nucleoside triphosphatases. AP’s are the best-known group of enzymes involved in DOP utilization by phytoplankton (Lin et al., 2016). These P-esterases are widely distributed in prokaryotic and eukaryotic microorganisms yet show only limited homology across these two groups (Lin et al., 2012). Furthermore, AP’s are much better characterized in prokaryotes than microbial eukaryotes (Shaked et al., 2006; Xu et al., 2006, 2010; Lin et al., 2011, 2013, 2015, 2016). For example, major prokaryotic AP isoforms include PhoA, PhoX, and PhoD (Luo et al., 2009, 2011). All of these have been detected in seawater, with PhoD being the most prevalent, followed by PhoX (Luo et al., 2009; Sebastian and Ammerman, 2009; Kathuria and Martiny, 2010; Luo et al., 2011). Substrate specificities of the AP isoforms are not completely characterized, but they may exhibit a wide degree of flexibility. For example, E. coli PhoA is also a polyphosphatase (Lorenz and Schroder, 2001; Huang et al., 2018).
Multiple putative AP’s have been recognized in the genome, transcriptome, and proteome of T. pseudonana CCMP1335 (Armbrust et al., 2004; Dyhrman et al., 2012), and at least one of these has been recognized for its resemblance to bacterial PhoA (Lin et al., 2016). Using BLASTP translated genome searches of T. pseudonana CCMP1335 and T. oceanica CCMP1005 with bacterial PhoA, PhoD, and PhoX queries, additional putative AP homologs were identified herein (Table 3). However, none of these sequences were apparent in the exoproteome data of either diatom species (Figure 7), suggesting that these enzymes may be exclusively cell-associated and ruling them out from participating in the P-hydrolysis dynamics observed in culture filtrates. Exoproteome samples revealed a number of other putative phosphatases, the diversity of which was biased in favor of P-anhydride degradation (Figure 7), consistent with the observed preferential hydrolysis of P-anhydrides in culture filtrates (Figures 2–4). The mechanisms leading to the appearance of these phosphatases in the exoproteome remain unclear, but may potentially include active secretion, passive dislodging of cell surface-associated enzymes during cultivation, and/or adventitious release during filtrate preparation.
Nearly all of the phosphatase hits present in the T. pseudonana CCMP1335 exoproteome (Figure 7) were also detected in its cell-associated transcriptome and proteome, which were reported in a previous study (Dyhrman et al., 2012). The only exception was the P-diesterase (GenBank EED94265). However, this sequence also could not be found in the JGI translated genome assemblies for T. pseudonana CCMP1335 utilized by Dyhrman et al. (2012). The absence of a protein sequence from the reference database precludes its identification in the experimental data. Thus, the absence of EED94265 in the proteome data from this previous study is at least partially a result of the different methods employed. As for the other putative phosphatases detected in the present study, none were upregulated in the T. pseudonana CCMP1335 transcriptome or proteome under P-deplete conditions (Dyhrman et al., 2012). In fact, three hits were upregulated under P-replete conditions: two GTPases (EED91276 in the proteome only; EED90008 in the transcriptome only) and one ATPase (transcriptome and proteome: EED92744). These results are consistent with the lack of upregulation of 3poly-P hydrolysis in the cell-free filtrate of this species under -P conditions (Figure 3E). On the other hand, ATP degradation was enhanced (∼2.5-fold) under low P availability in T. pseudonana CCMP1335 cell-free filtrates (Figure 3H), but this activity may not be regulated or sufficiently resolved at the transcriptional or translational levels.
Some of the exoproteome hits identified in this study may exhibit phosphatase activities by operating in the reverse direction of their annotated functions (e.g., ATP synthase components). Sequence information can identify candidate enzymes for a given biochemical function, however, these hits must be purified and assessed in vitro to verify their actual enzymatic reactivity. Furthermore, the presence of some protein hits in the exoproteomes may be unexpected. For example, an abundance of proteins affiliated with the ATP synthase complex were found in the exoproteomes of both diatom species. However, in some microorganisms, ATP synthase localizes in the cell membrane, utilizing the proton-motive force produced by the light activated proton-pump proteorhodopsin to generate ATP (Lin et al., 2016). In fact, proteorhodopsin expression may be an active aspect of diatom physiology (Marchetti et al., 2012; Marchetti et al., 2015). The potential localization of ATP synthase on the plasma membrane suggests that it could potentially become adventitiously dislodged without substantial cellular disruption or lysis (e.g., through daily physical mixing of cultures). Indeed, the release of cell-associated material was likely minimal during the preparation of diatom cell-free filtrates, as only 133 protein hits were detected in T. pseudonana CCMP1335 exoprotomes out of 1264 total protein hits reported in a prior study (Dyhrman et al., 2012).
Synthesis and Perspectives
The P-anhydride bond is essential to life. It serves as the universal basis of cellular energy transduction through the formation and hydrolysis of ATP and also plays diverse regulatory roles in the form of inorganic polyphosphates that are made by every cell in nature. As a widespread and labile constituent of marine DOP, P-anhydrides may also represent a bioavailable P source capable of supporting phytoplankton growth and productivity in the ocean. This P-anhydride pool has largely been overshadowed by the prevailing assumption that the much more abundant standing stock of marine P-esters is the most important DOP source. Based on results from this study, however, the possibility that P-anhydrides support a high degree, maybe even a majority, of DOP turnover and assimilation in diverse marine environments should be considered.
If P-esters are less preferred than P-anhydrides, then APA measurements may underestimate levels of P stress in oligotrophic systems, where phosphatase activity primarily functions in the acquisition of P by P-starved phytoplankton. In high-nutrient coastal systems, P-anhydrase activity may be constitutively expressed regardless of prevailing orthophosphate availability (Ammerman and Azam, 1991), suggesting that P-anhydrides may be a part of the highly labile DOP pool that is rapidly cycled in these environments (Benitez-Nelson and Buesseler, 1999). For example, in T. pseudonana, degradation of inorganic 3poly-P was just as high or higher under replete P conditions (Figures 3E,F). Furthermore, in T. pseudonana CCMP1335, this trend was consistent at the protein level, where each of the potential enzymes involved in 3poly-P degradation (Figure 7) were just as prevalent or more prevalent under P-rich conditions examined in a previous study (Dyhrman et al., 2012).
The degradation of dissolved P-anhydrides may be cryptic, with the relatively low abundance of this pool (Young and Ingall, 2010) masking rapid recycling rates. In situ P-anhydride degradation rates are dependent on the inherent degradation kinetics of the bulk P-anhydrase pool, as well as the in situ concentration of P-anhydride substrates. The maximum hydrolysis rates reported herein do not necessarily reflect in situ degradation rates, because actual P-anhydride levels may not be rate-saturating. In situ DOP utilization rates have been estimated based on standing stock DOP concentrations (Duhamel et al., 2011), but cryptic cycling commonly evades detection by concentration-based approaches (Canfield et al., 2010; Hansel et al., 2015; Berg et al., 2016). Rather, cryptic transformation rates may be more readily quantified by using high-sensitivity radiotracers amended at levels similar to in situ substrate concentrations. Biologically mediated cryptic pathways can also be illuminated by investigating the community-level metabolic potential, as manifest in environmental genome, transcriptome, and proteome sequence data. As a step toward this goal, the present study has identified a diversity of diatom-derived phosphatases that may drive P-anhydrase reactions in the marine environment.
In addition to radiotracers and bioinformatics approaches, the study of P-anhydride degradation in marine systems would also be advanced by the availability of representative labeled substrates. For example, P-ester degradation (conventionally referred to as APA) is routinely assessed using sensitive fluorogenic and chromogenic substrates (Hoppe, 2003; Dyhrman and Ruttenberg, 2006; Mahaffey et al., 2014; Davis and Mahaffey, 2017). By quantifying the inert, signal-bearing probe that is released during P hydrolysis, these substrates can be used to track P-hydrolysis rates independently of P dynamics, which is especially important at high cell densities. Synthesis of labeled inorganic poly-P has been described (Choi et al., 2010; Hebbard et al., 2014), but these substrates were unreactive in positive controls tested in this study (data not shown).
Future work should focus on methods to assess the P hydrolysis of diverse DOP compounds by whole cells. The application of such methods should confirm whether the DOP dynamics observed here in diatom cell-free filtrates reflect broader DOP nutritional preferences. For example, the P hydrolytic potential of diatom cell-free filtrates implies that these microorganisms have a capacity, and potentially a preference in the case of 3poly-P, to utilize a diversity of P sources for growth. If cultures had been amended with 3poly-P during stationary phase, the elevated potential for 3polyP hydrolysis (Figure 2) may have helped the cultures avoid crashing, although this was not tested. The P-hydrolysis of DOP sources is not necessarily coupled to the biological assimilation of enzymatically released P, however (Ammerman and Azam, 1985; Duhamel et al., 2017). For instance, heterotrophic bacteria are thought to degrade organic P sources as a carbon and/or nitrogen utilization strategy, and phosphate may accumulate in seawater as a result (Hoppe and Ullrich, 1999; Hoppe, 2003). Furthermore, if phosphatases are not actively degraded, then their activity can lead to the accumulation of dissolved inorganic P when other factors besides P availability limit plankton growth. In such a case, the accumulation of enzymatically released P may have other implications for marine ecosystem functioning. For example, the primary pathway for long-term P removal is the authigenic (or in situ) formation of marine calcium phosphate minerals (Ruttenberg, 2014). The direct precipitation of these minerals is kinetically inhibited in most marine environments but may involve inorganic poly-P as a reactive intermediate (Schulz and Schulz, 2005; Diaz et al., 2008). In fact, the inherently high capacity for P-anhydride degradation documented in diatom cultures and natural samples in this study suggests that pulsed or spatially-heterogeneous poly-P inputs could lead to the formation of transient, submicron-scale “hotspots,” which may be kinetically conducive to the formation of authigenic calcium phosphate minerals.
Data Availability Statement
The raw data supporting the conclusions of this manuscript are deposited at the Biological and Chemical Oceanography Data Management Office (http://bco-dmo.org) under project number 747715.
Author Contributions
JD, SD, and YT conceived the experiments and overall study design. JD, AH, JS, KB, DM, C-WC, and DP generated results and conducted the data analysis. All authors contributed to manuscript preparation.
Funding
This work was supported by the National Science Foundation under grants 1559124 (JD), 1736967 (JD), 1737083 (SD), and 1559087 (YT), as well as a Junior Faculty Seed Grant from the University of Georgia Research Foundation (JD). The Thermo-Fisher LTQ Orbitrap Elite Mass Spectrometer was purchased with funding from National Institutes of Health under grant S10RR028859.
Conflict of Interest Statement
The authors declare that the research was conducted in the absence of any commercial or financial relationships that could be construed as a potential conflict of interest.
Acknowledgments
We thank Colleen Hansel, Carl Lamborg and the science party and crew of the R/V Endeavor during the TORCH I cruise. We thank James Morrissey for his generosity in providing fluorogenic polyphosphate for our research, and Catherine Baker for synthesizing these substrates. We also thank Lee Ann DeLeo for her help with the preparation of graphics for this paper, and we acknowledge Mohit Jain for technical assistance with proteomics data analysis.
Supplementary Material
The Supplementary Material for this article can be found online at: https://www.frontiersin.org/articles/10.3389/fmars.2018.00380/full#supplementary-material
References
Alonso-Saez, L., and Gasol, J. M. (2007). Seasonal variations in the contributions of different bacterial groups to the uptake of low-molecular-weight compounds in northwestern Mediterranean coastal waters. Appl. Environ. Microbiol. 73, 3528–3535. doi: 10.1128/aem.02627-06
Ammerman, J. W., and Azam, F. (1985). Bacterial 5’-nucleotidase in aquatic ecosystems – A novel mechanism of phosphorus regeneration. Science 227, 1338–1340. doi: 10.1126/science.227.4692.1338
Ammerman, J. W., and Azam, F. (1991). Bacterial 5’-nucleotidase activity in estuarine and coastal marine waters: characterization of enzyme activity. Limnol. Oceanogr. 36, 1427–1436. doi: 10.4319/lo.1991.36.7.1427
Armbrust, E. V., Berges, J. A., Bowler, C., Green, B. R., Martinez, D., Putnam, N. H., et al. (2004). The genome of the diatom Thalassiosira pseudonana: ecology, evolution, and metabolism. Science 306, 79–86. doi: 10.1126/science.1101156
Benitez-Nelson, C. R., and Buesseler, K. O. (1999). Variability of inorganic and organic phosphorus turnover rates in the coastal ocean. Nature 398, 502–505. doi: 10.1038/19061
Berg, J. S., Michellod, D., Pjevac, P., Martinez-Perez, C., Buckner, C. R. T., Hach, P. F., et al. (2016). Intensive cryptic microbial iron cycling in the low iron water column of the meromictic Lake Cadagno. Environ. Microbiol. 18, 5288–5302. doi: 10.1111/1462-2920.13587
Berland, B. R., Bonin, D. J., Maestrini, S. T., and Pointer, J. R. (1972). Growth-potential bioassay of sea waters using algal cultures I. Comparison of methods of estimation. Int. Rev. Hydrobiol. 58, 933–944. doi: 10.1002/iroh.19720570608
Beversdorf, L. J., White, A. E., Björkman, K. M., Letelier, R. M., and Karl, D. M. (2010). Phosphonate metabolism of Trichodesmium IMS101 and the production of greenhouse gases. Limnol. Oceanogr. 55, 1768–1778. doi: 10.4319/lo.2010.55.4.1768
Björkman, K., and Karl, D. M. (1994). Bioavailability of inorganic and organic phosphorus compounds to natural assemblages of microorganisms in Hawaiian coastal waters. Mar. Ecol. Prog. Ser. 111, 265–273.
Björkman, K. M., and Karl, D. M. (2005). Presence of dissolved nucleotides in the North Pacific Subtropical Gyre and their role in cycling of dissolved organic phosphorus. Aquat. Microb. Ecol. 39, 193–203. doi: 10.3354/ame039193
Canfield, D. E., Stewart, F. J., Thamdrup, B., De Brabandere, L., Dalsgaard, T., Delong, E. F., et al. (2010). A cryptic sulfur cycle in oxygen-minimum-zone waters off the Chilean coast. Science 330, 1375–1378. doi: 10.1126/science.1196889
Choi, S. H., Collins, J. N. R., Smith, S. A., Davis-Harrison, R. L., Rienstra, C. M., and Morrissey, J. H. (2010). Phosphoramidate end labeling of inorganic polyphosphates: facile manipulation of polyphosphate for modulating its biological activities. Biochemistry 49, 9935–9941. doi: 10.1021/bi1014437
Davis, C. E., and Mahaffey, C. (2017). Elevated alkaline phosphatase activity in a phosphate-replete environment: influence of sinking particles. Limnol. Oceanogr. 62, 2389–2403. doi: 10.1002/lno.10572
Davis, C. E., Mahaffey, C., Wolff, G. A., and Sharples, J. (2014). A storm in a shelf sea: variation in phosphorus distribution and organic matter stoichiometry. Geophys. Res. Lett. 41, 8452–8459. doi: 10.1002/2014gl061949
Diaz, J., Ingall, E., Benitez-Nelson, C., Paterson, D., de Jonge, M. D., McNulty, I., et al. (2008). Marine polyphosphate: a key player in geologic phosphorus sequestration. Science 320, 652–655. doi: 10.1126/science.1151751
Diaz, J. M., Björkman, K. M., Haley, S. T., Ingall, E. D., Karl, D. M., Longo, A. F., et al. (2016). Polyphosphate dynamics at station ALOHA, North Pacific subtropical gyre. Limnol. Oceanogr. 61, 227–239. doi: 10.1002/lno.10206
Duhamel, S., Björkman, K. M., Doggett, J. K., and Karl, D. M. (2014). Microbial response to enhanced phosphorus cycling in the North Pacific Subtropical Gyre. Mar. Ecol. Prog. Ser. 504, 43–58. doi: 10.3354/meps10757
Duhamel, S., Björkman, K. M., Repeta, D. J., and Karl, D. M. (2017). Phosphorus dynamics in biogeochemically distinct regions of the southeast subtropical Pacific Ocean. Prog. Oceanogr. 151, 261–274. doi: 10.1016/j.pocean.2016.12.007
Duhamel, S., Björkman, K. M., Van Wambeke, F., Moutin, T., and Karl, D. M. (2011). Characterization of alkaline phosphatase activity in the North and South Pacific Subtropical Gyres: implications for phosphorus cycling. Limnol. Oceanogr. 56, 1244–1254. doi: 10.4319/lo.2011.56.4.1244
Duhamel, S., Dyhrman, S. T., and Karl, D. M. (2010). Alkaline phosphatase activity and regulation in the North Pacific Subtropical Gyre. Limnol. Oceanogr. 55, 1414–1425. doi: 10.4319/lo.2010.55.3.1414
Dyhrman, S. T., Chappell, P. D., Haley, S. T., Moffett, J. W., Orchard, E. D., Waterbury, J. B., et al. (2006). Phosphonate utilization by the globally important marine diazotroph Trichodesmium. Nature 439, 68–71. doi: 10.1038/nature04203
Dyhrman, S. T., Jenkins, B. D., Rynearson, T. A., Saito, M. A., Mercier, M. L., Alexander, H., et al. (2012). The transcriptome and proteome of the diatom Thalassiosira pseudonana reveal a diverse phosphorus stress response. PLoS One 7:e33768. doi: 10.1371/journal.pone.0033768
Dyhrman, S. T., and Ruttenberg, K. C. (2006). Presence and regulation of alkaline phosphatase activity in eukaryotic phytoplankton from the coastal ocean: implications for dissolved organic phosphorus remineralization. Limnol. Oceanogr. 51, 1381–1390. doi: 10.4319/lo.2006.51.3.1381
Fahmy, M. (2003). Water quality in the Red Sea coastal waters (Egypt): analysis of spatial and temporal variability. Chem. Ecol. 19, 67–77. doi: 10.1080/0275754031000087074
Fisher, T. R., Gustafson, A. B., Sellner, K., Lacouture, R., Haas, L. W., Wetzel, R. L., et al. (1999). Spatial and temporal variation of resource limitation in Chesapeake Bay. Mar. Biol. 133, 763–778. doi: 10.1007/s002270050518
Fisher, T. R., Peele, E. R., Ammerman, J. W., and Harding, L. W. (1992). Nutrient limitation of phytoplankton in Chesapeake Bay. Mar. Ecol. Prog. Ser. 82, 51–63. doi: 10.3354/meps082051
Fu, M. Z., Wang, Z. L., Pu, X. M., Xu, Z. J., and Zhu, M. Y. (2012). Changes of nutrient concentrations and N:P:Si ratios and their possible impacts on the Huanghai Sea ecosystem. Acta Oceanol. Sin. 31, 101–112. doi: 10.1007/s13131-012-0224-x
Gray, M. J., and Jakob, U. (2015). Oxidative stress protection by polyphosphate — New roles for an old player. Curr. Opin. Microbiol. 24, 1–6. doi: 10.1016/j.mib.2014.12.004
Guillard, R. R. L., and Ryther, J. H. (1962). Studies of marine planktonic diatoms. I. Cyclotella nana hustedt and Detonula Confervacea cleve. Can. J. Microbiol. 8, 229–239. doi: 10.1139/m62-029
Hansel, C. M., Ferdelman, T. G., and Tebo, B. M. (2015). Cryptic cross-linkages among biogeochemical cycles: novel insights from reactive intermediates. Elements 11, 409–414. doi: 10.2113/gselements.11.6.409
Hansen, H. P., and Koroleff, F. (1999). “Determination of dissolved inorganic phosphate,” in Methods of Seawater Analysis, eds K. Grasshoff, K. Kremling, and M. Ehrhardt (New York, NY: Wiley), 170–174.
Hardison, D. R., Sunda, W. G., Shea, D., and Litaker, R. W. (2013). Increased toxicity of Karenia brevis during phosphate limited growth: ecological and evolutionary implications. PLoS One 8:e58545. doi: 10.1371/journal.pone.0058545
Harrison, P. J., Hu, M. H., Yang, Y. P., and Lu, X. (1990). Phosphate limitation in estuarine and coastal waters of China. J. Exp. Mar. Biol. Ecol. 140, 79–87. doi: 10.1016/0022-0981(90)90083-o
Harrison, W. G., Azam, F., Renger, E. H., and Eppley, R. W. (1977). Some experiments on phosphate assimilation by coastal marine plankton. Mar. Biol. 40, 9–18.
Hebbard, C. F. F., Wang, Y., Baker, C. J., and Morrissey, J. H. (2014). Synthesis and evaluation of chromogenic and fluorogenic substrates for high-throughput detection of enzymes that hydrolyze inorganic polyphosphate. Biomacromolecules 15, 3190–3196. doi: 10.1021/bm500872g
Hoppe, H. G. (2003). Phosphatase activity in the sea. Hydrobiologia 493, 187–200. doi: 10.1023/a:1025453918247
Hoppe, H. G., and Ullrich, S. (1999). Profiles of ectoenzymes in the Indian Ocean: phenomena of phosphatase activity in the mesopelagic zone. Aquat. Microb. Ecol. 19, 139–148. doi: 10.3354/ame019139
Huang, R., Wan, B., Hultz, M., Diaz, J. M., and Tang, Y. (2018). Phosphatase-mediated hydrolysis of linear polyphosphates. Environ. Sci. Technol. 52, 1183–1190. doi: 10.1021/acs.est.7b04553
Huang, X. P., Huang, L. M., and Yue, W. Z. (2003). The characteristics of nutrients and eutrophication in the Pearl River estuary, South China. Mar. Pollut. Bull. 47, 30–36. doi: 10.1016/s0025-326x(02)00474-5
Ilikchyan, I. N., McKay, R. M. L., Zehr, J. P., Dyhrman, S. T., and Bullerjahn, G. S. (2009). Detection and expression of the phosphonate transporter gene phnD in marine and freshwater picocyanobacteria. Environ. Microbiol. 11, 1314–1324. doi: 10.1111/j.1462-2920.2009.01869.x
Karl, D. M. (2014). Microbially mediated transformations of phosphorus in the sea: new views of an old cycle. Annu. Rev. Mar. Sci. 6, 279–337. doi: 10.1146/annurev-marine-010213-135046
Karlson, B., Cusak, C., and Bresnan, E. (2010). Microscopic and Molecular Methods for Quantitative Phytoplankton Analysis. Paris: UNESCO.
Kathuria, S., and Martiny, A. C. (2010). Prevalence of a calcium-based alkaline phosphatase associated with the marine cyanobacterium Prochlorococcus and other ocean bacteria. Environ. Microbiol. 13, 74–83. doi: 10.1111/j.1462-2920.2010.02310.x
Kemp, W. M., Boynton, W. R., Adolf, J. E., Boesch, D. F., Boicourt, W. C., Brush, G., et al. (2005). Eutrophication of Chesapeake Bay: historical trends and ecological interactions. Mar. Ecol. Prog. Ser. 303, 1–29. doi: 10.3354/meps303001
Kim, I.-N., Lee, K., Gruber, N., Karl, D. M., Bullister, J. L., Yang, S., et al. (2014). Increasing anthropogenic nitrogen in the North Pacific Ocean. Science 346, 1102–1106. doi: 10.1126/science.1258396
Kornberg, A., Rao, N. N., and Ault-Riche, D. (1999). Inorganic polyphosphate: a molecule of many functions. Annu. Rev. Biochem. 68, 89–125. doi: 10.1146/annurev.biochem.68.1.89
Laurent, A., Fennel, K., Hu, J., and Hetland, R. (2012). Simulating the effects of phosphorus limitation in the Mississippi and Atchafalaya River plumes. Biogeosciences 9, 4707–4723. doi: 10.5194/bg-9-4707-2012
Letscher, R. T., and Moore, J. K. (2015). Preferential remineralization of dissolved organic phosphorus and non-Redfield DOM dynamics in the global ocean: impacts on marine productivity, nitrogen fixation, and carbon export. Global Biogeochem. Cycles 29, 325–340. doi: 10.1002/2014GB004904
Li, H., Veldhuis, M. J. W., and Post, A. F. (1998). Alkaline phosphatase activities among planktonic communities in the northern Red Sea. Mar. Ecol. Prog. Ser. 173, 107–115. doi: 10.3354/meps173107
Lin, H. Y., Shih, C. Y., Liu, H. C., Chang, J., Chen, Y. L., Chen, Y. R., et al. (2013). Identification and characterization of an extracellular alkaline phosphatase in the marine diatom Phaeodactylum tricornutum. Mar. Biotechnol. 15, 425–436. doi: 10.1007/s10126-013-9494-3
Lin, S. J., Litaker, R. W., and Sunda, W. G. (2016). Phosphorus physiological ecology and molecular mechanisms in marine phytoplankton. J. Phycol. 52, 10–36. doi: 10.1111/jpy.12365
Lin, X., Wang, L., Shi, X. G., and Lin, S. J. (2015). Rapidly diverging evolution of an atypical alkaline phosphatase (PhoA(aty)) in marine phytoplankton: insights from dinoflagellate alkaline phosphatases. Front. Microbiol. 6:868. doi: 10.3389/fmicb.2015.00868
Lin, X., Zhang, H., Huang, B. Q., and Lin, S. J. (2011). Alkaline phosphatase gene sequence and transcriptional regulation by phosphate limitation in Amphidinium carterae (Dinophyceae). J. Phycol. 47, 1110–1120. doi: 10.1111/j.1529-8817.2011.01038.x
Lin, X., Zhang, H., Huang, B. Q., and Lin, S. J. (2012). Alkaline phosphatase gene sequence characteristics and transcriptional regulation by phosphate limitation in Karenia brevis (Dinophyceae). Harmful Algae 17, 14–24. doi: 10.1016/j.hal.2012.02.005
Lomas, M. W., Burke, A. L., Lomas, D. A., Bell, D. W., Shen, C., Dyhrman, S. T., et al. (2010). Sargasso Sea phosphorus biogeochemistry: an important role for dissolved organic phosphorus (DOP). Biogeosciences 7, 695–710. doi: 10.5194/bg-7-695-2010
Lommer, M., Specht, M., Roy, A. S., Kraemer, L., Andreson, R., Gutowska, M. A., et al. (2012). Genome and low-iron response of an oceanic diatom adapted to chronic iron limitation. Genome Biol. 13:R66. doi: 10.1186/gb-2012-13-7-r66
Lorenz, B., and Schroder, H. C. (2001). Mammalian intestinal alkaline phosphatase acts as highly active exopolyphosphatase. Biochim. Biophys. Acta 1547, 254–261. doi: 10.1016/S0167-4838(01)00193-5
Luo, H. W., Benner, R., Long, R. A., and Hu, J. J. (2009). Subcellular localization of marine bacterial alkaline phosphatases. Proc. Nat. Acad. Sci. U.S.A. 106, 21219–21223. doi: 10.1073/pnas.0907586106
Luo, H. W., Zhang, H. M., Long, R. A., and Benner, R. (2011). Depth distributions of alkaline phosphatase and phosphonate utilization genes in the North Pacific Subtropical Gyre. Aquat. Microb. Ecol. 62, 61–69. doi: 10.3354/ame01458
Mahaffey, C., Reynolds, S., Davis, C. E., and Lohan, M. C. (2014). Alkaline phosphatase activity in the subtropical ocean: insights from nutrient, dust and trace metal addition experiments. Front. Mar. Sci. 1:73. doi: 10.3389/fmars.2014.00073
Marchetti, A., Catlett, D., Hopkinson, B. M., Ellis, K., and Cassar, N. (2015). Marine diatom proteorhodopsins and their potential role in coping with low iron availability. ISME J. 9, 2745–2748. doi: 10.1038/ismej.2015.74
Marchetti, A., Schruth, D. M., Durkin, C. A., Parker, M. S., Kodner, R. B., Berthiaume, C. T., et al. (2012). Comparative metatranscriptomics identifies molecular bases for the physiological responses of phytoplankton to varying iron availability. Proc. Nat. Acad. Sci. U.S.A. 109, E317–E325. doi: 10.1073/pnas.1118408109
Martin, P., Dyhrman, S. T., Lomas, M. W., Poulton, N. J., and Van Mooy, B. A. S. (2014). Accumulation and enhanced cycling of polyphosphate by Sargasso Sea plankton in response to low phosphorus. Proc. Nat. Acad. Sci. U.S.A. 111, 8089–8094. doi: 10.1073/pnas.1321719111
Martinez, A., Tyson, G. W., and DeLong, E. F. (2010). Widespread known and novel phosphonate utilization pathways in marine bacteria revealed by functional screening and metagenomic analyses. Environ. Microbiol. 12, 222–238. doi: 10.1111/j.1462-2920.2009.02062.x
Mazard, S., Wilson, W. H., and Scanlan, D. J. (2012). Dissecting the physiological response to phosphorus stress in marine Synechococcus isolates. J. Phycol. 48, 94–105. doi: 10.1111/j.1529-8817.2011.01089.x
Michelou, V. K., Lomas, M. W., and Kirchman, D. L. (2011). Phosphate and adenosine-5 ’-triphosphate uptake by cyanobacteria and heterotrophic bacteria in the Sargasso Sea. Limnol. Oceanogr. 56, 323–332. doi: 10.4319/lo.2011.56.1.0323
Moore, C. M., Mills, M. M., Arrigo, K. R., Berman-Frank, I., Bopp, L., Boyd, P. W., et al. (2013). Processes and patterns of oceanic nutrient limitation. Nat. Geosci. 6, 701–710. doi: 10.1038/ngeo1765
Moore, C. M., Mills, M. M., Langlois, R., Milne, A., Achterberg, E. P., La Roche, J., et al. (2008). Relative influence of nitrogen and phosphorus availability on phytoplankton physiology and productivity in the oligotrophic sub-tropical North Atlantic Ocean. Limnol. Oceanogr. 53, 291–305. doi: 10.4319/lo.2008.53.1.0291
Moore, L. R., Ostrowski, M., Scanlan, D. J., Feren, K., and Sweetsir, T. (2005). Ecotypic variation in phosphorus acquisition mechanisms within marine picocyanobacteria. Aquat. Microb. Ecol. 39, 257–269. doi: 10.3354/ame039257
Nicholson, D., Dyhrman, S., Chavez, F., and Paytan, A. (2006). Alkaline phosphatase activity in the phytoplankton communities of Monterey Bay and San Francisco Bay. Limnol. Oceanogr. 51, 874–883. doi: 10.4319/lo.2006.51.2.0874
Oudot-Le Secq, M.-P., Grimwood, J., Shapiro, H., Armbrust, E. V., Bowler, C., and Green, B. R. (2007). Chloroplast genomes of the diatoms Phaeodactylum tricornutum and Thalassiosira pseudonana: comparison with other plastid genomes of the red lineage. Mol. Genet. Genomics 277, 427–439. doi: 10.1007/s00438-006-0199-4
Repeta, D. J., Ferrón, S., Sosa, O. A., Johnson, C. G., Repeta, L. D., Acker, M., et al. (2016). Marine methane paradox explained by bacterial degradation of dissolved organic matter. Nat. Geosci. 9, 884–887. doi: 10.1038/NGEO2837
Rivkin, R. B., and Swift, E. (1979). Diel and vertical patterns of alkaline phosphatase activity in the oceanic dinoflagellate Pyrocystis noctiluca. Limnol. Oceanogr. 24, 107–116. doi: 10.4319/lo.1979.24.1.0107
Ruttenberg, K. C. (2014). “The global phosphorus cycle,” in Treatise on Geochemistry: Biogeochemistry, Vol. 10, eds D. M. Karl and W. H. Schlesinger (Amsterdam: Elsevier), 499–588.
Saad, E. M., Longo, A. F., Chambers, L. R., Huang, R., Benitez-Nelson, C., Dyhrman, S. T., et al. (2016). Understanding marine dissolved organic matter production: compositional insights from axenic cultures of Thalassiosira pseudonana. Limnol. Oceanogr. 61, 2222–2233. doi: 10.1002/lno.10367
Sanudo-Wilhelmy, S. A., Tovar-Sanchez, A., Fu, F. X., Capone, D. G., Carpenter, E. J., and Hutchins, D. A. (2004). The impact of surface-adsorbed phosphorus on phytoplankton Redfield stoichiometry. Nature 432, 897–901. doi: 10.1038/nature03125
Schulz, H. N., and Schulz, H. D. (2005). Large sulfur bacteria and the formation of phosphorite. Science 307, 416–418. doi: 10.1126/science.1103096
Sebastian, M., and Ammerman, J. W. (2009). The alkaline phosphatase PhoX is more widely distributed in marine bacteria than the classical PhoA. ISME J. 3, 563–572. doi: 10.1038/ismej.2009.10
Shaked, Y., Xu, Y., Leblanc, K., and Morel, F. M. M. (2006). Zinc availability and alkaline phosphatase activity in Emiliania huxleyi: implications for Zn-P co-limitation in the ocean. Limnol. Oceanogr. 51, 299–309. doi: 10.4319/lo.2006.51.1.0299
Strickland, J. D. H., and Parsons, T. R. (1972). A Practical Handbook Of Seawater Analysis. Ottawa, ON: Fisheries Research Board of Canada.
Thingstad, T. F., Krom, M. D., Mantoura, R. F. C., Flaten, G. A. F., Groom, S., Herut, B., et al. (2005). Nature of phosphorus limitation in the ultraoligotrophic eastern Mediterranean. Science 309, 1068–1071. doi: 10.1126/science.1112632
Turner, R. E., and Rabalais, N. N. (2013). Nitrogen and phosphorus phytoplankton growth limitation in the northern Gulf of Mexico. Aquat. Microb. Ecol. 68, 159–169. doi: 10.3354/ame01607
Wanner, B. L., and McSharry, R. (1982). Phosphate-controlled gene expression in Escherichia coli K12 using Mudl-directed lacZ fusions. J. Mol. Biol. 158, 347–363. doi: 10.1016/0022-2836(82)90202-9
White, A. E., Watkins-Brandt, K. S., Engle, M. A., Burkhardt, B., and Paytan, A. (2012). Characterization of the rate and temperature sensitivities of bacterial remineralization of dissolved organic phosphorus compounds by natural populations. Front. Microbiol. 3:276. doi: 10.3389/fmicb.2012.00276
Wilkins, A. S. (1972). Physiological factors in the regulation of alkaline phosphatase synthesis in Escherichia coli. J. Bacteriol. 110, 616–623.
Wu, J. F., Sunda, W., Boyle, E. A., and Karl, D. M. (2000). Phosphate depletion in the western North Atlantic Ocean. Science 289, 759–762. doi: 10.1126/science.289.5480.759
Xu, J., Yin, K. D., He, L., Yuan, X. C., Ho, A. Y. T., and Harrison, P. J. (2008). Phosphorus limitation in the northern South China Sea during late summer: influence of the Pearl River. Deep Sea Res. I Oceanogr. Res. Pap. 55, 1330–1342. doi: 10.1016/j.dsr.2008.05.007
Xu, Y., Boucher, J. M., and Morel, F. M. M. (2010). Expression and diversity of alkaline phosphatase ehap1 in Emiliania huxleyi (Prymnesiophyceae). J. Phycol. 46, 85–92. doi: 10.1111/j.1529-8817.2009.00788.x
Xu, Y., Wahlund, T. M., Feng, L., Shaked, Y., and Morel, F. M. M. (2006). A novel alkaline phosphatase in the coccolithophore Emiliania huxleyi (Prymnesiophyceae) and its regulation by phosphorus. J. Phycol. 42, 835–844. doi: 10.1111/j.1529-8817.2006.00243.x
Young, C. L., and Ingall, E. D. (2010). Marine dissolved organic phosphorus composition: insights from samples recovered using combined electrodialysis/reverse osmosis. Aquat. Geochem. 16, 563–574. doi: 10.1007/s10498-009-9087-y
Keywords: dissolved organic phosphorus, alkaline phosphatase activity, polyphosphate, phosphoester, phosphoanhydride, phosphorus stress, Thalassiosira, diatom
Citation: Diaz JM, Holland A, Sanders JG, Bulski K, Mollett D, Chou C-W, Phillips D, Tang Y and Duhamel S (2018) Dissolved Organic Phosphorus Utilization by Phytoplankton Reveals Preferential Degradation of Polyphosphates Over Phosphomonoesters. Front. Mar. Sci. 5:380. doi: 10.3389/fmars.2018.00380
Received: 13 May 2018; Accepted: 28 September 2018;
Published: 25 October 2018.
Edited by:
Wolfgang Koeve, GEOMAR Helmholtz-Zentrum für Ozeanforschung Kiel, Helmholtz-Gemeinschaft Deutscher Forschungszentren (HZ), GermanyReviewed by:
Michael William Lomas, Bigelow Laboratory for Ocean Sciences, United StatesMonika Nausch, Leibniz Institute for Baltic Sea Research (LG), Germany
Copyright © 2018 Diaz, Holland, Sanders, Bulski, Mollett, Chou, Phillips, Tang and Duhamel. This is an open-access article distributed under the terms of the Creative Commons Attribution License (CC BY). The use, distribution or reproduction in other forums is permitted, provided the original author(s) and the copyright owner(s) are credited and that the original publication in this journal is cited, in accordance with accepted academic practice. No use, distribution or reproduction is permitted which does not comply with these terms.
*Correspondence: Julia M. Diaz, anVsaWEuZGlhekBza2lvLnVnYS5lZHU=