- 1National Institute of Water and Atmospheric Research, Hamilton, New Zealand
- 2National Institute of Water and Atmospheric Research, Auckland, New Zealand
- 3Institute of Marine Science, University of Auckland, Auckland, New Zealand
Nursery habitats provide increased survival and growth and are a crucial early life-stage component for many fish and invertebrate populations. The biogenic structures that provide this nursery function, however, are increasingly degraded. Therefore, any effort to conserve, restore or replace habitat with artificial structure should be guided by an understanding of the value provided by that nursery habitat. Here, we experimentally manipulated structure across a number of sites by inserting pinnind bivalve mimics into the seabed and deploying video cameras to observe the response of post-settlement stage snapper, Chrysophrys auratus (Forster in Bloch and Schneider 1801). We also collected a range of environmental variables across these sites to determine the relative importance to snapper of benthic vs. pelagic productivity. While the abundance of snapper was low, our results demonstrated a strong association to structure relative to control plots. The environmental variable with the highest correlation to snapper abundance was the abundance of zooplankton eaten by snapper. This result was well supported by the dominance of zooplankton over small benthic invertebrates in snapper gut contents, and the weak influence of benthic infauna in our regression models. These regressions also demonstrated that when combined with zooplankton abundance, turbidity had a negative relationship to snapper abundance. This highlights the importance of relatively clear water in estuaries, which allows post-settlement snapper to more efficiently consume the zooplankton that are present in the water column. The third component that post-settlement snapper require is of course the presence of benthic structure. While benthic habitat structure was the strongest factor affecting juvenile snapper abundance, we did not find any correlations to suggest that this importance was related to energetic sheltering and access to locations with high food flux.
Introduction
Nursery habitats are a critical requirement for a number of fish species with separate juvenile and adult life stages (Beck et al., 2001; Heck et al., 2003; Dahlgren et al., 2006). Protection from predation and food availability are the most likely explanations for this juvenile habitat association (Heck et al., 2003). Given the obligate nature of the nursery habitat association and the often degraded state of the benthic habitats that provide it (e.g., Orth et al., 2006), conserving, restoring, or providing artificial habitat structure to ensure that juvenile life stage requirements are satisfied is an important consideration for the maintenance of adult fish populations and the services that they provide (Townsend et al., 2014). Understanding the environmental factors that drive the abundance of nursery habitat occupying juvenile fish, while not the entire picture (Beck et al., 2001), is an important consideration for habitat and fishery management.
Snapper, Chrysophrys auratus, (= Pagrusauratus) (Perciformes–Sparidae), are a recreationally and commercially important fish species that are abundant in the coastal waters of northern New Zealand (Parsons et al., 2014). Post-settlement stage snapper [ < 60 mm Fork Length (FL)] occupy shallow estuarine locations for a few months after settling over summer and/or autumn before dispersing to a range of habitats/locations. High abundances of post-settlement stage snapper are usually associated with habitat structure, whereas immediately adjacent bare sediment sites are usually unoccupied (Parsons et al., 2013, 2016). Because interactions between post-settlement snapper and predators have rarely been observed, an alternative explanation to describe their affinity to structure has been developed. This theory relates to energetic sheltering benefits that structure could provide at sites which have high water velocity, but also a high associated flux of the pelagic zooplankton food that post-settlement snapper prefer (Parsons et al., 2015, 2018). In more turbid estuaries this theory may not apply, as visual feeding is more difficult, and benthic prey (which don't have a flux) dominate post-settlement snapper diet (Lowe et al., 2015).
In the present study we conducted a controlled structure manipulation experiment as a means of understanding the response of post-settlement snapper to structure across a number of sites. We also collected a range of environmental and habitat variables from each of these sites, so we could understand how snapper abundance and response to structure was influenced by these variables. Of primary interest was the relative importance of benthic vs. pelagic variables, as the estuary we conducted this experiment within was relatively turbid (Lowe et al., 2015), suggesting that either benthic or pelagic productivity could be important.
Materials and Methods
Study Site and Overall Sampling Approach
Sampling was conducted within Mahurangi Harbor, northeastern New Zealand (Figure 1). Mahurangi Harbor is a drowned river valley with an area of c. 24.5 km2 (Feeney and Challis, 1984). While Mahurangi Harbor is relatively turbid and potentially a lower value habitat for juvenile snapper compared to some other estuaries within northeastern New Zealand (Lowe et al., 2015), it still hosts a large population of juvenile snapper (Morrison and Carbines, 2006) and offers one of the largest estuarine habitat areas to juvenile snapper within the Hauraki Gulf, the location of New Zealand's largest snapper population (Parsons et al., 2014).
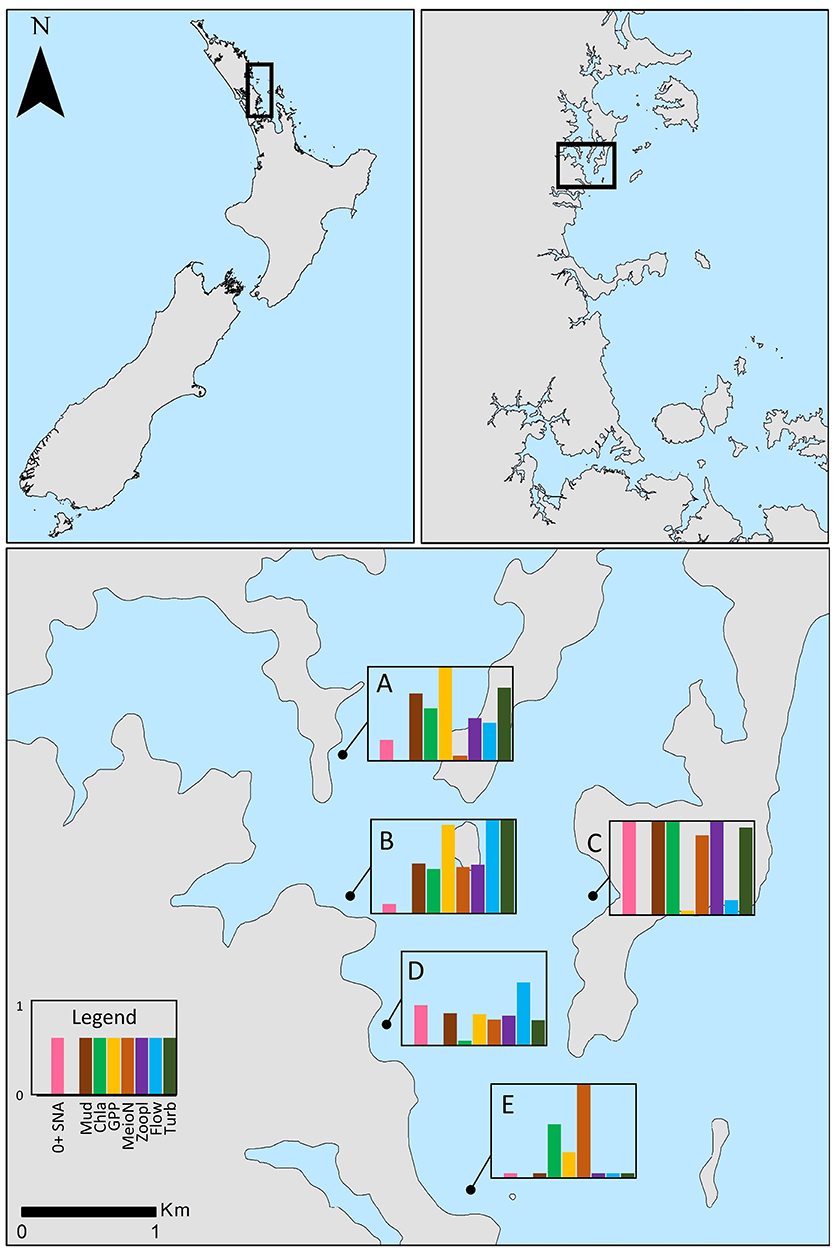
Figure 1. New Zealand (upper left) and the region north of Auckland (upper right) where the study was conducted. Bottom panel shows the positions of the five study sites in Mahurangi Harbor. Water depth at high tide was 5–8 m at all sites; only a small portion of the main harbor channel near the mouth is >10 m water depth. Bar graphs depict the relative importance of habitat structure to post-settlement snapper (pink bars = abundance of snapper amongst structure minus abundance in control plots, SNA diff) and the relative magnitudes of selected environmental variables (see legend) across sites. All variables were standardized to run from 0 to 1 for ease of comparison. Mud, sediment mud content (%); Chla, sediment chlorophyll a content (μg g−1); GPP, gross primary production (μmol O2 m−2 h−1); MeioN, abundance of meiofauna per core (ostracods, nematodes, and benthic copepods); Zoopl, abundance of zooplankton that dominate post-settlement snapper diet found in bottom water; Flow, average current velocity (m s−1); Turb, light attenuation coefficient, Kd, a proxy for turbidity.
Different components of sampling were conducted over an almost 3 year period (March 2015 to December 2017), with most sampling events taking place during March, the time period when the post-settlement juvenile snapper occupy sheltered inshore estuaries and harbors such at the Mahurangi (Parsons et al., 2014). Our approach centered around the quantification of post-settlement snapper abundance at five sites, all 5–8 m water depth,within Mahurangi Harbor (Figure 1: Site A: −36.4848°S, 174.7165°E, Site B: −36.4910°S, 174.7174°E; Site C: −36.4942°S, 174.7346°E, Site D: −36.5044°S, 174.7229°E; Site E: −36.51282°S, 174.7286°E) and relating this to environmental variables at those same sites. We implemented a structure manipulation experiment at these sites to aid in the quantification of post-settlement snapper abundance and to determine the importance of structure to post-settlement snapper. Animal ethics approval was not required for this study as per the local legislation. All sampling was conducted during daytime hours.
Structure Manipulation Experiment and Post-Settlement Snapper Abundance
To assess the effect of structure on post-settlement snapper abundance we created patches of high structure availability at five sites within Mahurangi Harbor (Figure 1). The structure element we used to create these patches was intended to mimic the valves of the pinnid bivalve Atrina zelandica, which protrude up to 25 cm above the sediment surface, can form extensive biogenic reefs (Cummings et al., 1998), and have been found associated with high abundances of post-settlement snapper at other locations (Parsons et al., 2016). Atrina mimics consisted of triangular segments cut from 100 mm diameter polyvinyl chloride (PVC) pipe with approximate dimensions of 40 cm high by 12 cm wide. In December 2015 (the time of peak snapper spawning, Parsons et al., 2014) divers created patches of Atrina mimics at each site by marking out a 2 × 2 m area with a quadrat and then haphazardly pushing 25 Atrina mimics into the sediment within the quadrat so that c. 10–15 cm of each mimic protruded above the sediment. Divers then measured 15 m from that patch before repeating this process so that n = 3 Atrina mimic patches were created over a 30 m section of seabed. Following identical spacing, n = 3 bare sediment control plots were identified over the next 30 m of seabed, but were unmarked. Previous investigations have demonstrated that post-settlement snapper are resident to patches separated by c. 10 m (Parsons et al., 2013), so 15 m spacing was adequate for us to treat each patch within a site as independent.
The patches of Atrina mimics were left in place until March 2016, the time of year when post-settlement snapper abundance should be at its peak (Parsons et al., 2014). At this time we quantified the fish communities associated with Atrina mimics (and adjacent control plots) by simultaneously deploying six video cameras at each site. We used GoPro Hero 3 or Hero 4 cameras mounted on 15 cm high steel stands, which divers deployed facing each Atrina mimic patch or bare sediment control plot. Cameras were positioned 1 m from the edge of Atrina patches or control plots (the diver would measure 1 m from the camera to indicate where the control plot edge was for the video observer). Divers also took sediment cores (n = 3; see more detailed description below) immediately adjacent to Atrina patches and control plots to quantify sediment grain size, organic matter content, pigment content and invertebrate community characteristics. Cameras were left to run for a minimum of 60 min before being retrieved via a surface float.
Video Analysis
The first aspect of video analysis was to determine if water visibility was adequate to enumerate fish abundance. For all video replicates water visibility was ≥1 m, enabling a clear view of fish over the Atrina mimics or control plots. Next, post-settlement snapper abundance was assessed using a subsampling procedure similar to that of Schobernd et al. (2014). Here, 10 random times were selected within the hour of video footage obtained from each camera deployment (excluding the first 10 min to remove the potential of diver disturbance). At each of these times, 2 min of video footage was observed (ensuring that the random times selected were ≥2 min apart), counting the maximum number of post-settlement snapper observed at one time. The average of these counts (Avg SNA) was then used to represent snapper abundance for each video replicate. Only snapper that were around the nearest edge of the Atrina mimic patch or closer to the camera were counted. For bare sediment sites, a similar depth of field was estimated from footage of the diver making measurements while the camera was set in place. In terms of distinguishing between post-settlement and older aged snapper, it is important to note that this survey was conducted during March. Therefore, post-settlement snapper should be c. 6 cm or c. 100% smaller in length than the next oldest age class (1 + snapper) (Francis, 1994), providing adequate resolution to determine which snapper belonged to the post-settlement and ≥1+ age classes.
Environmental Variables
Benthic Productivity
Incubation chambers were deployed to the seabed at the five sites and sampled by SCUBA divers on two consecutive days during March 2015. A 20 m seabed transect was established at each site, and seven pairs of light and dark chambers were deployed at random positions along each transects length. The chambers (8 cm internal diameter polycarbonate plastic domes with sampling ports) were used to enclose small patches of unmanipulated soft-sediment habitat (0.016 m2) together with 0.85 L of overlying water. The enclosed chamber waters were sampled initially (one 60 ml water sample collected per chamber at T = 0) and again 2–4 h later (i.e., a final sampling at T = end). Dissolved oxygen concentration was measured using a handheld oxygen probe (YSI ProODO) and samples were filtered through a glass fiber filter (Whatman GF/C, pore size 1.2 μm) for later analysis of dissolved inorganic nutrients using standard methods for seawater on an Astoria-Pacific 300 series segmented flow auto-analyser with detection limits of 1 mg m−3 for N and P. Changes in dissolved oxygen during the incubation were used to assess rates of microphytobenthic productivity, with the light chambers providing information on net photosynthetic oxygen production in the presence of sunlight, and dark chambers providing information on total community oxygen utilization. Fluxes of dissolved inorganic nutrients (nitrate-plus-nitrite nitrogen, ammonium nitrogen, and dissolved reactive phosphorus) were calculated to assess the influence of photosynthetic uptake on rates of nutrient exchange from sediment to water column. Fluxes of dissolved oxygen and inorganic nutrients were calculated as (CT = end-CT = 0) × V/(A × T), where C was the solute concentration at the start or end of the incubation, V was the volume of water enclosed in the chamber (constant), A was the area of sediment enclosed (constant), and T was the elapsed time between initial and final water samplings.
Benthic Infauna and Sediment Characteristics
In March of 2015 and 2016, benthic soft-sediment habitat characteristics were assessed by SCUBA divers at the five study sites. On the same days as the chamber incubations (March 2015, described above), 20 small cores of sediment (3 cm internal diameter) were collected along a 20 m seabed transect at each site. Sediment particle size distribution and organic matter content in the top 2 cm of sediment was assessed from five replicate sediment cores site−1 time−1; sediment pigment concentrations in the top 2 cm of sediment (chlorophyll a and pheophytin) were also assessed from five replicate cores site−1 time−1; identities and abundances of sediment invertebrates were quantified from five replicate samples site−1 time−1, with each sediment invertebrate sample comprised of two amalgamated 3 cm internal diameter × 5 cm deep cores.
Sediment grain size, organic matter content and pigment samples were kept frozen and in the dark until analysis and processed according to standard protocols (Gatehouse, 1971; Mook and Hoskin, 1982; Sartory, 1982; Lohrer et al., 2012). Briefly, sediment organic matter content was determined from the change in sediment dry weight (%) after combustion in a muffle furnace at 400°C for 6 h. Sediment chlorophyll a content (Chla) was determined spectrophotometrically after extracting pigments from sediments by boiling in ethanol and using an acidification step (addition of 1 drop of 1 M HCl to sample cuvette) to separate degradation products (e.g., phaeophytin) from Chla. Sediment particle size distribution (% dry weight of different grain size categories) was determined after wet sieving samples across 2,000, 1,000, 500, 250, and 63 μm mesh screens, and by pipetting to differentiate silt (3.9–63 μm) and clay (< 3.9 μm). We operationally define particles < 63 μm (i.e., silt + clay) as “mud”.
The sediment invertebrate samples were sieved in the field across a 125 μm mesh screen and preserved in 70% isopropyl alcohol until processing. At the laboratory, a red stain (Rose Bengal) was added to the isopropanol solution to facilitate sorting, and all invertebrates per sample (including nematodes, ostracods, and copepods) were identified to the lowest practicable taxonomic level possible under a dissecting microscope (Leica M80, 60 × main magnification with 10 × magnification binocular eye pieces) and given the condition of the items in the samples. During March 2016, sediment grain size, organic matter content, pigment content and invertebrate community characteristics were again evaluated using identical methodologies, except that 3 replicate samples were collected immediately adjacent to Atrina mimic patches and 3 samples were collected from nearby control plots at each site (see above).
Zooplankton
Zooplankton were sampled to describe the potential diet items of post-settlement snapper that may occur within the water column. Sampling was undertaken during the daytime on five different days, at the five sites between December 2015 and March 2016 (this encompasses the seasonal period when post-settlement snapper are present within estuarine waters such as Mahurangi Harbor). Water samples were collected with a plankton pump that consisted of a petrol powered impellor pump connected to a 6.5 cm diameter hose that was long enough to reach the seabed. The hose was lowered to the seabed and held in place with an attached metal pole. A 50 cm long metal arm protruded below the end of the hose. This ensured that the water sample was always taken at a consistent elevation above the seabed. We chose an elevation of 50 cm as we deemed this would allow us to obtain near seabed water samples, which is where post-settlement snapper feeding is likely to occur. The pump was then run until a 1200 l water sample had passed through a 250 μm sieve (measured by repetitively filling a 20 l bucket). Samples were washed off the sieve and fixed in a 10% formalin solution, and then drained and preserved in 70% ethanol within a week. All invertebrates were then sorted, counted, and identified down to the lowest practical taxonomic level possible under a dissecting microscope and given the condition of the items in the samples.
Water Flow and Light Attenuation
Water current velocities were simultaneously measured at the five sites (Figure 1) during December 2017 using five Nortek 2 MHz Aquadopp's [Acoustic Doppler Current Profilers (ADCPs)]. ADCPs were deployed on the sea bed facing the water surface in a bottom mounted upward-looking configuration. ADCP acoustic transducers were nominally positioned between 0.2 and 0.35 m above the sea bed. ADCP data were collected in bursts with an interval between bursts of 10 min. Water flow at each of the sites was measured over the same period, which encompassed approximately two complete tidal cycles. Summary statistics of the burst-averaged horizontal current speed in the bin closest to the sea bed were used in subsequent analysis.
Along with each current meter, an Odyssey PAR (photosynthetically active radiation) sensor was deployed to the seabed at each site to gather information on the light available to microphytobenthic primary producers. One PAR logger was also deployed in air to capture data on incident sea surface sunlight radiation. Based on water depth and differences between incident and seabed PAR levels, light extinction coefficients, Kd, were calculated for each site. Kd is a proxy for water column turbidity, as light penetration diminishes quickly with depth in turbid water relative to clear water.
Post-Settlement Snapper Diet
In addition to the environmental variables described above we wanted to describe post-settlement snapper diet, which may guide us in identifying particular benthic infauna or zooplankton taxa that may be important variables. Over several days during March 2015 and March 2016 we deployed opera house fish traps (Morrison and Carbines, 2006, length: 100 cm, width: 60 cm, height: 60 cm; 1 cm mesh) to capture post-settlement snapper to assess their diet. Multiple traps were deployed at each site, with some baited (using chopped up pilchard, Sardinops neopilchardus, as bait) and others un-baited. Traps would be deployed for a period of up to several hours before being checked and re-deployed. In 2016 traps were only deployed after video deployments for that site had been completed. All juvenile snapper captured were immediately euthanized before administering an injection of a 10% formalin solution (salt water buffered) into the gut cavity and then immersed into the same solution. Gut contents were later obtained by making a ventral incision, removing and opening the fore and hindgut and washing the sample into a vial using a 70% ethanol mixture. All invertebrates were then sorted, counted and identified down to the lowest practical taxonomic level.
Statistical Analyses
Benthic infauna community data from 2016 were visualized using non-metric multidimensional scaling (nMDS) and the effects of site (five levels, random factor) and treatment (i.e., structure vs. control, two levels, fixed factor) on benthic infauna community data were tested in a two-way non-parametric permutational analysis of variance PERMANOVA, PRIMER 7.0.13. The significance of the site × treatment interaction term was also assessed, and post-hoc pairwise comparisons were made when required to address specific hypotheses (e.g., significance of structure vs. control within sites). Identical analyses were also conducted for the meiofauna component (i.e., sediment dwelling ostracods, nematodes, and harpacticoid copepods), and sediment chlorophyll a content. Because benthic infauna communities in 2016 did not differ by treatment (i.e., structure vs. control), data from all six cores per site collected in 2016 were combined and compared to the 2015 data in a similar analysis (but with a fixed factor for “year” with two levels i.e., 2015 vs. 2016).
Next we assessed the effect of additional structure on post-settlement snapper. The primary response variable of interest was the abundance of post-settlement snapper (Avg SNA), with three replicate data points per site from Atrina mimics (i.e., structure), and also from adjacent control plots. Cameras failed on two occasions (i.e., two lost observations of 30 total), but the data set was otherwise well balanced. The effects of site (5 levels) and treatment (structure vs. control) on post-settlement snapper abundance were considered as random and fixed factors, respectively, in a two-way non-parametric permutational analysis of variance (PERMANOVA, PRIMER 7.0.13). The significance of the site × treatment interaction term was also assessed, and post-hoc pairwise comparisons were made when required to address specific hypotheses (e.g., significance of structure vs. control within sites).
The final part of our analysis was to relate post-settlement snapper abundance to all the ancillary environmental data collected. Our approach was to average each variable by site, generating a single independent value for each variable at each of the five sites. For snapper, the response variables used were the total of the Avg SNA observations from all replicates within a site regardless of treatment, and the difference in Avg SNA between a site's Atrina mimic and control replicates. These new variables were termed “total SNA” and “SNA diff,” respectively, with the latter indicating the relative importance of structure to post-settlement snapper at each of the sites. We related the patterns in these response variables to each site's combination of environmental variables using draftsman's plots and the RELATE and BEST procedures in PRIMER 7.0.13. Finally, regression models with a maximum of two independent explanatory variables were used to find the combination of environmental variables that best predicted the response of post-settlement snapper to structure (based on Akaike's Information Criterion, p-values, and adjusted r2 values). Models with more than two independent variables generally suffered from multi-collinearity (variance inflation) and overfitting. All explanatory variables were standardized to run between 0 and 1 prior to inclusion in the models, so that the relative strengths of the contributing variables could be evaluated by comparing the magnitudes of the coefficients.
Results
General Description of Environmental Variables Across Sites
The five sites sampled in the harbor differed significantly in their environmental characteristics. The inner estuary sites A, B, and C had muddy sediments, high sediment organic matter content, and high water column turbidity, relative to sites D and E, which were located closer to the mouth (Figure 1). Site B, at the confluence of Pukapuka Inlet and the main harbor channel, had the fastest average tidal current speed, with nearest neighboring sites D and A next fastest. All sites were net autotrophic during the period assessed (i.e., oxygen fluxes in light chambers were positive, with photosynthetic oxygen production exceeding total consumption). Sites A and B had the highest gross oxygen production (1,600 and 1,570 μmol m−2 h−1, respectively, relative to 1,110–1,260 μmol m−2 h−1 at the other sites).
The benthic invertebrate communities investigated in 2016, six months after experimentally adding Atrina mimics to the seabed, did not differ between structured and control plots (Site PPERM = 0.001, Treatment PPERM = 0.207, Site × Treatment interaction PPERM = 0.775; Figure 2B). Similar results were also obtained for analyses conducted on meiofauna abundance (PPERM = 0.349) and sediment chlorophyll a content (PPERM 0.949). Analysis of 2015 and 2016 benthic infauna data demonstrated differences by site and year, with communities at all sites significantly different from one another in both 2015 and 2016 (PPERM < 0.05 for all post-hoc pairwise tests, based on Bray-Curtis similarities generated from 4th root transformed community data). The differences between years at a given site were generally greater than the differences among sites within a year (Figure 2A).
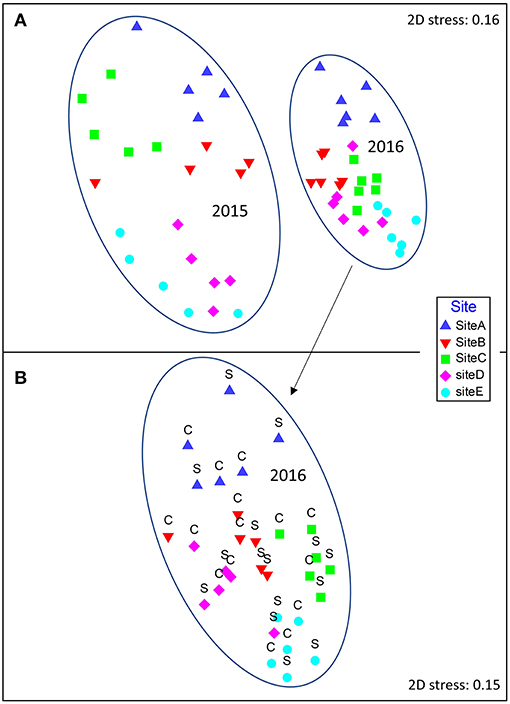
Figure 2. Non-metric multidimensional scaling (nMDS) plot of Bray-Curtis similarities based on log(x+1) transformed sediment invertebrate community sample data (organisms ≥ 0.125 mm). Points closer to one another in ordination space are more similar than those apart. The five sites in Mahurangi Harbor are indicated with different symbol colors and shapes (see legend on figure). (A) Data from the five sites in both years, (B) data from the five sites in 2016 only, with structured and control plot samples indicated by S and C, respectively. Stress value is an indication of how well the multivariate data are represented in two dimensions.
Nematodes were the most common sediment dwelling invertebrate at all sites in 2016, and other meiofaunal groupings such as ostracods and copepods were among the top five most abundant organisms at all sites. Site F had the highest total abundance of nematodes, total meiofauna, and polychaetes of the genus Pseudopolydora. Site A had the lowest abundance of Pseudopolydora sp., but had relatively high numbers of bivalves Arthritica bifurca and Phoxocephalidae amphipods. Theora lubrica, a mud-tolerant bivalve, was relatively common at sites A, B, and C (sites with relatively high sediment mud content). Oligochaetes and small syllid and paraonid polychaetes were other taxa that ranked in the top 10 in abundance at all five sites.
The abundance of zooplankton taxa that dominate snapper diet (here calanoid and cyclopid copepod, and unidentified crustaceans) in bottom water was greatest at the inner estuary sites (A, B, and C), relative to the sites closer to the mouth (D and E). Average sediment mud content and organic matter content were strongly correlated with zooplankton abundance (Pearson's R = 0.87–0.95; Table 1). Correlations of all three variables with turbidity were high (Pearson's R = 0.80–0.84; Table 1).
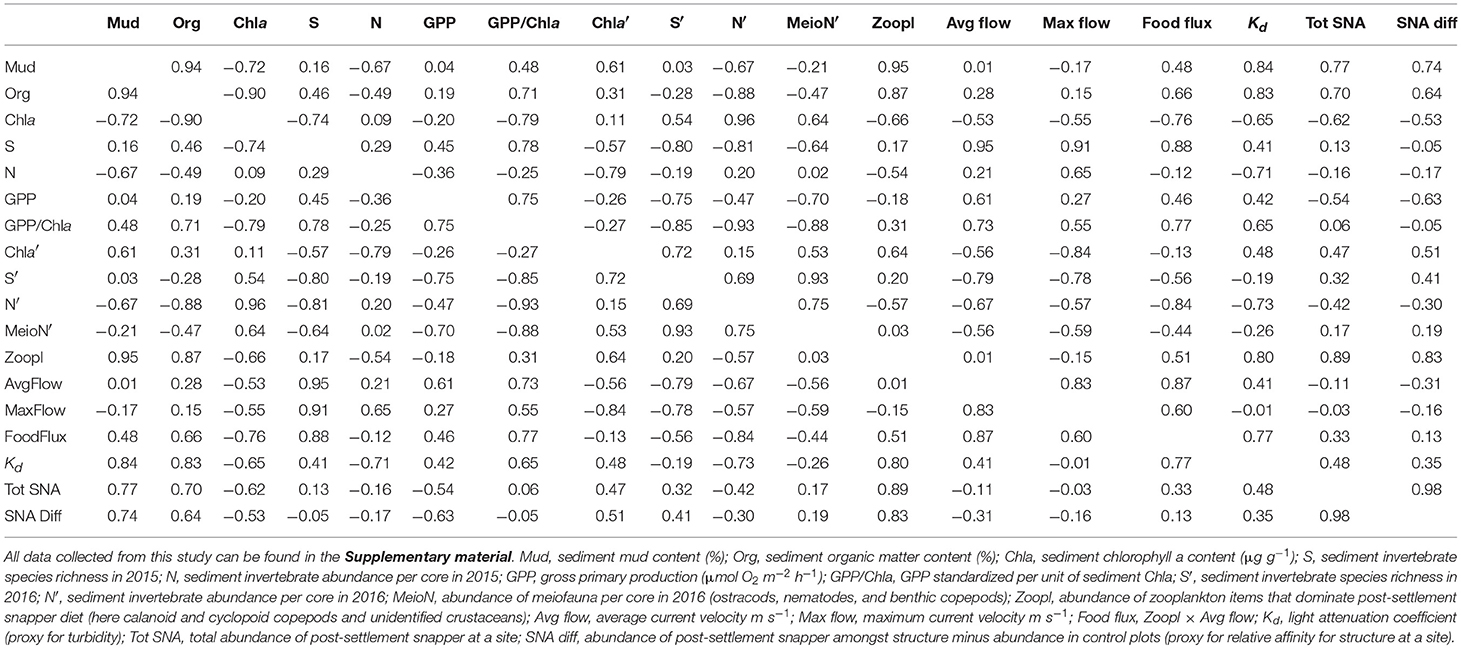
Table 1. Pearson product-moment correlation coefficients for environmental variables, based on averages calculated at each of the five study sites.
Effect of Additional Structure on Post-Settlement Snapper
The abundance of post-settlement snapper in Mahurangi Harbor was generally low, with a cumulative count of just 77 post-settlement snapper from 280 two-min video segments analyzed across all sites and treatments (using the maximum number of post-settlement snapper observed during each two-min video segment). The number of post-settlement snapper caught in opera house traps in 2015 and 2016 was also low (n = 34 total snapper collected).
The experimental addition of structure (i.e., Atrina mimics) was a significant factor explaining the abundance of post-settlement snapper at some sites, with more snapper present next to structure (compared to control patches) on average. Specifically, snapper abundance varied by site (PPERM = 0.013), and there was a significant site × treatment interaction (PPERM = 0.0496). The significant interaction term was the result of greater post-settlement snapper abundance near structure, relative to controls, at sites C, D, and A (Table 2). Although there were also more post-settlement snapper next to structure than at controls at Sites B and F, the effect of structure was not significant at these two sites (note there was only one fish recorded at Site E) (Table 2). As mentioned above, the addition of structure prior to the 2016 sampling did not have a significant effect at any site on invertebrate communities, meiofauna or sediment chlorophyll a content; even at site C, where the response of post-settlement snapper to the added artificial structure was the greatest.
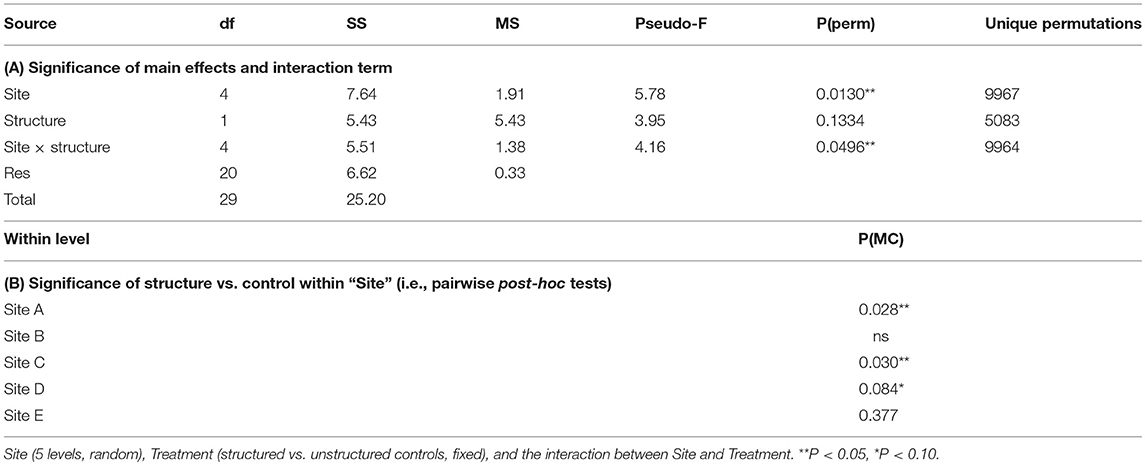
Table 2. Effects of experimentally added structure on the abundance of post-settlement snapper (Avg SNA), assessed using non-parametric permutational multivariate analysis of variance (PERMANOVA, Primer 7).
Relationship Between Post-Settlement Snapper Abundance and Environmental Variables
Although the resemblance matrix for total SNA present at each site was correlated with the resemblance matrix of environmental variables [Spearman rank correlation method, rho = 0.794, significance level 4.1% (i.e., α = 0.05)], the resemblance matrix for SNA diff (i.e., indicative of variation in the relative importance of structure to snapper across sites) was not significantly correlated with the resemblance matrix of environmental variables [Spearman rank correlation method, rho = 0.212, significance level 30.18% (i.e., α > 0.05)].
The best single environmental predictor of the response of post-settlement snapper to structure (SNA diff) across sites was the bottom water abundance of zooplankton items found to dominate post-settlement snapper diet (here calanoid and cyclopid copepod, and unidentified crustaceans) (p = 0.08, r = 0.59; Figures 3, 4). Snapper diet was pooled across all sites and both the 2015 and 2016 sampling events due to low sample size.
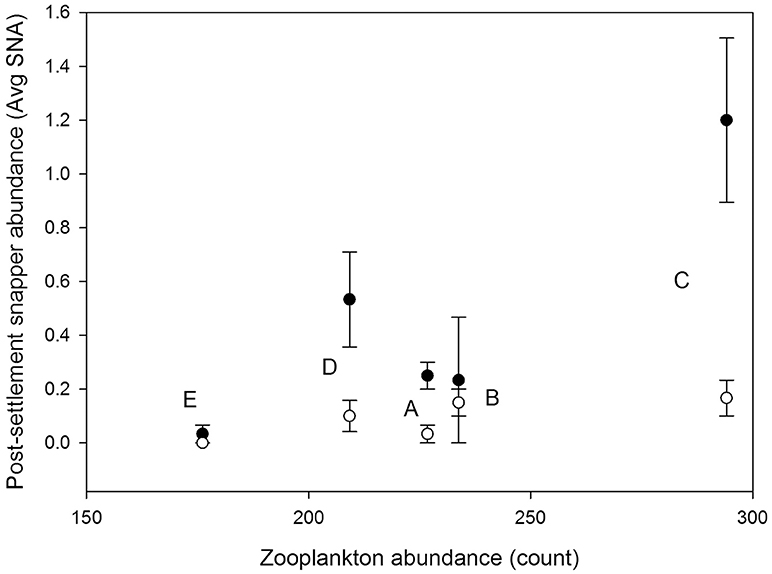
Figure 3. Average abundance of post-settlement snapper (Avg SNA) in structured (filled circle) and control (i.e., unstructured, open circle) habitat patches at each of the five study sites (E, A, D, B, C; labeled next to relevant data points). Error bars are ±1 standard error. The post-settlement snapper data are plotted vs. the average abundance of zooplankton items which dominate post-settlement snapper diet (calanoid and cyclopid copepods, and unidentified crustaceans) collected from near-bed bottom water at each site (see section Materials and Methods and Figure 4).
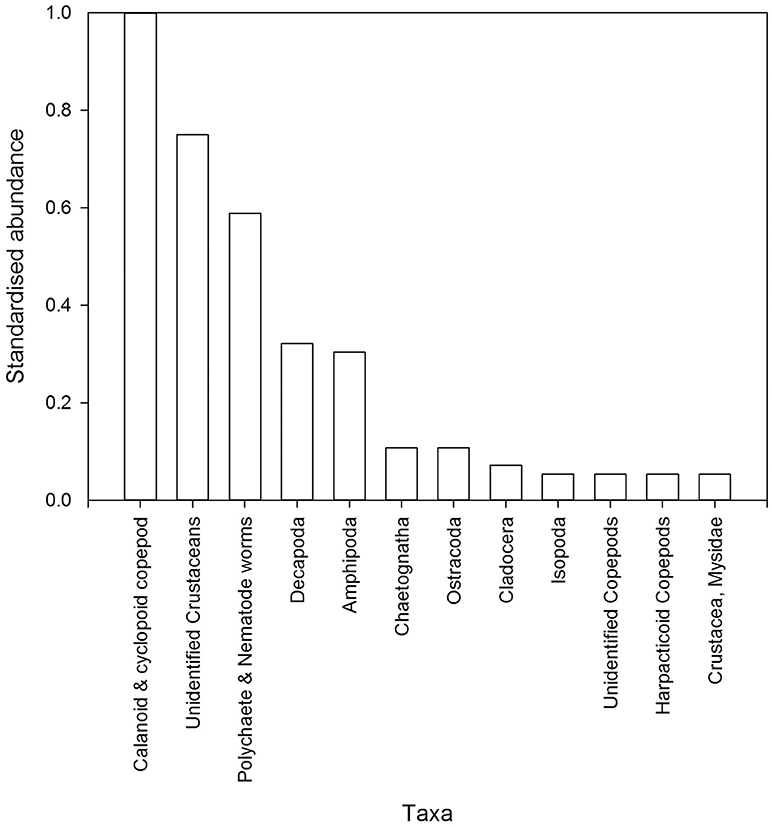
Figure 4. Abundance of invertebrates from post-settlement snapper gut contents (n = 35 individual fish) collected from all sites in 2015 and 2016. Abundances are standardized by the most abundant taxa and ordered from most to least abundant. Taxonomic nomenclature presented is not always at the same level, and in some cases has been pooled to likely snapper diet groups.
Overall, a two parameter model with zooplankton abundance and water column turbidity (Kd) had greatest explanatory power (p = 0.01, r = 0.98), with zooplankton abundance (parameter estimate 1.76, p = 0.0057) and turbidity (parameter estimate −0.84, p = 0.0163) showing positive and negative associations with the response of post-settlement snapper to structure (SNA diff), respectively. A model with organic matter content and GPP also performed well (parameter estimates 0.84 [p = 0.0036] and −0.71 [p = 0.0037], respectively; model p = 0.0044, r = 0.9913).
Discussion
Understanding the environmental and habitat drivers of abundance of a nursery stage juvenile fish has potential benefits for managers considering the best approaches to sustain fish populations (Adams et al., 2006; Nagelkerken, 2009). In the present study, the overall number of post-settlement snapper observed was low. For example, the average number of post-settlement snapper within 2 min video segments was < 1, whereas a similar study using artificial seagrass units in a nearby clear water harbor observed c. 6 snapper per 2 min video segment (Parsons et al., 2018). Despite this low abundance, the influence of the structure that we added to the seabed was strong (65 post-settlement snapper associated with structure vs. 12 from bare controls). This indicates the critical importance of structure as a driver of post-settlement snapper abundance, even within a somewhat degraded estuary (Lowe et al., 2015). The strong affinity to structure is also consistent with previous studies on fishes generally. For example, habitat structural complexity is an important determinant of fish assemblages on Hawaiian coral reefs and artificial reefs in the British Virgin Islands, and the abundance of young of the year rock sole (Lepidopsetta polyxystra) in Alaska (Friedlander and Parrish, 1998; Gratwicke and Speight, 2005; Stoner et al., 2007). Furthermore, multiple other studies conducted on post-settlement snapper have found similar results where higher abundances of snapper are associated with biogenic structures such as sponges or topographic complexity (Thrush et al., 2002; Compton et al., 2012; Lowe, 2013; Parsons et al., 2016), or artificial structures such as seagrass or bivalve mimics (Usmar, 2009; Parsons et al., 2013).
While the response to structure was undoubtedly the strongest driver of post-settlement snapper abundance, other environmental or habitat variables also had some influence. This was indicated by between site variation in the total abundance of post-settlement snapper (Total SNA) and the relative affinity of post-settlement snapper for structure between sites (SNA diff). Some previous studies conducted on snapper in New Zealand and Australia and the similar Pagrus major from Japan, have suggested that such variation in juvenile abundance is largely a response to benthic food availability (Tanaka, 1985; Francis, 1995, 1997; Sudo and Azeta, 2001; Hamer and Jenkins, 2004; Saunders et al., 2012). This is unlikely to be true for the present study, as post-settlement snapper diet (pooled across all sites) was dominated by pelagic zooplankton (not benthic infauna), the abundance of which was the most influential single explanatory variable of snapper abundance across sites. Alternatively, benthic invertebrates were observed to be poor predictors of snapper abundance. Therefore, it appears that the lack of affinity for structure and the preference for benthic food sources in these previous studies may be explained by a focus on larger juvenile snapper, which are known to prefer benthic food sources (Usmar, 2012), or entirely different life history patterns existing for juvenile snapper in different geographic regions.
Previous research has suggested that locations with high water flow may offer post-settlement snapper an increased flux of the pelagic zooplankton they prefer to eat, while benthic structure may help to reduce energy expenditure by providing shelter from fast water flow at these sites (Parsons et al., 2015, 2018). Other research has suggested that sediment induced turbidity is highly influential on post-settlement snapper health and abundance, and can cause a switch from a predominantly pelagic to predominantly benthic diet (Lowe et al., 2015). As such, as part of the present study we sought to quantify environmental characteristics, including benthic and water column food availability, turbidity and current speeds that potentially explain variation in the response of post-settlement snapper to structure across sites.
Our results demonstrated that the abundance of zooplankton (the post-settlement snapper diet component) was highest in muddy, organically enriched, inner estuary sites. While there is no immediate explanation for this relationship, post-settlement snapper abundance was positively related to zooplankton abundance (as described above). Alternatively, variables such as average current speed, maximum current speed, and food flux (zooplankton abundance multiplied by average current speed) were uncorrelated to post-settlement snapper abundance and structure use, and were among the weakest explanatory variables in our dataset. The lack of relationship between post-settlement snapper and food flux could be due to a number of explanations, including the potential for vertical migrations of zooplankton to disconnect water flow and accessible food abundance (Kimmerer et al., 2014). Beyond this, when turbidity was considered together with zooplankton abundance, it had a negative relationship with snapper abundance. Together these results confirm that post-settlement snapper respond to structure and pelagic food abundance (Parsons et al., 2015, 2016), however, at least in Mahurangi Harbor, this relationship isn't mediated by energetic gains related to food flux or hydrodynamic shelter provided by structure as it is for other fish species (Fausch and White, 1981). The negative response to turbidity further emphasizes the importance of pelagic over benthic food sources for the post-settlement stage, and is well aligned with the findings of Lowe et al. (2015), who also found a predominance of pelagic prey in the Mahurangi Harbor snapper analyzed in that study.
It is important to note the limitations of the dataset used in the present study. We collected environmental data between March 2015 and March 2017, which we compared with post-settlement snapper abundance and structure use from March 2016. Moreover, having to condense environmental variables down to simple site averages resulted in a loss of information and weakened our inferential power. A greater number of sites would have been beneficial, as this would have allowed us to include more than two variables within regression models, but was unachievable due to the labor intensive nature of conducting a controlled structure manipulation experiment by SCUBA.
Overall our results provide strong evidence that access to benthic habitat structure and an abundance of zooplankton prey, such as calanoid and cyclopoid copepods, are of primary importance to post-settlement stage snapper. Both the presence of biogenic structure and the ability to visually feed on zooplankton prey require relatively clear water with low sediment induced turbidity (Ellis et al., 2002; Thrush et al., 2004; Waycott et al., 2009), which was also identified as negatively related to post-settlement snapper abundance in the present study. Therefore, the identification and management of the most productive snapper nursery habitats (Parsons et al., 2014) should center on the three variables identified here, namely, turbidity, habitat structure and zooplankton abundance. Because turbidity, and to some extent the presence of habitat structure, can be estimated remotely, these variables could serve as starting points that are later field verified and supplemented with zooplankton abundance data. While the relationship between post-settlement snapper, turbidity and zooplankton are well described, we are now potentially less certain as to why post-settlement have such a high affinity for structure. The positive effect that structure may provide with regard to predation avoidance and food provision are obvious candidates (Beck et al., 2001), but it is the specific mechanisms (Nagelkerken, 2009) which remain elusive and yet may provide important context on the path to more effective nursery habitat conservation or restoration.
Author Contributions
AL and DP conceived the study and designed the experiment. AL, DP, LM, DB, and IM collected data and processed samples. AL and DP analyzed the data and wrote the manuscript, LM, DB, and IM wrote sections of the manuscript and provided revisions.
Funding
This work was supported by the NIWA Coasts and Oceans research programme 3 [Statement of Corporate Intent 2015/16].
Conflict of Interest Statement
The authors declare that the research was conducted in the absence of any commercial or financial relationships that could be construed as a potential conflict of interest.
Acknowledgments
We thank Samantha Parkes, Caroline Roberts, and Keren Spong for assistance in the laboratory and field.
Supplementary Material
The Supplementary Material for this article can be found online at: https://www.frontiersin.org/articles/10.3389/fmars.2018.00427/full#supplementary-material
References
Adams, A. J., Dahlgren, C. P., Kellison, G. T., Kendall, M. S., Layman, C. A., Ley, J. A., et al. (2006). Nursery function of tropical back-reef systems. Mar. Ecol. Prog. Series 318, 287–301. doi: 10.3354/meps318287
Beck, M. W., Heck, K. L., Able, K. W., Childers, D. L., Eggleston, D. B., Gillanders, B. M., et al. (2001). The identification, conservation, and management of estuarine and marine nurseries for fish and invertebrates. Bioscience 51, 633–641. doi: 10.1641/0006-3568(2001)051[0633:TICAMO]2.0.CO;2
Compton, T. J., Morrison, M., Leathwick, J. R., and Carbines, G. (2012). Ontogenetic habitat associations of a demersal fish species (Pagrus auratus) identified using boosted regression trees. Mar. Ecolo. Prog. Series 462, 219–230. doi: 10.3354/meps09790
Cummings, V. J., Thrush, S. F., Hewitt, J. E., and Turner, S. J. (1998). The influence of the pinnid bivalve Atrina zelandica (Gray) on benthic macroinvertebrate communities in softsediment habitats. J. Exp. Mar. Biol. Ecol. 228, 227–240. doi: 10.1016/S0022-0981(98)00028-8
Dahlgren, C. P., Todd, G. T., Adams, A. J., Gillanders, B. M., Kendall, M. S., Layman, C. A., et al. (2006). Marine nurseries and effective juvenile habitats: concepts and applications. Mar. Ecol. Prog. Series 312, 291–295. doi: 10.3354/meps312291
Ellis, J., Cummings, V. J., Hewitt, J. E., Thrush, S. F., and Norkko, A. (2002). Determining effects of suspended sediment on condition of a suspension feeding bivalve (Atrina zelandica): results of a survey, a laboratory experiment and a field transplant experiment. J. Exp. Mar. Biol. Ecol. 267, 147–174. doi: 10.1016/S0022-0981(01)00355-0
Fausch, K. D., and White, R. J. (1981). Competition between brook trout (Salvelinus fontinalis) and brown trout (Salmo trutta) for positions in a Michigan Stream. Can. J. Fish. Aqua. Sci. 38, 1220–1227. doi: 10.1139/f81-164
Feeney, C. M., and Challis, D. (1984). Water and Soil Management Issues In The Mahurangi Catchment, Estuary And Harbour. Auckland Regional Water Board Technical Publication No.29.
Francis, M. P. (1994). Growth of juvenile snapper, Pagrus auratus. NZ. J. Mar. Freshw. Res. 28, 201–218. doi: 10.1080/00288330.1994.9516608
Francis, M. P. (1995). Spatial and seasonal variation in the abundance of juvenile snapper (Pagrus auratus) in the north-western Hauraki Gulf. NZ J. Mar. Freshw. Res. 29, 565–579. doi: 10.1080/00288330.1995.9516688
Francis, M. P. (1997). Condition cycles in juvenile Pagrus auratus. J. Fish Biol. 51, 583–600. doi: 10.1111/j.1095-8649.1997.tb01514.x
Friedlander, A. M., and Parrish, J. D. (1998). Habitat characteristics affecting fish assemblages on a Hawaiian coral reef. J. Exp. Mar. Biol. Ecol. 224, 1–30. doi: 10.1016/S0022-0981(97)00164-0
Gatehouse, J. S. (1971). “Sedimentary analysis,” in Procedures in Sedimentology and Petrology ed. R. E. Carver (New York, NY: Wiley Interscience), 59–94.
Gratwicke, B., and Speight, M. R. (2005). Effects of habitat complexity on Caribbean marine fish assemblages. Mar. Ecol. Prog. Series 292, 301–310. doi: 10.3354/meps292301
Hamer, P. A., and Jenkins, G. P. (2004). High levels of spatial and temporal recruitment variability in the temperate sparid Pagrus auratus. Mar. Freshw. Res. 55, 663–673. doi: 10.1071/MF04024
Heck, K. L. Jr, Hays, G., and Orth, R. J. (2003). Critical evaluation of the nursery role hypothesis for seagrass meadows. Mar. Ecol. Prog. Series 253, 123–136. doi: 10.3354/meps253123
Kimmerer, W. J., Gross, E. S., and Macwilliams, M. L. (2014). Tidal migration and retention of estuarine zooplankton investigated using a particle-tracking model. Limnol. Oceanogr. 59, 901–906. doi: 10.4319/lo.2014.59.3.0901
Lohrer, A. M., Townsend, M., Rodil, I. F., Hewitt, J. E., and Thrush, S. F. (2012). Detecting shifts in ecosystem functioning: The decoupling of fundamental relationships with increased pollutant stress on sandflats. Mar. Pollut. Bull. 64, 2761–2769. doi: 10.1016/j.marpolbul.2012.09.012
Lowe, M. (2013). Factors Affecting the Habitat Usage of Juvenile Estuarine Fish in Northern New Zealand. PhD Thesis, University of Auckland, Auckland.
Lowe, M. L., Morrison, M. A., and Taylor, R. B. (2015). Harmful effects of sediment-induced turbidity on juvenile fish in estuaries. Mar. Ecol. Prog. Series 539, 241–254. doi: 10.3354/meps11496
Mook, D. H., and Hoskin, C. M. (1982). Organic determination by ignition: caution advised. Estuarine Coast. Shelf Sci. 15, 697–699.
Morrison, M., and Carbines, G. (2006). Estimating the abundance and size structure of an estuarine population of the sparid Pagrus auratus, using a towed camera during nocturnal periods of inactivity, and comparisons with conventional sampling techniques. Fish. Res. 82, 150–161. doi: 10.1016/j.fishres.2006.06.024
Nagelkerken, I. (2009). “Evaluation of nursery function of mangroves and seagrass beds for tropical decapods and reef fishes: patterns and underlying mechanisms,” in Ecological Connectivity Among Tropical Coastal Ecosystems, ed. I. Nagelkerken. (Dordrecht, Netherland: Springer Science and Business Media), 357–399.
Orth, R. J., Carruthers, T. J. B., Dennison, W. C., Duarte, C. M., Fourqurean, J. W., Heck, K. L., et al. (2006). A global crisis for seagrass ecosystems. BioScience 56, 987–996. doi: 10.1641/0006-3568(2006)56[987:AGCFSE]2.0.CO;2
Parsons, D., Morrison, M., Thrush, S. F., Middleton, C., Smith, M., Spong, K., et al. (2013). The influence of habitat structure on juvenile fish in a New Zealand estuary. Mar. Ecol. 34, 492–500. doi: 10.1111/maec.12050
Parsons, D. M., Buckthought, D., Middleton, C., and Mackay, G. (2016). Relative abundance of snapper (Chrysophrys auratus) across habitats within an estuarine system. NZ. J. Mar. Freshw. Res. 50, 358–370. doi: 10.1080/00288330.2016.1146310
Parsons, D. M., Macdonald, I., Buckthought, D., and Middleton, C. (2018). Do nursery habitats provide shelter from flow for juvenile fish? PLoS ONE 13:e0186889. doi: 10.1371/journal.pone.0186889
Parsons, D. M., Middleton, C., Spong, K. T., Mackay, G., Smith, M. D., and Buckthought, D. (2015). Mechanisms explaining nursery habitat association: how do juvenile snapper (Chrysophrys auratus) benefit from their nursery habitat? PLoS ONE 10:e0122137. doi: 10.1371/journal.pone.0122137
Parsons, D. M., Sim-Smith, C. J., Cryer, M., Francis, M. P., Hartill, B., Jones, E. G., et al. (2014). Snapper (Chrysophrys auratus): a review of life history and key vulnerabilities in New Zealand. NZ. J. Mar. Freshw. Res. 48, 256–283. doi: 10.1080/00288330.2014.892013
Sartory, D. P. (1982). Spectrophometric Analysis of Chlorophyll a in Freshwater Phytoplankton. Pretoria: Hydrological Research Institute, Department of Environmental Affairs.
Saunders, R. J., Fowler, A. J., and Gillanders, B. M. (2012). The use of food resources by 0+ snapper, Chrysophrys auratus, from northern Spencer Gulf, South Australia. Mar. Freshw. Res. 63, 680–686. doi: 10.1071/MF11266
Schobernd, Z. H., Bacheler, N. M., and Conn, P. B. (2014). Examining the utility of alternative video monitoring metrics for indexing reef fish abundance. Can. J. Fish. Aquat. Sci. 71, 464–471. doi: 10.1139/cjfas-2013-0086
Stoner, A. W., Spencer, M. L., and Ryer, C. H. (2007). Flatfish-habitat associations in Alaska nursery grounds: use of continuous video records for multi-scale spatial analysis. J. Sea Res. 57, 137–150. doi: 10.1016/j.seares.2006.08.005
Sudo, H., and Azeta, M. (2001). The microhabitat and size of gammarid species selectively predated by young red sea bream Pagrus major. Fish. Sci. 67, 389–400. doi: 10.1046/j.1444-2906.2001.00274.x
Tanaka, M. (1985). Factors affecting the inshore migration of pelagic larval and demersal juvenile red sea bream Pagrus major to a nursery ground. Trans. Am. Fish. Soc. 114, 471–477.
Thrush, S. F., Hewitt, J. E., Cummings, V. J., Ellis, J. I., Hatton, C., Lohrer, A., et al. (2004). Muddy waters: elevating sediment input to coastal and estuarine habitats. Front. Ecol. Environ. 2, 299–306. doi: 10.1890/1540-9295(2004)002[0299:MWESIT]2.0.CO;2
Thrush, S. F., Schultz, D., Hewitt, J. E., and Talley, D. (2002). Habitat structure in soft-sediment environments and abundance of juvenile snapper Pagrus auratus. Mar. Ecol. Prog. Series 245, 273–280. doi: 10.3354/meps245273
Townsend, M., Thrush, S. F., Lohrer, A. M., Hewitt, J. E., Lundquist, C. J., Carbines, M., et al. (2014). Overcoming the challenges of data scarcity in mapping marine ecosystem service potential. Ecosyst. Serv. 8, 44–55. doi: 10.1016/j.ecoser.2014.02.002
Usmar, N. R. (2009). Ontogeny and Ecology of Snapper (Pagrus auratus) in an Estuary, the Mahurangi Harbour. PhD thesis, University of Auckland, Auckland.
Usmar, N. R. (2012). Ontogenetic diet shifts in snapper (Pagrus auratus: Sparidae) within a New Zealand estuary. NZ. J. Mar. Freshw. Res. 46, 31–46. doi: 10.1080/00288330.2011.587824
Keywords: Pagrus auratus, juvenile fish nursery, habitat structure, environmental drivers, hydrodynamic variables, zooplankton, benthic infauna, diet
Citation: Lohrer AM, McCartain LD, Buckthought D, MacDonald I and Parsons DM (2018) Benthic Structure and Pelagic Food Sources Determine Post-settlement Snapper (Chrysophrys auratus) Abundance. Front. Mar. Sci. 5:427. doi: 10.3389/fmars.2018.00427
Received: 29 August 2018; Accepted: 26 October 2018;
Published: 19 November 2018.
Edited by:
Toyonobu Fujii, Tohoku University, JapanReviewed by:
Ulisses Miranda Azeiteiro, University of Aveiro, PortugalKusum Komal Karati, Centre for Marine Living Resources and Ecology (CMLRE), India
Copyright © 2018 Lohrer, McCartain, Buckthought, MacDonald and Parsons. This is an open-access article distributed under the terms of the Creative Commons Attribution License (CC BY). The use, distribution or reproduction in other forums is permitted, provided the original author(s) and the copyright owner(s) are credited and that the original publication in this journal is cited, in accordance with accepted academic practice. No use, distribution or reproduction is permitted which does not comply with these terms.
*Correspondence: Darren M. Parsons, ZGFycmVuLnBhcnNvbnNAbml3YS5jby5ueg==