- 1Marine Ecology Research Group, School of Biological Sciences, University of Canterbury, Christchurch, New Zealand
- 2National Institute of Water and Atmospheric Research Ltd, Christchurch, New Zealand
Shallow coastal rocky reefs worldwide have faced large-scale loss of biodiversity, yet little is known about the contributions of highly diverse multi-layered autotrophic species to ecosystem function over long periods. In this study we tested the role of functional diversity on net primary productivity (NPP) of macroalgal assemblages in one-off, and multi-year experiments. We removed canopy (perennial fucoid), mid-canopy and basal algae (turfing and encrusting algae) in intertidal assemblages and used in situ photorespirometry to measure recovery of NPP over 2 years. Experimental removal of the canopy caused a >50% decline in NPP, which remained significantly lower than undisturbed assemblages for up to 24 months. Removal of midcanopy and basal algae reduced NPP by >25%, but converged with controls after just 6–12 months. Canopy loss greatly reduced NPP for long periods and productivity began recovering only after the recruitment of the canopy-forming species. Subcanopy species composition varied with experimental disturbance treatment and through time, but consistently contributed to total assemblage NPP. High irradiance in the shallow intertidal zone may be vital to a maximizing the efficiency of light harvesting, and the spatial and temporal variations in species composition may play a critical role in buffering carbon fixation of macroalgal assemblages.
Introduction
Primary production is one of the most important functions of autotrophic assemblages, and macroalgae, the dominant primary producers of nearshore temperate marine ecosystems, are widely recognized as some of the most productive habitat-dominating organisms on a per-area basis (Mann, 1973; Gattuso et al., 2006). Macroalgae contribute significantly to coastal ecosystems (Anderson and Polis, 1998) and isotopic signatures of macroalgae can be found in organisms several kilometers offshore (Hill et al., 2006) and even in deep offshore basins (Fischer and Wiencke, 1992). However, large algae are susceptible to perturbations and biomass loss through a wide range of stressors (Schiel, 2006; Reed et al., 2011) including increased coastal run-off and sedimentation (Irving et al., 2009; Foster and Schiel, 2010), poor water quality (Foster and Schiel, 2010; Krause-Jensen et al., 2012), and increased temperatures (Schiel et al., 2004; Connell and Russell, 2010; Wernberg et al., 2010). There is increasing scrutiny of the interactions between multi-layered autotrophic assemblages in marine systems (Binzer and Sand-Jensen, 2002; Binzer et al., 2006; Miller et al., 2009; Harrer et al., 2013), particularly the potential for compensatory dynamics (Miller et al., 2009) and complementarity (Stachowicz et al., 2008; Tait et al., 2014; Bulleri et al., 2016) among benthic autotrophs. Yet the contribution of multi-layered autotrophic assemblages to primary productivity in the dynamic shallow marine environment has been poorly explored.
Most plant communities are vertically structured, resulting in light delivery that is variable to different layers (Mission et al., 2007; Sand-Jensen et al., 2007; Arkema et al., 2009; Mercado et al., 2009; Harrer et al., 2013) Because of high rates of photon capture within canopies, light can be a limiting resource in the subcanopy assemblage. In giant kelp forests [i.e., Macrocystis pyrifera (Linnaeus) C. Agardh], for example, light reaching the subcanopy is 90–98% less than that at the surface, with basal, reef-covering species receiving even less light (Neushul, 1971; Reed and Foster, 1984; Santelices and Ojeda, 1984). Primary productivity dynamics, however, have been mostly concerned with canopy species (Mann, 1973; Gollety et al., 2008; Reed et al., 2008; Migne et al., 2015). By comparison, the subcanopy of terrestrial forest ecosystems may contribute up to 30% of total ecosystem NPP (Mission et al., 2007), and the subcanopy of macroalgal assemblages could be equally important (Miller et al., 2009, 2011).
Light distribution within terrestrial forest canopies is critical for parameterizing photosynthetic models, including the relative contribution of diffuse and direct light (Roderick et al., 2001; Roderick, 2006), but the full canopy light-field has rarely been incorporated into marine photosynthetic models. This is surprising given the opportunity to directly measure integrated assemblage photosynthesis (Tait and Schiel, 2010), with the potential to calibrate and compare developing aquatic eddy-covariance methods for estimating whole ecosystem fluxes (Attard et al., 2014). Furthermore, the often compromised marine light environment is subject to variability on scales generally not seen in terrestrial systems. In particular, the lensing of light on the sea surface (Stramski and Legendre, 1992; Veal et al., 2010), the occlusion of light by suspended particles (both inorganic and organic), and changing tidal levels (Anthony et al., 2004) affect light attenuation to the benthos over multiple temporal and spatial scales, with largely unknown consequences to photosynthesis of multi-layered assemblages.
While total ecosystem models of NPP in terrestrial systems have developed beyond “big-leaf” models to better account for canopy and subcanopy interactions (Dai et al., 2004), there has been a general lack of integration of subcanopy photosynthesis into models of marine macrophyte production. Because there is a strong gradient of irradiance through macroalgal canopies (Reed and Foster, 1984; Harrer et al., 2013), different taxa or layers beneath canopies show photosynthetic responses that reflect their immediate light climate and their photoadapted state (Binzer et al., 2006). This has implications for the compensatory response of subcanopy layers to canopy loss (Miller et al., 2011), whereby subcanopy species inhabiting a low-light environment can respond positively to elevated radiation. These sorts of mechanisms may be manifested through time in natural communities, however, as cascading losses may occur in some cases or quick recovery in others.
Here we test the role of different canopy layers on net primary productivity (NPP) of algal assemblages and their ability to compensate for the loss of species using in situ photorespirometry (Tait and Schiel, 2010). We manipulated canopy and subcanopy species of assemblages in southern New Zealand and Oregon, USA, and tested how NPP responds over the full range of light intensities encountered in shallow intertidal systems. Furthermore, we tested changes in NPP dynamics of manipulated assemblages over 2 years (New Zealand only) to examine the functional roles of canopy species and some of the less conspicuous subcanopy species, which comprise most of the diversity in these systems (Lilley and Schiel, 2006; Schiel, 2006). To better understand species redundancy during recovery in the subcanopy, ephemeral subcanopy species (low biomass, high productivity), and perennial subcanopy species (high biomass, low productivity; primarily articulated calcareous algae) were further separated into two nominal components, basal algae (encrusting and articulate coralline algae) and midcanopy algae (perennial and ephemeral species beneath canopies, but above basal species).
In situ photorespirometry provides an instantaneous measure of the photosynthetic capacity of these assemblages with little alteration of natural conditions. This allowed NPP of assemblages to be followed through time along their natural trajectory of recovery. Through a series of experimental manipulations of the canopy, midcanopy, and basal turfing and encrusting components of algal assemblages we test, (a) the consequences of removing assemblage components on primary productivity, (b) whether and to what degree there is compensation for the loss of any assemblage components, and (c) the time trajectory over 2 years for any compensatory dynamics to occur.
Methods
Study Sites, Incubation Protocol and Standardization
Manipulation of layered macroalgal assemblages was done in two ways: (1) one-off manipulations of canopy and subcanopy components at two shore-heights in New Zealand and Oregon, and (2) tracking recovery dynamics of several canopy manipulations over 2 years in the mid shore of New Zealand.
One-off canopy manipulation experiments were done in assemblages dominated by the fucoid algae Hormosira banksii (Turner) Desicaine and Cystophora torulosa (R. Brown ex Turner) J. Agardh at Wairepo Reef, Kaikoura New Zealand (42° 25′ S, 173° 42′ E) and the fucoid algae Pelvetiopsis limitata (Setchell) N.L. Gardner and Fucus distichus (Linnaeus) at Fogarty Creek Reef, Oregon USA (44°51′ N, 124° 03′ W). The same three treatments were used in both locations: (1) intact assemblages, (2) removal of subcanopy assemblages (canopy only), and (3) removal of canopy species leaving the subcanopy assemblage intact. Photorespirometry incubations were performed on control and treatment assemblages at least 24 h after canopy and subcanopy removal. These experiments were done in austral summer in New Zealand (January 2009) and boreal summer in Oregon (June 2009).
Incubations employed custom-designed photorespirometry chambers (Tait and Schiel, 2010) which were sealed around target assemblages prior to incubations and removed afterwards to limit any long-term effects. This allowed the same assemblages to be resampled over time, while being exposed to natural conditions and successional processes. Oxygen concentrations were measured using a Hach LDO meter and water was exchanged at intervals of 10 min to avoid super-saturation of oxygen (Tait and Schiel, 2010). During incubations, light and temperature were measured using HOBO (Onset ©) loggers, with light converted to irradiance by cross-calibration with a Li-Cor LI 192 quantum sensor. All visible macro-invertebrates were hand-removed from the assemblages and a water pump was used to remove sediments and micro-invertebrates from coralline turfs to exclude most of the heterotrophic respiration. Incubation series during sunlight were run for 2–3 h per day (individual incubations being 10 min each), and incubation series at night (i.e., to measure respiration rates) were run for 1 h. All incubations (day and night) were done on rising tides when chambers were submerged.
To construct productivity-irradiance (P-E) curves and relevant photosynthesis parameters for each independent replicate plot, primary productivity data were collected under a range of natural light intensities from 0 to c. 2000 μmol m−2s−1. For this reason, data on a single replicate assemblage were often collected over several days, and an entire sampling run (i.e., all replicates of all treatments) could last up to 3 weeks. Because incubations were dependent on the timing of low tide (i.e., when it is possible to install the chambers), time of day for incubations was therefore random between sampling visits. Furthermore, low light values (e.g., below 500 μmol m−2s−1) occurred for several reasons: high cloud cover, turbid water, and no cloud cover but low sun angle (i.e., morning or evening incubations). While there are potentially confounding effects associated with circadian rhythms of macroalgal species and the differences in delivery of direct or diffuse light, randomization of chamber allocation across canopy treatments resulted in the even distribution of biases across treatments. It is possible, however, that differences between sampling visits for the long-term experiment were affected in some way by specific combinations in these confounding variables.
For analyses, NPP data were standardized either to grams dry weight of algal material (mg O2 gDW−1 h−1) or to area of reef substratum (g O2 m−2 h−1). Data standardized to reef area gave an ecologically relevant measure of production, which identified the potential carbon fixation of assemblages along a shore. Per-weight standardization was done for one-off canopy manipulations by harvesting, drying and weighing all algae following incubations. Weight-specific estimates give an indication of species-specific primary productivity, which depends on morphology and thallus thickness (Miller et al., 2009) and accounts for the large loss of biomass associated with recent canopy removal. However, in long-term manipulation experiments where recovery of photosynthetic rates was followed over a 2-year period, and assemblages could not be directly harvested, data were standardized by area of reef.
Contribution of Canopy and Subcanopy to NPP
In New Zealand, one-off tests of canopy and subcanopy removal were done on three replicate assemblages (n = 3) of each treatment (intact assemblage, canopy only, and subcanopy only) for assemblages dominated by C. torulosa and H. banksii over a period of 2 weeks. The subcanopy of both assemblages consisted primarily of the coralline turf Corallina officinalis (Linnaeus), and a range of red, brown and green macroalgae [browns: Carpophyllum maschalocarpum (Turner) Grev., Colpomenia bullosa (Saunders) Yamada, Halopteris virgata (Hook F. and Harv.) N.M. Adams, Cystophora scalaris (J. Agardh), reds: Champia novea-zealandia (J.D. Hooker and Harvey) Hooker, Lophothamnion hirtum (J.D. Hooker and Harvey) Womersley, and greens: Ulva intestinalis (Linnaeus), Ulva pertusa (Linnaeus)].
One-off tests of canopy (F. distichus and P. limitata) and subcanopy [predominantly Mazzaella affinis (Harvey) Fredericq, Mastocarpus papillatus (C. Agardh) Kützing, Endocladia muricata (J. Agardh), and Cladophora columbiana (Collins) N.L. Gardner] removal in Oregon were done in June 2009, with three replicate plots (n = 3) of each treatment (intact assemblage, canopy only and subcanopy only) in assemblages dominated by F. distichus and P. limitata over a period of 2 weeks.
Oxygen production data were collected over a range of light conditions (0– c.2000 μmol m−2s−1) and related to incident light intensity averaged over the period of the incubation. Furthermore, the photosynthetic parameters α, Pm, Ec, and R were calculated for each assemblage (H. banksii, C. torulosa, P. limitata, and F. distichus) and each experimental treatment (intact assemblage, canopy alone, and subcanopy alone).
Recovery of NPP Following Component Loss
To test the redundancy in species contributions to assemblage primary productivity over time, control and removal plots were set up in the middle of the algal zone (0.2 m above LAT) at Wairepo Reef, Kaikoura (Schiel et al., 2006). Three initial treatments were used: (1) unmanipulated control (“intact assemblage”), (2) the dominant midcanopy species were carefully cut away while maintaining the cover of the dominant canopy and basal encrusting and turfing coralline species (“canopy + basal”), and (3) the canopy was cut away leaving the midcanopy and basal assemblage intact (“basal + midcanopy”). A year later, a fourth treatment (“Canopy + midcanopy”) was added to test the role of basal species on NPP dynamics; the turfing and encrusting basal layer of mostly C. officinalis was carefully scraped away along with any attached epiphytes, leaving the canopy and midcanopy species intact. There were three replicate plots (n = 3) for each treatment. All removal treatments were a one-off pulse removal. The first three treatments were set up in November 2008 and the fourth treatment in November 2009. All plots were 50 × 50 cm within which a photorespirometry chamber was set up (a chamber enclosed a reef area of 314 cm2). The plots were sampled with a gridded quadrat to determine the cover of each species when the plots were initiated and then at 3, 6, 12, and 24 months.
The three initial treatments were analyzed directly after species removals, and at 3, 6, 12, and 24 months after removal. The “canopy + midcanopy” treatment was tested at the time of removal, and at 6 and 12 months after removal. All macroalgal species covering an area greater than 0.5% of the substratum within the 50 × 50 cm experimental plots were recorded and their percentage cover estimated at each sampling time.
Subcanopy Irradiance Environment
To gauge the light intensity reaching subcanopy species, understory irradiance was examined by comparing the light penetrating the canopy to that outside of canopies. Light intensity was logged every second during 2 h periods on four occasions (to cover a wide range of light conditions and tidal heights), during incoming tides above and below canopies of H. banksii (n = 4) in Kaikoura. Light was measured using HOBO (Onset Corporation) light loggers cross-calibrated with a cosine corrected LiCor LI-192 quantum sensor. An exponential function was then fitted to the raw HOBO data to convert lum/ft2 to irradiance as PAR (μmol m−2 s−1), similar to Long et al. (2012).
Four light loggers were placed beneath four algal canopies simultaneously (i.e., 16 light loggers beneath canopies and 2 loggers outside canopies). The four understory loggers were arranged to account for the angle of the sun relative to the macroalgal canopy (i.e., one logger unshaded, one shaded and two on either side of the canopy), with understory irradiance averaged between the four loggers (over 30 s intervals). Subcanopy irradiance was compared to ambient (outside canopy) irradiance, using loggers placed on nearby open reef outside of algal canopies. The maximum irradiance estimated was 2,160 μmol m−2 s−1 on a sunny day with light directly overhead; irradiance below 800 μmol m−2 s−1 was typically associated with cloud cover. Periods of tidal emergence of light sensors were filtered from the data set.
Statistical Analyses
P-E curves were analyzed by comparing photosynthetic parameters after fitting curves of the form:
Walsby (1997), where R = respiration rate, photosynthetic efficiency (α), and maximum primary productivity (Pm). Compensating irradiance (Ec), the irradiance at which photosynthesis and respiration are equal was also calculated. Differences in each of the four photosynthetic parameters across canopy removal treatments for one-off removals were analyzed using two-way ANOVA with the fixed factors of “canopy treatment” and “shore height” separated for New Zealand (upper shore, Hormosira banksii; lower shore, Cystophora torulosa) and USA (upper shore, Pelvetiopsis limitata; lower shore, Fucus distichus). For significant treatment effects Holm-Sidak comparisons were also made to examine differences between canopy treatment combinations for each photosynthetic parameter. For long-term recovery experiments, differences in each photosynthetic parameter were analyzed separately for each canopy treatment through time. Two-way ANOVA between time and canopy treatment was not done due to an imbalanced design (i.e., the “Canopy + midcanopy” treatment was initiated later than the other treatments).
The recovery of NPP (per reef area, g O2 m−2 h−1) over time was tested using factorial ANOVA of treatments, across three levels of irradiance and at five sampling periods, 0, 3, 6, 12, and 24 months (all fixed factors), following removal. These data were analyzed by binning data across treatments and time into irradiance ranges of 100–200 μmol m−2 s−1 (low), 800–1200 μmol m−2 s−1 (mid) and 1600+ μmol m−2 s−1 (high). Data were binned for this analysis for two reasons: (1) imbalance in the collection of data across a full irradiance range between sampling visits meant that some independent replicate plots could be described by many individual data-points (i.e., 10 min incubations), while some could be described by only a single data-point; and (2) each irradiance category was related to three major trends in the P-E curves (i.e., at low irradiance canopies alone often outperformed assemblages, at mid irradiance most canopy combinations were relatively equal, and high irradiance where assemblages often outperformed canopies). Holm-Sidak comparisons of canopy treatments over time were also used to analyze the point in time at which differences between canopy removal treatments and intact assemblages were non-significant.
Recovery of the percent cover of dominant species (H. banksii, C. torulosa, and C. officinalis) and the remaining species (combined) were analyzed separately by two-way ANOVA (“time” and “canopy treatment”).
Prior to ANOVA, variance heterogeneity was tested with Cochran's C-tests and removed with log transformation when required. Student-Newman-Keuls (SNK) tests were done for a posteriori comparisons of the means.
Results
Effects of Assemblage Component Removal on NPP
Removal of canopy and subcanopy components from macroalgal assemblages in New Zealand and Oregon (USA) showed that individual components (i.e., canopy only and subcanopy only) had saturating or photoinhibition responses to increasing irradiance, whereas intact assemblages showed a continuous rise in productivity (Figure 1). The canopy and subcanopy components of upper shore assemblages in New Zealand (Figure 1A) had saturating relationships with increasing irradiance, but intact assemblages had reduced productivity at low irradiance and elevated productivity at high irradiance relative to the canopy alone. Upper shore intact assemblages in Oregon (Figure 1B) had a similar continuous increase in productivity with irradiance, but the canopy and subcanopy components showed saturation at high irradiance. Lower shore assemblages in New Zealand (Figure 1C) and Oregon (Figure 1D) had saturation responses in canopy and subcanopy components, and a continuous rise in intact assemblage productivity with irradiance. While the canopy alone generally exceeded productivity of the intact assemblages at low to moderate irradiance, the presence of the subcanopy buffered the effects of saturation or photoinhibition of photosynthesis at high irradiance.
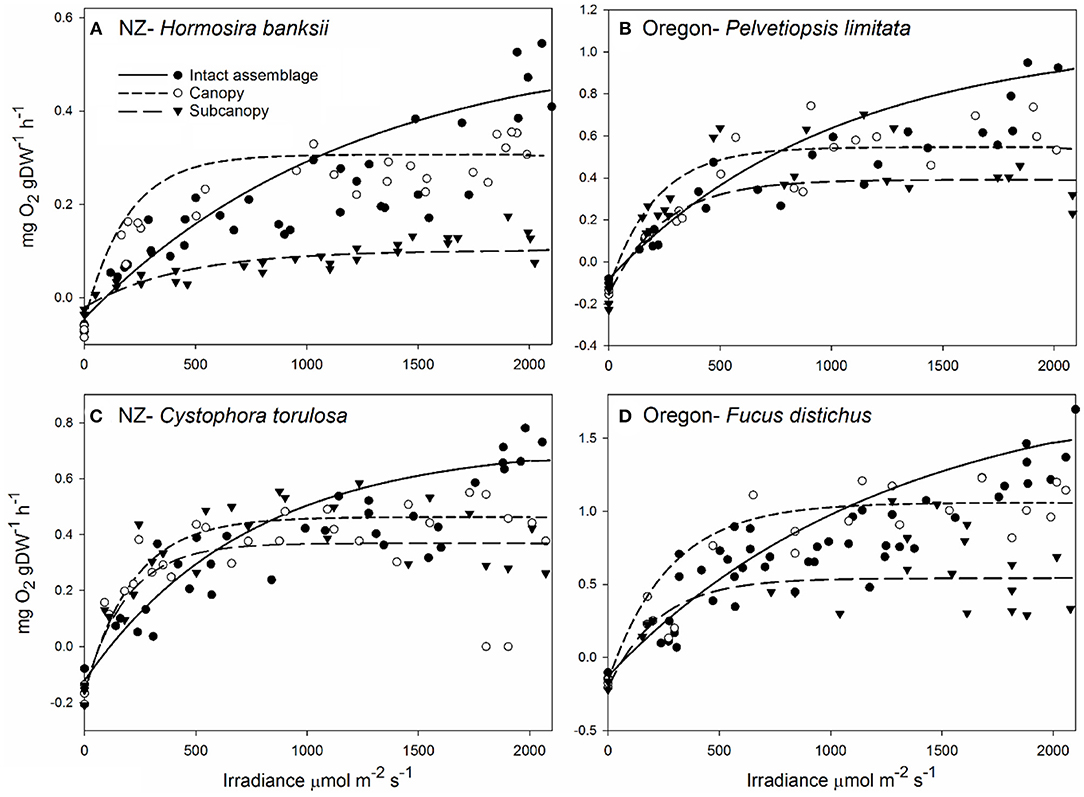
Figure 1. Primary productivity of four macroalgal assemblages in New Zealand and Oregon across a full range of natural irradiance. Assemblages were experimentally manipulated by removing components to examine the contribution of the canopy and subcanopy assemblages compared to intact assemblages. Treatments were intact assemblages (controls, solid line), canopy only (small dashed line), and subcanopy only (large dashed line). The four assemblages were the mid-high intertidal assemblages dominated by Hormosira banksii [(A), New Zealand] and Pelvetiopsis limitata [(B), Oregon, USA], and the mid-low intertidal assemblages dominated by Cystophora torulosa [(C), New Zealand] and Fucus distichus [(D), Oregon, USA]. Data were standardized by dry biomass (mg O2 gDW−1 h−1). Note the different scales of y-axes.
Analysis of the photosynthetic parameters across canopy treatments and shore heights showed that low shore New Zealand and Oregon (USA) macroalgal assemblages had higher photosynthetic efficiency (α), maximum productivity (Pm), and respiration (R) (Figure 1, Supplementary Tables 1, 2). Maximum productivity (Pm) and compensating irradiance (Ec) varied between treatments for both New Zealand and Oregon (USA) assemblages. Pm was significantly different between all canopy treatment combinations for both New Zealand and Oregon (USA) assemblages (Supplementary Tables 1, 2) and Ec was significantly lower in intact assemblages compared to the subcanopy alone in both New Zealand and Oregon (USA). New Zealand assemblages also showed lower respiration in the subcanopy compared to both the canopy alone and intact assemblages (Supplementary Table 1). There was also significantly higher photosynthetic efficiency (α) of the canopy alone compared to the subcanopy in New Zealand assemblages (Supplementary Table 1).
NPP and Assemblage Recovery
During the long-term experiments in New Zealand there was considerable variation in the productivity–irradiance (P-E) curves, among treatments and among times within treatments (Figure 2). Intact assemblages (Figure 2A) had consistent productivity dynamics across the 2-year study period. However, the saturating curves represent a relatively poor fit as highlighted by the gray shaded areas (Figure 2A), including: (i) under estimation of photosynthetic efficiency at low irradiance, particularly during winter, (ii) over estimation of photosynthesis at moderate light intensities, and (iii) under estimation of photosynthesis at high irradiance (Figure 2A). Removal of the midcanopy component caused a decline in assemblage productivity relative to control (i.e., intact assemblage) levels (Figure 2B), but showed recovery to control levels at 12 months. Removal of the canopy caused the greatest reduction in productivity (Figure 2C) and had not recovered to control levels after 24 months. Removal of the basal component also caused an initial drop in productivity, but this recovered to control levels by 12 months (Figure 2D).
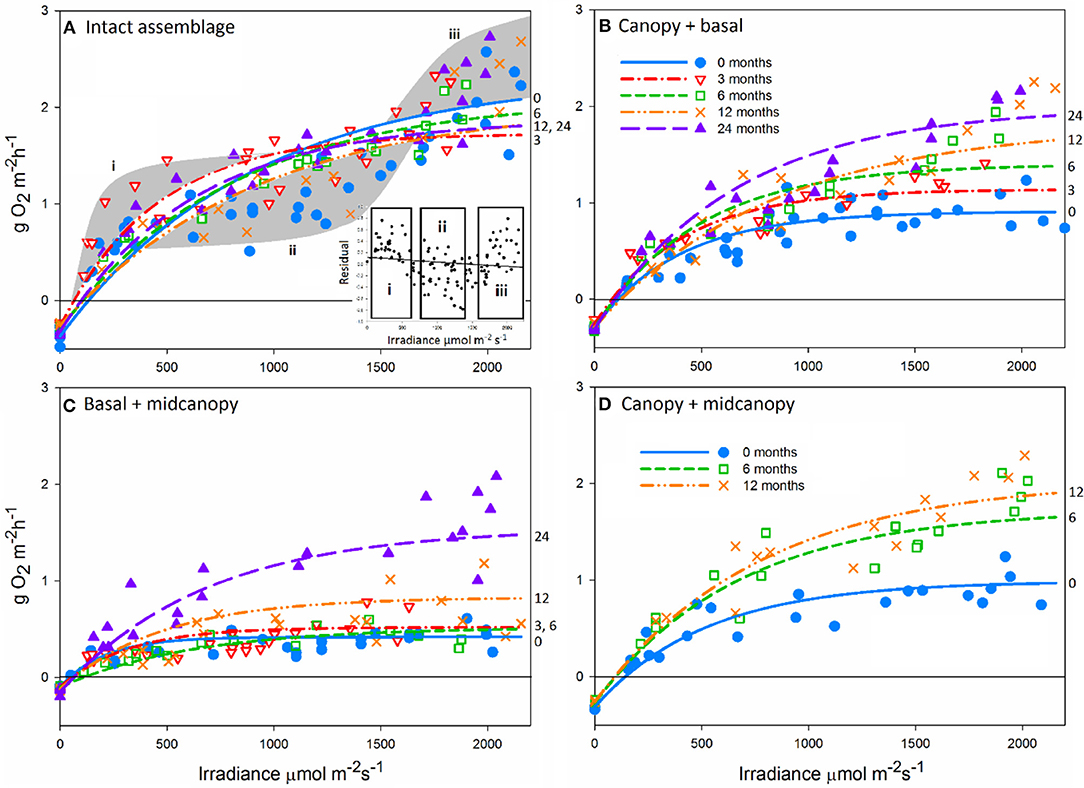
Figure 2. Productivity-irradiance (P-E) curves through time for experimentally manipulated in situ assemblages (standardized by reef area g O2 m−2 h−1), including intact assemblages (A), the canopy + basal [midcanopy removed; (B)], basal + midcanopy [canopy removed; (C)], and canopy + midcanopy [basal removed; (D)] treatments. All treatment assemblages fitted by saturating photosynthetic curves (Walsby, 1997) for each sampling period. Intact assemblages show relatively poor fit to saturating photosynthetic curves as highlighted by the gray shaded areas (A). The canopy + midcanopy treatment was initiated 12 months after other treatments and has P-E curves at 0, 6, and 12 months after treatment initiation. The gray area represent the deviation of residuals from predicted as represented by the inset graph on (A).
Analysis of changes in photosynthetic parameters over time for each canopy treatment showed little difference in maximum photosynthesis (Pm) for intact assemblages (Table 1). However, “canopy + basal,” and “canopy + midcanopy,” and “basal + midcanopy” had variable Pm during the recovery period associated with a large drop in NPP after the loss of midcanopy, basal and canopy components, respectively (Table 1; in all cases the reference for time 0 is the control, intact assemblage). Photosynthetic efficiency of intact assemblages (α) varied with seasonal sampling, particularly during winter (at 3 months). Increasing efficiency is indicative of adapting to lower ambient light intensities. All treatments had variable efficiency over time, with α generally increasing with the recovery of assemblages. Respiration rate (R) increased over time with the recovery of the “basal + midcanopy” treatment, but did not change significantly in all other treatments. Compensating irradiance (Ec), the point at which algae go from net respiration into net production, also varied at the 3-month winter sampling period, with lower compensating irradiance than during summer for the intact assemblages. Compensating irradiance (Ec) is calculated from α and R, and given the highly significant differences in α over time suggests that variation in light-use efficiency (α) was the main driver of Ec rather than R.
To gain a clearer view of NPP among treatments across times, we analyzed average productivity within three irradiance bins: low (100–200 μmol m−2s−1), mid (800–1200 μmol m−2s−1), and high (>1600 μmol m−2s−1) (Figures 3A–C, Supplementary Table 3). NPP per area varied relatively little over time at mid and low irradiances, except for peaks at 3 months in most treatments due to greater photosynthetic efficiency during winter (Figures 3A,B). The canopy removal treatment (basal + midcanopy) had lower NPP throughout the 24-month period. It was clear that at high irradiance for treatments with canopies, overall NPP was far greater than that at low and mid irradiance, reflective of the non-saturating curves seen in Figure 1. Statistically, there were significant interactions between irradiance and treatment (F4, 90 = 21.3, p < 0.001), recovery time and treatment (F8, 90 = 3.5, p = 0.001), and irradiance and time (irradiance x time F8, 90 = 2.2, p = 0.03). The interaction between irradiance and treatment was associated with increasing differences in NPP of treatments, particularly the narrowing gap between NPP of the basal + midcanopy treatment compared to intact assemblages during recovery.
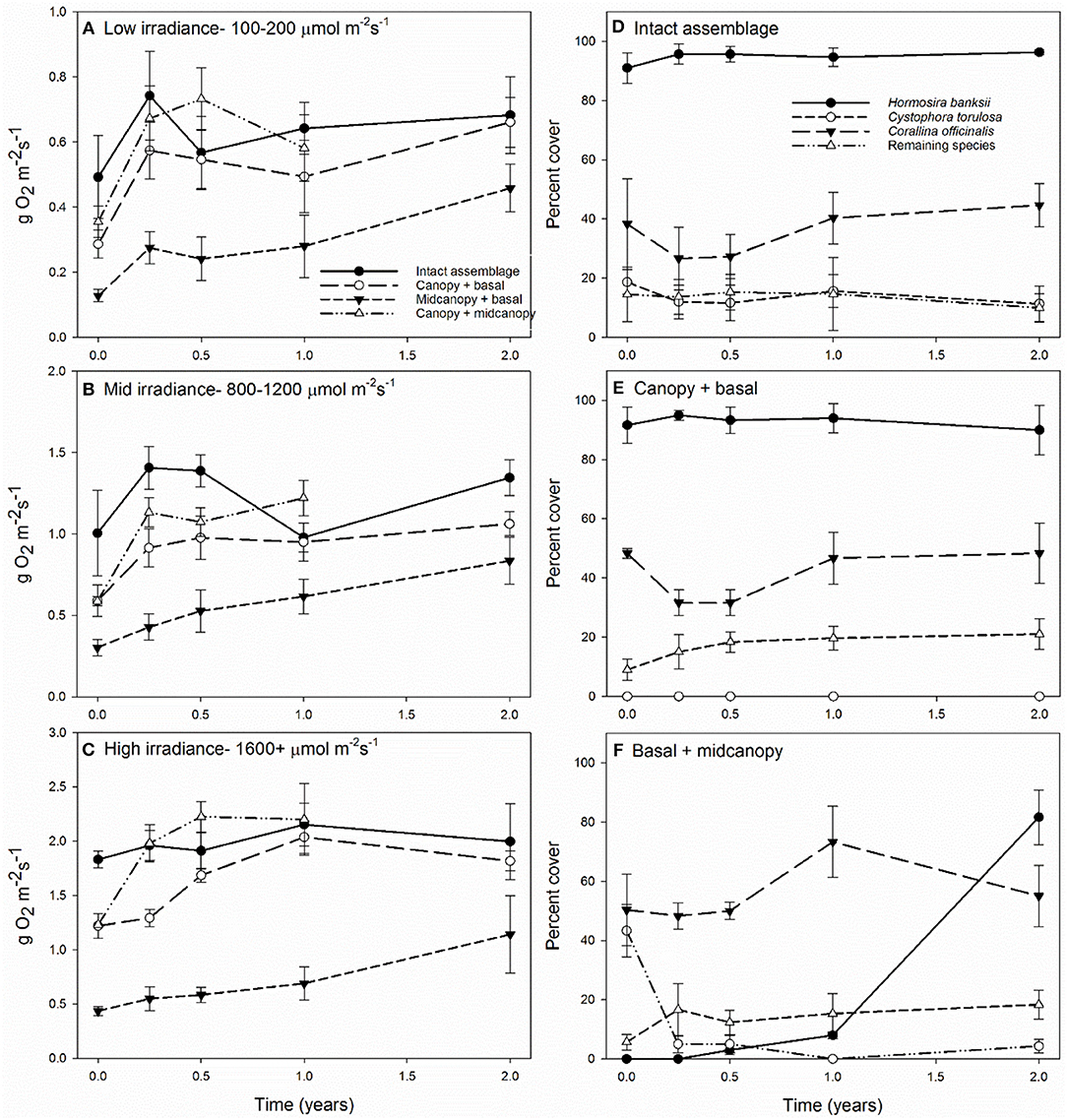
Figure 3. Primary productivity (±SE) at 0, 3, 6, 12, and 24 months after removal of assemblage components at low 100–200 μmol m−2 s−1 (A), mid 800–1200 μmol m−2 s−1 (B) and high 1600+ μmol m−2 s−1 (C) irradiance levels; and the change in percent cover of the dominant assemblage components for intact assemblages (D), canopy + basal (E), and basal + midcanopy (F). Percent cover shown for Hormosira banksii, Cystophora torulosa, Corallina officinalis, with all remaining species summed for a combined percent cover.
The persistently dominant species were H. banksii in the canopy, Corallina officinalis on the primary substratum, and C. torulosa in the midcanopy (Figures 3D,E). Most of the “remaining species” were ephemeral algae that came and went through time and were particularly evident in the basal + midcanopy treatment where the canopy had been removed (Figure 3F). It is these species that comprised most of the macroalgal species richness of the assemblages (Figure 4). Although the identity of these ephemeral species varied over time, the overall cover of these species changed little throughout the 2-year period and there was little difference in total cover between treatments. After the removal of the canopy (basal + midcanopy), the cover of several species declined greatly, including C. torulosa (Figure 3F) and Carpophyllum maschalocarpum (Figure 4). These species form canopies on the lower shore (C. torulosa) and the immediate subtidal zone (C. maschalocarpum), but occur in the subcanopy beneath H. banksii in the mid-high intertidal zone. The presence and cover of individual ephemeral species varied between treatments with several ephemeral species recruiting in relatively high densities into the basal + midcanopy plots. The delayed recovery of NPP in the canopy removal treatments (basal + midcanopy in Figures 3A–C) was associated with the slow recruitment of the dominant canopy-former H. banksii (Figure 3F). There were no significant changes in % cover over time in intact assemblages and canopy + basal treatments (F4, 90 = 0.30, p = 0.87 and F4, 90 = 0.44, p = 0.78 respectively), but there was a significant recovery in cover of some species (H. banksii) and decline in others (C. torulosa) in the basal + midcanopy treatment, as indicated by significant interaction between species and time (F12, 90 = 11.6, p < 0.0001).
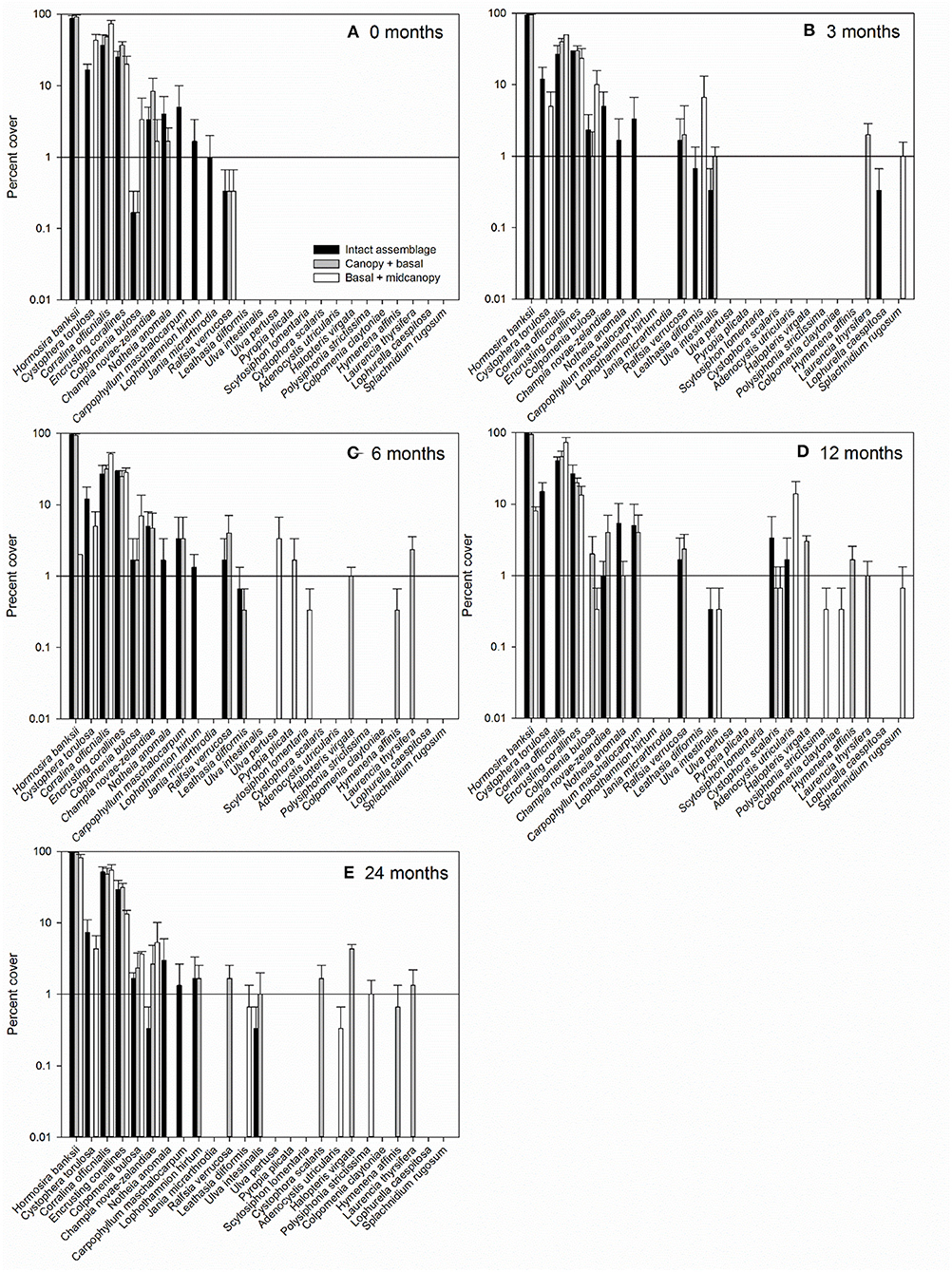
Figure 4. Average percent cover (±SE) of macroalgal species within control (intact assemblage) and experimental plots (canopy + basal, basal + midcanopy) 0 months (A), 3 months after removal (B), 6 months (C), 12 months (D), and 24 months after removal (E). Y-axis is log10 scale to better visualize presence of macroalgae with low percent cover.
Influence of Subcanopy Irradiance on Assemblage Productivity
Across a full range of light intensities, understory irradiance was only about 4% of above-canopy light levels (Figure 5A). Understory irradiance varied little when ambient light was less than c. 1,000 μmol m−2 s−1, but was increasingly variable beyond c. 1,200 μmol m−2 s−1. This variation was due to the movement of the canopy with water motion, constantly covering and exposing the subcanopy to shade and light flecks. Furthermore, the reduced distance that light must travel through the water column at midday means that the highest intensities and highest differential between sunlit and shaded intensities occur simultaneously. At reduced ambient light intensities, diffuse radiation caused by scattering of light by cloud cover reduced light flecks and shade, limiting variability in subcanopy irradiance. Compensating irradiance (Ec) of the subcanopy assemblage (at c. 30 μmol m−2 s−1) was reached beneath the canopy only when ambient light was around 400–800 μmol m−2 s−1, coincident with the intensity at which NPP of the full assemblage approximated that of the canopy alone and the point at which the subcanopy (midcanopy + basal) contributed positively to whole assemblage productivity (Figure 5B).
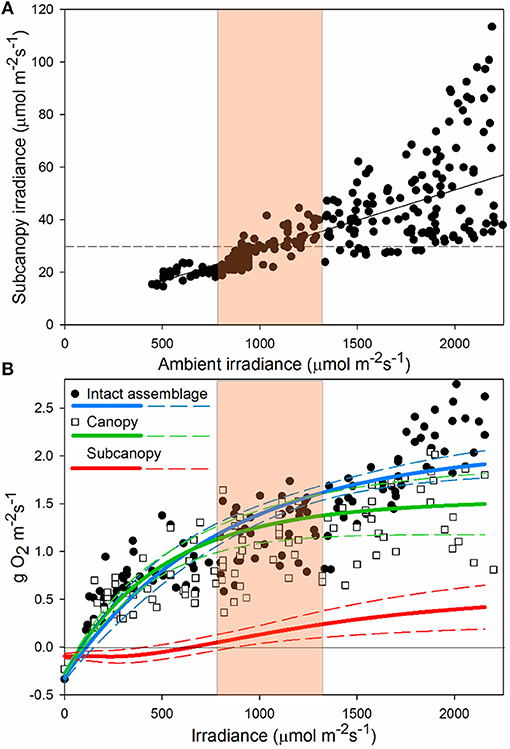
Figure 5. Relationship between ambient and subcanopy irradiance (A); dotted line indicates the average compensation point (irradiance level where photosynthesis and respiration are equal) of the subcanopy assemblage. Here we show the linear fit (F1, 304 = 342.5, P < 0.0001, r2 = 0.53), indicating increasing understory light levels and variance with increasing ambient light. Panel (B) shows NPP of “intact assemblages” and the “canopy” treatment, and the estimated contribution of the “subcanopy” to full assemblage NPP. P-E curves -(solid lines) and ± 1SD (dashed lines), are plotted for “intact assemblages” and the “canopy” treatments, with the difference between the two curves interpreted as “subcanopy” contribution to production. Highlighted on both graphs is the region of irradiance at which average subcanopy irradiance reaches compensation point of the subcanopy assemblage (A) and the corresponding point where productivity of intact assemblages deviates from assemblages with the subcanopy removed (B).
Discussion
Although there is an increasing literature on the importance of light distribution within macroalgal canopies on assemblage production dynamics [for review see (Binzer et al., 2006; Tait and Schiel, 2011; Tait et al., 2017)], there have been few attempts to understand whole assemblage and subcanopy production dynamics in situ [although see (Miller et al., 2011; Tait and Schiel, 2011; Harrer et al., 2013)]. Our study indicates that when fitted to full assemblages saturating curves (Walsby, 1997) often overestimated production at low light intensities and underestimated production at high light intensities. In particular, we note a potential tipping point beyond c. 600–1000 μmol m−2 s−1, above which NPP of complete assemblages increases beyond saturation of photosynthesis in the canopy. Although such a response fails to fit within a typical saturating relationship for photosynthesis, similar responses have been reported for terrestrial forest canopies (Mission et al., 2007; Mercado et al., 2009). The strong radiation gradient within canopies results in a low-efficiency, light saturated canopy, and a high-efficiency, light-limited subcanopy. This relationship is the basis for the “two-big-leaf” model for whole canopy photosynthesis which accounts for the photo-acclimated state of sunlit and shaded leaves (Dai et al., 2004). While such models are yet to be integrated into the whole ecosystem photosynthesis of marine macroalgae, results from our study clearly show that models of whole assemblage photosynthesis for macroalgae require more detailed assessment of the subcanopy contribution including radiative transfer of the canopy and separate integration of sunlit and shaded assemblage components (Dai et al., 2004).
Under a clear sky the canopies of terrestrial plants are often light saturated, yet increasing light to the shaded subcanopy buffers saturation of photosynthesis or photoinhibition, leading to a continuous rise in assemblage photosynthesis with increasing irradiance (Mercado et al., 2009). Macroalgal assemblages with canopy and subcanopy components responded in much the same way, with canopies alone prone to saturation of photosynthesis or photoinhibition. The presence of the subcanopy assemblage increased assemblage productivity above that of the canopy alone at high irradiance (i.e., above c. 1200 μmol m−2 s−1). Furthermore, the point at which whole assemblage photosynthesis exceeded that of the canopy alone was associated with crossing of the compensating light threshold of the subcanopy and an increase in the variability of light delivery. Increasing variability of irradiance in the subcanopy was associated with light transmission through canopies under clear skies where the differential between shade and sunlit intensities is greater than under diffuse radiation associated with cloud cover (Roderick et al., 2001).
Although the mechanisms resulting in an apparent high irradiance threshold in assemblage primary productivity are unclear, the variability in light delivery at high ambient intensities may greatly increase the photon flux density to the subcanopy in such a way that saturation or photoinhibition of whole assemblage photosynthesis does not occur. However, individual photosynthetic elements within an assemblage will undoubtedly be experiencing saturation or photoinhibition (Tait et al., 2017). Heterogeneous resources have been predicted as an important driver of ecosystem function (Tylianakis et al., 2008), and variable light delivery (i.e., flecking) to macroalgal thalli has been shown to increase rates of primary productivity relative to static light conditions (Dromgoole, 1988). Furthermore, there is increasing evidence that the diversity of photosynthetic pigments (Stomp et al., 2007; Striebel et al., 2009) and diversity of morphologies (Tait et al., 2014) within marine algae promote complementarity and enhance overall assemblage production through more complete use of the PAR spectrum (Tait et al., 2017). As more marine photosynthetic research leaves the confines of the laboratory, we believe the fine-scale dynamics of light distribution and delivery will be of utmost importance in determining the impacts of global changes on functioning of marine macrophyte assemblages.
There is substantial evidence that in the temperate marine intertidal environment, many ecosystem functions are largely dependent on canopy-forming algae (Bertness et al., 1999; Bruno et al., 2003; Schiel, 2006; Stachowicz et al., 2008). The removal of these species has a major effect on function, and in some cases these species have been shown to be functionally irreplaceable (Schiel, 2006). Despite the presence of functionally similar species in our study (i.e., perennial canopy-forming fucoid algae), no species replaced the role of Hormosira banksii as a canopy dominant (Schiel and Lilley, 2011), and recovery of NPP following canopy loss occurred only after H. banksii recruits re-established in these assemblages. Canopy-forming fucoids are autogenic engineers or foundation species due to their role in maintaining diversity (Bertness et al., 1999; Bruno et al., 2003; Schiel, 2006), and our results indicate that their role in primary productivity is a key ecosystem function that other species are unable to fulfill in much of the intertidal zone.
Niche complementary is often invoked to describe patterns of diversity and ecosystem function, and may explain how species-rich assemblages are able to use resources such as nitrogen (Bracken and Stachowicz, 2006) and light (Stomp et al., 2007; Striebel et al., 2009; Tait et al., 2014) more effectively than species-poor assemblages. Such complementarity is most readily observed in realistic assemblages (Bracken et al., 2008; Bracken and Williams, 2013), highlighting the importance of species composition and, in the case of light use, the importance of canopy structure. Loss of both canopy and subcanopy components greatly affected production dynamics in the short-term, but a suite of ephemeral algae largely compensated for the loss of the dominant perennial subcanopy species within 6–12 months. Recovery following canopy loss was markedly slower and only occurred after the dominant canopy species H. banksii recruited in high densities. Combined, these results show the critical role of canopy-forming macroalgae in maintaining function and assemblage composition, but also reveal a degree of functional redundancy in the spatially and temporally variable subcanopy assemblage.
Author Contributions
LT and DS wrote the manuscript text, contributed to the experimental design, completed the data collection, analyzed the data. LT prepared the figures.
Conflict of Interest Statement
The authors declare that the research was conducted in the absence of any commercial or financial relationships that could be construed as a potential conflict of interest.
Acknowledgments
We thank Paul South, Kerry South, and Stacie Lilley for help in the field, Bruce Menge for assistance during our North American research, the University of Canterbury for scholarship support, and the Andrew W Mellon Foundation of New York, the New Zealand Foundation for Research, Science and Technology (Coasts and Oceans OBI programme), and the National Science Challenge Sustainable Seas for research funding.
Supplementary Material
The Supplementary Material for this article can be found online at: https://www.frontiersin.org/articles/10.3389/fmars.2018.00444/full#supplementary-material
References
Anderson, W. B., and Polis, G. A. (1998). Marine subsidies of island communities in the gulf of California: evidence from stable carbon and nitrogen isotopes. Oikos 81, 75–80. doi: 10.2307/3546469
Anthony, K., Ridd, P. V., Orpin, A. R., Larcombe, P., and Lough, J. (2004). Temporal variation of light availability in coastal benthic habitats: effects of clouds, turbidty, and tides. Limnol. Oceanogr. 49, 2201–2211. doi: 10.4319/lo.2004.49.6.2201
Arkema, K. K., Reed, D. C., and Schroeter, S. C. (2009). Direct and indirect effects of giant kelp determine community structure and dynamics. Ecology 90, 3126–3137. doi: 10.1890/08-1213.1
Attard, K. M., Glud, R. N., McGinnis, D. F., and Rysgaard, S. (2014). Seasonal rates of benthic primary production in a Greenland Fjord measured by aquatic eddy correlation. Limnol. Oceanogr. 59, 1555–1569. doi: 10.4319/lo.2014.59.5.1555
Bertness, M. D., Leonard, G. H., Levine, J. M., Schmidt, P. R., and Ingraham, A. O. (1999). Testing the relative contribution of positive and negative interactions in rocky intertidal communities. Ecology 80, 2711–2726. doi: 10.1890/0012-9658(1999)080[2711:TTRCOP]2.0.CO;2
Binzer, T., and Sand-Jensen, K. (2002). Production in aquatic macrophyte communities: a theoretical and emperical study of the influence of spatial light distribution. Limnol. Oceanogr. 47, 1742–1750. doi: 10.4319/lo.2002.47.6.1742
Binzer, T., Sand-Jensen, K., and Middelboe, A.-L. (2006). Community photosynthesis of aquatic macrophytes. Limnol. Oceanogr. 51, 2722–2733. doi: 10.4319/lo.2006.51.6.2722
Bracken, M. E., Friberg, S. E., Gonzalez-Dorantes, C. A., and Williams, S. L. (2008). Functional consequences of realistic biodiversity changes in a marine ecosystem. Proc . Natl. Acad. Sci. U.S.A. 105, 924–928. doi: 10.1073/pnas.0704103105
Bracken, M. E., and Stachowicz, J. J. (2006). Seaweed diversity enhances nitrogen uptake via complementary use of nitrate and ammonium. Ecology 87, 2397–2403. doi: 10.1890/0012-9658(2006)87[2397:SDENUV]2.0.CO;2
Bracken, M. E., and Williams, S. L. (2013). Realistic changes in seaweed biodiversity affect mulitple ecosystem functions on a rocky shore. Ecology 94, 1944–1954. doi: 10.1890/12-2182.1
Bruno, J. F., Stachowicz, J. J., and Bertness, M. D. (2003). Inclusion of facilitation into ecological theory. Trends Ecol. Evol. 18, 119–125. doi: 10.1016/S0169-5347(02)00045-9
Bulleri, F., Bruno, J. F., Silliman, B. R., and Stachowicz, J. J. (2016). Facilitation and the niche: implications for coexistence, range shifts and ecosystem functioning. Funct. Ecol. 30, 70–78. doi: 10.1111/1365-2435.12528
Connell, S. D., and Russell, B. D. (2010). The direct effects of increasing CO2 and temperature on non-calcifying organisms: increasing the potential for phase shifts in kelp forests. Proc. R. Soc. B 277, 1409–1415. doi: 10.1098/rspb.2009.2069
Dai, Y., Dickinson, R. E., and Wang, Y. P. (2004). A two-big-leaf model for canopy temperature, photosynthesis, and stomatal conductance. J. Clim. 17, 2281–2299. doi: 10.1175/1520-0442(2004)017<2281:ATMFCT>2.0.CO;2
Dromgoole, F. I. (1988). Light fluctuations and the photosynthesis of marine algae II. Photosynthetic response to frequency, phase ratio and amplitude. Funct. Ecol. 2, 211–219.
Fischer, G., and Wiencke, C. (1992). Stable carbon isotope composition, depth distribution and fate of macroalgae from the Antarctic Peninsula region. Polar Biol. 12, 341–348. doi: 10.1007/BF00243105
Foster, M., and Schiel, D. R. (2010). Loss of predators and the collapse of southern California kelp forests (?): alternatives, explanations and generalizations. J. Exp. Mar. Biol. Ecol. 393, 59–70. doi: 10.1016/j.jembe.2010.07.002
Gattuso, J. P., Gentili, B., Duarte, C. M., Kleypas, J. A., Middelburg, J. J., and Antione, D. (2006). Light availability in the coastal ocean: impact on the distribution of benthic photosynthetic organisms and their contribution to primary productivity. Biogeosciences 3, 489–513. doi: 10.5194/bg-3-489-2006
Gollety, C., Migne, A., and Davoult, D. (2008). Benthic metabolism on a sheltered rocky shore: role of the canopy in the carbon budget. J. Phycol. 44, 1146–1153. doi: 10.1111/j.1529-8817.2008.00569.x
Harrer, S. L., Reed, D. C., Holbrook, S. J., and Miller, R. J. (2013). Patterns and controls of the dynamics of net primary production by understory macroalgal assemblages in giant kelp forests. J. Phycol. 49, 248–257. doi: 10.1111/jpy.12023
Hill, J. M., McQuaid, C. D., and Kaehler, S. (2006). Biogeographic and nearshore-offshore trends in stable isotope ratios of intertidal mussels and their food sources around the coast of southern Africa. Mar. Ecol. Prog. Ser. 318, 63–73. doi: 10.3354/meps318063
Irving, A. D., Balata, D., Colosio, F., Ferrando, G. A., and Airoldi, L. (2009). Light, sediment, temperature and the early life-history of the habitat alga Cystoseira barbata. Mar. Biol. 156, 1223–1231. doi: 10.1007/s00227-009-1164-7
Krause-Jensen, D., Markager, S., and Dalsgaard, T. (2012). Benthic and pelagic primary production in different nutrient regimes. Estuaries Coasts 35, 527–545. doi: 10.1007/s12237-011-9443-1
Lilley, S. A., and Schiel, D. R. (2006). Community affects following the deletion of habitat-forming alga from rocky marine shores. Oecologia 148, 672–681. doi: 10.1007/s00442-006-0411-6
Long, M. H., Rheuban, J. E., Berg, P., and Zieman, J. C. (2012). A comparison and correction of light intensity loggers to photosynthetically active radiation sensors. Limnol. Oceanogr. Methods 10, 416–424. doi: 10.4319/lom.2012.10.416
Mann, K. H. (1973). Seaweeds: their productivity and strategy for growth. Science 182, 975–981. doi: 10.1126/science.182.4116.975
Mercado, L. M., Bellouin, N., Sitch, S., Boucher, O., Huntingford, C., Wild, M., et al. (2009). Impact of changes in diffuse radiation on the global carbon land sink. Nature 458, 1014–1017. doi: 10.1038/nature07949
Migne, A., Gollety, C., and Davoult, D. (2015). Effect of canopy removal on a rocky shore community metabolism and structure. Mar. Biol. 162, 449–457. doi: 10.1007/s00227-014-2592-6
Miller, R. J., Reed, D. C., and Brzezinski, M. A. (2009). Community structure and productivity of subtidal turf and foliose algal assemblages. Mar. Ecol. Prog. Ser. 388, 1–11. doi: 10.3354/meps08131
Miller, R. J., Reed, D. C., and Brzezinski, M. A. (2011). Partitioning of primary production among giant kelp (Macrocystis pyrifera), understory macroalgae, and phytoplankton on a temperate reef. Limnol. Oceanogr. 56, 119–132. doi: 10.4319/lo.2011.56.1.0119
Mission, L., Baldocchi, D. D., Black, T. A., Blanken, P. D., Brunet, Y., Yuste, J. C., et al. (2007). Partitioning forest carbon fluxes with overstory and understory eddy-covariance measurments: a synthesis based on FLUXNET data. Agricult. Forest Meteorol. 144, 14–31. doi: 10.1016/j.agrformet.2007.01.006
Neushul, M. (1971). “Submarine illumination in Macrocystis,” in The Biology of Giant Kelp Beds (Macrocystis) in California, ed L. G. Jones (Lehre, Nova Hedwigia), 241–254.
Reed, D. C., and Foster, M. (1984). The effects of canopy shading on algal recruitment and growth in a giant kelp forest. Ecology 65, 937–948. doi: 10.2307/1938066
Reed, D. C., Rassweiler, A., and Arkema, K. K. (2008). Biomass rather than growth rate determines variation in net primary production by giant kelp. Ecology 89, 2493–2505. doi: 10.1890/07-1106.1
Reed, D. C., Rassweiler, A., Carr, M. H., Cavanaugh, K. C., Malone, D. P., and Siegel, D. A. (2011). Wave disturbance overwhelms top-down and bottom-up control of primary production in California kelp forests. Ecology 92, 2108–2116. doi: 10.1890/11-0377.1
Roderick, M. L. (2006). The ever-flickering light. Trends Ecol. Evol. 21, 3–5. doi: 10.1016/j.tree.2005.11.005
Roderick, M. L., Farquhar, G. D., Berry, S. L., and Noble, I. R. (2001). On the direct effect of clouds and atmospheric particles on the productivity and structure of vegetation. Oecologia 129, 21–30. doi: 10.1007/s004420100760
Sand-Jensen, K., Binzer, T., and Middelboe, A.-L. (2007). Scaling of photosynthetic production of aquatic macrophytes- a review. Oikos 116, 280–294. doi: 10.1111/j.0030-1299.2007.15093.x
Santelices, B., and Ojeda, F. P. (1984). Effects of canopy removal on the understory algal community of coastal forests of Macrocystis pyrifera from South America. Mar. Ecol. Prog. Ser. 14, 175–183. doi: 10.3354/meps014175
Schiel, D. R. (2006). Rivets or bolts? When single species count in the function of temperate rocky reef communities. J. Exp. Mar. Ecol. 338, 223–252. doi: 10.1016/j.jembe.2006.06.023
Schiel, D. R., and Lilley, S. A. (2011). Impacts and negative feedbacks in community recovery over eight years following removal of habitat-forming macroalgae. J. Exp. Mar. Biol. Ecol. 407, 108–115. doi: 10.1016/j.jembe.2011.07.004
Schiel, D. R., Steinbeck, J. R., and Foster, M. S. (2004). Ten years of induced ocean warming causes comprehensive changes in marine benthic communities. Ecology 85, 1833–1839. doi: 10.1890/03-3107
Schiel, D. R., Wood, S. A., Dunmore, R. A., and Taylor, D. I. (2006). Sediment on rocky intertidal reefs: effects on early post-settlement stages of habitat-forming seaweeds. J. Exp. Mar. Biol. Ecol. 331, 158–172. doi: 10.1016/j.jembe.2005.10.015
Stachowicz, J. J., Best, R. J., Bracken, M. S., and Graham, M. H. (2008). Complementarity in marine biodiversity manipulations: reconciling divergent evidence from field and mesocosm experiments. Proc. Natl. Acad.Sci. U.S.A. 105, 18842–18847. doi: 10.1073/pnas.0806425105
Stomp, M., Huisman, J., Stal, L. J., and Matthijs, H. C. (2007). Colorful niches of phototrophic microorganisms shaped by vibrations of the water molecule. ISME J. 1, 271–282. doi: 10.1038/ismej.2007.59
Stramski, D., and Legendre, L. (1992). Laboratory simulation of light focusing by water surface waves. Mar. Biol. 114, 341–348. doi: 10.1007/BF00349537
Striebel, M., Behl, S., Diehl, S., and Stibor, H. (2009). Spectral niche complementarity and carbon dynamics in pelagic ecosystems. Am. Nat. 174, 141–147. doi: 10.1086/599294
Tait, L. W., Hawes, I., and Schiel, D. R. (2014). Shining light on benthic macroalgae: mechanisms of complementarity in layered macroalgal assemblages. PLoS ONE 9:e114146. doi: 10.1371/journal.pone.0114146
Tait, L. W., Hawes, I., and Schiel, D. R. (2017).Integration of chlorophyll-a fluorescence and photorespirometry techniques to understand production dynamics in macroalgal communities. J. Phycol. 53, 476–485. doi: 10.1111/jpy.12524
Tait, L. W., and Schiel, D. R. (2010). Primary productivity of intertidal macroalgal assemblages: comparison of laboratory and in situ photorespirometry. Mar. Ecol. Prog. Ser. 416, 115–125. doi: 10.3354/meps08781
Tait, L. W., and Schiel, D. R. (2011). Legacy effects of canopy disturbance on ecosystem functioning in macroalgal assemblages. PLoS ONE 6:e26986. doi: 10.1371/journal.pone.0026986
Tylianakis, J. M., Rand, T. A., Kahmen, A., Klein, A. M., Buchmann, N., and Tscharntke, T. (2008). Resource heterogeneity moderates the biodiversity-function relationship in real world ecosystems. PLoS Biol. 6:e122. doi: 10.1371/journal.pbio.0060122
Veal, C. J., Carmi, M., Dishon, G., Sharon, Y., Michael, K., Tchernov, D., et al. (2010). Shallow-water wave lensing in coral reefs: a physical and biological case study. J. Exp. Biol. 213, 4304–4312. doi: 10.1242/jeb.044941
Walsby, A. E. (1997). Numerical integration of phytoplankton photosynthesis through time and depth in a water column. New Phytol. 136, 189–209. doi: 10.1046/j.1469-8137.1997.00736.x
Keywords: net primary productivity (NPP), macroalgae, ecosystem function, disturbance, compensation, complementarity, resilience, vegetation layering
Citation: Tait LW and Schiel DR (2018) Ecophysiology of Layered Macroalgal Assemblages: Importance of Subcanopy Species Biodiversity in Buffering Primary Production. Front. Mar. Sci. 5:444. doi: 10.3389/fmars.2018.00444
Received: 06 June 2018; Accepted: 05 November 2018;
Published: 26 November 2018.
Edited by:
Katherine Dafforn, Macquarie University, AustraliaReviewed by:
Mariana Mayer Pinto, University of New South Wales, AustraliaAngel Pérez-Ruzafa, Universidad de Murcia, Spain
Copyright © 2018 Tait and Schiel. This is an open-access article distributed under the terms of the Creative Commons Attribution License (CC BY). The use, distribution or reproduction in other forums is permitted, provided the original author(s) and the copyright owner(s) are credited and that the original publication in this journal is cited, in accordance with accepted academic practice. No use, distribution or reproduction is permitted which does not comply with these terms.
*Correspondence: Leigh W. Tait, bGVpZ2gudGFpdEBuaXdhLmNvLm56