The Precursor Hypothesis of Sponge Kleptocnidism: Development of Nematocysts in Haliclona cnidata sp. nov. (Porifera, Demospongiae, Haplosclerida)
- 1Research Centre for BioSystems, Land Use and Nutrition, Institute of Animal Ecology and Systematics, Justus Liebig University Giessen, Giessen, Germany
- 2Research Centre for BioSystems, Land Use and Nutrition, Institute of Applied Microbiology, Justus Liebig University Giessen, Giessen, Germany
- 3Biomedical Research Centre Seltersberg-Imaging Unit, Justus Liebig University Giessen, Giessen, Germany
- 4Department of Bioresources, Fraunhofer Institute for Molecular Biology and Applied Ecology, Giessen, Germany
Marine sponges thrive in benthic environments despite intense spatial competition and predator pressure. The sessile filter-feeders usually compensate their lack of physical defense and behavioral escape by a high level of bioactivity. In the stinging black sponge (Haliclona cnidata sp. nov.), these chemical defense mechanisms are complemented by “cellular weapons”—functional nematocysts likely acquired from cnidarians (kleptocnidism). Whereas, kleptocnidism might be facilitated by a close contact with cnidarian donors, preliminary investigations suggest that the stinging black sponge sustains nematocysts even if kept apart from cnidarians. As the underlying mechanisms causing this phenomenon remain unknown, the development of its nematocysts was studied in both presence and absence of potential cnidarian donors. First, we compared inherent nematocysts of adult sponge individuals with foreign nematocysts of co-cultivated cnidarians to identify potential donors. Second, we experimentally assessed the donor-independent and donor-dependent development of inherent and foreign nematocysts within cultures of sponge cell aggregates (SCAs). The inherent nematocysts comprised two specific types that both differed from those of the eight co-cultivated cnidarians. Specifically, we showed that the number of sponge-inherent nematocysts increased in SCAs over time in the absence of potential donors. Numbers of supplied foreign nematocysts, however, did not increase in the SCAs. We conclude that the observed increase of inherent nematocysts is due to the maturation of nematocyst precursor cells. Given these findings, we here propose the precursor hypothesis of sponge kleptocnidism. Accordingly, nematocyst precursors or immature nematocyte-nematocyst complexes might be initially acquired by sponges through filtration, maintained in sponge tissues, and nurtured to fully functioning nematocyte-nematocyst complexes. The underlying evolutionary processes are likely facilitated by bacteria-derived secondary metabolites and photosynthetically active dinoflagellates. Due to a simple body plan and the in vitro proliferation capacity of sponge cells, H. cnidata sp. nov. may serve as a novel evolutionary model system to further assess fundamental research questions regarding kleptocnidism. This study not only sheds new light on kleptocnidism in sponges, it also calls for a holobiontic view at defense mechanisms that involves the actual sponge, cnidarian nematocysts, dinoflagellate endosymbionts, and a complex microbial community.
Life Science Identifier (LSID): urn:lsid:zoobank.org:act:509A075A-5D5D-417F-811E-52D3421D48BC
Introduction
Marine sponges (phylum Porifera) are abundant and significant members of benthic environments with high ecological importance for bentho-pelagic coupling and symbiotic relationships (Taylor et al., 2007; Bell, 2008). Currently, about 8,500 sponge species are known with an estimated number of 15,000 species in total (Hooper and Van Soest, 2002; Van Soest et al., 2012). The majority belongs to the class Demospongia with Haliclona Grant, 1836 being the most species-rich genus (>450 species) (Redmond et al., 2013; Van Soest et al., 2018). Sponges are soft-bodied, sessile filter-feeders that are typically subject to spatial competition and predation by spongivores (Bell, 2008). Apart from spicules, which might function mainly as skeletal elements rather than antipredator defense (Thoms and Schupp, 2007), sponges lack true physical defense mechanisms and behavioral escape. Instead, their defense capacity is accounted for by the production of mostly bacteria-derived secondary metabolites, which can exhibit pronounced bioactivity such as antimicrobial, antifouling, and antifeedant activity, as well as cytotoxicity (Pawlik, 1993; Paul and Puglisi, 2004; Blunt et al., 2016).
In contrast to sponges, many cnidarians (phylum Cnidaria) use nematocysts (“stinging capsules”), secreted organelles from nematocytes (“stinging cells”), as defense as well as for food capture and locomotion (Kass-Simon and Scappaticci, 2002). These stinging cells are the defining autapomorphic trait of the Cnidaria (Marques and Collins, 2004) and 28 types of nematocysts have been reported (Kass-Simon and Scappaticci, 2002). Based on the alternative term for nematocyst (i.e., cnidocyst), the sum of all nematocyst types being present in a species is called cnidom (Fautin, 2009). However, some non-cnidarians like nudibranch gastropods can acquire functional nematocysts via feeding and apply these as “cellular weapons” (sensu Tardent, 1995) for their own defense (reviewed in Greenwood, 2009; Goodheart and Bely, 2017). This phenomenon is referred to as kleptocnidism and the sequestered nematocysts are called kleptocnidae. Kleptocnidism has evolved multiple times in at least four phyla—Ctenophora, Acoelomorpha, Platyhelminthes, and Mollusca (Goodheart and Bely, 2017). Among sponges, this trait has so far only been reported in a single species, the Australian taxon “Haliclona sp. 628,” which presumably sequesters the nematocysts from a coral donor (Garson et al., 1998; Russell et al., 2003). Kleptocnidism in the phylum Porifera is surprising, however, as filter-feeding sponges do not feed on cnidarian prey, which has been considered a prerequisite for sequestering nematocysts (Goodheart and Bely, 2017).
Another case of kleptocnidism in sponges potentially occurs in an undescribed sponge species—the “stinging black sponge.” In the 1990's, this Haliclona taxon appeared in tropical ornamental saltwater aquariums. It was subsequently introduced into the marine aquarium systems of the Research Center for Biosystems, Land Use and Nutrition (IFZ) at Justus Liebig University Giessen, Germany, in 2000, probably via “live rock” (i.e., coral pieces carrying algae and invertebrates). However, area of origin, natural habitat, and distribution of the sponge remain unknown. Surprisingly, handling of the sponge can cause immediate pain and microscopic analyses revealed that it contains nematocysts.
The origin of kleptocnidae in sponges has been ascribed to the uptake of nematocysts after competitive allelochemical interactions with corals (Russell et al., 2003). Moreover, for the Australian taxon Haliclona sp. 628, a single type of acroporid-like kleptocnidae has been identified (Russell et al., 2003). This implies both taxonomic specificity and defined origin of sponge nematocysts.
The situation seems to be different in the stinging black sponge, as our preliminary analyses indicate that specimens might sustain nematocysts even if kept apart from cnidarians in aquarium systems for longer periods of time. The major goal of this study is therefore to infer the mechanisms responsible for the maintenance of nematocysts in sponges. We hypothesize that nematocysts may develop in sponges without direct contact to a cnidarian donor. For testing this hypothesis, we used the undescribed stinging black sponge as a model taxon and studied the development of its nematocysts in both presence and absence of potential cnidarian donors.
Specifically, we:
• Identified the inherent nematocysts in adult sponge individuals and compared them to foreign nematocysts of eight potential cnidarian donors,
• Studied a donor-independent development of nematocysts by monitoring the number of inherent nematocysts in cultured aggregates of dissociated cells—sponge cell aggregates (SCAs)—over time in the absence of foreign nematocysts,
• Assessed a donor-dependent development of nematocysts by monitoring the number of foreign nematocysts over time in cultured SCAs that were exposed to nematocysts of the sea anemone Anemonia cf. manjano, and
• Formally described the stinging black sponge as a new species, Haliclona cnidata sp. nov., based on comparative morphological and phylogenetic analyses.
The ability of sponges to store functional nematocysts constitutes an exceptional defense mechanism that raises general questions about the complexity of their defense strategies and may shed new light on the interactions of marine organisms across phyla.
Materials and Methods
Material Studied
The stinging black sponge has been in long-term culture in the tropical marine aquarium systems of the Research Center for Biosystems, Land Use and Nutrition (IFZ) at Justus Liebig University Giessen (Giessen, Germany) since the year 2000. Material of the new species was collected directly from a tropical reef tank of the aquarium system (T5 light, salinity 33 S, 25°C, 900 L).
Nematocyst and Sponge Cell Aggregate (SCA) Analyses
Light Microscopic Investigations of Nematocysts
Nematocysts of the sponge were characterized morphologically in cross-sections of stained sponge samples [1.6% (v/v) cresyl violet acetate, Chem-Lab NV, Belgium] under a digital light microscope (VHX-2000, Keyence, Japan) and classified according to Östman (2000). The sponge nematocysts were compared to those of eight potential cnidarian donors, which had been cultivated with the stinging black sponge in the same tank for several years. The cnidarians comprised representatives of the orders Actinaria (Aiptasia diaphna, Anemonia cf. manjano, Entacmaea quadricolor), Alcyonacea (Anthelia sp., Capnella imbricata), Scleractinia (Acropora tumida, Montipora aequituberculata), and Zoantharia (Protopalythoa heliodiscus). Nematocysts were investigated in squash preparations of the cnidarian tissues (Figure S1). Samples were inspected with the Keyence microscope and nematocyst dimensions were obtained from 10 nematocysts per type. The Supplementary Video was recorded with the Keyence microscope and processed in DaVinci Resolve (version 14.1.0.108, Blackmagic Design, Australia).
Generation of Sponge Cell Aggregates
Sponge cell aggregates (SCA) were generated by dissociating sponge samples into single cells (modified after Custodio et al., 1998). Briefly, freshly collected sponge samples (ca. 10 g) were cut into 1 mm3 cubes with a sterile scalpel and washed for 20 min at 20°C with calcium- and magnesium-free artificial seawater containing 20 mM ethylenediaminetetraacetic acid (CMFSW-E, after Müller et al., 1976). The supernatant was discarded and replaced by fresh CMFSW-E. This washing step was repeated three times each for 40 min. The solution was then filtered through a 48 μm nylon mesh and centrifuged for 5 min at 500 g. The supernatant was discarded and the pellet was suspended in source seawater, supplemented with 100 μg mL−1 each of penicillin and streptomycin (Carl Roth GmbH, Germany). A volume of 6 mL of sponge cell suspension was transferred to sterile petri dishes. The dishes were placed on a rotary shaker (Thermomixer comfort, Eppendorf, Germany) for 48 h to facilitate the formation of SCAs.
Quantification of Sponge Inherent Nematocysts in SCA
To assess the development of inherent nematocysts, their number was quantified in SCAs over time. Twelve SCAs per dish (n = 8) were aliquoted into Greiner CELLSTAR® multiwell culture plates (Sigma-Aldrich, USA) containing 1 mL seawater supplemented with antibiotics (each 100 μg mL−1, see section Generation of Sponge Cell Aggregates). The plates were placed on a rotary shaker. Daily, one third of water was exchanged with fresh seawater supplemented with antibiotics (each 100 μg mL−1). After 4 days, the concentration of each antibiotic was increased to 110 μg mL−1. The number of nematocysts in the SCAs was then analyzed daily over a period of 8 days. Each day 8 SCAs were analyzed by subjecting one randomly chosen SCA per well to a consumptive microscopic analysis. For each SCA (n = 64) 4–14 images were acquired with the Keyence microscope at 500x magnification. Images were overlayed with a digital grid (50 × 50 μm) and the grid cells were assigned to one of three categories, (1) no sponge cells or inherent nematocyst, (2) sponge cells only, (3) sponge cells and inherent nematocyst. The numbers of nematocysts were normalized by extrapolating them to 200 grids with sponge cells. Nematocyst numbers were tested for significant differences between start and end of the experiment using the Wilcoxon rank sum test, as the data did not follow a normal distribution according to the Shapiro–Wilk test. Data analysis and generation of box-and-whiskers-plots were performed in RStudio (v. 1.1.423; R Studio Team, 2016).
Quantification of Foreign Nematocysts in SCA
The potential of foreign nematocysts to develop in the sponge was assessed by exposing SCAs to different concentrations of foreign nematocyst from Anemonia cf. manjano. The anemone has been selected due to its characteristic nematocysts and abundance in the tanks. SCAs were generated and analyzed according to the quantification protocol for inherent nematocysts. Anemone nematocysts were extracted using the modified protocol of McKay and Anderson (1988). Freshly collected tentacles of the anemone were sampled and transferred to a reaction tube containing seawater. To extract the nematocysts from the tissue without triggering a discharge, the tentacles were gently macerated with a mortar in the reaction tube. The suspension was centrifuged for 3 min at 2,000 g and the supernatant discarded. The obtained pellet was suspended in 70% (v/v) Percoll (Sigma-Aldrich, USA) in seawater and incubated for 5 min. The sample was centrifuged again for 3 min at 2,000 g, the supernatant was discarded and the pellet was suspended in 100 μL seawater. The extraction was performed 20 times to obtain a sufficient volume of anemone nematocyst suspension. The actual experiment comprised three treatments (each replicated once) plus one control and was performed over a period of 9 days. First, sponge cell suspensions were separated in petri dishes (n = 7) and exposed to anemone nematocyst suspensions in concentrations of 0 μL (C0, control), 100 μL (C100), 250 μL (C250), and 500 μL (C500) for 48 h. Then, SCAs were transferred and examined according to the quantification of sponge inherent nematocysts (see section Quantification of Sponge Inherent Nematocysts in SCA). For the assignment of the grid cells, the three categories (1) no sponge cells or inherent nematocyst, (2) sponge cells only, (3) sponge cells and inherent nematocyst were expanded by the category (4) sponge cells and foreign nematocyst.
Morphological and Skeletal Analyses of the Sponge
Morphological characteristics including growth form, body color, surface structure, skeletal architecture, as well as shape and size of the spicules were examined following the Systema Porifera guidelines (Hooper and Van Soest, 2002). Accordingly, the skeletal architecture was inspected in hand-cut sections of the ectosome and the choanosome with the Keyence digital microscope. The systematic assignment follows the “Systema Porifera” (Hooper and Van Soest, 2002). In addition, morphological characteristics were compared to descriptions of two potentially closely related species (Haliclona sp. 628, Garson et al., 1998; Russell et al., 2003; Haliclona vanderlandi De Weerdt and Van Soest, 2001).
For the analysis of spicules, two sponge samples were treated with nitric acid using a standard protocol (Kelly-Borges and Pomponi, 1992). Briefly, the sponge material was dissolved in nitric acid and spicules were concentrated by centrifugation. The nitric acid was then replaced by distilled water and samples were washed with ethanol and air-dried. Dimensions of spicules (n = 335) were measured as length × width [minimum – mean (standard deviation) – maximum] and subjected to frequency distribution analysis (histogram) to determine size categories.
For ultrastructural characterization by scanning electron microscopy (SEM) freshly collected sponge samples of two specimens were washed with 0.2 μm filtered, autoclaved seawater and pre-fixed for 1 h at 4°C in a solution of 0.1 M sodium cacodylate buffer (CB, pH 7.4) containing 8% sucrose, 1.5% paraformaldehyde, and 2.5% glutaraldehyde. Samples were then rinsed in CB buffer at 4°C, and cross-sections were prepared with a sterile razor blade and immersed in the fixative overnight at 4°C. After several washes in buffer, specimens were incubated in 2% OsO4, washed again in buffer and subsequently in dd H2O. Specimens were dehydrated in an ethanol series [30, 50, 70, 80, 90, 96, 100% (v/v)] (20 min each) after osmium fixation, critical point dried, mounted on SEM holders and gold sputtered. Samples were observed in a Zeiss DSM982 (Carl Zeiss AG, Germany) field emission scanning electron microscope (FESEM) at 3–5 kV. Images were recorded using a secondary electron (SE)-detector with the voltage of the collector grid biased to + 300 V in order to improve the signal-to-noise ratio and to reveal optimal topographical contrast. For element analysis, energy-dispersive X-ray microanalysis (EDX) was done at 15 kV acceleration voltage using a 10 mm2 Si(Li) detector (Oxford instruments plc, UK).
DNA Extraction, PCR, and Sequencing
Freshly collected sponge material was used for DNA isolation with the DNeasy Blood & Tissue Kit (Qiagen, Germany) according to the manufacturer's instructions. PCR amplifications of the mitochondrially encoded cytochrome c oxidase subunit I (COI) gene and the nuclear small-subunit ribosomal RNA (18S rRNA) gene were performed with the primers dgLCO149 and dgHCO2198 (Meyer et al., 2005) for COI, and primers A and B (Medlin et al., 1988), including the internal primer 400F18S (Redmond et al., 2007) and its reverse-complement, for the 18S rRNA gene. Fragments were amplified in 50 μL reactions comprising 1x DreamTaq Buffer, 0.1 mM dNTPs, 0.4 μM (for COI) and 0.5 μM (for 18S) of primers, 0.4 mg mL−1 BSA, 0.02 U μL−1 DreamTaq and DNase-free water (all reagents from Thermo Fisher Scientific, USA). Cycling conditions for COI gene amplification were as follows: initial denaturation at 95°C for 2 min followed by 37 cycles of denaturation for 40 s at 94°C, annealing for 40 s at 42°C and extension for 60 s at 72°C, and a final extension step of 10 min at 72°C. The 18S rRNA gene was amplified with the following cycling conditions. An initial denaturation step at 95°C for 2 min followed by 30 cycles of denaturation for 1 min at 95°C, annealing for 1 min at 60°C and extension for 90 s at 72°C, and a final extension step of 10 min at 72°C. Amplicon sizes were checked by agarose gel electrophoresis. Samples were purified with the QIAquick PCR Purification kit (Qiagen, Germany) according to the manufacturer's instructions and sent for Sanger sequencing (LGC Genomics, Germany). Sequences were submitted to the Sponge Barcoding Database (Wörheide et al., 2007) and GenBank (accession numbers MH373530, MH396488).
Phylogenetic Analyses
For phylogenetic reconstruction, datasets of 68 COI and 76 18S rRNA gene sequences were assembled based on GenBank BLAST searches and the studies of Redmond et al. (2011, 2013) (see Tables S1, S2). The datasets were not concatenated due to insufficient overlapping data for both genes. The alignment of the COI sequences was performed with the ClustalW algorithm in MEGA 5.2.2 (Tamura et al., 2011) and cropped to a length of 581 bp. For the 18S rRNA gene alignment, the online version of the multiple sequence alignment program MAFFT (version 7; Katoh and Standley, 2013) was used applying the Q-INS-i iterative refinement methods (Katoh and Toh, 2008) to account for secondary structure information. Regions with a sequence variability of >50% were excluded from the analyses, leaving a final alignment of 1,561 nucleotide positions.
Substitutional saturation was tested in DAMBE 6.4.4.1 (Xia and Lemey, 2009) according to Xia et al. (2003). Both datasets showed only little saturation even under the unlikely assumption of an asymmetrical tree (COI: Iss = 0.17, Iss.c = 0.36; 18S: Iss = 0.30, Iss.c = 0.50). The subsequent phylogenetic analyses were performed within a Bayesian framework in MrBayes 3.2.1 (Huelsenbeck and Ronquist, 2001). The General Time Reversible model incorporating invariant sites and gamma distribution (GTR+I+G) was inferred for both datasets using jModelTest 2.1.4 (Darriba et al., 2012) and applying the Akaike information criterion (AIC). For the COI analysis, settings were specified as follows: I = 0.4270, G = 0.6680, mcmc Nruns = 2, ngen = 50,000,000, samplefreq = 1,000, nchains = 4, and burnin = 0.1. Eunapius subterraneus (GenBank accession number FJ715439) was defined as outgroup. The analysis was stopped after 4,516,000 generations when it had reached an average standard deviation of split frequencies (ASDSF) of 0.01. For the 18S rRNA analysis, the same settings were chosen but priors were adjusted to I = 0.5300 and G = 0.6650. Aplysina fistularis (KC902180) was defined as outgroup. The analysis was stopped when the two parallel runs reached convergence (i.e., ASDSF < 0.005) at 12,842,000 generations. The likelihood of Effective Sample Sizes (ESSs) of the two parallel runs for both COI and 18S rRNA analyses were above 200, as inferred by Tracer v1.6 (Rambaut et al., 2014). Maximum clade credibility trees were visualized in FigTree v1.4.3 (http://tree.bio.ed.ac.uk/).
Results
Inherent Nematocysts of Adult Sponges and Potential Cnidarian Donors
Microscopic investigations revealed the occurrence of numerous, mostly intact nematocysts in adult sponge individuals (Figure 1). The nematocysts were either loosely distributed within the sponge mesohyl or occurred in clusters (Figure 1A). An in situ discharge of these inherent nematocysts could be observed following chemical (i.e., ethanol exposure; Figures 1B–D) or mechanical stimuli (i.e., stimulation of the sponge surface with a needle; Supplementary Video). The cnidom of the stinging black sponge comprised two types of nematocysts that could be distinguished morphologically. Nematocyst type 1 had a size of 41.5 ± 1.7 × 22.4 ± 1.3 μm [mean ± SD length × mean ± SD width] and an oval shape (Figure 1E). The discharged tubule was undifferentiated along its axis (isorhizous) and lacked a well-defined shaft (haploneme). The middle part of the tubule was armed with coiled, closely set spines (heterotrichous) (Figure 1F). In scanning electron micrographs, only type 1 was detected (Figures 1G,H). The heterotrichous tubule structure of type 1 consisted of parts without spines (Figure 1I), flanking the middle region equipped with coiled, closely set spines (Figure 1J). Nematocyst type 2 was smaller with a size of 17.1 ± 1.3 × 10.7 ± 0.8 μm and an oval shape with slightly pointed ends at the apical and basal poles (Figure 1K). Type 2 was characterized by a well-defined shaft (i.e., heteronemes) of ~capsule length (microbasic) with “initially penetrating large spines” (i.e., stylet, sensu Östman, 2000). Based on light microscopic investigation, the shaft likely had an unarmed base and large spines appeared to be present at the level of constriction (Figure 1L), which are features of pseudostenoteles. Furthermore, the apical pole might have an aperture closed by a cover (operculum). To infer a potential nematocyst donor for the sponge, the inherent nematocysts were compared to the respective cnidoms of eight abundant, co-cultivated cnidarian species. Light microscopic investigations revealed specific nematocyst types for the sea anemones Aiptasia diaphana, Anemonia cf. manjano, and E. quadricolor, the encrusting anemone P. heliodiscus, the soft corals Anthelia sp. and C. imbricata, and the stony corals A. tumida and M. aequituberculata (Figure S1). However, none of these nematocyst types matched morphologically the sponge inherent nematocysts.
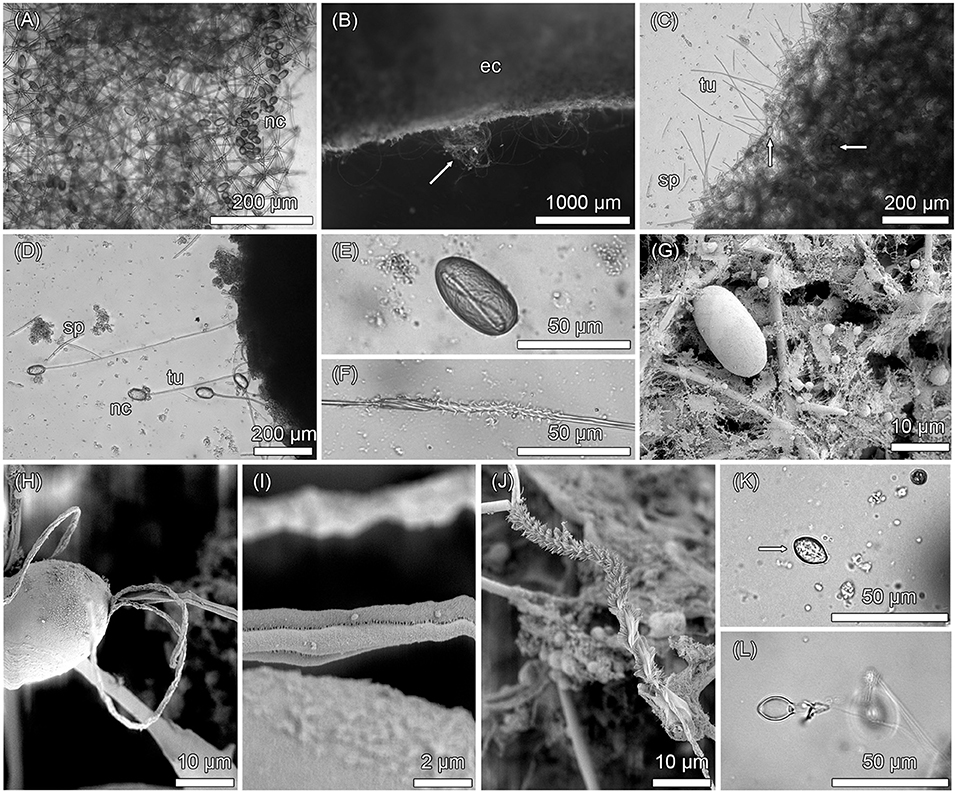
Figure 1. Inherent nematocysts in Haliclona cnidata sp. nov. Light microscopic images (A–F, K–L) and scanning electron micrographs (G–J) of nematocysts in the sponge. (A) Cresyl violet acetate staining showing battery-like agglomeration of nematocysts within the isodictyal sponge skeleton. (B) Tubules of discharged nematocysts (arrow) penetrating the sponge ectosome due to ethanol exposure. (C) Nematocysts (arrows) within mesohyl, discharged tubules, and scattered spiculae. (D) Tubules of four discharged type 1 nematocysts attached to sponge mesohyl. (E) Undischarged, heterotrichous isorhizous haploneme nematocyst (type 1) containing a coiled tubule. (F) Discharged, heterotrichous tubule of type 1 nematocyst. (G) Undischarged type 1 nematocyst within sponge mesohyl. (H) Type 1 nematocyst with everted tubule. (I) Spineless tubule structure of type 1 neamtocyst. (J) Close-up of heterotrichous tubule region of type 1 nematocyst equipped with closely-set spines arranged in helical rows. (K) Undischarged, microbasic heteroneme nematocyst (type 2, arrow). (L) Discharged shaft of type 2 nematocyst with spines at the level of constriction. ec, ectosome; nc, nematocyst; sp, spiculae; tu, tubules.
Donor-Independent Development of Inherent Nematocysts in SCAs
To address the potential of a donor-independent development of inherent sponge nematocysts, the changes of numbers of types 1 and 2 nematocysts in SCAs were assessed from day 1 to day 8. In total (types 1 and 2 combined), 2.9 ± 6.4 nematocysts were observed in 200 SCAs cells at day 1 and 24.8 ± 13.1 at day 8 (Figure 2). After 8 days, the total and individual numbers of types 1 and 2 nematocysts had increased in the SCAs significantly (p < 0.001, Wilcoxon rank sum test; Table 1).
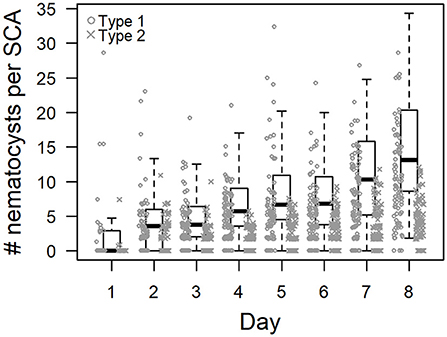
Figure 2. Donor-independent changes of inherent nematocyst numbers in sponge cell aggregates (SCA) of Haliclona cnidata sp. nov. Box-and-whiskers-plot showing the distribution of the extrapolated number of inherent nematocysts in 48-h-old SCA (n = 8) over eight consecutive days. Displayed are the median, first and third quartiles, and two whiskers for the total (sum of type 1 and type 2) number of nematocysts. Numbers of type 1 and type 2 are depicted individually. Nematocyst numbers differed significantly between day 1 and 8 (Table 1).
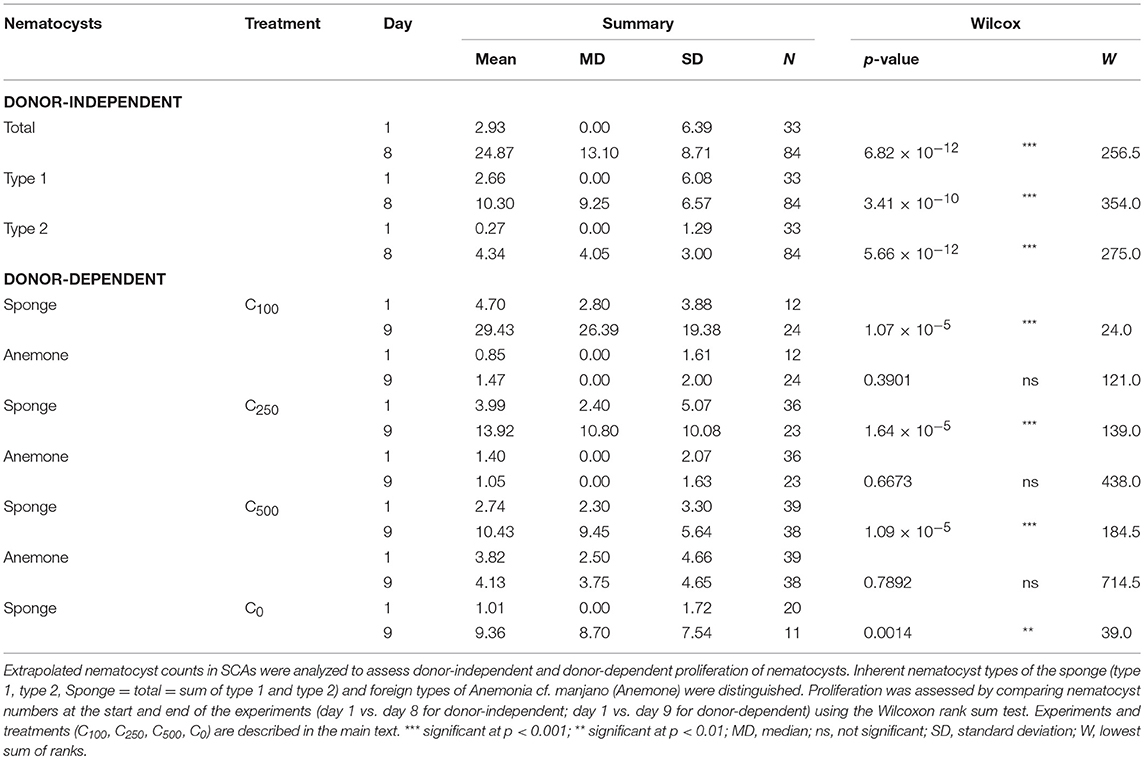
Table 1. Summary statistics for nematocyst counts in sponge cell aggregates (SCAs) of Haliclona cnidata sp. nov.
Donor-Dependent Development of Foreign Nematocysts in SCAs
The potential of foreign nematocysts to develop in SCAs was assessed by exposing SCAs to different volumes (C0, C100, C250, and C500) of nematocyst suspensions obtained from Anemonia cf. manjano. The foreign nematocysts of the anemone were narrow, elongated and had an average size of 17.8 ± 1.4 × 3.2 ± 0.5 μm. They could be easily distinguished from sponge inherent nematocysts. According to the first SCA experiment (see section Donor-Independent Development of Inherent Nematocysts in SCAs), the numbers of inherent nematocysts increased significantly in all four treatments (p < 0.001, Wilcoxon rank sum test; Table 1, Figure 3). However, the numbers of foreign nematocysts obtained from the anemone did not differ significantly between day 1 and 9 in the SCAs (p > 0.05, Wilcoxon rank sum test; Table 1, Figure 3).
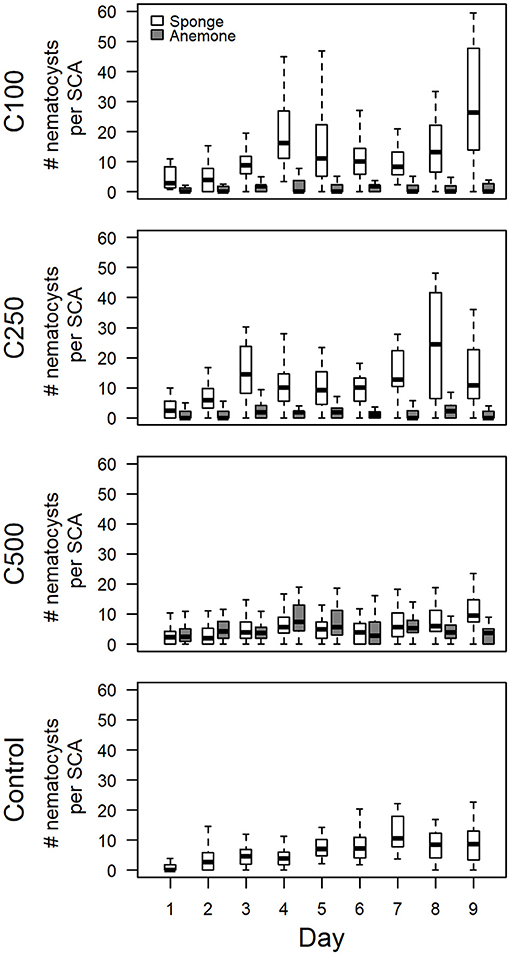
Figure 3. Donor-dependent changes of nematocyst numbers in sponge cell aggregates (SCAs) of Haliclona cnidata sp. nov. Box-and-whiskers-plot showing the distribution of the extrapolated number of sponge inherent and foreign anemone nematocysts in 48-h-old SCA over 9 consecutive days. Anemone nematocyst suspensions were applied to SCA within the first 48 h in various concentrations (C100; C250; C500; C0, control). Displayed are the median, first and third quartiles, and two whiskers for nematocysts of sponge (white) and anemone (gray). To enhance clarity, only the total numbers of sponge inherent nematocysts (i.e., sum of type 1 and type 2) are shown. Only numbers of sponge inherent nematocysts differed significantly between day 1 and 9 (Table 1).
Systematics and Taxonomy of Haliclona cnidata sp. nov.
1. Class Demospongiae Sollas, 1885
2. Subclass Heteroscleromorpha Cárdenas et al. (2012)
3. Order Haplosclerida Topsent, 1928
4. Family Chalinidae Gray, 1867
5. Genus Haliclona Grant, 1835
Haliclona cnidata sp. nov. (Figure 4A), the “stinging black sponge.”
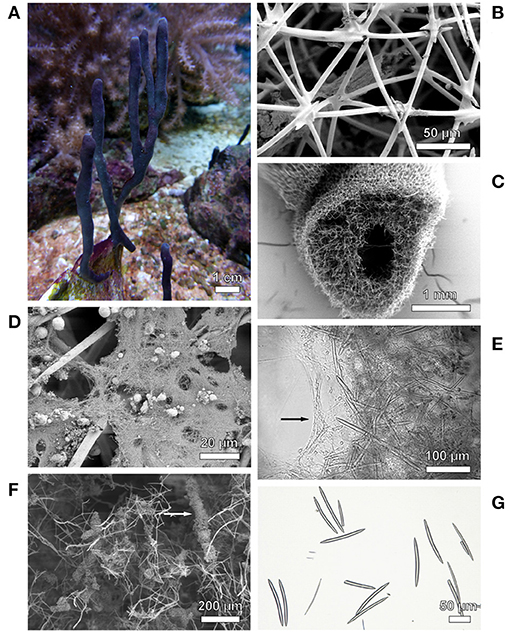
Figure 4. Photographs of Haliclona cnidata sp. nov. and its skeletal composition. (A) Haliclona cnidata sp. nov. in the marine aquarium system of Justus Liebig University Giessen. (B) Sponge ectosome showing isodictyal reticulated tangential skeleton. (C) Ectosomal and choanosomal structures. Spongin fibers: (D) collagen filaments, (E) compound fasciculate fibers in choanosome, (F) partially cored with detritus. (G) Megascleres of the type oxea. Depicted are scanning electron micrographs (B–D,F) and light microscopic images (E,G).
Material
Holotype/Paratypes: tropical marine aquarium systems of the Research Center for Biosystems, Land Use and Nutrition (IFZ) at Justus Liebig University Giessen, Giessen, Germany; collected by Johannes Schellenberg and Patrick Schubert from aragonite/coral rock substrate on 20 June 2018. Type material is deposited at the Senckenberg Research Institute and Natural History Museum (Senckenberg Gesellschaft für Naturforschung, Frankfurt/Main, Germany), inventory numbers SMF 12107 (holotype), SMF 12108 (paratypes). Additional paratypes and DNA preps are deposited at the Systematics and Biodiversity Collection at Justus Liebig University Giessen, Giessen, Germany (UGSB, see Diehl et al., 2018), inventory numbers UGSB 21994 (paratype) as well as 18538 and 18539 (DNA prep). Life Science Identifier (LSID): urn:lsid:zoobank.org:act:509A075A-5D5D-417F-811E-52D3421D48BC.
Morphological Description
Shape: arborescent-bifurcate branching erected growing fingers with encrusting base, spiny fibrous surface. Size: height up to 20 cm, diameter up to 7 mm. Color: dark gray to purple (normal light), weak brown to pale (low light), yellow-green (strong light); color in ethanol: pink and eventually white. Surface ornamentation: nematocysts and spicules forming a spiny fibrous shape, oscules flush with the surface at tips or irregularly scattered among the branches. Consistency: soft, fragile, easy torn; no mucus production or smell. Ectosomal skeleton: isodictyal reticulated tangential (Figure 4B). Choanosomal skeleton: alveolate, subisotropic, paucispicular reticulation with ill-defined uni- and secondary lines (Figure 4C); chelae and microscleres absent. Spongin: collagen filaments (Figure 4D) and compound fasciculate fibers (Figure 4E) partially cored with detritus (Figure 4F). Spicules: megascleres of the type oxea, slightly recurved in the midline, pointed ends (Figure 4G); two size categories 58.6–94.2(13.7)–129.0 × 1.0–3.9(1.5)–6.3 μm and 89.9–113.5(10.3)–135.2 × 6.5–8.3(1.1)–11.8 μm; consist of silicon dioxide (EDX analysis: dominant silicon signal [SiKα at 1.74 keV], prominent carbon signal [CKα at 0.28 keV] and oxygen signal [OKα at 0.54 keV]).
Ecology and Distribution
Tropical marine species, which grows on hard substrate. Natural distribution unknown.
Etymology
Derived from the Ancient Greek κνíδη (knídē, “nettle”), a synonym for nematocyst.
Additional Information
Adult individuals may harbor two types of cnidarian nematocysts in the sponge ectosome as well as single-celled dinoflagellates (Protista). The latter have a green to brown color, a spherical shape with a diameter of ~10 μm, and belong potentially to the genus Symbiodinium. Based on counting of Sybr Green I-stained bacterial cells and 16S rRNA gene amplicon next generation data (JS, unpublished data), the sponge most likely represents a high-microbial-abundance sponge (sensu Hentschel et al., 2006).
Phylogeny
The placement of H. cnidata sp. nov. within the phylogenies of the subclass Haploscleromorpha published by Redmond et al. (2011, 2013) was determined based on 18S rRNA and COI gene sequence data. The subclass Haploscleromorpha was proposed by Cárdenas et al. (2012) for marine Haplosclerida but later refined to Heteroscleromorpha (Morrow and Cárdenas, 2015; World Porifera Database, Van Soest et al., 2018). In the 18S rRNA gene based phylogeny (Figure 5), H. cnidata sp. nov. (GenBank accession number MH373530) clustered within clade A (sensu Redmond et al., 2013) and formed a distinct monophyletic group (BPP 0.88) with an undescribed Haliclona sp. from Florida (“Sp 5”; Richelle-Maurer et al., 2006; GenBank accession number AY734444). Based on COI data (Figure 6), H. cnidata sp. nov. (GenBank accession number MH396488) also clustered within clade A (sensu Redmond et al., 2011). Together with its sister taxon, the Indonesian H. vanderlandi (GenBank accession number JN242208), it formed a distinct and well supported clade (BPP 0.99).
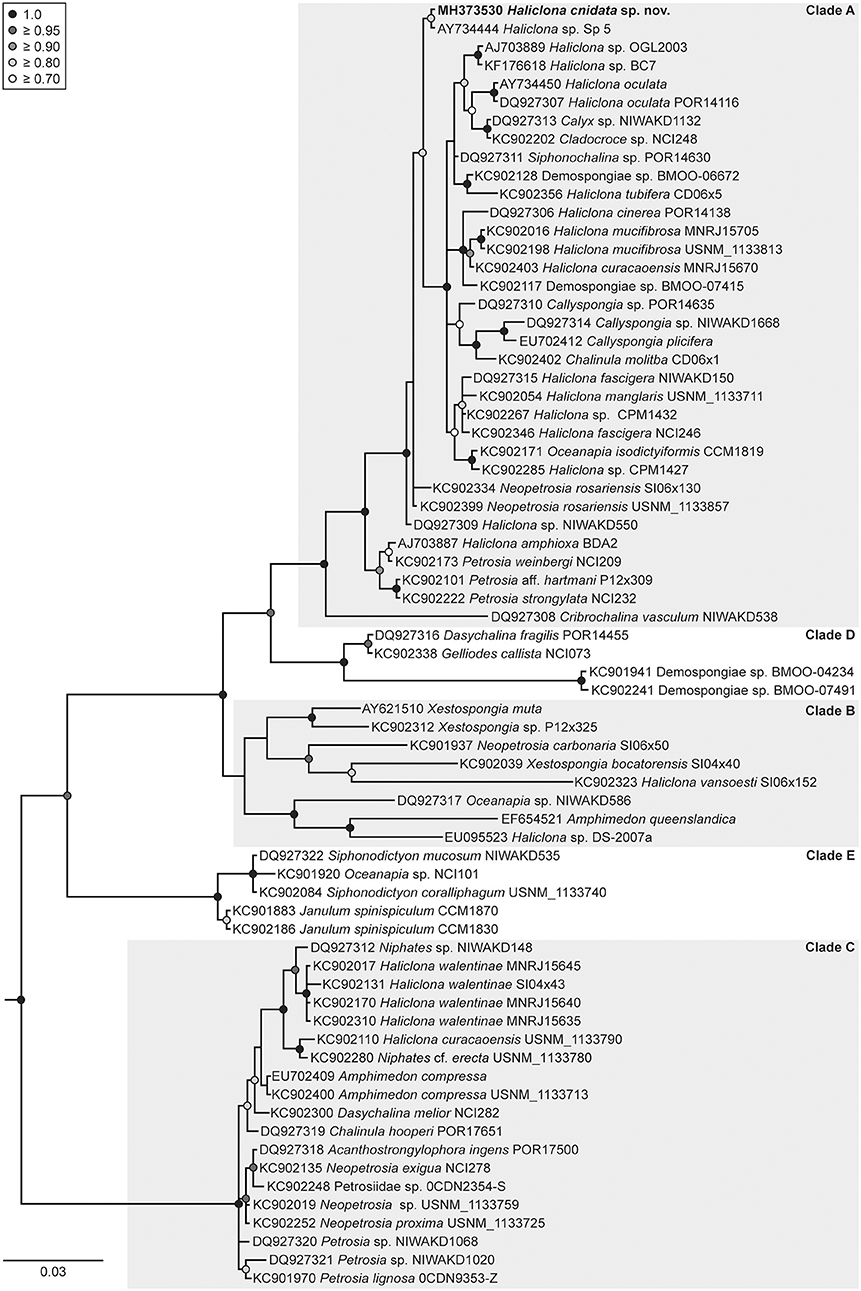
Figure 5. Phylogenetic placement of Haliclona cnidata sp. nov. based on the 18S rRNA gene. Bayesian maximum clade credibility tree depicting the placement of Haliclona cnidata sp. nov. (in bold) within the classification published of Redmond et al. (2013). The tree was rooted with the outgroup taxon Aplysina fistularis (KC902180). Additionally, Stelletta fibrosa (KC902062), Vetulina sp. (KC901963), Halisarca restingaensis (KC902130), Lamellodysidea sp. (KC902205), and Chelonaplysilla sp. (KC902373) were included as outgroup taxa according to Redmond et al. (2013). These outgroup taxa were removed from the tree a posteriori. Bayesian posterior probabilities (BPP) ≥ 0.7 are shown at nodes. The scale bar indicates substitutions per side according to the GTR+I+G model of sequence evolution.
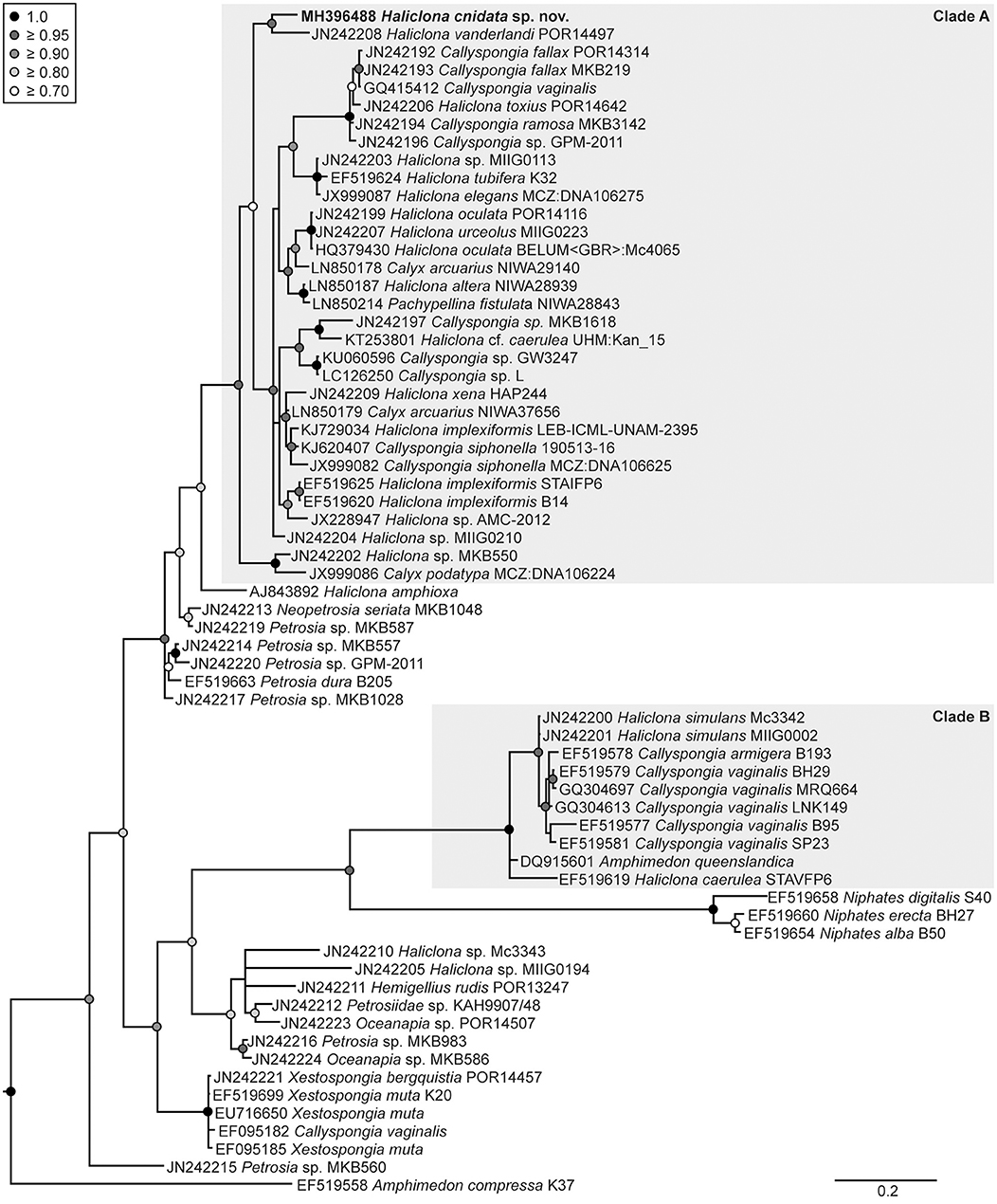
Figure 6. Phylogenetic placement of Haliclona cnidata sp. nov. based on the COI gene. Bayesian maximum clade credibility tree showing the placement of Haliclona cnidata sp. nov. (in bold) within the classification of Redmond et al. (2011). Two outgroup taxa, Eunapius subterraneus (FJ715439) and Baikalospongia intermedia (DQ167168), were included in the analysis (the former was used for rooting). Both outgroups were removed from the tree a posteriori. Bayesian posterior probabilities (BPP) ≥ 0.7 are shown at nodes. The scale bar indicates substitutions per side according to the GTR+I+G model of sequence evolution.
Remarks
Despite the taxonomic challenges in classifying Haliclona species (De Weerdt and Van Soest, 2001), the assignment to Haliclona, Grant 1835 is confirmed both by phylogenetic analyses and agreement with the following diagnostic characters of the genus according to “Systema Porifera” (Hooper and Van Soest, 2002). Haliclona species can be, among others, tube-shaped of purple color and soft consistency. Although the surface is rather smooth, it can be strongly punctated like H. cnidata sp. nov. Oscula are flush with the surface or at the top of chimneys. Ectosomal skeleton types comprise very regular, tangential, and isotropic reticulation. Choanosomal skeleton can be alveolate, paucispicular reticulation with ill-defined uni- and secondary lines. Megascleres can be oxeas and their typical length (80–250 μm × 5–10 μm) complies with H. cnidata sp. nov. The genus Haliclona comprises more than 450 species from which ~200 have been assigned to seven subgenera (Flagellia Van Soest, 2017, Gellius Gray, 1867, Halichoclona, Haliclona Grant, 1836, Reniera, Rhizoniera Griessinger, 1971, and Soestella De Weerdt, 2000) (Hooper and Van Soest, 2002; Van Soest et al., 2018). However, the subgenera of Haliclona seem to be polyphyletic (Redmond et al., 2011, 2013). Classification of H. cnidata sp. nov. as Flagellia can be excluded due to the absence of the characteristic flagellosigma (Van Soest, 2017). The subisotropic choanosomal skeleton of the species would justify an assignment to either Halichoclona, Soestella, or Reniera because species of the subgenera Haliclona, Rhizoniera, and Gellius have an anisotropic skeleton. Soestella can be likely excluded since rounded meshes and abundant choanosomal spaces are missing in the skeleton of H. cnidata sp. nov. Presence of spongin dissents from an assignment to Halichoclona, although the COI phylogeny indicates a close relationship. However, spongin is not restricted to the nodes as being characteristic for Reniera spp. Due to the inconsistencies and the apparent polyphyly of the subgenera, a distinct subgenus assignment of H. cnidata sp. nov. is pending. The presence of nematocysts in H. cnidata sp. nov. suggests a close relationship to the nematocyst bearing sponge Haliclona sp. 628 from the Great Barrier Reef Garson et al., 1998. We therefore compared H. cnidata sp. nov. with voucher material of Haliclona sp. 628 (voucher G304086, photograph provided by courtesy of Dr. M. Ekins), and the descriptions of Garson et al. (1998) and Russell et al. (2003). Both species are similar regarding the finger-like growth form, presence of dinoflagellates, and olive-brown color of the ectosome. However, they differ in several key characteristics, such as type of nematocysts, size of oxea, mucus production, endosome color, competitive interaction with corals, and preferred substrate. The single nematocyst type of Haliclona sp. 628 (“~15–20 mm in length and were composed of a spine-bearing butt and a long tightly coiled thread”; Garson et al., 1998, p. 369) is different from the cnidom of H. cnidata sp. nov. (see section Inherent Nematocysts of Adult Sponges and Potential Cnidarian Donors). Although both species share oxea that are slightly recurved in the mid-line (Garson et al., 1998), the size of oxea seem to be smaller in Haliclona sp. 628 with ~70 μm in length (own measurement based on microscopic images of Garson et al., 1998). Moreover, mucus production is absent for H. cnidata sp. nov. in contrast to Haliclona sp. 628 (Russell et al., 2003). The endosome of H. cnidata sp. nov. is pale while Haliclona sp. 628 is orange (Garson et al., 1998). H. cnidata sp. nov. shows no competitive interactions with corals and does not grow preferably on coral substrate; both are characteristic features of Haliclona sp. 628 (Garson et al., 1998). Unfortunately, a molecular comparison is not possible due to the lack of DNA sequence information for Haliclona sp. 628. Nonetheless, because of differences in morphological characteristic, the two species are likely not con-specific. The phylogenetic analyses based on the partial 18S rRNA gene (Figure 5) suggests a close relationship of H. cnidata sp. nov. to the poorly characterized “Haliclona sp. Sp 5” from Florida (Richelle-Maurer et al., 2006). However, given the conservative nature of the 18S rRNA gene, reliable species-level inferences remain impossible. In the COI phylogeny (Figure 6), H. cnidata sp. nov. clusters together with Haliclona (Halichoclona) vanderlandi from eastern Indonesia. However, the latter species exhibits aligned, laterally fused, thick-walled tubes that reach a diameter of up to 4 cm. H. vanderlandi also has a characteristic red color (De Weerdt and Van Soest, 2001). Moreover, the oxea have a large size of 174.9–202.4(11.8)–217.3 × 5.8–7.7(1.5)–10.1 μm (De Weerdt and Van Soest, 2001). These clear morphological differences strongly support the distinctness of H. cnidata sp. nov.
Discussion
Origin and Development of Nematocysts in Sponges—The Precursor Hypothesis of Sponge Kleptocnidism
The most striking feature of H. cnidata sp. nov. is the occurrence of mostly intact nematocysts, which have been observed in numerous specimens across different aquarium systems, regardless of the cnidarian species inventory in the respective tanks. We here provide the first experimental study that shows both the functionality and donor-independent development of nematocysts in the stinging black sponge. Both types of inherent nematocysts of H. cnidata sp. nov.—the heterotrichous isorhizous haploneme and the microbasic heteronemes with a stylet—belong to the common group Stomocnidae, which includes most of the penetrating nematocysts (Östman, 2000). Unfortunately, the microbasic hetereonemes (type 2) were not captured by SEM, which could have resolved the presence of an operculum. Such presence would restrict the potential donor of type 2 to a medusozoan origin (Reft and Daly, 2012). The arrangement of the nematocysts in the sponge is “battery”-like (sensu Kass-Simon and Scappaticci, 2002). They can penetrate the sponge ectosome and may thus serve as a highly specialized defense weapon in H. cnidata sp. nov. Kleptocnidism depends on the acquisition of nematocysts, as their production itself is a synapomorphic trait of cnidarians (Marques and Collins, 2004). Hence, the inherent nematocysts within H. cnidata sp. nov. must have originated initially from a cnidarian donor. Since the cnidom of the sponge comprised two different types, the inherent nematocysts might even originate from two distinct cnidarian species. Interestingly, none of the nematocysts of eight cnidarian species, which were co-cultivated with the stinging black sponge in our aquarium systems, resembled those found in the sponge. Noteworthy, a complete survey of all co-occurring cnidarians was not feasible but the investigated representatives comprised the abundant and conspicuous species and accounted for a broad range of cnidarian orders. Even though such a morphology-based comparison may have limitations due to the often high degree of polymorphism in nematocysts (Östman, 2000; Fautin, 2009), it is unlikely that the sponge acquired mature nematocysts. In fact, previous studies have shown that nematocysts and their encapsulating nematocytes stem from precursor cells that develop sequentially from nematoblasts into mature nematocyte-nematocyst complexes, and their localization is determined by a migration of either the precursor cells or the nematocyte to its ultimate site (Kass-Simon and Scappaticci, 2002; Fautin, 2009). Therefore, the observed donor-independent proliferation of inherent nematocysts in our SCAs (see section Donor-Independent Development of Inherent Nematocysts in SCAs) is likely related to a nematocyst precursor stage. Furthermore, the SCAs and most likely the adult sponge seem to provide a suitable environment for the development of these specific precursors. This assumption is also supported by (i) the occurrence of two specific nematocyst types in the adult H. cnidata sp. nov. (see section Inherent Nematocysts of Adult Sponges and Potential Cnidarian Donors) and (ii) the fact that the abundance of foreign nematocysts in the SCAs did not increase in our experiments, in contrast to the inherent types (see section Donor-Dependent Development of Foreign Nematocysts in SCAs). In this context, an unrestricted acquisition of nematocysts by H. cnidata sp. nov. seems unlikely. An autonomous production of nematocysts by the sponge can likely be excluded because it would at least require the genetic information of the cnidarian multi-potent interstitial stem cells. We believe that the sponge depends initially on the acquisition of immature cnidarian nematocytes (i.e., nematocyst precursors or immature nematocyte-nematocyst complexes).
Little is known about the acquisition of exogenous defense structures like the sequestration of nematocysts and the ecological implications for the sequestering species. Recently, Goodheart and Bely provided a generalized working model as well as traits facilitating the evolution of kleptocnidism in metazoans, i.e., preying on cnidarians, a soft-body, a branched digestive system that allows unhampered distribution of nematocysts, and the ability to regenerate (Goodheart and Bely, 2017). Interestingly, sponges comply mostly with these traits by having an aquiferous system throughout their soft body combined with regenerative power (Hooper and Van Soest, 2002), which likely facilitate the distribution of nematocysts. However, sponges do not prey on cnidarians.
The origin of kleptocnidae in sponges has previously been discussed for Haliclona sp. 628 in the light of the nematocyst-free congener Haliclona sp. 1031 (Garson et al., 1998; Russell et al., 2003). The authors hypothesized that the larvae of Haliclona sp. 628 settle preferably onto live Acropora nobilis corals. Eventually, the growing juvenile sponge causes necrosis of the coral tissue via secondary metabolites, and the sponge sequesters nematocysts and dinoflagellates of the coral. Their reasoning was supported, among others, by the preference of A. nobilis as substratum, the occurrence of dead coral tissue adjacent to the sponge, the presence of compounds within Haliclona sp. 628 that are toxic to corals, and variable amounts of nematocysts in the sponge depending on the presence of corals. This donor-dependent uptake of nematocyst, as proposed for Haliclona sp. 628 by Russell et al. (2003), is not fully compatible with our findings. Neither a preference for coral substratum nor competitive allelochemical interactions with corals have been observed for H. cnidata sp. nov. Moreover, we have shown that inherent nematocysts might develop in SCAs.
We therefore propose an alternative kleptocnidism model for the stinging black sponge. Accordingly, immature nematocytes might be acquired through filtration, either directly of free-floating forms, or indirectly via coral mucus or larvae. These immature nematocytes are maintained in the sponge and may even mature to specific types of fully functioning nematocyte-nematocyst complexes. Such maturation of sequestered nematocysts is known for some nudibranchs (Goodheart and Bely, 2017). Free-floating immature nematocytes may, for example, be released into the water body after corallivorous predator attacks either from predator feces (Martin, 2003; Goodheart and Bely, 2017) or potentially from injured coral tissues. Corallivory is widespread in marine ecosystems and has been observed across higher taxa (e.g., nudibranchs, flatworms, fishes; Cole et al., 2008; Greenwood, 2009; Goodheart and Bely, 2017). At a small scale, corallivory also occurs in the reef tanks used for cultivation of H. cnidata sp. nov. Alternatively, sponges could acquire immature nematocytes through filtration of secreted coral mucus (Brown and Bythell, 2005). Recently, Rix et al. (2016) showed that coral mucus is sequestered by sponges as an energy and nutrient source and they suggested that this biogeochemical cycling is ubiquitous in reef environments. Finally, cnidarian larvae could potentially serve as a vector for immature nematocytes (Kass-Simon and Scappaticci, 2002; Polato et al., 2013), attracted by sponge-associated bioactive substances. Bioassays with the dominant bioactive compound of Haliclona sp. 628, for example, revealed that haliclonacyclamine A selectively mediates settlement and metamorphosis of various larvae of invertebrates (Green et al., 2002; Roper et al., 2009). Moreover, the inhibition of nematocyst discharge in sponges might also be compound-mediated as suggested for nudibranchs and anemones (Greenwood et al., 2004). It is therefore likely that kleptocnidism in sponges is facilitated by specific bioactivity profiles. This would also explain why, on the one hand, specific nematocyst types were observed in sponge cnidoms and why, on the other hand, kleptocnidism is restricted to very few sponge species. The energetically-demanding production of such secondary metabolites in sponges (Thoms and Schupp, 2007), in turn, might be compensated through endosymbioses with photoautotrophic dinoflagellates (Russell et al., 2003) and bacterial communities (e.g., Taylor et al., 2007; Reveillaud et al., 2014). Although a correlation between bioactivity and kleptocnidism in sponges remain to be elucidated, H. cnidata sp. nov. contains both dinoflagellates and a complex bacterial community.
Conclusions and Outlook
In our study, we confirmed kleptocnidism in a second sponge species—the tropical marine H. cnidata sp. nov. Unexpectedly, our experiments demonstrated that different types of functional nematocysts can develop in this taxon without direct contact to a cnidarian donor. Specifically, we showed that these types do not match those of the abundant, co-occurring cnidarians and that the amount of sponge-inherent kleptocnidae in SCAs increased over time in the absence of potential donors. Based on these findings, we proposed the precursor hypothesis of sponge kleptocnidism: immature nematocytes might be (i) acquired by sponges through filtration, (ii) maintained in sponge tissues, and (iii) nurtured to specific types of fully functioning nematocyte-nematocyst complexes. The respective processes are likely facilitated by bacteria-derived secondary metabolites and acquired photosynthetically active dinoflagellates. Since adult cnidarians can pass on immature nematocytes to their offspring, it remains to be elucidated whether this could apply to sponges as well. Such a vertical transmission was beyond the scope of this research but provides an interesting hypothesis for future studies. Given the seemingly autonomous production of nematocysts in H. cnidata sp. nov. and the still unresolved question regarding their origin, we are currently addressing the sponge with its symbiotic partners in a hologenome sequencing project. Such a hologenomic focus could not only provide valuable insights on the origin of the nematocysts but also on the evolutionary processes among the symbiotic assemblages in the sponge holobiont. As kleptocnidism, so far, has been studied mostly in nudibranchs, H. cnidata sp. nov. may serve as a novel evolutionary model system. The simple body plan of sponges and the in vitro proliferation capacity of sponge cells via SCAs are ideal prerequisites for studying fundamental research questions regarding origin, maintenance, and deployment of inherent nematocysts. This study also extends the perception of defense mechanisms in sponges to a holobiontic perspective involving the actual sponge, cnidarian nematocysts, dinoflagellate endosymbionts, and a complex microbial community.
Author Contributions
JS, JR, PS, and TW conceived and designed the experiments. JS and JR performed the experiments. SG supported the phylogenetic analysis. JS, JR, MH, HS, PK, SG, PS, and TW wrote the manuscript. All authors contributed to data analysis, revised and approved the final manuscript version and are accountable for all aspects of the work.
Funding
JS and JR received financial support through scholarships of the CEMarin (Center of Excellence in Marine Sciences, Colombia).
Conflict of Interest Statement
The authors declare that the research was conducted in the absence of any commercial or financial relationships that could be construed as a potential conflict of interest.
Acknowledgments
We thank Dr. Merrick Ekins (Queensland Museum, South Brisbane, Australia) for providing a photograph of Haliclona sp. 628 (voucher G304086) and two reviewers for their valuable comments. We gratefully acknowledge Angelina Arnold, Nora Goldmann, Eva Heinrich, Sabine Agel, Barbara Hönig, and Anna Moebus (Justus Liebig University, Giessen) for their excellent technical assistance.
Supplementary Material
The Supplementary Material for this article can be found online at: https://www.frontiersin.org/articles/10.3389/fmars.2018.00509/full#supplementary-material
References
Bell, J. J. (2008). The functional roles of marine sponges. Estuar. Coast. Shelf. Sci. 79, 341–353. doi: 10.1016/j.ecss.2008.05.002
Blunt, J. W., Copp, B. R., Keyzers, R. A., Munro, M. H. G., and Prinsep, M. R. (2016). Marine natural products. Nat. Prod. Rep. 33, 382–431. doi: 10.1039/c5np00156k
Brown, B. E., and Bythell, J. C. (2005). Perspectives on mucus secretion in reef corals. Mar. Ecol. Prog. Ser. 296, 291–309. doi: 10.3354/meps296291
Cárdenas, P., Pérez, T., and Boury-Esnault, N. (2012). Sponge systematics facing new challenges. Adv. Mar. Biol. 61, 79–209. doi: 10.1016/B978-0-12-387787-1.00010-6
Cole, A. J., Pratchett, M. S., and Jones, G. P. (2008). Diversity and functional importance of coral-feeding fishes on tropical coral reefs. Fish. Fish 9, 286–307. doi: 10.1111/j.1467-2979.2008.00290.x
Custodio, M. R., Prokic, I., Steffen, R., Koziol, C., Borojevic, R., Brümmer, F., et al. (1998). Primmorphs generated from dissociated cells of the sponge Suberites domuncula: a model system for studies of cell proliferation and cell death. Mech. Ageing Dev. 105, 45–59. doi: 10.1016/S0047-6374(98)00078-5
Darriba, D., Taboada, G. L., Doallo, R., and Posada, D. (2012). jModelTest 2: more models, new heuristics and parallel computing. Nat. Methods 9:772. doi: 10.1038/nmeth.2109
De Weerdt, W., and Van Soest, R. W. (2001). Haliclona (Halichoclona) vanderlandi spec. nov. (Porifera: Demospongiae: Haplosclerida) from Indonesia. Zool. Verhandel. 334, 189–194.
Diehl, E., Jauker, B., Albrecht, C., Wilke, T., and Wolters, V. (2018). “GIEßEN: university collections: justus liebig university Gießen,” in Zoological Collections of Germany. Natural History Collections, ed L. Beck (Cham: Springer), 373–381. doi: 10.1007/978-3-319-44321-8_29
Fautin, D. G. (2009). Structural diversity, systematics, and evolution of cnidae. Toxicon 54, 1054–1064. doi: 10.1016/j.toxicon.2009.02.024
Garson, M. J., Flowers, A. E., Webb, R. I., Charan, R. D., and McCaffrey, E. J. (1998). A sponge/dinoflagellate association in the haplosclerid sponge Haliclona sp: cellular origin of cytotoxic alkaloids by Percoll density gradient fractionation. Cell Tissue Res. 293, 365–373. doi: 10.1007/s004410051128
Goodheart, J. A., and Bely, A. E. (2017). Sequestration of nematocysts by divergent cnidarian predators: mechanism, function, and evolution. Invertebr. Biol. 136, 75–91. doi: 10.1111/ivb.12154
Green, K. M., Russell, B., Clark, R. J., Jones, M., Garson, M. J., and Skilleter, G., et al. (2002). A sponge allelochemical induces ascidian settlement but inhibits metamorphosis. Mar. Biol. 140, 355–363. doi: 10.1007/s002270100698
Greenwood, P. G. (2009). Acquisition and use of nematocysts by cnidarian predators. Toxicon 54, 1065–1070. doi: 10.1016/j.toxicon.2009.02.029
Greenwood, P. G., Garry, K., Hunter, A., and Jennings, M. (2004). Adaptable defense: a nudibranch mucus inhibits nematocyst discharge and changes with prey type. Biol. Bull. 206, 113–120. doi: 10.2307/1543542
Hentschel, U., Usher, K. M., and Taylor, M. W. (2006). Marine sponges as microbial fermenters. FEMS Microbiol. Ecol. 55, 167–177. doi: 10.1111/j.1574-6941.2005.00046.x
Hooper, J. N. A., and Van Soest, R. W. (2002). Systema Porifera: A Guide to the Classification of Sponges. New York, NY: Kluwer Academic/Plenum Publishers.
Huelsenbeck, J. P., and Ronquist, F. (2001). MRBAYES: Bayesian inference of phylogenetic trees. Bioinformatics 17, 754–755. doi: 10.1093/bioinformatics/17.8.754
Kass-Simon, G. and Scappaticci, J. A. A. (2002). The behavioral and developmental physiology of nematocysts. Can. J. Zool. 80, 1772–1794. doi: 10.1139/z02-135
Katoh, K., and Standley, D. M. (2013). MAFFT multiple sequence alignment software version 7: improvements in performance and usability. Mol. Biol. Evol. 30, 772–780. doi: 10.1093/molbev/mst010
Katoh, K., and Toh, H. (2008). Improved accuracy of multiple ncRNA alignment by incorporating structural information into a MAFFT-based framework. BMC Bioinformatics 9:212. doi: 10.1186/1471-2105-9-212
Kelly-Borges, M., and Pomponi, S. A. (1992). The Simple Fool's Guide to Sponge Taxonomy. Fort Pierce, FL: Harbor Branch Oceanographic Institution.
Marques, A. C., and Collins, A. G. (2004). Cladistic analysis of Medusozoa and cnidarian evolution. Invertebr. Biol. 123, 23–42. doi: 10.1111/j.1744-7410.2004.tb00139.x
Martin, R. (2003). Management of nematocysts in the alimentary tract and in cnidosacs of the aeolid nudibranch gastropod Cratena peregrina. Mar. Biol. 143, 533–541. doi: 10.1007/s00227-003-1078-8
McKay, M. C., and Anderson, P. A. V. (1988). Preparation and Properties of Cnidocytes from the Sea Anemone Anthopleura elegantissima. Biol. Bull. 174, 47–53. doi: 10.2307/1541758
Medlin, L., Elwood, H. J., Stickel, S., and Sogin, M. L. (1988). The characterization of enzymatically amplified eukaryotic 16S-like rRNA-coding regions. Gene 71, 491–499. doi: 10.1016/0378-1119(88)90066-2
Meyer, C. P., Geller, J. B., and Paulay, G. (2005). Fine scale endemism on coral reefs: archipelagic differentiation in turbinid gastropods. Evolution 59, 113–125. doi: 10.1111/j.0014-3820.2005.tb00899.x
Morrow, C., and Cárdenas, P. (2015). Proposal for a revised classification of the Demospongiae (Porifera). Front. Zool. 12:7. doi: 10.1186/s12983-015-0099-8
Müller, W. E. G., Müller, I., Zahn, R. K., and Kurelec, B. (1976). Species-specific aggregation factor in sponges: VI. Aggregation receptor from the cell surface. J. Cell Sci. 21, 227–241.
Östman, C. (2000). A guideline to nematocyst nomenclature and classification, and some notes on the systematic value of nematocysts. Sci. Mar. 64, 31–46. doi: 10.3989/scimar.2000.64s131
Paul, V. J., and Puglisi, M. P. (2004). Chemical mediation of interactions among marine organisms. Nat. Prod. Rep. 21, 189–209. doi: 10.1039/b302334f
Pawlik, J. R. (1993). Marine invertebrate chemical defenses. Chem. Rev. 93, 1911–1922. doi: 10.1021/cr00021a012
Polato, N. R., Altman, N. S., and Baums, I. B. (2013). Variation in the transcriptional response of threatened coral larvae to elevated temperatures. Mol. Ecol. 22, 1366–1382. doi: 10.1111/mec.12163
R Studio Team (2016). RStudio: Integrated Development for R. Boston, MA: RStudio, Inc. Available online at: http://www.rstudio.com/
Rambaut, A., Succhard, M., and Drummond, A. (2014). Tracer v1.6. Available online at: http://beast.bio.ed.ac.uk/Tracer
Redmond, N. E., Morrow, C. C., Thacker, R. W., Diaz, M. C., Boury-Esnault, N., and Cárdenas, P., et al. (2013). Phylogeny and systematics of demospongiae in light of new small-subunit ribosomal DNA (18S) sequences. Integr. Comp. Biol. 53, 388–415. doi: 10.1093/icb/ict078
Redmond, N. E., Raleigh, J., Van Soest, R. W. M., Kelly, M., Travers, S. A. A., and Bradshaw, B., et al. (2011). Phylogenetic relationships of the marine Haplosclerida (Phylum Porifera) employing ribosomal (28S rRNA) and mitochondrial (cox1, nad1) gene sequence data. PLoS ONE 6:e24344. doi: 10.1371/journal.pone.0024344
Redmond, N. E., Van Soest, R. W. M., Kelly, M., Raleigh, J., Travers, S. A. A., and McCormack, G. P. (2007). Reassessment of the classification of the Order Haplosclerida (Class Demospongiae, Phylum Porifera) using 18S rRNA gene sequence data. Mol. Phylogenet. Evol. 43, 344–352. doi: 10.1016/j.ympev.2006.10.021
Reft, A. J., and Daly, M. (2012). Morphology, distribution, and evolution of apical structure of nematocysts in hexacorallia. J. Morphol. 273, 121–136. doi: 10.1002/jmor.11014
Reveillaud, J., Maignien, L., Murat Eren, A., Huber, J. A., Apprill, A., and Sogin, M. L., et al. (2014). Host-specificity among abundant and rare taxa in the sponge microbiome. ISME J. 8, 1198–1209. doi: 10.1038/ismej.2013.227
Richelle-Maurer, E., Boury-Esnault, N., Itskovich, V. B., Manuel, M., Pomponi, S. A., van de Vyver, G., et al. (2006). Conservation and phylogeny of a novel family of non-Hox genes of the Antp class in Demospongiae (porifera). J. Mol. Evol. 63, 222–230. doi: 10.1007/s00239-005-0294-x
Rix, L., Goeij, J. M., de, Mueller, C. E., Struck, U., Middelburg, J. J., and van Duyl, F. C., et al. (2016). Coral mucus fuels the sponge loop in warm- and cold-water coral reef ecosystems. Sci. Rep. 6:18715. doi: 10.1038/srep18715
Roper, K. E., Beamish, H., Garson, M. J., Skilleter, G. A., and Degnan, B. M. (2009). Convergent antifouling activities of structurally distinct bioactive compounds synthesized within two sympatric Haliclona demosponges. Mar. Biotechnol. 11, 188–198. doi: 10.1007/s10126-008-9132-7
Russell, B., Degnan, B., Garson, M. J., and Skilleter, G. (2003). Distribution of a nematocyst-bearing sponge in relation to potential coral donors. Coral Reefs 22, 11–16. doi: 10.1007/s00338-002-0271-4
Tamura, K., Peterson, D., Peterson, N., Stecher, G., Nei, M., and Kumar, S. (2011). MEGA5: molecular evolutionary genetics analysis using maximum likelihood, evolutionary distance, and maximum parsimony methods. Mol. Biol. Evol. 28, 2731–2739. doi: 10.1093/molbev/msr121
Taylor, M. W., Radax, R., Steger, D., and Wagner, M. (2007). Sponge-associated microorganisms: evolution, ecology, and biotechnological potential. Microbiol. Mol. Biol. Rev. 71, 295–347. doi: 10.1128/MMBR.00040-06
Thoms, C., and Schupp, P. (2007). Chemical defense strategies in sponges: a review. Porifera Res. 28, 627–637.
Van Soest, R. W. M. (2017). Flagellia, a new subgenus of Haliclona (Porifera, Haplosclerida). EJT. 351, 1–48. doi: 10.5852/ejt.2017.351
Van Soest, R. W. M., Boury-Esnault, N., Hooper, J. N. A., Rützler, K., de Voogd, N. J., Alvarez, B., et al. (2018). World Porifera database. Haploscleromorpha. Available online at: http://www.marinespecies.org/porifera/porifera.php?p=taxdetailsandid=607949 on 2018-08-27
Van Soest, R. W. M., Boury-Esnault, N., Vacelet, J., Dohrmann, M., Erpenbeck, D., Voogd, N. J., et al. (2012). Global diversity of sponges (Porifera). PLoS ONE 7:e35105. doi: 10.1371/journal.pone.0035105
Wörheide, G., Erpenbeck, D., and Menke, C. (2007). The Sponge Barcoding Project: aiding in the identification and description of poriferan taxa. Porifera Res. 28, 123–128.
Xia, X., and Lemey, P. (2009). “Assessing substitution saturation with DAMBE,” in The Phylogenetic Handbook: A Practical Approach to DNA and Protein Phylogeny, Vol. 2, eds P. Lemey, M. Salemi, and A.-M. Vandamme (Cambridge: Cambridge University Press), 615–630.
Keywords: Haliclona, nematocysts, phylogeny, new sponge species, kleptocnidism, SEM, sponge cell aggregates (SCA)
Citation: Schellenberg J, Reichert J, Hardt M, Schmidtberg H, Kämpfer P, Glaeser SP, Schubert P and Wilke T (2019) The Precursor Hypothesis of Sponge Kleptocnidism: Development of Nematocysts in Haliclona cnidata sp. nov. (Porifera, Demospongiae, Haplosclerida). Front. Mar. Sci. 5:509. doi: 10.3389/fmars.2018.00509
Received: 08 July 2018; Accepted: 20 December 2018;
Published: 14 January 2019.
Edited by:
Zhijun Dong, Yantai Institute of Coastal Zone Research (CAS), ChinaReviewed by:
Emilio Lanna, Federal University of Bahia, BrazilAllen Collins, National Oceanic and Atmospheric Administration (NOAA), United States
Copyright © 2019 Schellenberg, Reichert, Hardt, Schmidtberg, Kämpfer, Glaeser, Schubert and Wilke. This is an open-access article distributed under the terms of the Creative Commons Attribution License (CC BY). The use, distribution or reproduction in other forums is permitted, provided the original author(s) and the copyright owner(s) are credited and that the original publication in this journal is cited, in accordance with accepted academic practice. No use, distribution or reproduction is permitted which does not comply with these terms.
*Correspondence: Johannes Schellenberg, johannes.schellenberg@allzool.bio.uni-giessen.de