- National Oceanography Centre, University of Southampton, Waterfront Campus, Southampton, United Kingdom
The sieve mesh sizes used in benthic foraminiferal studies exert a strong influence on faunal densities and composition. We examined the consequences of including finer (63–150 μm) size classes in a study of Rose Bengal stained (‘live’) and dead foraminifera in 5 Megacorer samples (0–1 cm layer) from abyssal sites in the eastern Clarion-Clipperton Zone (CCZ; equatorial Pacific), a region with commercially significant deposits of polymetallic nodules. More than 60% of intact specimens originated from the finer (<150 μm) fractions, with over half being picked from 63 to 125 μm residues. Test fragments, mainly agglutinated tubes, were also abundant but were more evenly distributed between coarser and finer residues. The two fractions yielded the same main groups (a mixture of formal taxa and informal groupings) and were dominated by single-chambered forms (‘monothalamids’), the majority undescribed. Some were disproportionately abundant in finer fractions: rotaliids in the stained (‘live’), textulariids in the dead, and trochamminids, Lagenammina spp., Nodellum-like forms, saccamminids and spheres in both assemblages. However, the most striking difference was the much greater abundance of tiny, largely undescribed spherical agglutinated morphotypes in the <150-μm fractions. Our 5 samples yielded 462 morphospecies, of which 313 occurred in <150-μm fraction and 170 were confined to this fraction. Twelve of the 31 top-ranked species in the stained assemblage were more or less limited (>90%) to the finer fractions; the corresponding number for the stained + dead assemblage was 12 out of 35. Of the 46 most abundant species in the stained + dead assemblage, 35 were monothalamids (mainly spheres, Lagenammina spp., Nodellum-like forms, and saccamminids), the remainder being rotaliids (3), hormosinids (3), trochamminids (3) and textulariids (2). By far the most abundant species overall, a tiny agglutinated sphere, was almost entirely confined to the finer fractions. Although small foraminifera that pass through a 150-μm screen are time-consuming to analyze, they constitute an important part of abyssal Pacific assemblages and may include opportunistic species that respond to episodic food pulses as well as pioneer recolonizers of defaunated substrates. It is therefore important to consider them in studies of possible mining impacts on abyssal benthic communities.
Introduction
The use of different sized meshes to sieve sediment samples is an important issue in meiofaunal research, the mesh acting as a filter that strongly influences important assemblage metrics, notably faunal densities, biomass, diversity and taxonomic composition of taxa such as nematodes (Leduc et al., 2010). A mesh size of 32 μm is now the widely accepted lower limit for the metazoan meiofauna. In the case of foraminifera, however, different studies have used a variety of different sized meshes (Sen Gupta et al., 1987). The most common are 150, 125, and 63 μm, although larger (250 or 300 μm; e.g., Bernstein et al., 1978; Lutze and Coulbourne, 1984; Gooday et al., 2001) or smaller (45 or 32 μm; e.g., Gooday, 1986; Pawlowski and Lapierre, 1988; Pawlowski, 1991; Gooday et al., 1995; Nozawa et al., 2006; Szarek et al., 2007) sizes have been adopted occasionally. The need to analyze finer sieve fractions (63–125 μm or 63–150 μm) becomes more pressing in deep-sea settings, where benthic organisms are generally small in size compared to shallower waters (Thiel, 1975, 1983; Shirayama and Horikoshi, 1989). Although this involves a larger investment of time compared to the analysis of larger-sized fractions, Schröder et al. (1987) argue that the extra effort is worthwhile because valuable faunal information, including a large proportion of specimens belonging to environmentally important ‘indicator’ species, is lost by ignoring sieve residues <150 μm. For example, at bathyal depths in the North Atlantic, small-sized species include several that respond opportunistically to seasonally pulsed food inputs (Gooday and Hughes, 2002; Duchemin et al., 2007; Phipps et al., 2012).
Relatively few studies based on fine sieve fractions have been undertaken in the Pacific Ocean. Snider et al. (1984) analyzed the 45–150 and 150–300 μm fractions for meiofaunal organisms, mainly foraminifera, many of them single-chambered forms (monothalamids). More recently, Nozawa et al. (2006) used a 32 μm mesh in a study of foraminifera, again dominated by monothalamids, from the ‘Kaplan East’ site in the eastern Clarion-Clipperton Zone (CCZ, abyssal equatorial Pacific), a region where large tracts of seafloor are covered with commercially significant deposits of polymetallic nodules. Radziejewska et al. (2006) presented some preliminary observations of monothalamids and other foraminifera based on the >32-μm fractions of samples from the same general area. Ohkawara et al. (2009) described a tiny new spherical monothalamid that was abundant in samples from the Kaplan Central site in the deeper and more central part of the CCZ. The present contribution is part of the AYSSLINE (ABYSSal baseLINE) project, a baseline survey of foraminifera in two areas, also in the eastern CCZ, licensed by the International Seabed Authority for seabed exploration to the UK Seabed Resources Ltd. (UK-1 area), and Ocean Mineral Singapore Pte Ltd. (OMS area). The UK-1 area was sampled in 2013 and both areas were sampled in 2015 during ABYSSLINE research cruises. Previous studies on meiofaunal foraminifera within the framework of this project have described novel species that use radiolarian shells as microhabitats (Goineau and Gooday, 2015) and foraminiferal composition and diversity in >150 μm size fractions (Goineau and Gooday, 2017). Here, we extend this earlier research by describing assemblages of stained and dead benthic foraminifera retained on 63 and 125 μm mesh sieves and comparing them with assemblages in residues >150 μm. We address two main questions. (1) How much information on foraminiferal abundance and diversity is added by analyzing these finer fractions? (2) What are the differences in foraminiferal assemblage composition between the larger and smaller size fractions?
Materials and Methods
General information about the ABYSSLINE project is given by Glover et al. (2015). For environmental information about the study area see Amon et al. (2016) and Goineau and Gooday (2017). Pape et al. (2017) provide further environmental data (sediment granulometry, porosity and sorting, total organic carbon and total nitrogen content) for the GSR license area, located to the west of the UK-1 and OMS areas and in somewhat deeper water (∼4500 m). In general terms, our shipboard and laboratory methods followed those described by Goineau and Gooday (2017). We use the term ‘stained’ to refer to specimens that were inferred to be alive when stained.
Sampling
Samples were collected during two ABYSSLINE cruises at 5 sites (Supplementary Table S1) in three 30 × 30 km study areas (‘strata’) located within the UK-1 and OMS exploration contract areas (Figure 1). Two were obtained in Stratum A of the UK-1 license area, centered around 13°49′ N, 116°36′ W, during the first cruise (AB01, R/V Melville, cruise MV1313, 3–27 October 2013), two in UK-1 Stratum B, centered around 12° 28.9′ N, 116° 36.3′ W, and one in the OMS Stratum, centered around 12° 8.2′ N, 117° 17.7′ W during the second cruise (AB02: R/V Thomas G Thompson cruise TN319; 12 February to 25 March, 2015). In each case the samples were recovered using a hydraulically dampened Megacorer (BCMEGA OSIL Bowers & Connelly type), equipped with 12 polycarbonate coring tubes. As soon as possible after collection, the cores were sliced, using a metal plate, into 0.5-cm thick layers to 2 cm depth and 1-cm thick layers from 2 to 10 cm depth and each layer preserved separately in a 500 ml plastic bottle with 10% formalin buffered with borax. Any nodules present in the core were carefully removed in order to facilitate slicing and sediment adhering to them washed into the appropriate sample bottle.
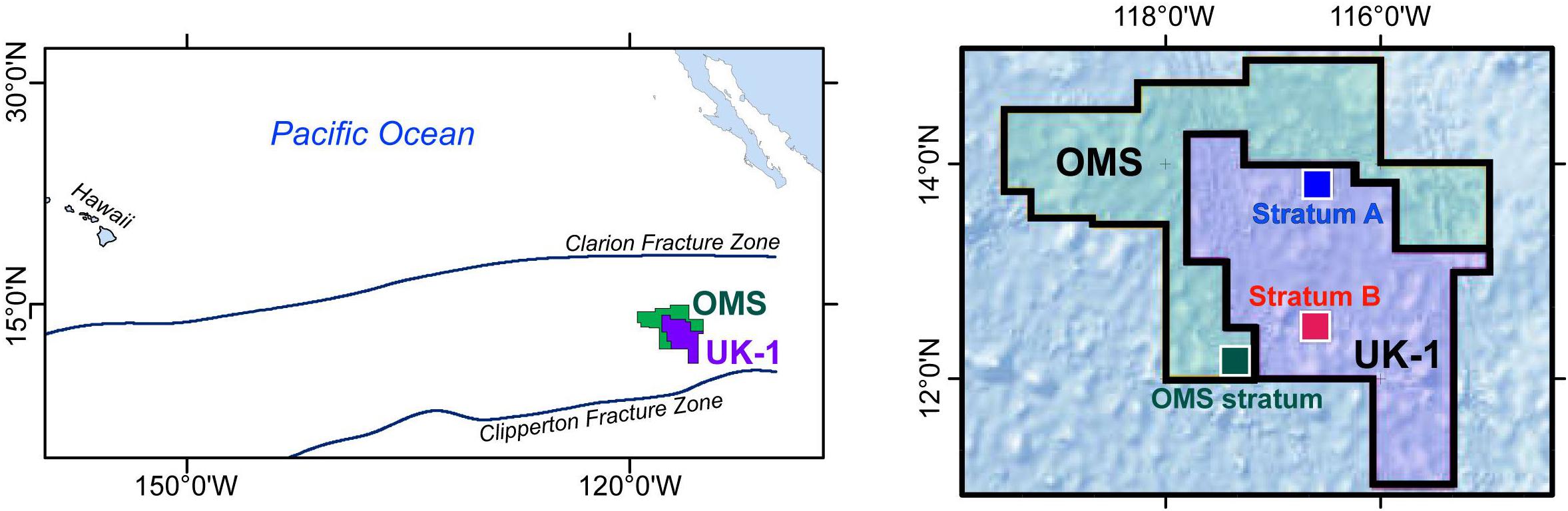
Figure 1. (Left) Location of the Ocean Mineral Singapore (OMS) and UK-1 exploration contract areas in the eastern Clarion-Clipperton Zone, equatorial Pacific. (Right) Location of UK-1 Strata A and B and the OMS stratum within the two contract areas.
Laboratory Processes
The 0–0.5 and 0.5–1.0 cm sediment layers were used for this study. In the laboratory, the volume of each slice was measured by settling the sediment in a graduated cylinder for 2 days (after which the volume stabilized) followed by splitting into 8 parts using a wet splitter (Jensen, 1982). One split was selected for analysis and its volume measured in the same way. It was then sieved on a series of meshes (300, 150, 125, 63 μm) and each residue stained overnight on the sieve in Rose Bengal solution (1 g in 1 l of tapwater). The stained residues were transferred in water to a Petrie dish and examined under a stereo-microscope. All foraminifera (stained and unstained) and metazoan meiofaunal animals were removed using a glass pipette. In most cases they were stored on cavity slides in a small volume of glycerol, the cavities being left uncovered in order to allow easy access to the specimens. Some delicate organic-walled foraminifera that shrank in glycerol were placed in 1.25 ml Nalgene® cryovials, while calcareous foraminifera, which were rare, were placed on dry slides in order to avoid dissolution in the glycerol. Foraminifera with stained cytoplasm and (if present) fresh stercomata were considered to have been alive when sampled (see Supplementary Material for discussion of criteria for distinguishing stained from dead tests).
All foraminiferal specimens were examined under an Olympus BH-2 compound microscope and photographed using an SLR digital camera (Canon EOS 350D) attached to the microscope. Calcareous and delicate organic-walled foraminifera were placed in a cavity slide with water for this purpose, but the majority of specimens were photographed in glycerol. Specimens were grouped into working morphospecies based mainly on observations of test morphology and wall structure, and assigned to genera or species where possible. Those that could not be placed in known taxa were given a descriptive working name.
Some of the methodological challenges involved in analyzing benthic foraminifera in sediment residues from the abyssal CCZ are outlined in the Supplementary Material.
Statistical Methods
T-tests to compare the relative abundances of major groups in the >150 and <150 μm fractions were computed using Excel. Based on complete specimens only (stained and stained plus dead combined), we used the open source software EstimateS (Version 9) (Colwell et al., 2012) to calculate the Fisher alpha (α) and Shannon (H’, using natural logarithm) diversity indices, and rarefied species richness values [E(S100)] – the expected number of species represented by 100 individuals) using the open source software EstimateS (Version 9) (Colwell et al., 2012).
Results
Abundance
We picked a total of 2341 complete stained (‘live’) foraminiferal tests, 1093 complete dead tests, and 1381 fragments (stained and dead combined) from the >300, 150–300, 125–150, and 63–125 μm fractions combined of the five 1/8th sample splits. Individual splits yielded 269–777 (=274–791.10 cm-2) stained tests, 125–329 (=127–335.10 cm-2) dead tests and 115–497 (=117–506.10 cm-2) test fragments.
A majority (62.2%) of complete stained foraminiferal tests originated from the two finer sieve fractions (<150 μm; i.e., 63–125 and 125–150 μm combined), with more than half (52.7% overall) being found in the 63–125 μm fraction alone (Table 1). The dead assemblage showed a similar proportion; 64.8% overall in the two finer fractions with 53.7% in the 63–125 μm fraction. Fragmented tests (live + dead combined, mainly tube fragments) were more evenly distributed between the two fractions, with 47.5% originating from the >150 μm and 52.5% from the <150 μm fraction (Table 2).
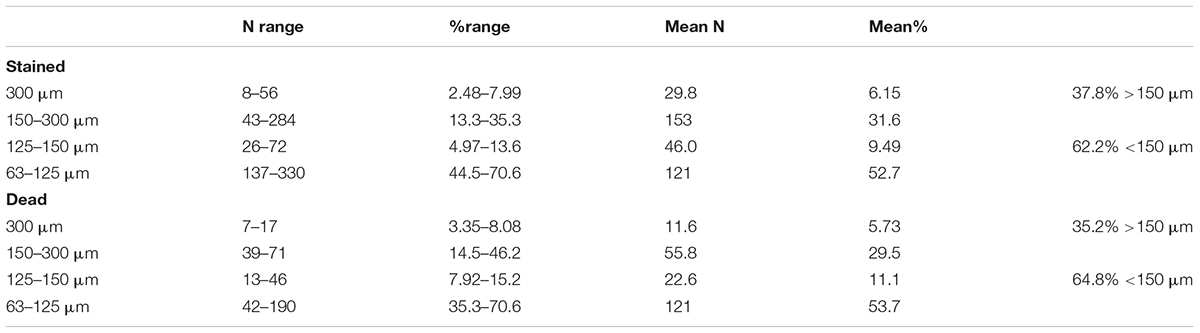
Table 1. Numbers and percentages of complete foraminiferal tests (stained + dead) in the 5 sample splits of which the >150 μm and 63–150 μm fractions were analyzed.

Table 2. Numbers and percentages of fragmentary foraminiferal tests (stained + dead) in the 5 sample splits of which the >150 μm and 63–150 μm fractions were analyzed.
Main Groups
Species were divided into a series of main categories, a mixture of formal taxa (e.g., rotaliids, hormosinids, trochamminids) and informal morphology-based groupings (e.g., spheres, tubes, and ‘other monothalamids’). The latter encompasses monothalamids that are difficult to catorgorise morphologically; some examples are illustrated in Supplementary Figures S1A–C,E–G, S2A,B. The same main taxa and morphological groupings are represented in both the >150 μm and <150 μm fractions (Tables 3, 4). Among groups represented by complete tests (stained and dead), most tend to be more abundant in absolute terms in the finer fractions. This difference is particularly clear in the case of rotaliids and trochamminids (Figures 2A,D–H and Supplementary Figures S3C–E, S4A–D), Lagenammina spp. (Supplementary Figures S5A–F), Nodellum-like forms (Supplementary Figure S6), saccamminids (Supplementary Figure S7), spheres (Figure 4; Supplementary Figure S8), and organic-walled forms (Supplementary Figures S9A–E) in the live assemblage, and textualariids (Figures 2B,C and Supplementary Figures S4E,F), trochamminids, Lagenammina spp., Nodellum-like forms, saccamminids, and spheres in the dead assemblage. On the other hand, complete tubes and spindles, chains, ‘other monothalamids’ and Komokiacea (‘komoki’; families Baculellidae and Komokiidae, putative monothalamids; Figure 3K and Supplementary Figure S1D) are more abundant in the coarser fractions, particularly in the case of stained specimens. The only groups that are significantly more abundant in the finer fractions, however, are the saccamminids and spheres (Tables 3, 4). The difference is particularly striking in the case of the stained spheres, which, on average, are some 5 times more abundant in the 63–150 μm than in the >150 μm fraction. This difference is highly significant (p < 0.001) for stained specimens (Table 3) and significant (p < 0.05) for the dead specimens (Table 4). These tiny spheres form a substantial proportion of the stained assemblage. They are largely undescribed and in most cases regarded as indeterminate since it was not possible to consistently distinguish morphologically distinct types amongst them. Some examples of the more common forms are shown in Figure 4 and Supplementary Figure S8.
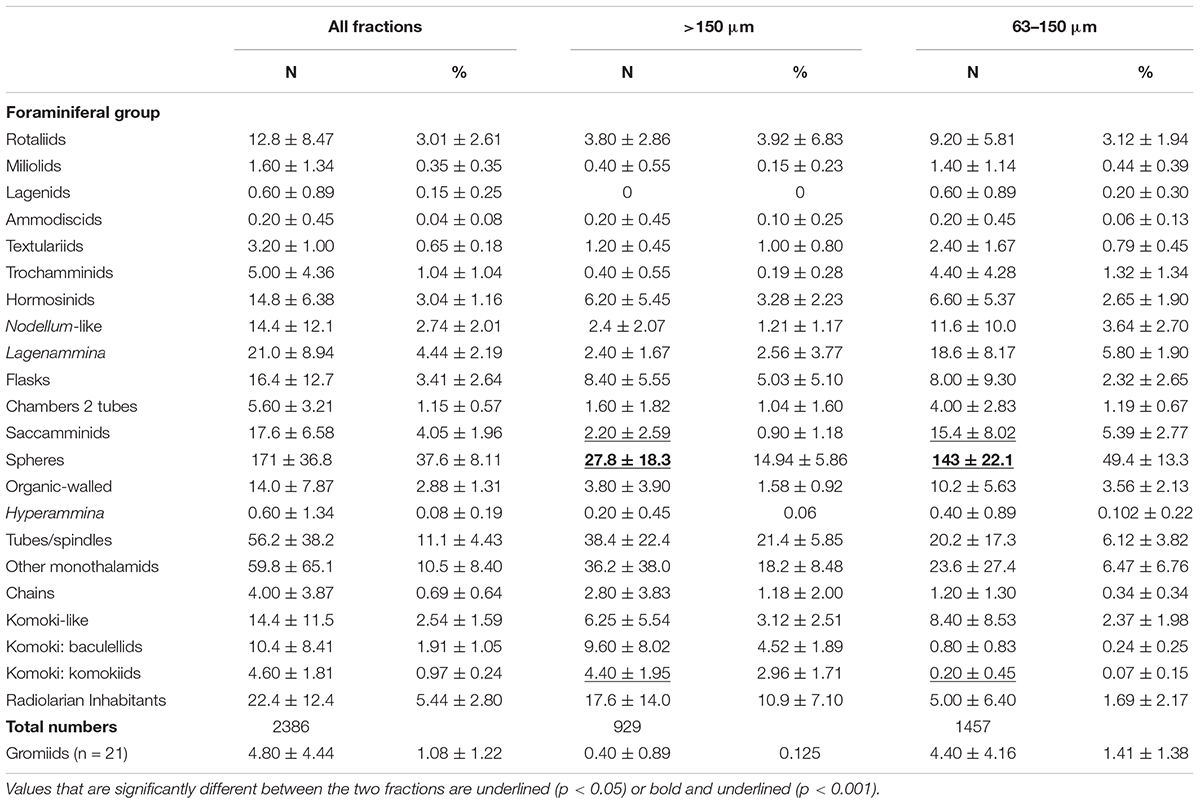
Table 3. Mean and percentage abundances (±standard deviations) of major groups based on complete stained specimens in the two size fractions and both fractions combined of 5 samples.
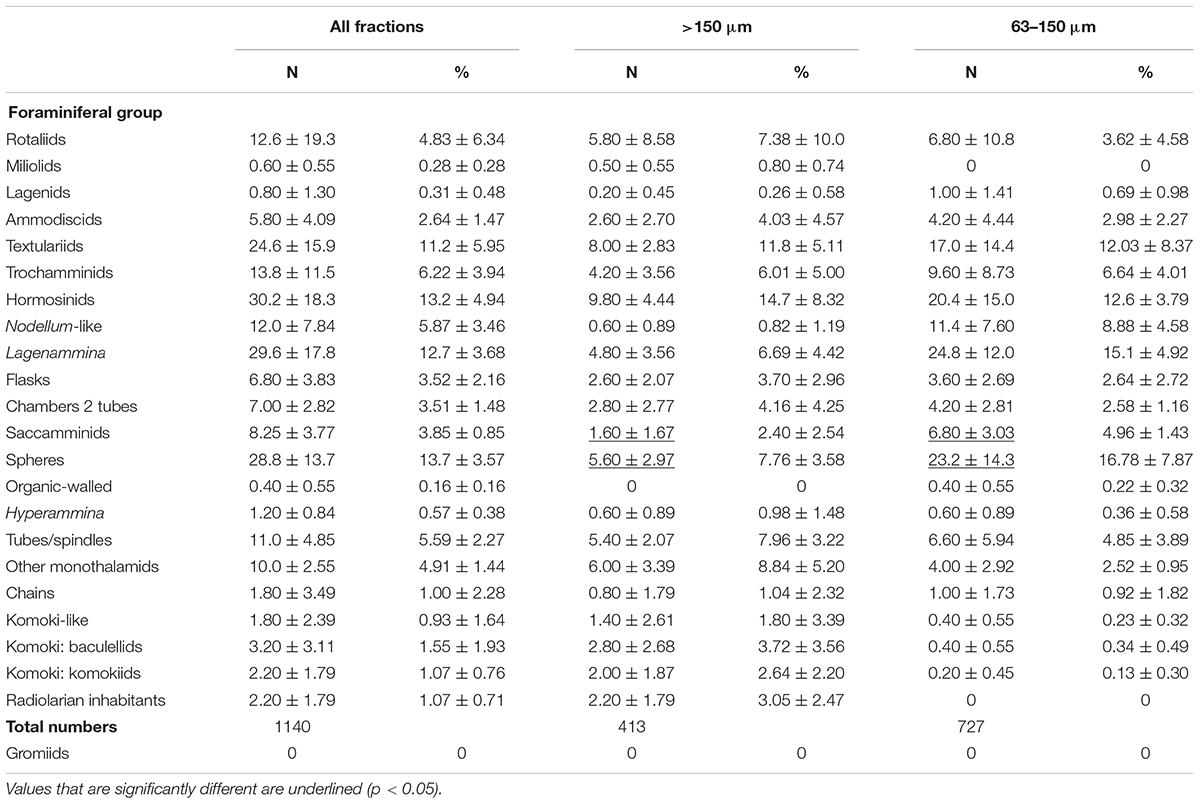
Table 4. Mean and percentage abundances (± standard deviations) of major groups based on complete dead specimens in the two size fractions and both fractions combined of 5 samples.
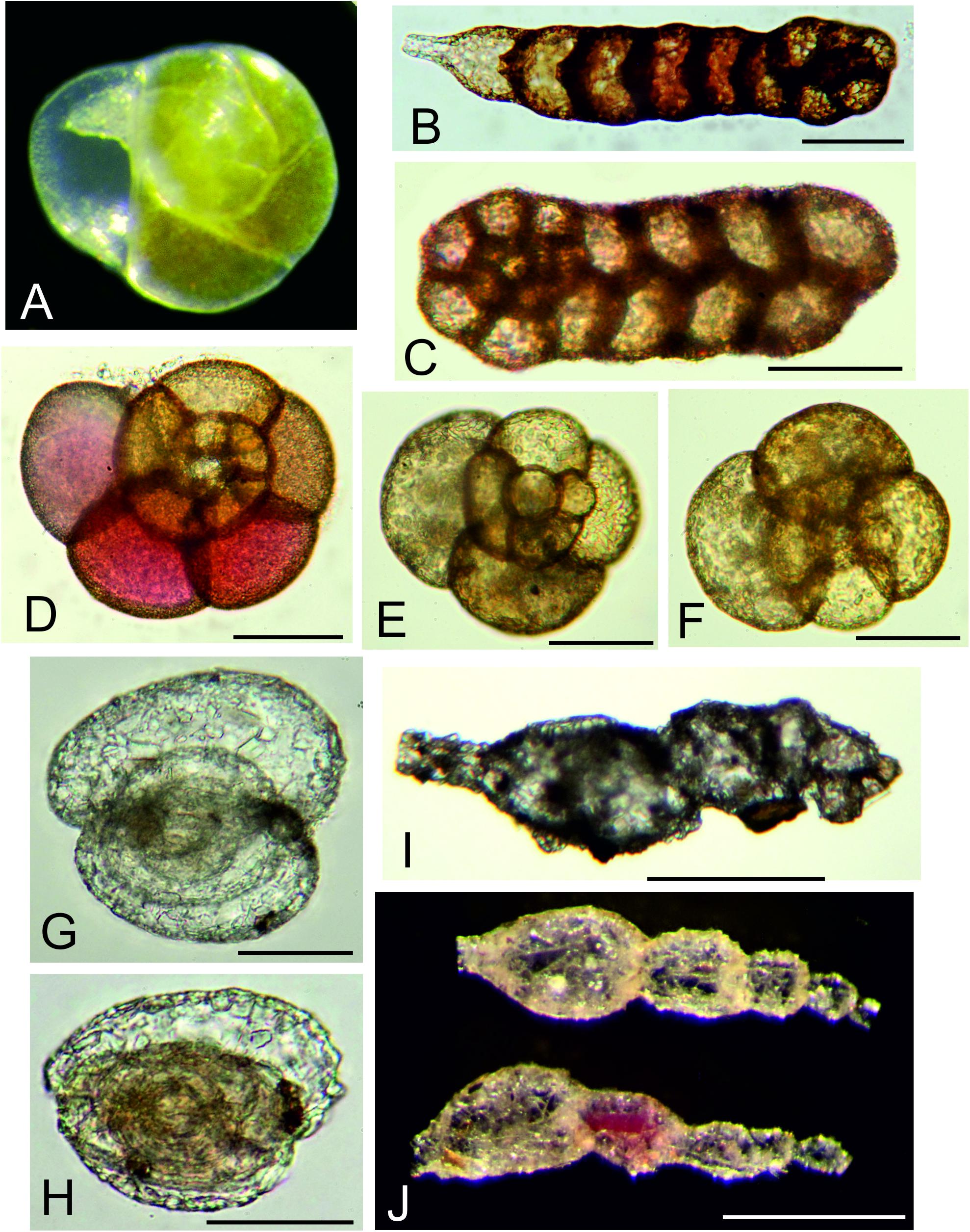
Figure 2. Multichambered foraminifera. (A) Epistominella exigua Brady; ‘live’ specimen with green cytoplasm, picked from >150 μm residue at sea. (B) Ammobaculites filiformis Earland; MC09, 0–0.5 cm, 63–125 μm fraction. (C) Spiroplectammina subcylindrica Earland; MC21 G 0–0.5 cm, 63–125 μm. (D) Trochamminacean sp. 1; MC21, 0.5–1.0 cm, 63–125 μm fraction. (E,F) Trochamminacean sp. 2; MC21, 0.5–1.0 cm, 63–125 μm fraction. (G,H) Adercotryma sp.; MC21, 0.5–1.0 cm, 63–125 μm fraction. (G) Rounded specimen. (H) More elongate specimen. (I) Reophax scorpiurus Montfort, MC11, 0.5–1.0 cm, 150–300 μm fraction. (J) Reophax sp. 1, MC05, 0–0.5 cm, >300 μm fraction. Scale bars = 50 μm (A–H), 250 μm (I), 500 μm (J).
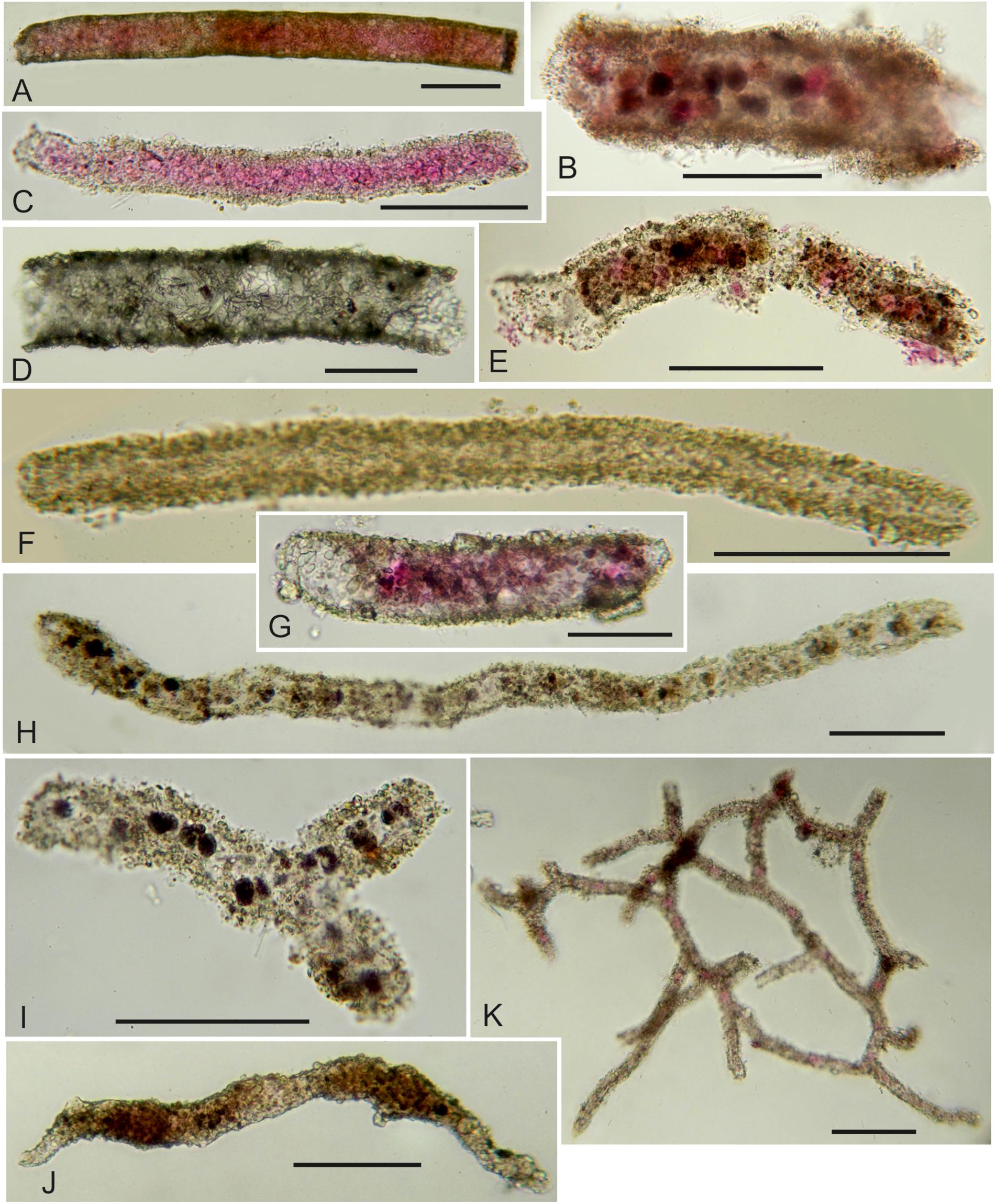
Figure 3. (A–F) Undescribed tubular foraminifera (fragments unless stated otherwise). (A) Stained; MC21, 0.5–1.0 cm, 63–125 μm fraction. (B) Stained with stercomata; MC13, 0–0.5 cm, 125–150 μm fraction. (C) Stained; MC11, 0–0.5 cm, 63–125 μm fraction. (D) Dead; MC13, 0–0.5 cm, 125–150 μm fraction. (E) Stained; MC09, 0–0.5 cm, 63–125 μm fraction. (F) Dead; MC21, 0.5–1.0 cm, 63–125 μm fraction. (G) Stained, complete test; MC05, 0.5–1.0 cm, 63–125 μm fraction. (H) Dead tubular fragment with stercomata; MC11, 0–0.5 cm, 63–125 μm fraction. (I) Complete tubular specimen; MC11, G, 0–0.5 cm, 63–125 μm fraction. (J) Marsipella sp.; MC11, 0–0.5 cm, 63–125 μm fraction. (K) Stained fragment of Reticulum (komokiacean); MC13, 0–0.5 cm, 125–150 μm fraction. Scale bars = 100 μm.
Test fragments (stained and dead combined) are dominated by tubes and spindle-shaped morphotypes (grouped together), with lesser contributions from komokiaceans (Baculellidae and Komokiidae) (Figure 3). However, the only clear differences between fractions are the greater average absolute and relative abundance of baculellid fragments in the coarser fractions and, to a lesser extent, of komokiids in the finer fractions (Supplementary Table S2). The differences were not significant (p > 0.05), however. In the case of the komokiids, it reflected the large number of small fragments of a Reticulum species (Figure 3K) in the 63–125 and 125–150 μm fractions of the MC13 sample.
Table 5 presents data for the calcareous and agglutinated taxa that have more robust tests and therefore the potential to survive in the fossil record. We include here all of the multichambered foraminifera (Globothalamea and Tubulothalamea) (Figure 2 and Supplementary Figures S3A–E, S4), together with species of Lagenammina (Supplementary Figures S5A–F), which, although monothalamous, have more or less rigid test walls and are known as fossils. Hormosinids (23.2% of stained and 25.1% of dead specimens) and Lagenammina species (40.8% of stained and 25.0% of dead specimens) are abundant constituents of the both stained and dead assemblages when the coarser and finer fractions are combined, and are joined in the dead assemblage by the textulariids (21.5%). Rotaliids are relatively more common in the stained (19.6%) than in the dead (8.38%) assemblage. The overall composition of the >150 μm and <150 μm assemblages is similar. However, hormosinids are relatively less abundant in the finer fractions (stained 19.1% vs. 33.7%; dead 23.2% vs. 30.2%), a pattern reversed in the case of Lagenammina spp. (stained 42.7% vs. 36.0%; dead 30.2% vs. 11.3%).
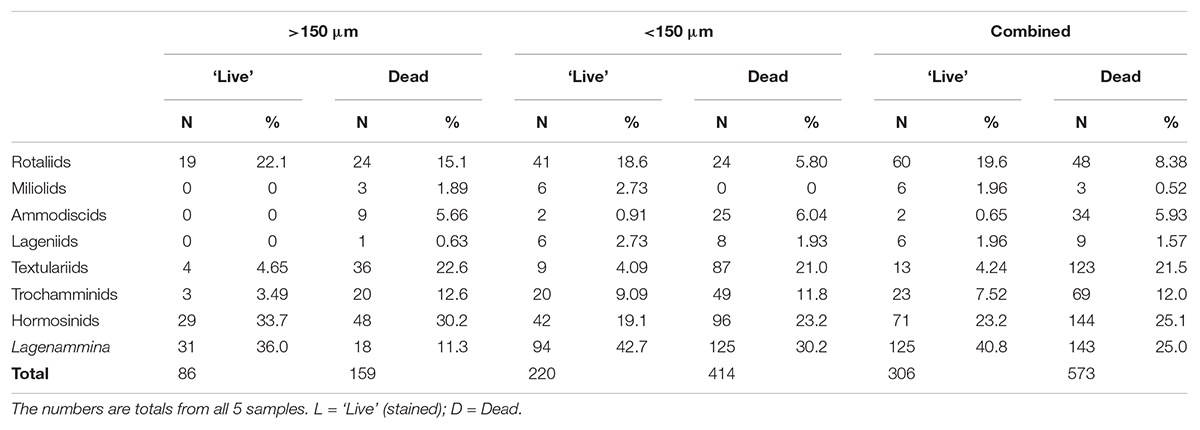
Table 5. Absolute and relative abundance of complete foraminifera belonging to more robust (fossilizable) taxa, mainly multichambered.
Common Species
Supplementary Tables S3–S6 detail the 30 or so most abundant species in the stained + dead and stained only assemblages; in each case, data for all fractions combined, and the <150 μm fraction only, are shown separately. All 44 species listed in these tables are illustrated in Figures 2–4 and the Supplementary Figures and brief descriptions are given the taxonomic notes included in the Supplementary Material. More than three-quarters (34) are monothalamids, among which spheres, Lagenammina species, and ‘other monothalamids’ make up the majority. Most of the multichambered species are textulariids, including several Reophax species. By far the most abundant species, both overall and in particular categories, is a small agglutinated sphere (Psammosphaerid sp. 20) that is almost entirely confined (99.4%) to the finer residues. Seven other species (Spiroplectammina subcylindrica, ‘lobed spheres,’ Lagenammina yellowish with long neck, Lagenammina sp. 9, Saccamminid sp. A, Saccamminid sp. 5) are found exclusively in the <150 μm fractions (stained + dead assemblage). The 30 most common species (stained + dead) in the <150-μm fraction include 23 that are also in the top 32 for the combined residues (>150 + <150 μm). In the case of the stained-only assemblage in the <150-μm fraction, the top 30 species include 22 that are also among the top-ranked species in the two residues combined; these include all of the 17 most abundant species.
Diversity
The five samples combined yielded a total of 462 provisional morphospecies of testate protists (stained and dead, complete and fragmentary tests) that were regarded as foraminifera. Of these, 54 were represented only by fragmented specimens, 279 occurred in the >150 μm fraction, 313 occurred in the <150-μm fractions and 170 were confined to these fine fractions (Table 6). The most diverse groups were the tubes with 77 species, 20 of them confined to fractions <150 μm, and the spheres, with 61 species, 25 confined to the fine fractions. Among multichambered groups, textulariids (32 species, 18 only in <150 μm fractions) and hormosinids (30 species, 6 only in <150 μm fractions) were the most specious. If the >150 μm dataset is expanded to include six additional sample splits for which the fine fractions were not analyzed (Goineau and Gooday, unpublished), then the number of morphospecies found only in the >63 μm fraction is halved from 170 to 84.
Detailed diversity metrics based on complete individuals are summarized in Table 7 (stained plus dead specimens combined) and Table 8 (stained specimens only). Species numbers and diversity indices [H′, Fisher α, E(S100)] are generally higher in samples that include the 63–125-μm plus 125–150-μm fractions compared to those based on only the 150–300-μm plus >300 μm fractions. Except for sample MC21, where stained specimens were 3.5 times more abundant in the finer than the coarser residues, Rank 1 Dominance (R1D) is higher in the case of the <150-μm assemblages. However, inclusion of the finer fractions has an inconsistent influence on R1D values when the two fractions are combined.
Gromiids, Unknown Testate Structures, and Metazoan Meiofauna
The <150-μm residues yielded 21 specimens with transparent organic tests that were regarded as gromiids based on the presence of an oral capsule (Hedley, 1960; Rothe et al., 2010). Most had more or less elongate, droplet-like tests that tapered toward the oral capsule (Supplementary Figures S9G,I), but a few were sausage-shaped (Supplementary Figure S9F) or spherical (Supplementary Figure S9H). They are tentatively assigned to 8 morphospecies, all confined to the fine fractions, although variability in test morphology made it difficult to discriminate between some of these forms.
The fine fractions (mainly the 63–125 μm fraction) of some samples also yielded a variety of more or less spherical, organic-walled structures that gave rise to relatively short blind-ending processes (Figure 5). They were concentrated in the MC11 and MC13 samples and included three main types. Type A varies in shape from spherical to oval or somewhat irregular and is characterized by a variable number (2–10) of short tubular extensions with longitudinal striations and ending in a thin-walled bulb. This was by far the commonest form, particularly in the MC13 sample where it was represented by 174 specimens. Type B has relatively longer, narrower, and often somewhat tapered tubular processes, sometimes ending in a small bulb. Three specimens were found inside other structures in the MC11 sample. Type C is spherical and was represented by 2 specimens from the MC13 sample with several (3 and 5) rounded bumps on the surface of the organic wall.
A total of 167 metazoan meiofaunal organisms (not counting 7 polychaete fragments without heads), all of which were well stained and assumed to have been alive when collected, were picked from the 5 sample residues (i.e., 1/8th splits of the top 1-cm layer of five 10-cm diameter sediment cores). This number corresponds to an overall density of 34.0 individuals per 10 cm2. Nematodes were the most common metazoans (62.3%), followed by harpacticoid copepods (19.7%), nauplii (15.6%), 2 ostracods, 1 bivalve and 1 kinorhynch.
Discussion
Stained complete foraminiferal tests were more than an order of magnitude more abundant in our five sample splits from the eastern CCZ than metazoan meiofaunal animals (i.e., 2341 stained tests compared with 167 metazoans, corresponding to overall densities of 496 and 34 individuals per 10 cm2, respectively). The metazoan densities are generally lower than those typical for the CCZ (11–394 individuals per 10 cm2 in nine studies; summarized in Radziejewska, 2014, Table 3.4 therein) and the mean values (88 ± 55 to 151 ± 54 individuals per 10 cm2) recently reported by Pape et al. (2017) from the GSR license area in the northeastern CCZ. Moreover, the proportion (62.3%) of nematodes is relatively low and the proportion of nauplii relatively high (15.6%), compared to previous studies. For example, nematodes represented 85–93% and nauplii 1.1–7.1% of the metazoan meiofauna in the study of Pape et al. (2017). These discrepancies probably reflect methodological differences. In particular, while the 63 μm lower limit used in the present study is normal for foraminifera (Murray, 2014), it is not appropriate for analyzing metazoan meiofauna, for which 32 μm is now the generally accepted lower limit (Leduc et al., 2010). Nevertheless, although metazoans were not adequately sampled, our results do suggest that stained foraminifera outnumber metazoan meiofaunal animals in CCZ sediments.
The nature of the organic-walled structures with blind-ending processes remains unresolved. They are almost certainly not dinoflagellate cysts (Dr. Ian Harding, University of Southampton, personal communication). The most likely identification, at least for the common forms from sample MC13 (Figures 5A–D), are that they are metazoan eggs, possibly those of a crustacean (Dr. Manuel Bringué; Geological Survey of Canada – Calgary).
The majority of complete stained (61.1%) as well as dead (63.8%) foraminiferal tests originated from the 63–150 μm fractions. These results are consistent with previous studies of deep-sea meiofauna, including foraminifera (e.g., Gooday, 1986; Schewe, 2001), as well as with the general tendency for small-sized organisms to become increasingly important with increasing water depth (Thiel, 1975, 1983). Duchemin et al. (2007) report that the 63–150 μm fraction of the top 1-cm of a core from 1000 m in the Bay of Biscay yielded the vast majority (97.5%) of stained foraminifera. In one of the most comprehensive studies, Phipps et al. (2012) found that stained foraminiferal densities in the 63–150 μm fraction of samples taken along a down-slope transect (262 – 4987 m water depth) on the Portuguese margin were consistently more than twice those in the >150 μm fraction. Interestingly, the proportion increased with depth, reaching a peak at 3908 m, where 88% of stained foraminifera were picked from the fine sieve residues.
Comparison With Modern Abyssal Assemblages
Fractions <150-μm were dominated by monothalamids, many of them more or less spherical forms. These tiny spheres represented an average of 37.6 ± 8.1% of complete stained foraminifera in all fractions combined and 49.4 ± 13.3% in the 63–150 μm fraction, with a significantly lower numbers in the 150–300 μm compared to the 63–150 μm fraction (Table 1). Many have delicate, fine-grained test walls and contain stercomata (Figures 4C–K). Numerous agglutinated spheres are also reported in previous studies of abyssal Pacific samples. According to Snider et al. (1984), spherical (‘sac-shaped’) morphotypes made up almost third (31.3%) of meiofaunal foraminifera in the central North Pacific (5800 m depth). Nozawa et al. (2006), found an even greater predominance (33.5 – 94.5%) of tiny, undescribed ‘psammosphaerids’ in small samples from the Kaplan East site, located immediately to the west of the UK-1/OMS area and at a similar water depth. The higher proportion probably reflects the fact that Nozawa et al. (2006) examined even finer fractions (>32 μm) fractions than those analyzed for the present study. Later, Ohkawara et al. (2009) established a new species, Saccammina minimus, based on numerous tiny agglutinated spheres from the deeper (∼5000 m) Kaplan Central site, located in the central part of the CCZ (∼14°N, 130°W). The majority of spheres in our samples are undescribed, but some are probably the same as S. minimus, while other somewhat larger forms resemble Thurammina albicans. Small agglutinated spheres also occur in the abyssal North Atlantic. They represented 7.2 – 12.4% of assemblages >63 μm at the Porcupine Abyssal Plain and an even higher proportion (17.8%, 21.0%) in two samples from the Cape Verde Abyssal Plain (Gooday, 1996). Very small agglutinated tests were reported by Gooday et al. (1995) in North Atlantic sediments sieved on 32 and 20 μm meshes. However, the spheres with a soft ‘fluffy’ wall and containing stercomata (Figures 4C–K), which are common in our samples, have not been observed in the Atlantic material.
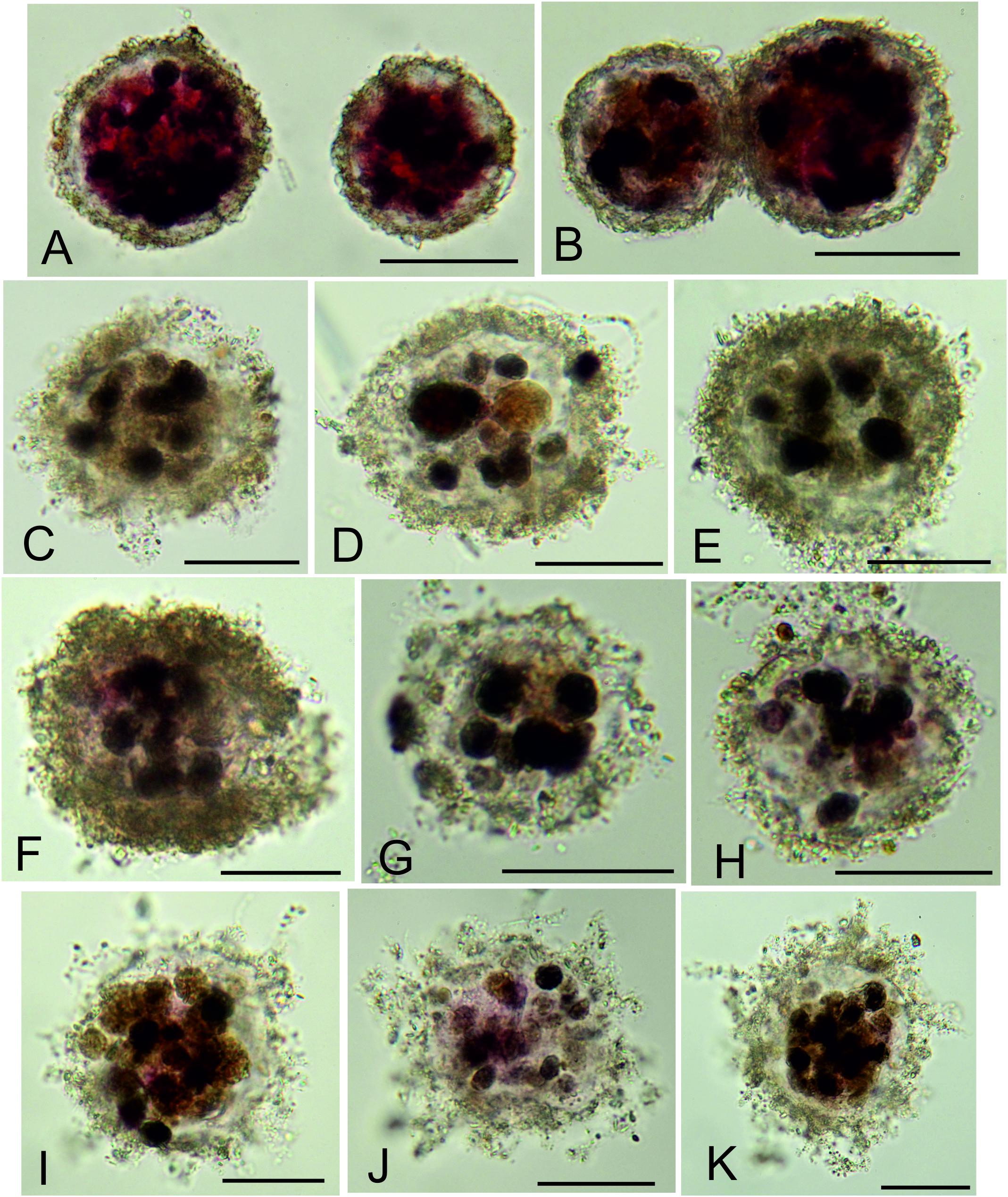
Figure 4. Undescribed spherical foraminifera from 63 to 125 μm fractions. (A,B) Psammosphaerid sp. 20, the most abundant morphospecies species in the stained assemblage; MC13, 0.5–1.0 cm. (C–H) Morphotype with delicate, fine-grained wall enclosing stercomata and sparse cytoplasm; all are from sample MC05. (I–K) Similar morphotype in which the wall includes an organic layer with finely agglutinated particles and gives rise to short filament-like structures; all are from sample MC05. Scale bars = 50 μm.
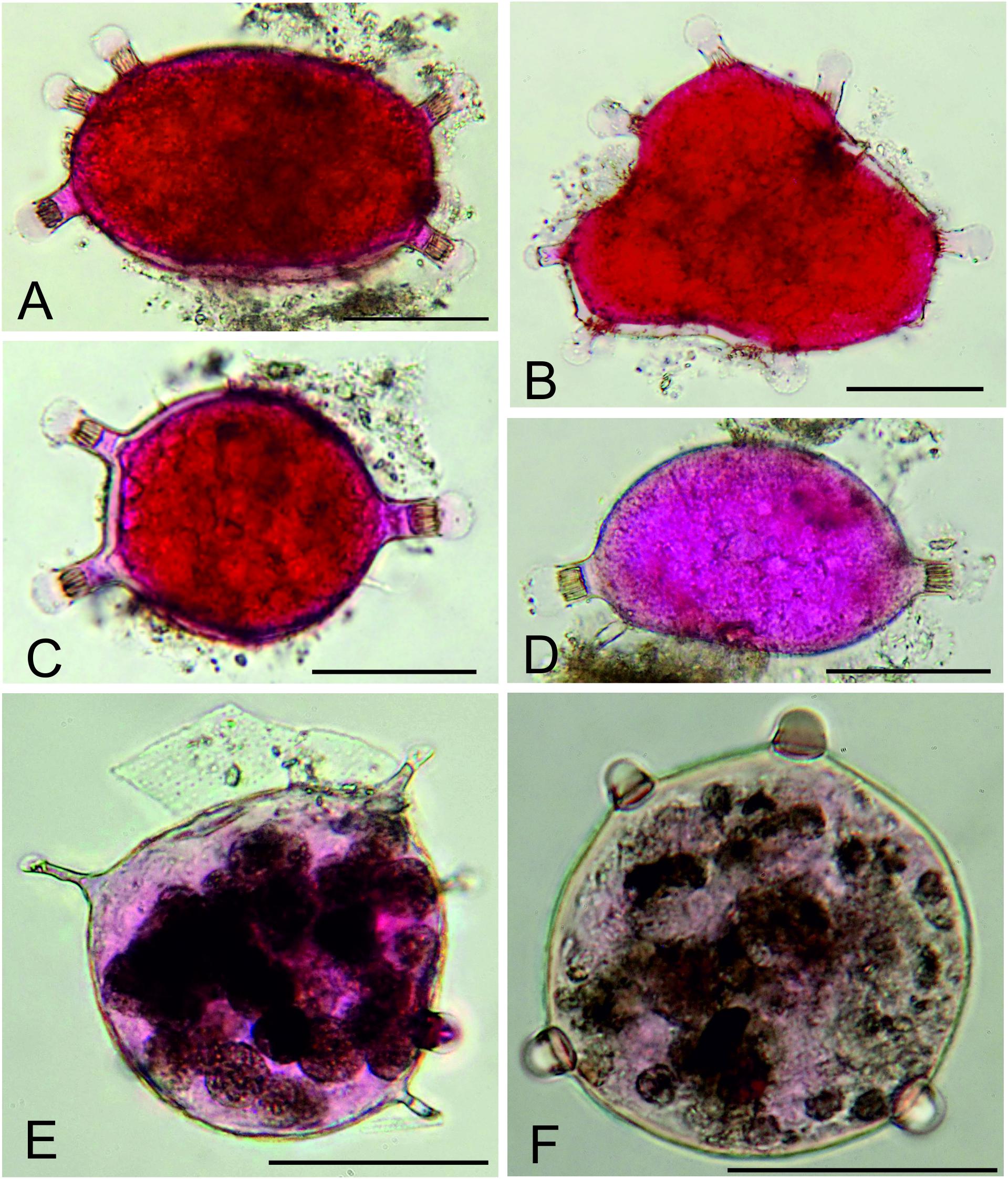
Figure 5. Unidentified organic-walled structures (?metazoan eggs) from 63–125 μm fractions. (A–C) Type A with short striated tubes ending in thin-walled bulbs; MC11, 0–0.5 cm layer. (D,E) Type B with relatively long slender tubes, sometimes ending in a small thin-walled bulb; MC11, 0–0.5 cm. (F) Type C with rounded bumps; MC13, 0.5–1.0 cm layer. Scale bars = 50 μm.
The finer residues yielded many tubular and spindle-shaped morphotypes (Figure 3). Stained specimens judged to be complete were again more common in the >150-μm (mean 21.4%) than the <150-μm (mean 6.12%) fraction (Table 3). Tubular fragments (stained as well as stained and dead combined) were also very numerous, representing >60% in both size fractions (Supplementary Table S2). Similar fragments are often common in abyssal samples. Bernstein et al. (1978) recorded 30,938 fragments compared to 5,079 complete foraminiferal tests in 5 unstained box-corer residues (>297 μm fraction) from the central North Pacific. Snider et al. (1984) likewise found numerous fragments in their North Pacific samples (42–1000-μm fraction). Based on the conservative assumption that all fragments of a similar type within a sediment layer and adjacent layers were derived from one individual, they calculated that 24% of monothalamids were tubular forms.
Our samples also yielded small, multichambered foraminfera that are similar to those reported in previous studies of modern abyssal benthic foraminifera. They include agglutinated species (e.g., Cribrostomoides subglobosum, Cyclammina trullisata, Cystammina galatea, Cystammina pauciloculata, Deuterammina grahami, Glomospira spp., Hormosinella ovicula, Hyperammina cylindrica, Lagenammina difflugiformis, Paratrochammina scotiaensis, Reophax scorpiurus, Spiroplectammina subcylindrica, Hormosinella distans, Veleroninoides wiesneri), and calcareous species [Epistominella exigua, Nuttallides umbonatus, Cibicidoides mundulus (as C. cf mundulus), Fissurina staphyllearia and Melonis pompiliodes] previously reported from abyssal depths in the North and equatorial Pacific by authors such as Smith (1973), Burministrova et al. (2007), and Enge et al. (2012). Many of these are well-known morphospecies that are widely distributed in modern ocean basins (Gooday and Jorissen, 2012; Holbourn et al., 2013).
Comparison With Fossil Assemblages
There are some similarities between the agglutinated foraminifera in our samples and certain assemblages of multichambered taxa of Late Cretaceous and Paleogene age recovered in Deep-Sea Drilling Project (DSDP) and Ocean Drilling Project (ODP) cores. Krasheninnikov described Upper Cretaceous faunas devoid of calcareous foraminifera in red-brown clays from the Pacific (1973) and Indian (1974) Oceans. He interpreted these as having been deposited in oceanic sediments below the CCD. They consisted mainly of small species, often with smooth, fine-grained test walls, assigned to genera such as Haplophragmoides, Labrospira, Trochammina, and Recurvoides. Similar abyssal faunas are known from the North Atlantic Plantagenet Formation, where they also include ‘rhizamminids’ and ‘fossil forms which resemble modern Komokiaceans’ (Kuhnt et al., 1989). However, our multichambered assemblages also contain some calcareous taxa (mainly rotaliids), reflecting their location close to, rather than well below, the CCD. In this respect, they have more in common with the mixed calcareous and agglutinated assemblages described by Hemleben and Troester (1984) from DSDP Hole 543A in the central Atlantic.
Species of Reophax and other hormosinids, which consistently constitute the most common multichambered foraminifera in our samples, are often not well represented in the Late Cretaceous assemblages, possibly a result of the low fossilization potential of their fairly delicate tests (Schröder, 1986). Some more robust species, however, are preserved as fossils in deep-sea sediments (e.g., Kuhnt and Moullade, 1991).
Possible Ecological Significance
Responses to Food Inputs
One rationale for including finer sieve fractions (63–150 μm) in ecological studies of bathyal and abyssal foraminifera is that small species often display opportunistic responses to seasonally pulsed food (‘phytodetritus’) inputs to the seafloor (e.g., Gooday, 1988, 2003; Gooday and Lambshead, 1989; Corliss and Silva, 1993; Silva et al., 1996; Ohga and Kitazato, 1997; Gooday and Rathburn, 1999; Gooday and Hughes, 2002; Kitazato et al., 2003; Heinz and Hemleben, 2006; Duchemin et al., 2007). This suggests that they play an active role in the processing fresh organic carbon on the ocean floor, an inference supported by in situ experimental studies using 13C-labeled algae (Moodley et al., 2002), some of which reveal different species-level responses to food inputs (Nomaki et al., 2005, 2006).
The deposition of phytodetritus on the deep-sea floor is well documented at temperate latitudes in the NE Atlantic. In the Pacific the phenomenon is reported from various sites along the North American continental margin (Beaulieu, 2002), notably at the intensively studied abyssal (4100 m depth) Station M on the Californian margin (Beaulieu and Smith, 1998). Here, as in the NE Atlantic, the presence of these deposits on the seafloor has a distinct seasonal component (Lauerman and Kaufmann, 1998). There is also evidence for seafloor phytodetritus in the abyssal equatorial Pacific. Deposition in this open ocean setting, however, is not seasonal (Beaulieu, 2002) and is linked to upwelling created by easterly trade winds (Smith and Demopoulos, 2003). Gardner et al. (1984) observed ‘dark globs of material’ that were ‘1–4 cm across and appear fluffy and organic in nature…’ in seafloor photographs taken at a site to the south of the central CCZ (4°N, 136°W; 4469 m depth). Smith et al. (1996) recovered phytodetritus in multicore samples collected along the 140°W line from 5°N to 5°S. Further east, and within the CCZ, Radziejewska (2002) observed a ‘very thin layer of a fluffy material, greenish-brown in color’ (i.e., phytodetritus) on the surfaces of some cores collected in 1997 in part of the IOM contract area (centered at 11° 04′N, 119° 40′W; 4380–4430 m depth) that had been subject 2 years earlier to an experimental disturbance designed to simulate the effects of nodule mining (see also Radziejewska, 2014). Samples of this material, which was very patchily distributed, contained chloropigments and intact diatoms. Phytodetritus was not present on the surfaces of cores recovered in 1995 or 2000. Its presence in 1997 was believed to be largely responsible for the greatly increased abundance of the nematode genera Desmoscolex and Pareudesmoscolex compared to 1995 (Radziejewska et al., 2001). Increases in metazoan megafauna in nodule-bearing parts of the IOM area was likewise attributed to the increased food supply originating from phytodetrital inputs (Radziejewska and Stoyanova, 2000).
Small lumps of phytodetritus-like material were occasionally found in our sieve residues, but these deposits were not observed on the surfaces of any megacores collected during the present study. However, our residues yielded small numbers of species, notably Alabaminella weddellensis and Epistominella exigua, which are known to exploit phytodetritual inputs in the abyssal NE Atlantic (e.g., Gooday, 1988, 1993). Epistominella exigua, in particular, contained bright green cytoplasm indicating ingestion of fresh chlorophyll-bearing particles (Figure 2A). Darker green cytoplasm is typical of Nuttallides umbonifera, the most common rotaliid in our samples (Supplementary Figure S3D). If inputs of phytodetritus do occur from time to time in the UK and OMS claim areas, as they appear to do in the nearby IOM area, then these and perhaps other species are likely to respond to this fresh food source with rapid reproduction and population growth. All but 2 of the 25 specimens (stained and dead) of A. weddellensis and E. exigua were extracted from residues <150 μm, demonstrating the importance of examining finer sediment fractions in order to recover small, opportunistic foraminiferal species that may be important players in the processing of fresh organic matter on the ocean floor.
Possible Importance in Recolonization
Alve (1999) reviewed how sediments that have been defaunated by natural or anthropogenic processes can be repopulated by benthic foraminifera. In relatively tranquil settings, initial recolonization may proceed in a series of stages, starting with quick-growing, opportunists that are succeeded by slower-growing, more specialized species. Relatively few studies of foraminiferal recolonization have been conducted in deep-water settings. Probably the best direct evidence comes from samples collected during 5 cruises (spring 1994, summer and winter 1996, summer 1998, spring 1999) in an area of the South China Sea (∼2500 m depth) blanketed by a layer of volcanic ash from the 1991 Mt Pinatubo eruption (Hess and Kuhnt, 1996; Hess et al., 2001; Kuhnt et al., 2005). Although the material involved was different, the ash fall to some extent mimicked the deposition of resuspended sediment expected to result from seabed mining. In samples sieved on a 63-μm mesh, the initial recolonizers of the 2- to 6-cm-thick ash layer appeared to be a small species of Textularia, followed by Reophax dentaliniformis and later by R. bilocularis and R. scorpiurus, the rotaliid Bolivina difformis and the miliolid Quinqueloculina seminula (Hess et al., 2001). A second wave of recolonizers included Trochammina spp., Adercotryma glomerata, and Subreophax guttifera.
Analysis of sediments deposited by turbidity currents in submarine canyons has provided insights into the recolonization by foraminifera of physically disturbed areas of seafloor. According to Duros et al. (2017), the initial recolonizers in the >150 μm fraction of a turbidite deposit at upper bathyal depths (983 m, 1454 m) in the Capbreton Canyon (Bay of Biscay) were Fursenkoina bradyi, R. dentaliniformis and Technitella melo. However, the 63–150 μm fraction from the 983-m site, as well as two shallower sites (251 m, 301 m), were dominated by juveniles of Bolivina subaenariensis, which was interpreted as representing a second stage of recolonization. The same species dominated low diversity assemblages (63–150-μm fraction) of two cores collected in the same canyon at a 600 m site that had been impacted by a turbidity current 1.5 years earlier (Hess et al., 2005; Hess and Jorissen, 2009). Here, B. subaenariensis may have been responding to organic matter associated with the turbidite, as well as participating in the recolonization process.
These studies suggest that azoic substrates, originating either from deposited material (ash) or physical disturbance of the seafloor (turbidite), are colonized by a succession of foraminiferal species. In both cases, the colonizing species were considered to have been infaunal. Some of these species are larger (e.g., Reophax spp., which are fairly common in our samples) and more likely to be retained in the >150-μm fraction, but smaller species or juveniles are also involved. Our finer fractions yielded small agglutinated species belonging to genera such as Ammobaculites, Spiroplectammina, and Textularia that are rare in the stained assemblages. It is possible that such species may be important during initial phases of recolonization following mining-related disturbances.
Conclusion
Is the considerable additional effort required to analyze foraminifera in 63–150 μm fractions of samples from areas that could be disturbed by seabed mining worthwhile? In the present study, the fine-fraction assemblages contained >60% of stained and dead test as well as 170 species that were not present in the coarser fractions. They also yielded greater absolute abundances than >150-μm fractions of several monothalamid groups, notably Lagenammina spp., Nodellum-like forms, saccamminids, and particularly spherical forms, as well as some multichambered taxa, namely rotaliids (stained only), hormosinids (dead only), textulariids (dead only), and trochamminids. An important part of the foraminiferal assemblage was therefore confined to these fine fractions. As argued elsewhere (e.g., Gooday et al., 2008; Goineau and Gooday, 2017), multichambered taxa, particularly the calcareous rotaliids, may be metabolically more active than stercomata-bearing monothalamids in deep-sea settings, and therefore more important in terms of ecosystem functioning. Although rotaliids are relatively uncommon in our samples, their greater absolute (but not relative) abundance in the <150-μm fractions provides one incentive for analyzing fine sieve residues. Another justification is the potentially important role that small multichambered opportunistic species could play in the recolonization of sediments disturbed by mining. Nevertheless, the fact remains that this work is very time-consuming and difficult to undertake routinely.
A number of studies have used foraminiferal faunal data and diversity metrics as bio-indicators of human impacts in marine environments (e.g., Jorissen et al., 2009 and references therein). This has led to the formulation of a series of recommendations, the FOMIBO initiative, aimed at standardizing methodologies applied in such studies (Schönfeld et al., 2012). One of the ‘mandatory’ recommendations is that, although samples should be sieved on a 63-μm mesh, detailed analysis should be based on the >125-μm fraction. However, they also add the ‘advisory’ recommendation that in some eutrophic settings, where small opportunistic species are abundant, it may also be necessary to analyze the finer 63–125-μm as well as the >125 μm fraction. A similar conclusion was reached by Sen Gupta et al. (1987). A sensible compromise in the case of baseline studies of foraminifera in Pacific nodule fields would therefore be to concentrate efforts on analyzing coarser residues from all samples but to examine finer fractions (63–150 μm), either quantitatively or qualitatively, in a subset of samples or sample splits. Similar approaches have been adopted by a number of authors working in the NE Atlantic deep-sea samples (e.g., Phipps et al., 2012; Duros et al., 2017). It may be particularly important to do this during monitoring exercises, i.e., where deep-sea sediments have been defaunated by seabed mining and are in the early stages of recolonization by meiofaunal organisms. To conclude, foraminifera and particularly monothalamids are a major constituent of benthic faunas in the CCZ nodule fields. Despite the methodological challenges that they pose, we would encourage other researchers to consider including them in studies of areas where seabed mining may occur in the future.
Data Availability
All datasets generated for this study are included in the manuscript and/or the Supplementary Files.
Author Contributions
AJG conceived the project, analyzed the <150 μm fractions, and prepared the figures and tables. AG analyzed the >150 μm fractions. Both authors integrated and analyzed the <150 μm and >150 μm fraction data. The manuscript was largely written by AG with contributions from AJG.
Funding
The ABYSSLINE project was funded through a commercial arrangement with UK Seabed Resources Ltd.
Conflict of Interest Statement
The authors declare that the research was conducted in the absence of any commercial or financial relationships that could be construed as a potential conflict of interest.
Acknowledgments
We are grateful to Craig Smith for his leadership of the overall ABYSSLINE project and particularly the AB01 and AB02 cruises, as well as Ivan Voltski (AB01) and Alexandra Weber (AB02) for their help with core collection and processing at sea. We would like to thank Dr. Ian Harding and Dr. Manuel Bringué for their advice regarding the identity of the unidentified organic-walled structures and two reviewers for their comments, which helped to improve the manuscript.
Supplementary Material
The Supplementary Material for this article can be found online at: https://www.frontiersin.org/articles/10.3389/fmars.2019.00114/full#supplementary-material
References
Alve, E. (1999). Colonization of new habitats by benthic foraminifera: a review. Earth Sci. Rev. 46, 167–185. doi: 10.1016/S0012-8252(99)00016-1
Amon, D. J., Ziegler, A. F., Dahlgren, T. G., Glover, A. G., Goineau, A., Gooday, A. J., et al. (2016). Insights into the abundance and diversity of abyssal megafauna in a polymetallic-nodule. Sci. Rep. 6:30492. doi: 10.1038/srep30492
Beaulieu, S. E. (2002). Accumulation and fate of phytodetritus on the sea floor. Oceanogr. Mar. Biol. Ann. Rev. 40, 171–232. doi: 10.1201/9780203180594.ch4
Beaulieu, S. E., and Smith, K. L. (1998). Phytodetritus entering the benthic boundary layer and aggregated on the sea floor in the abyssal NE Pacific: macro- and microscopic composition. Deep Sea Res. II 45, 781–815. doi: 10.1016/S0967-0645(98)00003-4
Bernstein, B. B., Hessler, R. R., Smith, R., and Jumars, P. A. (1978). Spatial dispersion of benthic Foraminifera in the abyssal central North Pacific. Limnol. Oceanogr. 23, 401–416. doi: 10.4319/lo.1978.23.3.0401
Burministrova, I. I., Khusid, T. A., Belyaeva, N. V., and Chekhovskaya, M. P. (2007). Agglutinated abyssal foraminifers of the equatorial Pacific. Oceanology 47, 824–832. doi: 10.1134/S0001437007060070
Colwell, R. K., Chao, A., Gotelli, N. J., Lin, S. Y., Mao, C. X., Chazdon, R. L., et al. (2012). Models and estimators linking individual-based and sample-based rarefaction, extrapolation and comparison of assemblages. J. Plant Ecol. 5, 3–21. doi: 10.1093/jpe/rtr044
Corliss, B., and Silva, K. A. (1993). Rapid growth of deep-sea benthic foraminifera. Geology 21, 991–994. doi: 10.1130/0091-7613(1993)021<0991:RGODSB>2.3.CO;2
Duchemin, G., Fontanier, F. C., Jorissen, F. J., Barras, C., and Griveaud, C. (2007). Living small-sized (63–150 μm) foraminifera from mid-shelf to mid-slope environments in the Bay of Biscay. J. Foramin. Res. 37, 12–32. doi: 10.2113/gsjfr.37.1.12
Duros, P., Silva Jacinto, R., Dennielous, B., Schmidt, S., Martinez Lamas, R., Gautier, E., et al. (2017). Benthic foraminiferal response to sedimentary disturbance in the Capbreton canyon (Bay of Biscay, NE Atlantic). Deep Sea Res. I 120, 61–75. doi: 10.1016/j.dsr.2016.11.012
Enge, A. J., Kucera, M., and Heinz, P. (2012). Diversity and microhabitats of living benthic foraminifera in the abyssal Northeast Pacific. Mar. Micropal. 9, 84–104. doi: 10.1016/j.marmicro.2012.08.004
Gardner, W. D., Sullivan, L. G., and Thorndike, E. M. (1984). Long-term photographic, current, and nephelometer observations of manganese nodule environments in the Pacific. Earth Planet. Sci. Lett. 70, 95–109. doi: 10.1016/0012-821X(84)90212-7
Glover, A. G., Dahlgren, T. G., Wiklund, H., Mohrbeck, I., and Smith, C. R. (2015). An end-to-end DNA taxonomy methodology for benthic biodiversity survey in the Clarion-Clipperton Zone, central Pacific abyss. J. Mar. Sci. Eng. 4:2. doi: 10.3390/jmse4010002
Gooday, A. J., and Jorissen, F. J. (2012). Benthic foraminiferal biogeography: controls on global distribution patterns in deep-water settings. Ann. Rev. Mar. Sci. 4, 237–262. doi: 10.1146/annurev-marine-120709-142737
Gooday, A. J., and Lambshead, P. J. D. (1989). Influence of seasonally deposited phytodetritus on benthic foraminiferal populations in the bathyal northeast Atlantic: the species response. Mar. Ecol. Prog. Ser. 58, 53–67.
Gooday, A. J., Nomaki, H., and Kitazato, H. (2008). “Modern deep-sea benthic foraminifera: a brief review of their biodiversity and trophic diversity,” in Biogeochemical Controls on Palaeoceanographic Environmental Proxies, Vol. 303, eds W. E. N. Austin and R. H. James, (London: Geological Society, Special Publications), 97–119.
Goineau, A., and Gooday, A. J. (2015). Radiolarian tests as microhabitats for novel benthic foraminifera: observations from the abyssal eastern equatorial Pacific (Clarion–Clipperton Fracture Zone). Deep Sea Res. I 103, 73–85. doi: 10.1016/j.dsr.2015.04.011
Goineau, A., and Gooday, A. J. (2017). Novel benthic foraminifera are abundant and diverse in an area of the abyssal equatorial Pacific licensed for polymetallic nodule exploration. Sci. Rep. 7:45288. doi: 10.1038/srep45288
Gooday, A. J. (1986). Meiofaunal foraminiferans from the bathyal Porcupine Seabight (northeast Atlantic): size structure, standing stock, taxonomic composition, species diversity and vertical distribution in the sediment. Deep Sea Res. 33, 1345–1373. doi: 10.1016/0198-0149(86)90040-3
Gooday, A. J. (1988). A response by benthic foraminifera to phytodetritus deposition in the deep sea. Nature 332, 70–73. doi: 10.1038/332070a0
Gooday, A. J. (1993). Deep-sea benthic foraminiferal species which exploit phytodetritus: characteristic features and controls on distribution. Mar. Micropaleontol. 22, 187–205. doi: 10.1016/0377-8398(93)90043-W
Gooday, A. J. (1996). Epifaunal and shallow infaunal foraminiferal communities at three abyssal NE Atlantic sites subject to differing phytodetritus regimes. Deep Sea Res. 43, 1395–1431. doi: 10.1016/S0967-0637(96)00072-6
Gooday, A. J. (2003). Benthic foraminifera (Protista) as tools in deep-water palaeoceanography: a review of environmental influences on faunal characteristics. Adv. Mar. Biol. 46, 1–90. doi: 10.1016/S0065-2881(03)46002-1
Gooday, A. J., Carstens, M., and Thiel, H. (1995). Micro- and nanoforaminifera from abyssal northeast Atlantic sediments: a preliminary report. Int. Rev. Ges. Hydrobiol. 80, 361–383. doi: 10.1002/iroh.19950800223
Gooday, A. J., and Hughes, J. A. (2002). Foraminifera associated with phytodetritus deposits at a bathyal site in the northern Rockall Trough (NE Atlantic): seasonal contrasts and a comparison of stained and dead assemblages. Mar. Micropaleontol. 46, 83–110. doi: 10.1016/S0377-8398(02)00050-6
Gooday, A. J., Hughes, J. A., and Levin, L. A. (2001). The foraminiferan macrofauna from three North Carolina (U.S.A.) slope sites with contrasting carbon flux: a comparison with the metazoan macrofauna. Deep Sea Res. I 48, 1709–1739. doi: 10.1016/S0967-0637(00)00098-4
Gooday, A. J., and Rathburn, A. E. (1999). Temporal variability in living deep-sea benthic foraminifera: a review. Earth Sci. Rev. 46, 187–212. doi: 10.1016/S0012-8252(99)00010-0
Hedley, R. H. (1960). The iron-containing shell of Gromia oviformis (Rhizopoda). Q. J. Microsc. Sci. 101, 279–293.
Heinz, P., and Hemleben, Ch (2006). Foraminiferal response to the Northeast Monsoon in the western and southern Arabian Sea. Mar. Micropaleontol. 58, 103–113. doi: 10.1016/j.marmicro.2005.10.001
Hemleben, C., and Troester, J. (1984). Campanian-Maestrichian deep-water foraminifers from Hole 543A, deep-sea drilling project. Initial Rep. DSDP 78, 509–532.
Hess, S., and Jorissen, F. J. (2009). Distribution patterns of living benthic foraminifera from Cap Breton canyon, Bay of Biscay: faunal response to sediment instability. Deep Sea Res. I 56, 1555–1578. doi: 10.1016/j.dsr.2009.04.003
Hess, S., Jorissen, F. J., Venet, V., and Abu-Zied, R. (2005). Benthic foraminiferal recovery after recent turbidite deposition in Cap Breton canyon, Bay of Biscay. J. Foramin. Res. 35, 114–129. doi: 10.2113/35.2.114
Hess, S., and Kuhnt, W. (1996). Deep-sea benthic foraminiferal recolonization of the 1991 Mt. Pinatubo ash layer in the South China Sea. Mar. Micropaleontol. 28, 171–197. doi: 10.1016/0377-8398(95)00080-1
Hess, S., Kuhnt, W., Hill, S., Kaminski, M. A., Holbourn, A., and de Leon, M. (2001). Monitoring the recolonization of the Mt. Pinatubo 1991 ash layer by benthic foraminifera. Mar. Micropaleontol. 43, 119–142. doi: 10.1016/S0377-8398(01)00025-1
Holbourn, A., Henderson, A., and McLeod, N. (2013). Atlas of Benthic Foraminifera. Hoboken, NJ: Willey-Blackwell. doi: 10.1002/9781118452493
Jorissen, F. J., Bicchi, E., Duchemin, G., Durrieu, J., Galgani, F., Cazes, L., et al. (2009). Impact of oil-based drill mud disposal on benthic foraminiferal assemblages on the continental margin off Angola. Deep Sea Res. II 56, 2270–2291. doi: 10.1016/j.dsr2.2009.04.009
Kitazato, H., Nakatsuka, T., Shimanaga, M., Kanda, J., Soh, W., Kato, Y., et al. (2003). Long-term monitoring of the sedimentary processes in the central part of Sagami Bay, Japan: rationale, logistics and overview of results. Prog. Oceanogr. 57, 3–16. doi: 10.1016/S0079-6611(03)00047-8
Kuhnt, W., Hess, S., Holbourn, A., Paulsen, H., and Salomon, B. (2005). The impact of the 1991 Mt. Pinatubo eruption on deep-sea foraminiferal communities: a model for the Cretaceous–Tertiary (K/T) boundary? Palaeogeogr. Palaeocl. Palaeoecol. 224, 83–107. doi: 10.1016/j.palaeo.2005.03.042
Kuhnt, W., Kaminski, M. A., and Moullade, M. (1989). Late Cretaceous deep-water agglutinated foraminiferal assemblages from the North Atlantic and its marginal seas. Geol. Rundsch. 78, 1121–1140. doi: 10.1007/BF01829336
Kuhnt, W., and Moullade, M. (1991). Quantitative analysis of Upper Cretaceous abyssal agglutinated foraminiferal distribution in the North Atlantic – paleoceanographic implications. Rev. Micropaleontol. 34,313–349.
Lauerman, L. M. L., and Kaufmann, R. S. (1998). Deep-sea epibenthic echinoderms and a temporally varying food supply: results from a one year time series in the N.E. Pacific. Deep Sea Res. II 45, 817–842. doi: 10.1016/S0967-0645(98)00004-6
Leduc, D., Probert, P. K., and Nodder, S. D. (2010). Influence of mesh size and core penetration on estimates of deep-sea nematode abundance, biomass and diversity. Deep Sea Res. I 57, 1354–1362. doi: 10.1016/j.dsr.2010.06.005
Lutze, G. F., and Coulbourne, W. T. (1984). Recent benthic foraminifera from the continental margin of northwest Africa: community structure and distribution. Mar. Micropaleontol. 8, 361–401. doi: 10.1016/0377-8398(84)90002-1
Moodley, L., Middelburg, J. J., Boschker, H. T. S., Duineveld, G. C. A., Pel, R., Herman, P. M. J., et al. (2002). Bacteria and Foraminifera: key players in a short-term deep-sea benthic response to phytodetritus. Mar. Ecol. Prog. Ser. 236, 23–29. doi: 10.3354/meps236023
Murray, J. W. (2014). Some trends in sampling modern living (stained) benthic foraminifera in fjord, shelf and deep sea: Atlantic Ocean and adjacent seas. J. Micropalaeontol. 34, 101–104. doi: 10.1144/jmpaleo2014-004
Nomaki, H., Heinz, P., Nakatsuka, T., Shimanaga, M., and Kitazato, H. (2005). Species-specific ingestion of organic carbon by deep-sea benthic foraminifera and meiobenthos: in situ tracer experiments. Limnol. Oceanogr. 50, 134–146. doi: 10.4319/lo.2005.50.1.0134
Nomaki, H., Heinz, P., Nakatsuka, T., Shimanaga, M., Ohkouchi, N., Ogawa, N. O., et al. (2006). Different ingestion patterns of 13C-labeled bacteria and algae by deep-sea benthic foraminifera. Mar. Ecol. Prog. Ser. 310, 95–108. doi: 10.3354/meps310095
Nozawa, F., Kitazato, H., Tsuchiya, M., and Gooday, A. J. (2006). ‘Live’ benthic foraminifera at an abyssal site in the equatorial Pacific nodule province: abundance, diversity and taxonomic composition. Deep Sea Res. I 51, 1406–1422. doi: 10.1016/j.dsr.2006.06.001
Ohga, T., and Kitazato, H. (1997). Seasonal changes in bathyal foraminiferal populations in response to the flux of organic matter (Sagami Bay, Japan). Terra Nova 9, 33–37. doi: 10.1046/j.1365-3121.1997.d01-6.x
Ohkawara, N., Kitazato, H., Uematsu, K., and Gooday, A. J. (2009). A minute new species of Saccammina (monothalamous Foraminifera; Protista) from the abyssal Pacific. J. Micropalaeontol. 28, 143–151. doi: 10.1144/jm.28.2.143
Pape, E., Bezerra, T. N., Hauquier, F., and Vanreusel, A. (2017). Limited spatial and temporal variability in meiofauna and nematode communities at distant but environmentally similar sites in an area of interest for deep-sea mining. Front. Mar. Sci. 4:205. doi: 10.3389/fmars.2017.00205
Pawlowski, J. (1991). Distribution and taxonomy of some benthic tiny foraminifers from the Bermuda Rise. Micropaleontology 37, 163–172. doi: 10.2307/1485556
Pawlowski, J., and Lapierre, L. (1988). Foraminifères benthiques actuels de l’Atlantique Nord-Est. Rev. Paléontol. 2, 873–878.
Phipps, M., Jorissen, F., Pusceddu, A., Bianchelli, S., and De Stigter, H. (2012). Live benthic foraminiferal faunas along a bathymetrical transect (282–4987 m) on the Portuguese margin (NE Atlantic). J. Foramin. Res. 42, 66–81. doi: 10.2113/gsjfr.42.1.66
Radziejewska, T. (2002). Responses of deep-sea meiobenthic communities to sediment disturbance simulating effects of polymetallic nodule mining. Internat. Rev. Ges. Hydrobiol. 87, 457–477. doi: 10.1002/1522-2632(200207)87:4<457::AID-IROH457>3.0.CO;2-3
Radziejewska, T. (2014). Meiobenthos in the Sub-Equatorial Pacific Abyss: a Proxy in Anthropogenic Impact Evaluation. Heidelberg: Springer. doi: 10.1007/978-3-642-41458-9
Radziejewska, T., Drzycimski, I., Galtsova, V. V., Kulangieva, L. V., and Stoyanova, V. (2001). “Changes in genus-level diversity of meiobenthic free-living nematodes (Nematoda) and harpacticoids (Copepoda Harpacticoida) at an abyssal site following experimental sediment disturbance,” in Proceedings 4th Ocean Mining Symposium, Szczecin, Poland, eds J. S. Chung and V. Stoyanova (Cupertino, CA: ISOPE), 38–43.
Radziejewska, T., Gooday, A. J., Koltan, M., and Szyrweil, E. (2006). Deep-sea non-calcareous Foraminifera: some examples from the Pacific’s abyssal nodule field. Meiofauna Mar. 15, 3–10.
Radziejewska, T., and Stoyanova, V. (2000). Abyssal epibenthic megafauna of the Clarion-Clipperton area (NE Pacific): changes in time and space versus anthropogenic environmental disturbance. Oceanol. Stud. 29,83–101.
Rothe, N., Gooday, A. J., Cedhagen, T., and Hughes, J. A. (2010). Biodiversity and distribution of the genus Gromia (Protista, Rhizaria) in the deep Weddell Sea (Southern Ocean). Polar Biol. 43, 69–81. doi: 10.1007/s00300-010-0859-z
Schewe, I. (2001). Small-sized benthic organisms of the alpha ridge, Central Arctic Ocean. Internat. Rev. Hydrobiol. 86, 317–335. doi: 10.1002/1522-2632(200106)86:3<317::AID-IROH317>3.0.CO;2-V
Schönfeld, J., Alve, E., Geslin, E., Jorissen, F. J., Korsun, S., Spezzaferri, S., et al. (2012). The FOBIMO (FOraminiferal BIo-MOnitoring) initiative—Towards a standardised protocol for soft-bottom benthic foraminiferal monitoring studies. Mar. Micropaleontol. 94–95, 1–13. doi: 10.1016/j.marmicro.2012.06.001
Schröder, C. J. (1986). Deep-water arenaceous foraminifera in the northwest Atlantic Ocean. Can. Tech. Rep. Hydrogr. Ocean Sci. 71, 1–191.
Schröder, C. J., Scott, D. B., and Medioli, F. S. (1987). Can smaller benthic foraminifera be ignored in paleoenvironmental analyses? J. Foramin. Res. 17, 101–105. doi: 10.2113/gsjfr.17.2.101
Sen Gupta, B. K., Shin, I. C., and Wendler, S. T. (1987). Relevance of specimen size in distribution studies of deep-sea benthic foraminifera. Palaios 2, 332–338. doi: 10.2307/3514758
Shirayama, Y., and Horikoshi, M. (1989). Comparison of the benthic size structure between sublittoral, upper slope and deep-sea areas of the Western Pacific. Int. Rev. Ges. Hydrobiol. 74, 1–13. doi: 10.1002/iroh.19890740102
Silva, K. A., Corliss, B. C., Rathburn, A. E., and Thunnell, R. C. (1996). Seasonality of living benthic foraminifera from the San Pedro Basin, California Borderland. J. Foramin. Res. 26, 71–93. doi: 10.2113/gsjfr.26.1.71
Smith, C. R., and Demopoulos, A. W. J. (2003). “The deep Pacific Ocean floor,” in Ecosystems of the Deep Oceans Ecosystems of the World 28, ed. P. Tyler (Amsterdam: Elsevier), 179–218.
Smith, C. R., Hoover, D. J., Doan, S. E., Pope, R. H., DeMaster, D. J., Dobbs, F. C., et al. (1996). Phytodetritus at the abyssal seafloor across 10° of latitude in the central equatorial Pacific. Deep Sea Res. II 43, 1309–1338. doi: 10.1016/0967-0645(96)00015-X
Smith, P. B. (1973). Foraminifera of the North Pacific Ocean, United States geological survey professional publication. 766, 1–17. doi: 10.3133/pp766
Snider, L. J., Burnett, B. R., and Hessler, R. R. (1984). The composition and distribution of meiofauna and nanobiota in a central North Pacific deep-sea area. Deep Sea Res. 31, 1225–1249. doi: 10.1016/0198-0149(84)90059-1
Szarek, R., Nomaki, H., and Kitazato, H. (2007). Living deep-sea benthic foraminifera from the warm and oxygen-depleted environment of the Sulu Sea. Deep Sea Res I 54, 145–176. doi: 10.1016/j.dsr2.2006.02.017
Thiel, H. (1975). The size structure of the deep-sea benthos. Internat. Rev. Ges. Hydrobiol. 60, 575–606. doi: 10.1111/gcb.12480
Keywords: polymetallic nodules, Clarion-Clipperton Zone, baseline survey, abyssal benthic foraminifera, sieve mesh size, biodiversity, recolonization
Citation: Gooday AJ and Goineau A (2019) The Contribution of Fine Sieve Fractions (63–150 μm) to Foraminiferal Abundance and Diversity in an Area of the Eastern Pacific Ocean Licensed for Polymetallic Nodule Exploration. Front. Mar. Sci. 6:114. doi: 10.3389/fmars.2019.00114
Received: 05 October 2018; Accepted: 25 February 2019;
Published: 02 April 2019.
Edited by:
Randi D. Rotjan, Boston University, United StatesCopyright © 2019 Gooday and Goineau. This is an open-access article distributed under the terms of the Creative Commons Attribution License (CC BY). The use, distribution or reproduction in other forums is permitted, provided the original author(s) and the copyright owner(s) are credited and that the original publication in this journal is cited, in accordance with accepted academic practice. No use, distribution or reproduction is permitted which does not comply with these terms.
*Correspondence: Andrew J. Gooday, YW5nQG5vYy5hYy51aw==