- 1ARC Centre of Excellence for Environmental Decisions, Centre for Biodiversity and Conservation Science, School of Biological Sciences, The University of Queensland, Brisbane, QLD, Australia
- 2School of Earth and Environmental Sciences, The University of Queensland, Brisbane, QLD, Australia
- 3Facultad de Ciencias Marinas, Universidad Autónoma de Baja California, Ensenada, Mexico
- 4Instituto de Investigaciones Oceanológicas, Universidad Autónoma de Baja California, Ensenada, Mexico
- 5Global-Change Ecology Research Group, School of Science and Engineering, University of the Sunshine Coast, Maroochydore, QLD, Australia
- 6Department of Zoology, Centre for African Conservation Ecology, Nelson Mandela University, Port Elizabeth, South Africa
Climate change is increasing the frequency and severity of marine heatwaves. A recent extreme warming event (2014–2016) of unprecedented magnitude and duration in the California Current System allowed us to evaluate the response of the kelp forest community near its southern (warm) distribution limit. We obtained sea surface temperatures for the northern Pacific of Baja California, Mexico, and collected kelp forest community data at three islands, before and after the warming event. The warming was the most intense and persistent event observed to date, with low-pass anomalies 1°C warmer than the previous extremes during the 1982–1984 and 1997–1998 El Niños. The period between 2014 and 2017 accounted for ∼50% of marine heatwaves days in the past 37 years, with the highest maximum temperature intensities peaking at 5.9°C above average temperatures for the period. We found significant declines in the number of Macrocystis pyrifera individuals, except at the northernmost island, and corresponding declines in the number of fronds per kelp individual. We also found significant changes in the community structure associated with the kelp beds: half of the fish and invertebrate species disappeared after the marine heatwaves, species with warmer affinities appeared or increased their abundance, and introduced algae, previously absent, appeared at all islands. Changes in subcanopy and understory algal assemblages were also evident; however, the response varied among islands. These results suggest that the effect of global warming can be more apparent in sensitive species, such as sessile invertebrates, and that warming-related impacts have the potential to facilitate the establishment of tropical and invasive species.
Introduction
Climate change is predicted to increase the frequency and severity of extreme climatic events (Solomon et al., 2007; Perkins et al., 2012; Sydeman et al., 2013; Frölicher et al., 2018) such as marine heatwaves (MHWs) (Hobday et al., 2016), which are impacting coastal marine ecosystems worldwide (Garrabou et al., 2009; Marba and Duarte, 2010; Smale and Wernberg, 2013; Wernberg et al., 2013, 2016; Cavole et al., 2016; Hughes T. P. et al., 2017; Oliver et al., 2017; Smale et al., 2019), as well as the human populations that depend on them (McCarthy et al., 2001; Harley et al., 2006). In the past decades, MHWs have already increased in frequency and severity, with a global average of 50% more MHW days registered per year (Frölicher et al., 2018; Oliver et al., 2018). Temperate foundation (habitat-forming) species, are reported to be sensitive to MHWs (Wernberg et al., 2019), especially to warmer water temperatures and to decreases in nutrient availability due to thermal stratification or to a weakening of coastal upwelling (Carr and Reed, 2015; Schiel and Foster, 2015). They are particularly susceptible to MHWs when located close to their equatorward (warm) range edges (Smale et al., 2019). Changes in the abundance and distribution of these foundation species could have consequences for the structure and function of the entire ecosystem (Harley et al., 2012; Schiel and Foster, 2015; Smale et al., 2019).
The temperate ecosystems of the California Current System (CCS) were recently exposed to a sustained and intense warming event. It began in November 2013 in the Gulf of Alaska (Gentemann et al., 2017), with positive (warm) sea surface temperature anomalies (SSTAs) in the upper ocean layer (∼100 m). By May 2014, it spread south to the coasts of Baja California, lasting until April 2015 (Di Lorenzo and Mantua, 2016). Nicknamed the “Blob,” temperature anomalies exceeded 3°C above previous climate records (Bond et al., 2015; Di Lorenzo and Mantua, 2016). Severe El Niño Southern Oscillation (Jacox et al., 2016) (nicknamed Godzilla) conditions (SSTAs of ∼2.3°C, as measured by el Niño 3.4 index) followed this event, extending the warming in Baja California until the end of 2016 (Di Lorenzo and Mantua, 2016; Jacox et al., 2016). Reports of biological changes across the entire food web of the CCS (Cavole et al., 2016; Sanford et al., 2019) followed these extreme conditions. However, limited knowledge exists regarding the corresponding responses of temperate coastal ecosystems.
Kelp forest ecosystems in the northeastern Pacific Ocean are distributed along the coasts of the CCS, from Alaska, United States, to their southern distribution limit in Baja California, Mexico (Graham et al., 2007; Carr and Reed, 2015; Schiel and Foster, 2015). The giant kelp, Macrocystis pyrifera, one of the foundation species in kelp forests of this region, creates a three-dimensional biogenic marine habitat that sustains diverse biological assemblages, and provides valuable ecosystem services (Foster and Schiel, 1985; Costanza et al., 1997; Carr and Reed, 2015). The occurrence of periodic warm oceanographic events, such as El Niño, which tend to generate warm and nutrient-poor environmental conditions, as well as episodic strong storms that dislodge M. pyrifera individuals (Dayton, 1985; Foster and Schiel, 1985; Byrnes et al., 2011), can cause the decrease or loss of M. pyrifera forests (Dayton and Tegner, 1984; Foster and Schiel, 1985; Zimmerman and Robertson, 1985; Edwards, 2004), and alter the structure of entire communities (Dayton and Tegner, 1984; Dayton et al., 1984, 1992; Tegner and Dayton, 1987; Schiel and Foster, 2015).
Kelp forest ecosystems located near temperate-subtropical transition zones, where conditions are more variable and extreme, are more vulnerable to warming events than those found at higher latitudes (Steneck et al., 2002; Edwards, 2004; Edwards and Estes, 2006; Johnson et al., 2011; Wernberg et al., 2013, 2016; Vergés et al., 2014). During El Niño 1997–1998, in the CCS, almost all of Baja California’s M. pyrifera forests disappeared, and while heavy losses were reported for central California, only minor impacts were observed for northern California (Edwards, 2004; Edwards and Estes, 2006). M. pyrifera forests are resilient to disturbance and quickly recover after catastrophic events due to their rapid responses to changes in nutrient supply, short lifespans, rapid growth rates, constant spore production, and propensity to generate a bank of microscopic stages that endure stressful conditions (Dayton et al., 1992; Reed et al., 1996; Ladah et al., 1999; Filbee-Dexter and Scheibling, 2014). Also, during El Niño 1997–1998, recovery rates for M. pyrifera were slower in Baja California than in central and northern California (Edwards, 2004; Edwards and Hernandez-Carmona, 2005; Edwards and Estes, 2006). The secular trend toward increasing temperatures might be challenging the capacity of M. pyrifera forests to recover near their southern (warm) limit in the CCS. This notion is supported by observations that their historical southern distribution edge has already suffered considerable poleward range contraction (Hernandez-Carmona et al., 2000), and that they might continue to contract poleward due to climate change.
Long-term, large-scale monitoring programs have proven to be extremely valuable to understand the response of complex marine ecosystems to extreme environmental changes (Hughes B. B. et al., 2017). But the high cost of collecting ecological data is shifting research efforts toward studies of sentinel species for climate change (early indicators of climate change), such as M. pyrifera (Krumhansl et al., 2016; Reed et al., 2016). It is particularly valuable to monitor such species when located in sentinel areas for climate change, that is regions such as Baja California, where ocean surface temperatures have changed rapidly, and are projected to continue to do so (Hobday and Pecl, 2014). Despite the sensitivity of Baja California’s kelp forest to warming events (Hernández Carmona, 1988; Ladah et al., 1999; Hernandez-Carmona et al., 2001; Edwards, 2004; Edwards and Hernandez-Carmona, 2005; Edwards and Estes, 2006; Torres-Moye et al., 2013; Beas-Luna and Ladah, 2014; Woodson et al., 2018), they have received far less attention than those further north (Ramírez-Valdez et al., 2017). Moreover, given the relevance of kelp forests near the southern end of their distributional range, understanding changes associated with an extreme environmental event might provide insights into how climate change will alter kelp forest ecosystems in the CCS.
Islands are vulnerable environments, where isolation can preclude recovery after an extreme event due to restricted adult migration, and larval input (Johnson and Black, 2006; Bell, 2008), making them good candidate sites in which to study ecosystem responses to MHWs. In December 2016, the Mexican government declared all Islands in the Pacific Coast of Baja California as a biosphere reserve. This action made them priority areas for conservation in the region, raising the need to understand the consequences of extreme events, which can endanger ongoing protection efforts in these vulnerable marine ecosystems.
The goal of this study is to provide a comprehensive report on the ecological impacts of the 2014–2016 warming event in the southern kelp forest communities of the CCS. We selected three islands, separated from each other at roughly 125 km intervals, to represent a latitudinal gradient off the coast of Baja California, and documented changes in community attributes from before to after the MHWs. We hypothesized that M. pyrifera kelp forests would suffer greatest impacts from the MHWs at the southernmost locations, since these are closer to the warm edge of their distribution limit, and that these changes would be reflected in the assemblage structure of invertebrates, fish, and algae.
Materials and Methods
Study Sites
Our study sites encompass three inhabited islands that are part of the Baja California Pacific Islands biosphere reserve in Mexico: Isla Todos Santos (ITS), Isla San Martin (ISM), and Isla San Jeronimo (ISJ) (Figure 1). Located in the CCS, these islands stretch along a 250 km latitudinal gradient (29.8°–31.8°N) near the southern biogeographic distribution limit of boreal populations of M. pyrifera. They are internationally recognized for their high diversity, the abundance of their flora and fauna, the relative natural integrity of their ecosystems, and their high levels of endemism (Samaniego-Herrera et al., 2007). The marine areas adjacent to these islands, support various fisheries, most of them targeting species dependent on M. pyrifera as a source of food and habitat (Montaño-Moctezuma et al., 2014).
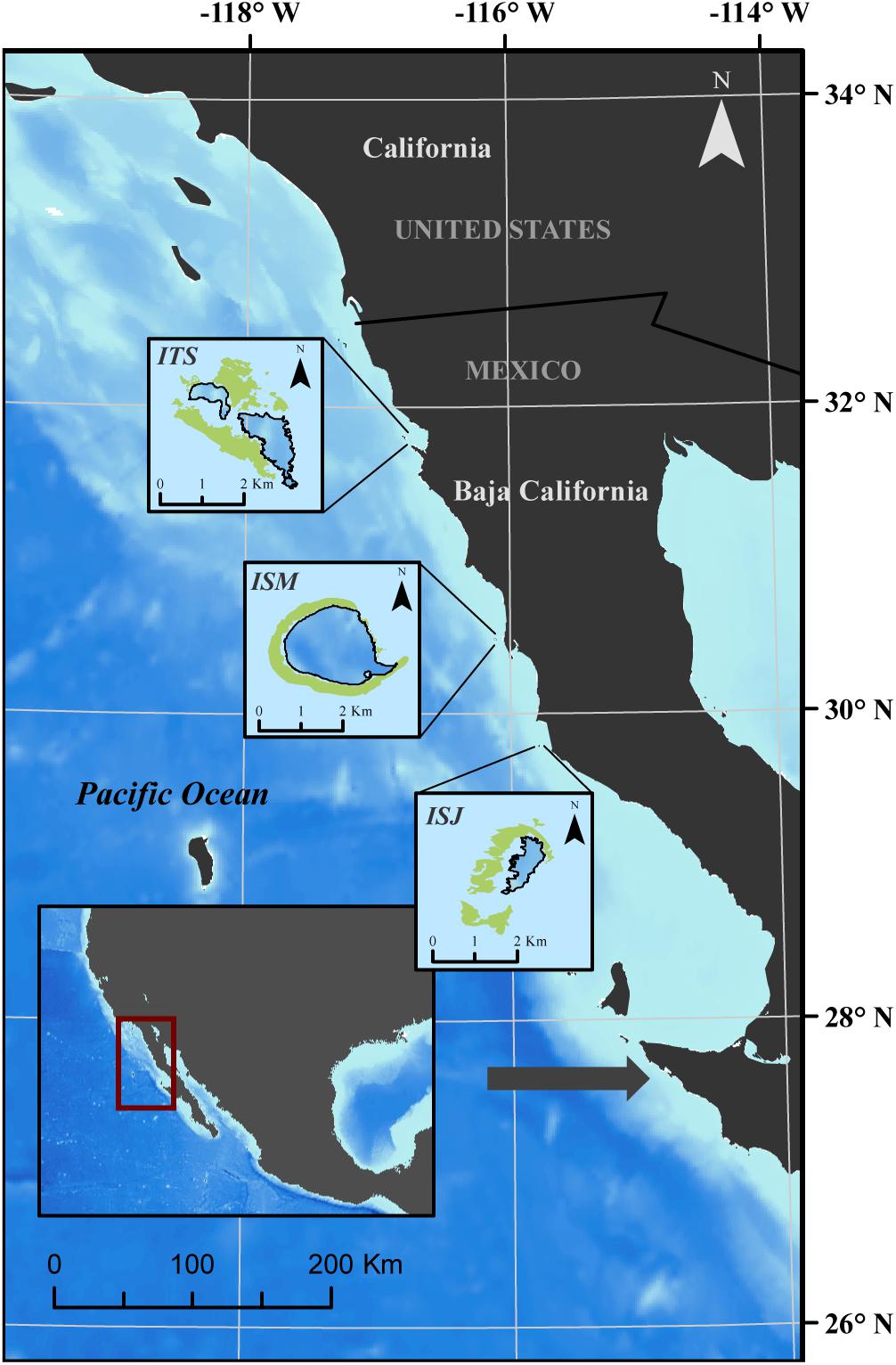
Figure 1. Location of Isla Todos Santos (ITS), Isla San Martin (ISM), and Isla San Jeronimo (ISJ) in Baja California, Mexico. Green polygons in the insets represent M. pyrifera beds at the three islands. The dark arrow in the main figure shows the southern distribution limit of M. pyrifera in the CCS.
Sea Surface Temperature
We obtained satellite-derived data of sea surface temperature by using the GHRSST Level 4 AVHRR Optimally Interpolated Global Blended Sea Surface Temperature Analysis (GDS version 2), from the National Centers for Environmental Information (2016), available at the physical oceanography distributed active archive center (PODAAC) of the NASA Jet Propulsion Laboratory (Last access: 2019-01-19). Daily sea surface temperature data are available at a 0.25° spatial resolution for 37 years (from September 1981 to present) (Reynolds et al., 2007; Banzon et al., 2016). To have a historical perspective of the strength of the 2014–2016 warming event, we calculated sea surface temperature anomalies for each 0.25° grid cell by subtracting the 1982–2018 local mean. We used a Fourier low-pass filter to show the contribution of interannual (basin-scale) processes (e.g., El Niño and the Blob) by removing signals with periods shorter than 18 months (cut off frequency), retaining inter-annual variability.
MHWs
We identified MHWs, defined by Hobday et al. (2016) as discrete prolonged anomalously warm-water events in a location, using the same daily sea surface temperature database for the 1982–2018 period. As with other studies of MHWs, we focused on upper percentiles of SSTAs, rather than on absolute SSTs. This approach accounts for the likelihood that marine populations are adapted to local temperature conditions, with anomalies indicating deviation from normal conditions that might elicit location-specific responses to thermal stress. We used the Matlab toolbox (Zhao and Marin, 2019) to identify periods of time where temperatures were above the 90th percentile threshold relative to the climatology (seasonally varying mean), and with a duration of at least 5 days. We identified the start and end dates and calculated the duration and intensity for each MHW event.
Biological Monitoring
We collected ecological data from the three islands. At each island, two sampling sites were selected (i.e., northern and southern sites). Within each of these sites, we used underwater survey techniques (depth ranges of 10–15 m) to characterize the assemblages of invertebrates, fish, and algae at three transects. Data were collected by experienced, trained divers to ensure constancy in survey methods, and species lists compiled over the years. We estimated abundance of M. pyrifera and fish in situ by census transects and assessed abundance of invertebrates and algae by analyzing video transects. To corroborate the results of the in situ estimate of M. pyrifera abundance and better understand the effects of the MHWs, we estimated kelp biomass using remote-sensed Landsat imagery. We conducted the field surveys in late autumn 2013 and 2016, but due to bad weather in 2016, we had to extend the field work to early winter 2017 (henceforth “2016”).
Census Transects
For each island and year, we conducted three fish transects of 30 m × 2 m (60 m2) at each sampling site (six transects in total). Divers recorded identity and abundance of fish species along each transect. To assess the importance of the MHWs for fish species with different thermal affinities, we classified fish species from north to south, based on the centroid of their latitudinal distribution (FishBase). We evaluated the density of M. pyrifera by counting all the individuals (sporophytes), taller than 1 m, and the number of fronds (also known as stipes) per individual (measured above the holdfast), reporting the number of individuals per unit area. In 2013, we counted the number of M. pyrifera fronds along the transects in a sub-sample of randomly picked 9 individuals: three at the beginning of the transect (0–10 m), three at 10–20 m, and three at 20–30 m. In 2016, we counted the fronds for all M. pyrifera individuals found along the transect.
Video Transects
For each island and year, we conducted three video transects at each sampling site (six transects in total). In 2013, the transects were 10 m × 1.5 m (15 m2), while in 2016 they were 20 m× 2 m (40 m2). We analyzed each video transect to estimate the number of individuals of all invertebrate species. We grouped algae into subcanopy, introduced and understory species, and reported as numbers of individuals per square meter for the subcanopy and introduced species (to account for different sampled areas). We grouped understory algae into coralline crustose, coralline articulated, red crustose, red branch, and brown algae and estimated per cent cover of each group by using the ImageJ software (Schneider et al., 2012). For this group, we manually captured images from the video transects in VLC Media Player software and improved if necessary with Microsoft Office Picture Manager 2010. Then we estimated the total area of each picture based on an object of known size. Finally, we manually selected the area covered by each algal group, and the software calculated the total area for each group as a percentage cover.
Satellite Images
We used multispectral Landsat imagery to estimate M. pyrifera canopy biomass at 30-m resolution for a 10-year period (from January 1, 2009 to December 31, 2018) following Cavanaugh et al. (2011). We analyzed each atmospherically corrected Landsat image using multiple endmember spectral unmixing (MESMA) (Roberts et al., 1998). This analysis enables estimates of kelp canopy area and biomass using observed relationships between MESMA-derived canopy fractions and diver-measured canopy biomass (Cavanaugh et al., 2011). These relationships were developed using data from the Santa Barbara Channel. There are 2 Landsat “scenes” that cover our study sites, and each scene has about 60 images between 1984-present, allowing us to determine seasonal canopy biomass for each 30 m × 30 m pixel.
Statistical Analysis
For all analyses comparing data collected before and after the MHWs, we used the parallel approach of modeling the response as a function of the crossed fixed factors Island (three levels: ITS, ISM, and ISJ) and Time (two levels: Before and After). In each instance, we assumed the six replicates taken at each time at each island (three replicates taken from two sites at each island) to comprise an independent, representative sample for that island. For univariate counts, we fit generalized linear models (GLMs) with Poisson errors, and simplified these based on standard log-likelihood ratio tests. For these models, we assessed approximate significance of pair-wise differences based on 95% confidence intervals. For multivariate responses, we used permutational multivariate analysis of variance (PERMANOVA) (Anderson, 2001) in the R package vegan (Oksanen et al., 2013) on the basis of Bray-Curtis distance matrices derived from square-root transformed data, and used non-Metric Multidimensional Scaling (nMDS) to visualize multivariate patterns in community structure. Where post hoc tests were required, we employed pairwise PERMANOVAs, with false-discovery-rate adjustment for multiple tests (Benjamini and Hochberg, 1995), using functions in the R package RVAideMemoire (Hervé, 2018). We used Dufrene-Legendre Indicator Species Analysis (Dufrêne and Legendre, 1997) to distinguish which species were contributing to significant clusters identified by these post hoc tests, using functions from the R Package labdsv (Roberts, 2018).
The time series of Landsat estimates of kelp biomass were the only long-term data available to explore whether observations from the period after the MHWs were beyond the bounds of natural variation for the period before the MHWs. We could not use standard linear modeling approaches in this instance, because observations would be expected to contain significant temporal autocorrelation, violating assumptions of independence. We therefore used automatic algorithms from the R package tsoutliers (López-de-Lacalle, 2019) to detect outliers from ARIMA (0,1,1) time series fitted to the log10(x + 1)-transformed data from each island. This approach has the advantage over a random walk model of smoothing the fit using an exponentially weighted moving average of preceding values, rather than placing excessive emphasis on the most recent observation. Outliers detected in this way can be assumed to arise from a process not inherent to that which generated the other observations in the time series (i.e., they are outside the natural variation associated with the time series).
We programmed all analyses in R version 3.5.2 (R Core Team, 2013), setting α = 0.05.
Results
Sea Surface Temperature Changes
Along the Baja California coast, 2013 was in a slightly cold stage, with negative (cold) anomalies and no evidence of the “Blob” (Figure 2A), until 2014, when the warming event became evident (Figure 2B). By 2015, the additional El Niño effect boosted the warm anomalies and extended their distribution offshore and along the coastal waters of Baja California (Figure 2C). Toward the end of 2016, this effect had weakened (Figure 2D), but warm water anomalies remained throughout 2017 (Figure 2E and Supplementary Figure S1). After removing the annual cycle, the average low-pass anomalies were more than 1°C warmer than the extreme El Niño 1982–1984 and 1997–1998 events (Supplementary Figure S1). The 2014–2016 warm-event anomaly registered on the three islands was the largest in extent and most prolonged in duration observed to date, with SSTAs slightly lower at the northernmost site (ITS) than the southernmost sites (ISM and ISJ), with anomalous temperatures peaking on summer 2015 at 2.15, 2.32, and 2.39°C above average, respectively (Supplementary Figure S1).
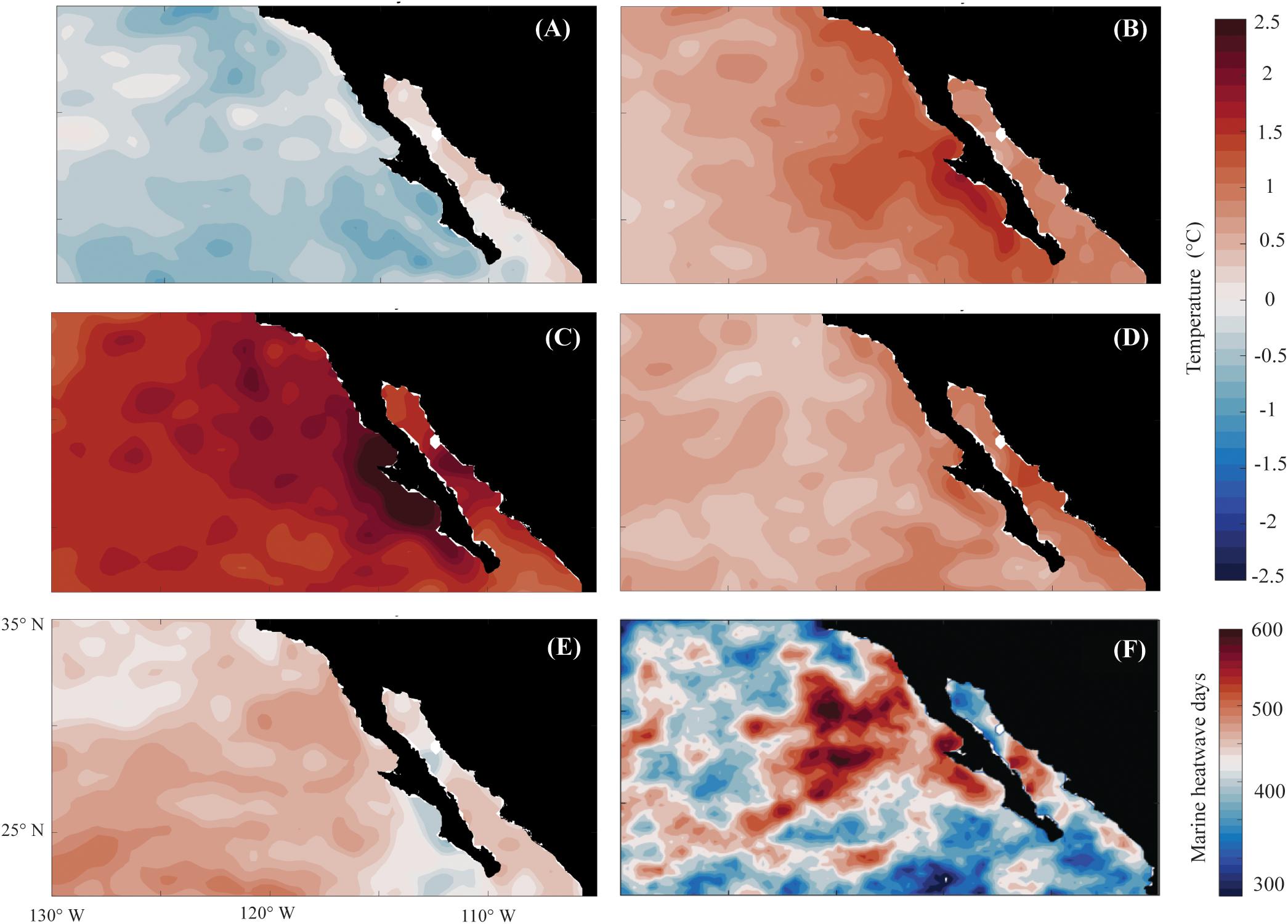
Figure 2. Mean low-pass sea surface temperature anomalies (°C) for (A) 2013, (B) 2014, (C) 2015, (D) 2016, (E) 2017, at Baja California, Mexico. (F) Total number of marine heatwave days [sensu Hobday et al. (2016)] detected for 2014–2017 period in Baja California.
Marine Heatwaves
Around 50% of the identified MHW days between 1982–2017 registered at the three study sites occurred during 2014–2017 (Figure 2F and Supplementary Figure S3A). The period 2014–2015 accounted for 545 MHW days at ITS (58%), 466 at ISM (51%), and 476 at ISJ (53%) (Figures 3A,C). The year with the highest number of MHW days registered was in 2015 for ITS (261 days), ISM (242 days) and ISJ (256 days) (Figures 3A,C). For ITS, the longest continuous MHW comprised 152 days, lasting from November 2014 until late April 2015, while ISM and ISJ registered events lasting 123 and 124 consecutive MHW days, respectively, between late August and late December 2015 (Supplementary Tables S1–S3). In autumn 2015, the highest maximum temperature during a MHW were identified for the three islands, peaking at 5.6, 5.9, and 5.7°C, respectively (Figures 3A,B and Supplementary Tables S1–S3). These values are higher than the maximum registered during previous strong 1982–1984 (4.1, 3.3, and 3.3°C) and 1997–1998 El Niños (3, 3.4, and 3.7°C) (Supplementary Figures S2, S3B).
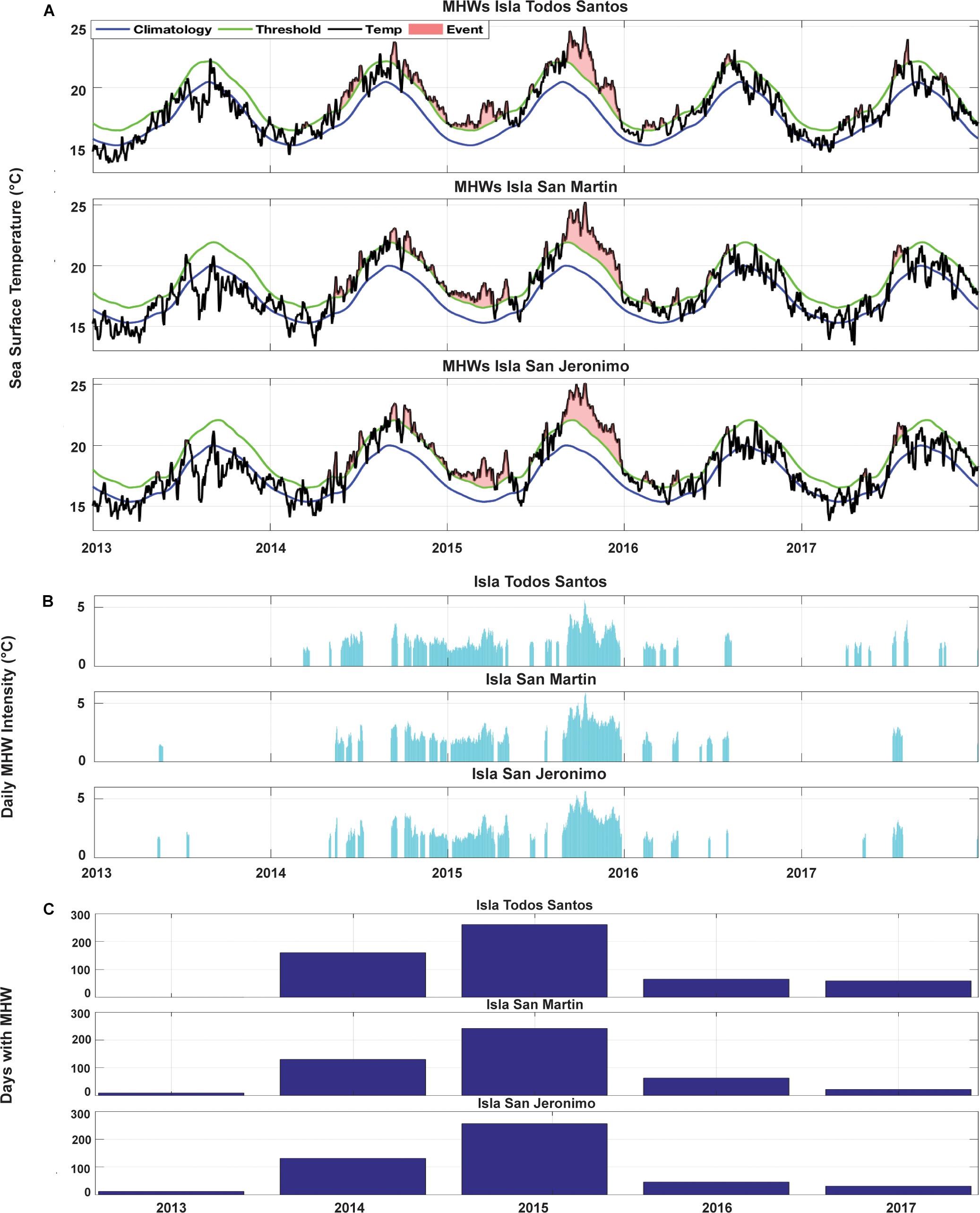
Figure 3. (A) Blue lines indicate the sea surface temperature (SST) climatology, green lines represent the 90th percentile that defines a marine heatwave (MHW), black lines are the SST time series, and the orange shaded region indicates all MHWs identified. (B) The daily MHW intensity, (C) the number of MHW days registered during 2013–2017 for Isla Todos Santos, Isla San Martin, and Isla San Jeronimo.
Changes in Macrocystis pyrifera
We detected significant interactions between Island and Time in our Poisson GLMs models of the numbers of M. pyrifera individuals per transect as well as the numbers of fronds per individual before and after the MHWs (Table 1). For the northernmost island, ITS, the number of individuals increased significantly after the MHWs. In contrast, we found significant decreases at the southern islands, ISM and ISJ, with only a few individuals per transect observed after the MHWs (Figure 4A). For all the islands we found a strong, consistent and significant decline in the numbers of fronds per M. pyrifera individual (Figure 4B).
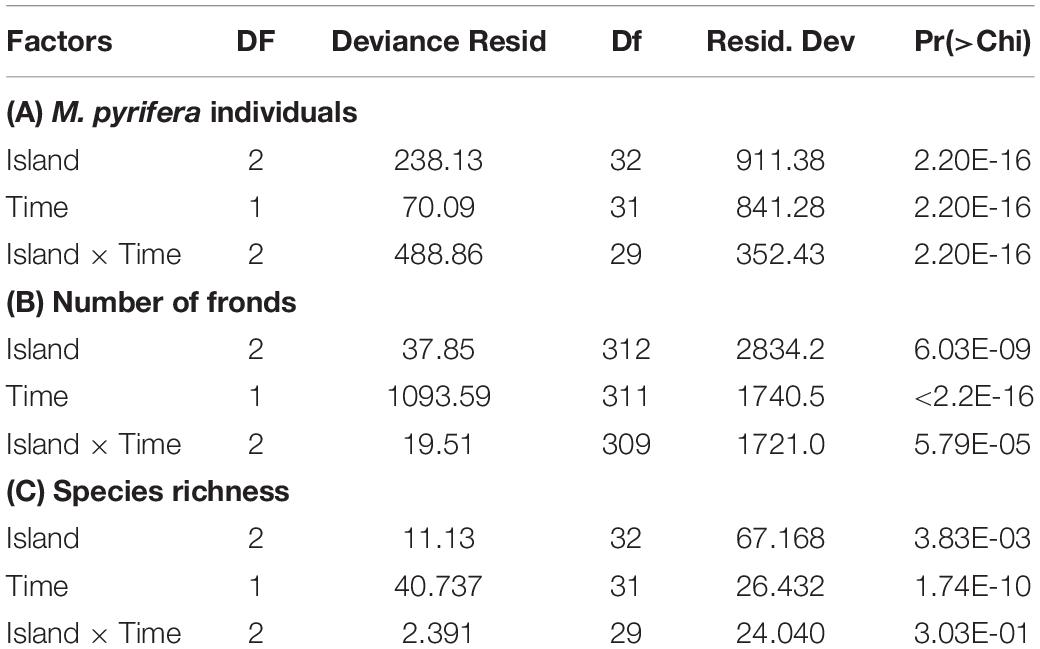
Table 1. Results from the analysis of deviance variance for Poisson generalized linear models (A) for the number of M. pyrifera individuals per 60-m2 transect, (B) for the number of fronds per M. pyrifera individuals, and (C) for species richness of the fish and invertebrate assemblage.
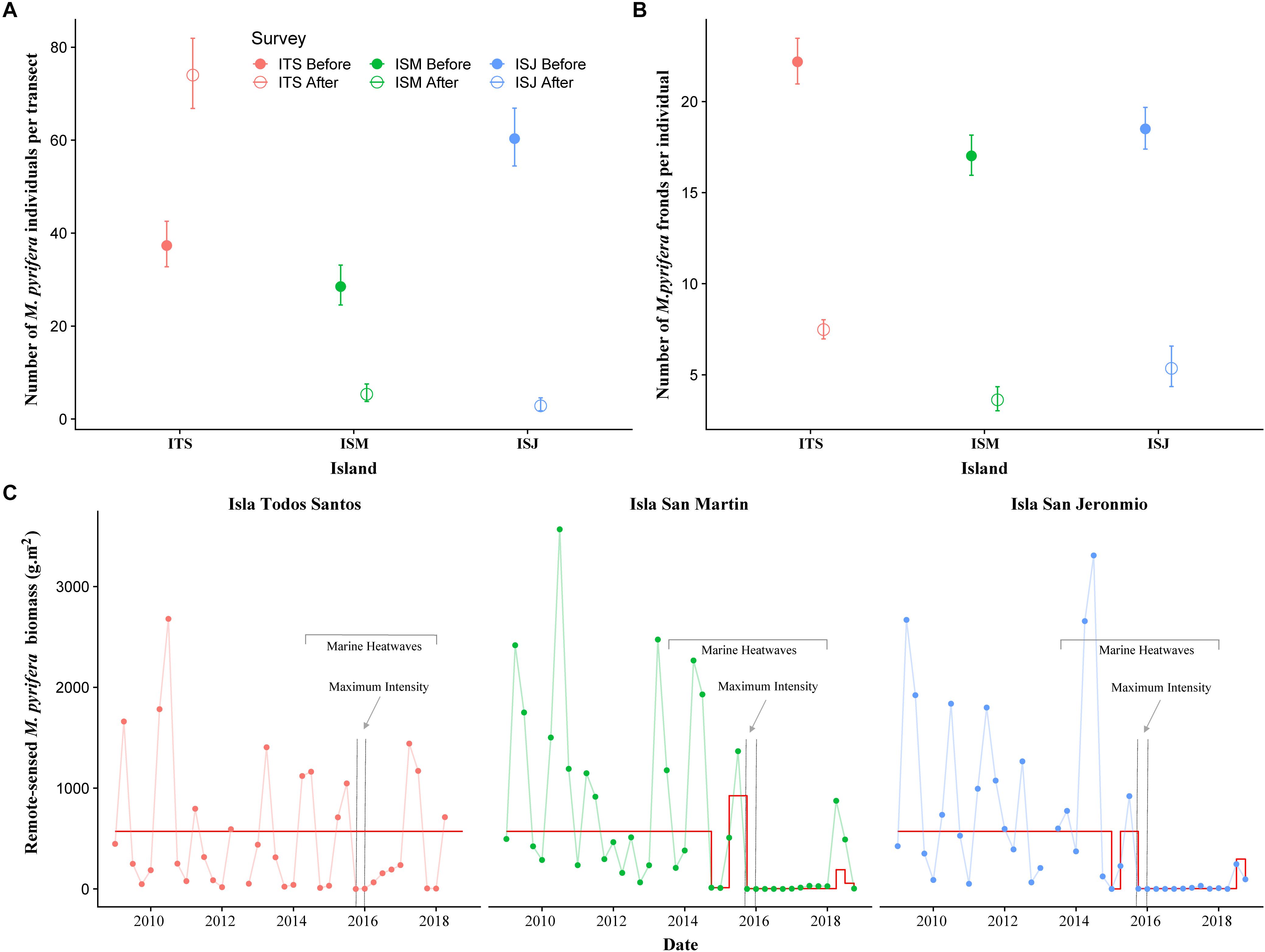
Figure 4. Mean estimated counts of (A) M. pyrifera individuals per 60 m2 transect and (B) number of fronds per individual for surveys before and after the MHWs (±95% confidence intervals) at Isla Todos Santos (ITS), Isla San Martin (ISM), and Isla San Jeronimo (ISJ). Estimates were drawn from models summarized in Table 1. (C) Time series of remote-sensed kelp seasonal mean biomass (g.m–2) at each island. Superimposed on each time series (in red) is the estimated deviation from the expectation of an ARIMA (0,1,1) model fitted to the log10(x + 1) transformed biomass data (deviations were back-transformed to the original scale). Dotted gray lines represent the duration of the MHW with the maximum intensity registered at each island during the 2013–2017 MHWs.
Remote-sensed kelp biomass estimates were highly variable, but we clearly detected the MHWs signal at both ISM and ISJ (Figure 4C). As was the case for number of M. pyrifera individuals (Figure 4A), kelp biomass at ITS contrasted from the pattern at ISM and ISJ, although in this case, the time series analysis suggested no long-term trend (Figure 4C). Irrespective, it was clear that kelp biomass during and after the MHWs at the southernmost islands, ISM and ISJ, was well beyond the bounds of natural variability observed prior to the MHWs. Qualitatively, satellite-derived kelp biomass estimates allowed us to document the loss of the kelp forests during the MHWs at all islands. Immediately after summer 2015, the satellite images did not detect kelp at the surface of the three islands, and the biomass remained negligible until the end of 2016 (Figure 4C). By winter and spring 2017, kelp biomass at ITS had recovered to previous seasonal values, but at ISM and ISJ there was no recovery, and values stayed very low until the end of the time series (end of 2018). Note that very low values of biomass do not necessarily mean that the kelp forest completely disappeared, since satellite images do not detect sub-surface smaller individuals.
Community Changes
Fish and Invertebrates
For the combined fish and invertebrate assemblages, our Poisson GLM of species richness (Figure 5A and Table 1) found significant and consistent declines from before to after the MHWs at all islands (significant main effect for Time). Species richness was significantly higher at ITS than the more-southerly islands (significant main effect for Island), but the analysis revealed no evidence that this decline differed by Island (no significant Time x Island interaction). Proportionally, we observed similar reductions for fish and invertebrate species richness at ITS (57 and 54%, respectively), but the decline in invertebrate species richness was greater at ISM (39 and 53%) and it was dramatic at ISJ (41 and 81%) (Figures 6, 7).
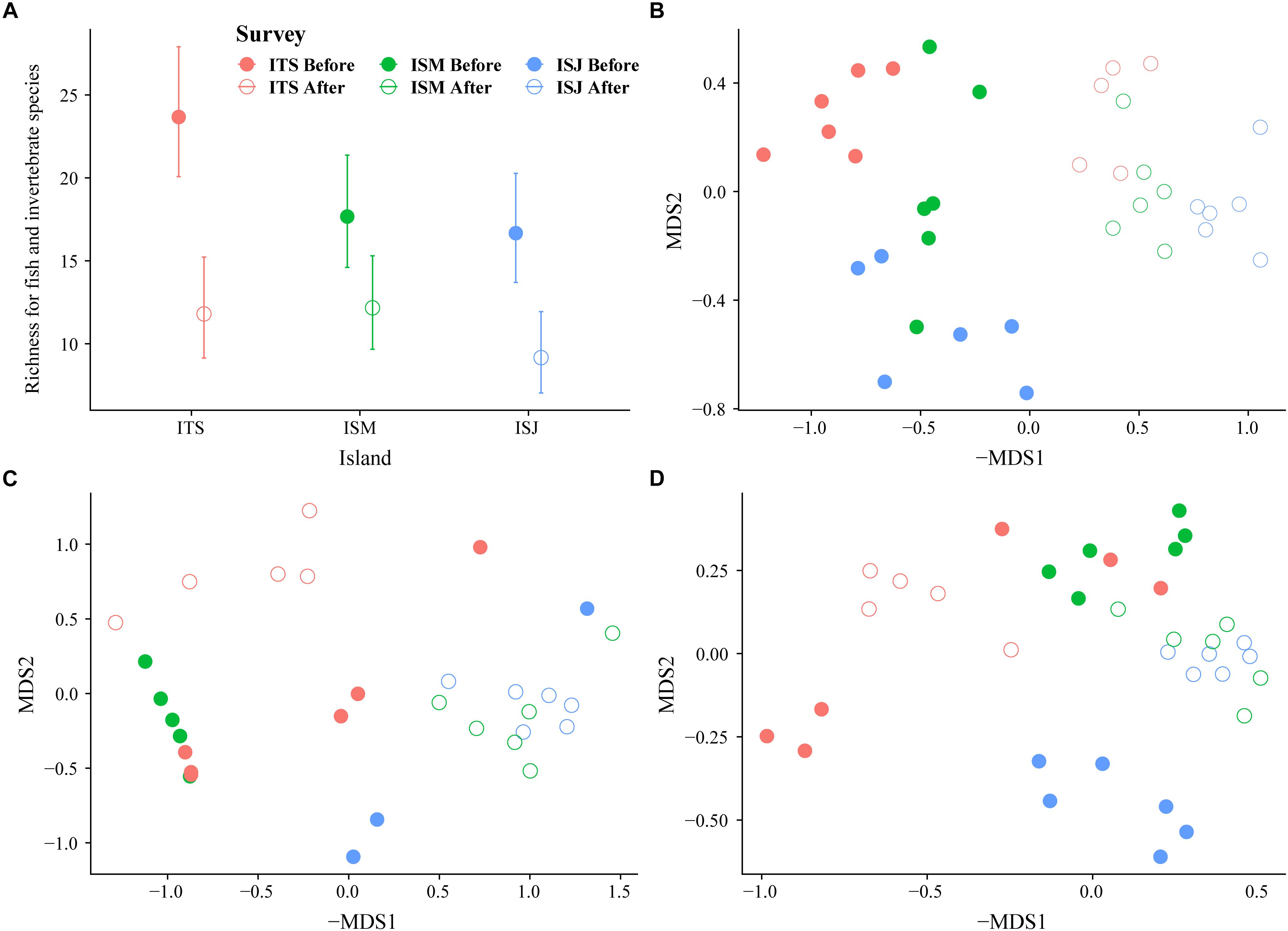
Figure 5. Community change from before to after the MHW. (A) Changes in fish and invertebrate mean species richness from before to after the MHWs (95% confidence intervals) at the three islands. Estimates were drawn from models summarized in Table 2. (B–D) Non-metric multidimensional (nMDS) scaling biplot of Bray-Curtis resemblance matrix computed on square-root transformed comparing (B) the fish and invertebrate, (C) the subcanopy, and (D) the understory algal assemblages among the three islands, Isla Todos Santos, Isla San Martin, and Isla San Jeronimo, and before and after the 2014–2016 warming event of the kelp forest community. Each symbol represents a transect.
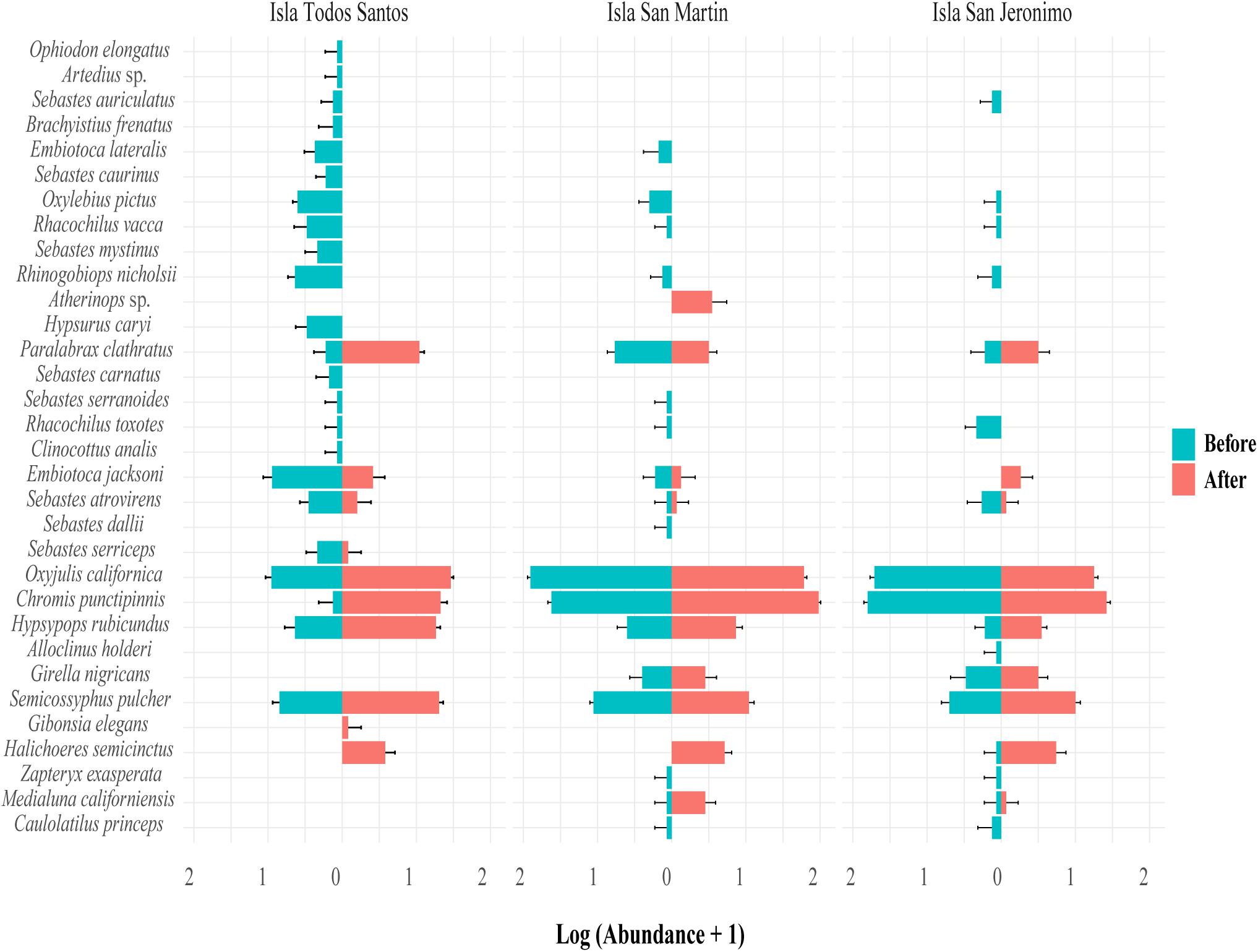
Figure 6. Abundance (mean number of individuals, n = two sites with three census transects, ±95% confidence intervals) of fish from before to after the 2014–2016 MHWs at Isla Todos Santos, Isla San Martin, and Isla San Jeronimo, in Baja California, Mexico. Fish species are organized based on their latitudinal distribution centroid, from north to south.
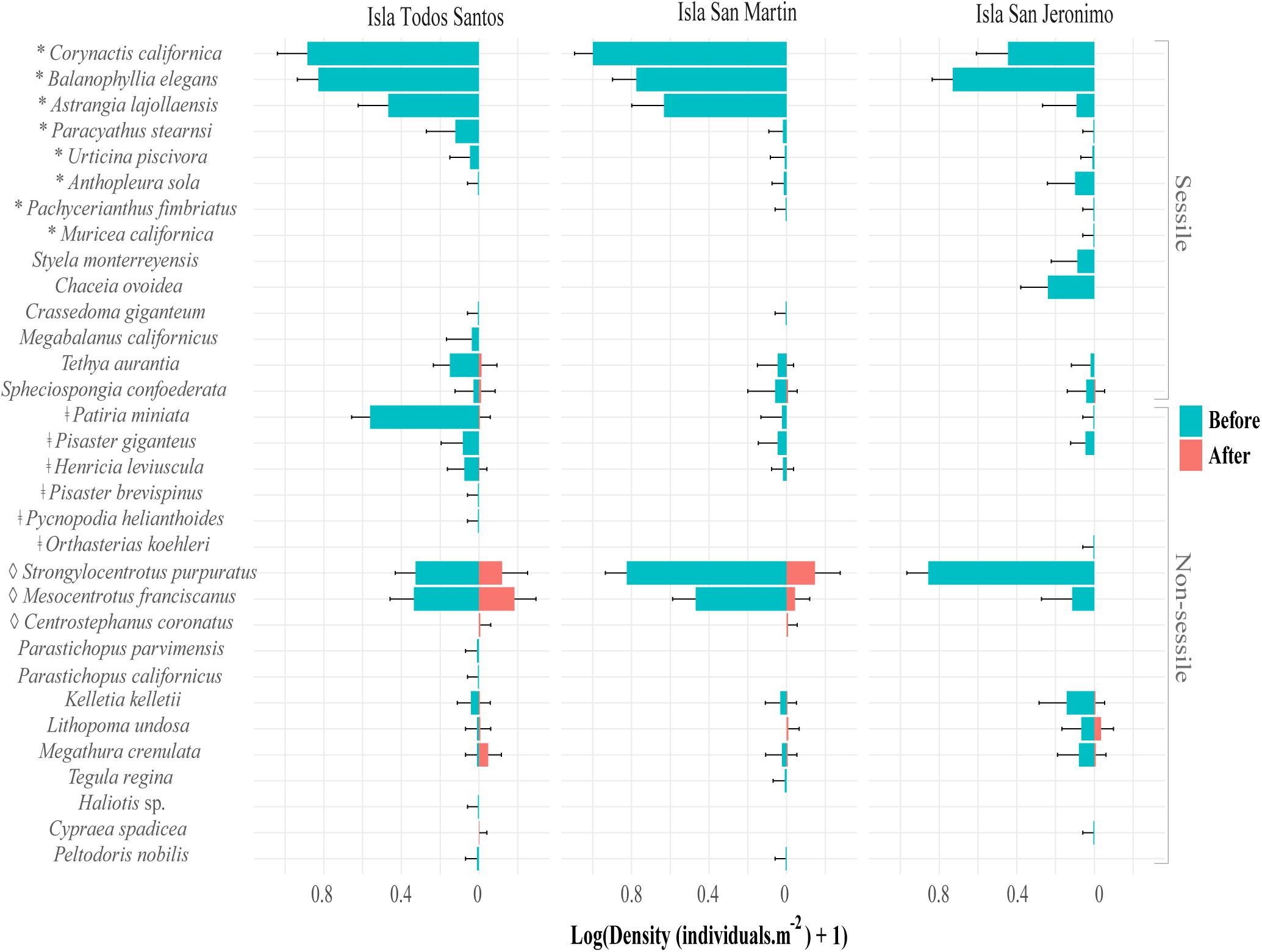
Figure 7. Density of invertebrate species (mean number of individuals.m–2, n = two sites with three video transects, ±95% confidence intervals) from before to after the 2014–2016 MHWs at Isla Todos Santos, Isla San Martin, and Isla San Jeronimo, in Baja California, Mexico. ∗Represents cnidaria species, ‡ sea star species, and ♢ sea urchin species.
Corresponding multivariate analysis of assemblage structure for fish and invertebrates (PERMANOVA) (Table 2) and associated post hoc tests (Supplementary Table S4A) revealed not only differences among islands and among times, but that the magnitude of the multivariate difference from before to after the MHWs differed by island (significant Time x Island interaction). The nMDS biplot shows clear differences in assemblage structure from before the MHWs to after (Figure 5B). Post hoc tests confirmed that significant changes in assemblage structure occurred from before the MHWs to afterward at each island (p < 0.043). Because we are most interested in species characterizing the periods before and after the MHWs (rather than differences among islands), we identified species with significant indicator-species values characteristic of these periods (Supplementary Table S6). Before the MHWs, assemblages were characterized by four fish species and fourteen invertebrate species (with the first five most important invertebrate species being more important indicator species than any of the fish). In accordance with the declining species richness in surveys after the MHWs, this period was characterized by four fish species and only one invertebrate species (Supplementary Table S6).
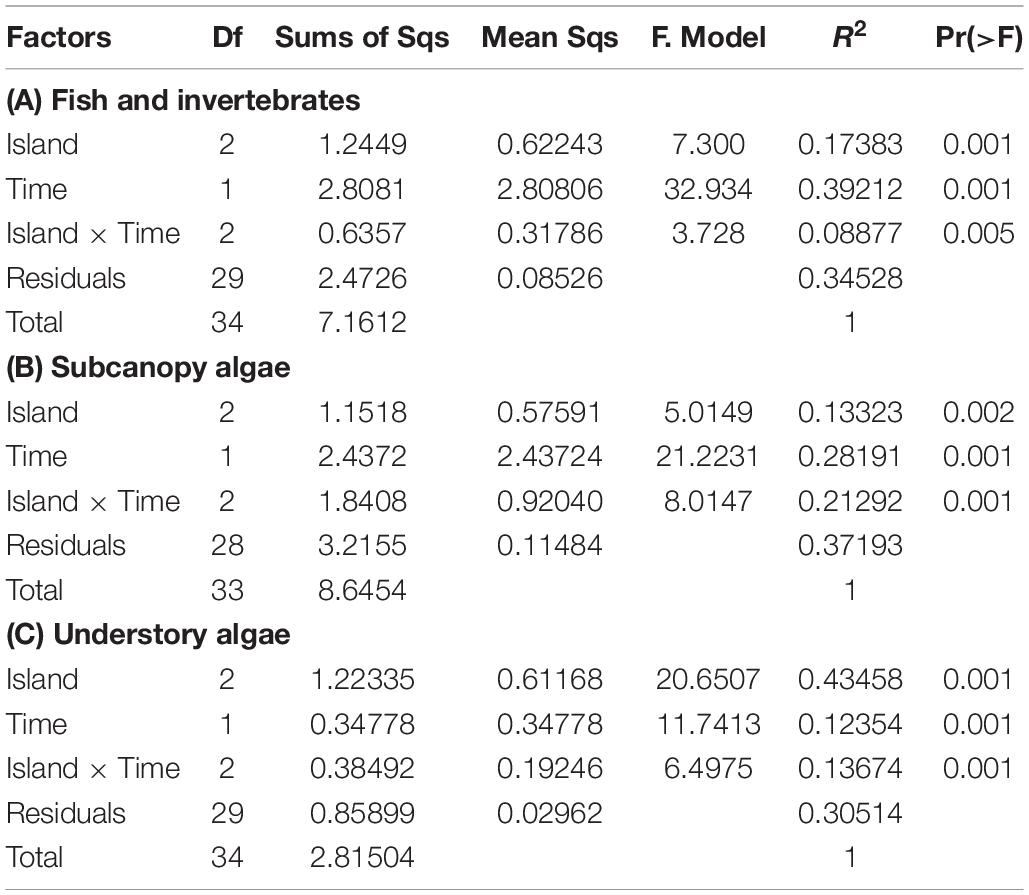
Table 2. Results from the analysis of multivariate variance for PERMANOVAs of response variables as a function of the crossed factors Island and Time based on Bray-Curtis distance matrices derived from square-root transformed data, for (A) density of fish and invertebrate, (B) relative abundance of subcanopy algae, and (C) percent cover of understory algae species per survey.
The loss or decrease of many species with cool-water affinities and the increase or appearance of some warm-affinity species (two species appeared after the MHWs) (Figure 6) drove the fish assemblage changes. The ten species exhibiting the northernmost distributions (based on their latitudinal centroid) (Supplementary Table S5) were absent at the three islands after the MHWs. All Sebastes species, except S. atrovirens and S. serriceps (which registered only one individual of each species at ITS in 2016), disappeared from the islands. S. atrovirens and S. serriceps are the most southerly of the Sebastes species recorded here, with distribution centroids in central California to central Baja California, compared to other Sebastes spp., which have centroids from northern California up to Alaska (FishBase). The three-characteristic fish species before the MHWs, Oxylebius pictus, Rhinogobiops nicholsii and Rhacochilus toxotes (Supplementary Table S6), have a centroid distribution from Oregon, north California and south California, respectively. Of the four-characteristic species after the MHWs, Halichoeres semicinctus, Hypsypops rubicundus, Paralabrax clathratus and Semicossyphus pulcher, all except P. clathratus have a southerly distribution, with centroids from northern to central Baja California (Supplementary Tables S5, S6).
A generalized decrease in species richness drove invertebrate assemblage changes (Figure 7). The number of species in all studied taxonomic groups declined from before to after the MHWs, with the loss of almost all sessile invertebrate species, especially cnidarians. Non-sessile species were also negatively affected. The number of echinoderm species decreased at ITS and ISM, with a notable decline at ISJ, where no echinoderms were present after the MHWs (Figure 7). Both red (Mesocentrotus franciscanus) and purple urchin (Strongylocentrotus purpuratus) densities decreased at ITS and ISM, while Centrostephanus coronatus, absent before the MHWs, appeared after at both islands (Figure 7). The starfish Henricia leviuscula and Patiria miniata were common before the MHWs, and almost disappeared afterward; furthermore, Pisaster giganteus disappeared from all islands (Figure 7). The only invertebrate species that was characteristic after the MHWs is C. coronatus (Supplementary Table S6), which has a southern distribution from southern California, United States, to the Galapagos Islands, Ecuador.
Subcanopy and Understory Algae
For both subcanopy and understory algae, PERMANOVA revealed not only that the assemblage structures differed significantly from before to after the MHWs (p < 0.0135 and 0.0063 for subcanopy and understory species, respectively), but also that the magnitude differed by island (Table 2). This pattern was reflected in corresponding nMDS biplots (Figures 5C,D) and associated post hoc tests (Supplementary Tables S4B,C). For subcanopy assemblage structure, only ISM and ITS differed significantly (p = 0.0086) before the MHWs (largely due to the substantial variability in assemblage structure within islands – Figure 5C). After the MHWs, assemblage structure differed significantly among ITS and the southernmost islands (p = 0.0086), but not between ISM and ISJ. For understory species, assemblage structure differed significantly among islands before the MHWs (p < 0.0298) and afterward (p < 0.0063), except between the southernmost islands. The limited number of species in each of these analyses meant that there were few significant indicator species in each instance. Previously absent introduced algae species, Undaria pinnatifida, appeared after the warming event at ITS, and Sargassum hornerii at ISM and ISJ (Figure 8A). For subcanopy species, Laminaria farlowii was characteristic of assemblages before the MHWs, while Ecklonia arborea, Cystoceira sp., and the introduced S. hornerii were characteristic afterward (Figure 8A and Supplementary Table S6). For the understory algal assemblages, red crustose algae were characteristic before the MHWs, with brown algae and articulated coralline species characteristic afterward (Figure 8B and Supplementary Table S6). In the three islands, the percent cover of non-coralline crustose algae increased after the warming event (Figure 8B).
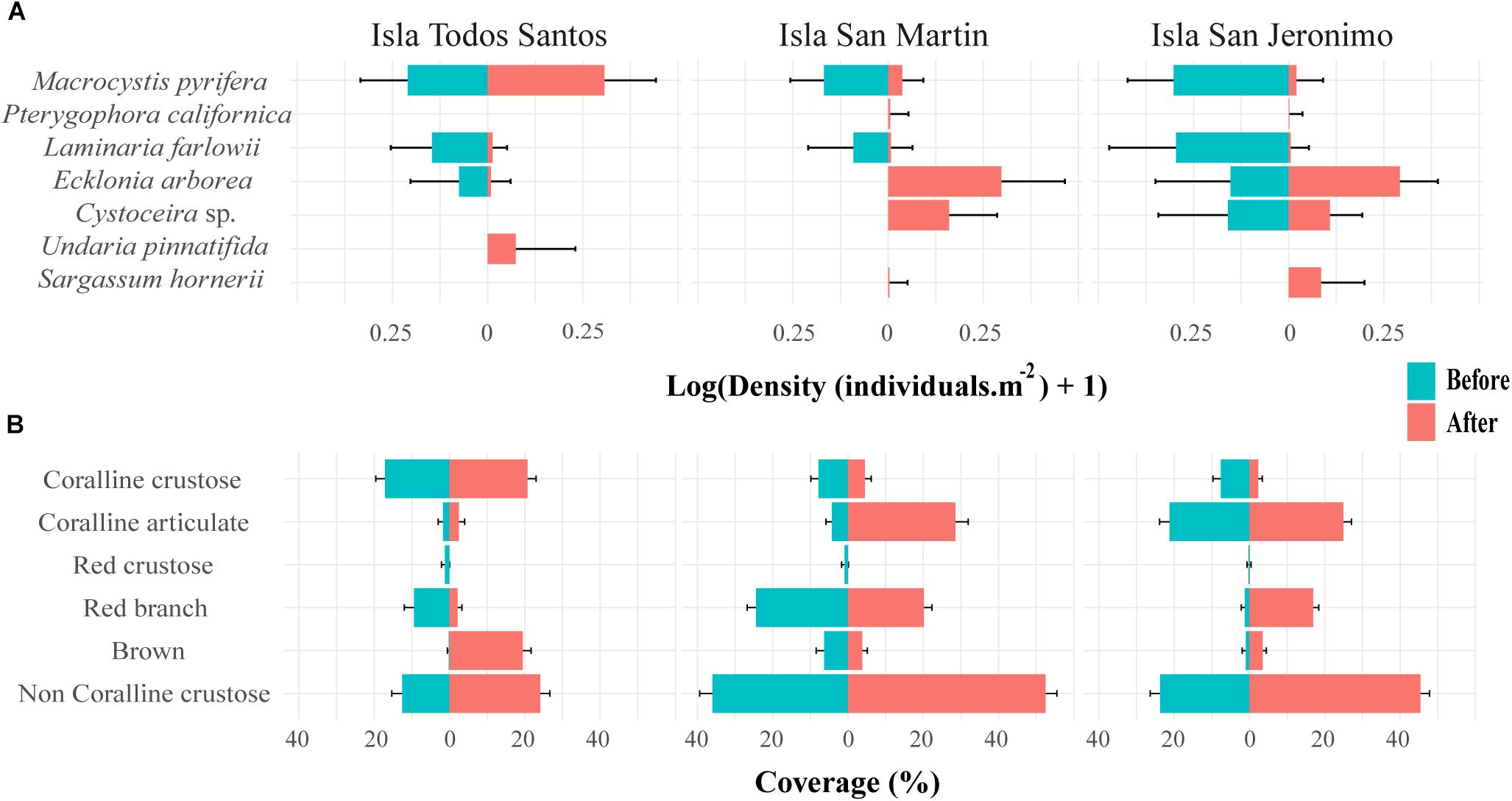
Figure 8. (A) Density of Macrocystis pyrifera, subcanopy algae, and introduced algae species (mean number of individuals.m–2, n = two sites with three video transects, ±95% confidence intervals). (B) Percent cover (mean, n = two sites with three video transects, ±95% confidence intervals) of understory algae from before to after the 2014–2016 MHWs at Isla Todos Santos, Isla San Martín, and Isla San Jeronimo, in Baja California, Mexico.
Discussion
Anthropogenic climate change is expected to remain one of the main threats to biological systems throughout the 21st century (Thomas et al., 2004; Pereira et al., 2010; Pecl et al., 2017), making it a central theme in ecology, and challenging science to understand and predict ecosystems’ responses to these changing conditions. Among the manifestations of climate change is the intensification of MHWs (Oliver et al., 2018), with corresponding impacts reported globally in different taxonomic groups and geographic areas (Garrabou et al., 2009; Marba and Duarte, 2010; Smale and Wernberg, 2013; Voerman et al., 2013; Short et al., 2015; Cavole et al., 2016; Oliver et al., 2017; Tuckett et al., 2017; Ruthrof et al., 2018; Smale et al., 2019). In this context, it is relevant to identify and monitor those areas where sentinel species for climate change are more responsive to warming and to MHWs in particular, such as near warm edge distribution limit. We evaluated changes in the kelp forest community structure after the extreme 2014–2016 warming event, near the southern distribution limit of M. pyrifera in the CCS. The timing of this study revealed the sensitivity of our study sites to the MHWs, with loss of M. pyrifera forests and significant assemblage changes for fish, invertebrates and algae.
MHWs and the Loss of M. pyrifera
The 2014–2016 warming was the most intense and sustained event, with the highest percentage of MHW days registered, in the 37-year historical series for the CCS. Contrary to past El Niños, which appeared after negative temperature anomalies, the strong 2015–2016 El Niño began with an already warmer ocean (“Blob”) (Jacox et al., 2016). The unprecedented combination of these two events preceded the loss of M. pyrifera after summer of 2015, corresponding with the maximum temperature anomalies, which peaked at 5.9°C warmer than the climatology (this heatwave lasted for around 4 months). These anomalies were ∼2°C warmer than any previous registered (El Niños), most likely with temperatures exceeding physiological thresholds for growth, survival, and reproduction of M. pyrifera (Zimmerman and Robertson, 1985; Hernández Carmona, 1988; Tegner et al., 1997; Hernandez-Carmona et al., 2001). The absence of significant wave disturbance during this event (Reed et al., 2016) suggests a combination of thermal stress and nutrient-poor waters (Whitney, 2015) as the primary drivers behind kelp canopy disappearance in Baja California. Our results are consistent with previous work that reports the sensitivity of M. pyrifera in southern California and Baja California to warm, nutrient-poor waters (Zimmerman and Kremer, 1984; Hernandez-Carmona et al., 2001) during El Niño events (Dayton and Tegner, 1984; Dayton, 1985; Zimmerman and Robertson, 1985; Tegner and Dayton, 1987, 1991; Hernández Carmona, 1988; Tegner et al., 1996; Ladah et al., 1999; Hernandez-Carmona et al., 2001; Edwards, 2004; Edwards and Hernandez-Carmona, 2005; Edwards and Estes, 2006).
Reed et al. (2016) found no ecosystem effects in M. pyrifera kelp forests at Santa Barbara, California, to the same extreme warming event. They argued that the variability of giant kelp biomass was high during the 15 studied years and that the observed decline was within normal variability. However, fewer impacts to kelp forests located at Santa Barbara are expected since they are located ∼1000 km north of the southern distribution limit (i.e., the upper limit of thermal tolerance of M. pyrifera). There, average SSTs are ∼3.3°C cooler than at our study sites, providing a greater population-level thermal safety margin (Pinsky et al., 2019). We found similar results in our northernmost site, ITS, located ∼400 km south of Santa Barbara (nevertheless satellite derived biomass estimates remained very low during autumn 2015 and all 2016). However, in the southernmost sites, ISM and ISJ, located 550 and ∼650 km south of Santa Barbara, respectively, we clearly detected the MHWs signal on M. pyrifera forests. Thus our results support previous studies in the CCS and other regions (Edwards, 2004; Edwards and Estes, 2006; Johnson et al., 2011; Wernberg et al., 2013, 2016; Horta e Costa et al., 2014), which reported kelp forest located near temperate-subtropical transition zones to be more vulnerable to historical warming events than those at higher latitudes. For example, following the MHWs of 2011 in Western Australia, Wernberg et al. (2016) reported a regime shift from a kelp to a seaweed turf dominated ecosystem in sites located near temperate-subtropical transition zones, but little or no change in communities at higher latitudes. Similarly, after 2017/2018 MHWs in New Zealand, Thomsen et al. (2019), found that Durvillaea spp. species located at warmer sites were more affected than those found in cooler ones. Although Reed et al. (2016), challenged the status of M. pyrfiera as a sentinel species for climate change, our work suggests that sentinel status should be evaluated in a site-by-site or regional basis, and reinforces the status of Baja California as a sentinel area for climate change (Hobday and Pecl, 2014).
Macrocystis pyrifera forests in the CCS have proven to be dynamic and resilient ecosystems (Dayton et al., 1992; Filbee-Dexter and Scheibling, 2014), recovering quickly after previous El Niño events. La Niña conditions that usually follow El Niño events bring cold, nutrient-rich waters, representing favorable conditions for M. pyrifera (Dayton et al., 1992; Tegner et al., 1997; Edwards and Estes, 2006). The lack of La Niña conditions after the 2014–2016 warming event and the persistence of anomalously high SST during 2017 (around 50 days registered as MHWs) is probably challenging the capacity of M. pyrifera to recover.
Nevertheless, after the warming event M. pyrifera recovered at ITS, the northernmost site. We found higher densities of individuals than before the MHWs, but with significantly lower number of fronds per individual, indicating the dominance of young M. pyrifera individuals. The presence of some productive individuals (≥16 fronds/individual) implies that M. pyrifera did not lose its reproductive capacity, since sporophylls begin to differentiate and have fertile sori when they have around 16 fronds/indivdual (Dayton et al., 1992). We found a strong effect of the MHWs on M. pyrifera densities and number of fronds per individual at the southernmost sites, ISM and ISJ, suggesting a very low density of fertile individuals. Biomass satellite time-series corroborate that kelp forest at ITS recovered after the MHWs, while at ISM and ISJ biomass values remained below average seasonal values throughout 2018. The three islands have similar mean SSTs, suggesting similar thermal safety margins, however, SSTAs and the maximum intensities registered during the MHWs were slightly higher at the southernmost sites than at ITS, which could explain the different latitudinal responses of the kelp forest to the extreme warming. However, it is also true that the differences in the anomalies were very small (∼0.2°C) between islands and other factors such as local seascape complexity, which might create “microclimates” (Woodson et al., 2018; Pinsky et al., 2019), could also contribute to the observed differences. Previous studies found that deeper sites represented local refugia and individuals living there could find and survive strong warming events such as El Niño, possibly serving as a source of spores and recruits to colonized shallower rocky areas (Ladah et al., 1999). Further research could test whether the observed differences are a result of local seascape variability, latitudinal gradients in SSTAs, or perhaps a combination of both.
Community-Level Impacts
The extreme conditions resulted in dramatic changes in the invertebrate, fish, and algal assemblages, with the loss of over half of invertebrate species. The significant reduction or disappearance of M. pyrifera can have profound effects on other members of the community (Graham, 2004) due to a release of resources (space and light) (Reed and Foster, 1984; Dayton, 1985) and a decrease in food availability (Ebeling et al., 1985; Harrold and Reed, 1985). Although we found evident changes, we cannot separate between the effects of high SSTs and loss of canopy on the community, since both are synergistically affecting all food web levels. Importantly, we found that M. pyrifera dynamics at the two sothernmost islands in our study immediately after the MHWs were beyond the limits of natural variation in the previous 5 years, and remained that way until the end of our study in 2018. Although all other metrics we analyzed represented only two periods, a year before and a year after the MHW, this finding that kelp biomass was significantly and persistently impacted by the MHW gives us some confidence in attributing community-wide impacts to the effects of the MHWs.
Loss of Sessile Invertebrates and Sea Stars
After the warming event, almost all sessile invertebrates disappeared, and all cnidarians were absent. Sessile invertebrates are highly sensitive to extreme temperature conditions due to their inability to escape from unfavorable conditions (Przeslawski et al., 2008) and their dependence on phytoplankton and suspended organic matter as food sources that might decrease during warming events (e.g., during El Niño events, when upwelling is suppressed). Reports of mass mortality of sessile species and bleaching of corals in temperate and tropical ecosystems (Berkelmans and Willis, 1999; Hoegh-Guldberg, 1999; Cerrano et al., 2000; Marshall and Baird, 2000; Perez et al., 2000; Garrabou et al., 2001, 2009; Caputi et al., 2014; Couch et al., 2017; Hughes T. P. et al., 2017) suggest that SSTs at our sites probably peaked at values in excess of their physiological tolerances (Lejeusne et al., 2010). Moreover, almost all sea star species disappeared or dramatically decreased their abundance. In 2014, an epidemic outbreak of wasting disease from Alaska to Mexico led to mass mortalities of many sea star species (Hewson et al., 2014). Anomalously warm water conditions in the CCS have been suggested to favor the spread of the densovirus linked to this disease and thus increase the mortality rate in sea stars (Eckert et al., 2000; Hewson et al., 2014).
Loss of Cold-Water Species and Increase in Some Warm Affinity Ones
For the entire community, we report the loss or decrease in abundance of many species with cold-water affinities and the appearance or increase in abundance of some species with warm-water affinities. The tropicalization of fish species near temperate transition zones has already been attributed as the primary driver of composition change in the fish assemblage (Wernberg et al., 2013, 2016; Caputi et al., 2014; Horta e Costa et al., 2014; Vergés et al., 2014; Smale et al., 2019). The loss of the ten fish species having the most northerly distribution centroids suggests SSTs peaked above their thermal tolerances and favored species with stronger affinity to warmer waters, such as H. semicinctus (Fishbase). We also reported the presence of the sea-urchin C. coronatus at ITS and ISM, which has the southernmost distribution range of invertebrate species described in this study. Similar patterns favoring C. coronatus have been reported during El Niño 2009–2010, where populations in one site at Oaxaca (Pacific southern Mexico) increased (López-Pérez et al., 2016), and during El Niño 2015 in southern California, with reports of poleward range expansion (Freiwald et al., 2016). Another example is a species from the same genus, Centrostephanus tenuispinus, which was the main winner after an extreme MHW in 2011 on the west coast of Australia (Smale et al., 2017).
The effect of warm water temperatures was also evident in kelp forest subcanopy species L. farlowii and E. arborea. L. farlowii was the characteristic subcanopy species before the MHWs, probably due to the absence (or very low densities) of the competitively superior Pterygophora californica (Tegner et al., 1997). In 2016, abundance of L. farlowii dramatically decreased, despite favorable niche conditions (light and space) after the loss of M. pyrifera forest. The sensitivity of Laminaria species to warm water conditions (Schiel et al., 2004) might explain its decrease after the extreme warming. On the contrary, abundances of E. arborea, known to be favored during El Niño conditions due to its tolerance to warm, nutrient-poor conditions (Dayton et al., 1984; Hernandez-Carmona et al., 2000; Matson and Edwards, 2007), increased at the two southernmost islands.
The influence of the warming event on the understory algae was less evident and more variable than for the rest of the community. For the southernmost islands (ISM and ISJ), we found a decrease in cover of coralline crustose algae, which have been reported to be sensitive to MHWs (Martin and Gattuso, 2009; Smale and Wernberg, 2013; Voerman et al., 2013; Wernberg et al., 2013, 2016; Short et al., 2015; Thomsen and South, 2019). However, the role of heatwaves in regulating abundance of coralline algae is still not clearly understood, since other factors, such as the loss of canopy cover (Irving et al., 2004, 2005; Flukes et al., 2014) or ocean acidification (McCoy and Kamenos, 2015), might also be influential for this group. The presence of coralline crustose algae can be facilitated by canopy-forming kelp species as they exclude other macroalgal competitors (Thomsen and South, 2019). This could explain the decrease of this taxon at the southernmost islands after the MHWs, while at the northernmost site, their cover and the abundance of M. pyrifera remained high. Little attention has been paid to coralline crustose algae, despite representing important habitats, and food sources for diverse epifaunal invertebrates (Graham et al., 2007; Thomsen and South, 2019), as well as providing chemical cues for abalone settlement (Morse et al., 1979; Miner et al., 2006). A generalized increase in the coverage of non-crustose coralline understory algae at all sites suggests that the combined loss of canopy kelp, sessile invertebrates, and coralline crustose algae might have modified light conditions and liberated free space for recruits (Reed and Foster, 1984), which probably favored the rest of the understory assemblage (Reed and Foster, 1984; Dayton et al., 1992; Tegner et al., 1997; Clark et al., 2004).
The Appearance of Invasive Species
Finally, we report the appearance of two invasive macroalgal species, U. pinnatifida at ITS and S. hornerii at ISM and ISJ. In Baja California, U. pinnatifida was first detected in 2003 (Aguilar-Rosas et al., 2004) and S. hornerii in 2005 (Aguilar-Rosas et al., 2007). In 2013, both species appeared during monitoring surveys at the protected side of the three islands, but were absent from the exposed sides (our study sites) (Montaño-Moctezuma et al., 2014). U. pinnatifida is an opportunistic cosmopolitan invasive species (Lowe et al., 2000) able to tolerate a wide range of temperatures (Morita et al., 2003), allowing this species to colonize empty spaces left by canopy-forming kelps. Nevertheless, without disturbance, U. pinnatifida rarely establishes under a dense canopy (Valentine and Johnson, 2003; Thomsen and South, 2019; Thomsen et al., 2019). Similar findings have been reported in other parts of the world as a result of both MHWs (Thomsen et al., 2019) and manipulated removal experiments (Thomsen and South, 2019). For example, in summer 2017–2018, strong MHWs in New Zealand caused the decline of the canopy-forming kelp species Durvillaea spp., and a successive subsequent invasion by U. pinnatifida (Thomsen and South, 2019; Thomsen et al., 2019). Our study supports previous research that opportunistic and invasive species are usually outcompeted by native species, but adverse conditions can leave empty niche space and trigger their expansion (Valentine and Johnson, 2003, 2004; Occhipinti-Ambrogi, 2007; Epstein and Smale, 2017; South et al., 2017). Although it is difficult to predict the long-term effect of invasive species, more frequent MHWs might increase the probability that invasive species colonize and perhaps displace native species.
General Implications
With one-time observation before and after, the ecological changes we describe cannot be confidently attributed to the MHWs. However, we chose our study sites on inhabited islands, since they are less impacted by cumulative human activities (Arafeh-Dalmau et al., 2017) than coastal areas, but are vulnerable ecosystems due to their isolation (Johnson and Black, 2006; Bell, 2008) making them ideal sites to understand kelp forests response to MHWs. Our study sites span a 250-km latitudinal gradient (almost half of the distribution of kelp in Baja California), allowing us to capture a good portion of the biogeographic variability of the region. There is also strong circumstantial evidence that the MHWs were, indeed, the driver of the observed changes: not only was there a corroborating signal in remote-sensed time series of kelp biomass, but we are unaware of any other plausible human-induced changes during the 2013–2018 period that could have altered the community structure in this way. Nevertheless, considering that the response of communities to the same disturbance might vary within the same region (Wernberg et al., 2013, 2016; Thomsen et al., 2019) and even within sites (Woodson et al., 2018), we acknowledge the need to understand the dynamics of these ecosystems at broader spatial and temporal scales and emphasize that our results should not be extrapolated without due care.
Kelp forest coverage has been reduced worldwide (Krumhansl et al., 2016; Wernberg et al., 2019), in part, due to climate change. Moreover, in some areas, their historical distribution limit has contracted poleward (Johnson et al., 2011; Wernberg et al., 2013, 2016), with clear signs of tropicalization of temperate ecosystems (Vergés et al., 2014). If positive temperature anomalies persist (2018 was still an anomalously warm year), the chronic effects of these warming events might shift Baja California’s ecosystems to a new alternative state, potentially precipitating range contractions for M. pyrifera and other species. Although future ecological states are difficult to predict in such dynamic ecosystems, our findings might be an early indication of a possible tropicalization of Baja California’s kelp forest (Cheung et al., 2013) under climate change, emphasizing the need to monitor and manage these ecosystems.
Southern kelp forests of the CCS are a shared marine resource between the south of California and the north of Baja California (Ramírez-Valdez et al., 2017). Advancing the understanding of the ecological impacts of MHWs (Hobday and Pecl, 2014) in this transboundary region to promote adequate management strategies will require a binational effort (Aburto-Oropeza et al., 2018). Thus, the protection of these valuable ecosystems needs immediate actions and close collaboration between southern California and Baja California (Arafeh-Dalmau et al., 2017). If we fail to act, once-abundant, extensive M. pyrfiera forests could experience important local declines, as they have in other regions (Johnson et al., 2011), leading to detrimental ecological and socio-political outcomes (Frölicher and Laufkötter, 2018).
Data Availability
All datasets generated for this study are included in the manuscript and/or the Supplementary Files.
Author Contributions
GM-M, GT-M, and NA-D designed the initial project with the final review of RB-L, JM, and DS. NA-D, GT-M, RB-L, and JM collected the data. NA-D, GM-M, and DS conducted the data analysis and interpretation and were discussed by all other authors. NA-D and GM-M drafted the work and was revised by all other authors. All authors approved the version to be published and agreed to be accountable for all aspects of the work in ensuring that questions related to the accuracy or integrity of any part of the work are appropriately investigated and resolved.
Funding
We gratefully acknowledge the following sources of funding that supported this research: PROMEP No. 10676. UABC–CA47 to GM-M and GT-M, PADI Foundation for the research grant to NA-D (CGA App 21977), UABC 19va-385 to RB-L and GM-M, UCMexus-CONACyT CN-17-117 to RB-L and GM-M, and the Fundación Bancaria “La Caixa” for the Postgraduate Fellowship (LCF/BQ/AA16/11580053) to NA-D.
Conflict of Interest Statement
The authors declare that the research was conducted in the absence of any commercial or financial relationships that could be construed as a potential conflict of interest.
Acknowledgments
We are deeply thankful to the Kelp Forest Ecological Monitoring Group at Universidad Autónoma de Baja California and to all the SCUBA divers involved in the fieldwork. We thank Arturo Ramírez Valdez and Luis Aguilar Rosas for their essential contribution to establish the monitoring program. Also, we thank Kyle Cavanaugh and Tom Bell for providing Satellite kelp cover data.
Supplementary Material
The Supplementary Material for this article can be found online at: https://www.frontiersin.org/articles/10.3389/fmars.2019.00499/full#supplementary-material
References
Aburto-Oropeza, O., Johnson, A. F., Agha, M., Allen, E. B., Allen, M. F., González, J. A., et al. (2018). Harnessing cross-border resources to confront climate change. Environ. Sci. Policy 87, 128–132.
Aguilar-Rosas, L. E., Aguilar-Rosas, R., Kawai, H., Uwai, S., and Valenzuela-Espinoza, E. (2007). New record of sargassum filicinum harvey (Fucales, Phaeophyceae) in the pacific coast of mexico. Algae 22, 17–21. doi: 10.4490/algae.2007.22.1.017
Aguilar-Rosas, R., Aguilar-Rosas, L. E., Avila-Serrano, G., and Marcos-Ramírez, R. (2004). First record of undaria pinnatifida (Harvey) SURINGAR (laminariales, phaeophyta) on the pacific coast of mexico. Botanica Marina 47, 255–258.
Anderson, M. J. (2001). A new method for non-parametric multivariate analysis of variance. Austral Ecol. 26, 32–46. doi: 10.1111/j.1442-9993.2001.01070.pp.x
Arafeh-Dalmau, N., Torres-Moye, G., Seingier, G., Montaño-Moctezuma, G., and Micheli, F. (2017). Marine spatial planning in a transboundary context: linking Baja California with California’s network of marine protected areas. Front. Mar. Sci. 4:150. doi: 10.3389/fmars.2017.00150
Banzon, V., Smith, T. M., Chin, T. M., Liu, C., and Hankins, W. (2016). A long-term record of blended satellite and in situ sea-surface temperature for climate monitoring, modeling and environmental studies. Earth Syst. Sci. Data 8, 165–176. doi: 10.5194/essd-8-165-2016
Beas-Luna, R., and Ladah, L. B. (2014). Latitudinal, seasonal, and small-scale spatial differences of the giant kelp, macrocystis pyrifera, and an herbivore at their southern range limit in the northern hemisphere. Botanica Marina 57, 73–83.
Bell, J. J. (2008). Connectivity between island Marine Protected Areas and the mainland. Biol. Conserv. 141, 2807–2820. doi: 10.1038/s41598-018-23004-2
Benjamini, Y., and Hochberg, Y. (1995). Controlling the false discovery rate: a practical and powerful approach to multiple testing. J. R. Stat. Soc. Ser. B 57, 289–300. doi: 10.1111/j.2517-6161.1995.tb02031.x
Berkelmans, R., and Willis, B. (1999). Seasonal and local spatial patterns in the upper thermal limits of corals on the inshore Central Great Barrier Reef. Coral Reefs 18, 219–228. doi: 10.1007/s003380050186
Bond, N. A., Cronin, M. F., Freeland, H., and Mantua, N. (2015). Causes and impacts of the 2014 warm anomaly in the NE Pacific. Geophys. Res. Lett. 42, 3414–3420. doi: 10.1002/2015gl063306
Byrnes, J. E., Reed, D. C., Cardinale, B. J., Cavanaugh, K. C., Holbrook, S. J., and Schmitt, R. J. (2011). Climate-driven increases in storm frequency simplify kelp forest food webs. Glob. Change Biol. 17, 2513–2524. doi: 10.1111/j.1365-2486.2011.02409.x
Caputi, N., Jackson, G., and Pearce, A. (2014). The Marine Heat Wave Off Western Australia During the Summer of 2010/11: 2 Years On. Fremantle, WA: Western Australian Fisheries and Marine. Fisheries Research Report No.250.
Carr, M. H., and Reed, D. C. (2015). Shallow Rocky Reefs and Kelp Forests. Berkeley, CA: University of California Press.
Cavanaugh, K. C., Siegel, D. A., Reed, D. C., and Dennison, P. E. (2011). Environmental controls of giant-kelp biomass in the santa barbara channel. California. Mar. Ecol. Prog. Ser. 429, 1–17. doi: 10.3354/meps09141
Cavole, L. M., Demko, A. M., Diner, R. E., Giddings, A., Koester, I., Pagniello, C. M., et al. (2016). Biological impacts of the 2013–2015 warm-water anomaly in the northeast pacific: winners, losers, and the future. Oceanography 29, 273–285.
Cerrano, C., Bavestrello, G., Bianchi, C. N., Cattaneo-Vietti, R., Bava, S., Morganti, C., et al. (2000). A catastrophic mass-mortality episode of gorgonians and other organisms in the Ligurian Sea (North-western Mediterranean), summer 1999. Ecol. Lett. 3, 284–293. doi: 10.1046/j.1461-0248.2000.00152.x
Cheung, W. W., Watson, R., and Pauly, D. (2013). Signature of ocean warming in global fisheries catch. Nature 497:365. doi: 10.1038/nature12156
Clark, R., Edwards, M., and Foster, M. (2004). Effects of shade from multiple kelp canopies on an understory algal assemblage. Mar. Ecol. Prog. Ser. 267, 107–119. doi: 10.3354/meps267107
Costanza, R., d’Arge, R., De Groot, R., Farber, S., Grasso, M., Hannon, B., et al. (1997). The value of the world’s ecosystem services and natural capital. Nature 387:253.
Couch, C. S., Burns, J. H., Liu, G., Steward, K., Gutlay, T. N., Kenyon, J., et al. (2017). Mass coral bleaching due to unprecedented marine heatwave in papahānaumokuākea marine national monument (Northwestern Hawaiian Islands). PLoS One 12:e0185121. doi: 10.1371/journal.pone.0185121
Dayton, P. K. (1985). Ecology of kelp communities. Annu. Rev. Ecol. Syst. 16, 215–245. doi: 10.1146/annurev.ecolsys.16.1.215
Dayton, P. K., Currie, V., Gerrodette, T., Keller, B. D., Rosenthal, R., and Tresca, D. V. (1984). Patch dynamics and stability of some California kelp communities. Ecol. Monogr. 54, 253–289. doi: 10.2307/1942498
Dayton, P. K., Tegner, M. J., Parnell, P. E., and Edwards, P. B. (1992). Temporal and spatial patterns of disturbance and recovery in a kelp forest community. Ecol. Monogr. 62, 421–445. doi: 10.2307/2937118
Dayton, P. K., and Tegner, N. J. (1984). Catastrophic storms, el nino, and patch stability in a southern california kelp community. Science 224, 283–285. doi: 10.1126/science.224.4646.283
Di Lorenzo, E., and Mantua, N. (2016). Multi-year persistence of the 2014/15 north pacific marine heatwave. Nat. Clim. Change 6:1042. doi: 10.1038/nclimate3082
Dufrêne, M., and Legendre, P. (1997). Species assemblages and indicator species: the need for a flexible asymmetrical approach. Ecol. Monogr. 67, 345–366. doi: 10.1890/0012-9615(1997)067\%5B0345:saaist\%5D2.0.co;2
Ebeling, A., Laur, D., and Rowley, R. (1985). Severe storm disturbances and reversal of community structure in a southern california kelp forest. Mar. Biol. 84, 287–294. doi: 10.1007/bf00392498
Eckert, G. L., Engle, J. M., and Kushner, D. J. (2000). “Sea star disease and population declines at the channel islands,” in Proceedings of the Fifth California Islands Symposium, Camarillo, CA.
Edwards, M., and Hernandez-Carmona, G. (2005). Delayed recovery of giant kelp near its southern range limit in the North Pacific following El Niño. Mar. Biol. 147, 273–279. doi: 10.1007/s00227-004-1548-7
Edwards, M. S. (2004). Estimating scale-dependency in disturbance impacts: El Niños and giant kelp forests in the northeast Pacific. Oecologia 138, 436–447. doi: 10.1007/s00442-003-1452-8
Edwards, M. S., and Estes, J. A. (2006). Catastrophe, recovery and range limitation in NE pacific kelp forests: a large-scale perspective. Mar. Ecol. Prog. Ser. 320, 79–87. doi: 10.3354/meps320079
Epstein, G., and Smale, D. A. (2017). Undaria pinnatifida: a case study to highlight challenges in marine invasion ecology and management. Ecol. Evol. 7, 8624–8642. doi: 10.1002/ece3.3430
Filbee-Dexter, K., and Scheibling, R. E. (2014). Sea urchin barrens as alternative stable states of collapsed kelp ecosystems. Mar. Ecol. Prog. Ser. 495, 1–25. doi: 10.3354/meps10573
Flukes, E., Johnson, C., and Wright, J. (2014). Thinning of kelp canopy modifies understory assemblages: the importance of canopy density. Mar. Ecol. Prog. Ser. 514, 57–70. doi: 10.3354/meps10964
Foster, M. S., and Schiel, D. R. (1985). Ecology of Giant Kelp Forests in California: a Community Profile. Technical Report FWS/OBS-85(7.2) ON: TI86900095 Moss Landing, CA: Moss Landing Marine Labs.
Freiwald, J., Wisniewski, C., Abbott, D., and Game. (2016). Northward range extension of the crowned sea urchin (Centrostephanus coronatus) to monterey bay, california. California Fish 102, 37–40.
Frölicher, T. L., Fischer, E. M., and Gruber, N. (2018). Marine heatwaves under global warming. Nature 560:360. doi: 10.1038/s41586-018-0383-9
Frölicher, T. L., and Laufkötter, C. (2018). Emerging risks from marine heat waves. Nat. Commun. 9:650. doi: 10.1038/s41467-018-03163-6
Garrabou, J., Coma, R., Bensoussan, N., Bally, M., Chevaldonné, P., Cigliano, M., et al. (2009). Mass mortality in northwestern mediterranean rocky benthic communities: effects of the 2003 heat wave. Glob. Change Biol. 15, 1090–1103. doi: 10.1111/j.1365-2486.2008.01823.x
Garrabou, J., Perez, T., Sartoretto, S., and Harmelin, J. (2001). Mass mortality event in red coral Corallium rubrum populations in the Provence region (France, NW Mediterranean). Mar. Ecol. Prog. Ser. 217, 263–272. doi: 10.3354/meps217263
Gentemann, C. L., Fewings, M. R., and García-Reyes, M. (2017). Satellite sea surface temperatures along the West Coast of the United States during the 2014–2016 northeast Pacific marine heat wave. Geophys. Res. Lett. 44, 312–319. doi: 10.1002/2016gl071039
Graham, M. H. (2004). Effects of local deforestation on the diversity and structure of southern California giant kelp forest food webs. Ecosystems 7, 341–357.
Graham, M. H., Vasquez, J. A., and Buschmann, A. H. (2007). Global ecology of the giant kelp macrocystis: from ecotypes to ecosystems. Oceanogr. Mar. Biol. 45, 39–88. doi: 10.1201/9781420050943.ch2
Harley, C. D., Anderson, K. M., Demes, K. W., Jorve, J. P., Kordas, R. L., Coyle, T. A., et al. (2012). Effects of climate change on global seaweed communities. J. Phycol. 48, 1064–1078. doi: 10.1111/j.1529-8817.2012.01224.x
Harley, C. D., Randall Hughes, A., Hultgren, K. M., Miner, B. G., Sorte, C. J., Thornber, C. S., et al. (2006). The impacts of climate change in coastal marine systems. J. Phycol. 9, 228–241. doi: 10.1111/j.1461-0248.2005.00871.x
Harrold, C., and Reed, D. C. (1985). Food availability, sea urchin grazing, and kelp forest community structure. Ecology 66, 1160–1169. doi: 10.2307/1939168
Hernández Carmona, G. (1988). Evaluación, Crecimiento y Regeneración de Mantos de MACROCYSTIS Pyrifera en la Costa Occidental de la Península de Baja California, México. Instituto Politécnico Nacional. México: Centro Interdisciplinario de Ciencias Marinas.
Hernandez-Carmona, G., Garcia, O., Robledo, D., and Foster, M. (2000). Restoration techniques for Macrocystis pyrifera (Phaeophyceae) populations at the southern limit of their distribution in Mexico. Botanica Marina 43, 273–284.
Hernandez-Carmona, G., Robledo, D., and Serviere-Zaragoza, E. (2001). Effect of nutrient availability on Macrocystis pyrifera recruitment and survival near its southern limit off Baja California. Botanica Marina 44, 221–229.
Hervé, M. (2018). RVAideMemoire: Testing and Plotting Procedures for Biostatistics. R Package Version 0.9-69–63.
Hewson, I., Button, J. B., Gudenkauf, B. M., Miner, B., Newton, A. L., Gaydos, J. K., et al. (2014). Densovirus associated with sea-star wasting disease and mass mortality. Proc. Natl. Acad. Sci. U. S. A. 111, 17278–17283. doi: 10.1073/pnas.1416625111
Hobday, A. J., Alexander, L. V., Perkins, S. E., Smale, D. A., Straub, S. C., Oliver, E. C., et al. (2016). A hierarchical approach to defining marine heatwaves. Prog. Oceanogr. 141, 227–238. doi: 10.1016/j.pocean.2015.12.014
Hobday, A. J., and Pecl, G. T. (2014). Identification of global marine hotspots: sentinels for change and vanguards for adaptation action. Rev. Fish Biol. 24, 415–425. doi: 10.1007/s11160-013-9326-6
Hoegh-Guldberg, O. (1999). Climate change, coral bleaching and the future of the world’s coral reefs. Mar. Freshwater Res. 50, 839–866.
Horta e Costa, B., Assis, J., Franco, G., Erzini, K., Henriques, M., Gonçalves, E. J., et al. (2014). Tropicalization of fish assemblages in temperate biogeographic transition zones. Mar. Ecol. Prog. Ser. 504, 241–252. doi: 10.3354/meps10749
Hughes, B. B., Beas-Luna, R., Barner, A. K., Brewitt, K., Brumbaugh, D. R., and Cerny-Chipman, E. B. (2017). Long-term studies contribute disproportionately to ecology and policy. BioScience 67, 271–281.
Hughes, T. P., Kerry, J. T., Álvarez-Noriega, M., Álvarez-Romero, J. G., Anderson, K. D., Baird, A. H., et al. (2017). Global warming and recurrent mass bleaching of corals. Nature 543:373. doi: 10.1038/nature21707
Irving, A. D., Connell, S. D., and Elsdon, T. S. (2004). Effects of kelp canopies on bleaching and photosynthetic activity of encrusting coralline algae. J. Exp. Mar. Biol. Ecol. 310, 1–12. doi: 10.1016/j.jembe.2004.03.020
Irving, A. D., Connell, S. D., Johnston, E. L., Pile, A. J., and Gillanders, B. M. (2005). The response of encrusting coralline algae to canopy loss: an independent test of predictions on an Antarctic coast. Mar. Biol. 147, 1075–1083. doi: 10.1007/s00227-005-0007-4
Jacox, M. G., Hazen, E. L., Zaba, K. D., Rudnick, D. L., Edwards, C. A., Moore, A. M., et al. (2016). Impacts of the 2015–2016 El niño on the california current system: early assessment and comparison to past events. Geophys. Res. Lett. 43, 7072–7080. doi: 10.1002/2016gl069716
Johnson, C. R., Banks, S. C., Barrett, N. S., Cazassus, F., Dunstan, P. K., Edgar, G. J., et al. (2011). Climate change cascades: shifts in oceanography, species’ ranges and subtidal marine community dynamics in eastern Tasmania. J. Exp. Mar. Biol. 400, 17–32. doi: 10.1016/j.jembe.2011.02.032
Johnson, M., and Black, R. (2006). Islands increase genetic subdivision and disrupt patterns of connectivity of intertidal snails in a complex archipelago. Evolution 60, 2498–2506. doi: 10.1111/j.0014-3820.2006.tb01885.x
Krumhansl, K. A., Okamoto, D. K., Rassweiler, A., Novak, M., Bolton, J. J., Cavanaugh, K. C., et al. (2016). Global patterns of kelp forest change over the past half-century. Proc. Natl. Acad. Sci. U. S. A. 113, 13785–13790.
Ladah, L. B., Zertuche-González, J. A., and Hernández-Carmona, G. (1999). Giant kelp (Macrocystis pyrifera, Phaeophyceae) recruitment near its southern limit in Baja California after mass disappearance during ENSO 1997–1998. J. Phycol. 35, 1106–1112. doi: 10.1046/j.1529-8817.1999.3561106.x
Lejeusne, C., Chevaldonné, P., Pergent-Martini, C., Boudouresque, C. F., and Pérez, T. (2010). Climate change effects on a miniature ocean: the highly diverse, highly impacted Mediterranean Sea. Trends Ecol. Evol. 25, 250–260. doi: 10.1016/j.tree.2009.10.009
López-de-Lacalle, J. (2019). tsoutliers. Available at: https://cran.r-project.org/web/packages/tsoutliers/vignettes/tsoutliers-intro.pdf (accessed May 25, 2019).
López-Pérez, A., Guendulain-García, S., Granja-Fernández, R., Hernández-Urraca, V., Galván-Rowland, L., Zepeta-Vilchis, R., et al. (2016). Reef community changes associated with the 2009–2010 El Niño in the southern mexican pacific1. Pacific Sci. 70, 175–191.
Lowe, S., Browne, M., Boudjelas, S., and De Poorter, M. (2000). 100 of the World’s Worst Invasive Alien Species: a Selection from the Global Invasive Species Database. Auckland: Invasive Species Specialist Group.
Marba, N., and Duarte, C. M. (2010). Mediterranean warming triggers seagrass (Posidonia oceanica) shoot mortality. Glob. Change Biol. 16, 2366–2375. doi: 10.1111/j.1365-2486.2009.02130.x
Marshall, P., and Baird, A. (2000). Bleaching of corals on the great barrier reef: differential susceptibilities among taxa. Coral Reefs 19, 155–163. doi: 10.1007/s003380000086
Martin, S., and Gattuso, J.-P. (2009). Response of mediterranean coralline algae to ocean acidification and elevated temperature. Glob. Change Biol. 15, 2089–2100. doi: 10.1002/ece3.475
Matson, P. G., and Edwards, M. S. (2007). Effects of ocean temperature on the southern range limits of two understory kelps, Pterygophora californica and Eisenia arborea, at multiple life-stages. Mar. Biol. 151, 1941–1949. doi: 10.1007/s00227-007-0630-3
McCarthy, J. J., Canziani, O. F., Leary, N. A., Dokken, D. J., and White, K. S. (2001). Climate Change 2001: Impacts, Adaptation, and Vulnerability: Contribution of Working Group II to the Third Assessment Report of the Intergovernmental Panel on Climate Change. Cambridge: Cambridge University Press.
McCoy, S. J., and Kamenos, N. A. (2015). Coralline algae (Rhodophyta) in a changing world: integrating ecological, physiological, and geochemical responses to global change. J. Phycol. 51, 6–24. doi: 10.1111/jpy.12262
Miner, C. M., Altstatt, J. M., Raimondi, P. T., and Minchinton, T. E. (2006). Recruitment failure and shifts in community structure following mass mortality limit recovery prospects of black abalone. Mar. Ecol. Prog. Ser. 327, 107–117. doi: 10.3354/meps327107
Montaño-Moctezuma, G., Torres-Moye, G., Aguilar-Rosas, L., and Ramirez-Valdez, A. (2014). Estructura de la Comunidad de Peces, Macroinvertebrados y Macroalgas Asociada a los Bosques de Macrocystis pyrifera de la Región Ensenadense de Baja California. Información Base para una Propuesta de Monitoreo. Informe Final de Proyecto Financiado por Fondo Sectorial de Investigación Ambiental. Información Base para una Propuesta de Monitoreo. Informe Final de Proyecto Financiado por Fondo Sectorial de Investigación Ambiental. La Paz: SEMARNAT-INE-CONACYT.
Morita, T., Kurashima, A., and Maegawa, M., (2003). Temperature requirements for the growth of young sporophytes of Undaria pinnatifida and Undaria undarioides (Laminariales, Phaeophyceae). Phycol. Res. 51, 266–270. doi: 10.1111/j.1440-1835.2003.t01-4-.x
Morse, D. E., Hooker, N., Jensen, L., and Duncan, H. (1979). “Induction of larval abalone settling and metamorphosis by γ-aminobutyric acid and its congeners from crustose red algae: II: applications to cultivation, seed-production and bioassays; principal causes of mortality and interference,” in Proceedings of the World Mariculture Society banner, Hoboken, NJ.
Occhipinti-Ambrogi, A. (2007). Global change and marine communities: alien species and climate change. Mar. Pollut. Bull. 55, 342–352. doi: 10.1016/j.marpolbul.2006.11.014
Oksanen, J., Blanchet, F. G., Kindt, R., Legendre, P., Minchin, P. R., O’hara, R., et al. (2013). Package ‘Vegan’. Community Ecology Package, Version, 2(9).
Oliver, E. C., Benthuysen, J. A., Bindoff, N. L., Hobday, A. J., Holbrook, N. J., Mundy, C. N., et al. (2017). The unprecedented 2015/16 Tasman Sea marine heatwave. Nat. Commun. 8:16101. doi: 10.1038/ncomms16101
Oliver, E. C., Donat, M. G., Burrows, M. T., Moore, P. J., Smale, D. A., Alexander, L. V., et al. (2018). Longer and more frequent marine heatwaves over the past century. Nat. Commun. 9:1324. doi: 10.1038/s41467-018-03732-9
Pecl, G. T., Araújo, M. B., Bell, J. D., Blanchard, J., Bonebrake, T. C., Chen, I.-C., et al. (2017). Biodiversity redistribution under climate change: impacts on ecosystems and human well-being. Science 355:eaai9214. doi: 10.1126/science.aai9214
Pereira, H. M., Leadley, P. W., Proença, V., Alkemade, R., Scharlemann, J. P., Fernandez-Manjarrés, J. F., et al. (2010). Scenarios for global biodiversity in the 21st century. Science 330, 1496–1501. doi: 10.1126/science.1196624
Perez, T., Garrabou, J., Sartoretto, S., Harmelin, J.-G., Francour, P., and Vacelet, J. (2000). Mortalité massive d’invertébrés marins: un événement sans précédent en méditerranée nord-occidentale. Comptes Rendus de l’Académie des Sciences-Series III-Sciences de la Vie 323, 853–865. doi: 10.1016/s0764-4469(00)01237-3
Perkins, S., Alexander, L., and Nairn, J. (2012). Increasing frequency, intensity and duration of observed global heatwaves and warm spells. Geophys. Res. Lett. 39:L20714.
Pinsky, M. L., Eikeset, A. M., McCauley, D. J., Payne, J. L., and Sunday, J. M. (2019). Greater vulnerability to warming of marine versus terrestrial ectotherms. Nature 569:108. doi: 10.1038/s41586-019-1132-4
Przeslawski, R., Ahyong, S., Byrne, M., Woerheide, G., and Hutchings, P. (2008). Beyond corals and fish: the effects of climate change on noncoral benthic invertebrates of tropical reefs. Glob. Change Biol. 14, 2773–2795. doi: 10.1111/j.1365-2486.2008.01693.x
Ramírez-Valdez, A., Aburto-Oropeza, O., Arafeh Dalmau, N., Beas-Luna, R., Caselle, J. E., Castorani, M. C., et al. (2017). “Mexico-california bi-national initiative of kelp forest ecosystems and fisheries,” in Paper Presented at the White Paper for the Environmental Working Group of the UC-Mexico Initiative, California, CA.
Reed, D., Washburn, L., Rassweiler, A., Miller, R., Bell, T., and Harrer, S. (2016). Extreme warming challenges sentinel status of kelp forests as indicators of climate change. Nat. Commun. 7:13757. doi: 10.1038/ncomms13757
Reed, D. C., Ebeling, A. W., Anderson, T. W., and Anghera, M. (1996). Differential reproductive responses to fluctuating resources in two seaweeds with different reproductive strategies. Ecology 77, 300–316. doi: 10.2307/2265679
Reed, D. C., and Foster, M. S. (1984). The effects of canopy shadings on algal recruitment and growth in a giant kelp forest. Ecology 65, 937–948. doi: 10.2307/1938066
Reynolds, R. W., Smith, T. M., Liu, C., Chelton, D. B., Casey, K. S., and Schlax, M. G. (2007). Daily high-resolution-blended analyses for sea surface temperature. J. Clim. 20, 5473–5496. doi: 10.1175/2007jcli1824.1
Roberts, D. (2018). labdsv: Ordination and Multivariate Analysis for Ecology. R Package Version 1.8-0; 2016.
Roberts, D. A., Gardner, M., Church, R., Ustin, S., Scheer, G., and Green, R. (1998). Mapping chaparral in the Santa Monica Mountains using multiple endmember spectral mixture models. Remote Sens. Environ. 65, 267–279. doi: 10.1016/s0034-4257(98)00037-6
Ruthrof, K. X., Breshears, D. D., Fontaine, J. B., Froend, R. H., Matusick, G., Kala, J., et al. (2018). Subcontinental heat wave triggers terrestrial and marine, multi-taxa responses. Sci. Rep. 8:13094. doi: 10.1038/s41598-018-31236-5
Samaniego-Herrera, A., Peralta-García, A., and Aguirre-Muñoz, A. (2007). Vertebrados de las Islas del Pacífico de Baja California. Baja California: Guía de campo.
Sanford, E., Sones, J. L., García-Reyes, M., Goddard, J. H., and Largier, J. L. (2019). Widespread shifts in the coastal biota of northern California during the 2014–2016 marine heatwaves. Sci. Rep. 9:4216.
Schiel, D. R., and Foster, M. S. (2015). The Biology and Ecology of Giant Kelp Forests. Oakland, CA: Univ of California Press.
Schiel, D. R., Steinbeck, J. R., and Foster, M. S. (2004). Ten years of induced ocean warming causes comprehensive changes in marine benthic communities. Ecology 85, 1833–1839. doi: 10.1890/03-3107
Schneider, C. A., Rasband, W. S., and Eliceiri, K. W. (2012). NIH Image to ImageJ: 25 years of image analysis. Nat. Methods 9:671. doi: 10.1038/nmeth.2089
Short, J., Foster, T., Falter, J., Kendrick, G. A., and McCulloch, M. T. (2015). Crustose coralline algal growth, calcification and mortality following a marine heatwave in Western Australia. Cont. Shelf Res. 106, 38–44. doi: 10.1016/j.csr.2015.07.003
Smale, D. A., and Wernberg, T. (2013). Extreme climatic event drives range contraction of a habitat-forming species. Proc. R. Soc. B Biol. Sci. 280:20122829. doi: 10.1098/rspb.2012.2829
Smale, D. A., Wernberg, T., Oliver, E. C., Thomsen, M., Harvey, B. P., Straub, S. C., et al. (2019). Marine heatwaves threaten global biodiversity and the provision of ecosystem services. Nat. Clim. Change 9, 306–312. doi: 10.1038/s41558-019-0412-1
Smale, D. A., Wernberg, T., and Vanderklift, M. A. (2017). Regional-scale variability in the response of benthic macroinvertebrate assemblages to a marine heatwave. Mar. Ecol. Prog. Ser. 568, 17–30. doi: 10.3354/meps12080
Solomon, S., Qin, D., Manning, M., Averyt, K., and Marquis, M. (2007). Climate Change 2007-the Physical Science Basis: Working Group I Contribution To The Fourth Assessment Report of the IPCC. Cambridge: Cambridge university press.
South, P. M., Floerl, O., Forrest, B. M., and Thomsen, M. S. (2017). A review of three decades of research on the invasive kelp Undaria pinnatifida in australasia: an assessment of its success, impacts and status as one of the world’s worst invaders. Mar. Environ. Res. 131, 243–257. doi: 10.1016/j.marenvres.2017.09.015
Steneck, R. S., Graham, M. H., Bourque, B. J., Corbett, D., Erlandson, J. M., Estes, J. A., et al. (2002). Kelp forest ecosystems: biodiversity, stability, resilience and future. Environ. Conserv. 29, 436–459. doi: 10.1017/s0376892902000322
Sydeman, W. J., Santora, J. A., Thompson, S. A., Marinovic, B., and Lorenzo, E. D. (2013). Increasing variance in North Pacific climate relates to unprecedented ecosystem variability off California. Glob. Change Biol. 19, 1662–1675. doi: 10.1111/gcb.12165
Tegner, M., and Dayton, P. (1991). Sea urchins, El Ninos, and the long term stability of southern california kelp forest communities. Mar. Ecol. Prog. Ser. 77, 49–63. doi: 10.3354/meps077049
Tegner, M., Dayton, P., Edwards, P., and Riser, K. (1997). Large-scale, low-frequency oceanographic effects on kelp forest succession: a tale of two cohorts. Mar. Ecol. Prog. Ser. 146, 117–134. doi: 10.3354/meps146117
Tegner, M., Dayton, P. K., Edwards, P., and Riser, K. L. (1996). Is There Evidence for Long-term Climatic Change in Southern California Kelp Forests?. Berkeley, CA: California Univ.
Tegner, M. J., and Dayton, P. K. (1987). El Nino effects on southern California Kelp Forest Communities. In Advances in Ecological Research. Amsterdam: Elsevier.
Thomas, C. D., Cameron, A., Green, R. E., Bakkenes, M., Beaumont, L. J., Collingham, Y. C., et al. (2004). Extinction risk from climate change. Nature 427:145.
Thomsen, M. S., Mondardini, L., Alestra, T., Gerrity, S., Tait, L., South, P., et al. (2019). Local extinction of bull kelp (Durvillaea spp.) due to a marine heatwave. Front. Mar. Sci. 6:84. doi: 10.3389/fmars.2019.00084
Thomsen, M. S., and South, P. M. (2019). Communities and attachment networks associated with primary, secondary and alternative foundation species; a case study of stressed and disturbed stands of southern bull kelp. Diversity 11:56. doi: 10.3390/d11040056
Torres-Moye, G., Edwards, M. S., and Montaño-Moctezuma, C. G. (2013). Benthic community structure in kelp forests from the Southern California Bight. Ciencias Marinas 39, 239–252. doi: 10.7773/cm.v39i3.2250
Tuckett, C., de Bettignies, T., Fromont, J., and Wernberg, T. (2017). Expansion of corals on temperate reefs: direct and indirect effects of marine heatwaves. Coral Reefs 36, 947–956. doi: 10.1007/s00338-017-1586-5
Valentine, J. P., and Johnson, C. R. (2003). Establishment of the introduced kelp Undaria pinnatifida in Tasmania depends on disturbance to native algal assemblages. J. Exp. Mar. Biol. Ecol. 295, 63–90. doi: 10.1016/s0022-0981(03)00272-7
Valentine, J. P., and Johnson, C. R. (2004). Establishment of the introduced kelp Undaria pinnatifida following dieback of the native macroalga Phyllospora comosa in Tasmania. Australia. Mar. Freshwater Res. 55, 223–230.
Vergés, A., Steinberg, P. D., Hay, M. E., Poore, A. G., Campbell, A. H., Ballesteros, E., et al. (2014). The tropicalization of temperate marine ecosystems: climate-mediated changes in herbivory and community phase shifts. Proc. R. Soc. B Biol. Sci. 281:20140846. doi: 10.1098/rspb.2014.0846
Voerman, S. E., Llera, E., and Rico, J. M. (2013). Climate driven changes in subtidal kelp forest communities in NW Spain. Mar. Environ. Res. 90, 119–127. doi: 10.1016/j.marenvres.2013.06.006
Wernberg, T., Bennett, S., Babcock, R. C., De Bettignies, T., Cure, K., Depczynski, M., et al. (2016). Climate-driven regime shift of a temperate marine ecosystem. Science 353, 169–172. doi: 10.1126/science.aad8745
Wernberg, T., Krumhansl, K., Filbee-Dexter, K., and Pedersen, M. F. (2019). Status and Trends for the World’s Kelp Forests. In World Seas: An Environmental Evaluation. Amsterdam: Elsevier.
Wernberg, T., Smale, D. A., Tuya, F., Thomsen, M. S., Langlois, T. J., De Bettignies, T., et al. (2013). An extreme climatic event alters marine ecosystem structure in a global biodiversity hotspot. Nat. Clim. Change 3:78. doi: 10.1038/nclimate1627
Whitney, F. A. (2015). Anomalous winter winds decrease 2014 transition zone productivity in the NE Pacific. Geophys. Res. Lett. 42, 428–431. doi: 10.1002/2014gl062634
Woodson, C. B., Micheli, F., Boch, C., Al-Najjar, M., Espinoza, A., et al. (2018). Harnessing marine microclimates for climate change adaptation and marine conservation. Conserv. Lett. 12:e12609. doi: 10.1111/conl.12609
Zhao, Z., and Marin, M. (2019). A MATLAB toolbox to detect and analyze marine heatwaves. J. Open Source Softw. 4, 1124. doi: 10.21105/joss.01124
Zimmerman, R. C., and Kremer, J. N. (1984). Episodic nutrient supply to a kelp forest ecosystem in Southern California. J. Mar. Res. 42, 591–604. doi: 10.1357/002224084788506031
Keywords: kelp forest, extreme warming event, marine heatwaves, community structure, tropicalization, trailing edge
Citation: Arafeh-Dalmau N, Montaño-Moctezuma G, Martínez JA, Beas-Luna R, Schoeman DS and Torres-Moye G (2019) Extreme Marine Heatwaves Alter Kelp Forest Community Near Its Equatorward Distribution Limit. Front. Mar. Sci. 6:499. doi: 10.3389/fmars.2019.00499
Received: 20 April 2019; Accepted: 25 July 2019;
Published: 23 August 2019.
Edited by:
Thomas Wernberg, The University of Western Australia, AustraliaReviewed by:
Dan Alexander Smale, Marine Biological Association of the United Kingdom, United KingdomAlex Sen Gupta, University of New South Wales, Australia
Mads Solgaard Thomsen, University of Canterbury, New Zealand
Copyright © 2019 Arafeh-Dalmau, Montaño-Moctezuma, Martínez, Beas-Luna, Schoeman and Torres-Moye. This is an open-access article distributed under the terms of the Creative Commons Attribution License (CC BY). The use, distribution or reproduction in other forums is permitted, provided the original author(s) and the copyright owner(s) are credited and that the original publication in this journal is cited, in accordance with accepted academic practice. No use, distribution or reproduction is permitted which does not comply with these terms.
*Correspondence: Nur Arafeh-Dalmau, bi5hcmFmZWhkYWxtYXVAdXEubmV0LmF1; Gabriela Montaño-Moctezuma, Z21vbnRhbm9AdWFiYy5lZHUubXg=