- 1School of Biological and Marine Sciences, University of Plymouth, Plymouth, United Kingdom
- 2School of Computing Sciences, University of East Anglia, Norwich, United Kingdom
- 3School of Biological Sciences, University of Western Australia, Perth, WA, Australia
- 4The Marine Biological Association, The Laboratory, Citadel Hill, Plymouth, United Kingdom
Within atolls, deep water channels exert significant control over local hydrodynamic conditions; which are important drivers of planktonic distributions. To examine planktonic responses to oceanography, this study tested the effect of proximity and exposure to deep oceanic flushing through these channels on water properties and planktonic assemblages across four atolls (Diego Garcia, Salomon, Egmont, and Peros Banhos) in the British Indian Ocean Territory Marine Reserve. As this is the largest, most isolated and sparsely inhabited atoll complex in the world, it provides the perfect experimental conditions to test the effect of oceanic flushing without confounding factors related to anthropogenic development. Results are discussed in the context of ecosystem functioning. A total of 30 planktonic taxa and 19,539 individuals were identified and counted. Abundance was significantly different between atolls and significantly greater within inner regions in all atolls except southeast Egmont. Planktonic assemblage composition significantly differed between atolls and between inner and outer stations; exhibiting higher similarity between outer stations. Within outer stations of Diego Garcia, Peros Banhos, and Egmont, evidence suggesting oceanic flushing of cold, saline, and dense water was observed, however a longer time series is required to conclusively demonstrate tidal forcing of this water through deep water channels. Planktonic variability between inner and outer atoll regions demonstrates that broad comparisons between oceanic and lagoon regions fail to capture the complex spatial dynamics and hydrodynamic interactions within atolls. Better comprehension of these distributional patterns is imperative to monitor ecosystem health and functioning, particularly due to increasing global anthropogenic pressures related to climate change. The extensive coral bleaching described in this paper highlights this concern.
Introduction
Coral atolls are often characterized by a rich biodiversity of fishes, corals, and coralline algae, highly mobile consumers such as manta rays (Mccauley et al., 2014) and sea turtles (Mendonca and Ehrhart, 1982), and apex predators (Sheppard et al., 2012) such as reef sharks (Tickler et al., 2017). The greater productivity within atolls relative to the surrounding oligotrophic tropical waters (Rayner and Drew, 1984; Letessier et al., 2016) likely supports such diverse assemblages. As such they have been claimed to be “oases of life in an oceanic desert” (Odum and Odum, 1955; Johannes and Gerber, 1974).
Such atoll productivity may be maintained by high nutrient recycling, through microbial food webs and coral symbionts (Hatcher and Frith, 1985; Falter et al., 2004), and geomorphology. Geomorphological features such as deep water channels are thought to affect productivity within atolls, by facilitating oceanic exchange between lagoon and oceanic waters; controlled by the tidal forcing (Pugh and Rayner, 1981; Kench, 1998; Chevalier et al., 2017; Green et al., 2018). Without this oceanic exchange, temperature, salinity, and oxygen may reach extreme, uninhabitable levels (Pugh and Rayner, 1981). Thus, the nutrient enrichment (Thompson and Golding, 1981; Thomson and Wolanski, 1984; Wolanski et al., 1988) and environmental stability exerted by these deep water channels through water renewal likely plays a key role in ecosystem functioning.
Investigating the role of atoll hydrodynamics on natural ecosystem functioning is almost always confounded by anthropogenic influences such as nutrient input through urban run-off, overfishing, sedimentation, coastal development, pollution, and tourism (Knowlton and Jackson, 2008; Riegl et al., 2012; Firth et al., 2016). However, atolls within the Chagos Archipelago, Indian Ocean, represent a unique opportunity to investigate these relationships in the absence of direct human pressures. The Chagos Archipelago is a large area of atolls and submerged banks within the British Indian Ocean Territory (BIOT), situated in the southern region of the Lakshadweep-Maldives-Chagos ridge (Sheppard et al., 2012). Established within BIOT is a designated no-take marine reserve of approximately 550,000 km2. The marine reserve includes all of the atolls and waters of the BIOT Exclusive Economic Zone (EEZ), with the exception of the atoll of Diego Garcia (Sheppard et al., 2012). There are no human inhabitants within the archipelago, save for those associated with the military base on Diego Garcia. As such, the BIOT marine reserve is the largest, most isolated uninhabited atoll complex in the world.
Plankton are extremely important to the functioning of atoll lagoon systems. They are key to conserving biodiversity and productivity (Bozec et al., 2004; Alldredge and King, 2009) as they play a vital role in food webs, linking primary producers to multiple trophic levels e.g., reef fishes, manta rays, and reef sharks (Niquil et al., 1998; Alldredge and King, 2009; Tickler et al., 2017), participate in biogeochemical cycles (Roman et al., 1990; Legendre and Le Fèvre, 1991) and their abundance is known to influence pelagic and benthic faunal recruitment (Hughes et al., 2000; Grorud-Colvert and Sponaugle, 2009). Given their importance to ecosystem functioning (Hébert et al., 2017), investigating the spatial distribution, abundance, and diversity of plankton is vital for the monitoring of marine biodiversity and ecosystem health.
Within planktonic assemblages, zooplankton comprise taxa which reside in the plankton for early larval stages (meroplankton) and taxa which are planktonic for their entire life cycle (holoplankton) (Kennish, 2001). There are also small, often benthic animals translocated into the water column by hydrographic and behavioral processes (tychoplankton) (Kennish, 2001). Typically the abundance of zooplankton is greater within lagoons, compared to adjacent oceanic areas (Gerber, 1981; Pagano et al., 2012) and is dominated by meroplankton (Gerber, 1981; Achuthankutty et al., 1989; Pagano et al., 2017). This pattern highlights the importance of atolls as “nursery grounds” for the larval planktonic stages of tropical invertebrates and fishes.
A number of oceanographic processes are known to influence the distribution and community structure of zooplankton throughout the open ocean such as ocean fronts, mesoscale eddies, and upwelling (Kiørboe, 1997; Huggett, 2014; Lamont et al., 2014). Within lagoons, however, hydrodynamic drivers including tidal flushing and wind-driven circulation, and the associated variations in salinity and temperature, are highly influential (Dumas et al., 2012; Dupuy et al., 2016). Additionally, particulate organic matter and chlorophyll (Le Borgne et al., 1989; Carassou et al., 2010), increased terrestrial runoff and nutrient input (Carrillo Baltodano and Morales Ramírez, 2016; Dupuy et al., 2016) and zooplankton swimming behavior within tidal-currents may also affect the retention/aggregation of zooplankton in lagoons (Genin et al., 2005). Cold oceanic water spilling into atolls from deep channels may underpin zooplankton spatial and temporal distribution within the lagoons.
This study compared zooplankton metrics (number of taxa, abundance, and assemblage composition) between inner more sheltered regions with outer more exposed regions. To examine the hypothesis that deep oceanic water is flushed into the atoll, differences in water properties were compared between inner and outer regions. Four atolls in the BIOT were surveyed; Salomon, Egmont and Peros Banhos in the marine reserve, and Diego Garcia.
Materials and Methods
To enable comparison of these data between atolls and to other comparable locations, site characterization was first undertaken. Intertidal and subtidal samples were taken using cores and towed video, respectively (Figure 1). Plankton samples and CTD vertical profiles were collected concurrently following a transect, to capture the different regions of exposure in four coral reef atolls in the BIOT (Diego Garcia, Salomon, Egmont, and Peros Banhos), in the central Indian Ocean between 04°54′ to 07°39′S and 70°14′ to 72°37′E (Tickler et al., 2017; Figure 1). Each transect started in the most sheltered part of the atoll and ended at the exposed lagoon entrance (weather depending). Sampling took place from a 4 m skiff during daylight hours between 11th and 22nd May 2016. Permission was granted to undertake the work by the United Kingdom Foreign and Commonwealth Office.
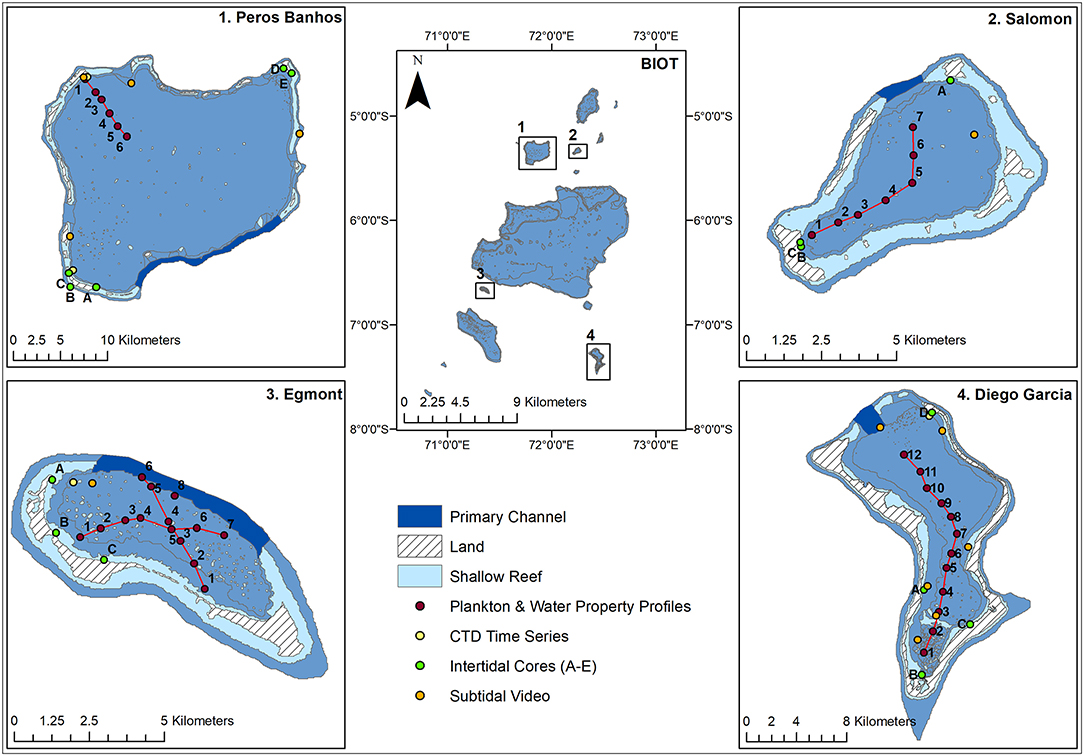
Figure 1. Map of BIOT with sampled atolls and their primary channels. Transects of plankton and water property profiles are shown, alongside CTD time series, intertidal cores, and subtidal video.
Site Characterization
Within each atoll, 3–5 intertidal sites and 1–5 subtidal sites were haphazardly selected to gain optimal coverage of each atoll, time allowing (Figure 1). To assess the intertidal sediment size, organic content and infauna, 3 replicates were sampled at the waterline of each site. Each replicate gathered a sediment sample using a small plastic corer (50 mm L × 50 mm D) to assess particle size and organic content, and an infauna sample using a bespoke stainless steel corer (250 mm L, 100 mm D) (Sheehan et al., 2015; Supplementary Figure 1). Sediment processing was conducted using a standard approach as described in Sheehan et al. (2010). Sediments from the large corer were sieved over a 1 mm mesh sieve. The samples did not contain any living fauna, bar 5 individuals of a featureless species of polychaete, hence no infauna data are presented. To assess habitat type and coral health, 3 × video transects of the subtidal sea bed at each site were recorded. Transects were recorded for 5 min and towed at <0.2 knots using a bespoke, non-contact, towed array, fitted with a Go Pro 4 silver and laser scaling (Supplementary Figure 1) for 3 × 5 min tows at each subtidal site (Figure 1). To analyse sub-tidal video transect recordings, each transect was split into frames at 3 s intervals. Poor quality and overlapping frames were removed, leaving high quality frames for analysis (Sheehan et al., 2013). The dominant habitat type and its bleaching status for each frame was recorded (Corals: Bommies, Rubble, Branching, and Massive; Sediment: Sand or Mounds in sand). In addition, any live corals (hard or soft), encrusting coralline algal/hydroid turf that colonizes dead coral, or reef fish were noted. Percent occurrence of dominant habitat and percent presence of the other variables were calculated for each replicate tow.
Plankton and Water Property Profiles
Along each transect, samples were taken at discrete stations separated 1 nautical mile apart for the larger atolls (Diego Garcia and Peros Banhos) and 0.5 nautical miles apart for the smaller atolls (Egmont and Salomon) (Figure 1). The position of each station was logged using a GPS Garmin Etrex 20x. At each station, a seabed-surface vertical sample was obtained by a plankton net (250 mm frame with a 250 μm mesh) and a Maestro CTD (RBR Maestro conductivity-temperature-depth sensor equipped with a Rinko dissolved oxygen sensor and a Seapoint Chlorophyll-α fluorometer, sampling at 12 Hz) (Supplementary Figure 1). Salinity data are henceforth expressed in practical salinity units (PSU). Each transect was completed between 30 and 70 min, minimizing the influence of non-steady dynamics, likely attributable to tides, on the results. From the density values derived from the Maestro CTD, the Brunt-Vaisala frequency, N;
where g = 9.81 m s−2 is the acceleration due to gravity, ρo is a reference density and z is the vertical distance, was calculated for 1 m vertical increments. Data were subsequently bin-averaged at 1 m vertical resolution in post-processing.
In addition to the vertical profiles, Valeport mini-CTDs were deployed at a depth of approximately 4 m and 2 m above the bed at the entrances to each lagoon, except Salomon in which the CTD malfunctioned (Figure 1). The mini-CTDs sampled at 1 Hz and were deployed at each site for a complete semidiurnal tidal cycle, with the intention of observing temporal changes in the water properties entering and leaving the lagoon. The deployments were coincident with the Maestro CTD vertical profile transects, enabling an assessment to be made of the temporal variability that may influence the interpretation of the spatial variability depicted by the Maestro CTD data. Due to entrapment of particles in the conductivity cell of the mini CTDs, the salinity data were unreliable, therefore only the temperature data from these instruments could be reliably used to indicate the susceptibility of a given site to cold water flushing from the open ocean.
Plankton samples were stored in 100% ethanol and later filtered through a 200 μm mesh and identified using light microscopy. Taxon were identified as described by Conway et al. (2003) to the highest level of taxonomic resolution as was feasible. For copepod abundance specifically, individuals were distinguished first by order and then a size fraction (small = < 2 mm, large = >2 mm). Identification was then conducted within these fractions.
Identifications were made using stereo microscopes: Leica MZ6 (magnification ×9.45–×60); Zeiss Discovery. V8 (magnification ×6.3–×120); and a bespoke compound microscope (magnification ×62.5–×625). For each microscope, size distinctions were made using an eyepiece graticule calibrated to a stage micrometer. Identification and enumeration was completed on 39 samples. Samples were counted in their entirety, excluding a singular occasion in which the abundance of gastropod larvae surpassed 5,500. In this sample (southwest Egmont Station 2, Figure 1), the count for this taxon was based on extrapolation of abundance within half the sample.
Data Analysis
Site Characterization
Site characterization data were tabulated but not analyzed as there were no a priori hypotheses to test.
Plankton and Water Property Profiles
Transects comprised evenly dispersed stations and ran from the most sheltered part of each atoll toward the primary channel where it was most exposed. To statistically compare plankton assemblages between inner sheltered and outer exposed regions, stations in the first half of each transect were considered “inner” and the remaining stations considered “outer” unless water property data provided a more informed division between categories (Figures 2, 3, Supplementary Figures 2–5). It was anticipated that deep oceanic flushing would be characterized by vertical isopycnals indicating lateral gradients in water properties as oceanic water entered the lagoon. Inner unflushed lagoons instead would demonstrate a degree of vertical stratification, as the absence of flushing by oceanic water enables persistent diurnal warming to enhance the near surface thermocline. As these data were limited to single time points, flushing extent could be underestimated. To further extrapolate these data, this study would need to be replicated to account for tidal, daily, seasonal, and annual variability.
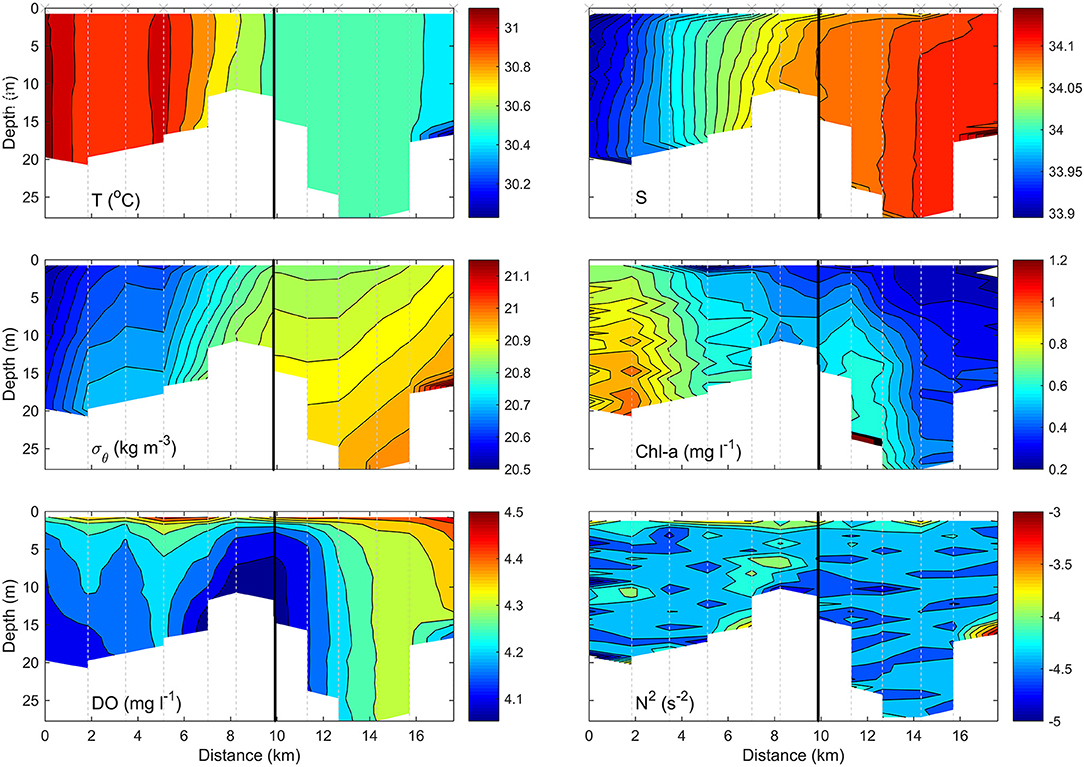
Figure 2. Example CTD transect, of Diego Garcia, indicating changes in temperature (T), salinity (S), density (σθ), chlorophyll-α (chl-α), dissolved oxygen saturation (DO), and the Brunt-Vaisala frequency squared (N2) with depth (m) and horizontal position (km). Black line indicates exposure boundaries, left (inner) and right (outer).
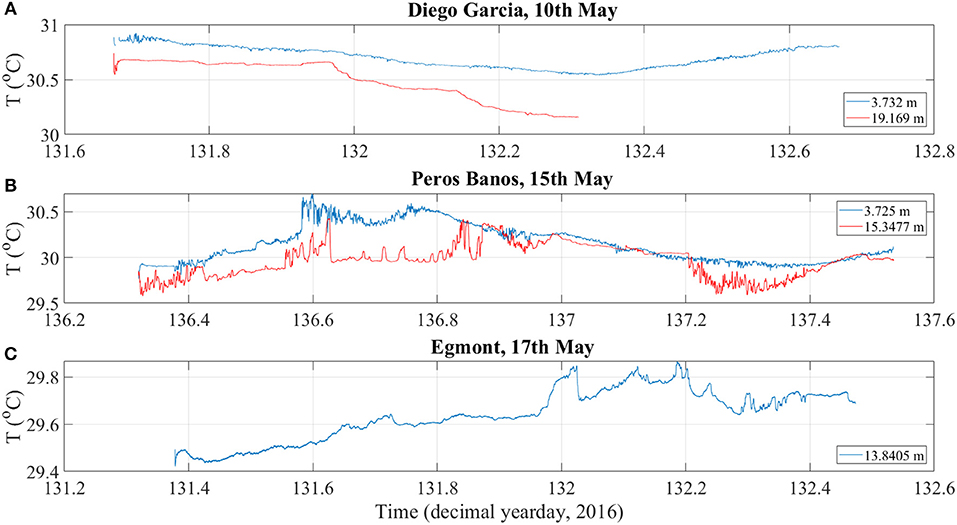
Figure 3. Temperature time series from Valeport miniCTDs deployed at the entrance to (A) Diego Garcia, (B) Peros Banhos, and (C) Egmont lagoons. With the exception of Egmont for which only the deep CTD was recovered, CTDs were located at a depth of approximately 4 m and a height above the bed of 2 m. For each instrument the mean depth throughout the record is indicated.
Permutational multivariate analysis of variance in Primer 7 (Anderson, 2001; Clarke and Warwick, 2001) was used to test for differences between Atolls (Fixed, 5 levels: Diego Garcia, Salomon, southwest Egmont, southeast Egmont, and Peros Banhos) and Exposure (Fixed, 2 levels: Inner and Outer) using two univariate metrics (number of taxa and abundance) and one multivariate metric (assemblage composition). All data were fourth root transformed and univariate data were based on Euclidean distance, while multivariate data were based on the Bray–Curtis similarity index (Bray and Curtis, 1957). Non-metric multi-dimensional Scaling (nMDS) was used to visualize differences between factors, while SIMPER routine (Clarke and Warwick, 2001) was used to interpret trends, which drove observed differences in assemblage composition.
Results
Atoll Habitat
The intertidal sediments in all four atolls comprised medium to very coarse sand (Folk and Ward, 1957) that had very poor to medium sorting, similar levels of low organic content and almost no infauna present (Supplementary Table 1).
The subtidal habitats were more variable (Supplementary Table 2). The habitat in Diego Garcia was dominated by a matrix of sandy mounds created by benthic organisms, covered in trails made by gastropods and hermit crabs (40%), areas of bleached Bommies (22.8%) on sand and coral rubble (26.3%). There was very little occurrence of live coral (hard = 6.9%; soft = 0.4%). While some corals appeared to be recently bleached, others had been bleached for some time and were covered in red algae, green algae, and other encrusting organisms (8.9%). The breaking down of coral structure was evident throughout, with giant clams falling out of decomposing coral structure. Reef fishes still populated areas of bleached coral and were seen in 13.7% of the frames.
Across the four atolls, Salomon was the only place where live coral was recorded as a dominant habitat (43%). There was still bleached branching corals (9.1%) and bleached massive corals (13.2%) between areas of sand (10.5%) and mounds in sand (22.7%). Here only 13.5% of frames contained colonizing crust on the bleached coral and 43.2% of frames were populated with reef fishes.
Egmont was characterized by mostly bleached massive corals (64%), bleached branching corals (36%), and turf algae (32%). Reef fishes occurred in 68% of the frames. Although extensively bleached, there were still some live hard (3%) and soft corals present (4.4%).
In Peros Banhos, the south west site had steep slopes of degrading corals down to flatter sandy areas, especially table corals that had broken and were littered upside down across the slopes. The rest of the sites were fairly homogenous flat areas, characterized mostly by bleached massive coral (84.9%). There were examples of recently bleached branching corals with symbiotic porcelain crabs still present next to those that had bleached some time ago, evidenced by the encrusting pink algae (89.4%). Live hard (3%) and soft corals (4.4%) were observed across the massive dead hard coral reef. Despite the dead coral habitat, 76% of the frames were populated with reef fishes and sharks were also recorded. Subtidal habitat examples are shown in Figure 4.
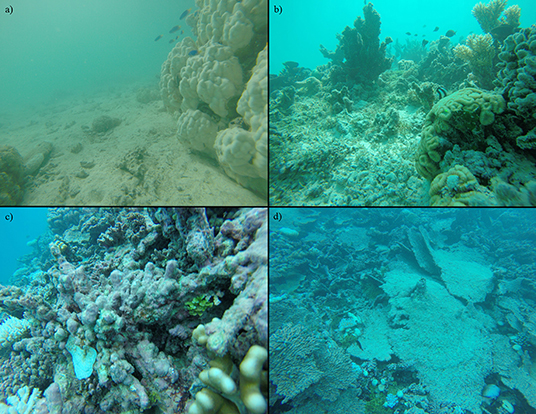
Figure 4. Images of the dominant habitat types (a) Bleached coral Bommie on sand in Diego Garcia (b) Examples of living coral in Salomon (c) Bleached coral encrusted with coralline algae in Peros Banhos (d) Broken bleached table coral in Peros Banhos.
Atoll Oceanography
The CTD time series data (Figure 3) was consistent with the hypothesis that these atolls are subject to intermittent inundations at depth of cold, oceanic water. CTD time series, presented as t, decimal year day for 2016, in particular demonstrated the extent to which the tidal currents may be flushing the lagoons with water of oceanic origin, thereby providing a potential source of nutrients. However, a longer time series is needed to conclusively demonstrate this.
CTD data from the time series moorings and the depth profile transects (Figure 2, Supplementary Figures 2–5) demonstrate that the region is strongly stratified, potentially from nonlinear internal waves of depression that are supported by near-surface stratification and manifest themselves as short period elevations in temperature (Hosegood et al., 2019). These effects may promote shear-induced mixing and the downward diffusion of heat accumulated in the surface layers during daytime.
Diego Garcia
The transect within Diego Garcia (Figure 2) began at the far southern extent of the lagoon in 10.68 m water depth, where the water is sheltered from the effects of the open sea and whose only entrance into the lagoon is ~20 km to the north, where the maximum depth recorded was 28.79 m. As such, there is no possibility of water entering the lagoon by any other means, such as over a surrounding reef. Temperature (T) was vertically homogenous throughout the lagoon and implies efficient vertical mixing throughout the water column. A lateral gradient was apparent as temperature decreased from ~31.05°C in the inner southern extent of the lagoon to <30.4°C toward the northern entrance. Similarly, salinity (S) was highest in the inner stations and reached 34.1, diminishing in an approximately linear manner horizontally toward outer stations, where S ~ 33.9. It should be noted that the apparent vertical homogeneity in T and S and simultaneous pronounced stratification in the density field is a relic of the color scale bar. Density only ranges from 20.83 to 20.99, a difference of 0.16, and a similarly subtle temperate and salinity gradient also exists but is not expressed in Figure 2. Density alternates between regions of stable but weaker stratification, in which N2 ~ 10−4.5 s−2, and regions in which isopycnals exhibited a significant inclination to the vertical, within which N2 > 10−4 s−2. The regions of maximum stratification coincided with parts of the lagoon in which dissolved oxygen (DO) was minimum, specifically the central part of the lagoon in which the channel narrows, and the southern extent of the lagoon where S was lowest. This suggests terrestrial input of freshwater, given the temperatures were highest.
Notably, there was evidence of a cold intrusion of deeper water entering the outer stations of the lagoon; the cold, saline, and therefore dense water was accompanied by decreased DO but had a negligible signature in chlorophyll-α (chl-α), which varied only in the horizontal. Distinctly higher concentration of chl-α >1 mg l−1, was observed at the enclosed inner end of the lagoon and dropped to background levels of <0.3 mg l−1 toward the extent of the outer stations.
CTD time series showed evidence of colder water entering the lagoon near the bed (Figure 3). Whilst a semidiurnal variation in temperature was apparent near the surface, where temperature decreased from 30.83°C at t = 131.7 to 30.55°C ~6.5 h later, before recovering back to initial values. The temperature at a depth of 19 m decreased more abruptly at t = 131.95 and 132.125, reaching 30.2°C from an initial temperature of 30.6°C. The accelerated decrease in temperature at these points suggests pulses of cooler water were advected into the lagoon and is consistent with the near-bed intrusion of cold water observed during the Maestro CTD transect.
Salomon
Despite the similar water depth range recorded within Salomon (11.62–28.72 m) compared to Diego Garcia (10.68–28.78 m), the vertical structure in Salomon was markedly different (Supplementary Figure 2). Pronounced and persistent stratification was evident across the entire transect. In the inner eastern portion of the transect, temperatures were lowest, T < 29.4°C, and increased toward the center the lagoon where a pronounced maximum of T > 29.8°C occurred in the surface layer of 5 m depth. The S did not replicate the vertical structure, indicating a lateral gradient that was strongest, O (0.05 km−1), toward the western inner portion of the transect, but decreased such that the S in the central lagoon was approximately homogenous with S ~ 34.3 compared to 34.5 in the west. In the center of the lagoon, closest to the entrance in the north, there was no evidence of deep, cold water exchange with the open ocean.
Similarly to Diego Garcia, DO was minimum where the stratification was strongest. In this case toward the beginning of the transect, where N2 >10−3.5 s−2, and DO <4.4 mg l−1. Stratification was elevated in a near surface layer, in the outer region, that is consistent with insolation effects, i.e., the generation of a diurnal thermocline, but also near the bed in a layer of 5 m thickness, that deepens from 10 m depth at the outer extent of the transect to 20 m toward the center of the lagoon. Chlorophyll-α was low, at <0.3 mg l−1, in the inner portion of the lagoon but increased substantially to >1 mg l−1 near the bed in the center of the lagoon. This is directly beneath a localized near surface region of elevated DO, but adjacent to the near bed minimum in DO.
Egmont
The CTD transect starting in the southeast of Egmont had a depth range of 4.99–21.57 m (Supplementary Figure 3) and exhibited highest salinity, S ~ 34.26, near to the land (furthest from the entrance). The transect beginning in the southwest (9.15–24.34 m depth range) (Supplementary Figure 4) indicated minimum salinities in the innermost stations, S ~ 34.18, increasing vertically downwards to 34.28 at the bed toward the center of the lagoon but with little lateral variability. As S increased with depth, so did chl-α to a maximum concentration of 0.7 mg l−1 at 15 m depth. In comparison, chl-α in the southwest transect reached 1.2 mg l−1 in inner stations. S and chl-α appear to therefore be positively correlated, with higher S suggesting higher chl-α concentrations. As T did not necessarily follow S (Figure 3), due to insolation effects that vary on short timescales of hours, the chl-α appears to be related to the incursion of oceanic water from outside the atolls.
In outer Egmont, temperature exhibits a general increasing trend during deployment, with occasional, abrupt decreases in temperature, demonstrated by the CTD time series, suggest the intrusion of colder water into the outer lagoon as pulses, such as at t = 132.02 (Figure 3).
Peros Banhos
The maximum water depth in Peros Banhos was not reached by the plankton net or the maestro CTD as there were concerns that the kit may become entangled and lost. The maximum water depth reached in Peros Banhos was 7.09–37.95 m. The Peros Banhos transect began at the northern edge of the lagoon near to an open channel that was not the primary channel. It was the most sheltered part of this lagoon, however penetration of cold, saline water through this channel into the lagoon was detected (Supplementary Figure 5). Temperature <29.2°C and S > 34.5 at depths of >30 m in the inner extent of the transect were markedly different from the overlying water properties. Near surface stratification (N2 > 10−3.5 s−2) was consistent with diurnal heating, particularly given the timing of the transect during the afternoon, but the high density of the near-bed intrusion also generated a strong density interface at a depth of 30 m, across which N2 ~ 10−3 s−2. The water of apparent oceanic origin exhibited high levels of chl-α, >1.5 mg l−1, and low DO ~ 4.0 mg l−1. The concentrations of chl-α in the near-bed layer were the highest observed within all of the lagoons. The persistent stratification throughout the water column, but especially at the top of the near-bed chlorophyll-rich layer, would have prevented the diffusion of chl-α vertically to the overlying water.
CTD time series (Figure 3) demonstrated high frequency fluctuations in temperature throughout the tidal cycle. A pronounced decrease in temperature at t = 137.21, contribute to our hypothesis that the lagoons we studied may be subject to intermittent inundations at depth of cold, oceanic water. There is a lack of clear tidal periodicity associated with these temperature time series, although we note that a weak tidal signal may be obscured by lower frequency variability over a given 12 h period. Longer time series should be undertaken to evidence tidal influence.
Number of Taxa and Abundance
The plankton samples contained 30 taxa (holoplankton = 17 & meroplankton = 13) and 19,539 individuals (Figure 5, Supplementary Table 3). Frequently observed holoplankton included crustaceans such as calanoid copepods >2 mm (Figure 5A), chordates such as appendicularians (Figure 5B), radiolarians such as acantharians, chaetognaths (Figure 5C), and calanoid copepods <2 mm (Figure 5D). Common meroplankton included crustaceans such as decapod larvae (Figure 5E) and molluscs such as gastropod larvae (Figure 5F). Fairly large fish larvae (~4 mm) were also encountered (Figure 5G) and annelids such as polychaete larvae (Figure 5H).
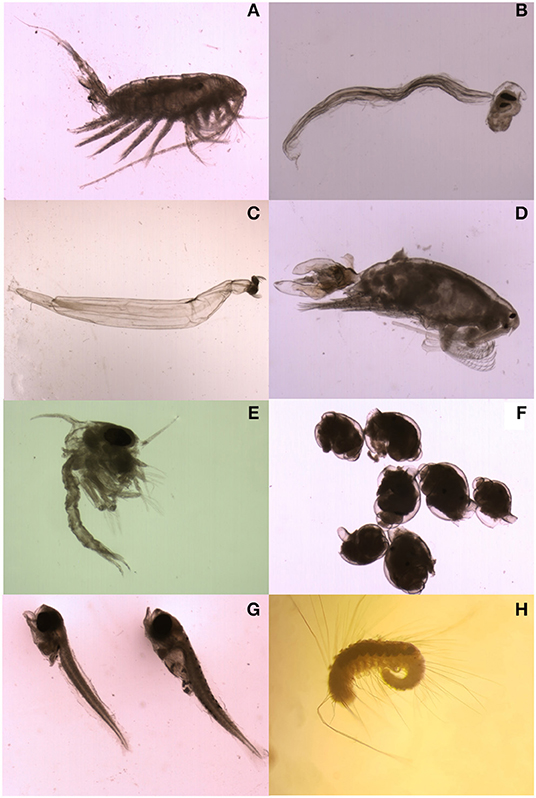
Figure 5. Images of zooplankton of interest to this study, holoplankton (A) Calanoid Copepod <2 mm (Centropages spp. 1.55 mm length) (B) Appendicularian (2.07 mm) (C) Chaetognath (6.88 mm) (D) Calanoid copepods >2 mm (Labidocera stylifera, 2.88 mm) and meroplankton (E) Decapod larvae (~2 mm) (F) Gastropod larvae (~1 mm) (G) Fish larvae (~4 mm), (H) Polychaete larvae (~3.5 mm). (A–G) were taken with an Infinity camera mounted to a Zeiss Discovery. V8.
The following data are presented as mean ± SE. Number of taxa were not statistically different between atolls or between inner and outer regions (Supplementary Table 4). However, the abundance was significantly different between atolls and inner (In) and outer (Out) regions (Atoll: P = 0.001, Pseudo-F = 0.87; Exposure: P = 0.01, Pseudo-F = 4.36, Supplementary Table 4). Abundance was consistently higher within inner regions across all atolls, excluding southeast Egmont (Figure 6). The greatest abundance occurred within inner stations in southwest Egmont (In = 2636 ± 1434.08, Out = 185.50 ± 26.80, Supplementary Table 3). Additionally, southwest Egmont had the largest difference between exposure regions (2450.50 individuals), driven by an extremely high abundance of gastropod larvae within the inner stations. Diego Garcia possessed the next greatest mean abundance (In = 458.57 ± 83.29, Out = 322 ± 60.28), followed by southeast Egmont (In = 150 ± 20.42, Out = 359.33 ± 194.01), Peros Banhos (In = 242.67 ± 49.65, Out = 87 ± 36.67), and Salomon (In = 141.25 ± 45.94, Out = 117 ± 47.62).
Zooplankton Assemblage Structure
The assemblage composition was significantly different between atolls (P = 0.0001, Pseudo-F = 6.39, Supplementary Table 4) and between inner and outer stations (P = 0.005, Pseudo-F = 2.94). The interaction between atoll and exposure was also significantly different (P = 0.0002, Pseudo-F = 2.29). The two atolls in the north, Peros Banhos, and Salomon were most similar to each other, Diego Garcia is the most isolated atoll of the group and was significantly different to the other three atolls, as was Egmont SW, however Egmont SE was similar to assemblages in Peros Banhos inner and outer regions, but only the outer region for Salomon with a significantly different assemblage to the inner region (Supplementary Table 4). Overall, the assemblage compositions within inner stations were more dispersed than those in outer stations (MVDISP: Inner = 1.06, Outer = 0.92, Figure 7).
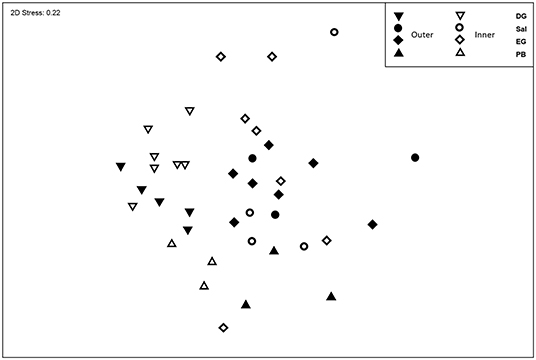
Figure 7. Non-metric Multidimensional Scaling Plot of the assemblage composition of zooplankton, between atolls and regions of Inner and Outer stations.
Of the holoplankton, calanoid copepods <2 mm comprised Acartia spp., Acrocalanus spp., Bestolina spp., Calanidae (Juvenile), Calanopia spp., Calanopia spp. (Juvenile), Calocalanus pavo, Candacia catula, Candacia spp., Candacia spp. (Juvenile), Canthocalanus spp., Centropages furcatus, Centropages orsinii, Centropages spp., Clausocalanus spp., Cosmocalanus darwinii, Labidocera laevidentata, Labidocera spp., Paracalanidae (Juvenile), Paracalanus spp., Tortanus spp. Calanoid copepods >2 mm included Calanopia spp., Labidocera spp., Labidocera stylifera, Labidocera styliferi/koryeri, Nannocalanus spp., Paraeuchaeta spp., Subeucalanus spp., Tortanus spp., Undinula spp. Poecilostomatoid copepods <2 mm included Corycaeus spp., Farranula spp., Oncaeidae. Cyclopoid copepods <2 mm were represented by Oithona spp. The singular Monstrilloid copepod <2 mm was Monstrilla sp.
Calanoid copepods <2 mm were the most dissimilar group between inner and outer regions (3.87 mean dissimilarity ± 1.25). They were most common to inner regions (In = 3.57, Out = 3.05, Figure 8, Supplementary Table 3). This trend was consistent among all atolls, except southeast Egmont which supported a higher abundance at outer stations. Additionally, within Egmont atoll, a calanoid copepod (<2 mm) from the family Tortanidae was observed and is believed to be a species that is not yet described. Calanoid copepods >2 mm were more abundant within outer regions (In = 1.09, Out = 1.22). This was also true for Salomon, southeast Egmont, and Peros Banhos. Within southwest Egmont, they were uniformly abundant, though within Diego Garcia they were present at greater abundance within inner stations. Similarly chaetognaths were relatively dissimilar between regions (3.37 mean dissimilarity ± 1.19) and typically occurred more frequently within outer stations (In = 1.8, Out = 1.85). This was reflected in all atolls except Peros Banhos. Contrary to this, appendicularians were more common at inner regions (In = 1.2, Out = 0.92). This occurrence was mirrored across all atolls barring southwest Egmont and within Peros Banhos where they were only present in the inner region of the atoll.
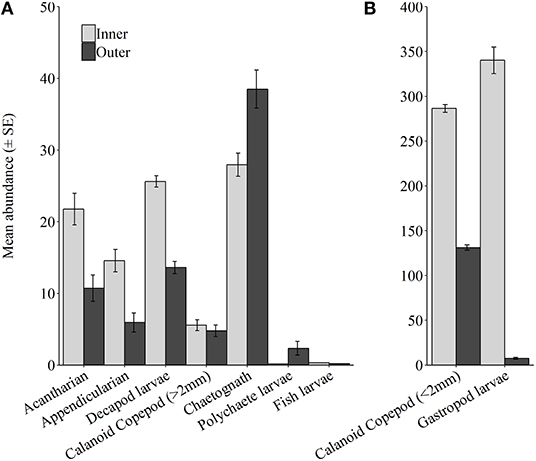
Figure 8. Mean abundance (± SE) for common taxa and/or taxa of ecological interest, at Inner and Outer stations across all atolls. (A) Taxa group 1, (B) Taxa group 2, Taxa have been separated into groups for graphical representation.
Of the meroplankton, gastropod larvae were the most dissimilar (4.26 mean dissimilarity ± 0.91) and dominated inner regions (In = 1.91, Out = 1.17). This pattern was consistent in Diego Garcia and southwest Egmont, and Peros Banhos, where gastropod larvae were only present within inner stations. However, within Salomon and southeast Egmont more individuals were found within outer stations. Decapod larvae were the second most dissimilar meroplankton group (2.23 mean dissimilarity ± 1.01) and were present at greater abundance within inner stations (In = 2.11, Out = 1.7) and this was consistent across atolls, except Diego Garcia. Polychaete larvae, however, were encountered more often within outer regions (In = 0.1, Out = 0.45). This was detected within Salomon and across southwest Egmont, and Peros Banhos wherein polychaete larvae only occurred within the outer region of the atoll. Polychaete larvae were not observed within Diego Garcia or southeast Egmont. There were no obvious trends in fish larval abundance across BIOT. Fish larvae were observed only within Diego Garcia, the outer region of Southwest Egmont and the inner region of Peros Banhos; abundance was consistently low.
Discussion
Diego Garcia, Salomon, Egmont, and Peros Banhos all shared similar intertidal beach habitats characterized by course sands with low organic content. Subtidally, the extent of bleaching was the most consistent and extensive feature. Diego Garcia was dominated by bleached bombies and sandy mounts, Egmont and Peros Banhos were characterized by bleached massive and degrading corals, whereas Salomon still supported live corals as a dominant habitat type. Despite bleaching effects, reef fishes were still observed in all atolls.
Zooplankton assemblages comprised different compositions depending on whether they were in the inner or outer atoll regions. Assemblages also varied between the atolls that were located furthest apart (Diego Garcia to all other atolls), and were more similar between atolls that were closer (Peros Banhos and Salomon). Overall, in the outer regions of BIOT atolls, planktonic assemblages were more similar between atolls, than inner station assemblages between atolls; which were more diverse. The water property and the plankton data support the hypothesis that atolls have regional differences which is likely to be the result of increased isolation of inner stations from oceanic exposure relative to outer regions. Shorter water residence time and frequent flushing with increasing proximity to channels within these outer regions, likely selects for community similarities by facilitating planktonic transport both in and out of the atolls. These exchanges are known to not only modify the composition of the planktonic community but also the abundance (Hamner et al., 2007; Pagano et al., 2017). Evidence suggesting the flushing of oceanic water was observed within outer regions of Diego Garcia, Peros Banhos, and Egmont; typically demonstrated by cold, saline, and dense water. However, a longer time series is required to conclusively demonstrate tidal forcing of this water through deep water channels. Tidal forcing through atoll channels has been documented (Green et al., 2018) and the pumping of cold water from depth demonstrated within canyons (Walter et al., 2012; Walter and Phelan, 2016) and to shallow coral reefs, driven by tidal bores (Leichter et al., 1996; Woodson, 2018). Given the density and low temperatures of these oceanic intrusions, deeper within the water column, it is clear that this flushing could not have originated from surface flow over the shallow reefs that surround some atolls. No evidence of oceanic flushing was found within outer stations in Salomon atoll, which was the only atoll where live coral was observed as a dominant habitat type. This may be a result of the tidal phase at the time of sampling, which may have been conducive for the flushing out of oceanic water and/or oceanic exchange may be occurring in a location not represented within the transect. Further detail on water properties within Chagos atolls, specifically temperature, can be found in Sheppard et al. (2017).
Abundance of plankton was shown to be consistently lower within outer regions, except southeast Egmont. Transport of plankton through channels adjacent to the coral reef may account for this reduction, as lower abundance of zooplankton following passage over the coral rim of atolls is well documented and has been linked to ingestion by the reef (Fabricius and Metzner, 2004; Wyatt et al., 2010) and planktivorous fish (Achuthankutty et al., 1989; Hamner et al., 2007). Increased surface temperatures and chlorophyll within some of the inner regions may also be driving the distinctions between inner and outer assemblages (Carassou et al., 2010).
The greater abundance of zooplankton present within outer stations in southeast Egmont is intriguing. Although not formally tested in this study, internal waves could perhaps account for this pattern as they are known to influence planktonic distributions (Shanks, 1983; Lennert-Cody and Franks, 1999) and have been observed in the BIOT (Hosegood et al., 2019). Turbulence associated with internal waves (Moum et al., 1992) may increase predator-prey contact rates and have an overall positive effect on plankton feeding rates (Kiørboe, 1997; Saiz et al., 2003), thus altering the structure of planktonic assemblages. In addition, variability in oceanographic parameters believed important to planktonic distributions, such as temperature, salinity, water velocity, and chlorophyll, may be associated with internal bores created by breaking internal waves (Leichter et al., 1996).
Egmont atoll was also distinctive due to the greatest difference between number of taxa in inner and outer stations, on the south-westerly transect. The remarkable abundance of zooplankton within the inner stations is represented mostly by an extreme abundance of gastropod larvae. Abundance of gastropod larvae has previously been positively related to solar radiation duration (Carassou et al., 2010); given the insolation effects evident within Egmont atoll it is possible that this might be influencing their abundance and distribution. Alternatively, the sampling may have captured a spawning event. This increased occurrence of gastropod larvae in inner regions is replicated across most atolls with other meroplankton, such as decapod larvae, also common to these areas. Increased occurrence of meroplankton within atolls is commonly documented (Gerber, 1981; Achuthankutty et al., 1989; Pagano et al., 2017) and specifically within the Chagos Archipelago, greater availability and longer residence of larvae has been linked to the weaker currents within lagoons (Riegl et al., 2012). Therefore, the co-occurrence of increased zooplanktonic biomass within these areas perhaps suggests the importance of these inner, less exposed areas as nursery grounds for larval species.
Although oceanographic processes are known drivers of zooplankton distribution and abundance (Kiørboe, 1997; Huggett, 2014; Lamont et al., 2014) and interactions within this study are evident, it is possible that behavioral responses and life history traits may also play a role, particularly for copepods; a major contribution to the zooplanktonic taxa in this study. Copepods have been observed to maintain position by active movements against the flow, migrating down to low current regions and attaching to substrate (Genin et al., 2005; Shang et al., 2008). As such their retention within lagoons has been observed. Of the copepods, calanoids <2 mm were more commonly found within inner regions, and the opposite was true for those >2 mm. Larger and oceanic copepods are likely present in higher densities at outer stations due to the inward flushing of ocean currents. The lower numbers observed within inner stations could also indicate higher predation rates, as it has been suggested that larger-sized individuals are actively-selected by predators (Suchman and Sullivan, 2000; Van Deurs et al., 2014). The dominance of smaller copepods in inner regions of atolls, could therefore suggest a size-based predation refuge. Additionally, these regions are likely to experience greater fluctuations in water properties, due to lack of interaction with regulatory, stable water bodies. The dominating presence of calanoids <2 mm, mostly represented by Acartia spp. and Centropages spp., in these areas could be indicative of this. Species of these genera produce resting eggs, which allow the persistence of copepod taxa when environmental conditions become unfavorable for growth and reproduction (Guerrero and Rodriguez, 1998). Greater retention of eggs within areas of reduced flushing could account for the increased abundance observed.
The variability in zooplankton distribution and assemblage composition within atolls, with respect to oceanic exposure, demonstrates that past studies detailing broad comparisons between oceanic and lagoon areas may underestimate differences, if samples are only taken in the outer regions of atolls. Interactions with the water properties in these systems is clearly complex and requires more extensive studies to identify and determine how deep water flushing influences planktonic communities as they are transported both in and out of atolls. If a predictable planktonic zonation exists within these systems, comprehension of such ecological patterns is imperative for an indication of ecosystem health and functioning, particularly when such patterns are likely to be disrupted in the near future by global anthropogenic pressures such as climate change (Beaugrand, 2003). This is particularly concerning given the importance of zooplankton to ecosystem functioning (Hébert et al., 2017). Given the widespread bleaching evident in this study, both from past and recent bleaching events, such changes are likely already at work. BIOT atolls are clearly not exempt from global issues such as climate change, and the high degree of localized protection does not change that. However, irrespective of such bleaching, the diversity and abundance of holoplankton and reef-associated fauna in the meroplankton in the region demonstrates that these atolls are dynamic and potentially resilient systems (Sheppard et al., 2012), and as such are important to preserve.
Continued monitoring of relatively pristine sites such as the Chagos Archipelago presents an invaluable opportunity to assess near-natural ecosystem functioning within coral atolls, provide ecological baselines and demonstrate best-case scenarios for impacts of climate change to reef systems. However, the lack of ecological and oceanographic data to currently support such investigations is a major hindrance. Further investigation of the spatiotemporal dynamics of these planktonic communities in response to oceanographic processes within BIOT is therefore necessary to continue and sustain management of these habitats, in the midst of such environmental uncertainty world-wide.
Data Availability
The datasets generated for this study are available on request to the corresponding author.
Author Contributions
ES, PH, MA, and JM conceived this research. ES, JM, and DT gathered the data. ES, PH, CG, and MW analyzed the data. All authors wrote the manuscript.
Funding
The project was primarily funded by the motor yacht Pangaea for vessel time, salaries, and research support (JM, ES, and CG). With additional support from the Marine Institute, Plymouth, UK (ES).
Conflict of Interest Statement
The authors declare that the research was conducted in the absence of any commercial or financial relationships that could be construed as a potential conflict of interest.
Acknowledgments
The authors acknowledge the motor yacht Pangaea for vessel time, and its crew for support in the field. We thank Dr. Shanta Barley and Louis Masarei from the University of Western Australian and Dr. David Conway from The Marine Biological Association, United Kingdom. We are grateful for the validated maps from the Millennium Coral Reef Mapping Project provided by the Institute for Marine Remote Sensing, University of South Florida and Institut de Recherche pour le Développement, Centre de Nouméa, with support from NASA (http://imars.marine.usf.edu/MC/). We also thank the United Kingdom Foreign and Commonwealth Office who permitted this work and provided supplies of ethanol.
Supplementary Material
The Supplementary Material for this article can be found online at: https://www.frontiersin.org/articles/10.3389/fmars.2019.00512/full#supplementary-material
References
Achuthankutty, C., Nair, S., Haridas, P., and Madhupratap, M. (1989). Zooplankton composition of the Kalpeni and Agatti atolls, Lakshadweep archipelago. Indian J. Marine Sci. 18, 151–154.
Alldredge, A. L., and King, J. M. (2009). Near-surface enrichment of zooplankton over a shallow back reef: implications for coral reef food webs. Coral Reefs 28, 895–908. doi: 10.1007/s00338-009-0534-4
Anderson, M. J. (2001). A new method for non-parametric multivariate analysis of variance. Aust. Ecol. 26, 32–46. doi: 10.1111/j.1442-9993.2001.01070.pp.x
Beaugrand, G. (2003). Long-term changes in copepod abundance and diversity in the north-east Atlantic in relation to fluctuations in the hydroclimatic environment. Fish. Oceanogr. 12, 270–283. doi: 10.1046/j.1365-2419.2003.00248.x
Bozec, Y. M., Gascuel, D., and Kulbicki, M. (2004). Trophic model of lagoonal communities in a large open atoll (Uvea, Loyalty islands, New Caledonia). Aquat. Living Resour. 17, 151–162. doi: 10.1051/alr:2004024
Bray, J. R., and Curtis, J. T. (1957). An ordination of the upland forest communities of Southern Wisconsin. Ecol. Monogr. 27, 325–349. doi: 10.2307/1942268
Carassou, L., Le Borgne, R., Rolland, E., and Ponton, D. (2010). Spatial and temporal distribution of zooplankton related to the environmental conditions in the coral reef lagoon of New Caledonia, Southwest Pacific. Marine Pollut. Bull. 61, 367–374. doi: 10.1016/j.marpolbul.2010.06.016
Carrillo Baltodano, A., and Morales Ramírez, Á. (2016). Changes in abundance and composition of a Caribbean coral reef zooplankton community after 25 years. Int. J. Trop. Biol. Conserv. 64, 1029–1040. doi: 10.15517/rbt.v64i3.21449
Chevalier, C., Devenon, J. L., Pagano, M., Rougier, G., Blanchot, J., and Arfi, R. (2017). The atypical hydrodynamics of the Mayotte Lagoon (Indian Ocean): effects on water age and potential impact on plankton productivity. Estuar. Coast. Shelf Sci. 196, 182–197. doi: 10.1016/j.ecss.2017.06.027
Clarke, K. R., and Warwick, R. M. (2001). Change in Marine Communities: An Approach to Statistical Analysis and Interpretation: Primer E. Plymouth: Plymouth Marine Laboratory.
Conway, D. V. P., White, R. G., Hugues-Dit-Ciles, J., Gallienne, C. P., and Robins, D. B. (2003). Guide to the Coastal and Surface Zooplankton of the South-Western Indian Ocean. Plymouth: Occasional Publication of the Marine Biological Association of the United Kingdom, No 15. doi: 10.13140/2.1.1554.0165
Dumas, F., Le Gendre, R., Thomas, Y., and Andréfouët, S. (2012). Tidal flushing and wind driven circulation of Ahe atoll lagoon (Tuamotu Archipelago, French Polynesia) from in situ observations and numerical modelling. Marine Pollut. Bull. 65, 425–440. doi: 10.1016/j.marpolbul.2012.05.041
Dupuy, C., Pagano, M., Got, P., Domaizon, I., Chappuis, A., Marchessaux, G., et al. (2016). Trophic relationships between metazooplankton communities and their plankton food sources in the Iles Eparses (Western Indian Ocean). Marine Environ. Res. 116, 18–31. doi: 10.1016/j.marenvres.2016.02.011
Fabricius, K. E., and Metzner, J. (2004). Scleractinian walls of mouths: predation on coral larvae by corals. Coral Reefs 23, 245–248. doi: 10.1007/s00338-004-0386-x
Falter, J. L., Atkinson, M. J., and Merrifield, M. A. (2004). Mass-transfer limitation of nutrient uptake by a wave-dominated reef flat community. Limnol. Oceanogr. 49, 1820–1831. doi: 10.4319/lo.2004.49.5.1820
Firth, L. B., Knights, A. M., Bridger, D., Evans, A., Mieszkowska, N., Moore, P., et al. (2016). Ocean sprawl: challenges and opportunities for biodiversity management in a changing world. Oceanogr. Mar. Biol. 54, 189–262. doi: 10.1201/9781315368597-5
Folk, R. L., and Ward, W. C. (1957). Brazos river bar: a study in the significance of grain size parameters. J. Sediment. Petrol. 27, 3–26. doi: 10.1306/74D70646-2B21-11D7-8648000102C1865D
Genin, A., Jaffe, J. S., Reef, R., Richter, C., and Franks, P. J. (2005). Swimming against the flow: a mechanism of zooplankton aggregation. Science 308, 860–862. doi: 10.1126/science.1107834
Gerber, R. P. (1981). Species composition and abundance of lagoon zooplankton at Enewetak atoll, Marshall Islands. Atoll Res. Bull. 247, 1–22. doi: 10.5479/si.00775630.247.1
Green, R. H., Lowe, R. J., and Buckley, M. L. (2018). Hydrodynamics of a tidally forced coral reef atoll. J. Geophys. Res. 123, 7084–7101. doi: 10.1029/2018JC013946
Grorud-Colvert, K., and Sponaugle, S. (2009). Larval supply and juvenile recruitment of coral reef fishes to marine reserves and non-reserves of the upper Florida Keys, USA. Marine Biol. 156, 277–288. doi: 10.1007/s00227-008-1082-0
Guerrero, F., and Rodriguez, V. (1998). Existence and significance of Acartia grani resting eggs (Copepoda: Calanoida) in sediments of a coastal station in the Alboran Sea (SE Spain). J. Plankton Res. 20, 305–314. doi: 10.1093/plankt/20.2.305
Hamner, W. M., Colin, P. L., and Hamner, P. P. (2007). Export-import dynamics of zooplankton on a coral reef in Palau. Marine Ecol. Prog. Ser. 334, 83–92. doi: 10.3354/meps334083
Hatcher, A. I., and Frith, C. A. (1985). The control of nitrate and ammonium concentrations in a coral reef lagoon. Coral Reefs 4, 101–110. doi: 10.1007/BF00300868
Hébert, M.-P., Beisner, B. E., and Maranger, R. (2017). Linking zooplankton communities to ecosystem functioning: toward an effect-trait framework. J. Plankton Res. 39, 3–12. doi: 10.1093/plankt/fbw068
Hosegood, P. J., Nimmo-Smith, W. A. M., Proud, R., Adams, K., and Brierley, A.S. (2019). Internal lee waves and baroclinic bores over a tropical seamount shark ‘hot-spot’. Prog. Oceanogr. 172, 34–50. doi: 10.1016/j.pocean.2019.01.010
Huggett, J. A. (2014). Mesoscale distribution and community composition of zooplankton in the Mozambique Channel. Deep Sea Res. II 100, 119–135. doi: 10.1016/j.dsr2.2013.10.021
Hughes, T. P., Baird, A. H., Dinsdale, E. A., Moltschaniwskyj, N. A., Pratchett, M. S., Tanner, J. E., et al. (2000). Supply-side ecology works both ways: the link between benthic adults, fecundity, and larval recruits. Ecology 81, 2241–2249. doi: 10.1890/0012-9658(2000)081[2241:SSEWBW]2.0.CO;2
Johannes, R., and Gerber, R. (1974). “Import and export of net plankton by an Eniwetok coral reef community”, in Proc 2nd Int Coral Reef Symp (Brisbane, QLD), 97–104.
Kench, P. S. (1998). Physical processes in an Indian Ocean atoll. Coral Reefs 17, 155–168. doi: 10.1007/s003380050110
Kennish, M. J. (2001). Zooplankton of the barnegat bay–little egg harbor estuary. J. Coast. Res. 163–166. Available online at: https://www.jstor.org/stable/25736231.
Kiørboe, T. (1997). Small-scale turbulence, marine snow formation, and planktivorous feeding. Sci. Mar. 61, 141–158.
Knowlton, N., and Jackson, J. B. (2008). Shifting baselines, local impacts, and global change on coral reefs. PLoS Biol. 6:e54. doi: 10.1371/journal.pbio.0060054
Lamont, T., Barlow, R. G., Morris, T., and Van Den Berg, M. A. (2014). Characterisation of mesoscale features and phytoplankton variability in the Mozambique Channel. Deep Sea Res. II 100, 94–105. doi: 10.1016/j.dsr2.2013.10.019
Le Borgne, R., Blanchot, J., and Charpy, L. (1989). Zooplankton of tikehau atoll (Tuamotu archipelago) and its relationship to particulate matter. Mar. Biol. 102, 341–353. doi: 10.1007/BF00428486
Legendre, L., and Le Fèvre, J. (1991). “From individual plankton cells to pelagic marine ecosystems and to global biogeochemical cycles,” in Particle Analysis in Oceanography, ed S. Demers (Berlin; Heidelberg: Springer Berlin Heidelberg), 261–300.
Leichter, J. J., Wing, S. R., Miller, S. L., and Denny, M. W. (1996). Pulsed delivery of subthermocline water to Conch Reef (Florida Keys) by internal tidal bores. Limnol. Oceanography 41, 1490–1501. doi: 10.4319/lo.1996.41.7.1490
Lennert-Cody, C. E., and Franks, P. J. S. (1999). Plankton patchiness in high-frequency internal waves. Mar. Ecol. Prog. Ser. 186, 59–66. doi: 10.3354/meps186059
Letessier, T. B., Cox, M. J., Meeuwig, J. J., Boersch-Supan, P. H., and Brierley, A. S. (2016). Enhanced pelagic biomass around coral atolls. Mar. Ecol. Prog. Ser. 546, 271–276. doi: 10.3354/meps11675
Mccauley, D. J., Desalles, P. A., Young, H. S., Papastamatiou, Y. P., Caselle, J. E., Deakos, M. H., et al. (2014). Reliance of mobile species on sensitive habitats: a case study of manta rays (Manta alfredi) and lagoons. Mar. Biol. 161, 1987–1998. doi: 10.1007/s00227-014-2478-7
Mendonca, M. T., and Ehrhart, L. M. (1982). Activity, population size and structure of immature Chelonia mydas and Caretta caretta in Mosquito Lagoon, Florida. Copeia 1982, 161–167. doi: 10.2307/1444280
Moum, J. N., Hebert, D., Paulson, C. A., and Caldwell, D. R. (1992). Turbulence and internal waves at the equator. Part I: statistics from towed thermistors and a microstructure profiler. J. Phys. Oceanogr. 22, 1330–1345. doi: 10.1175/1520-0485(1992)022<;1330:TAIWAT>2.0.CO;2
Niquil, N., Jackson, A. J., Legendre, L., and Delesalle, B. (1998). Inverse model analysis of the planktonic food web of Takapoto Atoll (French Polynesia). Mar. Ecol. Prog. Ser. 165, 17–29. doi: 10.3354/meps165017
Odum, H. T., and Odum, E. P. (1955). Trophic structure and productivity of a windward coral reef community on Eniwetok Atoll. Ecol. Monogr. 25, 291–320. doi: 10.2307/1943285
Pagano, M., Rodier, M., Guillaumot, C., Thomas, Y., Henry, K., and Andréfouët, S. (2017). Ocean-lagoon water and plankton exchanges in a semi-closed pearl farming atoll lagoon (Ahe, Tuamotu archipelago, French Polynesia). Estuar. Coast. Shelf Sci. 191, 60–73. doi: 10.1016/j.ecss.2017.04.017
Pagano, M., Sagarra, P. B., Champalbert, G., Bouvy, M., Dupuy, C., Thomas, Y., et al. (2012). Metazooplankton communities in the Ahe atoll lagoon (Tuamotu Archipelago, French Polynesia): spatiotemporal variations and trophic relationships. Mar. Pollut. Bull. 65, 538–548. doi: 10.1016/j.marpolbul.2012.01.025
Pugh, D. T., and Rayner, R. F. (1981). The tidal regimes of three indian ocean atolls and some ecological implications. Estuar. Coast. Shelf Sci. 13, 389–407. doi: 10.1016/S0302-3524(81)80036-9
Rayner, R. F., and Drew, E. A. (1984). Nutrient concentrations and primary productivity at the Peros Banhos and Salomon atolls in the Chagos Archipelago. Estuar. Coast. Shelf Sci. 18, 121–132. doi: 10.1016/0272-7714(84)90100-8
Riegl, B. M., Sheppard, C. R., and Purkis, S. J. (2012). Human impact on atolls leads to coral loss and community homogenisation: a modeling study. PLoS ONE 7:e36921. doi: 10.1371/journal.pone.0036921
Roman, M. R., Furnas, M. J., and Mullin, M. M. (1990). Zooplankton abundance and grazing at Davies Reef, Great Barrier Reef, Australia. Mar. Biol. 105, 73–82. doi: 10.1007/BF01344272
Saiz, E., Calbet, A., and Broglio, E. (2003). Effects of small-scale turbulence on copepods: the case of Oithona davisae. Limnol. Oceanogr. 48, 1304–1311. doi: 10.4319/lo.2003.48.3.1304
Shang, X., Wang, G., and Li, S. (2008). Resisting flow–laboratory study of rheotaxis of the estuarine copepod Pseudodiaptomus annandalei. Mar. Freshw. Behav. Physiol. 41, 91–106. doi: 10.1080/10236240801905859
Shanks, A. L. (1983). Surface slicks associated with tidally forced internal waves may transport pelagic larvae of benthic invertebrates and fishes shoreward. Mar. Ecol. Prog. Ser. 13, 311–315. doi: 10.3354/meps013311
Sheehan, E., Coleman, R., Thompson, R., and Attrill, M. (2010). Crab-tiling reduces the diversity of estuarine infauna. Mar. Ecol. Prog. Ser. 411, 137–148. doi: 10.3354/meps08668
Sheehan, E. V., Bridger, D., Cousens, S. L., and Attrill, M. J. (2015). Testing the resilience of dead maerl infaunal assemblages to the experimental removal and re-lay of habitat. Mar. Ecol. Prog. Ser. 535, 117–128. doi: 10.3354/meps11400
Sheehan, E. V., Stevens, T. F., Gall, S. C., Cousens, S. L., and Attrill, M. J. (2013). Recovery of a temperate reef assemblage in a marine protected area following the exclusion of towed demersal fishing. PLoS ONE 8:e83883. doi: 10.1371/journal.pone.0083883
Sheppard, C., Sheppard, A., Mogg, A., Bayley, D., Dempsey, A. C., Roche, R., et al. (2017). Coral bleaching and mortality in the Chagos Archipelago. Atoll Res. Bull. 613, 1–26. doi: 10.5479/si.0077-5630.613
Sheppard, C. R., Ateweberhan, M., Bowen, B. W., Carr, P., Chen, C. A., Clubbe, C., et al. (2012). Reefs and islands of the Chagos Archipelago, Indian Ocean: why it is the world's largest no-take marine protected area. Aquat. Conserv. 22, 232–261. doi: 10.1002/aqc.1248
Suchman, C. L., and Sullivan, B. K. (2000). Effect of prey size on vulnerability of copepods to predation by the scyphomedusae Aurelia aurita and Cyanea sp. J. Plankton Res. 22, 2289–2306. doi: 10.1093/plankt/22.12.2289
Thompson, R. O. R. Y., and Golding, T. J. (1981). Tidally induced ‘upwelling’ by the Great Barrier Reef. J. Geophys. Res. 86, 6517–6521. doi: 10.1029/JC086iC07p06517
Thomson, R. E., and Wolanski, E. J. (1984). Tidal period upwelling within Raine Island Entrance Great Barrier Reef. J. Mar. Res. 42, 787–808. doi: 10.1357/002224084788520792
Tickler, D. M., Letessier, T. B., Koldewey, H. J., and Meeuwig, J. J. (2017). Drivers of abundance and spatial distribution of reef-associated sharks in an isolated atoll reef system. PLoS ONE 12:e0177374. doi: 10.1371/journal.pone.0177374
Van Deurs, M., Koski, M., and Rindorf, A. (2014). Does copepod size determine food consumption of particulate feeding fish? ICES J. Mar. Sci. 71, 35–43. doi: 10.1093/icesjms/fst090
Walter, R. K., and Phelan, P. J. (2016). Internal bore seasonality and tidal pumping of subthermocline waters at the head of the Monterey submarine canyon. Cont. Shelf Res. 116, 42–53. doi: 10.1016/j.csr.2016.01.015
Walter, R. K., Woodson, C. B., Arthur, R. S., Fringer, O. B., and Monismith, S. G. (2012). Nearshore internal bores and turbulent mixing in southern Monterey Bay. J. Geophys. Res. 117:C07017. doi: 10.1029/2012JC008115
Wolanski, E., Drew, E., Abel, K. M., and O'brien, J. (1988). Tidal jets, nutrient upwelling and their influence on the productivity of the alga Halimeda in the Ribbon Reefs, Great Barrier Reef. Estuar. Coast. Shelf Sci. 26, 169–201. doi: 10.1016/0272-7714(88)90049-2
Woodson, C. B. (2018). The fate and impact of internal waves in nearshore ecosystems. Ann. Rev. Mar. Sci. 10, 421–441. doi: 10.1146/annurev-marine-121916-063619
Keywords: plankton, Chagos Archipelago, Indian Ocean, atoll, marine protected area, exposure, oceanography, coral bleaching
Citation: Sheehan EV, Hosegood P, Game CA, Attrill MJ, Tickler D, Wootton M, Johns DG and Meeuwig JJ (2019) The Effect of Deep Oceanic Flushing on Water Properties and Ecosystem Functioning Within Atolls in the British Indian Ocean Territory. Front. Mar. Sci. 6:512. doi: 10.3389/fmars.2019.00512
Received: 30 April 2019; Accepted: 05 August 2019;
Published: 20 August 2019.
Edited by:
Michael Arthur St. John, Technical University of Denmark, DenmarkReviewed by:
Charles Gordon Hannah, Department of Fisheries and Oceans, CanadaKusum Komal Karati, Centre for Marine Living Resources and Ecology (CMLRE), India
Copyright © 2019 Sheehan, Hosegood, Game, Attrill, Tickler, Wootton, Johns and Meeuwig. This is an open-access article distributed under the terms of the Creative Commons Attribution License (CC BY). The use, distribution or reproduction in other forums is permitted, provided the original author(s) and the copyright owner(s) are credited and that the original publication in this journal is cited, in accordance with accepted academic practice. No use, distribution or reproduction is permitted which does not comply with these terms.
*Correspondence: Chloe A. Game, Y2hsb2UuZ2FtZUB1ZWEuYWMudWs=