- 1Monterey Bay Aquarium Research Institute, Moss Landing, CA, United States
- 2Monterey Bay National Marine Sanctuary, National Ocean Service, National Oceanic and Atmospheric Administration, Monterey, CA, United States
- 3Moravian College, Bethlehem, PA, United States
Corals and sponges in rocky deep-sea environments are foundation species postulated to enhance local diversity by increasing biogenic habitat heterogeneity and enriching local carbon cycling. These key groups are highly vulnerable to disturbances (e.g., trawling, mining, and pollution) and are threatened by expansive changes in ocean conditions linked to climate change (acidification, warming, and deoxygenation). Once damaged by trawling or other disturbances, recolonization and regrowth may require centuries or longer, highlighting the need for stewardship of these deep-sea coral and sponge communities (DSCSCs). To this end, the sustainability of DSCSCs may be enhanced not only by protecting existing communities, but also repopulating disturbed areas using active restoration methods. Here, we report one of the first studies to explore methods to restore deep-sea coral populations by translocating coral fragments of multiple coral species. Branches of deep-sea corals were collected by ROV from 800 to 1300 m depth off central California and propagated into multiple fragments once at the surface. These fragments were then attached to “coral pots” using two different methods and placed in the same habitat to assess their survivorship (n = 113 total fragments, n = 7 taxa, n = 7 deployment groups). Mean survivorship for all translocated coral fragments observed within the first 365 days was ∼52%, with the highest mortality occurring in the first 3 months. In addition to an initial temporal sensitivity, survival of coral fragments varied by attachment method and among species. All coral fragments attached to coral pots using zip ties died, while those attached by cement resulted in differential survivorship over time. The latter method resulted in 80–100% fragment survivorship after 1 year for Corallium sp., Lillipathes sp., and Swiftia kofoidi, 12–50% for the bamboo corals Keratoisis sp. and Isidella tentaculum, and 0–50% for the bubblegum corals Paragorgia arborea and Sibogagorgia cauliflora. These initial results indicate differences in sensitivities to transplanting methods among coral species, but also suggest that repopulation efforts may accelerate the recovery of disturbed DSCSCs.
Introduction
Human impacts in the deep-sea are increasing from direct extractive activities (e.g., fishing, mineral extraction), pollution (e.g., oil spills, trash), and expansive changes in ocean conditions linked to anthropogenic climate change (ocean acidification, warming, and deoxygenation). While the integrated impacts of human activities in the deep-sea remain largely unknown, there is clear evidence that bottom trawling for fishes and invertebrates alone is leaving a global footprint from the nearshore to >1000 m depth (Amoroso et al., 2018). Catastrophes such as the 2010 BP Deepwater Horizon oil spill in the Gulf of Mexico also demonstrated that an oil spill from a single platform can have far ranging effects spanning from ecological impacts in the deep-sea to economic depression for the adjacent coastal communities—with the full potential impacts still unknown (McCrea-Strub et al., 2011; White et al., 2011; Sumaila et al., 2012). Although the Magnuson-Stevens Fishery Conservation and Management Act (2006) establishes a legal mandate to minimize negative impacts to these and other ecosystems within the United States Exclusive Economic Zone, the slow rates of recovery for some damaged deep-sea assemblages suggest that active restoration efforts may be beneficial.
Recovery and resilience of impacted deep-sea ecosystems may be enhanced by efforts to propagate species that form key biogenic structures. Species that form biogenic structures are hypothesized to facilitate positive species interactions, further promoting both biodiversity and ecosystem function (Bruno and Bertness, 2000; Bruno et al., 2003). In deep-sea ridge and seamount systems, suspension feeders such as corals and sponges typically dominate the benthos and provide critical biogenic habitat for a variety of associated deep-sea fishes and invertebrates (Rogers, 1999; Koslow et al., 2000; Roark et al., 2005; Stone, 2006; Love et al., 2007; Baillon et al., 2012; Bourque and Demopoulos, 2018). Furthermore, suspension feeding by these organisms may enhance carbon sequestration (Murray et al., 1994; Cathalot et al., 2015; Kahn et al., 2015). Thus, efforts to translocate healthy or rehabilitated corals and sponges may accelerate the recovery of local diversity and ecosystem function in deep-sea coral and sponge communities (DSCSCs) that have been disturbed or destroyed by human activity.
Understanding how to actively enhance or facilitate the recovery of disturbed DSCSCs is a new frontier for restoration science. Van Dover et al. (2014) evaluated hypothetical scenarios of ecological restoration of the deep sea but lacked empirical data from active restoration studies, bringing to light a major gap in applied scientific knowledge. Although experimental restoration of the deep-sea corals Lophelia pertusa (∼ 500 m in depth) and Oculina varicosa (∼60–120 m) has been somewhat successful in the Gulf of Mexico (Koenig et al., 2005; Brooke et al., 2006; Brooke and Young, 2009), studies examining the sensitivity of multiple coral taxa to differential handling and processing remain a gap in knowledge for all deep-sea efforts. However, insights gained from these initial efforts, in combination with information from coral reef restoration studies from shallow habitats (<30 m), may serve as a more comprehensive guide to expanding mitigation strategies for the deep sea.
While studies of both asexual and sexual propagation of shallow water corals are prevalent, we focused on translocating coral fragments from multiple coral taxa as a first step to understanding the feasibility and facilitation of DSCSC recovery in the Eastern Pacific for several reasons. Enhancing coral reef recovery via translocation or transplanting coral fragments are presently proposed to be more advantageous than using sexual propagation methods due to the cost-effectiveness and requirements for technical knowledge (Jaap, 2000; Bowden-Kerby, 2001; Epstein et al., 2001; Spieler et al., 2001; Rinkevich, 2008; Edwards et al., 2010; Villanueva et al., 2012; Barton et al., 2015). That is, propagation of corals by harnessing gametes or larvae requires biological, culturing, and processing knowledge that may be more complicated than fragmenting corals and attaching fragments to a surface. For example, harnessing the sexual propagation of Acropora corals in shallow water systems required knowledge of when these corals were likely to broadcast gametes, culturing the gametes through fertilization and larval development, creating larval settlement surfaces, determining the optimal time for larval batch exposure to settlement cues, determining the optimal time for newly settled larvae outplanting, how to outplant or attach the new recruits to a natural reef, and many other steps in the process that required additional resources (Boch and Morse, 2012). Horoszowski-Fridman et al. (2011) investigated the reproductive output of transplanted coral fragments versus natural coral colonies and concluded that the reproductive role of coral transplants needs further attention. Various attachment strategies for scleractinia coral fragments have been reviewed and discussed (Barton et al., 2015) and insights from in situ experimental studies with shallow water gorgonians (20–25 m) are also available (Lasker, 1990; Linares et al., 2008a, b). Overall, the survivorship of both fragmented and sexually propagated corals has been shown to be most sensitive during the first post-transplant year and more successful for fragment transplants than sexually propagated corals (Epstein et al., 2001; Boch and Morse, 2012). In over three decades of shallow water coral restoration research, survivorship of asexually propagated corals has typically ranged 30–40% after the first year in situ.
In 2013, deep-sea coral and sponge communities (DSCSCs) were discovered at Sur Ridge (36°N; 122°W, Figure 1A) by collaborators from the Monterey Bay National Marine Sanctuary (MBNMS, National Oceanographic Atmospheric Administration) and the Monterey Bay Aquarium Research Institute (MBARI). Recognizing the need to establish active deep-sea coral restoration strategies in addition to protecting these highly diverse habitats, the collaborators began coral translocation studies to examine the feasibility of active deep-sea mitigation as an additional option along with protection. Here, we report insights gained from ∼3 years of deep-sea coral translocation studies and discuss some of the steps needed to potentially overcome current limitations. As there are no previous publications that report details of active deep-sea coral restoration approaches in the Eastern Pacific, we conducted our translocation experiments to determine the factors that may enhance survivorship by specifically examining coral processing protocols, the sensitivity of various deep-sea coral species to translocation stress, and temporal sensitivity in survivorship as an initial foundation for further investigation.
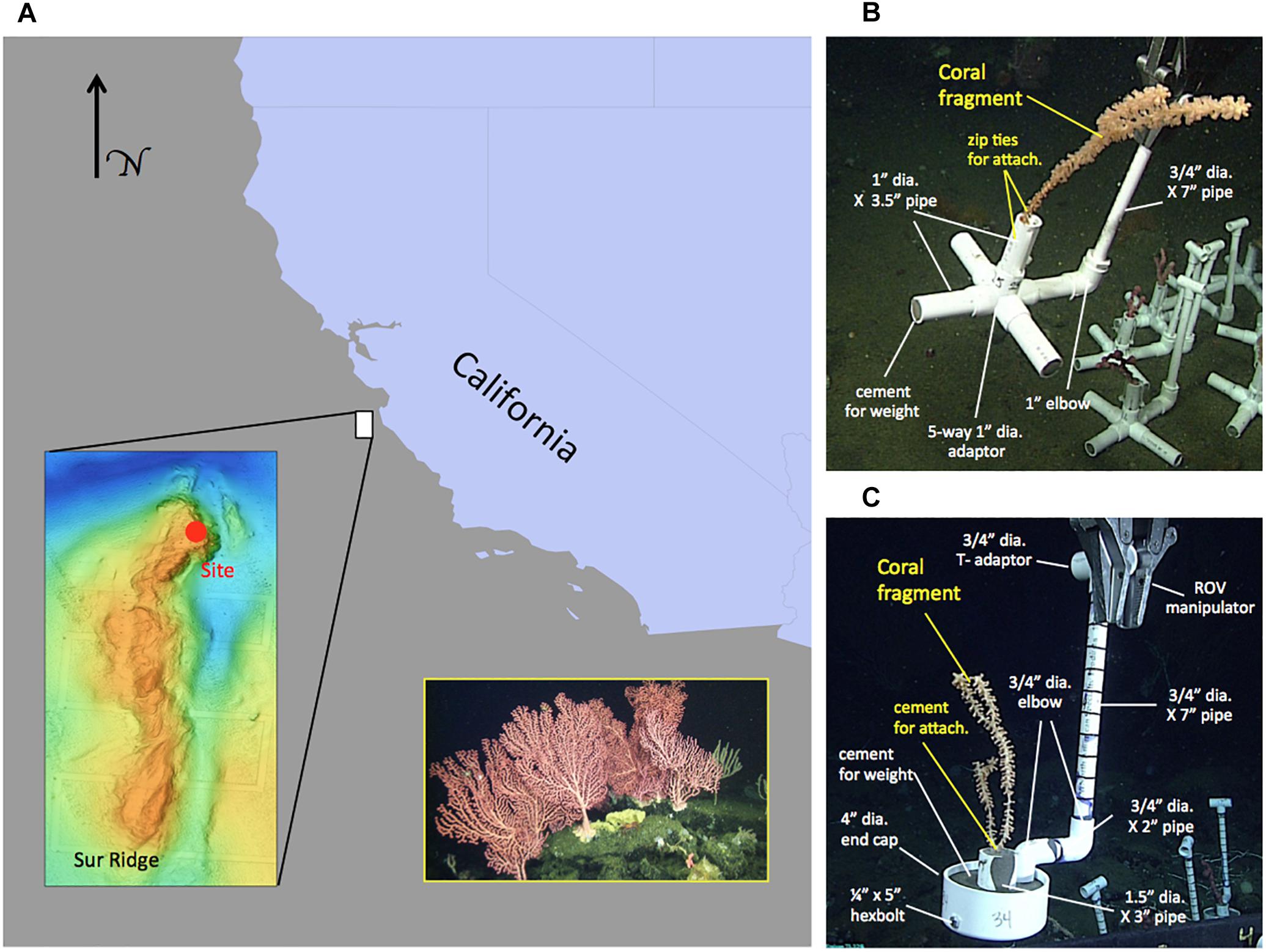
Figure 1. Map of the study area and schematic of coral pots with different coral attachment methods. (A) Location of Sur Ridge with respect to California, United States coastline. Lower-left inset: multi-beam sonar map of Sur Ridge. Solid red circle in the sonar map indicates the general location of the study site. Lower-right inset: photograph of an example coral and sponge cluster in the study area taken from the ROV Doc Ricketts. (B) Example coral pot using the zip tie method for attaching coral fragments in a moveable module. These coral pots (v.1) were also used with the cement attachment method (sans zip ties) in follow-up deployments. (C) Example coral pot v.2 using the cement attachment method for attaching a coral fragment in a moveable module. Note: all coral pot materials are Schedule 40 standard poly vinyl chloride (PVC) with the exception of the cement and galvanized steel hexbolt and nut.
Methods
Study Site, Environmental Exposure, and General Coral Processing
Coral translocation studies were conducted in DSCSCs at Sur Ridge, a prominent rocky ridge ∼60 km west of Point Sur, CA (Figure 1A). Coral taxa of interest were identified by the onboard taxonomic experts and using the Sur Ridge Field Guide created by the MBNMS and MBARI collaboration1. Seven coral taxa (Corallium sp., Lillipathes sp., Swiftia kofoidi, Keratoisis sp., Isidella tentaculum, Paragorgia arborea, and Sibogagorgia cauliflora; Table 1) common at Sur Ridge were included in the translocation studies.
Collection and translocation studies of coral fragments were conducted using the remotely operated vehicle (ROV) Doc Ricketts, operated from the R/V Western Flyer—a 36-meter long, small water-plane area twin hull vessel capable of deploying, operating, and recovering tethered remotely operated vehicles for oceanographic research. The Doc Ricketts has high-definition cameras, a suite of environmental sensors, robotic arms with manipulators, and storage containers (“bioboxes”) for samples. A high-resolution navigation system integrated with the R/V Western Flyer enabled repeated visits to specific locations on Sur Ridge for these studies. The data from a SBE 19 CTDO instrument (Sea-Bird Scientific, Bellevue, WA) mounted on the ROV Doc Ricketts during all of the ROV dives are reported to show the approximate ambient temperature, dissolved oxygen, and salinity levels experienced by the corals during the descent and ascent of ROV operations, during the collection of the donor coral branches and translocation of processed coral fragments. A sample temperature series logged within a biobox is also reported to provide a proxy of temperature experienced by the coral fragments within the biobox relative to the ambient temperature measurements.
Branches of selected coral species were collected from one or more colonies in situ that appeared to be visually “healthy” and stored in bioboxes for transport to the surface. After the ROV was recovered shipboard, branches of coral colonies (Corallium sp., Lillipathes sp., Swiftia kofoidi, Keratoisis sp., Isidella tentaculum, Paragorgia arborea, and Sibogagorgia cauliflora) were cut into smaller fragments using stainless steel scissors and attached to transportable modules (“coral pots”) that facilitated ROV operations with the bioboxes. Each coral fragment was placed approximately 1 inch (∼2 cm) in depth at the bases of the fragments. Each coral pot holding a single coral fragment was labeled with a unique number and deployment group letter (Figures 1B,C). Once processed, coral pots were returned by ROV to the site of collection within Sur Ridge DSCSCs to avoid confounding the potential effects of translocation with other factors (Figure 1A). Overall, n = 8 translocated coral groups were deployed with a mix of coral taxa. Collection, translocation, and re-visitation of translocated deep-sea corals were executed by careful coordination among the ship’s crew, ROV pilots, and scientists.
Coral Fragment Attachment
Survival of coral fragments may be affected by how coral fragments are attached to a transportable substrate or module. To evaluate the effects of attachment methods on translocated coral survivorship, coral pots were fabricated using polyvinyl chloride (PVC) plumbing parts with two different attachment methods (∼US$20 per pot). For attachment version 1 (coral pot v.1), fragments from multiple species were attached to a PVC base using four zip ties which “loosely” fixed the fragments to the upright 1″ PVC in the center of the coral pot (Figure 1B). PVC cross-legs at the pot base were filled with Sakrete® fast setting cement patcher (Charlotte, NC, United States) to function as the weight—the total pot without coral weighed approximately 1 kg. For attachment version 2 (coral pot v.2), coral fragments from multiple species were “hard” fixed to the upright 1.5″ PVC in the center of coral pot using the fast setting cement patcher (Figure 1C). The 4″ diameter PVC end cap at the base was filled with cement to anchor and enclose the pot in an upright orientation. The volume of cement required to affix fragments to the center PVC varied with the size and taxon of fragments. Version 2 pots weighed approximately 1.2 kg in dry weight without coral fragments.
Transport Stress
Survival of translocated corals may also be particularly affected by the duration of exposure to potentially stressful environmental changes during transport. To evaluate the sensitivity of corals to transport stress, we examined the survival of corals collected and returned to their DSCSC on the same day with a similar group held overnight in shipboard aquaria then relocated after ca. 1 day. For this experiment, only attachment of fragments by cement (coral pot v.2) was used. For the same-day treatment, multiple species were collected and processed while the corals were held in the ROV biobox to avoid stress (Supplementary Figure 1A). Using a team of six people with designated responsibilities, processing and attachment of each coral fragment required an average of 7 min per fragment (n = 20 coral fragments of various taxa). Once all fragments were fixed in coral pots, the ROV was deployed to relocate the corals at their collection location on Sur Ridge. This same-day approach required ca. 2 h at the surface and 1 h to return the coral pots to depth. For the overnight treatment, corals were maintained in shipboard aquaria overnight using 50-gallon acrylic aquaria (n = 3) in a darkened, temperature-controlled room. Chilled seawater (5–6°C) was set to flow at 1 Lmin−1 and the lights were turned off except during periods when corals were processed (Supplementary Figure 1B). Donor coral branches were constantly immersed in large Tupperware containers during movement from the ROV biobox to shipboard aquaria—ensuring the corals would not be exposed to air. Once in aquaria, the collected corals were fragmented and attached to coral pots (v.2). On the day after collection and processing (approximately 12–18 h post collection), the coral pots were transferred to the ROV biobox in large Tupperware containers for translocation to the study sites. The biobox was prepared to receive the coral pots by filling the partitions with the same shipboard chilled seawater used for the aquaria and with ice packs to maintain seawater temperature similar to their collection location.
Coral Survivorship and Analysis
Coral translocation sites were revisited one or more times per year for two plus years to determine the survival of translocated coral fragments. Corals were determined to be alive if the observed fragments were present in the coral pot at the time of the census and exhibited normal polyp and tissue color. After approximately 3 years of repeated visits to the study sites, overall survivorship patterns are reported by attachment method and by transport stress treatment. Survivorship over time was statistically analyzed using the generalized linear model (GLM) and segmented model with the deployment group and duration of transport stress as a combined fixed factor due to the low number of replicates. Segmented model analysis was used to test for any temporal sensitivity in survivorship by group deployment and transport stress—i.e., non-linear survival vs. time (Muggeo, 2003, 2008). Model fits were compared using the corrected Akaike Information Criterion (AICc). Reduction of the AICc by more than 10 by the segmented model was assumed to be a conservative indicator of a break-point in survival. Statistics were performed using the software R and the packages “lme4,” “segmented,” and “AICcmodavg.” We also report the survivorship patterns of each coral taxon as points for further discussion.
Results
Environmental Variability and Exposure
Temperature, salinity, and dissolved oxygen data logged from 0 to 1400 m depth during the coral translocation studies are shown in Supplementary Figures 2A–D (n = 14 dives). During these ROV dives, the corals were generally exposed to depths of 940.13 ± 344 m (mean ± SD), temperatures of 4.4 ± 2.18°C, salinity of 34.39 ± 0.22 PSU, and dissolved oxygen levels of 0.73 ± 0.91 ml/l (Table 2). Temperature sensors on the ROV and within the ROV biobox indicate that corals in the biobox experienced approximately 3°C warming during the ascent and descent of the ROV. Temperatures in the biobox rose from 5.2 to 8.5°C during ROV decent over the first 30 min (Supplementary Figure 3). Subsequently, temperatures within the biobox dropped to ambient (4.5°C) over the following 45 min. For corals held overnight in shipboard aquaria, seawater temperature (digital handheld thermometer; n = 12 samples) was stable (5.73 ± 0.42°C) and approximately 1.3°C warmer than the bottom temperatures at Sur Ridge.
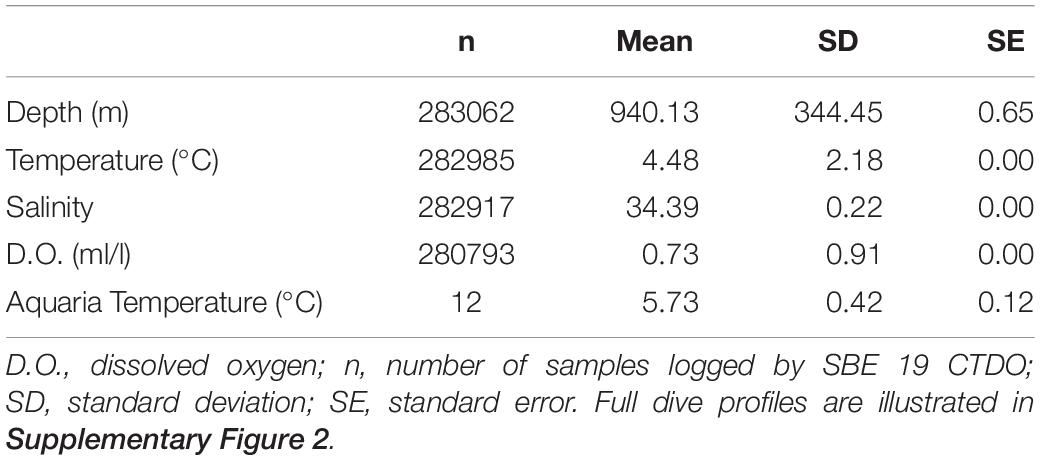
Table 2. General temperature, salinity, and dissolved oxygen conditions during ROV Doc Ricketts dives.
Coral Survivorship by Attachment and Temporal Sensitivity
Survival of coral fragments seemed to be dependent on the type of fragment attachment method—i.e., coral pot version—and non-linear over time. All Keratoisis sp., I. tentaculum, and P. arborea corals loosely attached by zip ties to a transportable module (coral pot v.1) did not survive (Figure 2A, black triangles). In contrast, coral fragments attached using cement (coral pot v.2) survived far better than those attached by zip-ties, but exhibited variable survival over time. Furthermore, some coral fragments survived 768 days displaying upright, colorful, and healthy polyps at the time of the census (see Figures 2C,D; Keratoisis sp. examples). Survivorship for all translocated coral fragments in coral pot v.2 observed within the first 365 days was ∼52.6%. At the end of the second year—i.e., between 563 and 685 days post-translocation—the overall mean survivorship was reduced to ∼23.9%. Survivorship of all the corals in coral pot v.2 was also significantly non-linear over time (Figure 2A and Table 3, p < 0.001). Segmented GLM analysis revealed that mortality rates were significantly higher in the first 105 ± 4.97 days (mean ± SE; Table 3, breaking point estimate; p < 0.05). After this initial period of approximately 3 months, the results indicate that overall survivorship rates became more stable with the slope of the second segment reaching near zero (Figure 2A and Table 3).
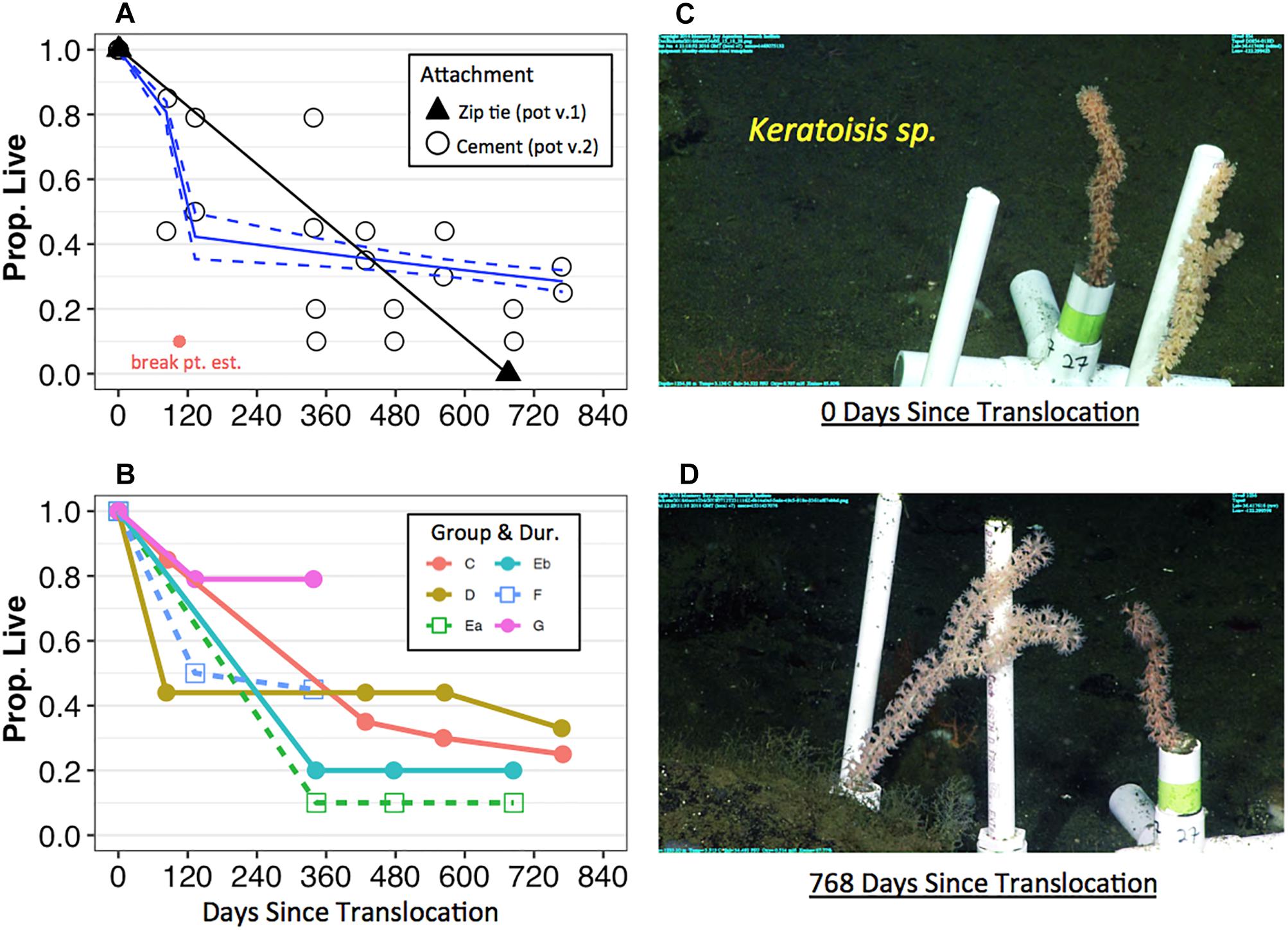
Figure 2. Survivorship of translocated deep-sea corals by attachment, deployment group, and transport treatment over time at Sur Ridge, California (coral pot v.2). (A) Solid black triangles represent the proportion of live coral over time relative to the total number of initial fragments using the zip tie attachment method (coral pot v.1). The triangle symbols that represent data for Keratoisis sp., I. tentaculum, and P. arborea overlap. Open black circles represent the proportion of live corals over time using the cement attachment method (coral pot v.2). Solid red circle represents the breaking point estimate (105.5 ± 4.9 SE days) for the coral pot v.2 data. Solid blue lines indicate segmented GLM fit with upper and lower confidence limit estimates (dashed blue lines). (B) Solid colored circles represent the proportion of live corals relative to the total number of initial fragments since the day of deployment—i.e., these corals were translocated to depth on the same day of collection. Open square symbols represent the proportion of live corals that were translocated on the day after collection. The numbers of coral fragments are reported in Table 1 for each deployment group and by taxa. (C) Example of Keratoisis sp. from Group D deployment—i.e., 0 days since translocation (June 4, 2016). (D) The same Keratoisis sp. samples from group D—768 days since translocation (July 12, 2018). The photo on the revisit could not be taken from exactly same camera angle but the #27 coral pot is clear in both panels (C,D). For panels (A,B), 0 days = the initial time when coral pots were translocated at depth.
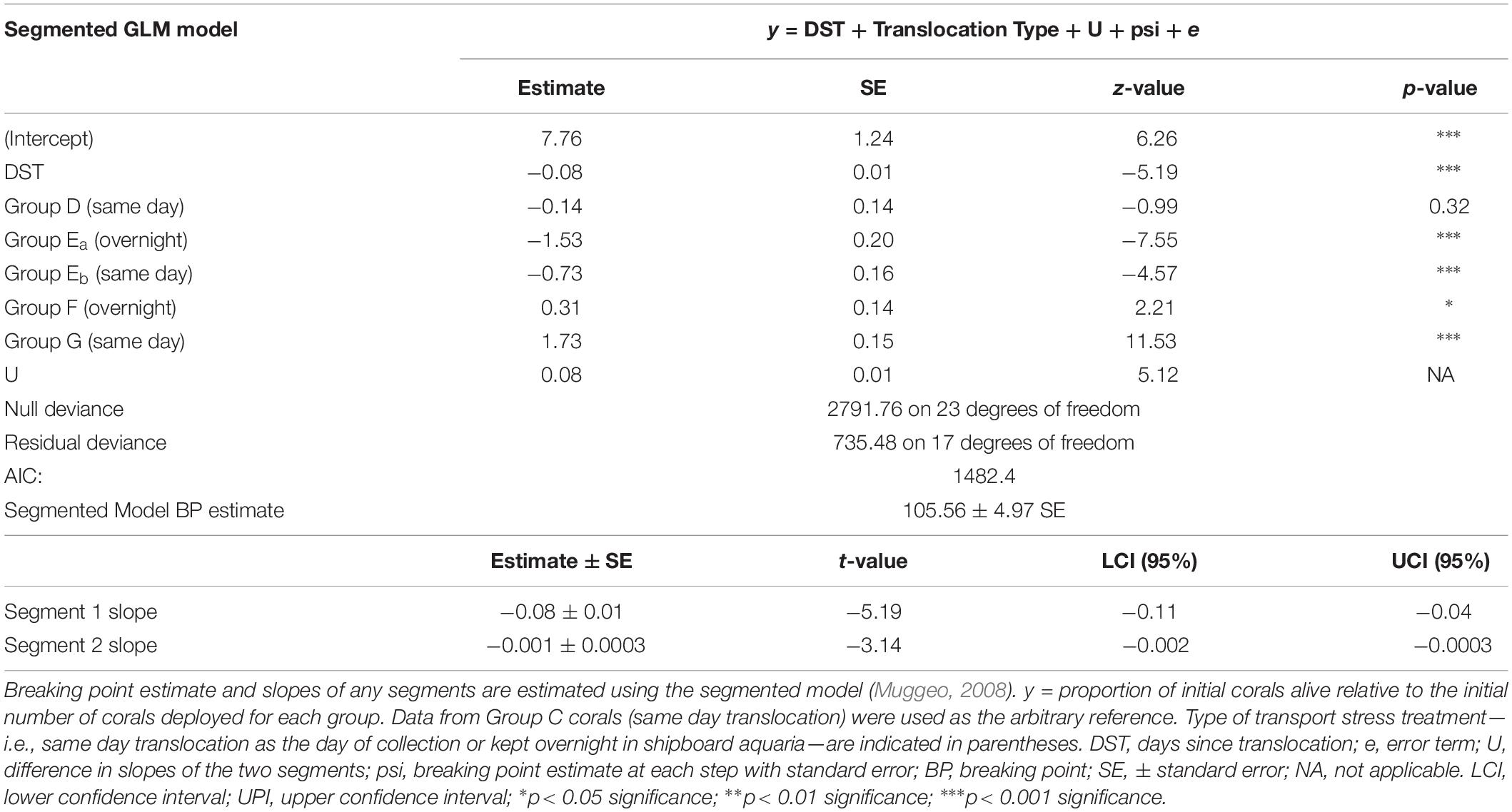
Table 3. Segmented generalized linear model (GLM) results and breaking point estimate for coral group survivorship over time.
Results of the cement attachment method (coral pot v.2) may have been partially due to a species-specific response. The precious coral Corallium sp. and the black coral S. kofoidi had the highest survivorship of all translocated coral taxa when translocated using coral pot v.2. Figure 3A shows an example of a Corallium sp. fragment with extended polyps indicating good “health” 133 days after translocation. All (100%) of the translocated Corallium sp. (n = 5) survived ca. one year and S. kofoidi exhibited high survival with 80–100% of the translocated corals surviving approximately 1–2 years (Figures 3B,C). Attachment of bamboo corals (Keratoisis sp. and I. tentaculum) using coral pot v.2 resulted in ∼30% and 0–50% survivorship respectively in the first year (Figures 3E,F). Attachment of bubble gum corals (P. arborea and S. cauliflora) resulted in the lowest survivorship over time for all corals deployed using coral pot v.2 (Figures 3G,H). 0–40% of P. arborea (Figure 3G, group C, Eb, and G) survived to the end of the first year. S. cauliflora coral fragments resulted in approximately 40% survivorship after the first year but ultimately declined to 0% over the subsequent year (Figure 3H, group C).
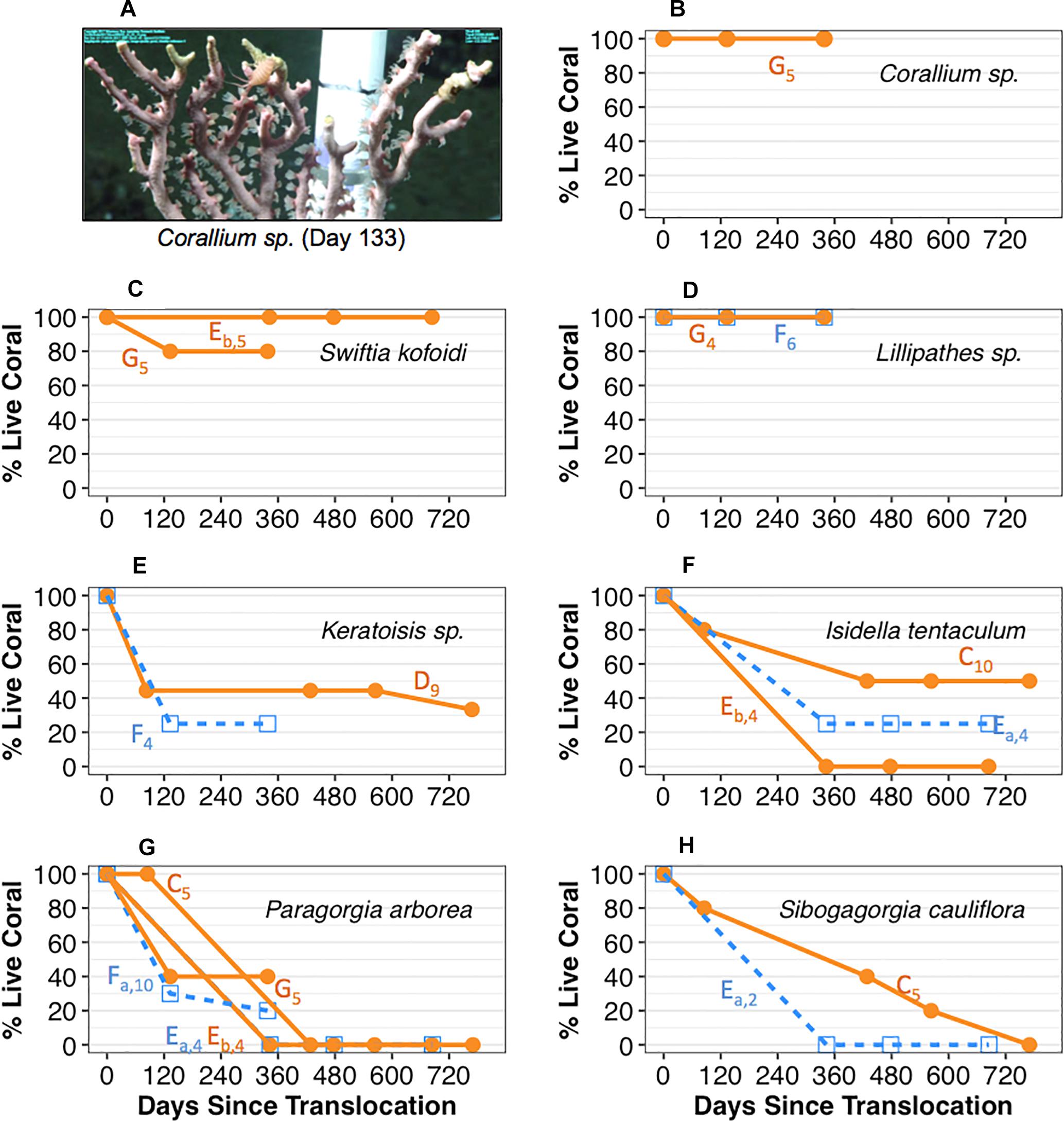
Figure 3. Survivorship by coral species and transport treatment (Sur Ridge, California). (A) An example image of Corallium sp. fragment with conspicuous polyp extension at 133 days post-translocation. (B) Percent live Corallium sp. fragments over time. (C) Percent live Swiftia kofoidi fragments over time. (D) Percent live Lillipathes sp. fragments over time. (E) Percent live Keratoisis sp. fragments over time. (F) Percent live Isidella tentaculum fragments over time. (G) Percent live Paragorgia arborea fragments over time. (H) Percent live Sibogagorgia cauliflora fragments over time. For panels (B–H), 0 days = the initial time when coral pots were translocated at depth. Open blue square data represent percent live of coral fragments that were exposed to overnight transport in shipboard aquaria; solid orange circle data represent survivorship for fragments that were translocated on the same day of collection. Coral group data by deployment are indicated by the letters next to each line along with the initial number of fragments at each deployment in subscript. All data represent results from deployment using the cement attachment method (coral pot v.2).
Transport Stress and Survivorship
Results from the GLM segmented model analysis indicate that proportional survivorship was not significantly dependent on coral deployment group or whether the corals had overnight treatment in shipboard aquaria (Figure 2B and Table 3). More specifically, while the survivorship rates of group D (same day translocation) did not significantly differ compared to the reference group C (same day translocation), the survivorship rates of groups E, F, and G did significantly differ regardless of transport duration treatment.
The responses to transport stress treatment may also have been partially due to a species-specific response. For the coral species tested for transport stress, survivorship due to treatment were mixed with the exception of Lillipathes sp. which resulted in 100% survivorship regardless of treatment (Figure 3D). For Keratoisis sp. and S. cauliflora, percent survivorship was generally lower for the coral fragments in the overnight treatment (Figures 3E,G,H; data denoted by open blue squares). Furthermore, survivorship of S. cauliflora declined more rapidly to 0% for the coral fragments in the overnight treatment (Figure 3H, group Ea) but this rapid decline may have been due to the limited samples (n = 2) with this deployment. However, survivorship of I. tentaculum and P. arborea due to transport stress treatment seemed to be mixed. For the former, 0–50% of the I. tentaculum [Figure 3F; group C (n = 10) and Eb (n = 4)] deployed survived ca. 2 years with the same day treatment but 25% survived the same amount of time when exposed to overnight treatment [Figure 3F; groups Ea (n = 4)]. For the latter coral species, corals deployed the same day treatment resulted in 0–40% survival (n = 5) whereas corals exposed to overnight treatment dropped to 0–20% at the end of the first year.
Discussion
Restoration of deep-sea communities is a new frontier for ocean science and resource management as human activities have increasingly broad and profound effects in the deep sea. The effects of trawling on deep-sea coral and sponge communities were perhaps the first deep-sea ecosystem impacts to be highlighted (Koslow et al., 2000; Van Dover, 2014; Van Dover et al., 2014; Amoroso et al., 2018). We now face continuing fishing impacts, as well as host of new local to global threats ranging from deep-sea mining to climate-linked changes in ocean conditions. The 2010 BP Deepwater Horizon oil spill and its enormous scope refocused our attention on anthropogenic impacts in the deep sea. Constraining further impacts using networks of Marine Protected Areas, a successful approach in shallow marine environments (McCook et al., 2010), shows promise, but has only been applied sparingly in the deep sea (Edgar et al., 2014). Beyond protection, active restoration of damaged deep-sea assemblages may provide meaningful mitigation, but has received little to no attention—this study is one of the first to explore methods to promote the recovery of impacted deep-sea coral populations by examining the performance of multiple coral taxa.
The high initial mortality of translocated corals observed in our investigation is similar to the general patterns observed in previous restoration studies of shallow water corals (Lasker, 1990; Epstein et al., 2001; Linares et al., 2008a, b; Edwards et al., 2010; Boch and Morse, 2012). High mortality rates shortly after translocation indicate that methods minimizing translocation stress should be one of the key factors to consider in future studies in both shallow and deep-sea environments. The variation in mortality among coral taxa observed also indicates that longer-term evaluations are needed and that successful translocation of multiple coral species will require different solutions depending on taxa. Furthermore, as the effects of habitat heterogeneity on deep-sea coral restoration success remain unknown, a more rigorous investigation of how the conditions at the source colony location and how the conditions at the translocation sites may influence the survivorship of translocated corals need further attention.
We recognize that we have not fully explored the full range of factors that may affect the survivorship of translocated coral fragments. Our results indicate that the attachment of coral fragments to a substrate may be critical for coral survival and different methods employed for mitigation efforts (e.g., zip tie, cement, or other unexplored options) may influence restoration success. Previous studies in shallow coral ecosystems indicate that the size of fragments (Linares et al., 2008b), variation in currents (Jokiel, 1978; Boch and Morse, 2012), protection from predation (Baria et al., 2010; Shaver et al., 2018), and having a nursery phase before transplanting (Shafir et al., 2006), can also have a major influence on the survival of translocated corals. Reproductive condition of translocated corals may also affect their survival and potentially the period required for corals to produce and release viable gametes. In our study, we observed that some polyps of Keratoisis sp., I. tentaculum, and Lillipathes sp. contained eggs at the time of initial translocation. Although eggs were visible within several translocated fragments of each of these species looked to be decreasing over time, additional studies are required to determine the survivorship and reproductive contribution as a function of initial reproductive condition.
Differences in survival rates among species were somewhat surprising. Bubblegum corals, which have broad, flexible proteinaceous branches, appeared robust during handling for translocation, but had very high rates of mortality compared to other taxa. Many factors may have contributed to high mortality rates, including the potentially toxic effects of cement or predation. While we did not have a persistent observation system to record any predatory behavior on the translocated corals, we did observe tissue sloughing from some bubble gum coral fragments (<4 months after translocation) followed by the fragments breaking off consistently at the base. Structural sensitivity of red gorgonian fragments at the base of fragments artificially attached to a natural substrate was discussed in a previous study; therefore high sensitivity at the point of attachment may be a general pattern for softer-bodied gorgonians (Linares et al., 2008a). In contrast, bamboo corals appeared to be highly fragile, but exhibited high survival up to ca. 2 year post-translocation. Thus, future studies should explore different attachment compounds and employ persistently present observation systems with a suite of sensors to help resolve questions related to factors such as attachment sensitivity and other factors such as flow and predation.
The cost of enhancing the restoration and recovery of deep-sea coral and sponge communities after anthropogenic disturbances will remain uncertain until all components of ecosystem services and the scale of active mitigation strategies can be explored. Spurgeon and Lindahl (2000) estimated that coral reef restoration costs could vary from US$13 K to US$100 M per hectare based on four case studies in shallow water coral reef systems. Additional estimates made on 10 case studies indicate that shallow water efforts have a median cost of ∼ US$500 K per hectare. In contrast, Van Dover et al. (2014) estimated that deep-sea restoration efforts will likely cost two to three orders of magnitude more than shallow water efforts based solely on direct costs. In our study, the use of the R/V Western Flyer and the ROV Doc Ricketts cost approximately US$30 k per day with the study site within 4 h from port. However, the cost of establishing viable translocation methods here should also include the costs of mapping the deep-sea coral and sponge communities and re-visiting mitigated areas so that outcomes of the translocation efforts can be assessed in a rigorous manner over time. Additional evaluations will also be needed to examine larger and cost-effective aquarium systems that minimize stress while transporting deep-sea corals over long distances and time periods, which may be necessary to mitigate large scale anthropogenic disturbances such as the BP Gulf of Mexico oil spill. Despite the need for further development, the relatively high survivorship of deep-sea coral fragments in pots constructed of low-cost (US$20) materials is a promising indication that developing active mitigation strategies for the DSCSCs could have merit. However, we also acknowledge that the use of PVC and cement materials is not an ideal module as a permanent transportable solution and additional bio-friendly materials will need to be explored.
The long-term survival of translocated corals, as well as their effect on DSCSC recovery over decades to centuries is yet to be determined. Considering the slow growth rates and high longevity of deep-sea corals (Andrews et al., 2002, 2005; Roark et al., 2005), it is natural to question if coral translocation will likely accelerate the recovery of damaged DSCSCs. Additionally, understanding the impacts of sourcing coral fragments from “healthy” versus “unhealthy” or “dying” donor colonies will be a critical step prior to implementation. For example, transplanting corals by fragmenting a limited number of source colonies versus fragmenting a limited number of corals that are prolific in one area to mitigate a disturbed area are likely to have different impacts on the source population but these questions have not been studied in the deep sea. Despite the gaps in knowledge, we will need to ask what role active mitigation will play in response to past, current, and future changes in the ocean due to increased human activity. Will establishing cost-effective restoration approaches that enhance gamete contact and approaches that generate corals that are more resilient to climate-related changes in ocean conditions better prepare deep-sea ecosystems for the future? Perhaps efforts to propagate other key associated taxa such as sponges that may enhance energy flow and carbon sequestration could help mitigate climate change driven changes in the deep sea (Murray et al., 1994; Cathalot et al., 2015; Kahn et al., 2015). Overall, the most effective strategies for mitigating damage in DSCSCs are uncertain but exploring the potential value of restoration options such as coral translocation and other approaches will help shape our efforts to protect and sustain these valuable and fragile deep-sea resources.
Author Contributions
CB, ADV, and JB designed the study. CB did the statistical analysis. CB, JB, ADV, EB, CK, JL, and CL conducted the translocation experiments. All authors listed made a substantial contribution to the writing of the manuscript.
Funding
This work was supported by the David and Lucile Packard Foundation through MBARI project 901007 and the Save the Earth Foundation.
Conflict of Interest Statement
The authors declare that the research was conducted in the absence of any commercial or financial relationships that could be construed as a potential conflict of interest.
Acknowledgments
We are extremely grateful to the crew of the R/V Western Flyer and ROV Doc Ricketts pilots. We are also grateful to the volunteers who helped with the deep-sea coral restoration study.
Supplementary Material
The Supplementary Material for this article can be found online at: https://www.frontiersin.org/articles/10.3389/fmars.2019.00540/full#supplementary-material
Footnotes
- ^ https://sanctuaries.noaa.gov/science/conservation/sur-ridge-field-guide-monterey-bay-national-mairne-sanctuary.html
References
Amoroso, R. O., Pitcher, C. R., Rijnsdorp, A. D., McConnaughey, R. A., Parma, A. M., Suuronen, R., et al. (2018). Bottom trawl fishing footprints on the world’s continental shelves. Proc. Natl. Acad. Sci. U.S.A. 115, E10275–E10282. doi: 10.1073/pnas.1802379115
Andrews, A. H., Caillet, G. M., Kerr, L. A., Coale, K. H., Lundstrom, C., and DeVogelaere, A. P. (2005). “Investigation of age and growth for three deep-sea corals from the Davidson Seamount off central California,” in Cold-Water Corals and Ecosystems. Erlangen Earth Conference Series, eds A. Freiwalk and J. M. Roberts (Berlin: Springer-Verlag).
Andrews, A. H., Cordes, E. E., Mahoney, M. M., Munk, K., Coale, K. H., Caillet, G. M., et al. (2002). Age, growth and radiometric age validation of a deep-sea, habitat-forming gorgonian (Primnoa resedaeformis) from the Gulf of Alaska. Hydrobiologia 471, 101–110.
Baillon, S., Hamel, J. F., Wareham, V. E., and Mercier, A. (2012). Deep cold-water corals as nurseries for fish larvae. Front. Ecol. Environ. 10, 351–356. doi: 10.1890/120022
Baria, M. V. B., Guest, J. R., Edwards, A. J., Alino, P. M., Heyward, A. J., and Gomez, E. D. (2010). Caging enhance post-settlement survival of juveniles of the scleractinian coral Acropora tenuis. J. Exp. Mar. Biol. Ecol. 394, 149–153. doi: 10.1016/j.jembe.2010.08.003
Barton, J. A., Willis, B. L., and Hutson, K. S. (2015). Coral propagation: a review of techniques for ornamental trade and reef restoration. Rev. Aquacult. 9, 238–256. doi: 10.1111/raq.12135
Boch, C. A., and Morse, A. N. C. (2012). Testing the effectiveness of direct propagation techniques for coral restoration of Acropora spp. Ecol. Eng. 40, 11–17. doi: 10.1016/j.ecoleng.2011.12.026
Bourque, J. R., and Demopoulos, A. W. J. (2018). The influence of different deep-sea coral habitats on sediment macrofaunal community structure and function. PeerJ. 6:e5276. doi: 10.7717/peerj.5276
Bowden-Kerby, A. (2001). Low-tech coral reef restoration methods modeled after natural fragmentation processes. Bull. Mar. Sci. 69, 915–931.
Brooke, S., Koenig, C. C., and Shephard, A. N. (2006). “Oculina banks restoration project: description and preliminary assessment,” in Proceedings of the 57th Gulf and Caribbean Fisheries Institute, St. Petersburg, FL. 607–620.
Brooke, S., and Young, C. M. (2009). In situ measurement of survival and growth of Lophelia pertusa in the northern Gulf of Mexico. Mar. Ecol. Prog. Ser. 397, 153–161. doi: 10.3354/meps08344
Bruno, J. F., and Bertness, M. D. (2000). “Habitat modification and facilitation in benthic marine communities,” in Marine Community Ecology, eds M. D. Bertness, S. Gaines, and M. E. Hay (Sunderland: Sinaeur), 201–218.
Bruno, J. F., Stachowicz, J. J., and Bertness, M. D. (2003). Inclusion of facilitation into ecological theory. Trends Ecol. Evol. 18, 119–125. doi: 10.1016/s0169-5347(02)00045-9
Cathalot, C., Van Oevelen, D., Cox, T. J. S., Kutti, T., Lavaleye, M., Duineveld, G., et al. (2015). Cold-water coral reefs and adjacent sponge grounds: hotspots of benthic respiration and organics carbon cycling in the deep sea. Front. Mar. Sci. 2:37. doi: 10.3389/fmars.2015.00037
Edgar, G. J., Stuart-Smith, R. D., Willis, T. J., Kininmonth, S., Baker, S. C., Banks, S., et al. (2014). Global conservation outcomes depend on marine protected areas with five key features. Nature 506, 216–220. doi: 10.1038/nature13022
Edwards, A. J., Job, S., and Wells, S. (2010). “Learning lessons from past reef-rehabilitation projects,” in Reef Rehabilitation Manual, ed. A. J. Edwards (St. Lucia: Coral Reef Targeted Research & Capacity Building for Management Program), 129–136.
Epstein, N., Bak, R. P. M., and Rinkevich, B. (2001). Strategies for gardening denude coral reef areas: the applicability of using different types or coral material for reef restoration. Restor. Ecol. 9, 432–442. doi: 10.1046/j.1526-100x.2001.94012.x
Horoszowski-Fridman, Y. B., Izhaki, I., and Rinkevich, B. (2011). Engineering of coral reef larval supply through transplantation of nursery-farmed gravid colonies. J. Exp. Mar. Biol. Ecol. 399, 162–166. doi: 10.1016/j.jembe.2011.01.005
Jokiel, P. L. (1978). Effects of water motion on reef corals. J. Exp. Mar. Biol. Ecol. 35, 87–97. doi: 10.2307/1542017
Kahn, A. S., Yahel, G., Chu, J. W., Tunnicliffe, V., and Leys, S. P. (2015). Benthic grazing and carbon sequestration by deep-water glass sponge reefs. Limnol. Oceanogr. 60, 78–88. doi: 10.1002/lno.10002
Koenig, C. C., Shephard, A. N., Reed, J. K., Coleman, F. C., Brooke, S. D., Brusher, J., et al. (2005). Habitat and fish populations in the deep-sea Oculina coral ecosystem of the Western Atlantic. Am. Fish. Soc. Symp. 41, 795–805.
Koslow, J. A., Boehlert, G. W., Gordon, M., Haedrich, R. L., Lorance, P., and Parin, N. (2000). Continental slope and deep-sea fisheries: implication for a fragile ecosystem. ICES J. Mar. Sci. 57, 548–557. doi: 10.1006/jmsc.2000.0722
Lasker, H. R. (1990). Clonal propagation and population dynamics of a gorgonian coral. Ecology 71, 1578–1589. doi: 10.2307/1938293
Linares, C., Coma, R., Mariani, S., Diaz, D., Hereu, B., and Zabala, M. (2008a). Early life history of the Mediterranean gorgonian Paramuricea clavata: implication for population dynamics. Invertebr. Biol. 127, 1–11. doi: 10.1111/j.1744-7410.2007.00109.x
Linares, C., Coma, R., and Zabala, M. (2008b). Restoration of threatened red gorgonian populations: an experimental and modeling approach. Biol. Conserv. 141, 427–437. doi: 10.1016/j.biocon.2007.10.012
Love, M., Yoklavich, M. M., Black, B. A., and Andrews, A. H. (2007). Age of black coral (Antipathes dendrochristos) colonies, with notes on associated invertebrate species. Bull. Mar. Sci. 80, 391–400.
McCook, L. J., Ayling, T., Cappo, M., Choat, J. H., Evans, R. D., De Freitas, D. M., et al. (2010). Adaptive management of the Great Barrier Reef: a globally significant demonstration of the benefits of networks of marine reserves. Proc. Natl. Acad. Sci. U.S.A. 107, 18278–18285. doi: 10.1073/pnas.0909335107
McCrea-Strub, A., Kleisner, K., Sumaila, U. R., Swartz, W., Watson, R., Zeller, D., et al. (2011). Potential impact of the Deepwater Horizon oil spill on commercial fisheries in the Gulf of Mexico. Fisheries 36, 332–336. doi: 10.1007/s00244-018-0568-0
Muggeo, V. M. R. (2003). Estimating regression models with unknown break-points. Stat. Med. 22, 3055–3071. doi: 10.1002/sim.1545
Muggeo, V. M. R. (2008). Segmented: an R package to fit regression models with broken-line relationships. R News 8, 20–25.
Murray, J. W., Barber, R. T., Roman, M. R., Bacon, M. P., and Feely, R. A. (1994). Physical and biological controls on carbon cycling in the equatorial Pacific. Science 266, 58–65. doi: 10.1126/science.266.5182.58
Rinkevich, B. (2008). Management of coral reefs: we have gone wrong when neglecting active reef restoration. Mar. Pollut. Bull. 56, 1821–1824. doi: 10.1016/j.marpolbul.2008.08.014
Roark, E. B., Guilderson, T. P., Flood-Page, S., Dunbar, R. B., Ingram, B. L., Fallon, S. J., et al. (2005). Radiocarbon-based ages and growth rates of bamboo corals from the Gulf of Alaska. Geophys. Res. Lett. 32:L04606. doi: 10.1029/2004GL021919
Rogers, A. D. (1999). The biology of Lophelia pertusa (Linnaeus 1758) and other deep-water reef-forming corals and impacts from human activities. Int. Rev. Hydrobiol. 84, 315–406. doi: 10.1002/iroh.199900032
Shafir, S., Van Rijn, J., and Rinkevich, B. (2006). Steps in the construction of underwater coral nursery, an essential component in reef restoration acts. Mar. Biol. 149, 679–687. doi: 10.1007/s00227-005-0236-6
Shaver, E. C., Burkepile, D. E., and Silliman, B. R. (2018). Local management actions can increase coral resilience to thermally-induced bleaching. Nat. Ecol. Evol. 2, 1075–1079. doi: 10.7924/G8348HFP
Spieler, R. E., Gilliam, D. S., and Sherman, R. L. (2001). Artificial substrate and coral reef restoration: what do we need to know to know what we need. Bull. Mar. Sci. 69, 1013–1030.
Spurgeon, J. P. G., and Lindahl, U. (2000). “Economics of coral reef restoration,” in Collected Essays on the Economics of Coral Reefs, ed. H. S. J. Cesar (Mombasa: CORDIO), 125–136.
Stone, R. P. (2006). Coral habitat in the Aleutian Islands of Alaska: depth distribution, fine-scale species associations, and fisheries interactions. Coral Reefs 25, 229–238. doi: 10.1007/s00338-006-0091-z
Sumaila, U. R., Cisneros-Montemayor, A. M., Dyck, A., Huang, L., Cheung, W., Jacquet, J., et al. (2012). Impact of the deepwater horizon well blowout on the economies of US Gulf fisheries. Can. J. Fish. Aquat. Sci. 69, 499–510. doi: 10.1139/f2011-171
Van Dover, C. L. (2014). Impacts of anthropogenic disturbances at deep-sea hydrothermal vent ecosystems: a review. Mar. Environ. Res. 102, 59–72. doi: 10.1016/j.marenvres.2014.03.008
Van Dover, C. L., Aronson, J., Pendleton, L., Smith, S., Arnaud-Haond, S., Moreno-Mateos, D., et al. (2014). Ecological restoration in the deep sea: desiderata. Mar. Policy 44, 98–106. doi: 10.1016/j.marpol.2013.07.006
Villanueva, R. D., Baria, M. V. B., and dela Cruz, D. W. (2012). Growth and survivorship of juvenile corals outplanted to degraded reef areas in Bolinao-Anda Reef Complex, Philippines. Mar. Biol. Res. 8, 877–884. doi: 10.1080/17451000.2012.682582
Keywords: deep-sea coral restoration, Corallium sp., Lillipathes sp., Swiftia kofoidi, Keratoisis sp., Isidella tentaculum, Paragorgia arborea, Sibogagorgia cauliflora
Citation: Boch CA, DeVogelaere A, Burton E, King C, Lord J, Lovera C, Litvin SY, Kuhnz L and Barry JP (2019) Coral Translocation as a Method to Restore Impacted Deep-Sea Coral Communities. Front. Mar. Sci. 6:540. doi: 10.3389/fmars.2019.00540
Received: 18 January 2019; Accepted: 16 August 2019;
Published: 28 August 2019.
Edited by:
Emanuele Di Lorenzo, Georgia Institute of Technology, United StatesReviewed by:
Andrew D. Thaler, Blackbeard Biologic Science and Environmental Advisors, United StatesErik Cordes, Temple University, United States
Copyright © 2019 Boch, DeVogelaere, Burton, King, Lord, Lovera, Litvin, Kuhnz and Barry. This is an open-access article distributed under the terms of the Creative Commons Attribution License (CC BY). The use, distribution or reproduction in other forums is permitted, provided the original author(s) and the copyright owner(s) are credited and that the original publication in this journal is cited, in accordance with accepted academic practice. No use, distribution or reproduction is permitted which does not comply with these terms.
*Correspondence: Charles A. Boch, Y2JvY2hAbWJhcmkub3Jn