- Département des Sciences Naturelles, Université du Québec en Outaouais, Gatineau, QC, Canada
Background: Commercial shipping is identified as a major source of anthropogenic underwater noise in several ecologically sensitive areas. Any development project likely to increase marine traffic can thus be required to assess environmental impacts of underwater noise. Therefore, project holders are increasingly engaging in underwater noise modeling relying on ships' underwater noise source levels published in the literature. However, a lack of apparent consensus emerges from the scientific literature as discrepancies up to 30 dB are reported for ships' broadband source levels belonging to the same vessel class and operating under similar conditions. We present a statistical meta-analysis of individual ships' broadband source levels available in the literature so far to identify which factors likely explain these discrepancies.
Methods: We collated ships' source levels from the published literature to construct our dataset. A Generalized Linear Mixed Model was applied to the dataset to statistically assess the contribution of intrinsic (i.e., related to ships' static and dynamic attributes) and extrinsic factors (i.e., related to both the protocol for hydroacoustic data acquisition and the noise data reduction procedure) to the reported broadband source levels.
Results: Amongst intrinsic factors, ships' speed-over-ground , ships' width , and ships' class (−6.07 to 2.08 dB; p-value ∈ [< 0.001 to 0.036]) have shown the strongest correlations with broadband source levels. The hydrophone-to-source closest point of approach and the correction for surface-image reflections (21.73 dB; p-value = 0.002) contribute the most to explain the reported ships' broadband source levels' variability amongst extrinsic factors.
Conclusions: Our meta-analysis confirms a consensus that speed regulation can effectively reduce instantaneous ships' source levels. Neglecting Lloyd's mirror effects through the abuse of non-corrected spreading laws for propagation loss directly leads to a generalized under-estimation of the ships' source levels retrieved from the literature. This could eventually be addressed by a wider adoption of standardized methods of hydrophone-based sound recordings and of data processing to homogenize results and facilitate their interpretation to conduct environmental impact assessment.
1. Introduction
The exposure of marine life (e.g., fishes, invertebrates, mammals) to anthropogenic noise remains a worldwide environmental issue for marine ecosystems (Williams et al., 2015). The magnitude of the underwater radiated noise attributed to the merchant fleet has shown a monotonic increase over the past few decades (Nolet, 2017) forcing the international authorities to suggest recommendations and new laws of mitigation in order to maintain control of this trend (IMO, 2014). Recently, commercial shipping activities, regarded as the main contributor to the anthropogenic underwater noise budget, have shown constant year-to-year increases and are a global-wide phenomenon, hence adding to the concerns (Clark et al., 2009).
The impact of the shipping noise on marine mammals is of critical importance considering the vital role of acoustics for numerous species. Anthropogenic noise alters the social behavior of marine mammals (Gomez et al., 2016), masks their communications (Erbe et al., 2016), and impedes their ability to appropriately forage (Tyack et al., 2011). Instantaneous high-amplitude events or long-term exposition to continuous underwater noise can lead to temporary or permanent injuries to their auditory system (NOAA, 2015).
In Canada, for many species listed as endangered according to Canada's Species at Risk Act (2002), underwater noise of anthropogenic origin is already being regarded as a threat to their recovery and is identified as such in their recovery plans. In the St. Lawrence River (Québec, Canada), anthropogenic underwater noise is thus identified as a threat to the recovery of both the St. Lawrence Estuary beluga population and the Northwest Atlantic blue whale.
In this context and considering the expected increase of the maritime traffic (Kaplan and Solomon, 2016), especially in the St. Lawrence River (Gouvernement du Québec, 2015), multi-stakeholder processes are underway to identify options to mitigate merchant ships' underwater radiated noise (e.g., Audoly et al., 2014). However, a prerequisite to an underwater noise reduction campaign concerns the ability to properly measure sound pressure through hydrophone-based observations and to accurately estimate the ships' source levels, a challenge undertaken worldwide by several research groups (Audoly et al., 2014; MacGillivray et al., 2019).
In order to quantify the underwater radiated noise from merchant ships through opportunistic hydrophone-based observations, numerous steps must be carefully carried out including the choice of hydrophones, location, and an accurate hydrophone calibration (Robinson et al., 2014). This also involves a detailed understanding of the complex physical processes and their mathematical representation that describe the acoustic propagation in anisotropic underwater environments (Erbe et al., 2016).
Merchant ships' underwater noise have several origins, the principal being machinery, propellers, and cavitation (Audoly et al., 2017). It is well-known that variations in merchant ships' source levels exist inside a given vessel class and from one class to another (see e.g., Figure 2 of Veirs et al., 2016). Proper characteristics to each ship (e.g., architecture, type of engines, maintenance of the propellers and hull) and conditions of operation (e.g., speed, load) can have an impact on the source levels. For this work, these factors will be referred to as intrinsic in a sense that they originate from the ships' own static and dynamic characteristics.
Alternatively, large, and often intra-class, discrepancies on source levels are reported in the literature, hence suggesting a certain uncertainty on the measurements attributed to factors extrinsic to the ships themselves. These extrinsic factors refer to the data campaign protocol and the mathematical calculations required to convert received levels of sound at the hydrophones into source levels at the position of the ship's position. To list a few, we can think about the experimental design for data acquisition (e.g., hydrophones' locations) or how certain physical processes (e.g., surface-image reflections) are handled during the data processing phases. Although underwater radiated noise propagation is directly related to the medium's chemical and geophysical properties, measurements of source levels found in the literature are usually reported without corresponding error bars or uncertainties, hence making study-to-study comparison quite complicated.
In this context where regulators, natural resources and conservation managers are required to identify new ways to attenuate the underwater radiated noise attributed to the merchant fleet in a growing number of ecologically sensitive areas, the important variability in the results reported by acoustic experts in the literature needs further investigation. This motivated a meta-analysis to shed some light on the apparent discrepancies reported for source levels of merchant ships and to identify the contribution of quantifiable intrinsic and extrinsic factors responsible for those.
2. Definitions and Scope
In this work, merchant ships include bulk carriers, unclassified cargo ships1, container ships, passenger ships, tankers, and vehicles carriers. We followed the terminology regarding ships' source levels described in ISO 17208-1 (2016), ISO 18405 (2017), and ISO 17208-2 (2019).
1. Radiated Noise Level (RNL): Level of the product of the distance from a ship reference point of a sound source and the far field root-mean-square sound pressure at that distance for a specified reference value.
2. Monopole Source Level (MSL): Mean-square sound pressure level at a distance of 1 m from a hypothetical monopole source, placed in a (hypothetical) infinite uniform lossless medium.
Source levels derived from underwater recordings that neglect surface-image reflections (i.e., Lloyd's mirror effects) are said to be RNL measurements. MSL values can be retrieved, in first approximation, using correction factors (listed in Appendix A of ISO 17208-2, 2019) applied to RNL measurements that were previously obtained using the standardized protocol described in ISO 17208-1 (2016). Higher precision can be obtained by using numerical algorithms (Collins, 1993; Porter and Liu, 1994) for the backpropagation of the received levels of noise instead of relying on the distance normalization of the standard spherical geometrical wave dilution. This latter method not only corrects for surface-image reflections but also compensates for the wave absorption of the seabottom sediments, bathymetric variations and channeling effects attributed to speed-of-sound gradients.
3. Objectives
The aims are to:
1.(a) Identify published studies that provide frequency-integrated (i.e., broadband) source levels for individual merchant ships;
(b) Characterize inter-study variability in source level measurements;
(c) Collate the data related to field campaigns and data processing.
2. Identify the contribution of quantifiable intrinsic and extrinsic factors that statistically explain the variability of the source level measurements. Emphasis will be accorded to extrinsic factors that can be objectively estimated from the information provided in each study. This excludes the precision of the hydrophone calibration as details of the pre-observations lab manipulations are rarely discussed in the selected studies.
3. Characterize the contribution of the ships' speed to the overall noise budget reported in the studies. Transiting speed is of particular interest as it appears to be a manageable factor to reduce the ships' radiated noise.
By achieving these objectives, we will provide key information and clarification about the interpretation of the ships' source levels reported in the literature. This will support ongoing management processes seeking to understand and mitigate the ships' radiated noise. This work will also be informative for the noise modeling endeavors carried out in the context of environmental impact assessment (e.g., Chion et al., 2017; Pennucci and Jiang, 2018).
4. Method
To identify studies that report opportunistic source level measurements of individual merchant ships and to quantitatively explore their variability, we first carried out a literature review using a keywords approach in databases of scholarly literature (Google Scholar, Scopus). The query used on these search engines is here listed as:
1. “Ship” AND
2.(a) “source levels” OR
(b) “sound signature” OR
(c) “acoustic signature” OR
(d) “noise signature” OR
(e) “radiated noise.”
A close examination of the list of references of each returned hit was also carried out in order to identify articles and reports that have failed to be returned by the keywords combination mentioned above. Only studies displaying broadband source level measurements in units of dB re 1 μPa · m for individual merchant ships were selected. All source level measurements for single recordings were gathered in a unique datasheet in order to investigate agreements and discrepancies between studies and conduct subsequent analysis.
The fact that each selected study has its own protocol/methodology for data acquisition creates a non-independence of the datasheet's intra-study data i.e., a measurement taken from a specific study will likely be more similar to another measurement taken from the same study than a measurement taken arbitrarily from another study. This signifies that a standard generalized linear model (GLM) cannot be used in order to estimate how intrinsic and extrinsic factors contribute to explain the variability in reported ships' source levels. In our case, the data non-independence requires the use of a generalized linear mixed model (GLMM). The term “mixed” indicates that the model implies the use of at least one fixed effect (i.e., a variable for which we wish to quantify the effect on reported source levels) and at least one random effect (authors-specific in our case). Random effects are not calculated but they are used to indicate to the model that intra-study data are not independent which results in a proper estimation of the model's residual deviation and a non-biased quantification of the fixed variables' uncertainty.
GLMM analysis was conducted with the function lmer of the lme4 package (Bates et al., 2015) using RStudio version 1.1.442 with R version 3.4.4. Confidence intervals and p-values (via Wald-statistics approximation) were calculated with the function sjt.lmer of the sjPlot package (Lüdecke, 2018). GLMMs were run using different combinations of fixed variables in order to minimize the Akaike information criterion (AIC) and explore the contribution of intrinsic and extrinsic factors to the variability in source level measurements. More specifically, extrinsic factors, in terms of methodological and technical parameters (see Table 1), will be regarded as possible sources for the inter-study variability.
Finally, we reviewed how the different studies characterized the relationship between ships' speed and source levels, either based on broadband measurements or from empirical models for ships' RNL/MSL predictions. This will deepen our understanding of the role played by speed on the ships' radiated noise.
5. Results
5.1. Characterization of the Selected Studies
All in all, 2,275 single transits from 9 different studies are reported in this work. Technical details for each recording missions and a description of the data presented by each study are provided in Tables 1, 2, respectively.
We emphasize, in Table 1, that all but one studies retained for this work backpropagated their received levels of noise to the sources' positions using variations of the geometrical spreading model while none of them reported having used corrections for surface-image reflections. From these specific studies, the retrieved RNLs (see section 2) are said to be surface-affected by the Lloyd's mirror effects (see e.g., Gassmann et al., 2017) and are hence referred to as dipole observations. SMRU Canada (2014) displays surface-corrected MSL measurements (see section 2) referred to as monopole observations in Table 1.
Vessel classes explored in this work are listed in italic in the right-hand column of Table 2. Whisker plots of each sample of merchant ships are plotted in Figure 1, hence illustrating the variability in broadband source level measurements between each study.
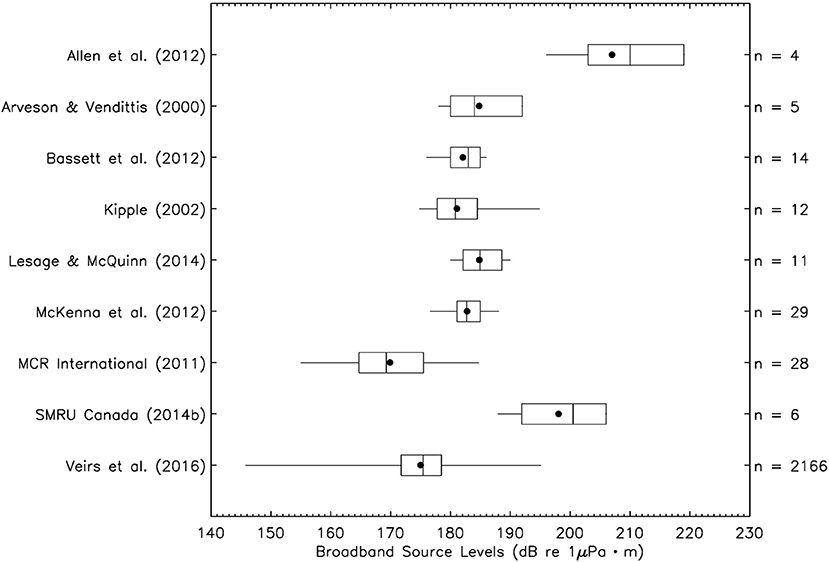
Figure 1. Broadband source levels for merchant ships reported from studies listed in Table 1. The right-hand ordinate provides the number of vessels with available data published in each work. Whisker plots show the minimal value, the 25, 50, 75% quartiles, the maximal value, and the mean () of each sample.
5.2. Factors Explaining the Ships' Source Levels' Variability
Intrinsic factors available for the GLMM analysis are the ships' length (ℓ), width (b), speed (v), and classes (see section 2). Draft (d) and water displacement (∝ ℓ × b × d) were not considered since the d parameter is missing from the Veirs et al.'s (2016) database (which accounts for the large majority of the 2,275 transits used in this work). The general consensus suggests that the logarithm of intrinsic factors, besides ships' classes, should be used to predict source level values (see Table A1).
Since we have no indications on how source level measurements behave with extrinsic factors that are linked to the methodological parameters and techniques of data processing, we chose to include them as linear predictors to the GLMM analysis. The extrinsic factors tested in the GLMM analysis are the lower and upper thresholds of the hydrophones' frequency bandwidth (f0, f1), the distance corresponding to the closest point of approach, the height of the water column at the hydrophones' position, the hydrophones' deployment depth, and the source approximation (i.e., whether RNL or MSL values were obtained). For the height of the water column and the deployment depth, we chose the largest value when an interval or more than one value are listed in Table 1. The source approximation was quantified as a standard Heaviside function that equals 1 when MSL values were gathered and 0 otherwise.
Different combinations of log10(intrinsic factors) + extrinsic factors were tested in an attempt to minimize the AIC using authors-specific random effects (see section 4). This was achieved using the v, b, class, CPA, and source approximation parameters while the 4 outliers provided by Allen et al. (2012) were ignored hereafter. The best-fitted model's coefficients are provided in Table 3 and Equation (1) which indicate the correlation between intrinsic/extrinsic factors with the ships' source level values.
where v0 = 1 knot, b0 = 1 meter, r0 = 1 nautical mile are reference values, and:
where the class reference was bulk carriers. Note that, in GLMM, qualitative parameters are always compared to the group's first element in alphabetical order.
Equation (3) suggests that cargo and container ships may have the noisiest acoustical footprint which agrees with the results presented by Jalkanen et al. (2018).
5.3. Ships' Speed vs. Source Levels Relation
This subsection explores the linearity between log10(v) and source levels according to (1) the observational data collected in this work (see section 5.3.1) and (2) the empirical models for source level predictions available in the literature (see section 5.3.2).
5.3.1. Observations
Speed is an intrinsic factors that can be regulated in order to favor an instantaneous noise attenuation of the merchant fleet. Our GLMM analysis revealed a positive correlation between a ship's source levels and speed. Therefore, a deeper analysis of the ships' speed vs. source levels relation is here developed by individually investigating each selected study. Results are provided in the upper panel of Table 4 and highly suggest a positive correlation between the magnitude of the RNL/MSL measurements and the ships' speed. Slopes, in the log10(v)-space, range from 11.71 to 49.94 with a median of roughly 21 that agrees relatively well with the estimate found in Table 3. Authors typically point out however that this relation is subject to variations from one ship to another, some ships are even likely to produce more noise at speeds below their optimal cruising speed.
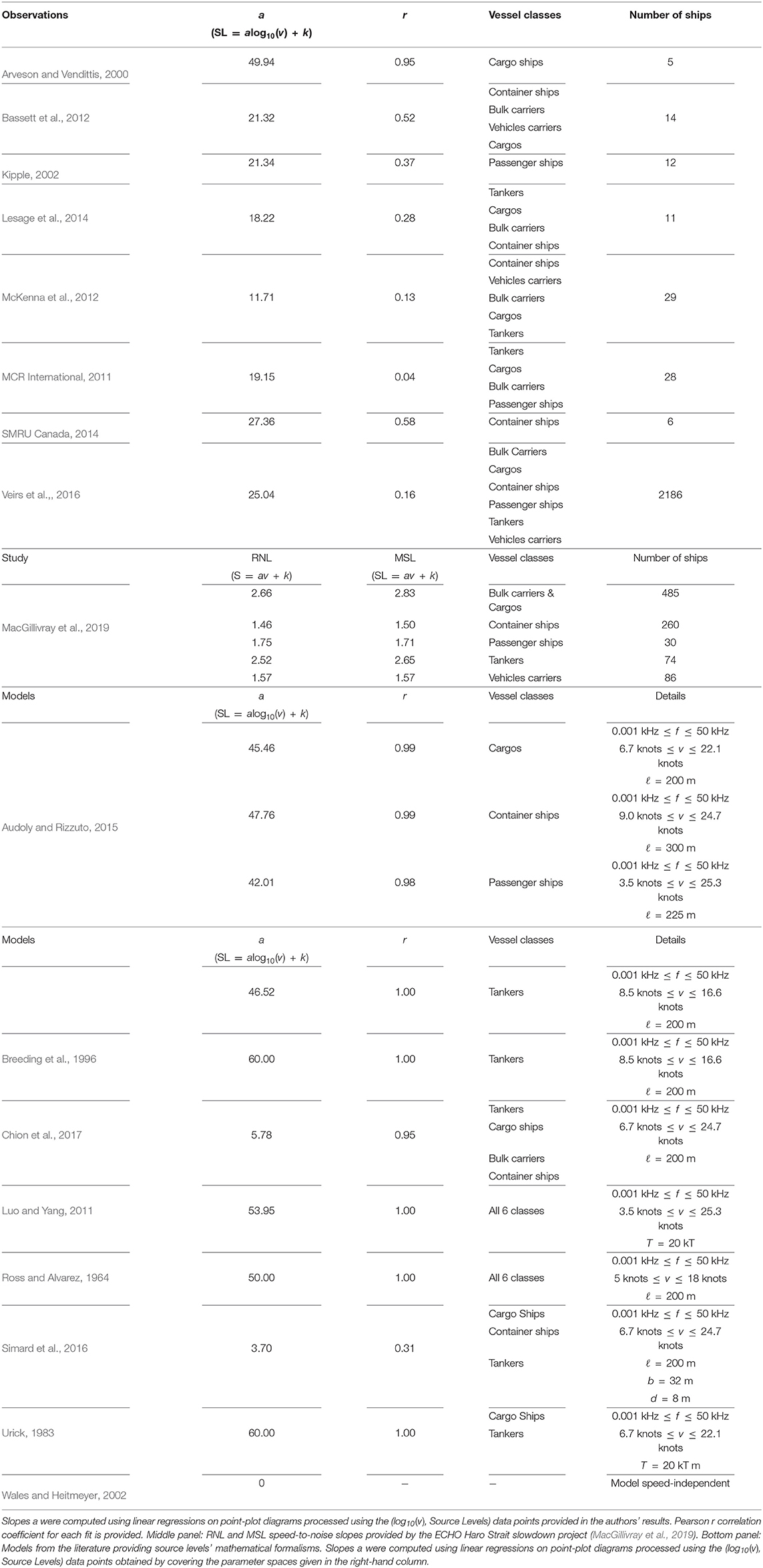
Table 4. Source levels vs. ships' Speed Relation. Upper panel: Observational studies listed in Tables 1, 2.
A study properly engineered to investigate the impact of a speed reduction on the ambient noise and the noise emitted by merchant ships was recently conducted under the Echo Program in the water basin of the Port of Vancouver (MacGillivray et al., 2019). This voluntary vessel slowdown trial provided source level measurements for merchant ships both inside and outside a speed reduction area in which the proposed speed limit was 11 knots. The source levels' variations of transiting ships were therefore solely attributed to a speed decrease since all other ship-related factors were kept constant between measurements. This approach makes the Port of Vancouver's vessel slowdown trial a valuable source of information in order to understand the impact of speed regulation on the levels of noise emitted by merchant ships. Both RNL and MSL noise-to-speed slopes were provided by the authors and are listed in Table 4's middle panel.
MacGillivray et al. (2019) reveals that a 40% speed reduction in this sector results in a MSL decrease of about 10 dB. Slopes are typically a factor 2–4 steeper than what we found for data in Table 3 (if GLMMs are processed with a source levels ∝ v model). Depending on the ship class, the noise-to-speed slopes vary from 1.4 to 2.8 dB knot−1 and appear to be slightly steeper for MSL measurements when compared to RNLs. A similar behavior can also be retrieved from Table 4's upper panel with the SMRU Canada (2014) study showing the second steepest relation between source levels and log10(v).
Even though a variability exists from one study to another, a large consensus seems established in the scientific community that speed reduction does indeed favors a decrease of the noise budget attributed to the merchant fleet (e.g., Audoly et al., 2017). However, this reduction of the instantaneous underwater noise radiated comes at the expense of an increase of the time spent by ships in a speed-restricted zone, hence potentially exposing nearby marine mammals to noise pollution for longer periods of time (McKenna et al., 2013; Chion et al., 2017).
5.3.2. Models
Empirical source level models listed in Table 5 can also be used to estimate how broadband ships' source levels vary with speed changes. As in Table 2, vessel classes explored in this work are listed in italic in Table 5. Results of the source levels ∝ log10(v) regressions are provided in the bottom panel of Table 4. All models that numerically depend on the speed parameter predict an increase of the noise radiated with increasing speed. Models were tested for standard dimensions in terms of length, width, draft, and water displacement (see the right-hand column in Table 4's bottom panel). Speed limits correspond to the minimal and maximal speeds retrieved, for each specific vessel class, from our 2,275 ships database (see Supplementary Material). The noise-to-speed slopes, in the log10(v)-space, range between 3.7 and 60, with a mean value of 48, roughly three times steeper than what was obtained from the GLMM approach in Table 3.
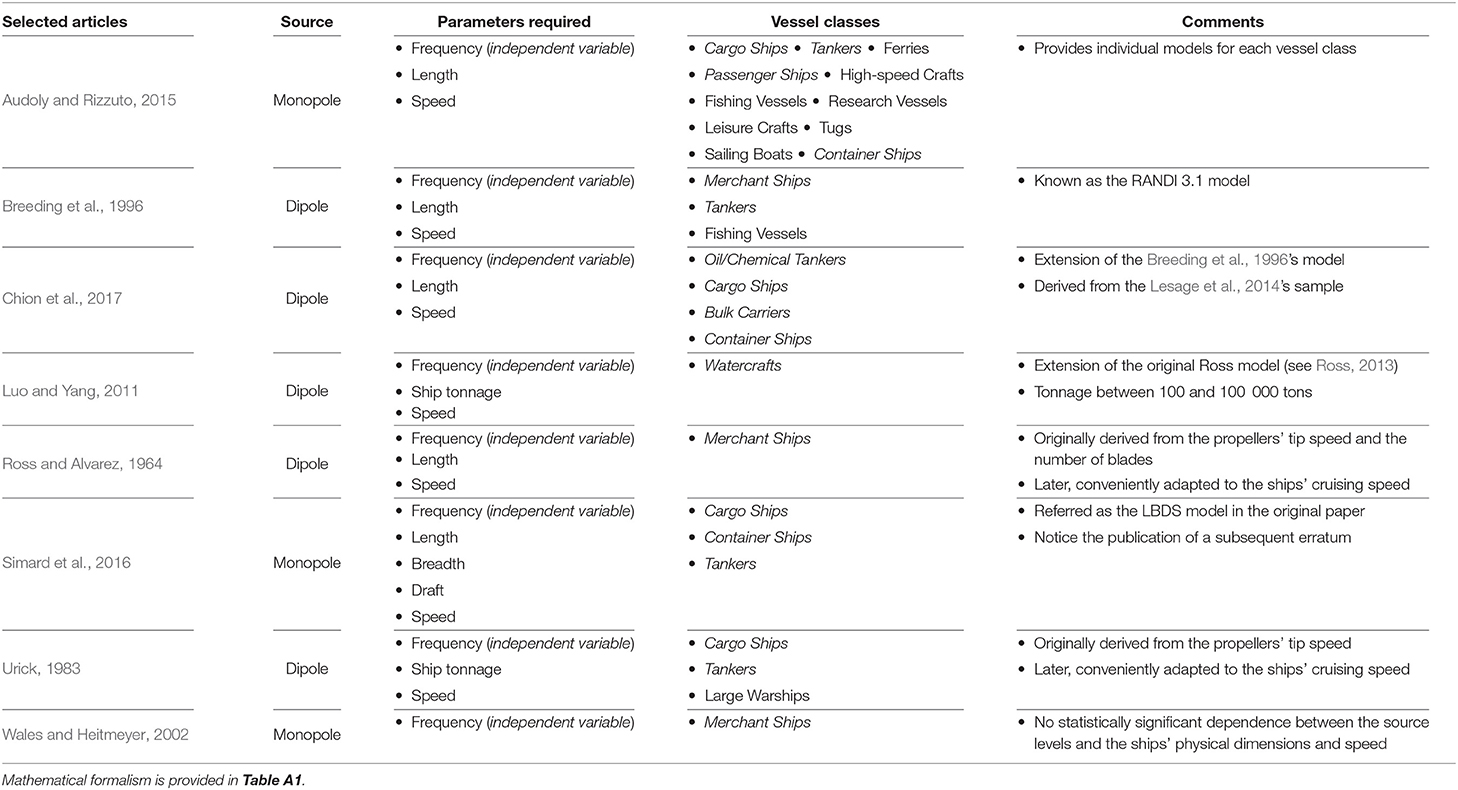
Table 5. Details of the theoretical models published in the literature that serve as RNL/MSL predictors.
The mathematical formalism of each source level models listed in Table 5 is provided in Table A1.
6. Discussion
6.1. Impact of the Experimental Methodology
This work identifies two extrinsic factors, proper to the experimental design and the data processing approach, that may impact the post-processed value of ships' broadband source levels (see Table 3). Our GLMM analysis reveals that the values computed for broadband source levels will (1) decrease with the ships' closest point of approach increasing, and (2) increase when the methodological approach leads to MSL measurements (by opposition to range-independent RNLs). The following subsections detail these behaviors.
6.1.1. Closest Point of Approach
The closest point of approach between a ship and an array of hydrophones requires a compromise in order to increase the chances of good data quality. Distances below a few hundreds meters signify that the hydrophones could be located close to the ship's near field in which the approximation of a point source no longer holds. At such close range, noise is radiated from numbers of different points along the hull, each being characterized by its own source-to-receptor separation. Alternatively, very large CPAs require the use of numerical algorithms in order to properly estimate the transmission loss in complex environments that include variations of the geophysical properties of the underwater terrain and the physico-chemical characteristics of the body of water between the hydrophones and the source. In this work, 8 out of 9 studies retained present RNLs that were processed using geometrical spreading laws (see Table 1). Therefore, the impact attributed to complex underwater environments on the measurement of reliable source levels cannot be properly assessed. The data sample assembled in this work does show a decrease of the calculated source levels with greater CPAs to the source. This suggests that the error propagation caused by ignoring the underwater complexity in RNL measurements leads to an under-estimation of the true source level values.
6.1.2. RNL vs. MSL
Table 3 reveals that the use of spreading laws to backpropagate levels of sound received at the hydrophones to the sources' positions without adding corrective terms to compensate surface-image reflections may lead to an underestimation of the ships' source levels by as much as 35 dB (i.e., upper limit of the confidence interval) which is clearly assessed in Panels (a) and (b) of Farcas et al.'s (2016) Figure 12. This contributes to explain, for example, median source level values in the vicinity of 200 dB re 1 μPa · m reported by Simard et al. (2016), results that are similar to those listed in SMRU Canada (2014). Given that 8 out 9 observational studies reported in this work (see Table 1) and 5 out 8 source level models (see Table 5) are based on RNL measurements, our GLMM analysis supports the need to more rigorously assess what is the best-suited [to the studys needs] numerical algorithm regarding the backpropagation processing (see Table 1 of Farcas et al., 2016).
7. Study's Limitations
This study would definitely benefit from the addition of more MSL measurements in order to properly assess the impact of the monopole vs. dipole approach on source levels' variability.
Other extrinsic factors (i.e., related to the field campaign) that likely play a role on the determination of the source level values cannot be easily quantified a posteriori and are beyond the scope of this paper.
7.1. Directionality and Recommended Hydrophone Angles
Usually treated as a point source in its far field, noise emitted by a ship is in fact directional and anisotropic. Hence, the alignment between a ship and an hydrophone will play a role in the sound levels recorded (Arveson and Vendittis, 2000; Gassmann et al., 2017).
The hydrophone angle, sustained between the source-to-hydrophone line and the sea surface, appears to lead to smaller source level measurements when small angles (< 1°) are involved (e.g., see results from Veirs et al., 2016). Standard protocols (e.g., ANSI, 2009; ISO 17208-1, 2016) recommend to average three (3) simultaneous recordings of a source at hydrophone angles of 15°, 30°, and 45° (see Figure 1 of ISO 17208-1, 2016). For the sake of comparison, an hydrophone angle of 0.2° results in source level values 5 to 10 dB lower than what is obtained for a 10° angle in the 0.020−1 kHz bandwidth using a spreading law for backpropagation calculation (Gassmann et al., 2017). This difference is somewhat reduced to 3–7 dB when correcting for surface-image reflections (cf. using Equation 3 in Gassmann et al., 2017).
7.2. Estimation of the Ship Source Depth
For MSL calculations, uncertainty on the determination of the ships' source depth will have an impact on the transmission loss profiles predicted and, therefore, on the value computed for the source levels. Numerical algorithms for backpropagation such as BELLHOP (Porter and Liu, 1994) and RAM (Collins, 1993) have proven to be highly sensitive to the value chosen for the source's depth as an input parameter. Estimations off by few meters have shown variations in MSL measurements up to 10 dB at low frequencies (Arveson and Vendittis, 2000; Gassmann et al., 2017).
Although the importance of the source's depth on MSL predictions have been demonstrated (see e.g., Figure 3 of Gassmann et al., 2017), this parameter is rarely discussed in observational studies, hence making it difficult to quantitatively estimate its impact on the data presented in this current study.
7.3. Other Factors
Methods of calibration of the hydrophones and the conditions in which these are stocked before deployment will impact the electrical response of the hydrophones to sound (Dakin and Heise, 2015). Calibration in laboratory will have a precision of ± (0.5–2) dB while in situ underwater calibration will have a precision of ± (3–6) dB (Dakin and Heise, 2015).
The reader will also note that the Veirs et al.'s (2016) sample represents the large majority of the data available for this study (see Figure 1). This may be at the origin of certain statistical bias in the quantification of fixed effects (e.g., the closest point of approach) on the values calculated for source levels.
Environmental conditions will also impact the magnitude of the received levels of sound at the hydrophones (e.g., sea roughness, rain lapping, strong winds, waves, currents). The subtraction of this background noise is not trivial and makes it difficult to properly isolate ships' acoustic signatures. Finally, gradients in speed of sound, attributed to a stratification in water temperature, acidity and/or salinity, will induce sound refraction and create tunneling effects that can contaminate sound samples recorded by hydrophones located at very large distances.
8. Conclusion
This work constitutes a literature review and a meta-analysis of the studies aiming at opportunistically assess the levels of noise emitted by merchant ships at the source. It is particularly aimed at supporting the interpretation of the variability in ships' broadband source levels reported in the literature. We specifically focused on the apparent lack of consensus throughout the literature and identify the common ground between different studies aiming at opportunistically estimate of ships' source levels and their contributing factors.
The main results of our study are:
1. Our analysis revealed a positive correlation between source level measurements and ships' speed-above-ground i.e., source levels ~ 15.39 dB × . Limitations in transiting should definitely be considered as measure of mitigation in order to maintain underwater noise attributed to merchant ships within reasonable levels.
2. We have demonstrated that differences in methodological protocols for opportunistic measurements of ships' underwater noise contribute to the inter-study variability reported for source level values of merchant ships. This is reflected by the presence of both CPA and Source extrinsic factors as statistically significant explanatory variables in the best-fitted GLMM describing source levels; see Equation (1). That said, our results support the necessity to use standardized approaches to conduct hydrophone-based recordings of underwater noise sources. The backpropagation methods used to estimate ships' source levels from hydrophone measurements also needs to be adapted to both the experimental setup and environmental characteristics to control as much as possible for the biasing factors. In particular, the commonly used geometrical spreading laws are clearly unadapted to some complex underwater environments, leading to an under-estimation of the backpropagated source levels.
3. Error estimation and propagation need to be refined as source level measurements provided in the literature never include envelopes of uncertainty.
This study recommends that:
1. Narrowband or 1/3-octave band measurements of ships' source levels instead of broadband values should be made available to the scientific community. Our study demonstrated that the interpretation of broadband source levels is subject to confusion, hence making the comparison between studies that focus on ships' underwater radiated noise particularly difficult. The publication of narrowband measurements would definitely benefit our field of study and contribute to facilitate the data interpretation of secondary users of ships' source level measurements.
2. In order to properly quantify the impact of individual intrinsic factors (e.g., speed, load, draft, working engines) on the underwater noise radiated by merchant ships and ultimately mitigate them, control experiments could be designed in order to favor the simultaneous control and monitoring of the factors contributing to ships' noise. This way, the impact of a single factor (e.g., ships' speed, ships' load, number of engines in operation) can be quantified while others are kept constant. This approach, although more costly, will help to gain a better understanding of onboard noise sources and could serve as a baseline to improve the interpretation of the growing number of ships' source level data coming from opportunistic measurements.
Data Availability Statement
The datasets for this manuscript are not publicly available because Data were provided during the submission process. Requests to access the datasets should be directed to Y2xlbWVudC5jaGlvbkB1cW8uY2E=.
Author Contributions
Review of literature, data processing, and statistical analysis were conducted by DL. CC was responsible for reaching out to authors in order to obtain methodological details that were not a priori available in the original studies. Responsibilities for the redaction, figures, and tables processing of this manuscript were equally shared between DL and CC. JD contributed to the revision of the early versions of this paper and also provided funding to support this work.
Funding
The funding to support this research project was provided by the Ministère des Forêts, de la Faune et des Parcs du Québec, the Department of Fisheries and Oceans Canada (contract number F5211-170397), and JD.
Conflict of Interest
The authors declare that the research was conducted in the absence of any commercial or financial relationships that could be construed as a potential conflict of interest.
Acknowledgments
The authors would like to thank the teams of specialists that generously made their data available to the public and those authors who kindly responded to our questionnaire regarding certain clarifications about specific details of their protocol. The authors are also grateful to I. McQuinn, V. Nolet, and V. Lesage for judicious remarks regarding this work and to Pr. A. Dupuch for her advice about the statistical analyses.
Supplementary Material
The Supplementary Material for this article can be found online at: https://www.frontiersin.org/articles/10.3389/fmars.2019.00714/full#supplementary-material
Footnotes
1. ^Simply referred as “cargo ships” by the different studies selected in section 4, these ships likely include a mixture of non-categorized bulk carriers, container ships, oil/chemical tankers, vehicles carriers and subcategories.
2. ^One can estimate, in Panel (a), a transmission loss of approximately 35 dB between the source and the diagram's lower-left corner. Applying this loss to the received levels illustrated in Panel (b)'s lower-left corner and backpropagating to the position of the source yields a RNL roughly 25 dB re 1 μPa · m short of the MSL value.
References
Allen, J. K., Peterson, M. L., Sharrard, G. V., Wright, D. L., and Todd, S. K. (2012). Radiated noise from commercial ships in the gulf of maine: implications for whale/vessel collisions. J. Acoust. Soc. Am. 132, EL229–EL235. doi: 10.1121/1.4739251
ANSI (2009). Quantities and Procedures for Description and Measurements of Underwater Sound From Ships - Part 1: General Requirements. Technical report, Acoustical Society of America Standards Secretariat.
Arveson, P. T., and Vendittis, D. J. (2000). Radiated noise characteristics of a modern cargo ship. J. Acoust. Soc. Am. 107, 118–129. doi: 10.1121/1.428344
Audoly, C., Gaggero, T., Baudin, E., Folegot, T., Rizzuto, E., Mullor, R. S., et al. (2017). Mitigation of underwater radiated noise related to shipping and its impact on marine life: a practical approach developed in the scope of aquo project. J. Ocean. Eng. 42, 373–387. doi: 10.1109/JOE.2017.2673938
Audoly, C., and Rizzuto, E. (2015). Ship Underwater Radiated Noise Patterns. Technical Report, AQUO European Collaborative Project, Deliverable D2.1.
Audoly, C., Rousset, C., and Leissing, T. (2014). “Aquo project–modelling of ships as noise source for use in an underwater noise footprint assessment tool,” in INTER-NOISE and NOISE-CON Congress and Conference Proceedings (Melbourne, VIC: Institute of Noise Control Engineering), 862–871.
Bassett, C., Polagye, B., Holt, M., and Thomson, J. (2012). A vessel noise budget for admiralty inlet, puget sound, washington (usa). J. Acoust. Soc. Am. 132, 3706–3719. doi: 10.1121/1.4763548
Bates, D., Mächler, M., Bolker, B., and Walker, S. (2015). Fitting linear mixed-effects models using lme4. J. Stat. Softw. 67, 1–48. doi: 10.18637/jss.v067.i01
Breeding, J. E., Pflug, L. A., Bradley, M., and Walrod, M. H. (1996). Research Ambient Noise Directionality (randi) 3.1 Physics Description. Technical report, Naval Research Lab Stennis Space Center MS.
Canada's Species at Risk Act (2002). Canada's Species at Risk Act. Technical report, Committee on the Status of Endangered Wildlife in Canada.
Chion, C., Lagrois, D., Dupras, J., Turgeon, S., McQuinn, I. H., Michaud, R., et al. (2017). Underwater acoustic impacts of shipping management measures: Results from a social-ecological model of boat and whale movements in the st. lawrence river estuary (canada). Ecol. Model. 354, 72–87. doi: 10.1016/j.ecolmodel.2017.03.014
Clark, C. W., Ellison, W. T., Southall, B. L., Hatch, L., Van Parijs, S. M., Frankel, A., et al. (2009). Acoustic masking in marine ecosystems: intuitions, analysis, and implication. Mar. Ecol. Prog. Ser. 395, 201–222. doi: 10.3354/meps08402
Collins, M. D. (1993). A split-step padé solution for the parabolic equation method. J. Acoust. Soc. Am. 93, 1736–1742. doi: 10.1121/1.406739
Dakin, T., and Heise, K. (2015). Guidelines for Installing Cabled Hydrophones for Monitoring Marine Mammals, Vessels and Other Sources of Underwater Noise. Technical report, Fisheries and Oceans Canada.
Erbe, C., Reichmuth, C., Cunningham, K., Lucke, K., and Dooling, R. (2016). Communication masking in marine mammals: a review and research strategy. Mar. Pollut. Bull. 103, 15–38. doi: 10.1016/j.marpolbul.2015.12.007
Farcas, A., Thompson, P. M., and Merchant, N. D. (2016). Underwater noise modelling for environmental impact assessment. Environ. Impact Assess. Rev. 57, 114–122. doi: 10.1016/j.eiar.2015.11.012
Gassmann, M., Wiggins, S. M., and Hildebrand, J. A. (2017). Deep-water measurements of container ship radiated noise signatures and directionality. J. Acoust. Soc. Am. 142, 1563–1574. doi: 10.1121/1.5001063
Gomez, C., Lawson, J. W., Wright, A. J., Buren, A. D., Tollit, D., and Lesage, V. (2016). A systematic review on the behavioural responses of wild marine mammals to noise: the disparity between science and policy. Can. J. Zool. 94, 801–819. doi: 10.1139/cjz-2016-0098
Gouvernement du Québec (2015). La stratégie Maritime à l'Horizon 2030 - plan d'Action 2015-2020. Technical report, Stratégie Maritime.
IMO (2014). Guidelines for the Reduction of Underwater Noise From Commercial Shipping to Address Adverse Impacts on Marine Life. Technical report, MEPC.1/Circ.833.
ISO 17208-1 (2016). Underwater Acoustics - Quantities and Procedures for Description and Measurements of Underwater Sound From Ships - Part 1: General Requirements. Technical report, International Standardization Organization.
ISO 17208-2 (2019). Underwater Acoustics - Quantities and Procedures for Description and Measurements of Underwater Sound From Ships - Part 2: Determination of Source Levels From Deep Water Measurements. Technical report, International Standardization Organization.
ISO 18405 (2017). Underwater Acoustics - Terminology. Technical report, International Standardization Organization.
Jalkanen, J.-P., Johansson, L., Liefvendahl, M., Bensow, R., Sigray, P., Östberg, M., et al. (2018). Modelling of ships as a source of underwater noise. Ocean Sci. 14, 1373–1383. doi: 10.5194/os-14-1373-2018
Kaplan, M. B., and Solomon, S. (2016). A coming boom in commercial shipping? The potential for rapid growth of noise from commercial ships by 2030. Mar. Policy 73, 119–121. doi: 10.1016/j.marpol.2016.07.024
Kipple, B. (2002). Southeast Alaska Cruise Ship Underwater Acoustic Noise. Technical report, Glacier Bay National Park and Preserve.
Lesage, V., McQuinn, I. H., Carrier, D., Gosselin, J.-F., and Mosnier, A. (2014). Exposure of the Beluga (Delphinapterus leucas) to Marine Traffic Under Various Scenarios of Transit Route Diversion in the St. lawrence Estuary. Technical report, Fisheries and Oceans Canada.
Lüdecke, D. (2018). sjplot: Data Visualization for Statistics in Social Science. Available online at: https://CRAN.R-project.org/package=sjPlot (accessed December 18, 2018).
Luo, J., and Yang, Y. (2011). Simulation model of ship-radiated broadband noise. in Signal Processing, Communications and Computing (ICSPCC) (Xi'an: IEEE), 1–5.
MacGillivray, A. O., Li, Z., Hannay, D. E., Trounce, K. B., and Robinson, O. M. (2019). Slowing deep-sea commercial vessels reduces underwater radiated noise. J. Acoust. Soc. Am. 146, 340–351. doi: 10.1121/1.5116140
McKenna, M. F., Ross, D., Wiggins, S. M., and Hildebrand, J. A. (2012). Underwater radiated noise from modern commercial ships. J. Acoust. Soc. Am. 131, 92–103. doi: 10.1121/1.3664100
McKenna, M. F., Wiggins, S. M., and Hildebrand, J. A. (2013). Relationship between container ship underwater noise levels and ship design, operational and oceanographic conditions. Sci. Rep. 3:1760. doi: 10.1038/srep01760
MCR International (2011). Final Report Describing Measurements of Ship Noise Taken From r/v Song of the Whale in the English Channel and the Hebrides in June and August 2011. Technical report, International Fund for Animal Welfare.
NOAA (2015). Guidance for Assessing the Effects of Anthropogenic Sound on Marine Mammal Hearing Underwater Acoustic Threshold Levels for Onset of Permanent and Temporary Threshold Shifts. Technical report, National Oceanic and Atmospheric Administration.
Pennucci, G., and Jiang, Y.-M. (2018). Extracting acoustic source information of shipping noise for dynamic ambient noise modelling. J. Ship. Ocean Eng. 8, 10–20. doi: 10.17265/2159-5879/2018.01.002
Porter, M. B., and Liu, Y.-C. (1994). Finite-element ray tracing. Theor. Comput. Acoust. 2, 947–956.
Robinson, S. P., Lepper, P. A., and Hazelwood, R. A. (2014). Good Practice Guide for Underwater Noise Measurement. Technical report, National Measurement Office, Marine Scotland, The Crown Estate.
Ross, D., and Alvarez, F. F. (1964). Radiated underwater noise of surface ships. US Navy J. Underw. Acoust. 14:331.
Simard, Y., Roy, N., Gervaise, C., and Giard, S. (2016). Analysis and modeling of 255 source levels of merchant ships from an acoustic observatory along St. lawrence seaway. J. Acoust. Soc. Am. 140, 2002–2018. doi: 10.1121/1.4962557
Tyack, P. L., Zimmer, W. M. X., Moretti, D., Southall, B. L., Claridge, D. E., Durban, J. W., et al. (2011). Beaked whales respond to simulated and actual navy sonar. PLoS ONE 6:e17009. doi: 10.1371/journal.pone.0017009
Veirs, S., Veirs, V., and Wood, J. D. (2016). Ship noise extends to frequencies used for echolocation by endangered killer whales. PeerJ 4:e1657. doi: 10.7717/peerj.1657
Wales, S. C., and Heitmeyer, R. M. (2002). An ensemble source spectra model for merchant ship-radiated noise. J. Acoust. Soc. Am. 111, 1211–1231. doi: 10.1121/1.1427355
Williams, R., Wright, A. J., Ashe, E., Blight, L. K., Bruintjes, R., Canessa, R., et al. (2015). Impacts of anthropogenic noise on marine life: publication patterns, new discoveries, and future directions in research and management. Ocean Coast. Manag. 115, 17–24. doi: 10.1016/j.ocecoaman.2015.05.021
Appendix
Mathematical Formalism of Empirical Source Level Models
Equations for the empirical source level models mentioned in sections 5.2 and 5.3.2. are provided in Table A1.
Keywords: review of literature, ships' source levels, acoustic techniques, hydrophone-based observations, statistical methods
Citation: Chion C, Lagrois D and Dupras J (2019) A Meta-Analysis to Understand the Variability in Reported Source Levels of Noise Radiated by Ships From Opportunistic Studies. Front. Mar. Sci. 6:714. doi: 10.3389/fmars.2019.00714
Received: 01 February 2019; Accepted: 06 November 2019;
Published: 26 November 2019.
Edited by:
Christine Erbe, Curtin University, AustraliaReviewed by:
Christ De Jong, Netherlands Organisation for Applied Scientific Research (TNO), NetherlandsFrancine Kershaw, Natural Resources Defense Council, United States
Alexander Orion MacGillivray, JASCO Applied Sciences (Canada) Ltd., Canada
Copyright © 2019 Chion, Lagrois and Dupras. This is an open-access article distributed under the terms of the Creative Commons Attribution License (CC BY). The use, distribution or reproduction in other forums is permitted, provided the original author(s) and the copyright owner(s) are credited and that the original publication in this journal is cited, in accordance with accepted academic practice. No use, distribution or reproduction is permitted which does not comply with these terms.
*Correspondence: Clément Chion, Y2xlbWVudC5jaGlvbkB1cW8uY2E=