Impacts of Acidic Seawater on Early Developmental Stages of Fucus gardneri at Burrard Inlet, British Columbia
- Department of Biological Sciences, Centre for Cell Biology, Development, and Disease, Simon Fraser University, Burnaby, BC, Canada
Increases in stressors associated with climate change such as ocean acidification and warming temperatures pose a serious threat to intertidal ecosystems. Of crucial importance are the effects on foundational species, such as fucoid algae, a critical component of rocky intertidal shorelines around the world. The impact of climate change on adult fronds of fucoid algae has been documented but effects on early developmental stages are not as well understood. In particular, ocean acidification stands to impact these stages because zygotes and embryos are known to maintain internal pH and develop a cytosolic pH gradient during development. To assess the effects of seawater acidification on early development, zygotes of Fucus gardneri were exposed to artificial seawater (ASW) buffered to conditions that approximate current global averages and extend largely beyond future projections. Exposure to acidic seawater had significant effects on embryonic growth. Specifically, rhizoid elongation, which occurs by a process known as tip growth, was significantly reduced with each 0.5 unit drop in pH. When pH was decreased from 8.0 to 7.5, which is similar to levels that have been observed in Burrard Inlet, there was reduction in rhizoid growth rate of almost 20%. Under more extreme conditions, at pH 6, rhizoid growth rates were reduced by 64% in comparison to embryos exposed to seawater at pH 8.0. On the other hand, acidic seawater had no effect on earlier processes; zygotes became multicellular embryos with well-formed rhizoids on a similar time course within the first 24 h of development, even when exposed to pH 6, an extreme pH well below what is expected in the future. This suggests that zygotes can maintain an internal pH that allows germination and cell division to occur. Tip growth, however, depends on the extended maintenance of an internal pH gradient. It is therefore possible that disruptions to this gradient could account for the observed reductions in rhizoid elongation. Under acidic conditions proton influx into the cell becomes energetically more favorable than at pH 8, and expulsion would be more difficult. This could disrupt the cytosolic pH gradient and in turn affect rhizoid growth.
Introduction
Fucoid algae are found on rocky intertidal shores around the world. They are foundational species in the ecosystems they occupy as they provide food, shelter, and habitat for many other organisms (Schiel, 2006). They are generally known as ecosystem engineers because they form extensive canopies that provide refuge for other organisms, especially during low tides. Their presence is associated with increases in species diversity and abundance at a variety of sites including locations impacted by industrial activities or contaminants, making them indicator species for the habitats they occupy (Watt and Scrosati, 2013; Bellgrove et al., 2017; Lauze and Hable, 2017; Scrosati, 2017).
To persist in the intertidal zone, algal populations must be able to survive, grow, and reproduce despite fluctuating and often stressful conditions. Mature fronds of fucoid algae are well-known for their ability to tolerate dehydration and heat; in the summer months they can recover after hours of exposure when tides are low during the day (Ferreira et al., 2014). Increases in the stress imposed on intertidal species are expected as our climate changes in coming years. In addition to warmer temperatures, ocean waters are also becoming more acidic, a phenomenon that is driven by increasing levels of anthropogenically produced carbon dioxide (CO2). As atmospheric CO2 levels rise, gas exchange between the sea surface and the air causes a corresponding increase in the amount of CO2 in the water. Once dissolved, CO2 reacts with water to produce carbonic acid which dissociates into hydrogen, bicarbonate, and carbonate ions. The increase in hydrogen acidifies the seawater and reduces the amount of carbonate ions present [reviewed in Doney et al. (2009)]. Globally, the pH of ocean waters has fallen from roughly 8.2 in pre-industrial times to 8.1 and predictions estimate further decreases of up to 0.32 pH units by the end of the century if CO2 production continues unabated (Orr et al., 2005; IPCC, 2014). Regional ocean pH can also deviate substantially from global averages. As part of the California Current System, the southern coast of British Columbia is subject to periodic penetration of acidified waters from offshore upwelling events followed by downwelling episodes that have the opposite effect (Johannessen and Macdonald, 2009; Chan et al., 2017) These patterns produce dynamic fluctuations in intertidal seawater pH, causing it to reach levels as low as 7.3 at some sites (Marliave et al., 2011). In future decades, further declines in seawater pH are expected as atmospheric CO2 levels continue to rise.
Ocean acidification that comes from an influx of CO2 can have a wide range of impacts on marine organisms. The increase in CO2 in the seawater can be beneficial for primary producers like eelgrass and algae, since they can utilize the carbon for photosynthesis and growth (Zimmerman et al., 1997; Olischläger et al., 2012; Koch et al., 2013). However, as CO2 levels rise, the ocean’s carbonate chemistry also changes, making it difficult for calcifying seaweeds and invertebrates to build calcium carbonate skeletal structures or shells because carbonate ions in the seawater are less prevalent (Gazeau et al., 2013; Hofmann and Bischof, 2014). Studies on fucoid algae evaluated the effects of increased CO2 on vegetative growth of germlings (greater than 8 weeks old) and adult fronds with conflicting results (Gutow et al., 2014; Graiff et al., 2015; Al-Janabi et al., 2016), possibly arising from differential effects of increased photosynthetic activity resulting from higher CO2 levels and the negative effects of reduced pH on cellular activities. As hydrogen ions become more concentrated in the external environment, the ability to maintain cytoplasmic pH at normal levels within cells may become more difficult. This is particularly important for algal species related to Fucus which also depend on the formation and maintenance of pH gradients in the cytoplasm during embryonic growth and development (Gibbon and Kropf, 1994).
Zygotes of fucoid algae are initially spherical in shape and over the first few hours of development there is a cytoplasmic reorganization that results in the formation of a protrusion, the rhizoid, from one pole. This process is called germination; it is turgor driven and occurs at a site where the cell wall has been weakened. The first cell division is an asymmetric one that bisects the zygote into two cells with different shapes and developmental fates. The spherical thallus cell is cleaved into a ball of cells that eventually gives rise to most of the mature alga, including the reproductive and photosynthetic fronds. The rhizoid cell, on the other hand, contains the protrusion that formed at germination; it elongates by extension of the tip apex in a process known as tip growth, eventually developing into the holdfast that anchors the alga to the substratum. The rhizoid also undergoes several rounds of cell divisions, but in this case the cell plates are all oriented parallel to one another and perpendicular to the growth axis. As development proceeds, the rhizoid continues to elongate via tip growth of the apical-most rhizoid cell, producing a long file of cells [reviewed in Kropf (1997), Bisgrove and Kropf (2007), and Hable (2014)].
Tip growth is a turgor-driven process that also involves focused delivery of cell wall and membrane materials to the growing tip. It occurs in specific cells of organisms from a range of taxa. In addition to brown algal rhizoids, fungal hyphae, as well as pollen tubes and root hairs of plants all elongate via tip growth (Gow et al., 1984; Cárdenas, 2009; Bascom et al., 2018). This type of cell expansion correlates with gradients of Ca2+ and H+ ions that form in the cytoplasm parallel with the direction in which the cell is elongating, with the highest concentrations of each ion found in the apical regions near the growing tip. These gradients are thought to have an important role in tip growth by driving the activities of different regulatory proteins and processes along the cell and restricting growth to extension from the apical-most region [reviewed in Obermeyer and Feijó (2017), Bascom et al. (2018)].
In tip growing cells an internal pH gradient is established and maintained within the cytoplasm by controlling proton fluxes into and out of the cell. Protons flow in at the tip and are used in metabolic processes or expelled in basal regions of the cell, creating a gradient that is most acidic at the apex [Takeuchi et al., 1988; reviewed in Obermeyer and Feijó (2017)]. In fucoid algae the first detectable cytosolic pH gradient forms at the presumptive rhizoid pole early in zygotic development, well before rhizoid growth begins. The magnitude of this gradient is initially small, with a difference of less than 0.1 pH units, but it increases as development proceeds. At the time of germination, the emerging rhizoid is approximately 0.1 units more acidic than the cytoplasm in the thallus which is maintained close to pH 7.5 (Gibbon and Kropf, 1993, 1994; Kropf et al., 1995b). The gradient continues to intensify; at the 2-celled stage the rhizoid tip has acidified to a pH of 7.2 generating a differential of 0.3–0.5 pH units between the tip and the cytoplasm further back at the base (Gibbon and Kropf, 1994). Dissipation of the gradient by treatment with H+ buffers inhibits rhizoid growth, indicating that the gradient is important for embryonic development (Kropf et al., 1995a). Since rhizoid formation and tip growth depend on creating and maintaining an intracellular pH gradient, these stages of the life cycle stand to be impacted by ocean acidification if zygotes and embryos cannot adequately cope with higher proton concentrations in their environment. In this study, the effects of seawater acidification on early development of Fucus gardneri at Burrard Inlet were assessed. Zygotes and embryos were cultured in artificial seawater (ASW) solutions buffered to a pH value that ranged from 8 to 6. These conditions were chosen to approximate current global averages, encompass levels that have already been observed at the Barnet Marine Park, and include more extreme conditions. Three key aspects of early development were examined: the ability of zygotes to form a rhizoid, the timing of rhizoid formation, and the rate of rhizoid growth. The goal of this study was to examine whether seawater pH would affect early developmental processes and determine whether embryos from this population could withstand extreme acidity.
Materials and Methods
Algal Collection and Culture Conditions
Early developmental stages of fucoid algae were sourced from a population of F. gardneri growing at the Barnet Marine Park located on Burrard Inlet in Burnaby, British Columbia (Figure 1). Burrard inlet lies in an urban region with several large municipalities and industrial sites situated along its shorelines. A sawmill and several export terminals which include facilities for petroleum and sulfur are located near the Barnet Marine Park and marine biota within its boundaries are exposed to contaminants associated with occasional releases from these facilities. In addition to chemical contaminants, inlet waters are also subject to increasing levels of stressors associated with climate change. Sea surface temperatures fluctuate throughout the year, dipping to approximately 5°C in the winter and often reaching highs of 20°C or more during the summer months (NASA JPL, 2019). Furthermore, fluctuations in the pH of seawater collected from the park at the time of sampling ranged from 8.2 down to 7.4. Despite these fluctuating conditions and the presence of multiple types of contaminants, the intertidal zone at this site harbors a robust population of F. gardneri.
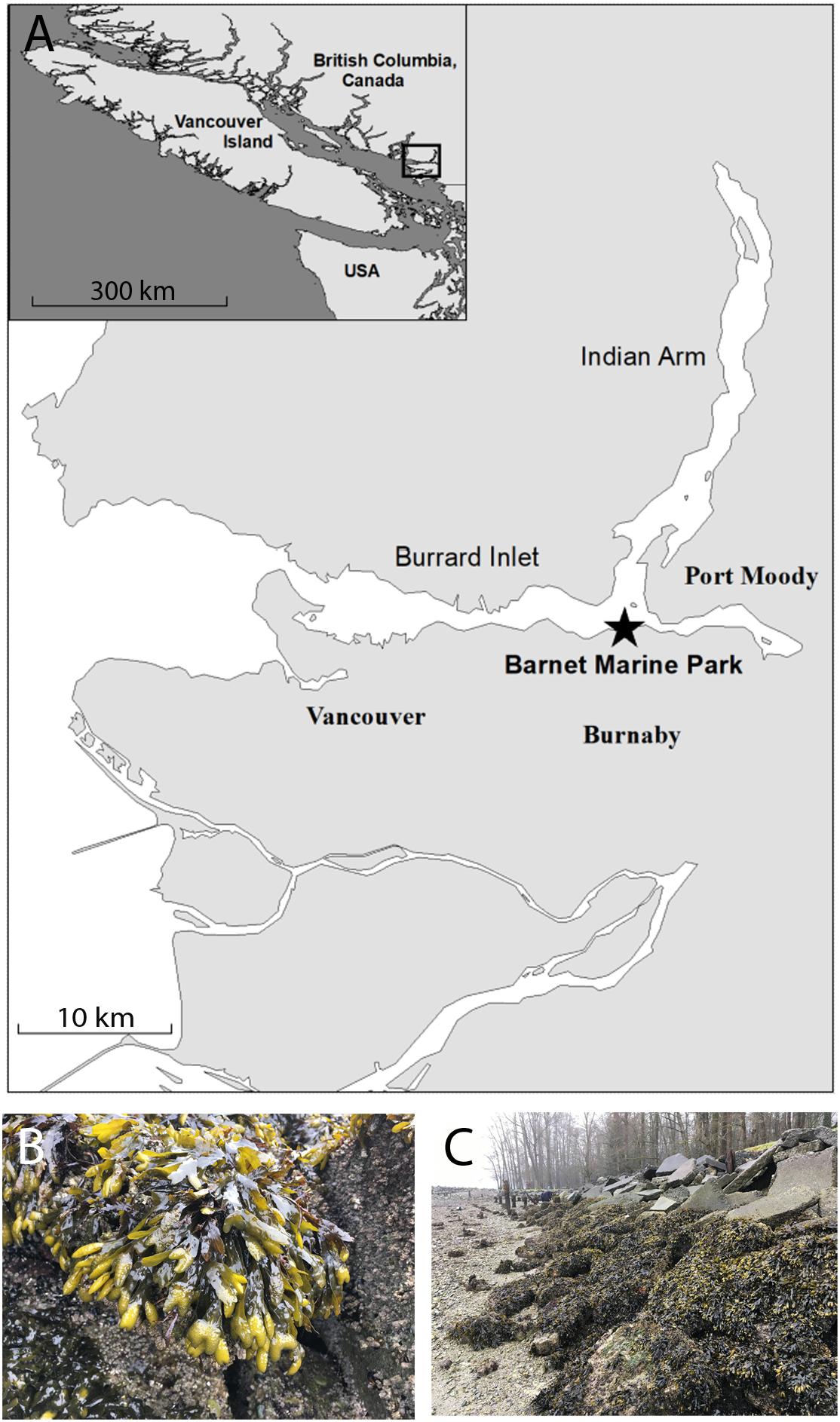
Figure 1. The Barnet Marine Park harbors a robust population of F. gardnerii. Reproductive fronds were collected from a site within the Barnet Marine Park (49°17′31.9′′N 122°55′34.1′′W), located in an industrialized area in the city of Burnaby, approximately 15 km east of Vancouver (A). Inset shows the general location of the park with respect to Vancouver Island. A population of healthy F. gardnerii covering discarded pavement blocks and boulders in the intertidal zone at the site as viewed at low tide (B,C). Scale bars are 10 and 300 km in the map and inset, respectively; star in A denotes collection site.
Sexually mature fronds were collected at low tide and stored in the dark at 4°C for at least 3 days until use. Zygote release was induced by incubating receptacles under approximately 115 μmol/m2/s light for 1 h at 15°C in buffered ASW (10 mM KCl, 9 mM CaCl2 (Fisher Scientific), 0.45 M NaCl (ACP Chemicals), 16 mM MgSO4 (Caledon Laboratories), 10 mM tris base (Invitrogen), 0.04 mg/ml chloramphenicol (Sigma-Aldrich); Bisgrove and Kropf, 2001). The pH was adjusted to 8.0, 7.5, 7.0, 6.5, or 6.0 using concentrated HCl. These pH values were chosen to approximate current conditions and extend well beyond the 0.32-unit reduction projected to occur over the next 80 years (IPCC, 2014). On the coast of British Columbia, ocean pH dips to levels that are substantially lower than global averages of 8.1 (Marliave et al., 2011; IPCC, 2014). We observed fluctuations down to 7.4 at Barnet Marine Park; if conditions at this site follow global projections, pH could drop to levels as low as 7.0 by the end of the century. We extended our analysis to include more extreme conditions, down to pH 6.0, to fully assess sensitivities and determine how resilient zygotes were to low pH.
Zygotes were collected by rinsing fronds with seawater at the respective pH then filtering the solution containing the zygotes through a 300 μm nylon mesh to separate them from the fronds and other debris. Zygotes were rinsed twice with fresh ASW at the appropriate pH, distributed into three separate petri dishes per pH treatment, and incubated at 15°C in unidirectional light (115 μmol/m2/s). The time of fertilization was considered to be 30 min after fronds were placed in ASW.
Experimental Design and Data Analysis
To assess the effects of seawater pH on germination, four separate trials, each using a different batch (collection) of fronds, were conducted as follows. Receptacles from a single algal collection were induced to release zygotes in seawater of the appropriate pH (described above). For each of the five pH treatments, zygotes were aliquot into three petri dishes and incubated until 24 h after fertilization (AF). Each petri dish was divided into four equal sections, and a picture was taken from a random location within each section, for a total of four pictures per dish. The percent of zygotes that germinated in each petri dish was calculated by determining the proportion that had well-formed rhizoids and an average was taken from the three replicate plates. The four treatment averages from each trial were averaged together to determine the overall germination percentage at each seawater pH.
The effects of seawater pH on the timing of germination were assessed by averaging the results from three trials. Each trial consisted of three replicate plates, exposed to two different pH levels, pH 8 or the more extreme pH 6. These two pH levels were initially chosen to see whether there were different effects on the timing of germination. Since zygotes in both treatments followed the same time course there was no reason to test pH levels between 8 and 6. Since germination was expected to begin around 10 h AF in zygotes exposed to pH 8, a window of 10–14 h AF was chosen for analysis. Four photos, one from each section of the plate, were taken at 10, 12, and 14 h AF. Averages for the three trials were determined by averaging germination percentages at each timepoint for each pH treatment, as described above.
Rhizoid growth rates were assessed between 1- and 6-days AF in four separate trials. Each trial consisted of five pH treatments, each with three replicate plates. Ten germinated zygotes were randomly selected and measured from photographs taken of each of the four sections on every plate for a total of 40 rhizoid lengths per plate. Lengths were measured from the base of the thallus to the tip of the rhizoid on day 1 and day 6 from the same plates. The difference in lengths were used to calculate growth rates in μm/day by the following formula: (average length 6 days AF – average length 1 day AF)/5 days. Growth rates from three replicate plates were averaged together and the four averages from each trial were averaged to determine the average growth rates for each pH treatment.
In all experiments, photographs were taken using an Olympus SZX16 microscope equipped with a Retiga 4000R digital camera and Q-Capture Pro 6.0 software. Rhizoid lengths were measured using ImageJ software1. Statistical analyses were performed using JMP 14 software. One- or two-way ANOVA analyses followed by post hoc Tukey tests were used to test for differences between means. Levene’s test and normal quantile plots were also used in JMP to assess ANOVA assumptions of normality and homogeneity of variance (see Supplementary Tables S1–S4).
Results
The effects of reduced pH on three key aspects of early development were examined; the ability of zygotes to form a rhizoid, the timing of rhizoid formation, and the rate of rhizoid growth.
Germination Occurred in Zygotes Exposed to Acidic ASW
To determine whether early developmental processes could occur in acidified seawater the percent of zygotes that were able to germinate was assessed. Zygotes were allowed to develop until 24 h AF which is several hours past the time when these events typically occur. This provided ample time for rhizoid formation in all pH treatments. Embryos cultured in seawater at pH 8, which approximates the global average, developed from spherical shaped cells into multicellular embryos with well-formed rhizoids Figure 2). Embryos cultured in more acidic seawater were similar in appearance. The majority of embryos were multicellular, had germinated, and had rhizoids that appeared to be similar in size, regardless of seawater pH. Zygotes, therefore, were able to form an axis and germinate even in seawater as acidic as pH 6. To assess whether acidic conditions affected the ability of zygotes to germinate, the percent of zygotes that formed rhizoids was determined in each pH treatment (Figure 2G). At pH 8, an average of 90.6% (±4.71 SD) of embryos had rhizoids. At lower pH levels, the average percent of embryos with rhizoids ranged from 88.2 to 94.3% (±2.71 to 5.67 SD). This level of germination is high and statistically indistinguishable from the levels observed for embryos developing at pH 8 (p ≥ 0.339; one-way ANOVA with post hoc Tukey’s test) (see Supplementary Table S1). We therefore conclude that zygotes were able to form a developmental axis and initiate rhizoid growth, regardless of the pH of the seawater.
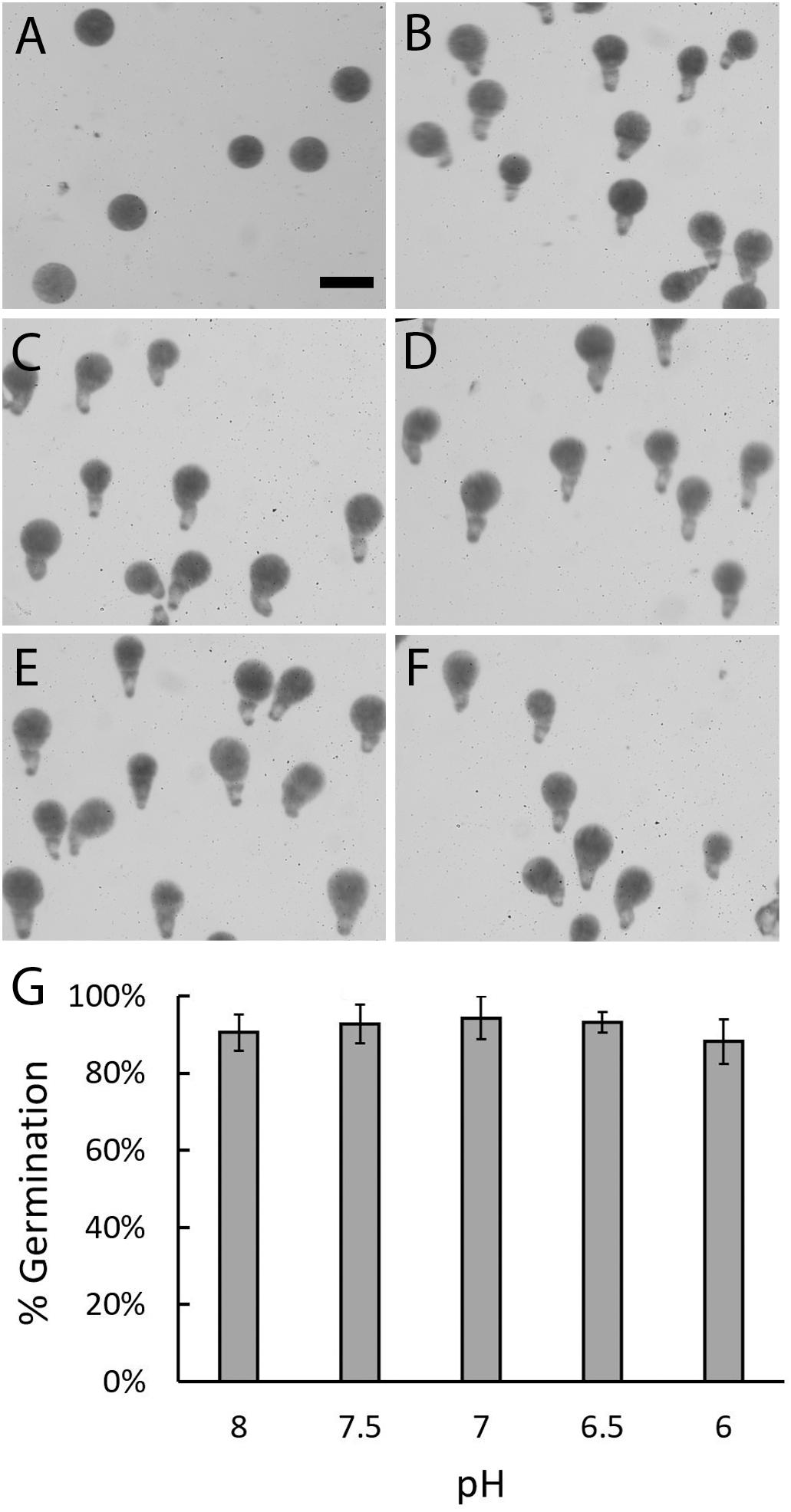
Figure 2. Zygotes can form rhizoids under acidic conditions. Most zygotes were spherical in shape at 10 h AF, regardless of pH (A). At 24 h AF, most embryos cultured in ASW at pH 8 (B), 7.5 (C), 7 (D), 6.5 (E), or 6 (F) had well-formed rhizoids. Quantification of zygotes/embryos that germinated by 24 h AF revealed similar percentages in all five pH treatments (G). Bars depict the average percent of embryos with rhizoids with standard deviations (SDs). Averages represent data from four trials (N = 1910–2334 zygotes per trial). Size bar in (A) is 100 μm and applies to all photographs.
Zygotes Exposed to Basic or Acidic Seawater Formed Rhizoids at the Same Time
The above analysis revealed that rhizoid formation could occur under acidic conditions. However, since zygotes were analyzed several hours after germination, any developmental delays could have been overlooked. Therefore, the effects of acidic seawater on the timing of germination was assessed. Zygotes were incubated under two different conditions, pH 8 and a highly acidic treatment, pH 6. When examined 10 h AF, most zygotes in both treatments were still spherical in shape (Figure 3). Only a few had small protrusions, indicative of the earliest signs of germination. At 12 h AF, the majority of zygotes had formed small rhizoids in both treatments and by 14 h AF, rhizoids on germinated zygotes appeared longer than they were at 12 h AF. These visual observations suggested that most zygotes germinated between 10 and 12 h AF.
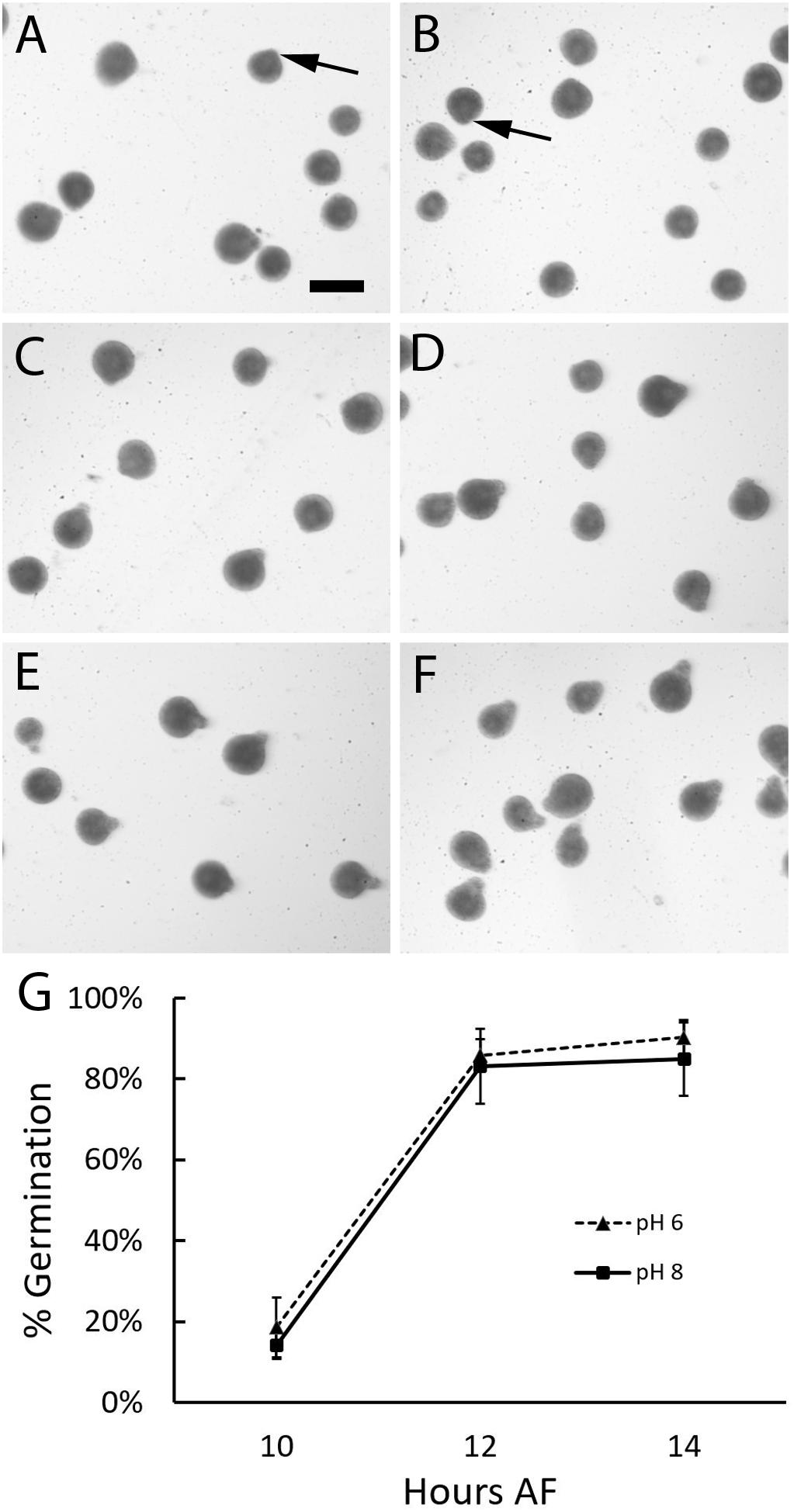
Figure 3. Zygotes germinate at the same time regardless of seawater pH. At 10 h AF small bulges consistent with the onset of rhizoid formation (arrows) were found on a few zygotes developing in ASW at pH 6 (A) and pH 8 (B). Longer rhizoids were observed on more of the zygotes examined 12 (C,D) and 14 (E,F) hours AF at pH 6 (C,E), and pH 8 (D,F). On average the percent of zygotes that had formed rhizoids at 10, 12, or 14 h AF were similar at pH 6 and 8 (G). Data points depict the average percent of zygotes with rhizoids and bars represent SDs. Averages represent data from three trials (N = 2967-4968 zygotes per trial). Size bar in (A) is 100 μm and applies to all photographs.
To determine whether there were any differences in the timing of germination between treatments, we calculated the percent of zygotes that had formed rhizoids at each timepoint. At 10 h AF an average of 18.6 and 14.2% (±7.34 and 3.47 SD) of zygotes had germinated in pH 6 and 8, respectively, indicating that germination had begun in both treatments. Two hours later these numbers increased to 85.9 and 83.2% (±3.96 and 9.25 SD), indicating that most zygotes had germinated by 12 h AF. Subsequent increases in the number of individuals with rhizoids were small; at 14 h AF an average of 90.3 and 85.0% (±4.37 and 9.06 SD) of zygotes were germinated at pH 6 and 8, respectively. At each timepoint, a comparison of the average percent of zygotes that formed rhizoids revealed no difference between the two pH treatments (two-way ANOVA with post hoc Tukey’s test; 0.546 < p < 0.952; Supplementary Table S2). Thus, germination occurred at the same time regardless of whether zygotes were cultured in seawater at pH 8 or the more acidic pH 6.
Seawater Acidity Correlates With Reductions in Embryonic Growth Rates
Once formed, rhizoids elongate by tip growth, a process that could be affected by low extracellular pH since it involves the formation of an intracellular pH gradient. To evaluate this possibility, rhizoids were assessed 6 days AF and at this time embryos exposed to different pH levels were all multicellular with long rhizoids. This indicates that normal developmental processes like cell division and tip growth still occurred, regardless of pH (Figure 4). However, embryos exposed to acidic seawater had rhizoids that were visibly shorter than those cultured in ASW at pH 8.0, suggesting that reductions in external pH influence tip growth.
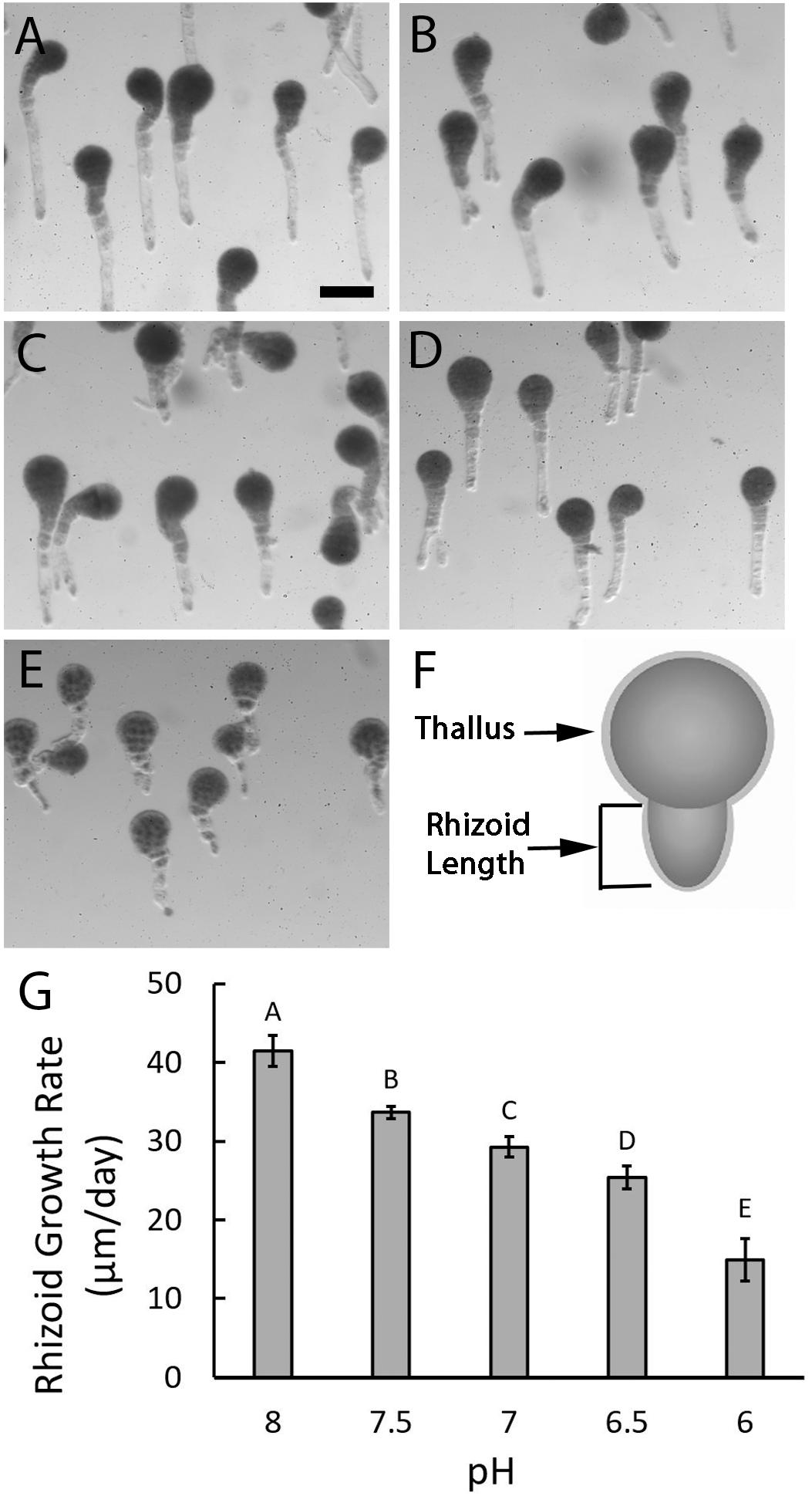
Figure 4. Rhizoid elongation rates are reduced in acidic seawater. Embryos growing in ASW at pH 8 (A) had long rhizoids when observed 6 days AF while those cultured in ASW at pH 7.5 (B), 7.0 (C), 6.5 (D), and 6.0 (E) were, respectively, shorter. Rhizoid lengths were measured from the base of the thallus to the tip of the rhizoid (F) on randomly selected embryos 1- and 6-days AF. Growth rates were determined by dividing the amount of growth that occurred between day 1 and day 6 by the 5 days. Quantification of rhizoid growth rates revealed progressive decreases with each 0.5 unit decrease in pH (G). Bars depict the average rate of rhizoid growth with SDs. Average growth rates represent data from four trials (N = 600 embryos on day 1 as well as day 6, for each trial). Different letters above the bars denote means that are statistically different (one-way ANOVA with post hoc Tukey’s test; 0.001 ≤ p ≤ 0.046). Size bar in (A) is 100 μm and applies to all photographs.
Embryos developing in ASW at pH 8.0 had rhizoids that elongated 41.48 μm/day (±1.95 SD), whereas embryos developing in ASW at pH 7.5, grew approximately 20% slower at 33.64 μm/day (±0.78 SD). At pH 7.0 growth rates dropped to 29.3 μm/day (±1.28 SD), almost a 30% reduction from pH 8.0. Further reductions in growth rates were observed with each 0.5 unit drop in pH to a minimum of 14.93 μm/day (±2.71 SD) in embryos developing at pH 6.0, a 64% reduction from pH 8.0. Statistical analyses confirmed that the decreases in growth rates observed with each incremental decline in pH were significantly different (one-way ANOVA with post hoc Tukey’s test, 0.001 ≤ p ≤ 0.046; Supplementary Table S3). Taken together, these results indicate that acidic conditions impair embryo development by reducing rhizoid elongation rates.
Discussion
Fucoid algae are integral components of the intertidal ecosystems that they occupy, providing food and shelter for many other species. Seaweeds growing in this environment are exposed to constantly changing and often stressful conditions, which are predicted to intensify with climate change. In coming years oceans are expected to acidify; global averages are predicted to decrease 0.32 units by the end of the century (Orr et al., 2005; IPCC, 2014). Any negative impacts of these changes on foundational species, like fucoid algae, could have cascading effects for entire intertidal ecosystems if their ability to persist in the long term is threatened (Schiel, 2006; Watt and Scrosati, 2013). In a thriving population adult stages must be able to produce viable offspring that can cope with future conditions. In fucoid algae, the importance of maintaining internal pH during early development has been shown (Gibbon and Kropf, 1994). In the current study, the effects of seawater acidification on embryonic growth and development were investigated.
Zygotes and embryos were grown in seawater buffered to conditions that approximate current global averages, encompass levels that have already been observed in Burrard Inlet, and include more extreme conditions. During the first 10–12 h AF zygotes of fucoid algae must maintain internal pH at a level compatible with cytosolic processes and generate a pH gradient in the cytoplasm along the rhizoid/thallus axis. Seawater acidification and the concomitant increases in H+ concentrations outside the cell could impact these processes since internal pH is regulated, at least in part, by controlling proton fluxes across the plasma membrane. If seawater acidification had interfered with cellular regulation of internal pH, we would expect to have seen an effect on the ability of zygotes to form a rhizoid/thallus axis and germinate. We found that seawater pH had no effect on zygotic development prior to germination. This conclusion is based on two lines of evidence. First, germination rates were equivalent and high for zygotes developing in all five pH treatments. Secondly, rhizoid formation occurred on the same developmental time-course, regardless of whether zygotes were incubated in seawater at pH 6 or 8. This suggests that when the pH of the external medium is reduced, zygotes are either able to regulate internal pH or can develop under a range of cytoplasmic pH conditions, at least prior to germination.
In a previous study, it was shown that zygotes of this stage were able to maintain a stable cytosolic pH of about 7.5 (within 0.2 units) when exposed to seawater with pH as low as 6.2 (Gibbon and Kropf, 1993). Consistent with these previous findings, we found zygotes were able to germinate on time (10–12 h AF) even in seawater with pH as low as 6.
At later developmental stages, reductions in external pH had negative impacts on growth. After germination, there were significant declines in rhizoid growth rate as pH was incrementally decreased. Even in ASW with a pH as high as 7.5, the growth rate was reduced by 19% compared to zygotes developing at pH 8. Rhizoid elongation continued to decline with further decreases in pH, culminating in a 64% reduction in growth at pH 6 when compared to zygotes growing in ASW at pH 8. At these stages of development, embryonic growth occurs in large part through polarized extension of the rhizoid tip, via a process known as tip growth. This type of cell expansion, which is conserved across diverse eukaryotic phyla, depends on the presence of a pH gradient parallel with the axis of elongation which is maintained by controlling proton concentrations in the cell [Gow et al., 1984; Gibbon and Kropf, 1994; Cárdenas, 2009; reviewed in Obermeyer and Feijó (2017) and Bascom et al. (2018)]. Previous studies using zygotes of a fucoid algae (Silvetia compressa, formerly known as Pelvetia fastigiata) have shown that the internal pH gradient is required for rhizoid elongation and that when the gradient is abolished, subsequent tip growth is inhibited. In addition, there is a correlation between the magnitude of the gradient and rate of growth (Gibbon and Kropf, 1994). Since we found that reductions in external pH also impaired rhizoid elongation, we propose that acidic seawater affects the ability of the embryo to maintain an internal pH gradient.
In tip growing cells, a pH gradient is formed when H+ ions move in at the growing tip where cytoplasmic pH is lowest and are either used in metabolic processes or expelled further back toward the base of the cell [Gibbon and Kropf, 1994; reviewed in Obermeyer and Feijó (2017)]. In embryos of fucoid algae growing in seawater at pH 8, H+ ions are more concentrated in the cytoplasm than outside the cell (Figure 5A). In this scenario, protons entering the cell at the tip move inward against their concentration gradient and passively flow outward further back along the rhizoid or are metabolized in the cytoplasm. As the external pH decreases from 8 to 7 or lower, the proton concentration in the surrounding medium would become higher than inside the cell (Figures 5B,C). It is now energetically favorable for protons to enter at the tip as they would be moving down a concentration gradient. Removal near the base, however, is more difficult because it would be unfavorable to expel excess protons that are not used up in metabolic processes within the cell. If external pH levels are too low, an excess of protons inside the cell could build up and affect the magnitude of the pH gradient, which would in turn inhibit rhizoid elongation (Gibbon and Kropf, 1994). In this report, significant reductions in rhizoid growth were observed with each incremental increase in seawater acidity, consistent with the idea that under acidic conditions it is more challenging for embryos to maintain a pH gradient in the rhizoid. To regulate proton flow in acidic seawater, a change in transporter activity at different regions in the rhizoid may be required if metabolic activities are insufficient to remove excess protons. Although, the mechanisms that regulate internal pH in fucoid algae are not well understood, studies using pharmalogical agents have implicated H+-ATPase and Na+/H+ antiporter activities as contributors (Gibbon and Kropf, 1993). Seawater acidification could also impact tip growth in additional ways. For example, the activity of enzymes in the cell wall could be affected by external pH, as each enzyme has a pH optimum where its activity is maximal. If enzymes involved in cell wall synthesis are not working efficiently, turgor driven growth may become slightly de-localized, allowing rhizoids to expand laterally as well as at the tip. This could result in shorter, slightly wider rhizoids. Regardless of the mechanisms, it appears to be difficult for embryos to adjust when the proton concentration is high in the external seawater, given that we observed reductions in rhizoid elongation rates under acidic conditions.
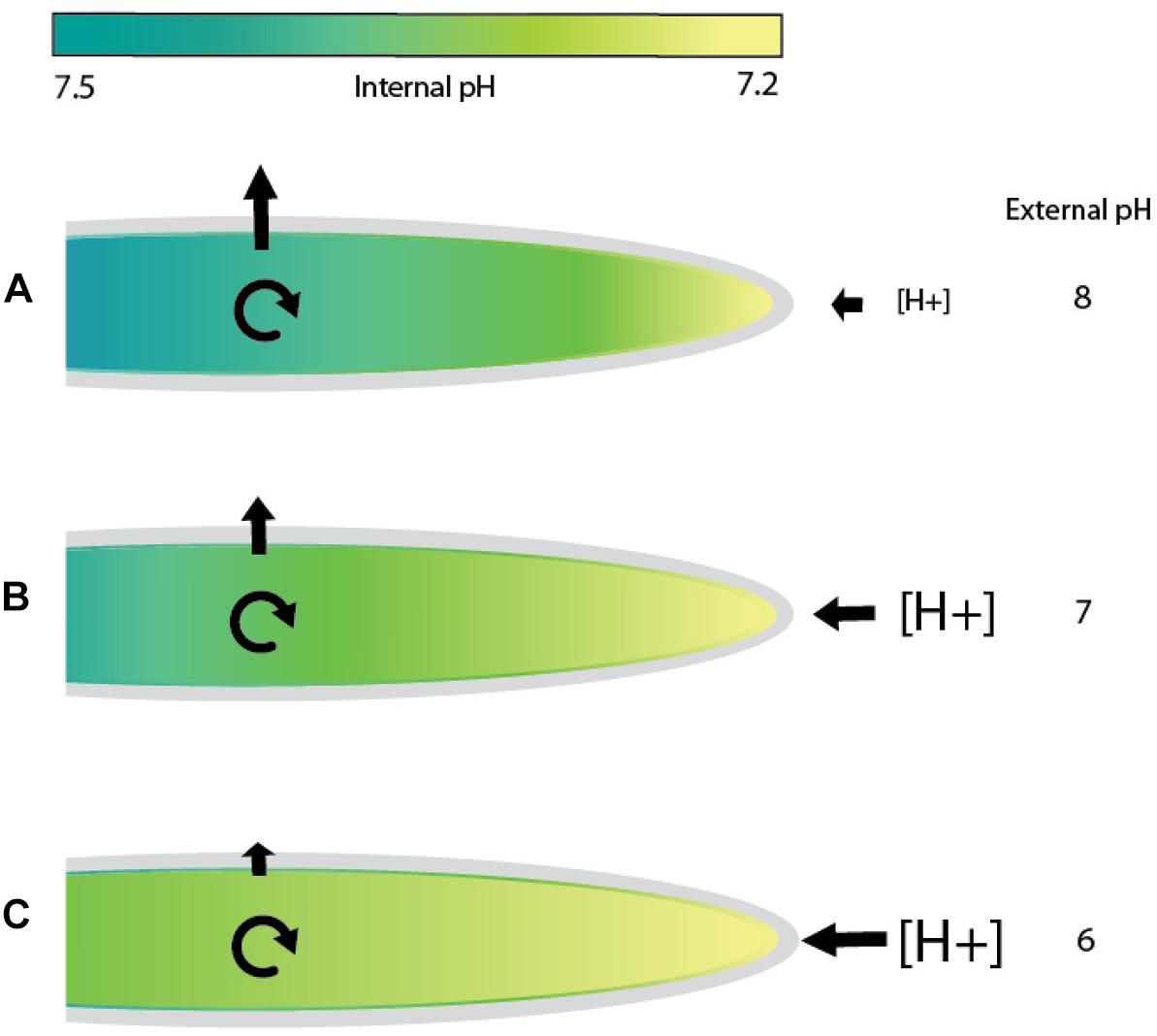
Figure 5. Model of proton flow in rhizoids exposed to seawater of differing pH values. In seawater at pH 8, tip growing rhizoids of fucoid algae maintain an internal gradient with a magnitude of 0.3–0.5 pH units (Gibbon and Kropf, 1994). Diagrams depict examples of cells with a pH of 7.2 at the growing tip and 7.5 further back at the base (indicated by differences in the color of the cytoplasm). (A) In seawater at pH 8, proton concentrations are higher inside the growing rhizoid than outside. Hydrogen ions would be transported into the rhizoid at the tip against a concentration gradient and are either metabolized or flow passively out of the cell further back near the base of the rhizoid. (B) At an external pH of 7, the concentration of protons would be slightly higher outside the cell than in the cytoplasm at the rhizoid tip, a condition that favors a passive influx of protons. However, expelling H+ions near the base would be energetically unfavorable as the proton concentration is higher outside the cell. (C) At an extremely acidic pH, such as 6, proton flow into the tip will be highly favorable, perhaps flooding the cytoplasm with H+ ions. Maintaining the internal pH gradient at normal levels would be more difficult as proton expulsion is now occurring against a large concentration gradient. This is shown in (B) and (C), where internal gradients would be disrupted by excess protons. Cyclic arrows indicate proton removal via metabolic processes and straight arrows represent proton movement across the plasma membrane. The colored bar indicates the magnitude of the cytosolic pH gradient. Increasing font sizes of [H+] symbolizes higher hydrogen ion concentrations in the external seawater.
Whether populations of fucoid algae can adapt or acclimate to more acidic conditions over the long term will depend on their ability to optimize enzyme activity, alter proton transport, or adjust metabolic activities enough to sustain optimal rhizoid growth. As it stands now, embryos are best adapted to growth in seawater at pH 8 and they cannot maintain optimal growth under more acidic conditions, since significant decreases in rhizoid elongation were observed with each incremental drop in seawater pH. As seawater pH decreases and H+ efflux becomes more difficult, embryos must alter their physiology to more efficiently remove excess protons. Currently pH fluctuates between a high of 8.2 down to 7.4 at the Barnet Marine Park. Given these fluctuations, it may be difficult for embryos to adjust to acidic conditions if changes in the localization patterns of the relevant transporters are required. It would be interesting to assess whether preexposure to acidic conditions of fronds with developing eggs and sperm, affects the sensitivity of subsequent embryonic development to low pH. However, this is difficult to address as reproductive fronds contain eggs at different stages of development and the fronds may be exposed to field conditions that change with weather and discharges from nearby industries in the weeks preceding collection, including fluctuations in pH levels. An alternative possibility would be to assess the effects of acidic seawater on embryos of fucoid algae from other sites with more stable pH levels closer to 8, and/or less impacted by anthropogenic inputs.
Regardless of their ability to adjust, embryos are still able to grow, even at the most acidic pH, which is well below projections for global ocean acidification by the end of the century (IPCC, 2014). Under current conditions the assemblage of fucoid algae at the Barnet Marine Park is surviving. This suggests that the impacts on embryonic growth from fluctuations in pH at this site have not yet been enough to have a major impact on the population as whole, although whether it has been regressing over time is unknown. This study, however, shows that future declines in seawater pH will impair embryonic growth. This shift could compromise the ability of the population to persist in the long term since embryos are thought to be more vulnerable to predation and other environmental stressors than mature algae and other stressors such as temperature are also expected to increase in the coming years.
Data Availability Statement
All datasets generated for this study are included in the article/Supplementary Material.
Author Contributions
BS and KO were responsible for sample collections in the field, and conducting experimental trials, data collection and analysis. BS and SB prepared the manuscript including: figures, statistical analyses, interpretation of results, and written portions. SB was the principal investigator who conceived the idea for the project, oversaw the research, and provided mentorship, lab facilities, as well as funding. BS, KO, and SB collaborated on experimental design and methodology.
Funding
This study was supported by the Natural Sciences and Engineering Research Council of Canada (NSERC) Discovery Grant (327163-2012) awarded to SB: provided primary funding for research project including materials, supplies, and support for lab work and field collections. NSERC undergraduate research award to BS: provided stipend to conduct research for one semester. Article processing charges were supported by the Simon Fraser University’s Open Access Author Fund.
Conflict of Interest
The authors declare that the research was conducted in the absence of any commercial or financial relationships that could be construed as a potential conflict of interest.
Acknowledgments
We thank Hae-Ryoung Kim for his technical assistance in both the field and lab.
Supplementary Material
The Supplementary Material for this article can be found online at: https://www.frontiersin.org/articles/10.3389/fmars.2019.00755/full#supplementary-material
Footnotes
References
Al-Janabi, B., Kruse, I., Graiff, A., Winde, V., Lenz, M., and Wahl, M. (2016). Buffering and amplifying interactions among OAW (Ocean Acidification & Warming) and nutrient enrichment on early lifestage fucus vesiculosus L. (Phaeophyceae) and their carry over effects to hypoxia impact. PLoS One 11:e0152948. doi: 10.1371/journal.pone.0152948
Bascom, C. S. Jr., Hepler, P. K., and Bezanilla, M. (2018). Interplay between Ions, the cytoskeleton, and cell wall properties during tip growth. Plant Physiol. 176, 28–40. doi: 10.1104/pp.17.01466
Bellgrove, A., Mckenzie, P. F., Cameron, H., and Pocklington, J. B. (2017). Restoring rocky intertidal communities: lessons from a benthic macroalgal ecosystem engineer. Mar. Pollut. Bull. 117, 17–27. doi: 10.1016/j.marpolbul.2017.02.012
Bisgrove, S. R., and Kropf, D. L. (2001). Cell wall deposition during morphogenesis in fucoid algae. Planta 212, 648–658. doi: 10.1007/s004250000434
Bisgrove, S. R., and Kropf, D. L. (2007). Asymmetric cell divisions: zygotes of fucoid algae as a model system. Plant Cell Monogr. 9, 323–341. doi: 10.1007/7089_2007_134
Cárdenas, L. (2009). New findings in the mechanisms regulating polar growth in root hair cells. Plant Signal. Behav. 4, 4–8. doi: 10.4161/psb.4.1.7341
Chan, F., Barth, J. A., Blanchette, C. A., Byrne, R. H., Chavez, F., Cheriton, O., et al. (2017). Persistent spatial structuring of coastal ocean acidification in the California current system. Sci. Rep. 7:2526. doi: 10.1038/s41598-017-02777-y
Doney, S. C., Fabry, V. J., Feely, R. A., and Kleypas, J. A. (2009). Ocean acidification: the other CO2 problem. Annu. Rev. Mar. Sci. 1, 169–192. doi: 10.1146/annurev.marine.010908.163834
Ferreira, G., Arenas, F., Martinez, B., Hawkins, S. J., and Jenkins, S. R. (2014). Physiological response of fucoid algae to environmental stress: comparing range centre and southern populations. New Phytol. 202, 1157–1172. doi: 10.1111/nph.12749
Gazeau, F., Parker, L. M., Comeau, S., Gattuso, J., O’Connor, W. A., Martin, S., et al. (2013). Impacts of ocean acidification on marine shelled molluscs. Mar. Biol. 160, 2207–2245. doi: 10.1007/s00227-013-2219-3
Gibbon, B. C., and Kropf, D. L. (1993). Intracellular pH and Its regulation in pelvetia zygotes. Dev. Biol. 157, 259–268. doi: 10.1006/dbio.1993.1130
Gibbon, B. C., and Kropf, D. L. (1994). Cytosolic pH gradients associated with tip growth. Science 263, 1419–1421. doi: 10.1126/science.263.5152.1419
Gow, N. A. R., Kropf, D. L., and Harold, F. M. (1984). growing hyphae of achlya bisexualis generate a longitudinal pH gradient in the surrounding medium. J. Gen. Microbiol. 130, 2967–2974. doi: 10.1099/00221287-130-11-2967
Graiff, A., Bartsch, I., Ruth, W., Wahl, M., and Karsten, U. (2015). Season exerts differential effects of ocean acidification and warming on growth and carbon metabolism of the seaweed fucus vesiculosus in the western baltic sea. Front. Mar. Sci. 2:112. doi: 10.3389/fmars.2015.00112
Gutow, L., Rahman, M. M., Bartl, K., Saborowski, R., Bartsch, I., Wiencke, C., et al. (2014). Ocean acidification affects growth but not nutritional quality of the seaweed fucus vesiculosus (Phaeophyceae. Fucales). J. Exp. Mar. Bio. Ecol. 453, 84–90. doi: 10.1016/j.jembe.2014.01.005
Hable, W. E. (2014). Rac1 signaling in the establishment of the fucoid algal body plan. Front. Plant Sci. 5:690. doi: 10.3389/fpls.2014.00690
Hofmann, L. C., and Bischof, K. (2014). Ocean acidification effects on calcifying macroalgae. Aquat. Biol. 22, 261–279. doi: 10.3354/ab00581
IPCC (2014). “Climate change 2014: synthesis report,” in Contribution of Working Groups I, II and III to the Fifth Assessment Report of the Intergovernmental Panel on Climate Change, eds R. K. Pachauri and L. A. Meyer, (Geneva: IPCC), 151.
Johannessen, S. C., and Macdonald, R. W. (2009). Effects of local and global change on an inland sea: the strait of georgia, British Columbia, Canada. Clim. Res. 40, 1–21. doi: 10.3354/cr00819
Koch, M., Bowes, G., Ross, C., and Zhang, X. H. (2013). Climate change and ocean acidification effects on seagrasses and marine macroalgae. Glob. Change Biol. 19, 103–132. doi: 10.1111/j.1365-2486.2012.02791.x
Kropf, D. L. (1997). Induction of polarity in fucoid zygotes. Plant Cell 9, 1011–1020. doi: 10.1105/tpc.9.7.1011
Kropf, D. L., Henry, C. A., and Gibbon, B. C. (1995a). Measurement and manipulation of cytosolic pH in polarizing zygotes. Eur. J. Cell Biol. 68, 297–305.
Kropf, D. L., Money, N. P., and Gibbon, B. C. (1995b). Role of cytosolic pH in axis establishment and tip growth. Can. J. Bot. 73, 126–130. doi: 10.1139/b95-235
Lauze, J. F., and Hable, W. E. (2017). Impaired growth and reproductive capacity in marine rockweeds following prolonged environmental contaminant exposure. Bot. Mar. 60, 137–148. doi: 10.1515/bot-2016-0067
Marliave, J. B., Gibbs, C. J., Gibbs, D. M., Lamb, A. O., and Young, S. J. F. (2011). “Biodiversity stability of shallow marine benthos in strait of georgia, british columbia, canada through climate regimes, overfishing and ocean acidification,” in Biodiversity Loss in a Changing Planet, eds O. Grillo and G. Venora, (London: IntechOpen).
NASA JPL (2019). Data from: Multi-Scale Ultra-High Resolution (MUR) SST Analysis fv04.1, Global, 0.01°, 2002-Present, Daily. Griddap Dataset. Available at http://coastwatch.pfeg.noaa.gov/erddap/griddap/jplMURSST41.html (accessed August, 2019).
Obermeyer, G., and Feijó, J. (2017). Pollen Tip Growth: From Biophysical Aspects to Systems Biology. London: Springer Nature, 1–424. doi: 10.1007/978-3-319-56645-0
Olischläger, M., Bartsch, I., Gutow, L., and Wiencke, C. (2012). Effects of ocean acidification on different life-cycle stages of the kelp Laminaria hyperborea (Phaeophyceae). Bot. Mar. 55, 511–525. doi: 10.1515/bot-2012-0163
Orr, J. C., Fabry, V. J., Aumont, O., Bopp, L., Doney, S. C., Feely, R. A., et al. (2005). Anthropogenic ocean acidification over the twenty-first century and its impact on calcifying organisms. 437, 681–686. doi: 10.1038/nature04095
Schiel, D. R. (2006). Rivets or bolts? When single species count in the function of temperate rocky reef communities. J. Exp. Mar. Biol. Ecol. 338, 233–252. doi: 10.1016/j.jembe.2006.06.023
Scrosati, R. A. (2017). Community-level facilitation by macroalgal foundation species peaks at an intermediate level of environmental stress. Algae 32, 41–46. doi: 10.4490/algae.2017.32.2.20
Takeuchi, Y., Schmid, J., Caldwell, J. H., and Harold, F. M. (1988). Transcellular ion currents and extension of neurospora crassa hyphae. J. Membr. Biol. 101, 33–41. doi: 10.1007/BF01872817
Watt, C. A., and Scrosati, R. A. (2013). Bioengineer effects on understory species richness, diversity, and composition change along an environmental stress gradient: experimental and mensurative evidence. Estuar. Coast. Shelf Sci. 123, 10–18. doi: 10.1016/j.ecss.2013.02.006
Keywords: ocean acidification, fucoid algae, rhizoid elongation (tip growth), effects of climate change, algal development
Citation: Schiltroth BWJ, Ohori KT and Bisgrove SR (2019) Impacts of Acidic Seawater on Early Developmental Stages of Fucus gardneri at Burrard Inlet, British Columbia. Front. Mar. Sci. 6:755. doi: 10.3389/fmars.2019.00755
Received: 02 July 2019; Accepted: 21 November 2019;
Published: 05 December 2019.
Edited by:
Iris Eline Hendriks, University of the Balearic Islands, SpainReviewed by:
Whitney E. Hable, University of Massachusetts Dartmouth, United StatesValentina Asnaghi, University of Genoa, Italy
Copyright © 2019 Schiltroth, Ohori and Bisgrove. This is an open-access article distributed under the terms of the Creative Commons Attribution License (CC BY). The use, distribution or reproduction in other forums is permitted, provided the original author(s) and the copyright owner(s) are credited and that the original publication in this journal is cited, in accordance with accepted academic practice. No use, distribution or reproduction is permitted which does not comply with these terms.
*Correspondence: Sherryl R. Bisgrove, sbisgrov@sfu.ca