Sperm Motility Impairment in Free Spawning Invertebrates Under Near-Future Level of Ocean Acidification: Uncovering the Mechanism
- 1Department of Biology and Evolution of Marine Organisms, Stazione Zoologica Anton Dohrn, Naples, Italy
- 2Department of Sciences, University of Basilicata, Potenza, Italy
Ocean acidification (OA) refers to the decrease in ocean water pH resulting from the increasing absorption of atmospheric CO2. This will cause changes in the ocean’s carbonate chemistry system with a resulting impact on reproduction of marine organisms. Reproduction is the fundamental process that allows the conservation of the species; in free-spawning marine invertebrates, this process is highly sensitive to changes in seawater quality and chemistry. To date, the majority of the studies concerned OA effects on reproduction has been focused on embryo and larval development. Despite several evidence for the impairment of reproductive success by environmental perturbations through altering gamete quality, sperm physiological responses to OA are poorly investigated. In this study, we evaluated the effects of exposure to acidified seawater (AcSW) (pH 7.8), which approximate the predicted global averages for oceanic surface waters at the end of this century, on sperm quality of the mussel Mytilus galloprovincialis and the ascidian Ciona robusta by evaluating several endpoints, such as motility, vitality, mitochondrial activity, oxidative state, and intracellular pH (pHi). Following sperm exposure to AcSW, the percentage of motile spermatozoa, mitochondrial activity and pHi decreased in comparison to the current seawater pH of 8.1, whereas vitality and oxidative state were unaffected by the low external pH in both the species. In broadcast spawners, a relationship between sperm intracellular pH and the initiation of motility are well known. Spermatozoa are immotile in the testes and motility is induced after the spermatozoa are released into seawater; the alkaline pH of seawater, in fact, increases the pHi activating motility and mitochondrial respiration. The results of this study suggest that the lowering of seawater pH as predicted to occur for 2100, through the inhibition of pHi increase, prevent sperm motility activation. Sperm motility is a key determinant of fertilization success; consequently, a corresponding drop in fertilization success would be expected with important implications for the fitness and the survival of marine invertebrates.
Introduction
Ocean acidification (OA) is a claimed issue of global change started over than 250 years ago when the industrial era has taken place (Feely et al., 2008). It can be defined as a long-term decrease in oceanic water pH mainly due to the human-driven intensification of the atmospheric carbon dioxide (CO2) (Doney et al., 2009). Oceans work as moderator of climate change. As one of the largest natural reservoirs of carbon, they absorb approximately a quarter of anthropogenic CO2 (generated by fossil fuel, deforestation and other industrial and agricultural activities) emitted into the atmosphere playing a critical role in the global carbon cycle. The atmospheric man-made CO2 dissolves in the seawater increasing the bicarbonate ions and decreasing the carbonate ions. These have already caused a drop of the global mean seawater pH levels of 0.1 units compared with pre-industrial levels (pH 8.2) (Orr et al., 2005) and are projected to decrease the oceanic surface waters pH by approximately 0.3–0.5 pH units by the end of the century (Pachauri et al., 2014: synthesis report. Contribution of Working Groups I, II and III to the fifth assessment report of the Intergovernmental Panel on Climate Change 2014, Meehl et al., 2007). It has been well documented that marine organisms with calcium carbonate structures are most sensitive to OA (Kroeker et al., 2010; Gazeau et al., 2014). Laboratory and mesocosm experiments showed that OA causes substantial changes in overall calcification rates for many species of marine calcifiers including corals, coccolithophores, foraminifera, pteropods, echinoderms, bivalves (Feely et al., 2008). In addition, a meta-analysis combination of different taxonomic groups revealed that survival, growth, and reproduction were all negatively affected by OA (Kroeker et al., 2010).
Reproduction is a fundamental aspect in the life cycle of organisms allowing the conservation of the species. It strongly depends on gamete quality, which is defined as the ability to fertilize or to be fertilized, and subsequently develop into a normal embryo (Bobe and Labbé, 2010). Gamete quality has demonstrated to be highly sensitive to changes in seawater quality and chemistry (Byrne et al., 2008, 2010; Gallo and Tosti, 2019). In marine organisms characterized by external fertilization, gametes are released into the seawater and, consequently, are directly exposed to chemical and physical stressors. These can produce adverse effects on gamete quality and, therefore on fertilization success, embryo development, larval viability and subsequently species fitness and survival (Tosti, 1994). To date, the adverse effects of OA on embryo and larval development are widely documented. Exposure to acidified seawater (AcSW) has been demonstrated to negatively affect fertilization and embryo and/or larval development in different species of sea urchin (Kurihara and Shirayama, 2004; Carr et al., 2006; Havenhand et al., 2008; Byrne et al., 2009; Brennand et al., 2010; Lenz et al., 2019) bivalve molluscs (Kurihara et al., 2007; Parker et al., 2009; Guo et al., 2015; Kong et al., 2019) and polychaetes (Díaz-Castañeda et al., 2019). On the contrary, the effects of OA on gamete quality is poorly investigated despite several evidence for the impairment of reproductive success by chemo-physical stressors through altering the gamete quality (Gallo et al., 2011, 2016, 2018a,b; Gallo and Tosti, 2013, 2015; Boni et al., 2016; Gallo, 2018; Rotini et al., 2018).
Sperm quality is defined as the ability to successfully fertilize an oocyte and, subsequently, to allow the development of normal embryo (Bobe and Labbé, 2010). It is related to various cellular parameters such as morphology, viability, motility, intracellular pH (pHi), membrane and DNA integrity, mitochondrial activity, and oxidative status (Bobe and Labbé, 2010; Cabrita et al., 2014). Nowadays, very little is known on the impacts of OA exposure on marine invertebrate spermatozoa. Studies on sperm physiological responses to OA are scarce and mainly focused on few sperm quality parameters, in particular motility, in different broadcast spawning marine invertebrates such as sea urchins, corals, oysters, polychaetes (Christen et al., 1982; Levitan, 2000; Kupriyanova and Havenhand, 2005; Havenhand and Schlegel, 2009; Morita et al., 2010; Schlegel et al., 2014, 2015) while ascidians and mussels despite their ecological importance, remain understudied. To fill this gap, the present study aimed at investigating sperm quality in Mytilus galloprovincialis and Ciona robusta after direct exposure to AcSW (pH 7.8) predicted for the end of this century. The ascidian C. robusta and the mussel M. galloprovincialis are benthic, filter feeding organisms living in shallow water. They are characterized by external fertilization, a pelagic larval stage and a benthic adult stage (Satoh, 1994; Parker et al., 2013). The mussel M. galloprovincialis provides an essential ecosystem services including habitat structure for benthic organisms, acts as a biological filter to purify polluted estuarine water, and is a food source for other organisms (Parker et al., 2013). The ascidian C. robusta plays an important ecological role in marine biodiversity and ecosystem functioning; it is able to grow in eutrophic environments and to colonize natural and artificial substrates in protected harbors (Shenkar and Swalla, 2011). In this study, the effects of sperm exposure to OA have been assessed by evaluating several endpoints, such as motility, vitality, mitochondrial activity, oxidative state, and pHi, in order to provide valuable insight into the impact of near-future OA on marine invertebrate reproduction elucidating the possible mechanism of OA induced reproductive impairment.
Materials and Methods
Animal Collection
Adult specimens of C. robusta and M. galloprovincialis were collected from the Gulf of Naples (Italy) in January and February 2018, when animals are sexually mature. After collection, they were transported to Stazione Zoologica Unit of Marine Resources for Research laboratory and maintained in aquaria with running seawater at 18°C (38 PSU salinity, pH 8.1 ± 0.1) for at least 7 days until the experiments. Once daily, the animals were fed with a mixture of marine microalgae (Shellfish Diet 1800tm, Reed Mariculture Inc., Campbell, CA, United States), approximately 250 × 106 microalgae per animal.
The ascidian C. robusta and the mussel M. galloprovincialis are not protected by any environmental agency in Italy and Europe.
Sperm Collection
After anesthetization of C. robusta on ice, spermatozoa were collected from the sperm duct with a Pasteur pipette and stored “dry” in a test tube on ice. To harvest spermatozoa from M. galloprovincialis, they were opened with a scalpel, their mantles were slightly cut and the resulting sperm mass was collected with a Pasteur pipette and stored “dry” in a test tube on ice.
In both species, an aliquot of spermatozoa collected from each male was diluted in filtered natural seawater (FNSW) to previously check morphology, motility and concentration under a light microscope using the Makler sperm counting chamber. Then dry spermatozoa of two individuals for each species were pooled and used for the experiments.
Acidified Seawater and Carbonate Chemistry
Natural seawater collected from an uncontaminated area in the Bay of Naples was used throughout the experiments after filtration by 0.22 μm membrane filter (Millipore; Milli Q, Medford, MA, Unite States). Two pH levels were compared in this study: the present seawater pH of 8.1 (control; CTRL) and the lower pH of 7.8 representing the OA scenario in 2100. The desired experimental pH level was obtained by bubbling CO2 through FNSW by means of a bubbling system supplying CO2, which was plugged with a pH controller adjusted at pH 7.8; once the required pH was achieved, the supply of CO2 was halted via an automated feedback relay system.
Chemical parameters of seawater were determined: the pH (pHNBS) was measured using a pH meter (Mettler Toledo FiveEasy) calibrated with NBS standard buffers, dissolved oxygen, salinity and temperature were measured with a YSI 85 multiparameter meter, total alkalinity was determined using an automatic titrator system (Mettler Toledo G20S) interfaced with the data acquisition software LabX. The pCO2 and dissolved organic carbon were calculated as a function of pHNBS, temperature, salinity, and total alkalinity by using the open source program CO2SYS (Pierrot et al., 2006) with the constants from Mehrbach et al. (1973) refit by Dickson and Millero (1987), and KHSO4 dissociation constant from Dickson (1990) (Table 1). Soon after collection, aliquots of dry spermatozoa were added to test chambers containing 1 ml of ambient (CTRL) and AcSW to achieve the final sperm concentration needed for each quality parameter assessment, limiting the time lag between the first and last aliquot preparation. After 30 and 60 min of incubation, the seawater pH was checked to make sure it has not changed and then spermatozoa were tested for different quality parameters. The exposure time was selected based on sperm longevity time after spawning, i.e., 1 h for the ascidian C. robusta and 4 h for the mussel M. galloprovincialis (Sedano et al., 1995; Bolton and Havenhand, 1996). For each species, each experiment consisted of four experimental groups: spermatozoa exposed to ambient seawater for 30 and 60 min and spermatozoa exposed to AcSW for 30 and 60 min. Each experiment was performed five times with three technical replicates at 18°C, which is equivalent to the seawater temperature during the spawning period.
Motility
After adding 1% bovine serum albumin in FNSW in 1:1 v/v ratio to prevent sperm from adhering to the glass surfaces, an aliquot of sperm suspension (4 × 106 spermatozoa/ml) was placed on a Makler sperm counting chamber, in which spermatozoa form in a monolayer. Sperm movement was recorded for 2 s at 50 frames s–1 using a digital camera (Axiocam 506 mono, Ziess, Germany) mounted on a microscope (Axio Imager Z.2, Zeiss, Germany). Videos in uncompressed AVI format were directly analyzed using the free Computer Assisted Sperm Analyzer software that is a plugin open source for the software ImageJ (Wilson-Leedy and Ingermann, 2007). Acquisition parameters were set on those used to analyze fish sperm motility; expect for sperm size, which was modified in each species according to the sperm characteristics to accurately detect motile spermatozoa, i.e., 35 and 50 maximum sperm size (pixels) in C. robusta and M. galloprovincialis, respectively (Supplementary Table S1). Three different randomly selected microscopic fields were scanned in each semen sample. The percentage of motile spermatozoa was calculated as a mean of that measured by software in the three scans for each microscopic field.
Mitochondrial Membrane Potential (MMP)
JC-1 (5,50,6,60-tetrachloro-1,10,3,30-tetraethylbenzimidazolylca rbocyanine iodide; Life Technologies, Milan, Italy) is a lipophilic vital probe commonly used to detect the mitochondrial membrane potential (MMP) (Cottet-Rousselle et al., 2011). Passing through the membrane cells, it leads to the detection of mitochondrial activity, since it has reversible transformations from aggregate to monomer forms as MMP changes. The aggregate form emits red fluorescence (∼595 nm) while monomer form emits green fluorescence (∼535 nm) (Boni et al., 2016; Gallo et al., 2016, 2018c). Aliquots of spermatozoa exposed to pH 8.1 and pH 7.8, with a concentration of 1 × 106 spermatozoa/ml, were stained by adding 5 μM JC-1, diluted from a 7.7 mM stock solution in DMSO. The final concentration of DMSO was 0.5%, which was tested in preliminary studies assessing that this concentration did not exert significant effect on sperm quality parameters in ascidian and mussel (Di Matteo et al., 2009; Shiba and Inaba, 2014). Spermatozoa were incubated for 30 min at 18°C and then centrifuged for 15 min at 800 × g at 4°C, the pellet resuspended in FNSW and transferred to quartz cuvette for spectrofluorometric analysis using 488 nm excitation and 500–650 nm emission wavelengths. MMP value was calculated as red to green fluorescence emission peaks ratio. A positive control was prepared by exposing JC-1 stained spermatozoa to the mitochondrial uncoupler carbonyl cyanide m-chlorophenyl hydrazone (5 μM, CCCP, Life technologies, Milan, Italy) that perturbs and shifts MMP from red to green fluorescence (low MMP).
Intracellular Reactive Oxygen Species (ROS) Determination
In order to evaluate the intracellular reactive oxygen species (ROS) levels, two different oxidation-sensitive fluorescent probes were employed: the 2′,7′-dichlorodihydrofluorescein diacetate (H2DCFDA; Life technologies, Milan, Italy), specific for the detection of the intracellular content of hydrogen peroxides (H2O2), and the hydroethidine (HE; Life technologies, Milan, Italy), which allows to analyze the intracellular content of superoxide anions (O2–).
The fluorophore H2DCF-DA passively penetrates cell membranes and is incorporated into hydrophobic regions of the cell. The de-acetylation of H2DCFDA by cellular esterases forms the non-permeating oxidant-sensitive compound, 2′,7′-dichlorodihydrofluorescein (H2DCF). This is oxidized by H2O2 to the highly fluorescent 2′,7′-dichlorofluorescein (DCF) which emits fluorescence at ∼530 nm in response to 488 nm excitation wavelengths. Sperm aliquots (5 × 106 cell/ml) were stained by adding 10 μM H2DCF-DA and incubated for 30 min in the dark at 18°C. Then, sperm suspensions were washed by centrifugation for 15 min at 800 × g and 4°C, the pellet was resuspended in FNSW and incubated for additional 30 min. Subsequently, after a wash, the pellet was resuspended in FNSW and transferred to the quartz cuvette for spectrofluorometric analysis. A positive control was prepared by incubating sperm suspension with 25 μM hydrogen peroxide. Fluorescence intensity peak was measured at emission wavelength of ∼530 nm using 488 nm excitation wavelength and related to H2O2 intracellular levels (Gallo et al., 2018b).
The probe HE is the sodium borohydride-reduced form of ethidium. It is freely permeable to cells and, when oxidized by O2–, it generates two red fluorescent products whose emission spectra overlap: the unspecific oxidation product, ethidium, which binds to DNA emitting at 610 nm in response to 488-nm excitation, and the highly specific red fluorescent product, 2-hydroxyethidium. This is selectively detected by setting the excitation wavelength at 350 nm (Robinson et al., 2006).
Aliquots of spermatozoa (30 × 106 spermatozoa/ml) were loaded with 20 μM HE and incubated for 30 min in the dark at 18°C. After that, samples were transferred to FNSW and then to the quartz cuvette for spectrofluorometric analysis. A positive control was prepared by incubating the sperm suspension with 20 μM menadione, a redox active quinone that generates O2–. Fluorescence intensity peaks were measured recording fluorescent emission spectra from 600 to 650 nm after excitation at ∼350 nm wavelength.
Lipid Peroxidation
The fluorescent membrane probe C11-BODIPY581/591 (Life Technology, Milan, Italy) was used to quantify lipid peroxidation of plasma membranes. As fatty acid analog, this lipophilic probe is easily incorporated into membranes. It is sensitive to fatty acid oxidation shifting from red (590 nm) to green (510 nm) fluorescence upon oxidation. Sperm suspensions (2 × 107 spermatozoa/ml) were incubated for 30 min in the dark at 18°C with 5 μM C11-BODIPY581/591 in DMSO. Then, spermatozoa were centrifuged and the pellet resuspended in FNSW. A positive control was prepared by incubating the samples with two peroxidation promoters (150 μM ferrous sulfate and 750 μM vitamin C). Then spectrofluorometric analysis was performed recording the fluorescence intensity spectra at 500–650 nm emission wavelengths, after excitation at 488 nm. Lipid peroxidation value was determined as a ratio between fluorescence emission peak value at ∼520 nm and the sum of fluorescence emission peak values at ∼520 and ∼590 nm.
Intracellular pH (pHi)
The pH sensitive fluorescent chromophore 2′,7′-bis-(2-carboxyethyl)-5-(and-6) carboxyfluorescein acetoxymethyl ester (BCECF-AM; Life Technology, Milan, Italy) was used to evaluate the pHi. It freely diffuses through the plasma membrane and, in the cell, it is hydrolyzed by esterases resulting in the cytoplasmic indicator BCECF whose fluorescence intensity depends on the pHi. The ester form of BCECF (5 μM BCECF-AM) was loaded to the sperm suspensions (1 × 106 cell/ml) and incubated in the dark at 18°C for 30 min. Then, the suspensions were centrifuged at 800 × g at 4°C for 15 min, the sperm pellet was resuspended in FNSW and transferred to the quartz cuvette for spectrofluorometric analysis. The pHi value was measured by alternately exciting the samples at 440 nm and 490 nm and expressing as a ratio the variation of fluorescence emission peak values at 535 nm. This ratio was converted in pHi by using a calibration curve (Supplementary Figure S1). The latter was constructed on each experiment by incubating sperm suspensions in a calibration buffer solution (135 mM KCl, 5 mM HEPES, 290 mOsm) at pH 6.5, 7.2, and 8.0, in presence of 5 μM nigericin (Sigma Aldrich, Milan, Italy). Nigericin equilibrates intracellular and extracellular pH acting as antiporter, promoting K+/H+ exchange. As consequence, the pHi value derived from the linear relationship between external (and internal) concentrations of K+ and the fluorescence intensity ratio between 490 and 440 nm excitation.
Sperm Viability
Sperm viability was evaluated using the LIVE/DEAD Sperm Viability Kit (Life Technologies, Milan, Italy). This kit is made up of a SYBR-14 stock solution (1 mM in DMSO) and a propidium iodide solution (PI; 2.4 mM solution in water). The SYBR-14 selectively permeates cells characterized by membrane integrity (live spermatozoa) while PI is able to permeate only cells that lost their membrane integrity (dead spermatozoa). Fluorescent staining was carried out as reported in Gallo et al., 2018a. Briefly, an aliquot of 5 × 106 spermatozoa/ml was stained by adding SYBR-14 (final concentration 100 nM) and incubated in the dark for 15 min. Then, PI (final concentration 12 μM) was added to the sample and incubated for other 15 min. Samples were washed and then resuspended in FNSW. As final step using the spectrofluorometer (Shimadzu RF-5301PC, Japan) and a quartz microtube (10 × 4 mm, high precision, Hellma Analytics, Müllheim, Germany),we detected the specific fluorescent emission peaks. The excitation wavelength was 488 nm for SYBR-14 and 545 nm for PI. The emission spectra were recorded at 500–530 nm and at 570–650 nm, respectively. Sperm viability was determined as a ratio between live spermatozoa (SYBR-14 stained) and the sum of dead (PI-stained) and live spermatozoa.
Statistical Analysis
Data were reported as mean ± standard error (SE) and were tested for normal distribution by the Shapiro–Wilk test and for variance homogeneity by Leven’s test. Since the two assumptions were accepted, the two-way analysis of variance (ANOVA) followed by Tukey test was performed to test for significant differences between CTRL and AcSW exposed spermatozoa and among the time exposure groups. For values expressed as percentages, such as motility, data were analyzed after arcsine transformation to achieve normality. For the pHi, whose values were not characterized by a continuous distribution, a transformation in H+ concentration was applied. The significance level was set at P ≤ 0.05.
Results
Motility
In M. galloprovincialis, after 30 min exposure to FNSW, the percentage of motility in control spermatozoa was 66.9 ± 3.9% and it significantly dropped at 50% ± 2.0 after 60 min (P ≤ 0.01). After 30 min and 60 min of exposure to AcSW, the motility rate significantly decreased compared to the control spermatozoa (54.4 ± 4.5% vs. 34.4 ± 4.7%; P ≤ 0.05). A significant difference between the two time exposure groups was also observed (P ≤ 0.05) (Figure 1A).
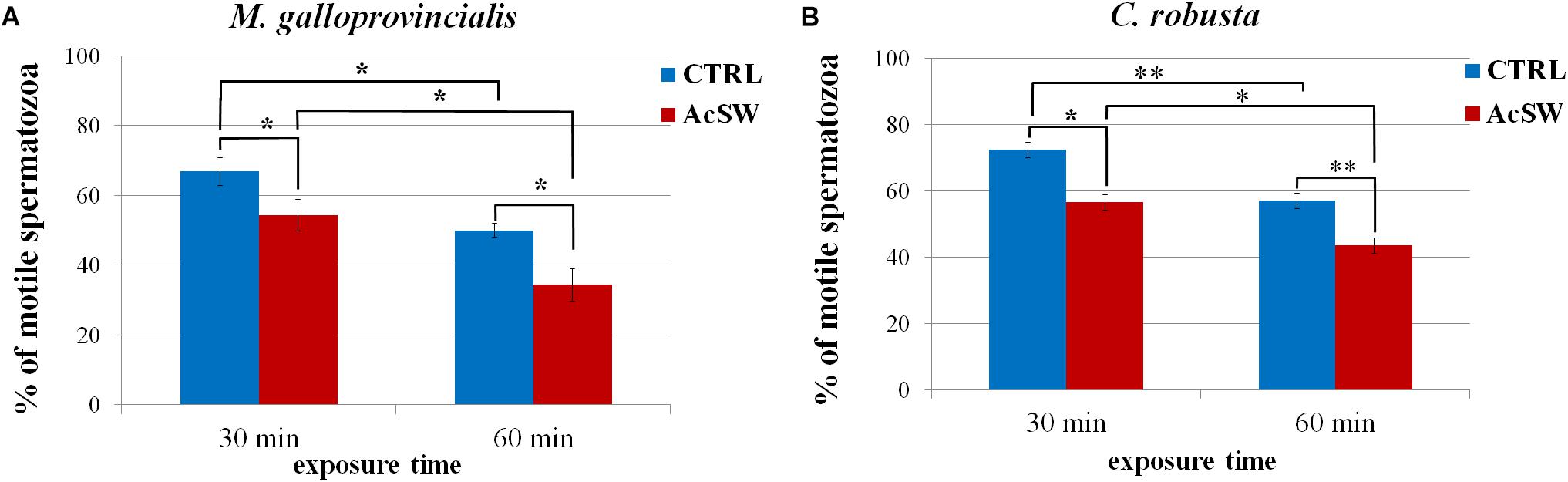
Figure 1. Effects of acidified seawater (AcSW) on sperm motility in M. galloprovincialis (A) and C. robusta (B) (mean ± SE). The percentage of motile spermatozoa was assessed in spermatozoa (CTRL) exposed to filtered natural seawater with a natural pH of 8.1 and in spermatozoa exposed to (AcSW) with a pH value of 7.8. ∗ and ∗∗ indicate significant (P ≤ 0.05) and high significant (P ≤ 0.01) differences.
Similarly, in C. robusta, the percentage of motile spermatozoa significantly (P ≤ 0.001) decreased in AcSW compared to control spermatozoa (72.4 ± 2.4% vs. 56.5 ± 2.4% after 30 min exposure and 57.0 ± 2.4% vs. 43.5 ± 2.4% after 30 min exposure). In addition, also in C. robusta there was a significant variation between the two exposure time groups (P ≤ 0.01) (Figure 1B).
Mitochondrial Membrane Potential (MMP)
Sperm exposure to AcSW significantly affected mitochondrial activity in both species. In particular, in M. galloprovincialis, in untreated spermatozoa the MMP values were 14.9 ± 2.1 and 10.5 ± 1.1 after 30 and 60 min, respectively. These significantly decreased after exposure to AcSW for 30 and 60 min (10.1 ± 0.2 and 8.0 ± 0.6; respectively; P ≤ 0.05) (Figure 2A). The MMP in C. robusta control spermatozoa was 6.6 ± 0.6 at 30 min and 5.5 ± 0.6 at 60 min. The exposure to AcSW significantly decreased MMP value, which was 5.7 ± 0.6 and 3.9 ± 0.6 after 30 and 60 min of exposure, respectively (P ≤ 0.05). Furthermore, a significant difference was found between these two exposure times (P ≤ 0.05) (Figure 2B).
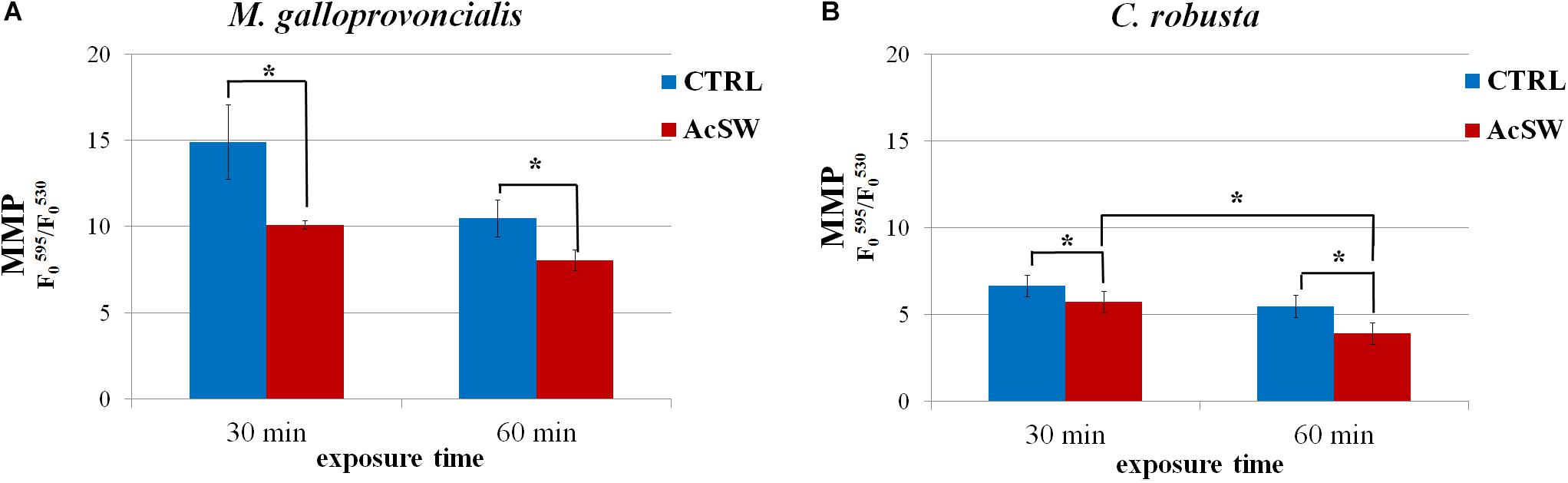
Figure 2. Effects of acidified seawater (AcSW) on mitochondrial membrane potential (MMP) in M. galloprovincialis (A) and C. robusta (B) spermatozoa (mean ± SE). MMP value was calculated as red (∼595 nm) to green (∼535 nm) fluorescence emission peak ratio in spermatozoa exposed for 30 and 60 min to filtered natural seawater with a natural pH value of 8.1 (CTRL) and to AcSW with a pH value of 7.8. ∗indicates statistically significant differences (P ≤ 0.05).
Intracellular Reactive Oxygen Species (ROS)
Intracellular ROS levels were not significantly affected by AcSW exposure in both species. In M. galloprovincialis, the H2O2 levels in the control spermatozoa were 88.1 ± 6.9 at 30 min and 81.7 ± 5.7 at 60 min. They did not significantly change after sperm exposure to AcSW (84.0 ± 6.3 and 77.7 ± 5.2 after 30 and 60 min exposure, respectively) (Figure 3A). Similarly, the intracellular content of O2– in control spermatozoa was 14.6 ± 1.7 and 15.7 ± 3.0 at 30 and 60 min exposure, respectively, and it was not significantly different from those recorded in sperm exposed to AcSW (13.1 ± 1.6 and 13.2 ± 1.4 at 30 and 60 min, respectively) (Figure 3C).
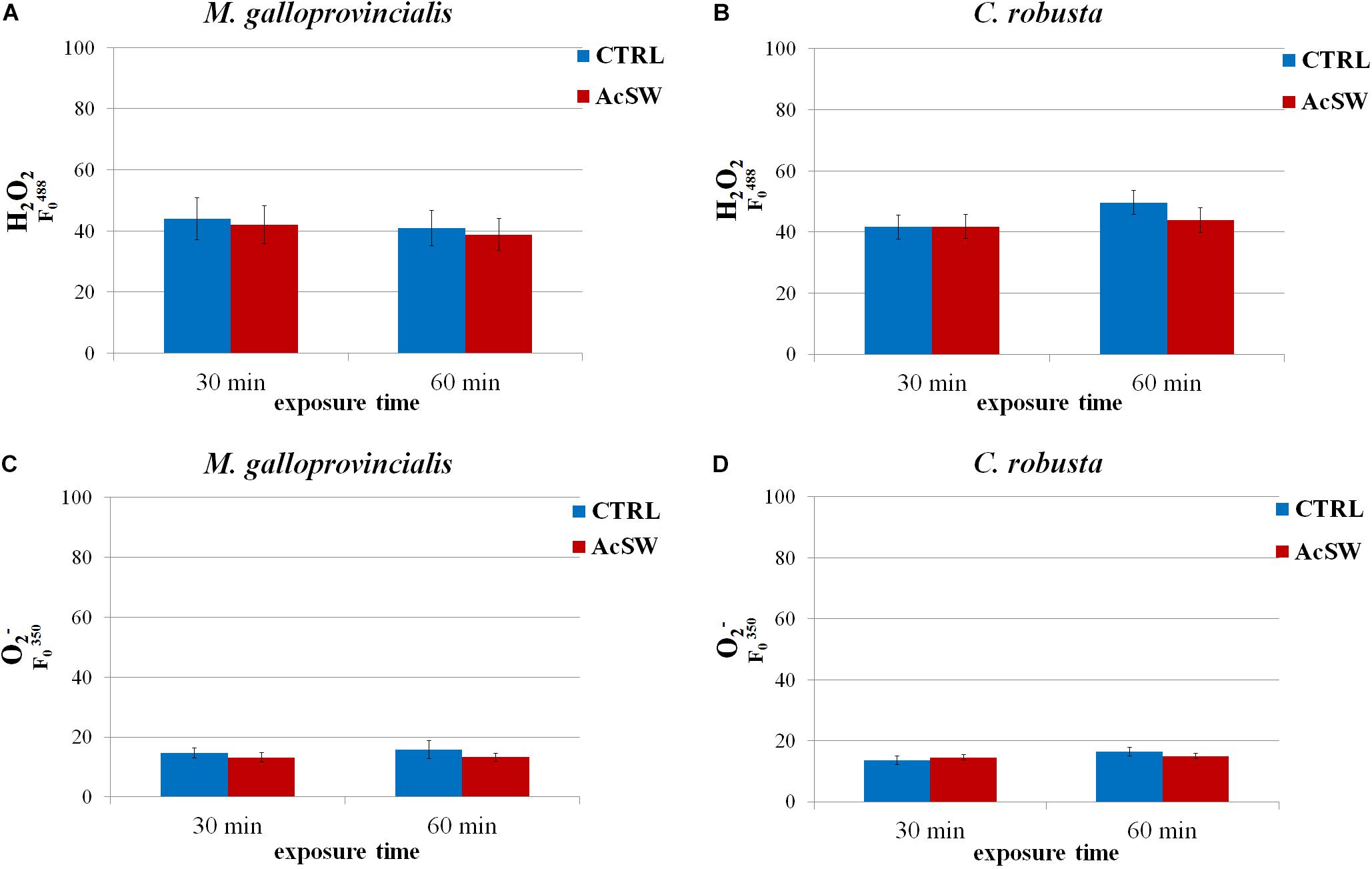
Figure 3. Effects of acidified seawater (AcSW) on intracellular levels of reactive oxygen species in spermatozoa of M. galloprovincialis and C. robusta. After exposure for 30 and 60 min to filtered natural seawater with a pH value of 8.1 (CTRL) and to AcSW with a pH value of 7.8, intracellular level of hydrogen peroxide (H2O2) (A,C) were measured at emission wavelength of ∼530 nm using 488 nm excitation wavelength and superoxide anion (O2–) (B,D) recording fluorescent emission spectra from 600 to 650 nm after excitation at ∼350 nm wavelength.
In C. robusta, following the exposure to AcSW for 30 and 60 min, no significant differences in H2O2 and O2– intracellular levels were observed in comparison to control spermatozoa (H2O2: 41.6 ± 3.9 vs. 41.7 ± 3.9 and 49.6 ± 3.9 vs. 43.8 ± 3.9 after 30 min and 60 min exposure, respectively; O2–:14.5 ± 0.8 vs. 13.6 ± 0.8 at 30 min and 16.4 ± 0.8 vs. 14.9 ± 0.8 at 60 min) (Figures 3B,D).
Lipid Peroxidation
Lipid peroxidation in spermatozoa of both species was not significantly affected by the AcSW exposure. M. galloprovincialis control spermatozoa showed a value of lipid peroxidation of 41.0 ± 1.6 after 30 min and 42.0 ± 3.1 after 60 min exposure. Following exposure to AcSW for the two exposure time groups, these values did not significantly change (44.9 ± 2.3 at 30 min and 48.5 ± 2.0 at 60 min) (Figure 4A). In C. robusta spermatozoa, plasma membrane lipid peroxidation was 58.3 ± 1.9 and 54.4 ± 1.9 at 30 and 60 min, respectively. No significant differences were observed in spermatozoa exposed to AcSW at the both exposure time (54.7 ± 1.9 at 30 min and 52.1 ± 1.9 at 60 min) (Figure 4B).
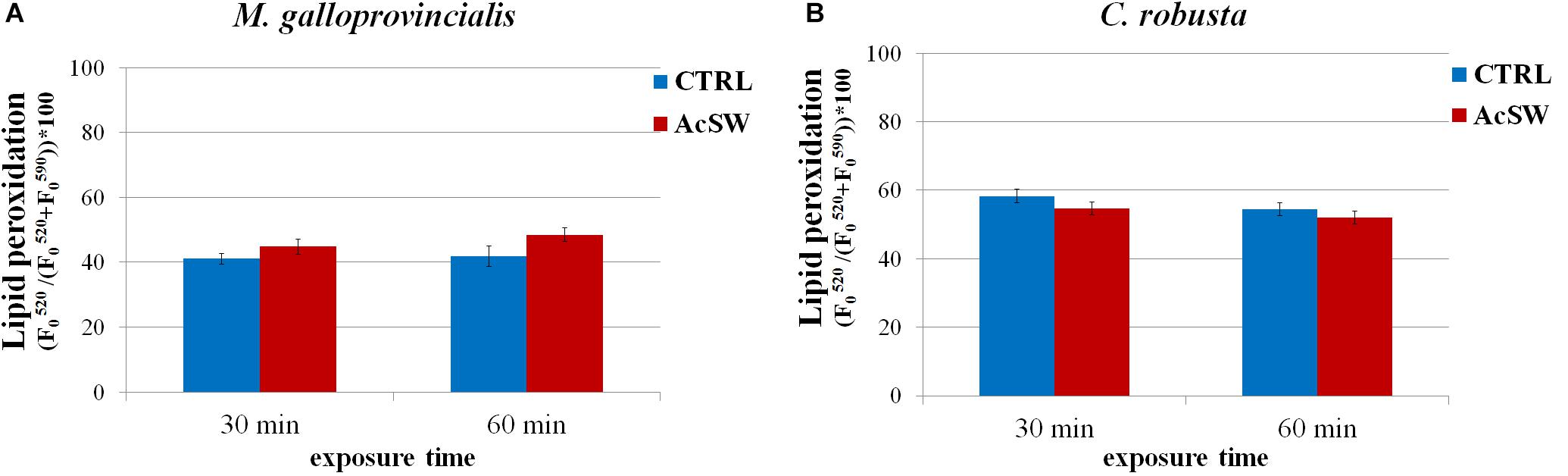
Figure 4. Effects of acidified seawater (AcSW) on plasma membrane lipid peroxidation in spermatozoa of M. galloprovincialis (A) and C. robusta (B) (mean ± SE). Lipid peroxidation was determined as a ratio between fluorescence emission peak value at ∼520 nm and the sum of fluorescence emission peak values at ∼520 and ∼590 nm after exposure for 30 and 60 min to filtered natural seawater with a natural pH of 8.1 (CTRL) and to AcSW with a pH of 7.8.
Intracellular pH (pHi)
The pHi recorded in M. galloprovincialis control spermatozoa at both exposure times was 8.144 ± 0.002; and it significantly decreased after 30 and 60 min exposure to AcSW (7.842 ± 0.007 and 7.92 ± 0.01; respectively) (Figure 5A).
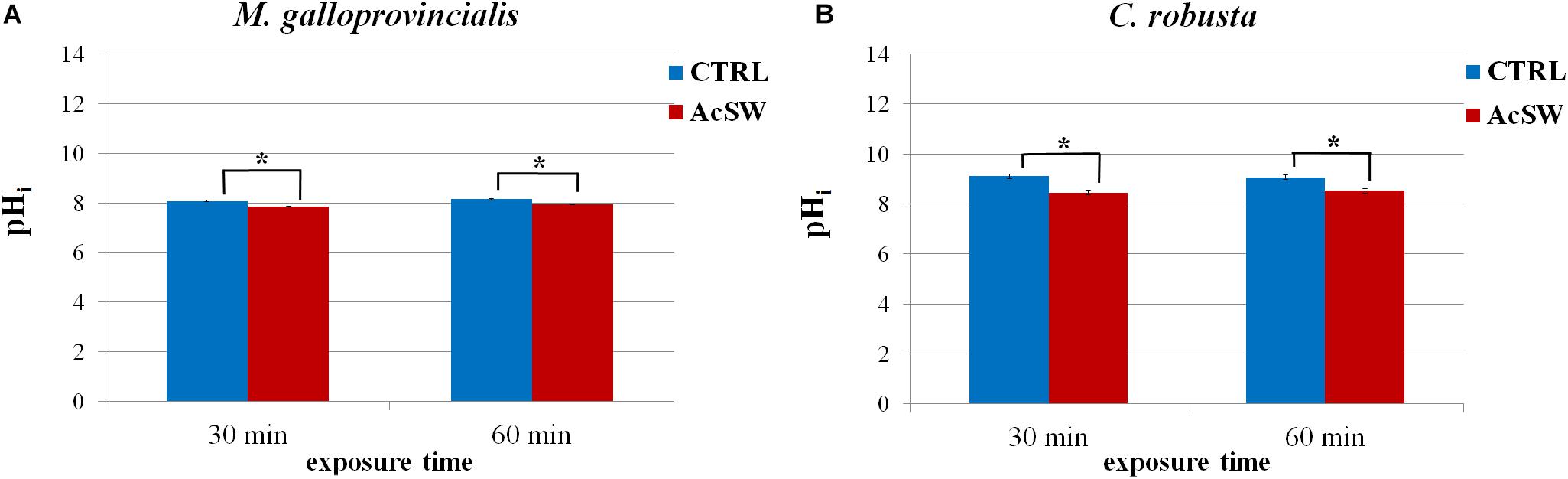
Figure 5. Effect of acidified seawater (AcSW) on intracellular pH of M. galloprovincialis (A) and C. robusta (B) spermatozoa (mean ± SE). pHi was evaluated by using the probe BCECF-AM after sperm exposure for 30 and 60 min to filtered natural seawater with a pH value of 8.1 (CTRL) and to AcSW with a pH value of 7.8. ∗ indicates significant (P ≤ 0.05) differences.
Similarly, in C. robusta, control spermatozoa showed a pHi value of 9.1 ± 0.1, which significantly dropped to 8.4 ± 0.1 and 8.5 ± 0.1 after 30 and 60 min exposure to AcSW, respectively (Figure 5B).
Sperm Viability
In M. galloprovincialis, sperm viability in the control group was 83.1 ± 1.8% and 84.1 ± 2.1% at 30 and 60 min exposure time, respectively. Following exposure to AcSW, no significant differences were recorded (79.6 ± 1.7% and 81.9 ± 2.2% at 30 and 60 min of exposure) in comparison to the control group (Figure 6A).
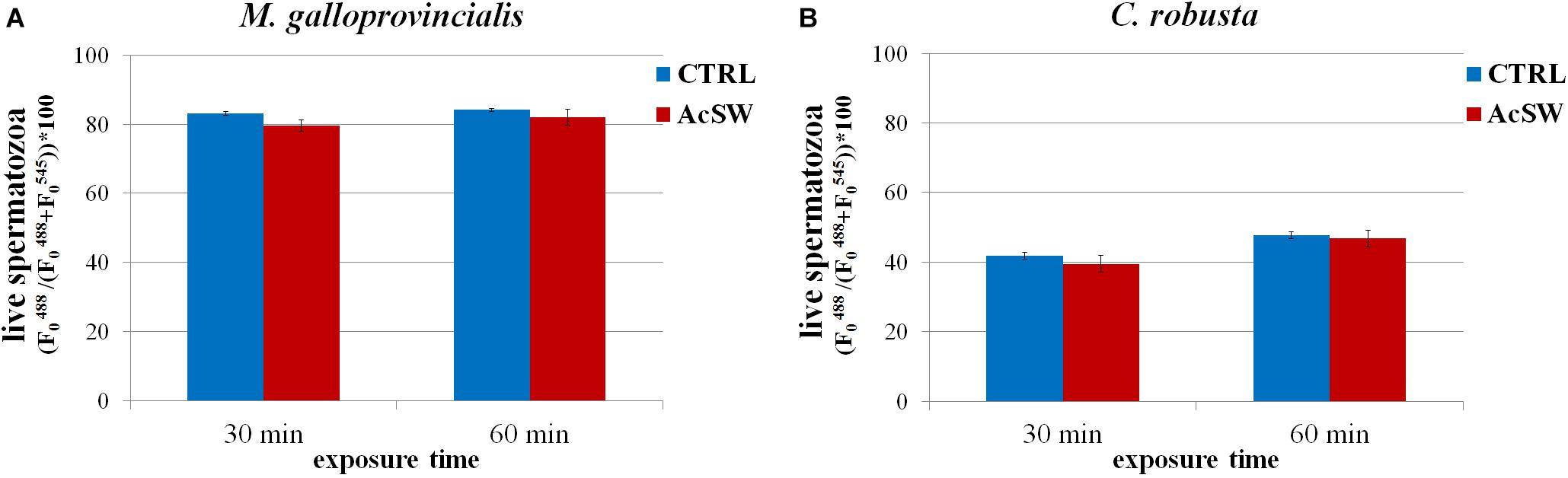
Figure 6. Effects of acidified seawater (AcSW) on sperm viability in M. galloprovincialis (A) and C. robusta (B) (mean ± SE). Viability was determined after exposure for 30 and 60 min to filtered natural seawater with a natural pH value of 8.1 (CTRL) and to AcSW with a pH vale of 7.8.
Similarly, in C. robusta, sperm viability did not significantly change after exposure to AcSW for 30 and 60 min (41.8% ± 2.4 vs. 39.5% ± 2.4 at 30 min and 47.7% ± 2.4 vs. 46.8% ± 2.4 at 60 min) (Figure 6B).
Discussion
Gamete quality is a key factor in reproductive success and its impairment may affect offspring quality with important implications for the fitness and the survival of marine animals.
In the present study, we evaluated the effects of exposure of M. galloprovincialis and C. robusta spermatozoa to AcSW at a pH value of 7.8 predicted for the surface oceans for the year 2100.
Like most marine invertebrates, these species release their gametes into the water column where fertilization occurs and new individuals develop (Evans and Sherman, 2013). The pH decrease of seawater where gametes are released therefore may affect gamete quality parameters strictly related to fertilization success.
Sperm quality is crucial to predict male reproductive success and depends on several factors; among these, environmental perturbations are considered key aspects (Gallo and Tosti, 2016). Recent literature reports different effects of OA on spermatozoa, many of these focused on motility (Levitan, 2000; Kupriyanova and Havenhand, 2005; Havenhand and Schlegel, 2009; Schlegel et al., 2014, 2015; Gallo et al., 2019). This is considered a reliable predictor for sperm quality (Au et al., 2002; Liu et al., 2011) since a positive relationship between this parameter and fertilization success has been demonstrated in many marine invertebrates (Levitan, 2000; Kupriyanova and Havenhand, 2005).
The impact of OA exposure on sperm motility was assessed in different marine species with contrasting results. In the sea urchin Psammechinus miliaris, sperm exposure to AcSW caused an enhancement of motility, whereas no effects were observed in the oyster Crassostrea gigas and in marine fish (Havenhand and Schlegel, 2009; Frommel et al., 2010). On the contrary, a decrease of the percentage of motile spermatozoa after exposure to acidified conditions was observed in many different marine species such as the sea urchin Heliocidaris erythrogramma (Havenhand et al., 2008), the polychaete Galeolaria caespitosa (Schlegel et al., 2014), the coral Acropora digitifera and the sea cucumber Holothuria spp. (Morita et al., 2010). In agreement with these studies, here we observed a reduction of the percentage of motile spermatozoa in C. robusta and M. galloprovincialis following exposure to AcSW.
Sperm motility is well documented to be correlated to pHi (Christen et al., 1982, 1983; Sendai and Aketa, 1991; Nakajima, 2005). In marine species, spermatozoa are stored in the gonads in a quiescent state, but following the release into seawater, they become activated, initiating both respiration and motility. Activation of spermatozoa is regulated by ionic composition of the seawater into which they are released (Christen et al., 1983; Tosti, 1994). Quiescent spermatozoa have an acid pHi that inhibits different enzymes, in particular the dynein ATPase responsible for most of the ATP hydrolysis and respiratory chain enzymes (Christen et al., 1983). Upon release into seawater, an influx of external sodium ions occurring into the spermatozoa triggers the release of intracellular H+ ions causing an increase of pHi. The pHi increase activates the dynein ATPase, which is a cytoskeletal motor protein, and enzymes of mitochondrial respiration that provides the energy for motility (Shapiro and Tombes, 1985). Here, we observed that the exposure to AcSW of C. robusta and M. galloprovincialis spermatozoa induced a decrease of pHi. We could suppose that CO2-AcSW alters the acid-base balance of the spermatozoa causing a significant reduction of pHi and, consequently, the inhibition of the flagellar motor driving motility. Furthermore, we demonstrated that OA negatively affected mitochondrial activity assessed by evaluating changes in MMP. In sea urchin spermatozoa, a positive correlation between MMP and motility after OA exposure was previously reported (Schlegel et al., 2015). Sperm energy metabolism is driven by mitochondrial activity (Gibbons, 1972; Mita and Nakamura, 1998), which is the principle source of energy required for motility. Therefore, the decrease of sperm motility under acidified conditions is likely due to reduced mitochondrial activity. The initiation of sperm motility at spawning is associated to an increase of mitochondrial activity resulting in supplement of the energy for motility (Dale, 2018). In the present study, the increase of MMP in spermatozoa exposed to FNSW with a pH value of 8.1 compared to those exposed to AcSW is clear evidence of sperm motility initiation.
On the other hand, despite a positive correlation between ROS production and MMP has been well documented (Suski et al., 2012), no change of intracellular levels of two different ROS species nor lipid peroxidation were here observed. These may be due to a rapid and efficient neutralization of ROS by antioxidants to preserve a cellular redox balance and to prevent potential damages that they may exert altering sperm function. This is also supported by a previous report demonstrating that in M. galloprovincialis seawater acidification exposure induced an increase of antioxidant defenses and, consequently, this species results more resistant to reduced seawater pH (Munari et al., 2018).
Altogether, the obtained results indicate that spermatozoa of the free spawning invertebrates released into seawater at a pH value of 7.8, as predicted to occur at the end of this century, are maintained in a repressed metabolic state as occurs in the testis. This hypothesis is also supported by the lack of effects of AcSW on sperm vitality here observed. The initiation of sperm motility occurring after spawning is a prerequisite process for the accomplishment of fertilization, and, in the free spawning invertebrates, the relationship between sperm motility and fertilization success is well described (Styan, 1998). In conclusion, future changes in ocean acidity through sperm motility impairment will potentially pose serious consequences for fertilization success and in turn for the survival of many marine species. Given the intrinsic importance of reproduction for the conservation of marine biodiversity and ecosystem functionality, it is clear the urgent need for further studies into the potential impacts of near-future levels of OA.
Data Availability Statement
The datasets generated for this study are available on request to the corresponding author.
Author Contributions
AG, RB, and ET designed the methodology. ME, RB, AC, and AG performed the experiments. AG and RB collected and analyzed the data. ET supervised the project. ME and AG wrote the manuscript with input from all authors that gave final approval for publication.
Funding
This study was supported by the Stazione Zoologica Anton Dohrn institutional funding. AG has been supported by a Stazione Zoologica Anton Dohrn post-doc fellowship.
Conflict of Interest
The authors declare that the research was conducted in the absence of any commercial or financial relationships that could be construed as a potential conflict of interest.
Acknowledgments
We wish to acknowledge Dr. Nuria Teixidò and Alice Mirasole for total alkalinity determination, Mr. A. Macina for technical support in animal maintenance, and Mr. V. Monfrecola for laboratory assistance.
Supplementary Material
The Supplementary Material for this article can be found online at: https://www.frontiersin.org/articles/10.3389/fmars.2019.00794/full#supplementary-material
References
Au, D. W., Chiang, M. W., Tang, J. Y., Yuen, B. B., Wang, Y. L., and Wu, R. S. (2002). Impairment of sea urchin sperm quality by UV-B radiation: predicting fertilization success from sperm motility. Mar. Pollut. Bull. 44, 583–589. doi: 10.1016/s0025-326x(01)00288-0
Bobe, J., and Labbé, C. (2010). Egg and sperm quality in fish. Gen. Comp. Endocr. 165, 535–548. doi: 10.1016/j.ygcen.2009.02.011
Bolton, T. F., and Havenhand, J. N. (1996). Chemical mediation of sperm activity and longevity in the solitary ascidians Ciona intestinalis and Ascidiella aspersa. Biol. Bull. 190, 329–335. doi: 10.2307/1543025
Boni, R., Gallo, A., Montanino, M., Macina, A., and Tosti, E. (2016). Dynamic changes in the sperm quality of Mytilus galloprovincialis under continuous thermal stress. Mol. Reprod. Dev. 83, 162–173. doi: 10.1002/mrd.22604
Brennand, H. S., Soars, N., Dworjanyn, S. A., Davis, A. R., and Byrne, M. (2010). Impact of ocean warming and ocean acidification on larval development and calcification in the sea urchin Tripneustes gratilla. PLoS One 5:e11372. doi: 10.1371/journal.pone.0011372
Byrne, M., Ho, M., Selvakumaraswamy, P., Nguyen, H. D., Dworjanyn, S. A., and Davis, A. R. (2009). Temperature, but not pH, compromises sea urchin fertilization and early development under near-future climate change scenarios. Proc. R. Soc. Lond. 276, 1883–1888. doi: 10.1098/rspb.2008.1935
Byrne, M., Oakes, D. J., Pollak, J. K., and Laginestra, E. (2008). Toxicity of landfill leachate to sea urchin development with a focus on ammonia. Cell Biol. Toxicol. 24, 503–512. doi: 10.1007/s10565-008-9099-1
Byrne, M., Soars, N., Selvakumaraswamy, P., Dworjanyn, S. A., and Davis, A. R. (2010). Sea urchin fertilization in a warm, acidified and high pCO2 ocean across a range of sperm densities. Mar. Environ. Res. 69, 234–239. doi: 10.1016/j.marenvres.2009.10.014
Cabrita, E., Martínez-Páramo, S., Gavaia, P. J., Riesco, M. F., Valcarce, D., Sarasquete, C., et al. (2014). Factors enhancing fish sperm quality and emerging tools for sperm analysis. Aquaculture 432, 389–401. doi: 10.1016/j.aquaculture.2014.04.034
Carr, R. S., Biedenbach, J. M., and Nipper, M. (2006). Influence of potentially confounding factors on sea urchin porewater toxicity tests. Arch. Environ. Con. Tox. 51, 573–579. doi: 10.1007/s00244-006-0009-3
Christen, R., Schackmann, R., and Shapiro, B. (1983). Metabolism of sea urchin sperm. Interrelationships between intracellular pH, ATPase activity, and mitochondrial respiration. J. Biol. Chem. 258, 5392–5399.
Christen, R., Schackmann, R. W., and Shapiro, B. (1982). Elevation of the intracellular pH activates respiration and motility of sperm of the sea urchin, Strongylocentrotus purpuratus. J. Biol. Chem. 257, 14881–14890.
Cottet-Rousselle, C., Ronot, X., Leverve, X., and Mayol, J. F. (2011). Cytometric assessment of mitochondria using fluorescent probes. Cytometry A 79, 405–425. doi: 10.1002/cyto.a.21061
Díaz-Castañeda, V., Cox, T. E., Gazeau, F., Fitzer, S., Delille, J., Alliouane, S., et al. (2019). Ocean acidification affects calcareous tube growth in adults and reared offspring of serpulid polychaetes. J. Exp. Biol. 222:jeb196543. doi: 10.1242/jeb.196543
Dickson, A. (1990). Standard potential of the reaction AgCl (s)? 1/2H2 (g) = Ag (s). HCl (aq), and the standard acidity constant of the ion HSO4-in synthetic seawater from 273.15 to 318.15K. J. Chem. Thermodyn. 22, 113–127. doi: 10.1016/0021-9614(90)90074-z
Dickson, A., and Millero, F. J. (1987). A comparison of the equilibrium constants for the dissociation of carbonic acid in seawater media. Deep Sea Res. Part A Oceanogr. Res. Pap. 34, 1733–1743. doi: 10.1016/0198-0149(87)90021-5
Di Matteo, O., Langellotti, A. L., Masullo, P., and Sansone, G. (2009). Cryopreservation of the Mediterranean mussel (Mytilus galloprovincialis) spermatozoa. Cryobiology 58, 145–150. doi: 10.1016/j.cryobiol.2008.09.015
Doney, S. C., Fabry, V. J., Feely, R. A., and Kleypas, J. A. (2009). Ocean acidification: the other CO2 problem. Annu. Rev. Mar. Sci. 1, 169–192.
Evans, J. P., and Sherman, C. D. (2013). Sexual selection and the evolution of egg-sperm interactions in broadcast-spawning invertebrates. Biol. Bull. 224, 166–183. doi: 10.1086/bblv224n3p166
Feely, R. A., Sabine, C. L., Hernandez-Ayon, J. M., Ianson, D., and Hales, B. (2008). Evidence for upwelling of corrosive “Acidified” water onto the continental shelf. Science 320, 1490–1492. doi: 10.1126/science.1155676
Frommel, A. Y., Stiebens, V., Clemmesen, C., and Havenhand, J. (2010). Effect of ocean acidification on marine fish sperm (Baltic cod: Gadus morhua). Biogeosciences 7, 3915–3919. doi: 10.5194/bg-7-3915-2010
Gallo, A. (2018). Toxicity of marine pollutants on the ascidian oocyte physiology: an electrophysiological approach. Zygote 26, 14–23. doi: 10.1017/S0967199417000612
Gallo, A., Boni, R., Buia, M. C., Monfrecola, V., Esposito, M. C., and Tosti, E. (2019). Ocean acidification impact on ascidian Ciona robusta spermatozoa: new evidence for stress resilience. Sci. Total Environ. 697:134100. doi: 10.1016/j.scitotenv.2019.134100
Gallo, A., Boni, R., Buttino, I., and Tosti, E. (2016). Spermiotoxicity of nickel nanoparticles in the marine invertebrate Ciona intestinalis (ascidians). Nanotoxicology 10, 1096–1104. doi: 10.1080/17435390.2016.1177743
Gallo, A., Boni, R., and Tosti, E. (2018a). Sperm viability assessment in marine invertebrates by fluorescent staining and spectrofluorimetry: a promising tool for assessing marine pollution impact. Ecotox. Environ. Saf. 147, 407–412. doi: 10.1016/j.ecoenv.2017.07.069
Gallo, A., Manfra, L., Boni, R., Rotini, A., Migliore, L., and Tosti, E. (2018b). Cytotoxicity and genotoxicity of CuO nanoparticles in sea urchin spermatozoa through oxidative stress. Environ. Int. 118, 325–333. doi: 10.1016/j.envint.2018.05.034
Gallo, A., Menezo, Y., Dale, B., Coppola, G., Dattilo, M., Tosti, E., et al. (2018c). Metabolic enhancers supporting 1-carbon cycle affect sperm functionality: an in vitro comparative study. Sci. Rep. 8:11769. doi: 10.1038/s41598-018-30066-9
Gallo, A., Silvestre, F., Cuomo, A., Papoff, F., and Tosti, E. (2011). The impact of metals on the reproductive mechanisms of the ascidian Ciona intestinalis. Mar. Ecol. 32, 222–231. doi: 10.3390/md11093554
Gallo, A., and Tosti, E. (2013). Adverse effect of antifouling compounds on the reproductive mechanisms of the ascidian Ciona intestinalis. Mar. Drugs 11, 3554–3568. doi: 10.3390/md11093554
Gallo, A., and Tosti, E. (2015). Reprotoxicity of the antifoulant chlorothalonil in ascidians: an ecological risk assessment. PLoS One 10:e0123074. doi: 10.1371/journal.pone.0123074
Gallo, A., and Tosti, E. (2016). New markers for the assessment of sperm quality. JFIV Reprod. Med. Genet. 4:e125.
Gallo, A., and Tosti, E. (2019). Effects of ecosystem stress on reproduction and development. Mol. Reprod. Dev. 86, 1269–1272. doi: 10.1002/mrd.23169
Gazeau, F., Alliouane, S., Bock, C., Bramanti, L., Lopez Correa, M., Gentile, M., et al. (2014). Impact of ocean acidification and warming on the Mediterranean mussel (Mytilus galloprovincialis). Front. Mar. Sci. 1:62. doi: 10.3389/fmars.2014.00062
Gibbons, B. H. (1972). Flagellar movement and adenosine triphosphatase activity in sea urchin sperm extracted with Triton X-100. ıJ. Cell. Biol. 54, 75–97. doi: 10.1083/jcb.54.1.75
Guo, X., Huang, M., Pu, F., You, W., and Ke, C. (2015). Effects of ocean acidification caused by rising CO2 on the early development of three mollusks. Aquat. Biol. 23, 147–157. doi: 10.3354/ab00615
Havenhand, J. N., Buttler, F., Thorndyke, M. C., and Williamson, J. E. (2008). Near-future levels of ocean acidification reduce fertilization success in a sea urchin. Curr. Biol. 18, R651–R652.
Havenhand, J. N., and Schlegel, P. (2009). Near-future levels of ocean acidification do not affect sperm motility and fertilization kinetics in the oyster Crassostrea gigas. Biogeosciences 6, 3009–3015. doi: 10.5194/bg-6-3009-2009
Kong, H., Jiang, X., Clements, J. C., Wang, T., Huang, X., Shang, Y., et al. (2019). Transgenerational effects of short-term exposure to acidification and hypoxia on early developmental traits of the mussel Mytilus edulis. Mar. Environ. Res. 145, 73–80. doi: 10.1016/j.marenvres.2019.02.011
Kroeker, K. J., Kordas, R. L., Crim, R. N., and Singh, G. G. (2010). Meta-analysis reveals negative yet variable effects of ocean acidification on marine organisms. Ecol. Lett. 13, 1419–1434. doi: 10.1111/j.1461-0248.2010.01518.x
Kupriyanova, E. K., and Havenhand, J. N. (2005). Effects of temperature on sperm swimming behaviour, respiration and fertilization success in the serpulid polychaete, Galeolaria caespitosa (Annelida: Serpulidae). Invertebr. Reprod. Dev. 48, 7–17. doi: 10.1080/07924259.2005.9652166
Kurihara, H., Kato, S., and Ishimatsu, A. (2007). Effects of increased seawater pCO2 on early development of the oyster Crassostrea gigas. Aquat. Biol. 1, 91–98. doi: 10.3354/ab00009
Kurihara, H., and Shirayama, Y. (2004). Effects of increased atmospheric CO2 on sea urchin early development. Mar. Ecol. Progr. Ser. 274, 161–169. doi: 10.3354/meps274161
Lenz, B., Fogarty, N. D., and Figueiredo, J. (2019). Effects of ocean warming and acidification on fertilization success and early larval development in the green sea urchin Lytechinus variegatus. Mar. Poll. Bull. 141, 70–78. doi: 10.1016/j.marpolbul.2019.02.018
Levitan, D. R. (2000). Sperm velocity and longevity trade off each other and influence fertilization in the sea urchin Lytechinus variegatus. Proc. R. Soc. Lond. 267, 531–534. doi: 10.1098/rspb.2000.1032
Liu, G., Innes, D., and Thompson, R. J. (2011). Quantitative analysis of sperm plane circular movement in the blue mussels Mytilus edulis, M. trossulus and their hybrids. J. Exp. Zool. A Ecol. Genet. Physiol. 315A, 280–290. doi: 10.1002/jez.674
Meehl, G. A., Stocker, T. F., Collins, W. D., Friedlingstein, P., Gaye, A. T., Gregory, J. M., et al. (2007). “Global climate projections. in climate change 2007: the physical science basis,” in Proceedings of the Contribution of Working Group I to the Fourth Assessment Report of the Intergovernmental Panel on Climate Change, eds S. Solomon, D. Qin, M. Manning, Z. Chen, M. Marquis, K. B. Averyt, et al. (Cambridge: Cambridge University Press).
Mehrbach, C., Culberson, C., Hawley, J., and Pytkowicx, R. (1973). Measurement of the apparent dissociation constants of carbonic acid in seawater at atmospheric pressure 1. Limnol. Oceanogr. 18, 897–907. doi: 10.4319/lo.1973.18.6.0897
Mita, M., and Nakamura, M. (1998). Energy metabolism of sea urchin spermatozoa: an approach based on echinoid phylogeny. Zool. Sci. 15, 1–10. doi: 10.2108/zsj.15.1
Morita, M., Suwa, R., Iguchi, A., Nakamura, M., Shimada, K., Sakai, K., et al. (2010). Ocean acidification reduces sperm flagellar motility in broadcast spawning reef invertebrates. Zygote 18, 103–107. doi: 10.1017/S0967199409990177
Munari, M., Matozzo, V., Gagné, F., Chemello, G., Riedl, V., Finos, L., et al. (2018). Does exposure to reduced pH and diclofenac induce oxidative stress in marine bivalves? A comparative study with the mussel Mytilus galloprovincialis and the clam Ruditapes philippinarum. Environ. Pollut. 240, 925–937. doi: 10.1016/j.envpol.2018.05.005
Nakajima, M. (2005). Reconstitution of circadian oscillation of cyanobacterial KaiC phosphorylation in vitro. Science 308, 414–415. doi: 10.1126/science.1108451
Orr, J. C., Fabry, V. J., Aumont, O., Bopp, L., Doney, S. C., Feely, R. A., et al. (2005). Anthropogenic ocean acidification over the twenty-first century and its impact on calcifying organisms. Nature 437, 681–686. doi: 10.1038/nature04095
Pachauri, R. K., Allen, M. R., Barros, V. R., Broome, J., Cramer, W., Christ, R., et al. (2014). “Climate change 2014:synthesis report,” in Proceedings of the Contribution of Working Groups I, II and III to the Fifth Assessment Report of the Intergovernmental Panel on Climate Change, eds R. Pachauri and L. Meyer, (Geneva: IPCC).
Parker, L., Ross, P., O’Connor, W., Pörtner, H., Scanes, E., and Wright, J. (2013). Predicting the response of molluscs to the impact of ocean acidification. Biology 2, 651–692. doi: 10.3390/biology2020651
Parker, L. M., Ross, P. M., and O’Connor, W. A. (2009). The effect of ocean acidification and temperature on the fertilization and embryonic development of the Sydney rock oyster Saccostrea glomerata (Gould 1850). Glob. Change Biol. 15, 2123–2136. doi: 10.1111/j.1365-2486.2009.01895.x
Pierrot, D., Lewis, E., and Wallace, D. (2006). MS Excel Program Developed for CO2 System Calculations. In: ORNL/CDIAC-105a. Oak Ridge, Tenn: Carbon Dioxide Information Analysis Center.
Robinson, K. M., Janes, M. S., Pehar, M., Monette, J. S., Ross, M. F., Hagen, T. M., et al. (2006). Selective fluorescent imaging of superoxide in vivo using ethidium-based probes. Proc. Natl. Acad. Sci. U.S.A. 103, 15038–15043. doi: 10.1073/pnas.0601945103
Rotini, A., Gallo, A., Parlapiano, I., Berducci, M., Boni, R., Tosti, E., et al. (2018). Insights into the CuO nanoparticle ecotoxicity with suitable marine model species. Ecotox. Environ. Saf. 147, 852–860. doi: 10.1016/j.ecoenv.2017.09.053
Schlegel, P., Binet, M. T., Havenhand, J. N., Doyle, C. J., and Williamson, J. E. (2015). Ocean acidification impacts on sperm mitochondrial membrane potential bring sperm swimming behaviour near its tipping point. J. Exp. Biol. 218, 1084–1090. doi: 10.1242/jeb.114900
Schlegel, P., Havenhand, J. N., Obadia, N., and Williamson, J. E. (2014). Sperm swimming in the polychaete Galeolaria caespitosa shows substantial inter-individual variability in response to future ocean acidification. Mar. Pollut. Bull. 78, 213–217. doi: 10.1016/j.marpolbul.2013.10.040
Sedano, F., Rodríguez, J., Ruiz, C., García-Martín, L., and Sánchez, J. (1995). Biochemical composition and fertilization in the eggs of Mytilus galloprovincialis (Lamarck). J. Exp. Mar. Biol. Ecol. 192, 75–85. doi: 10.1016/0022-0981(95)00062-v
Sendai, Y., and Aketa, K. (1991). Activation of Ca2+ transport system of sea urchin sperm by high external pH: 220 kD membrane glycoprotein is involved in the regulation of the Ca2+ Entry. (sea urchin sperm/external pH/intracellular pH/Ca2+ entry/acrosome reaction). Dev. Growth Diff. 33, 101–109. doi: 10.1111/j.1440-169x.1991.00101.x
Shapiro, B. M., and Tombes, R. M. (1985). A biochemical pathway for a cellular behaviour: pHi, phosphorylcreatine shuttles, and sperm motility. Bioessays 3, 100–103. doi: 10.1002/bies.950030303
Shenkar, N., and Swalla, B. J. (2011). Global diversity of Ascidiacea. PLoS One 6:e20657. doi: 10.1371/journal.pone.0020657
Shiba, K., and Inaba, K. (2014). Distinct roles of soluble and transmembrane adenylyl cyclases in the regulation of flagellar motility in Ciona sperm. Int. J. Mol. Sci. 15, 13192–13208. doi: 10.3390/ijms150813192
Styan, C. A. (1998). Polyspermy, egg size, and the fertilization kinetics of free-spawning marine invertebrates. Am. Nat. 152, 290–297. doi: 10.1086/286168
Suski, J. M., Lebiedzinska, M., Bonora, M., Pinton, P., Duszynski, J., and Wieckowski, M. R. (2012). Relation between mitochondrial membrane potential and ROS formation. Methods Mol. Biol. 810, 183–205. doi: 10.1007/978-1-61779-382-0_12
Tosti, E. (1994). Sperm activation in species with external fertilisation. Zygote 2, 359–361. doi: 10.1017/s0967199400002215
Keywords: ascidian, free spawning invertebrates, mussel, ocean acidification, sperm quality, motility
Citation: Esposito MC, Boni R, Cuccaro A, Tosti E and Gallo A (2020) Sperm Motility Impairment in Free Spawning Invertebrates Under Near-Future Level of Ocean Acidification: Uncovering the Mechanism. Front. Mar. Sci. 6:794. doi: 10.3389/fmars.2019.00794
Received: 05 August 2019; Accepted: 10 December 2019;
Published: 08 January 2020.
Edited by:
Peng Jin, Guangzhou University, ChinaReviewed by:
Hui Kong, Shanghai Ocean University, ChinaXinguo Zhao, Yellow Sea Fisheries Research Institute (CAFS), China
Copyright © 2020 Esposito, Boni, Cuccaro, Tosti and Gallo. This is an open-access article distributed under the terms of the Creative Commons Attribution License (CC BY). The use, distribution or reproduction in other forums is permitted, provided the original author(s) and the copyright owner(s) are credited and that the original publication in this journal is cited, in accordance with accepted academic practice. No use, distribution or reproduction is permitted which does not comply with these terms.
*Correspondence: Alessandra Gallo, alessandra.gallo@szn.it