- 1Kepley Biosystems Incorporated, Greensboro, NC, United States
- 2Department of Nanoengineering, Joint School of Nanoscience and Nanoengineering, North Carolina A&T State University, Greensboro, NC, United States
- 3Department of Anesthesiology, Critical Care, and Surgery, Duke University School of Medicine, Durham, NC, United States
- 4Ontario Veterinary College, University of Guelph, Guelph, ON, Canada
- 5LADUCA Regulatory, Quality, Compliance and Scientific Advisors LLC, East Brunswick, NJ, United States
- 6Department of Nanoscience, Joint School of Nanoscience and Nanoengineering, University of North Carolina at Greensboro, Greensboro, NC, United States
Horseshoe crab (HSC) hemolymph is the source of Limulus amebocyte lysate (LAL), a critical component in sterility testing that ensures drug and medical device safety for millions of patients every year. Wild HSC populations have been declining as a result of its use as whelk and eel bait, environmental changes, and its capture and bleeding for hemolymph by the biomedical industry, thus posing significant risks to species viability and the LAL raw material supply chain. We designed a controlled aquaculture habitat to husband HSCs and evaluated the effects of captivity on health markers (e.g., amebocyte density, hemocyanin levels, and LAL activity). We found HSC aquaculture to be practicable, with routine hemolymph harvesting resulting in high LAL quality, while safeguarding animal well-being with 100% HSC survival. Further, low-impact hemolymph harvesting via an indwelling catheter revealed rapid amebocyte rebound kinetics after consecutive 10% hemolymph extractions. Sustainable supplies of LAL could also be adapted to address daunting trends in septicemia and antimicrobial resistance. LAL is uniquely sensitive and specific for gram-negative bacteria, which represent 70–80% of pathogens that typically lead to sepsis. However, erratic results associated with interfering substances plagued efforts to adapt LAL for clinical use in the past. We report the development of a new LAL-based assay that can detect gram-negative bacteria and endotoxins in human blood without interference using aquaculture-derived LAL. Based on this research, sustainable LAL production from aquaculture could potentially satisfy industry needs with a fraction of one year’s current capture via year-round harvesting from a finite cohort of HSCs and expand raw materials supplies for potential future clinical applications.
Introduction
In 1983, the Limulus amebocyte lysate (LAL) assay was approved by the Food and Drug Administration (FDA) for pharmaceutical quality control testing and became the bacterial endotoxin test of choice for detection of gram-negative pathogens due to its simplicity, specificity, and sensitivity (Hochstein, 1990; Cooper, 2001). Early discoveries revealed instantaneous, rigid gel clot formation upon LAL exposure to lipopolysaccharides (LPS) at parts per trillion (Roslansky and Novitsky, 1991). The clotting cascade and LPS gel detection method are shown in Figure 1. As a highly toxic, heat-stable molecule, LPS comprises approximately 70% of the outer membranes of gram-negative bacteria. The unique sensitivity (0.05–50.0 EU/ml) and specificity of LAL characterize its potential for detecting LPS in human blood with important clinical indications as the most sensitive and rapid method for endotoxin detection (Food and Drug Administration [FDA], 1987; Hochstein, 1990) with specificity for up to 80% of pathogens that typically lead to sepsis (MacVane, 2017).
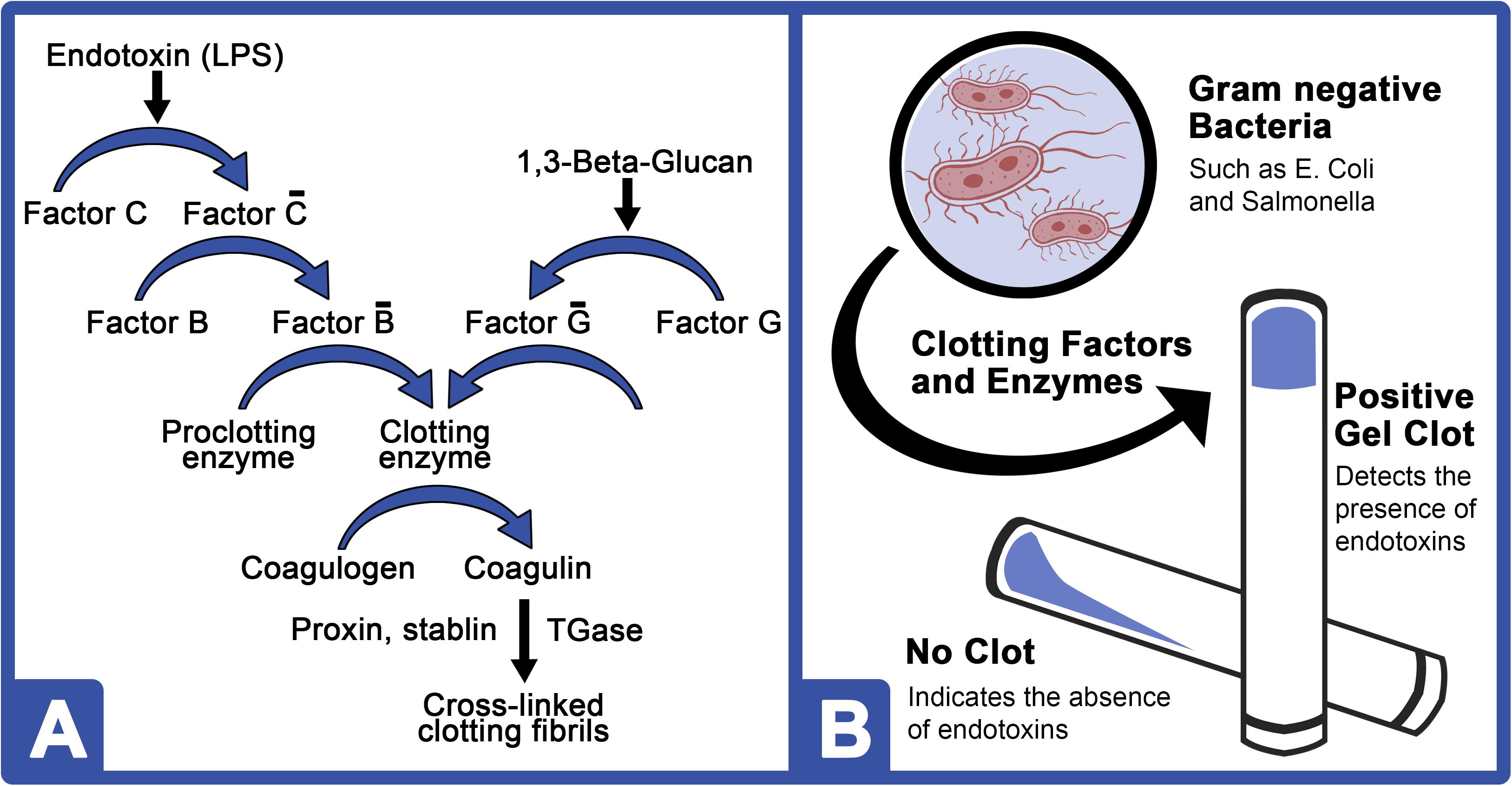
Figure 1. LPS and 1,3-β-D-glucan clotting cascades of the HSC amebocyte lysate. (A) Bacterial endotoxins (LPS) activate Factor C (C-bar), which then activates Factor B (B-bar). Activated Factor B converts the proclotting enzyme to a clotting enzyme and cleaves two coagulogen peptide bonds to form an insoluble coagulin gel. A similar pathway is shown for 1,3-β-D-glucan, which comprises the cell wall of most invasive fungal pathogens. (B) Schematic of a typical LPS-mediated LAL gel clot assay.
From a clinical perspective, a lack of tools for the early detection of blood-borne endotoxins, including LPS, often leads to inappropriate antibiotic administration (Baker et al., 2018). Antibiotics accelerate LPS shedding from the outer membrane of gram-negative bacteria and release inflammatory mediators (Jean-Baptiste, 2007). Early detection and timely, informed therapy could thus help decrease LPS levels during sepsis, potentially minimizing its toxic effects and improving patient survival rates (Berg and Gerlach, 2018); whereby, they increase by 7.6% per hour with appropriate antibiotic administration (Kumar et al., 2006). However, conventional laboratory methods for detecting bacteria in human blood have not advanced substantially since the advent of culture, which often requires 2–3 days for test results (Samuel, 2018).
Basal LPS levels are subject to significant variation due to an array of bacterial species and the preparation method used. Nonetheless, they are consistently higher in septic patients (0.96–3.45 EU/ml and 300 pg/ml) when compared to healthy subjects with ranges from 0.04 to 0.36 EU/ml and 5.1 pg/ml (Fleishman and Fowlkes, 1982; Casey et al., 1993; Bates et al., 1998; Ketchum et al., 1997; Strutz et al., 1999; Hurley et al., 2015). For FDA testing protocols, the acceptable safe (non-pyrogenic) endotoxin limit for injected and inhaled pharmaceutical products is ∼0.25–0.50 EU/ml (Williams, 2007; Hynes, 2016), which falls within the expected range of clinical insignificance.
However, LAL testing in human blood has been historically problematic, with considerable variability due to the presence of interfering substances that have either suppressed or spuriously activated the assay (Rowley et al., 1958; Keene et al., 1961; Oroszlam et al., 1966; Rudbach et al., 1966; Skarnes, 1966, 1968; Levin et al., 1970, 1971; Elin et al., 1975, 1976; Wardle, 1979; Freudenberg et al., 1980; Ulevitch et al., 1981; Yoshioka and Konno, 1984; Martel et al., 1985; Dawson, 1993; Elsbach and Weiss, 1993; Hurley, 1994, 1995; Marra et al., 1994; Gnauck et al., 2016). Early efforts to evaluate LAL for detection of endotoxins in patient blood were largely abandoned due to a lack of standardized procedures that often included extensive specimen manipulation (McCabe et al., 1972; Martinez et al., 1973; Stumacher et al., 1973; Feldman and Pearson, 1974; Elin et al., 1975; Zinner and McCabe, 1976; Gnauck et al., 2015). In particular, one published LAL method requires substantial sample dilution (up to 40-fold; Lonza Walkersville Inc, 2014), denaturing (heating at 70°C), and the addition of chemical inhibitors; whereas, dilution can limit sensitivity and overall complexity would lessen practicality as a rapid patient screening assay (Obayashi, 1984; Dawson, 2005; BioDtech Inc., 2014; Lonza Walkersville Inc, 2014). Anticoagulants, chemical inhibitors, and temperature-induced pH changes of the blood after preparation have also been shown to interfere with the reliability of the LAL assay (Gnauck et al., 2016). Further, the ratios and function of the clotting components in pooled LAL reagents from random wild horseshoe crab (HSC, Limulus polyphemus) harvesting are variable, which can affect reaction kinetics, LPS sensitivity, and result in batch-to-batch variation (Massignon et al., 1996).
From a conservation perspective, a clinical application for LAL may not be feasible, as the natural LAL resources are already strained by pharmaceutical sterility testing. Annually, some 600,000 HSCs are captured and bled to meet LAL demand, of which some 30% expire in the process (Atlantic States Marine Fisheries Commission [ASMFC], 2019). Current threats to wild HSC populations include not only biomedical harvesting but also habitat encroachment, global warming, and its use as bait for eel and whelk fisheries, which have negatively impacted population numbers (Krisfalusi-Gannon et al., 2018). The uncertain future of HSCs and the importance of LAL to modern medicine have prompted innovations for sustainable HSC cultivation (Krisfalusi-Gannon et al., 2018). As such, aquaculture has become a promising approach to restore dwindling populations. However, long-term efforts to husband adult HSCs have been largely unreported or unsuccessful for the last three decades (Carmichael and Brush, 2012). After 3–4 months of husbandry, research has indicated that hemolymph protein concentrations drop below reference ranges (3.4–11.8 mg/ml) and result in mortality, which is thought to be linked to nutritional deficiencies that give rise to panhypoproteinemia (Nolan and Smith, 2009).
The first objective of this study was to develop and optimize an indoor recirculating aquaculture system (RAS) for captive HSC husbandry that would facilitate repetitive LAL harvesting while maintaining animal wellbeing. A surgically implanted catheter was developed to achieve a low-impact, routine harvesting method. In this study, hemocyanin (Hc), amebocyte, and LAL activities were analyzed to examine the effect of aquaculture on adult HSCs. These health parameters play an essential role in immunity to defend against pathogens and ensure the overall health of the HSCs (Nagai et al., 2001). The rationale behind this objective was that controlled aquaculture could provide an alternative to current practices and foster conservation by establishing a more sustainable endotoxin testing resource from a finite cohort of captive HSCs. The expansion of LAL resources toward clinical medicine should also emphasize responsible methods of raw material collection. The establishment of an aquaculture model that could produce greater volumes of LAL reliably, sustainably, and economically would benefit nature and industry. Carefully controlled collection of LAL from monitored and well-maintained HSCs in aquaculture could increase LAL supply quantities, ensure species viability, and allow for new clinical innovations. The second objective of this study was to demonstrate the potential use of aquaculture-derived LAL as a rapid, reliable, and cost-effective method for detection of LPS as a marker for gram-negative bacteria that overcomes erratic results heretofore characteristic of testing human blood with LAL assays.
Materials and Methods
Husbandry in RAS
The indoor management and maintenance of HSCs was evaluated in a pair of RAS at a salinity of 19.5–22.0‰. Each RAS was equipped with two holding tanks (4 ft × 6 ft × 1 ft), a biofiltration tank with K1 media (Pentair, Minneapolis, MN, United States), and a clarifying (solids separation) tank (Figure 2). Ambient air supplied oxygen to the water via a piston air pump with supplemental air stones positioned in each tank (Nelson and Pade, Montello, WI, United States). Water was recirculated through biological filtration tanks, allowing for water purification, hygiene, and disease prevention. Water parameters were measured and adjusted as needed six times per week over the course of the study.
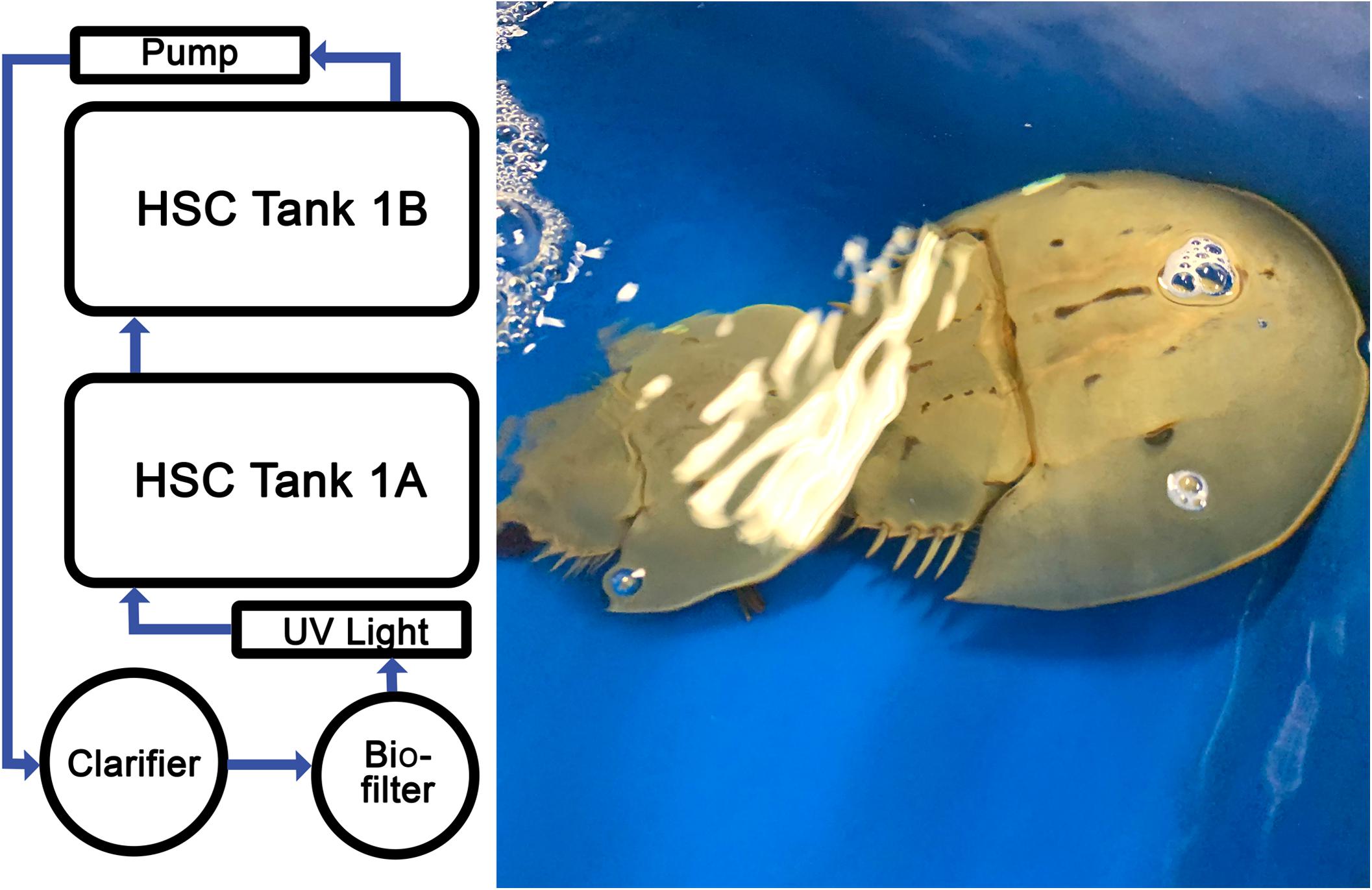
Figure 2. Recirculating aquaculture system (RAS). Temperature-controlled, saltwater system optimized for HSC aquaculture comprising multiple tanks, filtration units, UV sterility lights, and aeration system. Photograph of two HSCs in the RAS engaging in typical breeding behaviors.
HSCs were stocked at a density of one animal per 3 ft2. An isolated circular resin tank (diameter: 3.25 ft) equipped with a solids filter was used as a “hospital” tank to quarantine HSCs prior to RAS introduction and allow for recovery after catheter surgery (see Catheter Implantation section). HSCs (n = 24) were procured (Dynasty Marine Associates, Marathon, FL, United States) or acquired and maintained in accordance with collection and aquaculture operation permits (North Carolina Department of Environmental Quality, Division of Marine Fisheries; #1946771 and #1947050). A health assessment was recorded for each HSC upon intake (i.e., sex, weight, and carapace length/width, as well as appendage, carapace, eye, and mouth evaluation). HSCs sourced directly from the wild (“wild type”) were used as controls for some studies, from which hemolymph extracts were collected within 1 h of habitat removal to establish baselines.
In order to ensure HSC well-being and optimal hemolymph parameters, diet and feeding rates were established over a trial period of 6 weeks prior to the initiation of the study. Feed rates, dietary protein (DP), nutrient composition, and daily energy requirements were determined for natural feed sources and a commercially manufactured aquafeed (Classic Brood 46/12 Complete Feed, Skretting, Tooele, UT, United States). Initial observations revealed that HSCs fed at different rates per day (expressed as a percentage of the b/w of the HSC) depending on the diet (Tinker-Kulberg et al., under review). In this study, the nutrient composition of the selected commercial aquafeed after modification with gelatin (5% w/v) included protein (23%), lipids (6%), carbohydrates (2%), and moisture content (50%). Gelatin served as a binding agent to provide rigidity, extended water stability and nutrient retention; thus eliminating buoyancy (for sinking density) and affording HSCs time to forage on the tank floor to accommodate characteristic bottom scavenging. A daily feed rate of 3% of their b/w sustained (or increased) HSC weight (∼1.2% change on average) and serum protein levels (above 5.0 mg/ml) over the course of the study, with an associated survival rate of 100%. Any feed not consumed after 8 h was removed. Sodium bicarbonate was added to the RAS at 0.12 g per 1.0 g of feed to maintain alkalinity and pH.
Catheter Implantation
The catheter was composed of a radiopaque tube and a valve. For implantation, each HSC was gently immobilized, and the book gills were bathed with saltwater. Protective draping surrounded access to the pericardial membrane, and the region was prepped with 70% ethanol and betadine. An 18-gauge needle on the intravascular system was inserted at a 45° angle toward the prosoma (cephalothorax). Once the appearance of hemolymph was observed in the catheter, the guidewire was inserted and the device positioned. The needle was then removed; the site was cleaned; and a sterile, quick-drying topical tissue adhesive (VetbondTM, 3M, St. Paul, MN, United States) was applied. The catheter was capped with a sterile luer-lock, and it was affixed to the shell of the HSC in the dorsal prosoma region. Postoperative HSCs were monitored during recovery in the hospital tank. Using the implanted catheter, hemolymph was extracted for an initial health evaluation after 7 days, and the HSCs were reintegrated into the RAS cohort.
Hemolymph Collection
Hemolymph was collected via previously established methods for both the implanted and control cohorts (Coates et al., 2012; Anderson et al., 2013). Materials were rendered pyrogen-free by cleaning and overnight soaking in 1% E-Toxa-Clean(R) Solution (Sigma-Aldrich, St. Louis, MO, United States), heating at 200°C for 3 h, and rinsing with endotoxin-free water. For the control group without catheter insertion, the pericardial membrane was cleaned twice with 70% ethanol prior to hemolymph collection with a 22-gauge needle (Becton Dickson, NJ, United States) using a standard SOP (Armstrong and Conrad, 2008); for the implanted HSCs, it was collected using the catheters. An aliquot of the hemolymph was diluted (1:1) in a prechilled mixture of N-ethylmaleimide (0.125%), NaCl (3%), and Tris-HCl (0.5 M, pH 7.5) for amebocyte density analysis (Armstrong and Conrad, 2008; Coates et al., 2012; see Hemocyanin and Amebocyte Density section). Sterile tubes containing the balance of each harvest were centrifuged (1,000-rcf/5-min), and the supernatant was removed. Any samples that appeared clotted after centrifugation were not processed or analyzed. Amebocyte pellets were then washed with endotoxin-free NaCl (3% v/v), and both fractions were stored at −70°C pending analysis and LAL extraction.
Over the course of this research, to monitor the health of individual HSCs, 1 ml of hemolymph was extracted and analyzed for Hc concentration, amebocyte density, and LAL activity. For hemolymph extractions (e.g., 10%), blood volume was calculated as 25% (b/w), and the density was estimated at 0.00104 kg/ml (Hurton et al., 2005).
Hc and Amebocyte Density
Hc and amebocyte density were measured throughout the study as an indicator of HSC health. Total serum protein concentration was used as a proxy for Hc by diluting the hemolymph supernatant in Tris-HCl (0.1 M, pH 7.5) and measuring absorbance at 280 nm on a UV-Vis Spectrometer (Cary 6000i, Agilent Technologies). Dioxygen-bound Hc (Oxy-Hc) was likewise measured at 340 nm. The total protein concentration was calculated according to Nickerson and Van Holde (1971), using the absorption coefficient A280 = 1.39 mg–1 ml–1 cm–1 for Hc, and A350 = 0.223 mg–1 ml–1 cm–1 for Oxy-Hc. The percentage of Hc that was oxygen bound was determined using the ratio of Oxy-Hc (mg/ml) to Hc (mg/ml) × 100.
A 10 μl volume of the diluted aliquot was placed in a hemocytometer and analyzed microscopically. Since amebocytes are susceptible to rapid degranulation, a digital camera was used to record bright-field images from the hemocytometer, and counts were processed off-line using the ImageJ software (National Institutes of Health, Bethesda, MD, United States).
LAL Preparation and Analysis
LAL activity was assessed throughout the study as an indicator of HSC health and to investigate the effects of aquaculture on quality. LAL was prepared by thawing frozen amebocyte pellets on ice and then lysing them in pyrogen-free water at a 1:1 ratio of original hemolymph collection volume under gentle agitation over night at 4°C. Next, a chloroform extraction (1:1 v/v) of the lysate was performed for total protein measurement using a BCA kit (Pierce, Thermo Fisher Scientific, Waltham, MA, United States). All LAL extracts were aliquoted and frozen at −80°C pending analysis (no loss in LAL activity was observed in frozen samples for up to 6 months). Equal protein amounts of the LAL from aquaculture HSCs and commercial kits (E-ToxateTM, Sigma-Aldrich, St. Louis, MO, United States) were diluted to a total volume of 100 μl and tested for their respective and relative activities. All evaluated LAL samples were subjected to a single freeze–thaw cycle to preserve activity.
LAL activity was evaluated turbidimetrically. The commercial kit served as a control, and assays were performed following the manufacturer’s instructions (Sigma-Aldrich, St. Louis, MO, United States). Aquaculture and commercial LAL (100 μl) samples were gently mixed with 100 μl of standard endotoxin solution (final concentration range: 0–50 EU/ml) in a pyrogen-free, 96-well microplate and incubated at 37°C for 1 h. The conversion of coagulogen to coagulin was measured at 340 nm on a microplate reader (BioTek 800TM, BioTek Instruments, Winooski, VT, United States). The turbidity of the blank (endotoxin-free lysate) was subtracted from test values, and the relationship between endotoxin concentration and clot formation was measured against a standard curve derived from commercial LAL.
Equal amounts of amebocyte total protein isolated from the individual HSCs were pooled and analyzed for LAL activity in triplicate. A turbidimetric LAL assay was performed by incubating a standard endotoxin solution (50 EU/ml, Sigma Aldrich, St. Louis, MO, United States) with different concentrations of LAL (0–300 μg). Hemolymph extracted from wild-caught HSCs (“wild type”) was collected within 1 h of capture, from which LAL was processed and frozen at −80°C pending analysis and then used as a control; results were also compared to a commercial LAL kit (E-ToxateTM, Sigma Aldrich, St. Louis, MO, United States). Both controls were diluted to the same concentration as the aquaculture LAL.
Purified protein extracts (10 μg) from commercial, aquaculture, and wild-type LAL were subjected to SDS-PAGE in the presence of β-mercaptoethanol. The total protein concentration of each sample was determined with a BCA assay. Each sample was diluted in a 4 × NuPAGETM loading buffer (Invitrogen, Carlsbad, CA, United States) containing 2.5% β-mercaptoethanol and heated to 85°C for 3 min. Electrophoresis was performed on 8% tris-glycine gels (NovecTM Wedge Well, Invitrogen, Carlsbad, CA, United States) and stained with Coomassie Brilliant Blue R-250 (Bio-Rad, Hercules, CA, United States). A SeeBlue® Plus2 Pre-stained Protein Standard (Invitrogen, Carlsbad, CA, United States) was used as a molecular weight marker.
Aquaculture LAL Assay of Human Blood Specimens
To test the ability for LAL to detect endotoxins (LPS) in human blood, presumably endotoxin-negative human blood was freshly drawn from healthy volunteers using citrated collection tubes (3.2% buffered sodium citrate to inhibit clot formation). Aliquots were then spiked with various concentrations of LPS (Escherichia coli 055:B5, World Health Organization [WHO], 2012) or left neat as negative controls and incubated at room temperature for 15 min (to allow unbound LPS to disperse so as to mimic circulating endotoxins in patient specimens). Before LAL analysis, the samples were centrifuged; aliquots were removed and chemically treated with a matching volume of endotoxin-free reagents (to that of the initial volume) to remove blood components known to interact with LPS and inhibit LAL activation. LAL assays were then performed as described above and demonstrated consistent spiked bacterial concentrations.
To evaluate detection of intact bacteria in human blood, exponential growth of E. coli (Turbo Competent) was selectively achieved in LB broth containing 100 μg/ml of ampicillin to an optical density of 0.35. The culture was centrifuged to pellet the bacteria and washed twice to remove unbound LPS. A sample containing only the LB growth medium in the absence of bacteria was also processed in parallel, to account for any LPS contamination (negative “broth” control). Samples were then resuspended in isotonic buffer (0.9% NaCl), and a bacterial count (CFU/ml) was performed by plating serial dilutions on LB agar plates containing 100 μg/ml of ampicillin. Blood samples were then spiked with equal volumes of bacteria at different concentrations or with the negative “broth” control and incubated at RT for 15 min. All samples were chemically treated as with the LPS-spiked blood samples and then frozen at −70°C for 1 h to destabilize the E. coli cellular membranes. Samples were then thawed at RT, and LAL assays were performed.
LPS-spiked blood (0–50 EU/ml), E. coli-spiked blood (0–5.0 × 105 CFU/ml), and controls were incubated with LAL at 37°C for 1 h at a 1:1 dilution, and clot formation was evaluated. A standard LAL gel clot assay with LPS (0–50 EU/ml) or E. coli (0–5.0 × 105 CFU/ml) was also performed as a positive control. A clot remaining at the bottom of the tube after a gentle 180° inversion was considered positive, whereas if the sample remained liquid and flowed down the tube, the assay result was considered negative. Clots were rated as follows: firm, opaque gel remaining stable after 180° inversion (++); soft gel with moderate to considerate opacity and tube adhesion when rotated 90°; weak gel with slight-to-moderate opacity and adhesion of starch-like floccules to sides of the tube when slanted (±); and no visible increase in viscosity or opacity (–) (Bishop and White, 1986).
Data Analysis
Individual HSC cohorts were used for each respective analysis. HSC Cohort A (n = 6) was used to measure differences in Oxy-Hc and amebocyte density over an 88-day period. HSC Cohort B (n = 6) was used to measure LAL activity in aquaculture HSCs (after 88 days of husbandry) in comparison to LAL from wild-type HSCs and a commercially available LAL kit (E-ToxateTM, Sigma-Aldrich). HSC Cohort C (n = 4) was used to measure differences in amebocyte density, Hc concentrations, and LAL activity (after 6 months of husbandry) for the 10% bleed study. Cohort D (n = 4) served as a no-bleed control to the 10% bleed studies.
Individual HSC data (Oxy-Hc and amebocyte density) varied slightly from animal to animal but with a low standard deviation. The LAL activity derived from individual HSCs was also similar, with low standard deviation. For simplicity and assay accuracy, LAL activity was measured using pooled samples from the entire aquaculture cohort, composed of equal amounts of proteins from individual HSCs. The standard deviation reported for LAL activity represents each assay, performed in triplicate.
Results
HSC Aquaculture Husbandry
The RAS used for this HSC study was designed for conventional aquaculture operations and scalability using standard equipment. Specifically, it was configured to house 24 HSCs, with six in each of four groups. The RAS design, methods, and HSC viability with respect to repetitive bleeding and catheter tolerance were established during the study period (Figure 2).
Determining and maintaining optimal water quality parameters were especially important in assessing the feasibility of an indwelling catheter (Figure 3). Water temperatures were between 17.1 and 21.7°C (desired range: 15.0–25.0°C); pH was maintained from 7.5 to 8.0 (desired range: 7.5–8.8); and dissolved oxygen (DO) remained within 6.3–11.1 mg/L (desired range: >3.0–5.0) across all tanks. The target ammonia concentration was established at <1.0–3.0 ppm, with the average calculated at 0.732 (± 0.206) ppm across the study; and it stayed within the determined cautionary range (Timmons, 1994; Schreibman and Zarnoch, 2009; Ebeling and Timmons, 2010; Shelley and Lovatelli, 2011).
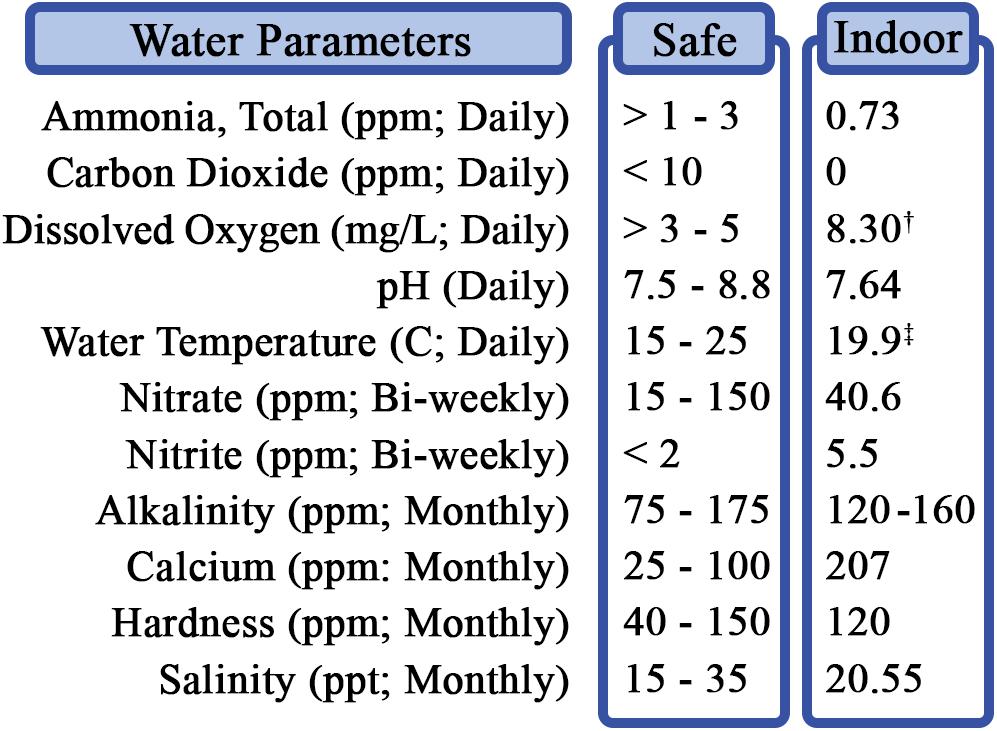
Figure 3. RAS water quality parameters and testing. Measurements were performed daily, weekly, biweekly, or monthly, as indicated. †Average dissolved oxygen range: 6.3–11.1 mg/L. ‡Average temperature range: 17.1–21.7°C.
All HSCs in the RAS thrived throughout a 12-month period, as measured by biologic markers, including LAL activity, bleeding frequency, and breeding behaviors (i.e., copulation and sperm and egg release) and remained healthy throughout the investigation (100% survival). Water parameters did not change significantly throughout the course of the study at the calculated HSC density and feeding rate. Immediate and long-term action plans for system perturbations were established and deemed convenient and effective. The results indicated that HSC RAS husbandry is practicable, that management protocols can be easily executed using conventional equipment, and that available feed inputs are nutritionally robust and affordable (feed cost per pound: $0.78).
HSC Health Assessment After Catheter Implantation
Intravascular catheters were inserted into the respective pericardial membranes of the HSCs, which allowed for routine hemolymph collection up to three times per month (see HSC Health Assessment After Repetitive Hemolymph Collection section; Figure 7) without repetitive membrane punctures (Figure 4). The procedure and device design were optimized over time and well tolerated by the HSCs, as all survived catheter implantation and continued to demonstrate robust health after surgery, as reflected in subsequent testing. The following parameters were analyzed at predetermined intervals (see HSC Health Assessment After Repetitive Hemolymph Collection section) throughout the study: total serum protein concentration; amebocyte concentration (cells/ml); and the LAL activity of amebocyte extracts in response to LPS.
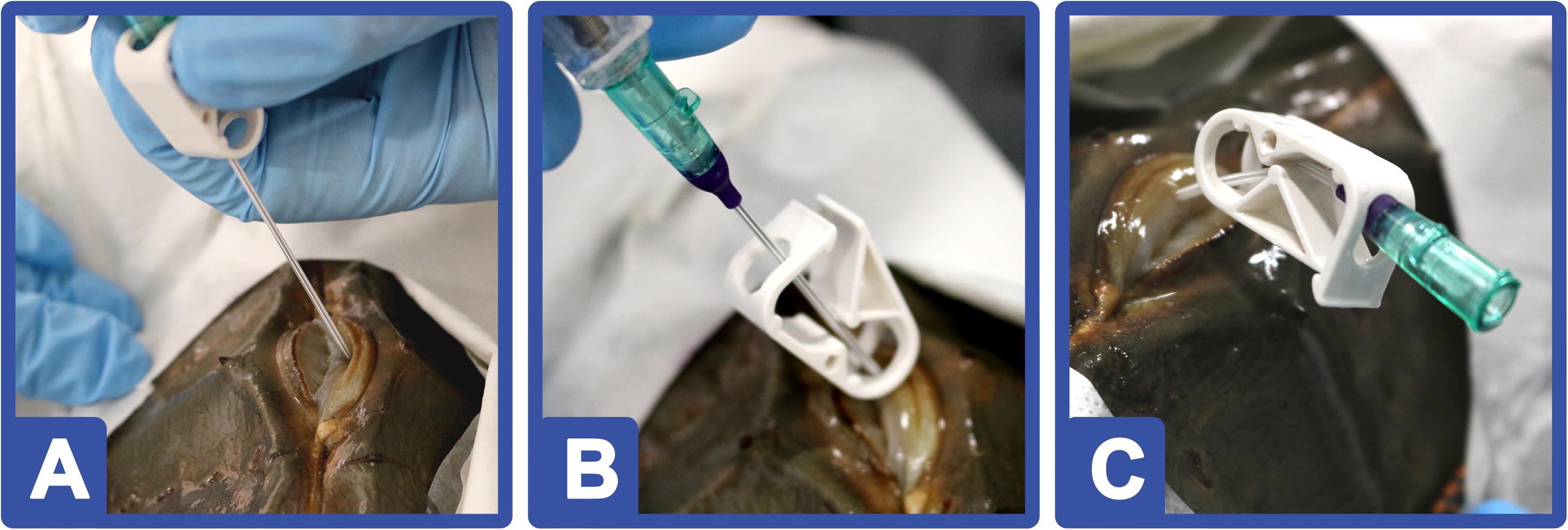
Figure 4. HSC catheter implantation: (A) An 18-gauge needle on an intravascular catheter is inserted into the pericardial membrane toward the prosoma. (B) Once hemolymph was observed in the catheter tubing, a guidewire was deployed. (C) After the catheter was positioned, the needle was retracted; the insertion site cleaned; and a topical adhesive applied. HSCs (n = 12) were monitored in a “hospital” tank for 7 days before returning to the RAS.
Hemocyanin concentration was examined over the course of 88 days (HSC Cohort A; n = 6). The proportion of Hc with dioxygen-bound Hc (i.e., Oxy-Hc) is an indicator of health status, and it remained within a desirable range of 85–95% across all HSCs (Figure 5A). Amebocyte concentration was used to assess immune function and as an indication of well-being versus stress (Coates et al., 2012). The HSC amebocyte density levels remained high throughout the study and correlated with optimal vitality (Figure 5B; Day 0: 1.806E+07; Day 88: 2.093E+07).
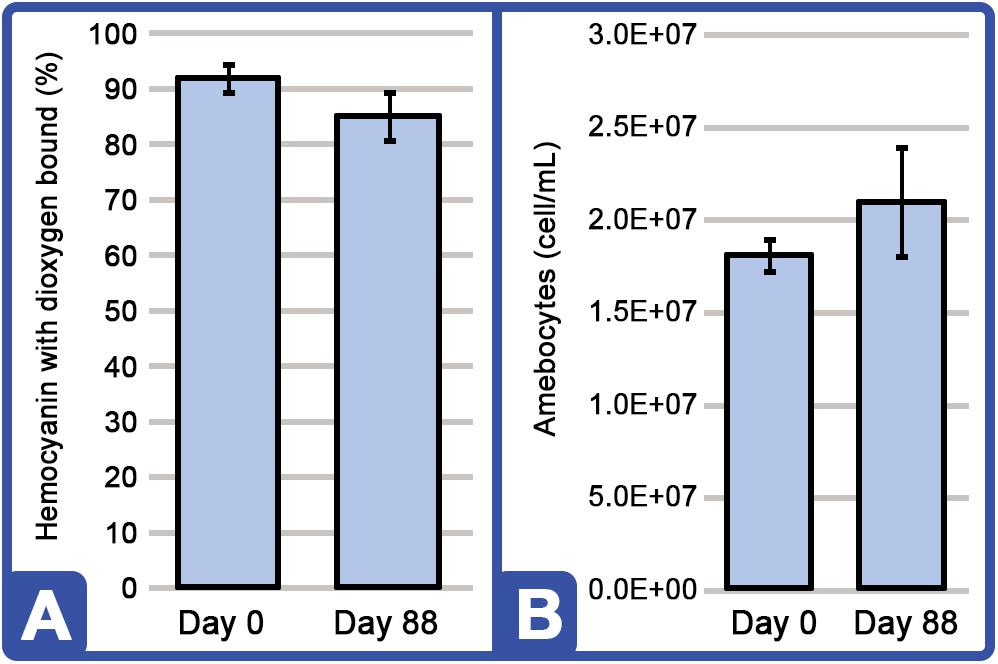
Figure 5. HSC dioxygen-bound hemocyanin (Oxy-Hc) and amebocyte density stability throughout the 88 days study. (A) Percentage of Oxy-Hc from aquaculture HSC hemolymph RAS. (B) Aquaculture HSC amebocyte density concentration. Results are represented as mean ± SD between individual HSCs in Cohort A (n = 6).
The aquaculture LAL (HSC Cohort B; n = 6) had similar activity to that of the wild HSCs, and both extracts revealed slightly increased activity compared with the lyophilized and preserved LAL from the commercial kit (although values were not determined to be statistically significant; Figure 6A). The activity of aquaculture LAL remained constant throughout the 88-day culture period (data not shown). At higher concentrations (150 μg), the enzymatic activity of aquaculture LAL was twofold higher than that of the commercial LAL at an LPS concentration of 50 EU/ml (Figure 6B). These findings suggest that fresh aquaculture lysates may contain a greater abundance of clotting factors per microgram of total extract than that contained by commercial sources, as evidenced by clot formation after 1 h of incubation and as LPS concentrations become saturated, typical of a zero-order kinetic reaction.
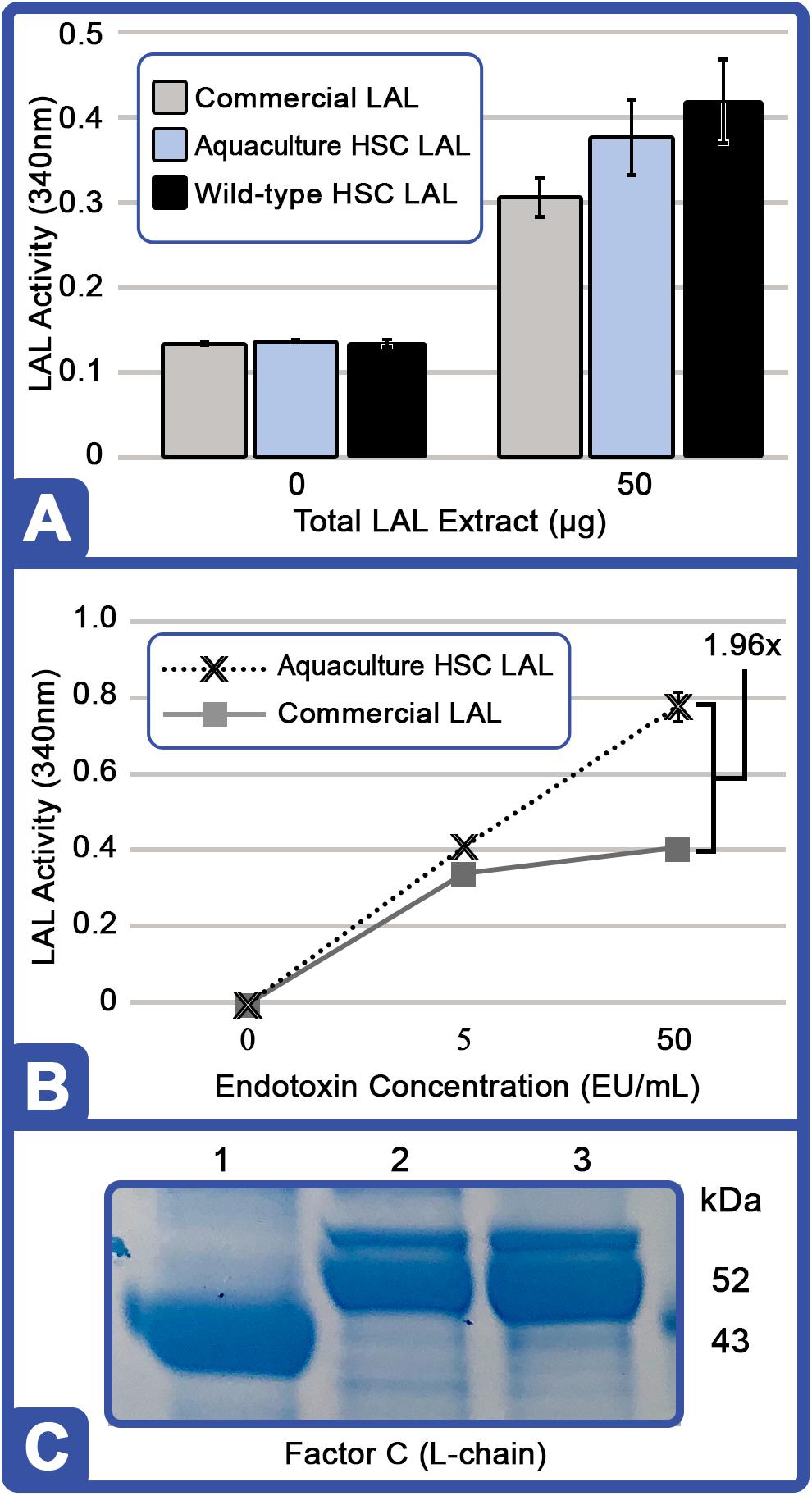
Figure 6. Evaluation and protein analysis of aquaculture-derived LAL at Day 88. (A) Degree of gel clot formation (measured at 340 nm) based on the total protein content of amebocyte extracts (50 μg) using lysates prepared from aquaculture HSC (equal amounts of protein pooled from individual HSC in Cohort B; n = 6); freshly removed from wild-captured HSC (wild-type); or a commercial kit (Sigma E-ToxateTM). The total LPS concentration used for each was 50 EU/ml. (B) Degree of clot formation (measured at 340 nm) of aquaculture LAL (150 μg total protein, equal amounts of protein pooled from individual HSC in Cohort B; n = 6) compared to a commercial kit (150 μg protein) at different endotoxin concentrations: 0, 5.0, and 50.0 EU/ml. (C) Coomassie blue staining of crude protein lysate (10 μg per well): commercial LAL (Lane 1), aquaculture LAL (Lane 2), and wild-type LAL (Lane 3). Aquaculture LAL is representative of equal amounts of protein pooled from individual HSC in Cohort B (n = 6). Molecular weight marker (SeeBlueTM Plus2; Invitrogen, Thermo Fisher Scientific) is indicated.
Crude LAL derived from the aquaculture cohort (HSC Cohort B; n = 6) and the wild control demonstrated similar protein banding; however, different patterns were also observed with the commercial LAL using Coomassie-stained tris-glycine denaturing gel (Figure 6C). One protein band, identified as the L-chain of Factor C, migrated faster in the commercial LAL (∼43 kDa) than in the aquaculture LAL (∼52 kDa), which was confirmed via Western blotting with rabbit polyclonal antibody (COCH, ABclonal, Inc., Woburn, MA, United States; data not shown). Factor C typically generates a 43 kDa subunit (L-chain) under denaturing conditions (Shibata et al., 2018), and thus, the aquaculture-derived Factor C L-chain subunit could migrate at a higher molecular weight due to posttranslational modifications. As lysates prepared from freshly harvested wild HSCs revealed, this higher molecular weight form of Factor C does not appear to be a consequence of aquaculture but rather an effect of commercial LAL preparation (Figure 6C).
HSC Health Assessment After Repetitive Hemolymph Collection
The effects of aquaculture on Hc and amebocyte rebound kinetics were evaluated after repetitive HSC harvests (10% of total hemolymph volume). Hemolymph was drawn at three intervals: Bleed 1 (10% hemolymph volume at time = 0); Bleed 2 (10% hemolymph volume at time = 16 days); and Bleed 3 (1 ml of hemolymph at time = 23 days, health assessment; Figure 7). HSC amebocyte density, total serum protein concentration, and LAL activity were thus measured to assess the impact of consecutive bleeding at t0 = 0 days, t1 = 16 days, and t3 = 23 days (n = 4; Figure 7).
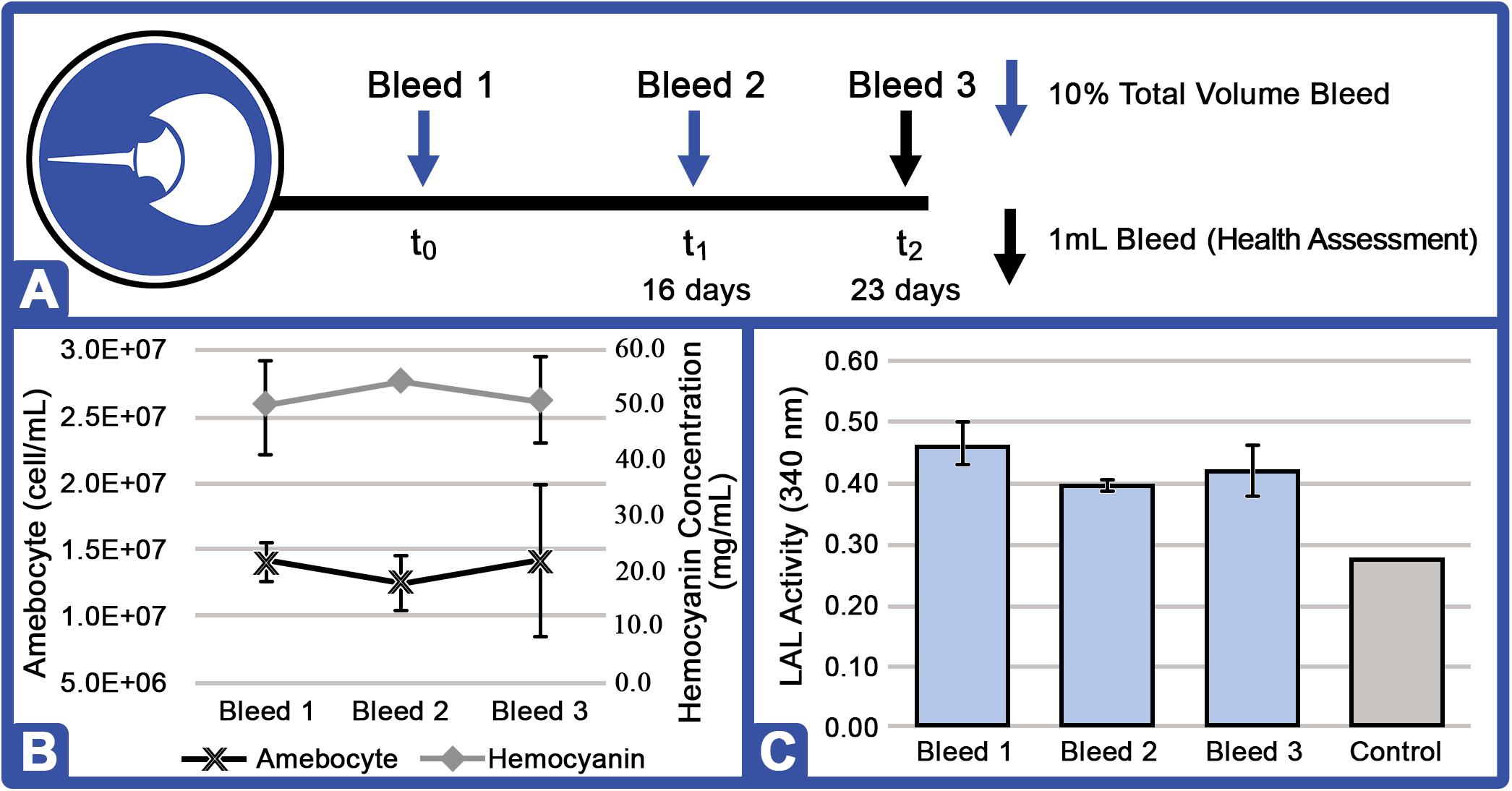
Figure 7. Amebocyte density, hemocyanin concentration, and LAL activity of aquaculture HSC after repeat hemolymph collection. (A) After 6 months of aquaculture, each HSC (n = 4) was bled at t0 and t1 (16 days after t0) for 10% total hemolymph volume and returned to the RAS, and a final health assessment sampling (1 ml bleed) was performed at t3 (23 days after t0). (B) Representative HSC amebocyte density and hemocyanin concentration. (C) Aquaculture HSC LAL activity after bleeds compared to control (commercial kit LAL; Sigma E-ToxateTM) at the same protein concentration (300 μg protein) exposed to 50 EU/ml of LPS.
Average serum protein levels recovered after each successive 10% hemolymph extraction (t0 vs. t1 = 10.2% Δ; t1 vs. t2 = 6.7% Δ; Figure 7A). Amebocyte density decreased slightly (−10.7% Δ) after the first collection (t0 vs. t1) and rebounded above baseline after the second (12.8% increase between t1 and t2, however; this increase was associated with a higher standard deviation among individual HSCs). LAL activity was notably higher after the second instance over the 7-day recovery period (t0 vs. t1 = −14.9% Δ and t1 vs. t2 = + 5.0% Δ; Figure 7C). As a “no-bleed” control, a health assessment specimen (1.0 ml) from a second HSC cohort (n = 4) was taken at the same time and analyzed in parallel, with no significant change in hemolymph parameters (data not shown).
Detection of Endotoxins and Gram-Negative Bacteria in Human Blood
Aquaculture LAL was evaluated for detection of endotoxins (LPS-spiked) in chemically treated blood samples and compared to commercial standards. The previously described method (Bishop and White, 1986) to evaluate clot integrity was followed [Figure 8: firm gels (++); soft gels (+); weak clots (±); and no gelation (–)]. LPS-spiked blood samples and LAL commercial standards yielded comparable degrees of gelation from 0.5 to 50.0 EU/ml (Table 1). Strong clots (++) formed at endotoxin levels of 12.5–50 EU/ml; soft gel clots (+) formed at 3.125–6.25 EU/ml, and weak gel clots (±) formed at 0.5 EU/ml in both LPS-spiked blood samples and LAL commercial standards, while at the lowest concentration of 0.05 EU/ml, no gel clots were discernible [(–) clot rating; Table 1]. The neat (negative) blood samples remained viscous yet revealed no gelation (–).
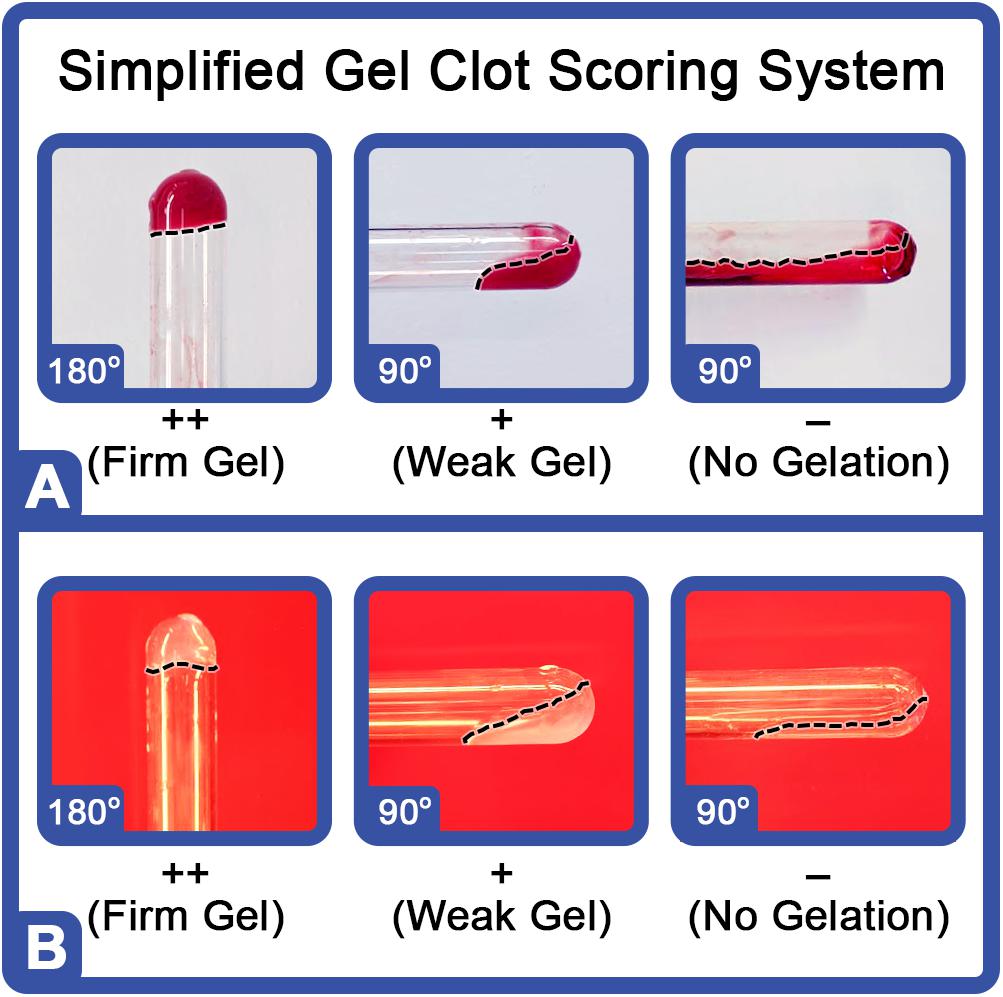
Figure 8. Visual scoring of gelation of LAL in the presence of LPS or bacteria. Representative results of LAL in the presence of LPS or bacteria in (A) human blood or (B) a standard control (endotoxin-free water). The gel clot detection range of 0.05–50.0 EU/ml was used in the test. Clot ratings: firm, opaque gel remaining stable after 180° inversion (++); soft gel with moderate to considerate opacity and tube adhesion when rotated 90° (+); weak gel with slight-to-moderate opacity and adhesion of starch-like floccules to sides of the tube when slanted (±); and no visible increase in viscosity or opacity (–) (Bishop and White, 1986).
Human blood with and without anticoagulant (heparin) demonstrated similar results: chemical treatment of the blood was required in order to detect LPS; whereas gel clots did not form in untreated blood samples (with or without anticoagulant) across the range of endotoxin concentrations (up to 50 EU/ml). This was consistent with previous studies showing that human blood inhibits LAL reactions (Martel et al., 1985; Lonza Walkersville Inc, 2014).
When E. coli was added to treated blood at concentrations between 0.5 and 500,000 CFU/mL, a firm gel clot formed [(++) clot rating; Table 2]. Interestingly, bacterial concentrations lower than 5,000 CFU/ml were not detectable in the absence of treated blood. To validate whether the treatment altered LPS activation of LAL, the LPS (0.05–50 EU/ml) was also added following chemical treatment, and clot formation was consistent with that of LPS spiked-blood samples prior to treatment (data not shown). Blood spiked with both free LPS and bacteria (exponential-phase) activated LAL clot formation (Tables 1, 2) with no false positives or false negatives observed. The “broth” control (no bacteria) did not form a gel clot, also suggesting that the bacterial growth medium was sufficiently washed away from the bacteria in the procedure and did not cause a false positive in the bacteria-spiked samples (Table 2).
Discussion
Current wild harvest practices typically correspond with the HSC spawning season (May and June), when they swarm beaches and allow for easy collection, despite subsequent exposure to the attendant temperature and potential hypoxia variables associated with boat and truck transport to and from bleeding facilities. This period represents an especially vulnerable metabolic phase for the HSCs, whereby coastal migration and breeding activities (laying and subsequently fertilizing millions of eggs) are energy intensive (Sullivan and Watson, 1974; Leschen and Correia, 2010; Malkoski, 2010; James-Pirri, 2012; Anderson et al., 2013; Owings et al., 2019). These factors combine at the most perilous time to subject HSCs to bleeding and hemolymph depletion from a migratory, reproductive, and ultimately, LAL resource quality perspective. They further underscore the rationale for end-users and regulators to require all commercial LAL to be sustainably sourced from aquaculture by a date certain upon scale-up for the sector.
Our results demonstrate the potential to practicably harvest hemolymph from aquaculture HSCs (two distinct 10% extractions per month or potentially 12–24 times annually), in contrast to singular, commercial harvest requiring wild capture, extended habitat extraction, puncture and bleeding (typically 30% of hemolymph volume), and transport back to the shore during spawning season. Every HSC received ample nutrition before and after in situ hemolymph harvest that was completed within minutes, rather than days outside the water, allowing for a more rapid recovery while eliminating hypoxia and myriad transport and habitat extraction risks.
HSC vitality was demonstrated, as reflected by stable Hc levels, amebocyte density, and aquaculture LAL activity (Figures 5, 6) over the course of the study. Both diet and water quality in indoor aquaculture were shown to be controllable and optimized while yielding well-fed, vigorous HSCs. Moreover, aquaculture demonstrated enhanced reactivity when compared to that in commercial kits, which may be an indicator of healthier and less stressed animals in an optimized environment. Aquaculture also enabled repetitive, low-impact bleeding via an indwelling catheter proximal to the water, thereby eliminating the risks of repeat membrane puncture, transport, and lengthy extraction periods likely to account for the current loss of up to 30% of the harvested wild crabs each year (Hurton and Berkson, 2006; Leschen and Correia, 2010).
Determination of optimal hemolymph collection intervals and volumes was essential for rapid recovery, highly reactive LAL production, and maintenance of animal health. Previous reports showed that 4 months was required to reestablish baseline amebocyte counts after a 30% bleed with “well-fed” captive HSCs in laboratory settings and outdoor tanks (Rudloe, 1983; Novitsky, 1984; Anderson et al., 2013); whereas, just 60% of baseline Hc concentrations were restored after 6 weeks (Coates et al., 2012; Anderson et al., 2013). In contrast, we found that HSCs bled at 10% recovered serum protein after 16 days, and a subsequent (second) 10% harvest appeared to trigger an increase in amebocyte counts after 7 days (Figure 7B). The reactivity of the LAL was notably higher after the second instance over the 7-day recovery period (Figure 7C). This rapid proliferation of circulating amebocytes may be a physiologic response to the initial bleeding (Hufgard, 2012).
HSC aquaculture has disruptive potential, especially given the threatened collapse of Asian populations (Akbar John et al., 2018), consequent pressure on Atlantic HSCs, and the self-evident global conservation benefits from species recovery in North America and preservation in Asia. HSC aquaculture could match industry needs for several years with the equivalent of 5–10% of one year’s annual catch, leaving nearly 600,000 HSCs in the wild each year thereafter. In fact, these findings suggest that ∼60,000 aquaculture HSCs could be sustainably bled 12–24 times annually and exceed current biomedical LAL demand. Notably, such a finite cohort would likewise amount to a fraction of the 30% of the harvested HSCs that would otherwise perish every year (Atlantic States Marine Fisheries Commission [ASMFC], 2019). Estimated average life spans of up to 20 years (Shuster, 1958) could further position HSCs as a highly productive aquaculture species with competitive economic potential.
This study also demonstrated the potential for a rapid, reliable, and cost-effective method for detection of LPS as a marker for gram-negative bacteria that overcomes erratic results heretofore characteristic of testing human blood with LAL assays. Separation of specific blood components and a proprietary chemical treatment were shown to isolate LPS and therefore gram-negative bacteria from inhibitors without heating or dilution, thereby allowing for low levels of detection using a gel clot assay (Figure 8).
In patients experiencing sepsis, blood concentrations of LPS as high as 3.5 EU/ml, or 300 ng/ml, and above, have been documented, although specific levels may vary with the type of bacteria (Brandtzaeg et al., 1989). Nonetheless, recovery of spiked LPS and bacteria from modified blood was achieved, even at low concentrations. The specimen preparation method resulted in LPS detection in clinically relevant ranges (3.125 EU/ml of free LPS and 1.0–500,000 CFU/ml of gram-negative bacteria; Tables 1, 2), which would be suitable for early screening of patients at risk for septicemia, even if asymptomatic, to inform appropriate clinical management. While this assay would screen for gram-negative pathogens, it could save valuable time from earlier initiation and results from cultures for identification and susceptibility given the length of time required to perform them (2–3 days). Ultimately, LAL clinical endotoxin screening could outperform current culture and PCR-based methods in speed, labor, and cost.
In addition to human diagnostic potential, a robust, sustainable source of LAL could play a vital role in other sectors. In the agricultural arena, nearly 80% of antibiotics in the US are sold for use in livestock feed (Martin et al., 2015). Intensive agriculture operations are used to raise large numbers of livestock in confined facilities, whereby animals are kept in close proximity. Identifying, isolating, and treating sick animals can prevent disease spread, avoid unnecessary antibiotic administration to healthy animals, reduce risks of antibiotic resistance, protect food supplies, and improve animal welfare. The factors driving research in this space include food security, global demand for animal-derived food products, and increasing incidence of zoonotic diseases at these facilities (Krehbiel, 2013). The same platform could also help ensure safety for agricultural personnel routinely exposed to such pathogens.
Other clinical and diagnostic applications for sustainable, abundant LAL-based assays could include helping municipal shelters in housing large populations of at-risk companion animals to reduce disease transmission, while save associated veterinary resources, and ultimately maximize adoption rates of healthy rescues. And food security applications could be used to prevent harmful pathogens from entering the supply chain, reduce costly management of food-borne illness, and protect public safety as well as food producers’ reputations.
Conclusion
HSC aquaculture with year-round, low-impact harvesting could supply current biomedical LAL demand from a fraction of the harvested HSCs that would otherwise perish every year (Atlantic States Marine Fisheries Commission [ASMFC], 2019), while eliminating the potentially deleterious effects of current practices. At scale, these findings suggest that ∼60,000 aquaculture HSCs (equivalent of 5–10% of one year’s annual catch) could be sustainably bled 12–24 times annually and exceed current biomedical LAL demand. Estimated average life spans of up to 20 years (Shuster, 1958) could further position HSCs as a highly productive aquaculture species with competitive economic potential.
Controlled-environment aquaculture and hemolymph collection could therefore alter the practice of wild harvest, expand the global LAL supply, and avert related viability threats, while building an irrefutable case for species conservation. Innovations like recombinant Factor C (rFC), an alternative to LAL assays, have emerged to address declining HSC populations (Bolden, 2019), with recent FDA approval for use of rFC in Eli Lilly’s EmgalityTM (galcanezumab). However, additional capital resources for equipment and end-user validation are also required to adopt such alternatives, which would be anticipated as significant drivers of industry demand and rapid acceptance of assays using abundant, sustainable, aquaculture-derived LAL.
These findings have demonstrated the potential for HSC aquaculture to alter the way that the current LAL industry operates and serve as a source of abundant, sustainable, and highly reactive LAL – with global conservation benefits from species recovery in North America and preservation in Asia. In turn, an expanded global supply of sustainable LAL raw material would provide a compelling rationale to develop new clinical, agricultural, veterinary, and food safety applications. Given the specter of antimicrobial resistance, these advances would address the urgent need for a new paradigm that moves from widespread antibiotic prophylaxis to dispositive, timely therapeutic administration for gram-negative pathogens consistent with human and veterinary disease management standards.
Data Availability Statement
All datasets generated for this study are included in the article/supplementary material.
Ethics Statement
The research on the HSCs (Limulus polyphemus) involved in this study did not require approval. HSCs are classified as an exempt invertebrate species. All HSC collection and aquaculture techniques and methods were reviewed by the North Carolina Department of Environmental Quality, Division of Marine Fisheries (Collection permit #1946771 and Aquaculture operation permit #1947050).
Author Contributions
RT-K, KD, TB, and AD conceived the research hypothesis and developed the research objectives and experimental plans. RT-K, KD, CK, and TB verified the materials and methods. RT-K, KD, LR, and AD conducted the research experiments. AD supervised the findings of this work. All authors prepared analysis of the primary research and contributed to the final writing of the manuscript. SA was a DVM and Ph.D. in Veterinary Nutrition with 25+ years of clinical nutrition experience and provided nutritional guidance. FL was an expert in blood coagulation, regulatory affairs, and bedside testing and provided diagnostic expertise for the analysis of clinical specimens in the LAL experimentation. JL was a critical care physician and cardiac anesthesiologist engaged in sepsis treatment, transfusion, and ECMO and provided experimental guidance on developing a LAL-based blood diagnostics for patient care.
Funding
The authors would like to acknowledge the following funding sources: National Science Foundation Small Business Innovation Research Grant program (Grant #1819562); North Carolina Board of Science & Technology; One North Carolina SBIR Matching Funds Program; and North Carolina Sea Grant (Grant #2017-R/MG-1712).
Conflict of Interest
AD, RT-K, TB, and LR were employed by Kepley BioSystems, Inc. KD and CK were full-time professors at the Joint School of Nanoscience and Nanoengineering. JL was a practicing critical care physician and cardiac anesthesiologist; he was also a Professor of Anesthesiology and Surgery (Cardiothoracic), and Co-Director of the Cardiothoracic Surgical Intensive Care Unit at Duke University Medical Center; and JL serves as the head of the advisory board for Kepley Biosystems, Inc. FL was President and Owner of LADUCA RCA LLC in New Jersey, an independent consultant and adviser to the medical industry, and serves on the advisory board at Kepley BioSystems, Inc. SA was an Assistant Professor of Clinical Nutrition at the Ontario Veterinary College, University of Guelph, and Owner of Sit, Stay, Speak Nutrition, LLC; SA specializes in nutritional coaching and serves as a scientific advisor for Kepley Biosystems, Inc. The authors alone are responsible for the content and writing of the article.
Acknowledgments
We thank our peers and collaborators at Gateway Research Park, Ecological Research and Development Group (Glenn Gauvry); Joint School of Nanoscience and Nanoengineering; National Science Foundation; North Carolina Board of Science & Technology; North Carolina Sea Grant; and the University of Georgia Marine Extension and Georgia Sea Grant. We thank Lauren Perdue, a dedicated senior at North East Guilford High School (McLeansville, NC), for her daily help in measuring aquaculture system water parameters and HSC feeding, as well as Melinda K. M. Goddard, Principal of ClienTell® Consulting, LLC, for editing the manuscript.
Abbreviations
ASMFC, Atlantic States Marine Fisheries Commission; BCA, Bicinchoninic acid assay; b/w, Body weight; CFU, Colony-forming unit; COCH, Cochlin; DO, Dissolved oxygen; HSC, Horseshoe crab; E. coli, Escherichia coli; EU, Endotoxin unit; g/mol, Grams per mole; kDa, kilodalton; kJ, kilojoules; Hc, Hemocyanin; LAL, Limulus amebocyte lysate; LB agar, Luria-Bertani agar; L-chain, Light chain; LPS, Lipopolysaccharide; mmol/L, Millimoles per liter; NaCl, Sodium chloride; nm, Nanometers; N-NH3–, Ammonia nitrogen; Oxy-Hc, Oxyhemocyanin; PCR, Polymerase chain reaction; ppm, Parts per million; RAS, Recirculating aquaculture system; rcf, Relative centrifugal force; rFC, Recombinant Factor C; SOP, Standard operating procedure; Tris-HCl, Tris(hydroxymethyl)aminomethane hydrochloride; v/v, Volume/volume; w/v, Weight/volume.
References
Akbar John, B., Nelson, B. R., Sheikh, H. I., Cheung, S. G., Wardiatno, Y., Dash, B. P., et al. (2018). A review on fisheries and conservation status of Asian horseshoe crabs. Biodiv. Conserv. 27, 3573–3598. doi: 10.1007/s10531-018-1633-8
Anderson, R. L., Watson, W. H. III, and Chabot, C. C. (2013). Sublethal behavioral and physiological effects of the biomedical bleeding process on the American horseshoe crab, Limulus polyphemus. Biol. Bull. 225, 137–151. doi: 10.1086/BBLv225n3p137
Armstrong, P., and Conrad, M. (2008). Blood collection from the American horseshoe crab, Limulus polyphemus. J. Vis. Exp. 20:e958. doi: 10.3791/958
Atlantic States Marine Fisheries Commission [ASMFC] (2019). Horseshoe Crab Stock Benchmark Stock Sssessment and Peer Review Report. Arlington, VA: ASMFC.
Baker, S. J., Payne, D. J., Rappuoli, R., and De Gregorio, E. (2018). Technologies to address antimicrobial resistance. Proc. Natl. Acad. Sci. U.S.A. 115, 12887–12895. doi: 10.1073/pnas.1717160115
Bates, D. W., Parsonnet, J., Ketchum, P. A., Miller, E. B., Novitsky, T. J., Sands, K., et al. (1998). Limulus amebocyte lysate assay for detection of endotoxin in patients with sepsis syndrome. Clin. Infect. Dis. 27, 582–591. doi: 10.1086/514713
Berg, D., and Gerlach, H. (2018). Recent advances in understanding and managing sepsis. F1000Research 7:1570. doi: 10.12688/f1000research.15758.1
BioDtech Inc. (2014). Accurate Endotoxin Detection in Human Plasma with ESP. Available online at: http://biodtechinc.com/wp-content/uploads/2014/09/ESP%E2%84%A2-Application-Note.pdf (accessed November 13, 2014).
Bishop, J. R., and White, C. H. (1986). Assessment of dairy product quality and potential shelf-life: a review. J. Food Protect. 49, 739–753. doi: 10.4315/0362-028X-49.9.739
Bolden, J. (2019). “Recombinant factor C in endotoxin detection and control,” in Pharma, Limulus, and Mammalian Systems, ed. K. L. Williams (Cham: Springer), 497–521. doi: 10.1007/978-3-030-17148-3_13
Brandtzaeg, P., Kierulf, P., Gaustad, P., Skulberg, A., Bruun, J. N., Halvorsen, S., et al. (1989). Plasma endotoxin as a predictor of multiple organ failure and death in systemic meningococcal disease. J. Infect. Dis. 159, 195–204. doi: 10.1093/infdis/159.2.195
Carmichael, R. H., and Brush, E. (2012). Three decades of horseshoe crab rearing: a review of conditions for captive growth and survival. Rev. Aquac. 4, 32–43. doi: 10.1111/j.1753-5131.2012.01059.x
Casey, L. C., Balk, R. A., and Bone, R. C. (1993). Plasma cytokine and endotoxin levels correlate with survival in patients with the sepsis syndrome. Ann. Intern. Med. 119, 771–778. doi: 10.7326/0003-4819-119-8-199310150-00001
Coates, C. J., Bradford, E. L., Krome, C. A., and Nairn, J. (2012). Effect of temperature on biochemical and cellular properties of captive Limulus polyphemus. Aquaculture 334, 30–38. doi: 10.1016/j.aquaculture.2011.12.029
Cooper, J. F. (2001). “Bacterial endotoxins test,” in Microbiology in Pharmaceutical Manufacturing, ed. R. Prince (Bethesda, MD: Parenteral Drug Association).
Ebeling, J. M., and Timmons, M. B. II (2010). Recirculating Aquaculture. New York, NY: Cayuga Aqua Ventures LLC.
Elin, R. J., Robinson, R. A., Levine, A. S., and Wolff, S. M. (1975). Lack of clinical usefulness of the Limulus test in the diagnosis of endotoxemia. New Engl. J. Med. 293, 521–524. doi: 10.1056/NEJM197509112931102
Elin, R. J., Sandberg, A. L., and Rosenstreich, D. L. (1976). Comparison of the pyrogenicity, Limulus activity, mitogenicity and complement reactivity of several bacterial endotoxins and related compounds. J. Immunol. 117, 1238–1242.
Elsbach, P., and Weiss, J. (1993). The bactericidal/permeability-increasing protein (BPI), a potent element in host-defense against gram-negative bacteria and LPS. Immunobiology 187, 417–429. doi: 10.1016/0952-7915(93)90088-A
Feldman, S., and Pearson, T. A. (1974). The Limulus test and gram-negative bacillary sepsis. Am. J. Dis. Child. 128, 172–174. doi: 10.1001/archpedi.1974.02110270046009
Fleishman, J., and Fowlkes, F. (1982). A comparison of pyrogenicity of bacterial endotoxins from a variety of gram-negative bacteria as determined by the LAL test. Prog. Clin. Biol. Res. 93, 131–140.
Food and Drug Administration [FDA] (1987). Guideline on Validation of the Limulus Amebocyte Lysate Test as an End-Product Endotoxin Test for Human and Animal Parenteral Drugs, Biological Products, and Medical Devices. Rockville, MD: Food and Drug Administration.
Freudenberg, M. A., Bøg-Hansen, T. C., Back, U., and Galanos, L. (1980). Interaction of lipopolysaccharides with plasma high-density lipoprotein in rats. Infect. Immun. 28, 373–380. doi: 10.1016/B978-0-08-024952-0.50044-2
Gnauck, A., Lentle, R. G., and Kruger, M. C. (2015). The Limulus amebocyte lysate assay may be unsuitable for detecting endotoxin in blood of healthy female subjects. J. Immunol. Methods 416, 146–156. doi: 10.1016/j.jim.2014.11.010
Gnauck, A., Lentle, R. G., and Kruger, M. C. (2016). Chasing a ghost?: issues with the determination of circulating levels of endotoxin in human blood. Crit. Rev. Clin. Lab. Sci. 53, 197–215. doi: 10.3109/10408363.2015.1123215
Hochstein, H. D. (1990). “Role of the FDA in regulating the Limulus amoebocyte lysate test,” in Clinical Applications of the Limulus Amoebocyte Lysate Test, ed. R. B. Prior (Boca Raton, FL: CRC Press), 37–51.
Hufgard, J. R. (2012). Amebocyte Diameter and Density After Partial Exsanguinations in Limulus Polyphemus Hemolymph. Terre Haute, IN: Rose-Hulman Research Publications.
Hurley, J. C. (1994). Concordance of endotoxemia with gram-negative bacteremia in patients with gram-negative sepsis: a meta-analysis. J. Clin. Microbiol. 32, 2120–2127. doi: 10.1128/jcm.32.9.2120-2127.1994
Hurley, J. C. (1995). Endotoxemia: methods of detection and clinical correlates. Clin. Microbiol. Rev. 8, 268–292. doi: 10.1128/CMR.8.2.268
Hurley, J. C., Nowak, P., Öhrmalm, L., Gogos, C., Armaganidis, A., and Giamarellos-Bourboulis, E. J. (2015). Endotoxemia as a diagnostic tool for patients with suspected bacteremia caused by gram-negative organisms: a meta-analysis of four decades of studies. J. Clin. Microbiol. 53, 1183–1191. doi: 10.1128/JCM.03531-14
Hurton, L., and Berkson, J. (2006). Potential causers of mortality for horseshoe crabs (Limulus polyphemus) during the biomedical bleeding process. Fish. Bull. 104, 293–298.
Hurton, L., Berkson, J., and Smith, S. (2005). Estimation of total hemolymph volume in the horseshoe crab Limulus Polyphemus. Mar. Freshw. Behav. Physiol. 2, 139–147. doi: 10.1080/10236240500064354
Hynes, M. D. (2016). Preparing for FDA Pre-Approval Inspections: A Guide to Regulatory Success. Boca Raton, FL: CRC Press.
James-Pirri, M. J. (2012). Assessment of spawning horseshoe crabs (Limulus polyphemus) at Cape Cod National Seashore, 2008–2009. Natural Resource Technical Report NPS/CACO/NRTR-2012/573 (Washington, DC: U.S. Department of the Interior).
Jean-Baptiste, E. (2007). Cellular mechanisms in sepsis. J. Intensive Care Med. 22, 63–72. doi: 10.1177/0885066606297123
Keene, W. R., Landy, M., and Shear, M. J. (1961). Inactivation of endotoxin by a humoral component VII: enzymatic degradation of endotoxin by blood plasma. J. Clin. Investig. 40, 302–310. doi: 10.1172/JCI104257
Ketchum, P. A., Parsonnet, J., Stotts, L. S., Novitsky, T. J., Schlain, B., Bates, D. W., et al. (1997). Utilization of a chromogenic Limulus amebocyte lysate blood assay in a multi-center study of sepsis. J. Endotoxin Res. 4, 9–16. doi: 10.1177/096805199700400102
Krehbiel, C. (2013). The role of new technologies in global food security: improving animal production efficiency and minimizing impacts. Anim. Front. 3, 4–7. doi: 10.2527/af.2013-0017
Krisfalusi-Gannon, J., Ali, W., Dellinger, K., Robertson, L., Brady, T. E., Goddard, M. K., et al. (2018). The role of horseshoe crabs in the biomedical industry and recent trends impacting species sustainability. Front. Mar. Sci. 5:185. doi: 10.3389/fmars.2018.00185
Kumar, A., Roberts, D., Wood, K. E., Light, B., Parrillo, J. E., Sharma, S., et al. (2006). Duration of hypotension before initiation of effective antimicrobial therapy is the critical determinant of survival in human septic shock. Crit. Care Med. 34, 1589–1596. doi: 10.1097/01.CCM.0000217961.75225.E9
Leschen, A. S., and Correia, S. J. (2010). Mortality in female horseshoe crabs (Limulus polyphemus) from biomedical bleeding and handling: implications for fisheries management. Mar. Freshw. Behav. Physiol. 43, 135–147. doi: 10.1080/10236241003786873
Levin, J., Poore, T. E., Zauber, N. P., and Oser, R. S. (1971). Detection of endotoxin in the blood of patients with sepsis due to gram-negative bacteria. New Engl. J. Med. 283, 1313–1316. doi: 10.1056/NEJM197012102832404
Levin, J., Tomasulo, P. A., and Oser, R. S. (1970). Detection of endotoxin in human blood and demonstration of an inhibitor. J. Lab. Clin. Med. 75, 903–911.
Lonza Walkersville Inc (2014). Certificate of Analysis, Product: Kinetic-QCL 192 Test Kit. Walkersville, MD: Lonza Walkersville Inc.
MacVane, S. H. (2017). Antimicrobial resistance in the intensive care unit: a focus on gram-negative bacterial infections. J. Intensive Care Med. 32, 25–37. doi: 10.1177/0885066615619895
Malkoski, V. (2010). Massachusetts 2010 Compliance Report to the Atlantic States Marine Fisheries Commission: Horseshoe Crab. Boston, MA: Division of Marine Fisheries.
Marra, M. N., Thornton, M. B., Snable, J. L., Wilde, C. G., and Scott, R. W. (1994). Endotoxin-binding and-neutralizing properties of recombinant bactericidal/permeability-increasing protein and monoclonal antibodies HA-1A and E5. Crit. Care Med. 22, 559–565. doi: 10.1097/00003246-199404000-00009
Martel, F., Adenot, N., Denhaut, G., Martin, F., Robert, F., and Chataing, B. (1985). Limulus test using a chromogenic method: application to the control of pyrogens in blood derivatives. Rev. Fr. Transfus. Immunohematol. 28, 237–250. doi: 10.1016/S0338-4535(85)80115-X
Martin, M. J., Thottathil, S. E., and Newman, T. B. (2015). Antibiotics overuse in animal agriculture: a call to action for health care providers. Am. J. Public Health 105, 2409–2410. doi: 10.2105/AJPH.2015.302870
Martinez, L. A., Quintiliani, R., and Tilton, R. C. (1973). Clinical experience on the detection of endotoxemia with the Limulus test. J. Infect. Dis. 27, 102–105. doi: 10.1093/infdis/127.1.102
Massignon, D., Lepape, A., Debize, G., Remillieux, M. F., De Pasquale, V., Banssillon, V., et al. (1996). Detection of gram-negative bacteremia in early sepsis by a quantitative chromogenic and kinetic endotoxin assay. Eur. J. Clin. Investig. 26, 596–601. doi: 10.1046/j.1365-2362.1996.1810531.x
McCabe, W. R., Kreger, B. E., and Johns, M. (1972). Type-specific and cross-reactive antibodies in gram-negative bacteremia. New Engl. J. Med. 287, 261–267. doi: 10.1056/NEJM197208102870601
Nagai, T., Osaki, T., and Kawabata, S. I. (2001). Functional conversion of hemocyanin to phenoloxidase by horseshoe crab antimicrobial peptides. J. Biol. Chem. 276, 27166–27170. doi: 10.1074/jbc.M102596200
Nickerson, K., and Van Holde, K. E. (1971). A comparison of molluscan and arthropod hemocyanin. I. Circular dichroism and absorption spectra. Comp. Biochem. Physiol. 39B, 855–872. doi: 10.1016/0305-0491(71)90109-X
Nolan, M. W., and Smith, S. A. (2009). “Clinical evaluation, common diseases, and veterinary care of the horseshoe crab, Limulus polyphemus,” in Biology and Conservation of Horseshoe Crabs, eds J. T. Tanacredi, M. L. Botton, and D. Smith (Boston, MA: Springer), 479–499. doi: 10.1007/978-0-387-89959-6_30
Novitsky, T. J. (1984). Discovery to commercialization: the blood of the horseshoe crab. Oceanus 27, 19–26.
Obayashi, T. (1984). Addition of perchloric acid to blood samples for colorimetric Limulus test using chromogenic substrate: comparison with conventional procedures and clinical applications. J. Lab. Clin. Med. 104, 321–330.
Oroszlam, S., McFarland, V. W., Mora, P. T., and Shear, M. J. (1966). Reversible inactivation of an endotoxin by plasma proteins. Ann. N. Y. Acad. Sci. 133, 622–628. doi: 10.1111/j.1749-6632.1966.tb52393.x
Owings, M., Chabot, C., and Watson, W. III (2019). Effects of the biomedical bleeding process on the behavior of the American horseshoe crab, Limulus polyphemus, in its natural habitat. Biol. Bull. 236, 207–223. doi: 10.1086/702917
Roslansky, P. F., and Novitsky, T. J. (1991). Sensitivity of Limulus amebocyte lysate (LAL) to LAL-reactive glucans. J. Clin. Microbiol. 29, 2477–2483. doi: 10.1128/jcm.29.11.2477-2483.1991
Rowley, D., Ali, N., and Jenkin, C. R. (1958). A reaction between fresh serum and lipopolysaccharides of gram-negative bacteria. Br. J. Exp. Pathol. 39, 90–98. doi: 10.1099/00221287-18-2-529
Rudbach, J. A., Anacker, R. L., Haskins, W. F., Johnson, A. G., Milner, K. C., and Ribi, E. (1966). Physical aspects of reversible inactivation of endotoxin. Ann. N. Y. Acad. Sci. 133, 629–643. doi: 10.1111/j.1749-6632.1966.tb52394.x
Rudloe, A. (1983). The effect of heavy bleeding on mortality of the horseshoe crab, Limulus polyphemus, in the natural environment. J. Invertebr. Pathol. 42, 167–176. doi: 10.1016/0022-2011(83)90059-9
Samuel, L. (2018). Direct detection of pathogens in bloodstream during sepsis: are we there yet? J. Appl. Lab. Med. 3, 631–642. doi: 10.1373/jalm.2018.028274
Schreibman, M. P., and Zarnoch, C. B. (2009). “Aquaculture methods and early growth of juvenile horseshoe crabs (Limulus polyphemus),” in Biology and Conservation of Horseshoe Crabs, eds J. Tanacredi, M. Botton, and D. Smith (Boston, MA: Springer), 501–511. doi: 10.1007/978-0-387-89959-6_31
Shibata, T., Kobayashi, Y., Ikeda, Y., and Kawabata, S. I. (2018). Intermolecular autocatalytic activation of serine protease zymogen factor C through an active transition state responding to lipopolysaccharide. J. Biol. Chem. 293, 11589–11599. doi: 10.1074/jbc.RA118.002311
Shuster, C. N. Jr. (1958). On Morphometric and Serological Relationships within the Limulidae, with Particular Reference to Limulus Polyphemus (L.). dissertation thesis, New York University, New York, NY.
Skarnes, R. C. (1966). The inactivation of endotoxin after interaction with certain proteins of normal serum. Ann. N. Y. Acad. Sci. 133, 644–662. doi: 10.1111/j.1749-6632.1966.tb52395.x
Skarnes, R. C. (1968). In vivo interaction of endotoxin with a plasma lipoprotein having esterase activity. J. Bacteriol. 95, 2031–2034. doi: 10.1128/jb.95.6.2031-2034.1968
Strutz, F., Heller, G., Krasemann, K., Krone, B., and Müller, G. A. (1999). Relationship of antibodies to endotoxin core to mortality in medical patients with sepsis syndrome. Intensive Care Med. 25, 435–444. doi: 10.1007/s001340050877
Stumacher, R. J., Kovnat, M. J., and McCabe, W. R. (1973). Limitations of the usefulness of the Limulus assay for endotoxin. New Engl. J. Med. 288, 1261–1264. doi: 10.1097/00003246-197305000-00022
Sullivan, J. D., and Watson, S. W. (1974). Factors affecting the sensitivity of Limulus lysate. Appl. Environ. Microbiol. 28, 1023–1026. doi: 10.1128/aem.28.6.1023-1026.1974
Timmons, M. B. (1994). Aquaculture reuse systems: engineering design and management. Dev. Aquac. Fish. Sci. 27:346.
Ulevitch, R. J., Johnston, A. R., and Weinstein, D. B. (1981). New function for high density lipoproteins: isolation and characterization of a bacterial-lipopolysaccharide-high density lipoprotein complex formed in rabbit plasma. J. Clin. Investig. 67, 827–837. doi: 10.1172/JCI110100
Wardle, N. (1979). Bacteraemic and endotoxic shock. Br. J. Hosp. Med. 21, 223–231. doi: 10.1136/jcp.33.9.888-a
Williams, K. L. (2007). Endotoxins: Pyrogens, LAL Testing and Depyrogenation. Boca Raton, FL: CRC Press.
Yoshioka, M., and Konno, S. (1984). Characteristics of endotoxin altering fractions derived from normal serum III. Isolation and properties of horse serumα 2-macroglobulin. J. Lab. Clin. Med. 104, 321–330.
Keywords: aquaculture, diagnostics, gram-negative bacteria, horseshoe crabs, Limulus amebocyte lysate, sepsis, sustainable
Citation: Tinker-Kulberg R, Dellinger K, Brady TE, Robertson L, Levy JH, Abood SK, LaDuca FM, Kepley CL and Dellinger AL (2020) Horseshoe Crab Aquaculture as a Sustainable Endotoxin Testing Source. Front. Mar. Sci. 7:153. doi: 10.3389/fmars.2020.00153
Received: 31 December 2019; Accepted: 28 February 2020;
Published: 01 April 2020.
Edited by:
Wen-Cheng Wang, National Taiwan Normal University, TaiwanReviewed by:
Bryan Raveen Nelson, Central China Normal University, ChinaAkbar John, International Islamic University Malaysia, Malaysia
Copyright © 2020 Tinker-Kulberg, Dellinger, Brady, Robertson, Levy, Abood, LaDuca, Kepley and Dellinger. This is an open-access article distributed under the terms of the Creative Commons Attribution License (CC BY). The use, distribution or reproduction in other forums is permitted, provided the original author(s) and the copyright owner(s) are credited and that the original publication in this journal is cited, in accordance with accepted academic practice. No use, distribution or reproduction is permitted which does not comply with these terms.
*Correspondence: Anthony L. Dellinger, YWRlbGxpbmdlckBrZXBsZXliaW9zeXN0ZW1zLmNvbQ==; YWRlbGxpbmdlckBnbWFpbC5jb20=