- 1Key Laboratory of Freshwater Fisheries and Germplasm Resources Utilization, Ministry of Agriculture and Rural Affairs, Freshwater Fisheries Research Center, Chinese Academy of Fishery Sciences, Wuxi, China
- 2International Joint Research Laboratory for Fish Immunopharmacology, Freshwater Fisheries Research Center, Chinese Academy of Fishery Sciences, Wuxi, China
- 3National Agricultural Research Center, Research Institute for Fisheries and Aquaculture, Szarvas, Hungary
Hepatic steatosis is the most common phenomenon of lipid metabolism disorder in farmed fish, but its molecular mechanism is poorly understood. Therefore, the present study was aimed to investigate hepatic steatosis induced by high-fat diet (HFD) and explore underlying mechanism in tilapia. The fish were fed on control diet or HFD for 90 days. The blood and liver tissues were collected to determine biochemical parameter, gene expression and protein level after 30, 60, and 90 days, and analyzed lipid accumulation, endoplasmic reticulum (ER) stress and autophagy. After 30 days of feeding, the plasmatic and hepatic lipid content (TG, TCH, LDL-C, and HDL-C) and fatty acid (FA) transportation (fabp1 and CD36) were enhanced significantly in HFD-fed tilapia. After 60 days, HFD feeding increased TG synthesis and free CH formation, and decreased FAs β-oxidation and biosynthesis in liver of tilapia. Further, with increasing lipid accumulation, ER stress was induced, which worsened hepatic steatosis via activating IRE1 signaling pathway in liver of HFD group after 90 days. Meanwhile, HFD feeding suppressed autophagy via impairing AMPK and TFEB pathways in tilapia liver after 90 days. Our results demonstrated that HFD feeding induced extensive lipid deposition, promoted ER stress, suppressed autophagy in tilapia liver. Interestingly, these pathological features were positively correlated with the duration of HFD feeding.
Introduction
Steatosis is the most common phenomenon of lipid metabolism disorder in liver of cultured fish, which results in reduction of growth, feed utilization rate, immunity, stress tolerance, etc. (Dai et al., 2019; Xu et al., 2019). Various predisposing factors for hepatic steatosis have been reported, such as nutritional imbalance, environmental stress and physiological dysfunction (Du, 2014). Among them, excessive lipid intake is a primary factor in the majority of cultured fish (Cao et al., 2019). Lipid, as a non-protein energy substitute, is essential for aquatic animal, and therefore, high-fat diet (HFD) has been frequently fed to farmed fish to provide more energy or replace partial protein (Watanabe, 1982; Boujard et al., 2004; Kim et al., 2006; Morais et al., 2007). Indeed, reasonable high fat in diet can improve the growth of fish (Vergara et al., 1999; Ghanawi et al., 2011).
In fish, the adverse effects induced by HFD have garnered much attention from researchers in recent years. Dietary high fat (15 and 16%) suppressed growth performance, reduced immune capacity and altered lipid metabolism in grass carp (Ctenopharyngodon idella) and tilapia (Oreochromis niloticus) (Ma et al., 2018; Tang et al., 2019). The lipid peroxidation and oxidative stress were caused by high dietary lipid levels (17–19.5%) in various fish species such as turbot (Scophthalmus maximus) and black seabream (Acanthopagrus schlegelii) (Jia et al., 2017; Jin et al., 2019). Moreover, HFD (11 or 12% fat) activated endoplasmic reticulum (ER) stress to mediate hepatic lipid secretion in blunt snout bream (Megalobrama amblycephala) (Cao et al., 2019), and worsen intestinal heath in tilapia (Limbu et al., 2019). Despite this, our knowledge about the underlying mechanisms of lipotoxicity induced by HFD is still very limiting.
Autophagy, a basic cellular process, is involved in the degradation of cell constituent, such as unfolded protein and damaged organelle. In the past decade, the key molecular pathways that regulate autophagy have be proposed, which consist mainly of autophagy (Atg) proteins (Martinez-Lopez and Singh, 2015). In mammalian cells, unc-51 like autophagy activating kinase 1 (ULK1) and Beclin 1 are required in initiation of autophagosome biogenesis, and Atg7, Atg5, Atg3 and LC3b (microtubule-associated protein 1 light chain-3B) are involved in autophagosome formation (Wu et al., 2018). The last phase of autophagic process is mediated by lysosomal pathway including transcription factor EB (TFEB) (Ide et al., 2011). Moreover, in response to cellular stress, AMP-activated protein kinase (AMKP) directly activates ULK1 to promote autophagy, while mammalian target of rapamycin (mTOR) suppresses autophagy by inhibiting ULK1 (Lavallard and Gual, 2014). It has been reported that HFD feeding impairs hepatic autophagic function to accelerate lipid accumulation (Tanaka et al., 2016), but activation of hepatic autophagy alleviates liver steatosis in mice (Lin et al., 2013). Unfortunately, in cultured fish, there are no published reports to investigate the relationship between autophagy and liver steatosis.
Tilapia (Oreochromis niloticus), a fast-growing, low-cost and well-studied fish species, is widely cultured in China, Asia, and Africa. In the intensive aquatic environment, it is a very common phenomenon that tilapia is overfed or fed on high-fat/high-sugar diet in order to improve growth, which may lead to frequent occurrence of fat deposition (Huang et al., 2007). Existing research has confirmed that HFD induces oxidative stress, reduces immune capacity and damages liver tissue in tilapia (Lin et al., 2018; Ma et al., 2018; Qiang et al., 2018; Xu et al., 2019). The lipid metabolic adaptation mechanisms have also evaluated in tilapia under high or low-fat diet feeding (He et al., 2015). However, the molecular mechanisms of hepatic steatosis induced by HFD are not entirely known in tilapia. Also the key molecules or signaling pathways which were involved in lipid metabolism are not well elucidated.
In this study, we evaluated possible molecular mechanism of hepatic steatosis in HFD-fed tilapia, and explored relationship between lipid accumulation and ER stress and autophagy after HFD feeding for 30, 60, and 90 days. Meanwhile, we analyzed multiple signaling pathways which mediated ER stress and autophagy including inositol-requiring enzyme 1 (IRE1), TFEB and AMPK pathways. Our results constituted novel insights into lipid accumulation induced by HFD in tilapia, which might be helpful for prevention and treatment of fatty liver injury in fish.
Materials and Methods
Ethics Statement
The experiment was performed taking into consideration the welfare of animal, and the use of fish was approved by the Freshwater Fisheries Research Centre (FFRC) of the Chinese Academy of Fishery Sciences, Wuxi, China.
Animal and Experimental Design
Juvenile tilapias (weight 35 ± 1.2 g) were provided by the farm in Freshwater Fish Research Center of Chinese Academy of Fishery Sciences (Wuxi, China). The fish were acclimated to lab condition in a recirculation system (temperature 29 ± 2°C; dissolved oxygen > 6 mg/L; pH 7.4–8.1) for 2 weeks, and fed on a control diet twice per day.
After acclimation, the fish were weighed and randomly distributed into two groups: control group and HFD group. Each group contained 40 fish tested in duplicate. The fish were fed on control diet (6% fat) or HFD (21% fat) at approximately 4% of their body weight twice per day (9:00 and 16:00) for 90 days. The formation of experimental diet is presented in Supplementary Table S1, and the diet preparation is based on previous reports (He et al., 2015; Sheng et al., 2016; Qiang et al., 2017).
After 30, 60, and 90 days of feeding, all fish were weighed and 10 fish were randomly netted from each group to get liver and blood tissues under anesthesia (100 mg/L MS-222, Sigma Diagnostics INS, St. Louis, MO, United States). The plasma was separated from blood by centrifugation (5000 rpm, 4°C, and 10 min) for analysis of blood biochemical parameters. The liver samples were flash-frozen in liquid nitrogen for measurement of enzymatic activity, gene and protein levels.
Biochemical Parameters Analysis
Plasma lipid parameters including triacylglycerol (TG), total cholesterol (TCH), free fatty acid (FFA), low density lipoprotein cholesterol (LDL-C), and high density lipoprotein cholesterol (HDL-C) were measured using commercially available kits (Nanjing Jiancheng Bioengineering Institute, Nanjing, China). The TG, TCH and free CH were also tested in liver.
Histological Evaluation
Histopathology of hepatic steatosis induced by HFD was evaluated by observing pathological section of hematoxylin and eosin (H&E) staining, Oil red O staining and transmission electron microscope (TEM). The H&E staining was performed as previously described by Jia et al. (2019). Liver tissues were fixed in Bouin’s solution for 24 h, then dehydrated with gradient alcohol and cut into thin slices (5–6 μm) after embedding in paraffin. The sections were deparaffinized, rehydrated, stained with H&E (Nanjing Jiancheng Bioengineering Institute), and examined under a light microscope. For Oil red O staining, flash-frozen liver was cut into 10–12 μm-thick sections, stained with Oil red O solution (Sigma-Aldrich) and photographed using microscope according to the method reported by Annika et al. (2013). For electron microscopy, liver samples were fixed with 2.5% glutaraldehyde solution for 2 h, posfixed with 1% osmium tetraoxide solution for 1h, and dehydrated in graded concentrations of ethanol. After dehydration, samples were embedded in epoxy resin, cut into ultra-thin sections (80 nm), and observed with Hitachi H7650 electron microscope (Hitachi High-Technologies, Japan) after staining with aqueous uranyl acetate and lead citrate (Matsumoto et al., 2010).
Fatty Acid Analysis
Liver fatty acids (FAs) were prepared as described by Chun-Yan et al. (2015) and Fan et al. (2019). Total lipids of liver were extracted with chloroform/methanol 2:1 (vol/vol) by Folch’s procedure (Folch et al., 1957). Fatty acid methyl esters were produced by incubating in 14% boron trifluoride-methanol solution at 60°C for 40 min. Methylated FAs were analyzed using gas chromatography (GC–MS; Shimadzu QP 2010 Ultra) equipped with A 100 m × 0.25 mm ID × 0.2 μm fused silica capillary column. Heneicosanoic acid (C21:0; Sigma-Aldrich) was used as the internal standard. Individual FA concentration was expressed as g/100 g.
Real-Time Quantitative PCR
Total RNA was extracted from liver using RNAiso Plus kit (Takara, Beijing, China). Complementary DNA (cDNA) was synthesized from total RNA (1 μg) by PrimeScript RT reagent Kit with gDNA Eraser (Takara). The relative expression of specific genes was detected using quantitative real-time PCR (qPCR) kit (TB Green Premix Ex Taq II, Takara). The reaction condition was as follows: one cycle 95°C for 30 s, then 40 cycles of 95°C for 5 s and 60–64°C for 1 min. Specific primers used for qPCR were listed in Supplementary Table S2. The values of genes expression were normalized by ubiquitin-conjugating enzyme (UCBE) (Chang et al., 2013), and the relative mRNA level was calculated by the 2–ΔΔCq method (Livak and Schmittgen, 2001; Bustin et al., 2009).
Western Blotting
The procedure of western blotting was performed as previous method described by Madamanchi and Runge (2001). Each protein sample from liver was subjected to SDS-PAGE gel (Beyotime) electrophoresis and transferred to polyvinylidene fluoride (PVDF) membrane (Beyotime). After blocking with QuickBlock buffer (Beyotime), the membrane was incubated with the specific primary antibody (overnight at 4°C) and secondary antibody (2 h), and then visualized using ECL reagent (Beyotime). The following primary antibodies or secondary antibody were used in this study: β-actin (Absin, abs119600), GRP78 (Proteintech, 11587-1-AP), EIF2α (Abcam, ab70542), and IgG (Beyotime, A0208).
Statistical Analysis
All data in this study are expressed as mean ± SEM (standard error of mean), calculated by SPSS software (version 20.0). Two-tail Student’s t-test was used for comparisons between control group and HFD group. P < 0.05 was considered statistically significant. Relationship between hepatic lipid content, ER stress and autophagy were analyzed based on Pearson correlation coefficient. Principal component analysis (PCA) was used to detect any correlation between experimental groups and performed by using Origin 2018 (Originlab, MA, United States). The standardized scores of the first two components which explained the highest variation were used to make biplots.
Results
Gross Phenotype and Histopathology Observation
To investigate steatosis in liver of tilapia fed on HFD, we observed liver appearance (Figures 1A,B), H&E-stained section (Figures 1C,D), oil red O-stained section (Figures 1E,F) and electron microscopic image (Figures 1G,H). As shown in Figure 1B, there was a presence of white and swollen liver in HFD group. Compared with control group, considerable steatosis was observed in liver of tilapia fed on HFD after 90 days (Figures 1C,D). The symptom of steatosis or fat accumulation was also proved by oil red O staining (Figure 1F). Electron microscopic examination further revealed the presence of lipid droplets in hepatocytes of HFD-fed tilapia (Figure 1H).
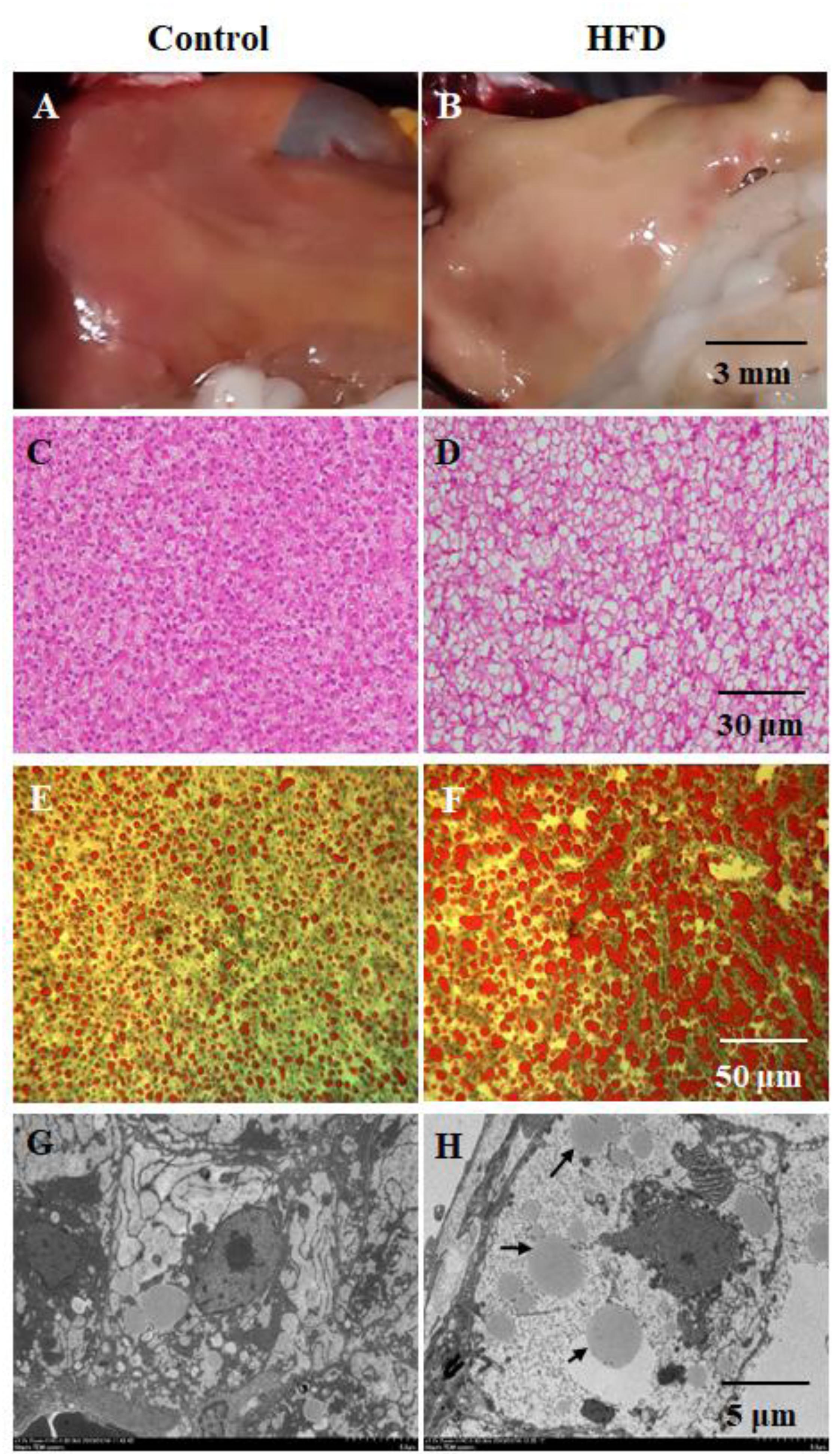
Figure 1. Gross phenotype and histopathology in tilapia fed on control diet and HFD at 90 day. (A,B) gross appearance of liver; (C,D) hematoxylin and eosin (H&E)-stained section of liver; (E,F) oil red O-stained section of liver; (G,H) electron microscopic image of liver, the black arrows indicates lipid droplets.
Lipid Content in Liver and Plasma
In plasma, the levels of TG, TCH, FFA, LDL-C, and HDL-C were significantly increased in HFD-fed fish from 30 days of feeding onward, except FFA at 30 days (Figures 2A–E). In liver, the contents of TG, free CH and TCH were also significantly increased in HFD-fed fish after 30 or 60 days of feeding (Figures 3E–G). As shown in Table 1, 28 kinds of FAs were detected in the liver of tilapia after 90 days of feeding under control diet and HFD administration. In HFD group, 14 kinds of FAs levels were higher than that in control group. Similarly, the levels of total saturated fatty acid (SFA), total monounsaturated fatty acid (MUFA), total polyunsaturated fatty acid (PUFA), n-3 PUFA, n-6 PUFA and total lipid were also clearly elevated in HFD group. Moreover, the body weight, survival, feed efficiency and LW/BW were not influenced by HFD feeding (Figures 3A–D).
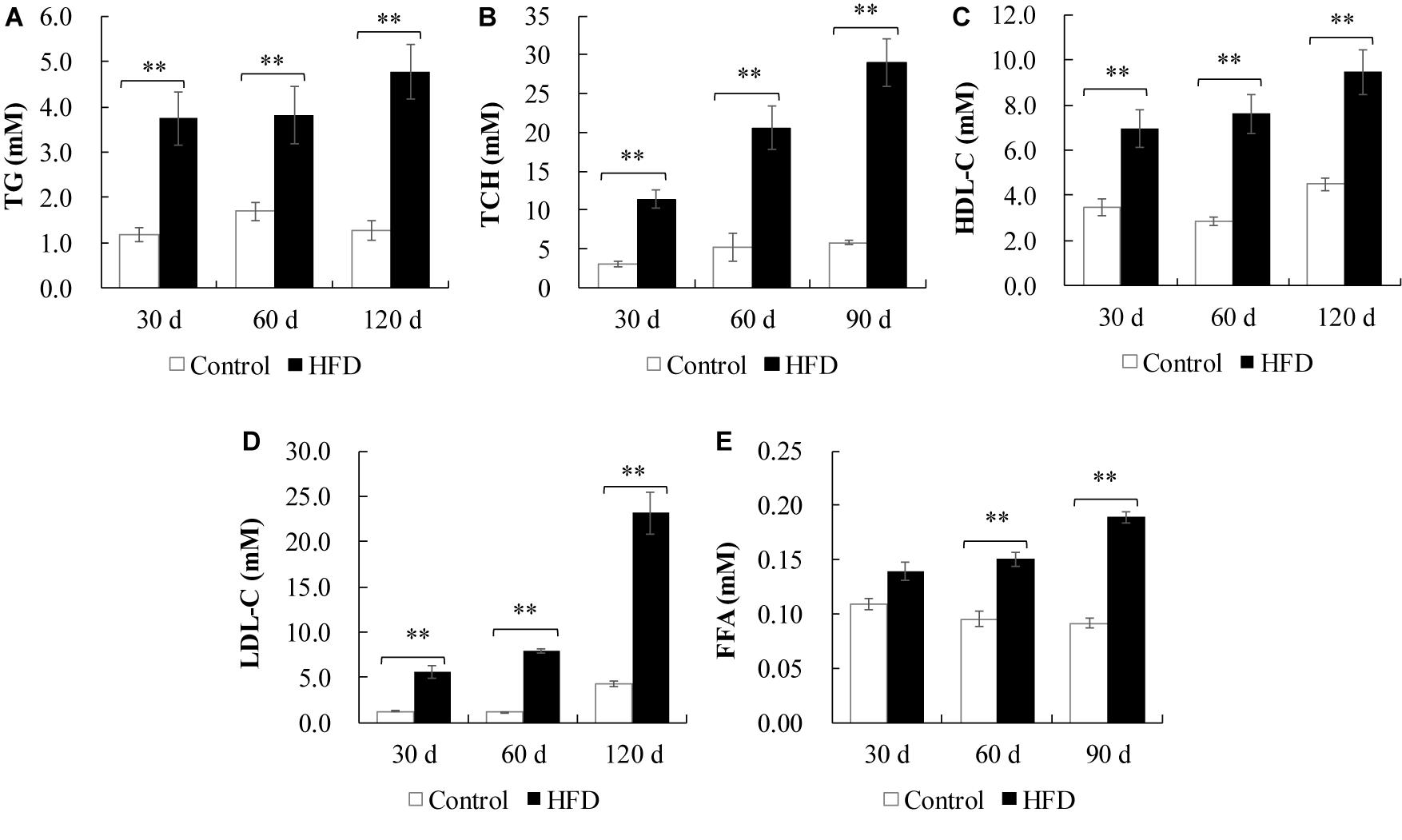
Figure 2. Plasma lipid parameters of tilapia fed on control diet and HFD for 90 days. (A) TG content; (B) TCH content; (C) HDL-C content; (D) LDL-C content; (E) FFA content. All values are expressed as mean ± SEM (n = 10); ∗∗P < 0.01 versus control at same time.
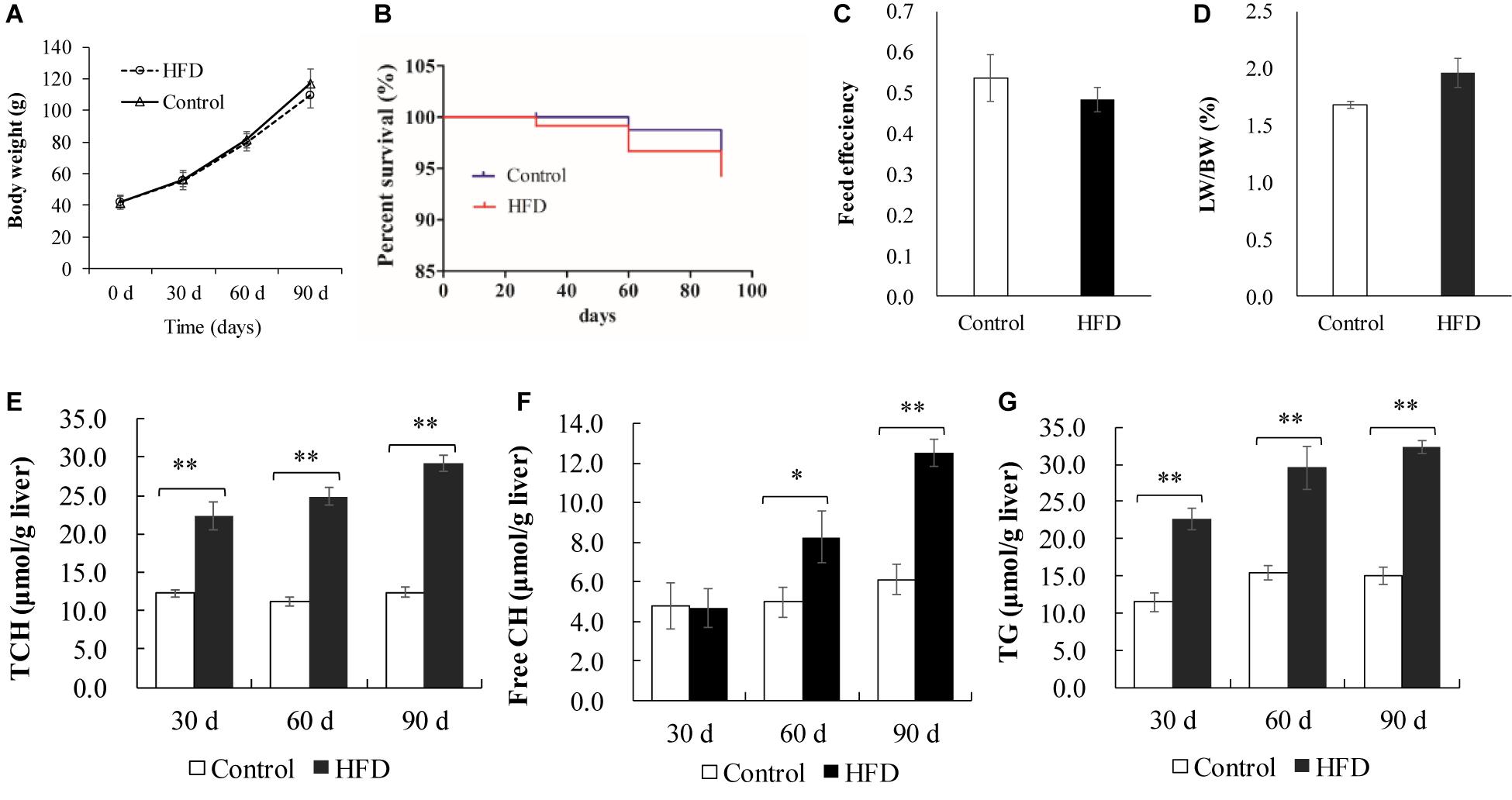
Figure 3. Growth performance and liver lipid content in control diet and HFD-fed tilapia. (A) Body weight and (B) survival of tilapia were measured every 30 days (n = 40–23). (C) Feed efficiency and (D) LW/BW were measured at 90 days (n = 20). (E–G) Changes of TCH, free CH and TG in liver (n = 10). All values are expressed as mean ± SEM. ∗P < 0.05 and ∗∗P < 0.01 versus control at same time. Feed efficiency = wet weight gain/dry feed intake; LW, liver weight; BW, body weight.
Fatty Acid Metabolism in Liver
To examine the effects of HFD on lipid metabolism in tilapia liver, we assessed the expression of lipid metabolism-related genes including hepatic TG metabolism, and FA uptake, synthesis and β-oxidation (Figure 4). HFD feeding enhanced mRNA levels of fatty acid binding protein 1 (fabp1) and cluster of differentiation 36 (CD36), which promoted FAs uptake in liver after 30 days and subsequent feeding (Figure 4A). Whereas the lower mRNA levels of lipoprotein (lpl) and low density lipoprotein receptor (ldlr) showed an inhibition in TG and FAs influx after 90 days of feeding (Figure 4A). The de novo synthesis of hepatic FAs was blocked in HFD group, where the mRNA levels of acetyl-CoA carboxylase (accα), FA synthase (fasn) and peroxisome proliferator-activated receptor γ (ppar-γ) were markedly downregulated after 30 or 60 days (Figure 4B). The data from Figure 4C revealed that TG synthesis genes including glycerol-3-phosphate acyltransferase 1 (gpat1), diacylglycerol acyltransferase 1 (dgat1) and dgat2 were obviously upregulated in liver of fish fed on HFD after 60 or 90 days. However, TG hydrolysis genes including adipose triglyceride lipase (atgl) and hormone sensitive lipase (hsl) were clearly downregulated in HFD-fed tilapia after 60 or 90 days (Figure 4C). HFD inhibited the expression of FA β-oxidation genes including ppar-α, carnitine palmitoyltransferase 1 (cpt-1), acyl-CoA oxidase (acox-1) in liver of fish after 60 and 90 days (Figure 4D). Moreover, HFD administration suppressed VLDL assembly via downregulating the genes expression of apolipoprotein (apoB-100) and microsomal triglyceride transfer protein (mttp) (Figure 4E). In addition, the genes related lipid droplet formation were not changed by HFD feeding (Figure 4F), despite abundant lipid droplets were observed in HFD-fed tilapia (Figure 1H). These data indicated that the fatty acid metabolism was disturbed in liver of HFD-fed tilapia.
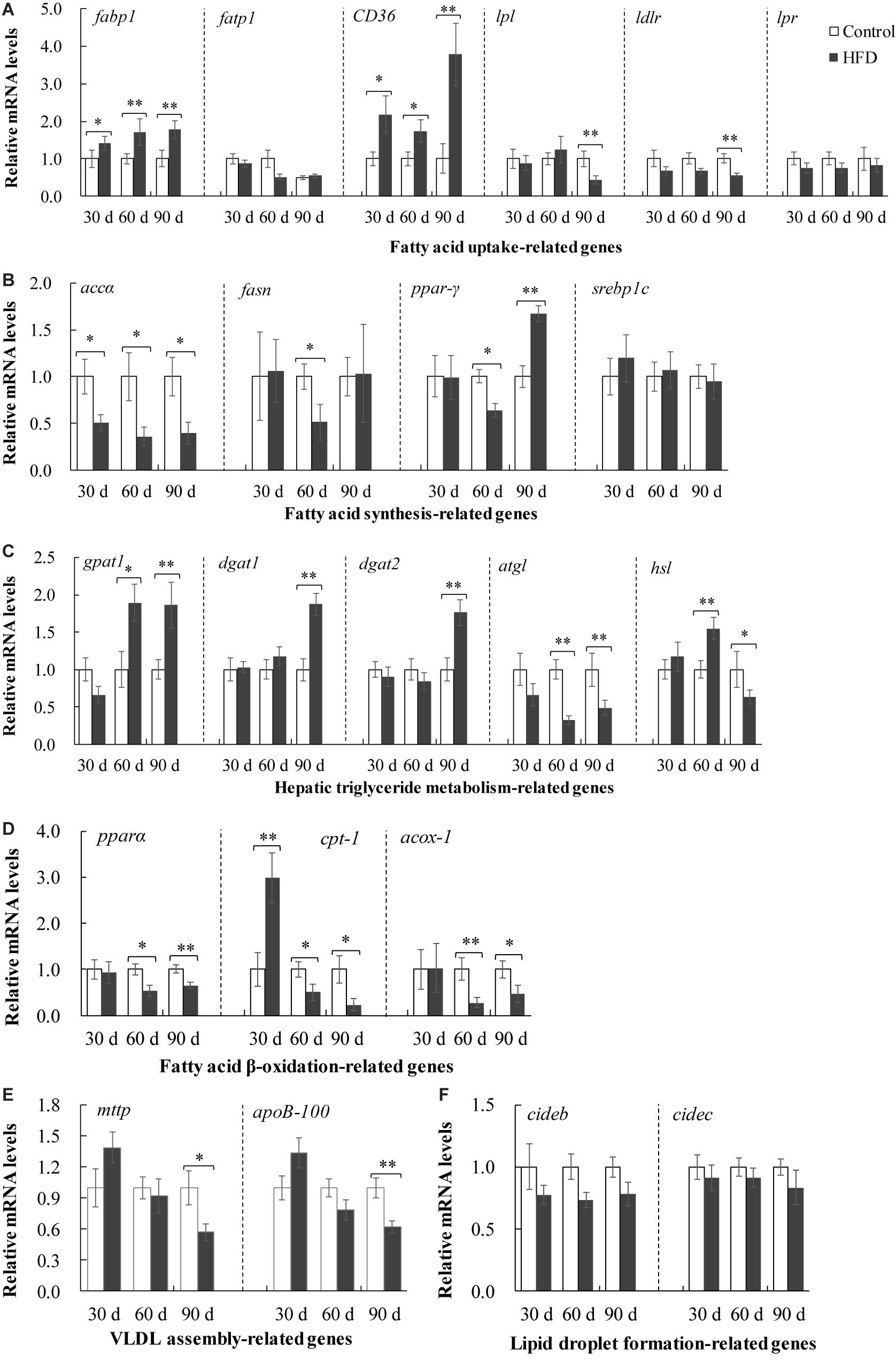
Figure 4. Expression of fatty acids metilapia fed on control diet and HFD for 90 days. (A) Expression of fatty acid uptake-related genes including fabp1, fatp1, CD 36, lpl, ldlr and lpr; (B) expression of fatty acid synthesis-related genes including accα, fasn, ppar-γ and srebp1c; (C) expression of hepatic triglyceride metabolism-related genes including gpat1, dgat1, dgat2, atgl and hsl; (D) expression of fatty acid β-oxidation-related genes including pparα, cpt-1 and acox-1; (E) expression of VLDL assembly-related genes including mttp and ApoB100; (F) expression of lipid droplet formation-related genes including cideb and cidec. The values are normalized to control values and expressed as means ± SEM (n = 10); ∗P < 0.05 and ∗∗P < 0.01 versus control at same time.
Cholesterol Metabolism in Liver
In FHD-treated tilapia, the cholesterol synthesis and uptake in liver were inhibited, as validated by lower mRNA levels of hepatic lipase (lipc), 3-Hydroxy-3-methylglutaryl-CoA reductase (hmgcr) and sterol regulatory element binding protein 2 (srebp-2) after 30, 60, or 90 days (Figure 5A). The data of cholesterol efflux showed a conflicting results (Figure 5B). HFD feeding upregulated the expression of sterol 7a hydroxylase (cyp7a1), but downregulated the expression of acetyl-co-enzyme A acetyltransferase 2 (acat2) and apolipoprotein A1 (apoa1) in the liver after 30, 60, or 90 days. Moreover, the liver X receptor (LXR) and farnesoid X receptor (FXR) were not altered by HFD administration (Figure 5C). These data indicated that HFD induced dysregulation of cholesterol metabolism in liver.
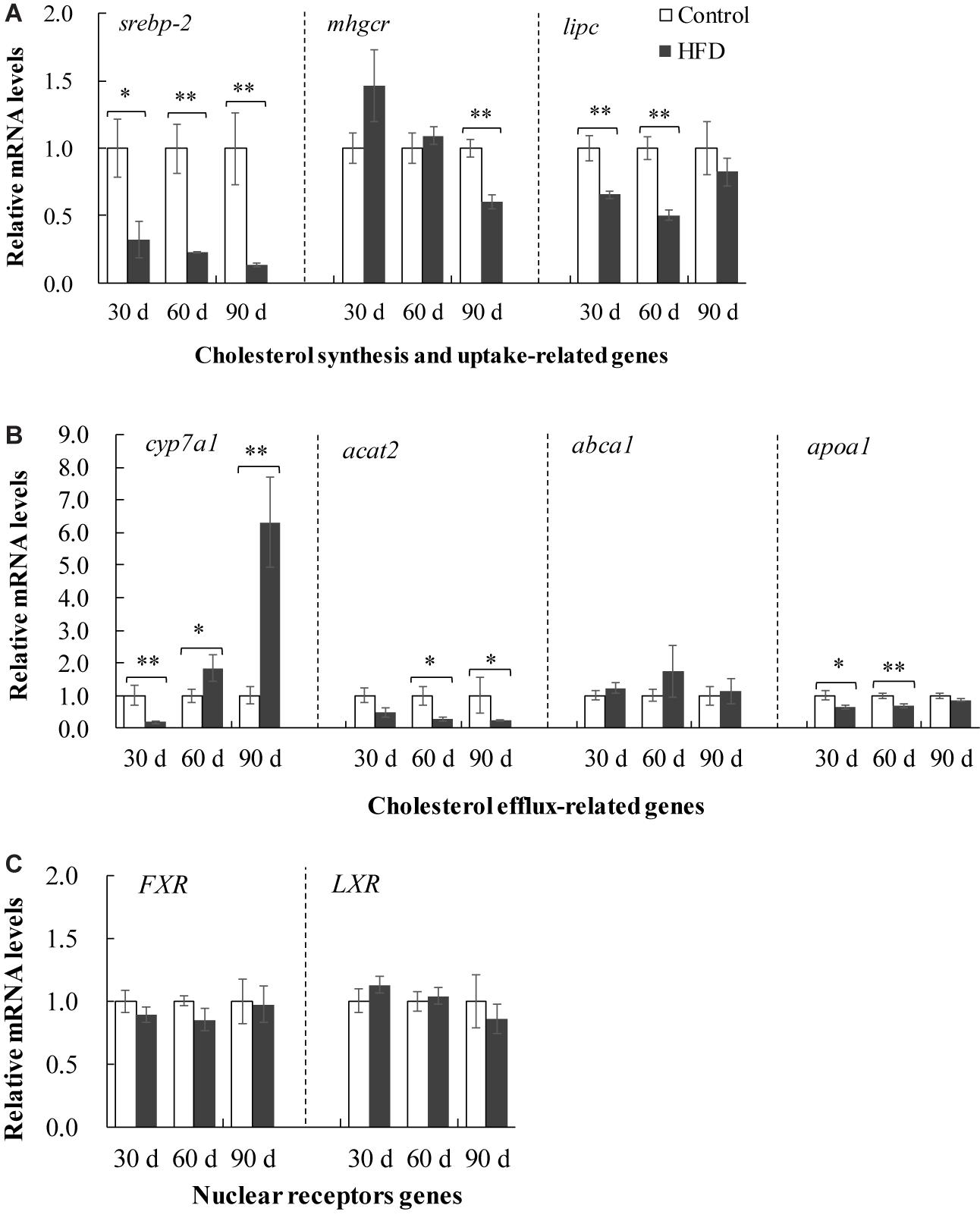
Figure 5. Expression of cholesterol metabolism-related genes in liver of tilapia fed on control diet and HFD for 90 days. (A) Expression of cholesterol synthesis and uptake-related genes including srebp-2, mhgcr and lipc; (B) expression of cholesterol efflux-related genes including cyp7a1, acat2, abca1 and apoa1; (C) expression of nuclear receptors genes including FXR and LXR. The values are normalized to control values and expressed as means ± SEM (n = 10); ∗P < 0.05 and ∗∗P < 0.01 versus control at same time.
Endoplasmic Reticulum Stress
ER stress participates in multiple physiological process including lipid metabolism (Kaplowitz et al., 2007), and thus, we detected several ER indicators. The mRNA levels of ire1, 78 kDa glucose-regulated protein (grp78), spliced X box binding protein 1 (xbp1s) and C/EBP-homologous protein (chop) were increased in the liver of HFD-fed tilapia after 90 days (Figures 6A–D). Similarly, the GRP78 protein expression was upregulated in the liver of HFD-fed tilapia after 90 days (Figure 6J). In addition, the mRNA levels of grp78, chop and calreticulin (crt) were also increased by FHD feeding after 30 or 60 days, whereas, the crt mRNA level was decreased by FHD feeding after 90 days (Figure 6E). Moreover, the mRNA levels of perk, eif2α, atf4 and atf6 were not influenced by HFD feeding (Figures 6F–I).
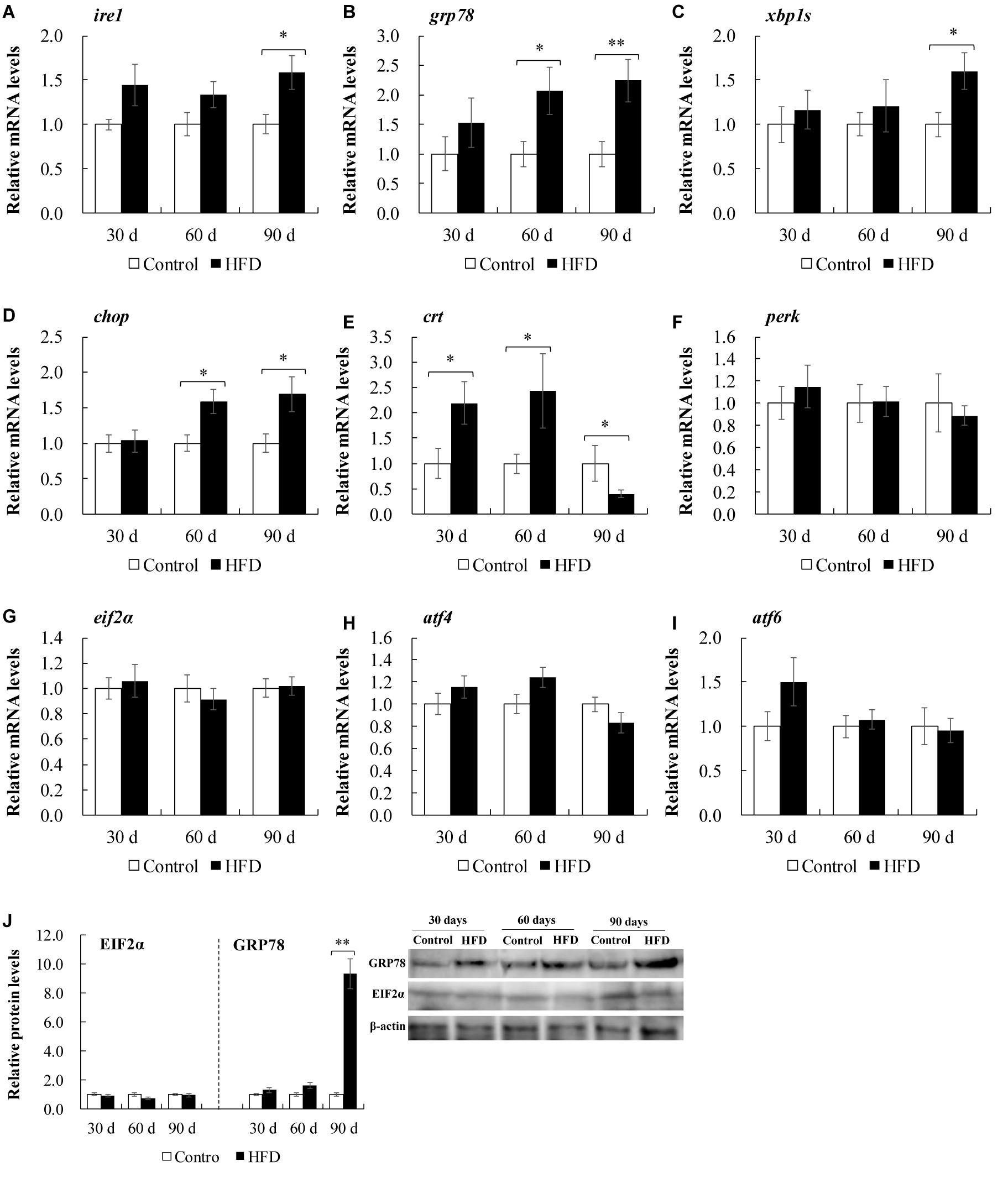
Figure 6. Expression of endoplasmic reticulum stress-related genes or proteins in liver of tilapia fed on control diet and HFD for 90 days. (A–I) Expression of endoplasmic reticulum stress-related genes (n = 10). (J) Representative immunoblotting and relative protein expression of GRP78 and EIF2α (n = 3). The values are normalized to control values and expressed as means ± SEM; ∗P < 0.05 and ∗∗P < 0.01 versus control at same time.
Autophagy
Lysosome-mediated autophagy plays an important role in hepatic physiology and pathogenesis of fatty liver. Compared with control group, HFD feeding caused a marked downregulation of tfeb, and its target genes including UV radiation resistance associated gene (uvrag) and mucolipin-1(mcoln-1) after 90 days (Figure 7A). Meanwhile, the data from Figures 7B,C revealed that the mRNA levels of ampk, becin1, lc3b, p62, atg7, and atg3 were clearly restrained in HFD-fed tilapia after 90 days of feeding, indicating that autophagy was suppressed by HFD treatment in liver of tilapia.
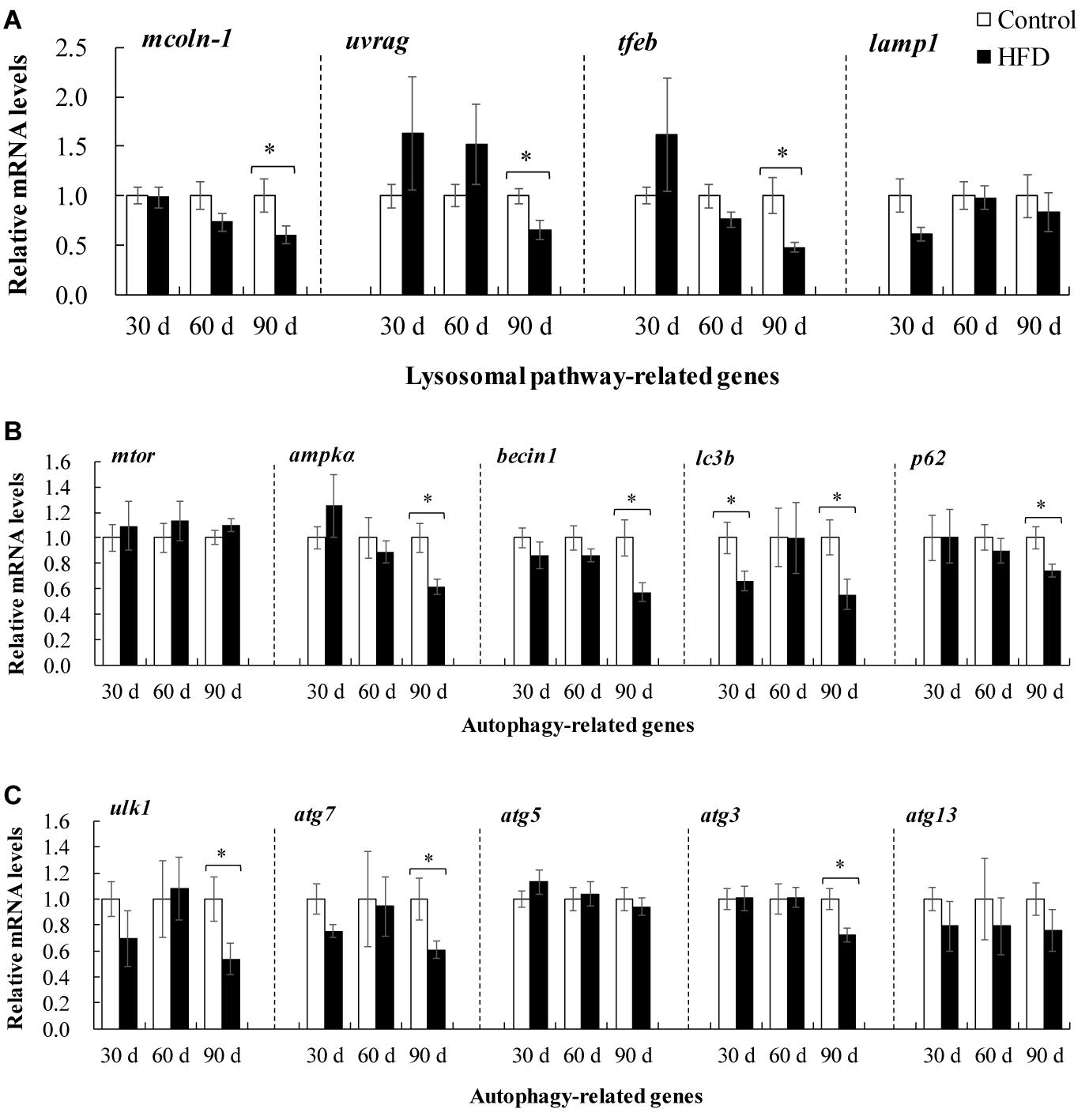
Figure 7. Expression of autophagy-related genes in liver of tilapia fed on control diet and HFD for 90 days. (A) Expression of lysosomal pathway-related genes including mcoln-1, uvrag, tfeb, and lamp1; (B,C) expression of autophagy-related genes mTOR, ampkα, becin1, lc3b, p62, ulk1, atg7, atg5, atg3 and atg13. The values are normalized to control values and expressed as means ± SEM (n = 10); ∗P < 0.05 versus control at same time.
Correlation Analysis and Principal Component Analysis (PCA)
Correlation analysis showed that hepatic TG accumulation was positively related to IRE1-XBP1s pathways, but negatively related to AMPK and TFEB pathways (Figure 8A). Meanwhile, there was a significant correlation between TCH and AMPK and TFEB pathways (Figure 8A). PCA showed the pathological changes induced by HFD were connected with the duration of feeding, and the adverse effects were more serious in tilapia fed on HFD for 90 days (Figure 8B).
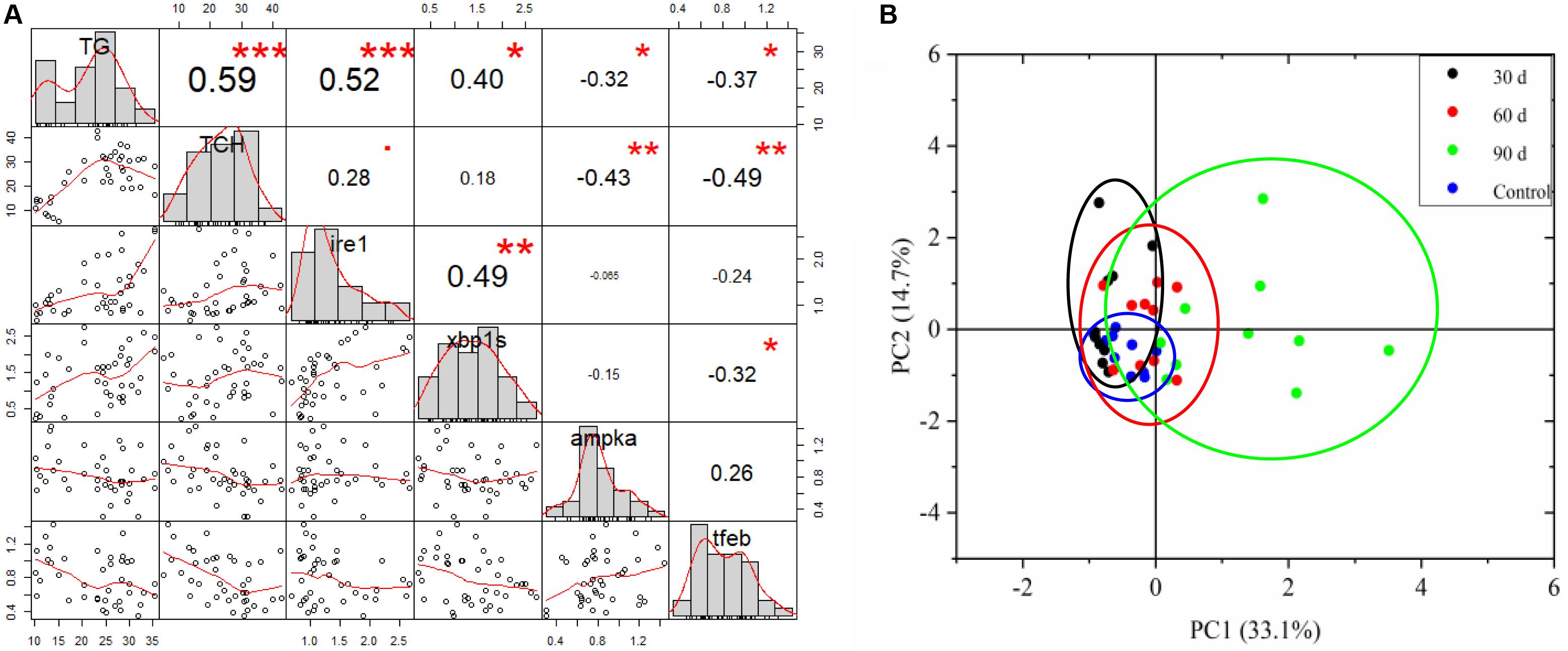
Figure 8. Correlation analysis and principal component analysis (PCA). (A) Relationship between hepatic lipid content and ER stress and autophagy, hepatic lipid mainly consisted of TG and TCH. (B) PCA score plot for all markers measured in tilapia fed control diet and HFD after 30, 60, and 90 days.
Discussion
Effects of HFD on Lipid Metabolism in Tilapia
Liver is the central organ that regulates the uptake, synthesis, secretion, catabolism and storage of lipid. The dysregulation of FAs and TG is a major reason of steatosis (Day and James, 1998; Bass and Merriman, 2005). This phenomenon was observed in the present study, where HFD feeding induced lipid deposits and steatosis in tilapia liver. One of the reasons for steatosis is excessive uptake of FAs from plasma (Bradbury, 2006). In this study, lipid content such as TG and FFA was raised in plasma after HFD feeding, which promoted FAs uptake into liver (Bass and Merriman, 2005). Meanwhile, HFD enhanced the genes expression of transporter proteins, such as FABP1, CD36 and FATP1 in liver, accelerating FAs influx into liver (Lu et al., 2014; An et al., 2017).
Another reason for steatosis is lower FA β-oxidation. In tilapia, our data displayed that HFD feeding downregulated genes expression of β-oxidation rate-limiting enzymes (CPT-1and ACOX-1), and pparα (a key regulator of β-oxidation) in the liver. Similar results were described in FHD-fed medaka (Oryzias latipes) and blunt snout bream (Matsumoto et al., 2010; Lu et al., 2014, 2017). Conversely, HFD feeding increased FA β-oxidation in zebrafish and grass carp (Dai et al., 2015; Li et al., 2016). Also, in human non-alcoholic fatty liver disease (NAFLD), the mixing results were reported (Reviewed by Kawano and Cohen, 2013). The potential reasons for these conflicting results are still unclear. It is possible that increased β-oxidation is an adaptive protection against FA-mediated hepatotoxicity, whereas, decreased β-oxidation shows a dysregulation of hepatic FAs, which accelerates lipid accumulation, and induces liver injury.
De novo lipogenesis is a major source of FA in hepatocyte, which is regulated by two rate-liming enzymes ACC and FASN. In rat and human liver, HFD boosted the FA de novo synthesis via upregulating ACC and FASN, and accelerated occurrence of NAFLD (Matsumoto et al., 2010; Tanaka et al., 2016; An et al., 2017). The upregulated acc and fasn were also observed in HFD fed medaka (Matsumoto et al., 2010). Nevertheless, in tilapia liver, we found that the de novo synthesis of FAs were limited by HFD feeding via downregulating accα and fasn genes expression, which was consistent with the results discovered in HFD-fed zebrafish (Dai et al., 2015). It is possible an adaptive protection mechanism against FAs accumulation in liver.
In liver, FAs can synthesize TG for storage in the form of lipid droplets or export in combination with VLDL. The high hepatic TG content occurred more frequently in HFD-induced NAFLD model (Dai et al., 2015; An et al., 2017). Here, we also provided evidence that HFD feeding increased TG content in tilapia liver, which is related to the dysregulation of hepatic TG. In HFD-treated tilapia, the mRNA levels of gpat1, dagat1, and dgat2 (three key enzymes for TG synthesis) were upregulated, but the mRNA levels atgl and hsl (two rate-limiting enzymes for TG lipolysis) were downregulated in liver. Meanwhile, the genes expression of mttp and ApoB100 (two key regulators of VLDL assembly) was obviously restrained by HFD in tilapia liver. These data suggested that HFD feeding promoted TG synthesis, inhibited TG lipolysis, and blocked TG export in tilapia liver, which may be the third reason of excessive lipid deposition and steatosis in tilapia liver (Buzzetti et al., 2016). Altogether, the FAs and TG overload in tilapia liver mainly resulted from increased FAs uptake, impaired FAs β-oxidation and blocked VLDL assembly under HFD feeding.
Apart from FAs and TG, the CH, more specifically, free CH is also a major lipotoxic molecule in the fatty liver injury. Hepatic free CH accumulation can induce ER stress, mitochondrial dysfunction, oxidative damage, inflammation and liver injury (Reviewed by Musso et al., 2013). Excess dietary fat or/and CH has been shown to cause high free CH content in liver, as well as steatosis, NAFLD and liver injury in different animal models (Subramanian et al., 2011; Savard et al., 2013; Dai et al., 2015; An et al., 2017). Our results were consistent with previous studies revealing higher hepatic CH content in HFD-fed tilapia.
In hepatocytes, CH homeostasis is maintained through a complex pathway including cholesterol uptake, de novo synthesis and efflux. Uptake from plasma lipoproteins is a source of hepatic CH, which is mediated by several membrane receptors including LDLR, and the scavenger receptor class B type I (SCARB1). In this study, decreased ldlr gene expression signified suppression of CH uptake from plasma in HFD-fed tilapia, which was supported by previous reports in mice (Savard et al., 2013). In addition, new production of CH, regulated by HMGCR and SREBP-2, is another source of hepatic CH. It is a potential reason for hepatic free CH accumulation in setting of NAFLD (Caballero et al., 2009; Min et al., 2012). However, some investigation observed opposite results that the genes expression of hmgcr and srebp-2 was downregulated and hepatic CH synthesis was limited in HFD-induced NAFLD models (Savard et al., 2013; Dai et al., 2015). In HFD-fed tilapia, we also found the decreased genes expression of hmgcr and srebp-2 in liver, indicating a limited hepatic CH synthesis. The inhibition of CH synthesis and uptake in HFD-fed tilapia liver may be an adaptive adjustment.
Hepatic CH efflux is associated with multiple pathways, including conversion to bile acids via catalysis of CYP7A1, export incorporation into VLDL, and secretion combination with nascent HDL particles with the assistance of Apoa1 (Ioannou, 2016). Our study discovered that upregulation of cyp7a1 gene expression promoted CH conversion to bile acids, but downregulation of acat2, apoa1, mttp, and apoB-100 genes expression restrained CH efflux in the liver of HFD-fed tilapia. Thus we speculated that the accumulation of hepatic CH mainly resulted from impaired VLDL assembly and HLD particles formation in HFD-fed tilapia liver. Our data partially confirmed early reports in NAFLD models (Min et al., 2012; Savard et al., 2013).
Effects of HFD on ER Stress in Tilapia
The ER is pivotal organelle with major function in hepatic lipid metabolism including lipid synthesis, storage and export. Unfolded protein response (UPR), a highly conserved pathway in ER, monitors the status of ER protein assembly and lipid metabolism, and serves to restore ER homeostasis (Gentile et al., 2011). It starts with the activation of three ER-localized proteins (IRE1, ATF6 and PERK), following the release of the chaperone GRP78 (Cnop et al., 2012). IRE1 activation promotes the XBP1s mRNA and subsequent transcription of molecular chaperones (e.g., CHOP). Activation of PERK causes phosphorylation of EIF2α, and upregulates ATF4 and molecular chaperones. Activation of ATF6 leads to its release from ER membrane and enters nuclear to target molecular chaperones. Notably, in mice and human, the lipid overload induced ER stress in liver, which led to chronic UPR, and then resulted in oxidative stress, apoptosis and inflammation (Urra et al., 2013). ER stress also promoted lipid accumulation and steatosis via inhibiting FAs β-oxidation in liver (Lebeaupin et al., 2018). In HFD-fed fish liver, IRE1-XBP1 pathway-mediated ER stress and ATF6 regulated ER dysfunction were observed, which brought about abnormal lipid metabolism (Dai et al., 2015; Cao et al., 2019). In this study, the upregulated mRNA levels of ire1, grp78, xbp1s, and chop, and protein level of GRP78 indicated that ER stress was a consequence of lipotoxicity induced by HFD in tilapia. As such, we surmised that ER stress participated in lipid accumulation and further fatty liver injury in tilapia via activating IRE1 pathway.
Effects of HFD on Autophagy in Tilapia
Autophagy plays important roles in regulation of hepatic lipid metabolism. In hepatocyte, LDs can be engulfed to form autophagosomes, and then generate autolysosomes by fusing to lysosome (Singh et al., 2009). Autolysosomes are hydrolyzed by lysosomal enzymes, and lipid products are released into cytosol to participate in other cellular process (Martinez-Lopez and Singh, 2015). In dietary models of NAFLD, autophagy was suppressed in liver, which accelerated hepatic steatosis and aggravated liver injury in NAFLD (Wu et al., 2018). Here, our results also revealed that HFD feeding impeded autophagy, which might be a mechanism of lipid accumulation and lipotoxicity induced by HFD in tilapia.
TFEB is a master regulator in lysosome biogenesis and function. Impaired TFEB inhibited lysosome biogenesis and autophagy, which deteriorated liver injury and steatosis (Chao et al., 2018). In HFD-induced mice hepatic steatosis, tfeb and its target genes (lamp1, mcoln, and uvrag) involved in lysosomal pathway were downregulated (Su et al., 2018). The similar results were also seen in HFD-fed tilapia liver. These data suggested that HFD feeding inhibited TFEB-mediated lysosomal pathway, and then suppressed autophagy in HFD-fed tilapia.
Autophagy can be regulated through different pathways including the classic AMPK pathway. Activation of AMPK induced autophagy to attenuate HFD-induced liver steatosis, but inhibition of AMPK abolished the improvement of liver steatosis and autophagy activation (Ren et al., 2019). In NAFLD mice, AMPK pathway was interfered by HFD treatment and affected the development of fatty liver injury (Qiang et al., 2016; Chen et al., 2019). These evidence was further confirmed in HFD-fed tilapia, where the mRNA levels of ampkα and ulk1 were significantly declined. Thus we speculated AMPK mediated autophagy via directly phosphorylating ULK1 in the liver of tilapia after HFD feeding, which accelerated the lipid accumulation and lipotoxicity.
Conclusion
In summary, the current study demonstrated that HFD feeding could induce steatosis via increasing FAs uptake, impairing FAs β-oxidation, blocking VLDL assembly and enhancing HDL particles formation (Figure 9). Meanwhile, HFD feeding induced ER stress via IRE1 pathway and suppressed autophagy via TFEB and AMPK pathways to worsen lipotoxicity in liver (Figure 9).
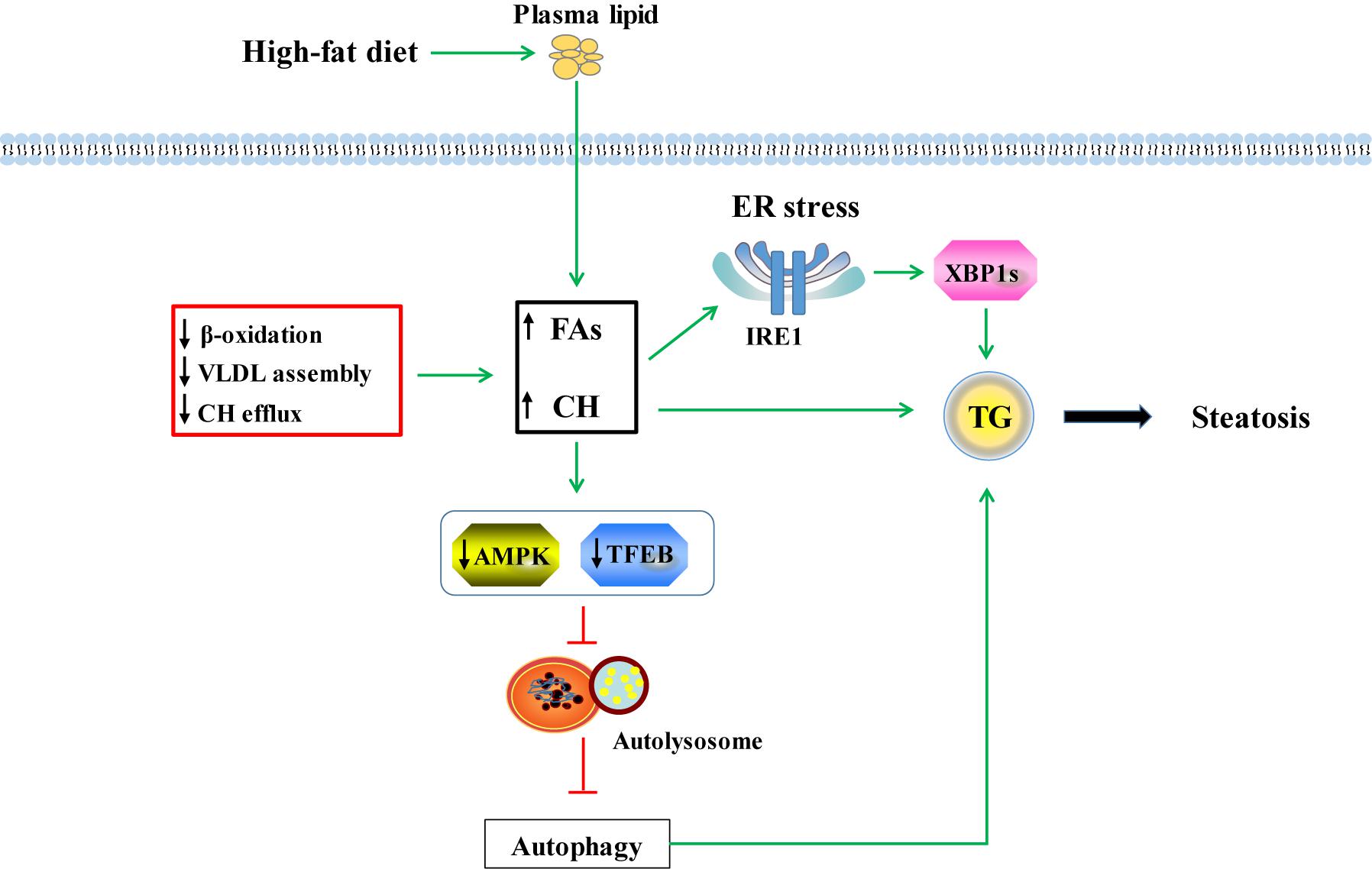
Figure 9. Possible mechanisms of lipotoxicity induced by HFD in tilapia. HFD feeding increased fatty acids (FAs) and free CH (cholesterol) via uptake from plasma. Meanwhile, suppression of FAs β-oxidation, VLDL assembly and CH efflux induced by HFD feeding also enhanced the hepatic FAs and CH content. Increased FAs and CH promoted TG formation which induced hepatic steatosis. Excessive FFA and free CH could cause ER stress and further accelerated steatosis by IRE1-XBP1s signaling pathway. In HFD-induced fatty liver, the decreased AMPK pathway inhibited formation of UKL1 and Atg13 complex, hampered autophagy proteins expression, and ultimately resulted in low autophagy. Moreover, the low autophagy was related to depressed lysosomal biogenesis mediated by TFEB pathway. Suppressed autophagy blocked decomposition of lipid droplets and worsened steatosis.
Data Availability Statement
All datasets generated for this study are included in the article/Supplementary Material.
Ethics Statement
The animal study was reviewed and approved by Freshwater Fisheries Research Centre (FFRC) of the Chinese Academy of Fishery Sciences.
Author Contributions
RJ designed the study and wrote the manuscript. L-PC, and J-LD provided technical assistance and analyzed the data for the study. QH and Z-YG conducted the experiments. GJ corrected writing and grammar mistakes. PX and G-JY directed the project and edited the manuscript. All authors read and approved the final version of the manuscript.
Funding
This work was supported by Jiangsu Provincial Natural Science Foundation of China (NO. BK20170218); National Natural Science Foundation of China (NO. 31702318); and by Central Public-interest Scientific Institution Basal Research Fund, Freshwater Fisheries Research Center, CAFS (2019JBFM10, 11).
Conflict of Interest
The authors declare that the research was conducted in the absence of any commercial or financial relationships that could be construed as a potential conflict of interest.
Supplementary Material
The Supplementary Material for this article can be found online at: https://www.frontiersin.org/articles/10.3389/fmars.2020.00363/full#supplementary-material
Abbreviations
ACAT2, acetyl-co-enzyme A acetyltransferase 2; ACC A, acetyl-CoA carboxylase; ACOX-1, acyl-CoA oxidase; AMKP, AMP-activated protein kinase; Apoa1, apolipoprotein A1; ApoB-100, apolipoprotein; ATF6, AMP-dependent transcription factor 6; Atg, autophagy; ATGL, adipose triglyceride lipase; CD36, cluster of differentiation 36; CHOP, C/EBP-homologous protein; CPT-1, carnitine palmitoyltransferase 1; CYP7a1, sterol 7a hydroxylase; DGAT1, diacylglycerol acyltransferase 1; EIF2 α, eukaryotic translation initiation factor 2 α; ER, endoplasmic reticulum; FABP1, fatty acid binding protein 1; FAs, fatty acids; FASN, FA synthase; FFA, free fatty acid; FXR, farnesoid X receptor; GPAT1, glycerol-3-phosphate acyltransferase 1; GRP78, 78 kDa glucose-regulated protein; H&E, hematoxylin and eosin; HDL-C, high density lipoprotein cholesterol; HFD, high-fat diet; HMGCR, 3-Hydroxy-3-methylglutaryl-CoA reductase; HSL, hormone sensitive lipase; IRE1, including inositol-requiring enzyme 1; LC3b, microtubule-associated protein 1 light chain-3B; LDL-C, low density lipoprotein cholesterol; LDLR, low density lipoprotein receptor; Lipc, hepatic lipase; LPL, lipoprotein; LXR, liver X receptor; Mcoln-1, mucolipin-1; mTOR, mammalian target of rapamycin; MTTP, microsomal triglyceride transfer protein; MUFA, monounsaturated fatty acid; NAFLD, non-alcoholic fatty liver disease; PERK, protein kinase R (PKR)-like endoplasmic reticulum kinase; PPAR- γ, peroxisome proliferator-activated receptor γ; PUFA, polyunsaturated fatty acid; PVDF, polyvinylidene; SFA, fluoride, saturated fatty acid; SREBP-2, sterol regulatory element binding protein 2; TCH, total cholesterol; TEM, transmission electron microscope; TFEB, transcription factor EB; TG, triacylglycerol; UCBE, ubiquitin-conjugating enzyme; ULK1, unc-51 like autophagy activating kinase 1; Uvrag, UV radiation resistance associated gene; VLDL, very low-density lipoproteins; XBP1s, spliced X box binding protein 1.
References
An, S., Zhao, L. P., Shen, L. J., Wang, S., Zhang, K., Qi, Y., et al. (2017). USP18 protects against hepatic steatosis and insulin resistance through its deubiquitinating activity. Hepatology 66, 1866–1884. doi: 10.1002/hep.29375
Annika, M., Hagberg, C. E., Lars, M., Ulf, E., and Annelie, F. (2013). Imaging of neutral lipids by oil red O for analyzing the metabolic status in health and disease. Nat. Protoc. 8, 1149–1154. doi: 10.1038/nprot.2013.055
Bass, N. M., and Merriman, R. B. (2005). “Fatty Acid Metabolism and Lipotoxicity in the Pathogenesis of NAFLD/NASH,” in Fatty Liver Disease, eds G. C. Farrell, J. George, P.d.l.M. Hall, and A. J. McCullough (Oxford: Blackwell Publishing Ltd), 109–122.
Boujard, T., Gélineau, A., Covès, D., Corraze, G., Dutto, G., Gasset, E., et al. (2004). Regulation of feed intake, growth, nutrient and energy utilisation in European sea bass (Dicentrarchus labrax) fed high fat diets. Aquaculture 231, 529–545.
Bradbury, M. W. (2006). Lipid metabolism and liver inflammation. I. Hepatic fatty acid uptake: possible role in steatosis. Am. J. Physiol. Gastrointest. Liver Physiol. 290, G194–G198.
Bustin, S. A., Benes, V., Garson, J. A., Hellemans, J., Huggett, J., Kubista, M., et al. (2009). The MIQE guidelines: minimum information for publication of quantitative real-time PCR experiments. Clin. Chem. 55, 611–622. doi: 10.1373/clinchem.2008.112797
Buzzetti, E., Pinzani, M., and Tsochatzis, E. A. (2016). The multiple-hit pathogenesis of non-alcoholic fatty liver disease (NAFLD). Metabolism 65, 1038–1048. doi: 10.1016/j.metabol.2015.12.012
Caballero, F., Fernandez, A., De Lacy, A. M., Fernandez-Checa, J. C., Caballeria, J., and Garcia-Ruiz, C. (2009). Enhanced free cholesterol, SREBP-2 and StAR expression in human NASH. J. Hepatol. 50, 789–796. doi: 10.1016/j.jhep.2008.12.016
Cao, X.-F., Dai, Y.-J., Liu, M.-Y., Yuan, X.-Y., Wang, C.-C., Huang, Y.-Y., et al. (2019). High-fat diet induces aberrant hepatic lipid secretion in blunt snout bream by activating endoplasmic reticulum stress-associated IRE1/XBP1 pathway. Biochim. Biophys. Acta 1864, 213–223. doi: 10.1016/j.bbalip.2018.12.005
Chang, G. Y., Xian, L. W., Tian, J., Wei, L., Fan, W., Ming, J., et al. (2013). Evaluation of reference genes for quantitative real-time RT-PCR analysis of gene expression in Nile tilapia (Oreochromis niloticus). Gene 527, 183–192. doi: 10.1016/j.gene.2013.06.013
Chao, X., Wang, S., Zhao, K., Li, Y., Williams, J. A., Li, T., et al. (2018). Impaired TFEB-mediated lysosome biogenesis and autophagy promote chronic ethanol-induced liver injury and steatosis in mice. Gastroenterology 155, 865–879.e12. doi: 10.1053/j.gastro.2018.05.027
Chen, X. Y., Cai, C. Z., Yu, M. L., Feng, Z. M., Zhang, Y. W., Liu, P. H., et al. (2019). LB100 ameliorates nonalcoholic fatty liver disease via the AMPK/Sirt1 pathway. World J. Gastroenterol. 25, 6607–6618. doi: 10.3748/wjg.v25.i45.6607
Chun-Yan, L. I., Zhang, X. Y., Zhang, Y. H., and Shang, X. U. (2015). Determination of fatty acids in mice serum and liver by optimized gas chromatography. Chin. J. Vet. Med. 51, 33–38.
Cnop, M., Foufelle, F., and Velloso, L. A. (2012). Endoplasmic reticulum stress, obesity and diabetes. Trends Mol. Med. 18, 59–68. doi: 10.1016/j.molmed.2011.07.010
Dai, W., Wang, K., Zheng, X., Chen, X., Zhang, W., Zhang, Y., et al. (2015). High fat plus high cholesterol diet lead to hepatic steatosis in zebrafish larvae: a novel model for screening anti-hepatic steatosis drugs. Nutr. Metab. 12:42. doi: 10.1186/s12986-015-0036-z
Dai, Y.-J., Cao, X.-F., Zhang, D.-D., Li, X.-F., Liu, W.-B., and Jiang, G.-Z. (2019). Chronic inflammation is a key to inducing liver injury in blunt snout bream (Megalobrama amblycephala) fed with high-fat diet. Dev. Comp. Immunol. 97, 28–37. doi: 10.1016/j.dci.2019.03.009
Day, C. P., and James, O. F. W. (1998). Steatohepatitis: A tale of two ‘Hits’? Gastroenterology 114, 842–845.
Du, Z. (2014). Causes of fatty liver in farmed fish: a review and new perspectives. J. Fish. China 38, 1628–1638.
Fan, Y., Ren, C., Meng, F., Deng, K., Zhang, G., and Wang, F. (2019). Effects of algae supplementation in high-energy dietary on fatty acid composition and the expression of genes involved in lipid metabolism in Hu sheep managed under intensive finishing system. Meat Sci. 157:107872. doi: 10.1016/j.meatsci.2019.06.008
Folch, J., Lees, M., and Stanley, G. S. (1957). A simple method for the isolation and purification of total lipides from animal tissues. J. Biol. Chem. 226, 497–509.
Gentile, C. L., Frye, M. A., and Pagliassotti, M. J. (2011). Fatty acids and the endoplasmic reticulum in nonalcoholic fatty liver disease. Biofactors 37, 8–16. doi: 10.1002/biof.135
Ghanawi, J., Roy, L., Davis, D. A., and Saoud, I. P. (2011). Effects of dietary lipid levels on growth performance of marbled spinefoot rabbitfish Siganus rivulatus. Aquaculture 310, 395–400.
He, A. Y., Ning, L. J., Chen, L. Q., Chen, Y. L., Xing, Q., Li, J. M., et al. (2015). Systemic adaptation of lipid metabolism in response to low- and high-fat diet in Nile tilapia (Oreochromis niloticus). Physiol. Rep. 3:e12485. doi: 10.14814/phy2.12485
Huang, K., Yang, H. K., Gan, H., Gong, Z. L., Gan, X., and Luo, Y. J. (2007). Effects of diet-supplemental choline on fatty liver pathological changes in tilapia(Oreochromis niloticus×O.aureus). J. Fishery Sci. China 14, 257–262.
Ide, S., Beroza, G. C. H., Kanamori, M., Kikuchi, A., and Huynh, T. (2011). TFEB links autophagy to lysosomal biogenesis. Science 1429, 1429–1433. doi: 10.1126/science.1204592
Ioannou, G. N. (2016). The role of cholesterol in the pathogenesis of NASH. Trends Endocrinol. Metab. 27, 84–95.
Jia, R., Li, Y., Cao, L., Du, J., Zheng, T., Qian, H., et al. (2019). Antioxidative, anti-inflammatory and hepatoprotective effects of resveratrol on oxidative stress-induced liver damage in tilapia (Oreochromis niloticus). Comp. Biochem. Physiol. Part C Toxicol. Pharmacol. 215, 56–66. doi: 10.1016/j.cbpc.2018.10.002
Jia, Y., Jing, Q., Niu, H., and Huang, B. (2017). Ameliorative effect of vitamin E on hepatic oxidative stress and hypoimmunity induced by high-fat diet in turbot (Scophthalmus maximus). Fish Shellfish Immunol. 67, 634–642. doi: 10.1016/j.fsi.2017.06.056
Jin, M., Pan, T. T., Cheng, X., Zhu, T. T., Sun, P., Zhou, F., et al. (2019). Effects of supplemental dietary L-carnitine and bile acids on growth performance, antioxidant and immune ability, histopathological changes and inflammatory response in juvenile black seabream (Acanthopagrus schlegelii) fed high-fat diet. Aquaculture 504, 199–209.
Kaplowitz, N., Than, T. A., Shinohara, M., and Ji, C. (2007). Endoplasmic reticulum stress and liver injury. Semin. Liver Dis. 27, 367–377.
Kawano, Y., and Cohen, D. E. (2013). Mechanisms of hepatic triglyceride accumulation in non-alcoholic fatty liver disease. J. Gastroenterol. 48, 434–441. doi: 10.1007/s00535-013-0758-5
Kim, K. D., Kim, K. M., Kim, K. W., Yong, J. K., and Lee, S. M. (2006). Influence of lipid level and supplemental lecithin in diet on growth, feed utilization and body composition of juvenile flounder (Paralichthys olivaceus) in suboptimal water temperatures. Aquaculture 251, 484–490.
Lavallard, V. J., and Gual, P. (2014). Autophagy and non-alcoholic fatty liver disease. Biomed Res. Int. 2014:120179. doi: 10.1155/2014/120179
Lebeaupin, C., Vallée, D., Hazari, Y., Hetz, C., Chevet, E., and Bailly-Maitre, B. (2018). Endoplasmic reticulum stress signalling and the pathogenesis of non-alcoholic fatty liver disease. J. Hepatol. 69, 927–947. doi: 10.1016/j.jhep.2018.06.008
Li, A., Yuan, X., Liang, X.-F., Liu, L., Li, J., Li, B., et al. (2016). Adaptations of lipid metabolism and food intake in response to low and high fat diets in juvenile grass carp (Ctenopharyngodon idellus). Aquaculture 457, 43–49.
Limbu, S. M., Ma, Q., Zhang, M.-L., and Du, Z.-Y. (2019). High fat diet worsens the adverse effects of antibiotic on intestinal health in juvenile Nile tilapia (Oreochromis niloticus). Sci. Total Environ. 680, 169–180. doi: 10.1016/j.scitotenv.2019.05.067
Lin, C.-W., Zhang, H., Li, M., Xiong, X., Chen, X., Chen, X., et al. (2013). Pharmacological promotion of autophagy alleviates steatosis and injury in alcoholic and non-alcoholic fatty liver conditions in mice. J. Hepatol. 58, 993–999. doi: 10.1016/j.jhep.2013.01.011
Lin, J.-J., Liu, Y.-C., Chang, C.-J., Pan, M.-H., Lee, M.-F., and Pan, B. S. (2018). Hepatoprotective mechanism of freshwater clam extract alleviates non-alcoholic fatty liver disease: elucidated in vitro and in vivo models. Food Funct. 9, 6315–6325. doi: 10.1039/c8fo01758a
Livak, K., and Schmittgen, T. (2001). Analysis of relative gene expression data using real-time quantitative PCR and the 2(-Delta Delta C(T)) Method. Methods 25, 402–408.
Lu, K. L., Wang, L. N., Zhang, D. D., Liu, W. B., and Xu, W. N. (2017). Berberine attenuates oxidative stress and hepatocytes apoptosis via protecting mitochondria in blunt snout bream Megalobrama amblycephala fed high-fat diets. Fish Physiol. Biochem. 43, 65–76. doi: 10.1007/s10695-016-0268-5
Lu, K. L., Xu, W. N., Wang, L. N., Zhang, D. D., Zhang, C. N., and Liu, W. B. (2014). Hepatic beta-oxidation and regulation of carnitine palmitoyltransferase (CPT) I in blunt snout bream Megalobrama amblycephala fed a high fat diet. PLoS One 9:e93135. doi: 10.1371/journal.pone.0093135
Ma, Q., Li, L. Y., Le, J. Y., Lu, D. L., Qiao, F., Zhang, M. L., et al. (2018). Dietary microencapsulated oil improves immune function and intestinal health in Nile tilapia fed with high-fat diet. Aquaculture 496, 19–29.
Madamanchi, N. R., and Runge, M. S. (2001). Western Blotting. Methods Mol. Med. 51:245. doi: 10.1385/1-59259-087-X:245
Martinez-Lopez, N., and Singh, R. (2015). Autophagy and lipid droplets in the liver. Annu. Rev. Nutr. 35, 215–237. doi: 10.1146/annurev-nutr-071813-105336
Matsumoto, T., Terai, S., Oishi, T., Kuwashiro, S., Fujisawa, K., Yamamoto, N., et al. (2010). Medaka as a model for human nonalcoholic steatohepatitis. Dis. Model. Mech. 3, 431–440. doi: 10.1242/dmm.002311
Min, H., Kapoor, A., Fuchs, M., Mirshahi, F., Zhou, H., Maher, J. W., et al. (2012). Increased hepatic synthesis and dysregulation of cholesterol metabolism is associated with the severity of nonalcoholic fatty liver disease. Cell Metab. 15, 665–674. doi: 10.1016/j.cmet.2012.04.004
Morais, S., Conceição, L. E. C., Rønnestad, I., Koven, W., Cahu, C., Infante, J. L. Z., et al. (2007). Dietary neutral lipid level and source in marine fish larvae: effects on digestive physiology and food intake. Aquaculture 268, 106–122.
Musso, G., Gambino, R., and Cassader, M. (2013). Cholesterol metabolism and the pathogenesis of non-alcoholic steatohepatitis. Prog. Lipid Res. 52, 175–191. doi: 10.1016/j.plipres.2012.11.002
Qiang, J., He, J., Yang, H., Sun, Y.-L., Tao, Y.-F., Xu, P., et al. (2017). Dietary lipid requirements of larval genetically improved farmed tilapia, Oreochromis niloticus (L.), and effects on growth performance, expression of digestive enzyme genes, and immune response. Aquac. Res. 48, 2827–2840.
Qiang, J., Tao, Y. F., Bao, J. W., Chen, D. J., Li, H. X., He, J., et al. (2018). High Fat Diet-Induced miR-122 regulates lipid metabolism and fat deposition in genetically improved farmed tilapia (GIFT, Oreochromis niloticus) Liver. Front. Physiol. 9:1422. doi: 10.3389/fphys.2018.01422
Qiang, X., Xu, L., Zhang, M., Zhang, P., Wang, Y., Wang, Y., et al. (2016). Demethyleneberberine attenuates non-alcoholic fatty liver disease with activation of AMPK and inhibition of oxidative stress. Biochem. Biophys. Res. Commun. 472, 603–609. doi: 10.1016/j.bbrc.2016.03.019
Ren, H., Wang, D., Zhang, L., Kang, X., Li, Y., Zhou, X., et al. (2019). Catalpol induces autophagy and attenuates liver steatosis in ob/ob and high-fat diet-induced obese mice. Aging 11, 9461–9477. doi: 10.18632/aging.102396
Savard, C., Tartaglione, E. V., Kuver, R., Haigh, W. G., Farrell, G. C., Subramanian, S., et al. (2013). Synergistic interaction of dietary cholesterol and dietary fat in inducing experimental steatohepatitis. Hepatology 57, 81–92. doi: 10.1002/hep.25789
Sheng, C. H., Jing, J. L., Lee, M. F., Liu, Y. C., and Pan, B. S. (2016). Freshwater clam extracts alleviate dyslipidaemia of tilapia fed a high-fat diet as an animal model. J. Funct. Foods 25, 559–567.
Singh, R., Kaushik, S., Wang, Y., Xiang, Y., Novak, I., Komatsu, M., et al. (2009). Autophagy regulates lipid metabolism. Nature 458, 1131–1135. doi: 10.1038/nature07976
Su, H., Li, Y., Hu, D., Xie, L., Ke, H., Zheng, X., et al. (2018). Procyanidin B2 ameliorates free fatty acids-induced hepatic steatosis through regulating TFEB-mediated lysosomal pathway and redox state. Free Radic. Biol. Med. 126, 269–286. doi: 10.1016/j.freeradbiomed.2018.08.024
Subramanian, S., Goodspeed, L., Wang, S., Kim, J., Zeng, L., Ioannou, G. N., et al. (2011). Dietary cholesterol exacerbates hepatic steatosis and inflammation in obese LDL receptor-deficient mice. J. Lipid Res. 52, 1626–1635. doi: 10.1194/jlr.M016246
Tanaka, S., Hikita, H., Tatsumi, T., Sakamori, R., Nozaki, Y., Sakane, S., et al. (2016). Rubicon inhibits autophagy and accelerates hepatocyte apoptosis and lipid accumulation in nonalcoholic fatty liver disease in mice. Hepatology 64, 1994–2014. doi: 10.1002/hep.28820
Tang, T., Hu, Y., Peng, M., Chu, W. Y., Hu, Y. J., and Zhong, L. (2019). Effects of high-fat diet on growth performance, lipid accumulation and lipid metabolism-related MicroRNA/gene expression in the liver of grass carp (Ctenopharyngodon idella). Comp. Biochem. Physiol. B Biochem. Mol. Biol. 234, 34–40. doi: 10.1016/j.cbpb.2019.04.006
Urra, H., Dufey, E., Lisbona, F., Rojas-Rivera, D., and Hetz, C. (2013). When ER stress reaches a dead end. Biochim. Biophys. Acta 1833, 3507–3517. doi: 10.1016/j.bbamcr.2013.07.024
Vergara, J. M., López-Calero, G., Robaina, L., Caballero, M. J., Montero, D., Izquierdo, M. S., et al. (1999). Growth, feed utilization and body lipid content of gilthead seabream (Sparus aurata) fed increasing lipid levels and fish meals of different quality. Aquaculture 179, 35–44.
Watanabe, T. (1982). Lipid nutrition in fish. Comp. Biochem. Physiol. Part B Comp. Biochem. 73, 3–15. doi: 10.3390/nu11102390
Wu, W. K. K., Zhang, L., and Chan, M. T. V. (2018). “Autophagy, NAFLD and NAFLD-Related HCC,” in Obesity, Fatty Liver and Liver Cancer, ed. J. Yu (Singapore: Springer), 127–138.
Keywords: steatosis, endoplasmic reticulum (ER) stress, autophagy, high-fat diet, Oreochromis niloticus
Citation: Jia R, Cao L-P, Du J-L, He Q, Gu Z-Y, Jeney G, Xu P and Yin G-J (2020) Effects of High-Fat Diet on Steatosis, Endoplasmic Reticulum Stress and Autophagy in Liver of Tilapia (Oreochromis niloticus). Front. Mar. Sci. 7:363. doi: 10.3389/fmars.2020.00363
Received: 25 March 2020; Accepted: 29 April 2020;
Published: 26 May 2020.
Edited by:
Kang-le Lu, Jimei University, ChinaReviewed by:
Xian Li, Institute of Oceanology (CAS), ChinaChanghong Cheng, Key Laboratory of South China Sea Fishery Resources Exploitation and Utilization, South China Sea Fisheries Research Institute (CAFS), China
Copyright © 2020 Jia, Cao, Du, He, Gu, Jeney, Xu and Yin. This is an open-access article distributed under the terms of the Creative Commons Attribution License (CC BY). The use, distribution or reproduction in other forums is permitted, provided the original author(s) and the copyright owner(s) are credited and that the original publication in this journal is cited, in accordance with accepted academic practice. No use, distribution or reproduction is permitted which does not comply with these terms.
*Correspondence: Pao Xu, eHVwQGZmcmMuY24=; Guo-Jun Yin, eWluZ2pAZmZyYy5jbg==