- 1Centre for Integrative Ecology, School of Life and Environmental Sciences, Deakin University, Burwood, VIC, Australia
- 2School of Biological Sciences, The University of Queensland, Brisbane, QLD, Australia
- 3International Atomic Energy, Monaco, Monaco
- 4Centre for Marine Ecosystems Research, School of Science, Edith Cowan University, Joondalup, WA, Australia
- 5Institut de Ciència i Tecnologia Ambientals, Departamento de Física, Universitat Autònoma de Barcelona, Barcelona, Spain
Historically, coastal “blue carbon” ecosystems (tidal marshes, mangrove forests, seagrass meadows) have been impacted and degraded by human intervention, mainly in the form of land acquisition. With increasing recognition of the role of blue carbon ecosystems in climate mitigation, protecting and rehabilitating these ecosystems becomes increasingly more important. This study evaluated the potential carbon gains from rehabilitating a degraded coastal tidal marsh site in south-eastern Australia. Tidal exchange at the study site had been restricted by the construction of earthen barriers for the purpose of reclaiming land for commercial salt production. Analysis of sediment cores (elemental carbon and 210Pb dating) revealed that the site had stopped accumulating carbon since it had been converted to salt ponds 65 years earlier. In contrast, nearby recovered (“control”) tidal marsh areas are still accumulating carbon at relatively high rates (0.54 tons C ha–1year–1). Using elevation and sea level rise (SLR) data, we estimated the potential future distribution of tidal marsh vegetation if the earthen barrier were removed and tidal exchange was restored to the degraded site. We estimated that the sediment-based carbon gains over the next 50 years after restoring this small site (360 ha) would be 9,000 tons C, which could offset the annual emissions of ∼7,000 passenger cars at present time (at 4.6 metric tons pa.) or ∼1,400 Australians. Overall, we recommend that this site is a promising prospect for rehabilitation based on the opportunity for blue carbon additionality, and that the business case for rehabilitation could be bolstered through valuation of other co-benefits, such as nitrogen removal, support to fisheries, sediment stabilization, and enhanced biodiversity.
Introduction
Coastal wetlands, such as seagrass meadows, tidal marshes, and mangrove forests, have the ability to sequester organic carbon in their sediments over millennial time scales at rates 30–50-fold greater than the soils of terrestrial forests (Duarte et al., 2013). Although coastal wetlands represent less than 3% of terrestrial forests coverage, they are able to sequester similar amounts of organic carbon annually (Duarte et al., 2013). These ecosystems not only remove CO2 from the atmosphere by storing carbon in their living biomass through photosynthesis, they also have the ability to trap externally produced organic carbon suspended in tidal flows and terrestrial runoff, which they continually accrete within their sediments over time (Mcleod et al., 2011). Carbon is therefore derived locally (termed “autochthonous” carbon) and externally (“allochthonous” carbon) resulting in this increased efficiency compared to terrestrial ecosystems. As global attention focuses on bio-sequestration as a tool to remove carbon emissions from the atmosphere, protecting and rehabilitating these coastal ecosystems from degradation become increasingly critical to climate change mitigation.
Historically, coastal ecosystems have been impacted and degraded by human intervention, mainly in the form of land acquisitions for agriculture, aquaculture, and coastal development (Wylie et al., 2016); eutrophication (Adam, 2002; Macreadie et al., 2017), and changes in sediment input resulting from the construction of dams, levees, and irrigation (Adam, 2002). Over 60% of coastal wetlands have been lost globally during the 20th century (Davidson, 2014). Based on current rates of loss, an estimated 30–40% of tidal marshes and seagrasses and an even greater percentage of mangroves could be lost in the next 100 years (Pendleton et al., 2012). Thus, rehabilitation projects can have a great impact on improving several ecosystem services directly and indirectly. For example, mangrove rehabilitation projects implemented in Mexico to increase carbon storage also benefited from coastal protection and water purification ecosystem services (Curado et al., 2013, 2014; Adame et al., 2015a). Such projects therefore not only assist in mitigating climate change, but also provide additional benefits to the surrounding environment and community.
Coastal wetlands may also face further stresses with keeping pace with sea level rise (SLR). Land elevation and the capacity to accrete sediment will be key factors when coping with this additional pressure (Adam, 2002). If the ability of coastal wetlands to accrete sediments exceeds the rate of SLR or if the surrounding environment allows for expansion inland (for example, the removal of any man-made barriers or infrastructure), these ecosystems may be able to survive or possibly increase in the future (Adam, 2002; Macreadie et al., 2017). However, Macreadie et al. (2017) found that Australian tidal marsh ecosystems, on average, accrete sediments at a rate of 2.09 ± 0.32 mm year–1, considerably slower than the global rate of 6.73 ± 0.07 mm year–1 (Duarte et al., 2013). It was calculated that a global SLR greater than 26 cm by 2100 would likely be greater than some ecosystems’ abilities to accrete sediment and increase elevation over this period of time (Macreadie et al., 2017). In addition, SLR can also have synergic effects with changes in the salinity and inundation conditions which can lead to reduced fitness for some marsh species (Gallego-Tévar et al., 2019).
Rehabilitation of these coastal wetlands may also offer potential economic incentives in the form of market-based, emission-trading schemes (Ullman et al., 2013; Wylie et al., 2016). Under such schemes, the amount of carbon stored in these ecosystems is measured and sold as carbon credits to those entities needing to offset their emissions (Wylie et al., 2016). These incentives have the potential to offer management agencies and conservation organizations a mechanism to finance or co-fund future rehabilitation projects (Macreadie et al., 2017). Although carbon sequestration and storage are important metrics when determining the carbon characteristics of the ecosystem, when assessing the carbon gains from rehabilitation, we cannot not rely solely on these data to give us an accurate evaluation of the site as it may take many years for a rehabilitated site to reach the functionality of a natural or recovered site (Osland et al., 2012; Burden et al., 2019). In addition, a number of studies have shown that carbon sequestration rates and carbon storage values vary among sites depending upon local factors such as hydrology, sediment type, and plant species present (e.g., Kelleway et al., 2016; Macreadie et al., 2017), which may influence the timeframe for functional rehabilitation.
In South Eastern Australia, an estimated 70% of tidal marshes have been lost since European settlement (Zann, 2000; Rogers et al., 2016). Port Phillip Bay in Victoria has experienced a ∼65% reduction in tidal marsh ecosystem cover post-European arrival (Ghent, 2004; Boon et al., 2011). This loss has been mainly due to increasing population pressures and land conversions for agriculture, coastal development, water treatment, and salt production (Boon et al., 2011). The Australian Government has started to recognize the need to protect and conserve tidal marsh ecosystems, listing subtropical and temperate coastal tidal marsh as a vulnerable ecological community under the Environment Protection and Biodiversity Conservation (EPBC) Act 1999 (Macreadie et al., 2017; Wegscheidl et al., 2017). As a result, any new or expanded development that impacts tidal marsh ecosystems will require an environmental assessment (Wegscheidl et al., 2017). Initially, interest in rehabilitating degraded tidal marsh sites has been motivated by establishing habitat for declining populations of migratory and resident shore and water birds (Purnell et al., 2015). To our knowledge, no previous research has evaluated the potential benefits of rehabilitating these ecosystems for carbon storage gains.
The aim of this study was to estimate and evaluate the potential blue carbon benefits of rehabilitating a tidal marsh ecosystem by restoring its natural tidal flow. To our knowledge, this is the first study that used soil carbon data from nearby rehabilitation sites to estimate potential carbon gains at a currently impacted site. Using a raster-based approach, we developed a proxy indication for the removal of tidal barriers, which were constructed for salt production. We compared the current organic carbon sequestration rate and organic carbon density (which hereafter will be referred to as carbon) among: (1) salt ponds (non-rehabilitated tidal marshes), (2) existing (or “recovered”) tidal marsh site adjacent to the ponds, and (3) the rehabilitated tidal marsh site. Within the recovered and rehabilitated sites, we also compared carbon sequestration rate and carbon density across three different categories of tidal marsh species present within the wet saltmarsh shrubland tidal marsh community. We hypothesized that there would be a difference in accumulated carbon stocks and sequestration rates among the site conditions and elevations. As part of our evaluation, we also estimated the recovered site’s capacity to keep pace with SLR and calculated the potential carbon credits to be gained following rehabilitation.
Materials and Methods
Study Site—Snake Island
Snake Island is located on the western shoreline of Port Phillip Bay, Victoria, Australia and is part of the Avalon Coastal Reserve and the Port Phillip Bay and Bellarine Peninsula Ramsar site (Figure 1). Large sections of this coastline were originally tidal marsh, until in the early 1950s Cheetham Saltworks constructed coastal barriers that restricted natural tidal flows, creating a number of shallow evaporative ponds used for salt production. Salt production at this site ceased in 2002 leaving the bund walls intact and the area disused. Many of the main bund walls remain compacted; however, native tidal marsh species such as Tecticornia arbuscula and Atriplex cinerea have re-colonized the smaller bund walls surrounding the salt ponds. This site is currently managed by Parks Victoria who is investigating options for its rehabilitation. Snake Island is primarily surrounded by agricultural farms with an operational gravel quarry on the north-eastern boundary and an abalone farm on the western boundary. The discharge water from the abalone farm is currently diverted into some of the larger salt ponds and used to maintain water levels for migratory and resident shorebirds and water birds that frequent the ponds.
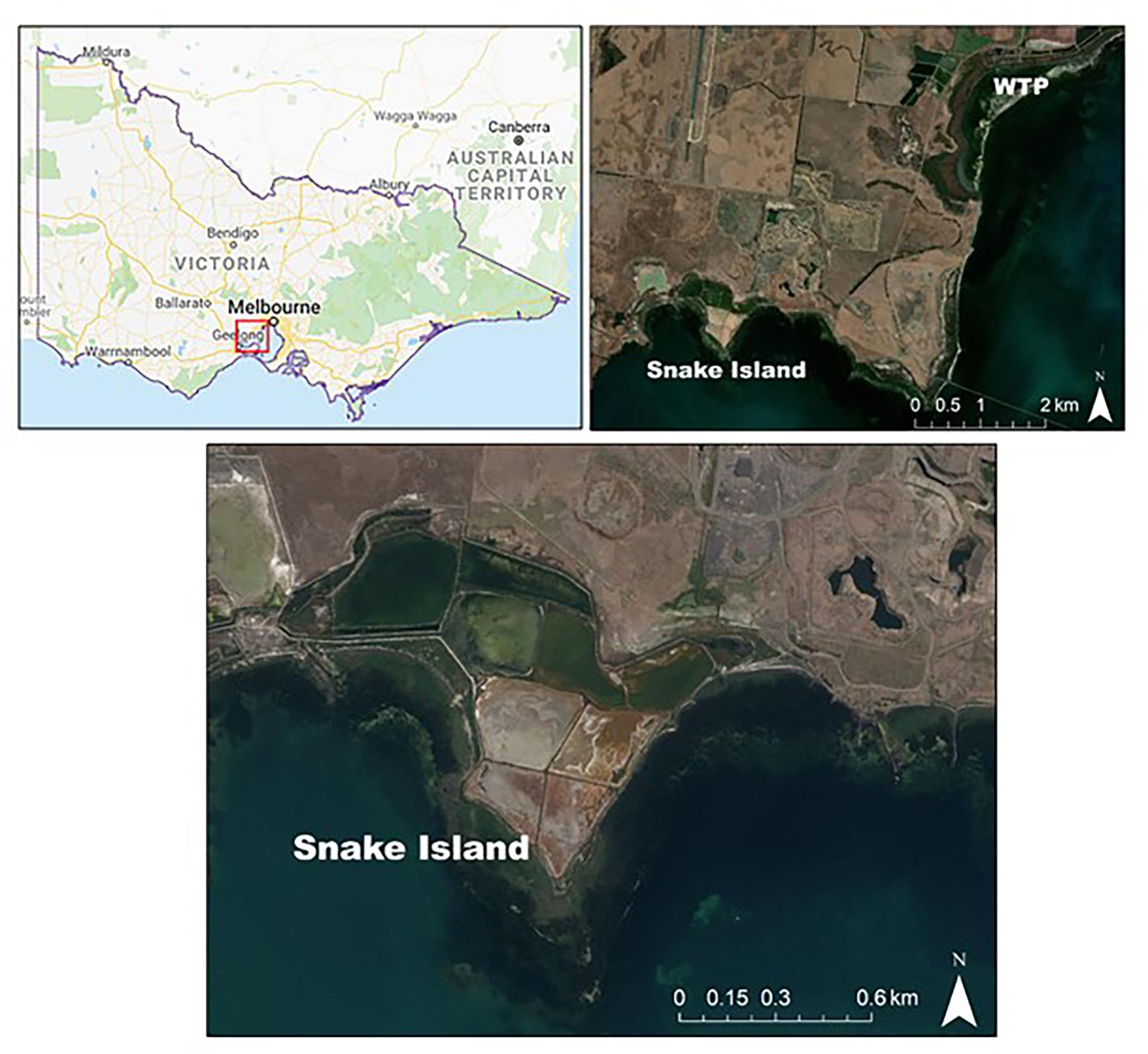
Figure 1. The study sites are located on the western shoreline of Port Phillip Bay, Victoria. The recovered tidal marsh and salt pond sites at Snake Island, Avalon and the recently rehabilitated tidal marsh site, formerly part of the Western Treatment Plant (WTP), Werribee (Map Source: ESRI DigitalGlobe, ArcGis 10.5.1).
In order to estimate the potential carbon gains following potential rehabilitation via tidal re-instatement of the disused salt ponds at Snake Island, it was important to measure soil carbon accumulation from local rehabilitation examples. Fortunately, two tidal marsh sites within 0.1–5 km of Snake Island have seen recovery post-disturbance or active rehabilitation efforts. During the initial construction of the salt ponds, the tidal marsh was cleared; however, in one section, the bund wall was removed and tidal marsh allowed to re-establish from 1952 (hereafter termed “recovered”). This site is mainly dominated by T. arbuscula (hereafter Tecticornia), Sarcocornia quinqueflora (hereafter Sarcocornia), and Suaeda australis (hereafter Suaeda) species. Nearby to this site, a large area of tidal marsh was cleared in the 1960s. The site was a former sewage treatment pond at the Western Treatment Plant (WTP) in Werribee, where bund walls were constructed and restricted natural tidal flows (Figure 1). This site was rehabilitated in 2010 by removing residual sewage material and biosolids. Part of the bund walls were removed and the pond graded to elevations that were consistent with the adjacent tidal marsh vegetation present in The Spit Nature Conservation Reserve (Ecology Australia, 2012). Tidal marsh plants have since recolonized and established naturally without any need for replanting. Here we term this site “rehabilitated.”
Field Sampling
Field sampling occurred in October 2017. Sediment cores at the recovered and rehabilitated sites were collected employing a stratified random sampling design (Howard et al., 2014). Three replicate cores were taken from random locations within three different categories of tidal marsh species presence and dominance. Given the difference in age of the tidal marsh sites (65 and 7 years old), the tidal marsh species present are different despite inhabiting similar tidal elevations. The recovered tidal marsh site is classified as a wet saltmarsh shrubland tidal marsh community and was split into three categories: (1) Sarcocornia (closest to seaward edge, located adjacent to intertidal seagrass and dominated by Sarcocornia, which is a groundcover species), Sarcocornia/Tecticornia (located at the transition zone between dominance by Sarcocornia and Tecticornia, which is a large shrub), and Tecticornia (furthest from seaward edge, with little tidal inundation and dominated by Tecticornia) (Figure 2a). At the newly rehabilitated site, the locations were similarly split into three zones: (1) Sarcocornia (closest to seaward edge and dominated by Sarcocornia), (2) Sarcocornia/Suaeda (covered equally by Sarcocornia and Suaeda), and (3) Sarcocornia/Tecticornia/Suaeda an equal mix of the three species (Figure 2b). These nine cores from each of the two rehabilitation sites were used for carbon analysis to calculate the mean carbon density (mg C cm–3) at each sample depth and inundation category. While both above- and below-ground plant biomass and soil are used to determine carbon stocks in coastal wetlands, this study focused on the below ground biomass + sediment (including roots, detritus, and sediment organic matter) in the calculations, since this component represents 65–95% of the total stored carbon for tidal marshes (Howard et al., 2014). An additional core was collected from each of the three different categories of tidal marsh species to determine the sediment/carbon accretion rates via 210Pb age dating. Two cores were also collected form the disused salt pond at Snake Island to act as a reference prior to rehabilitation—one for carbon analysis and one for 210Pb age dating.
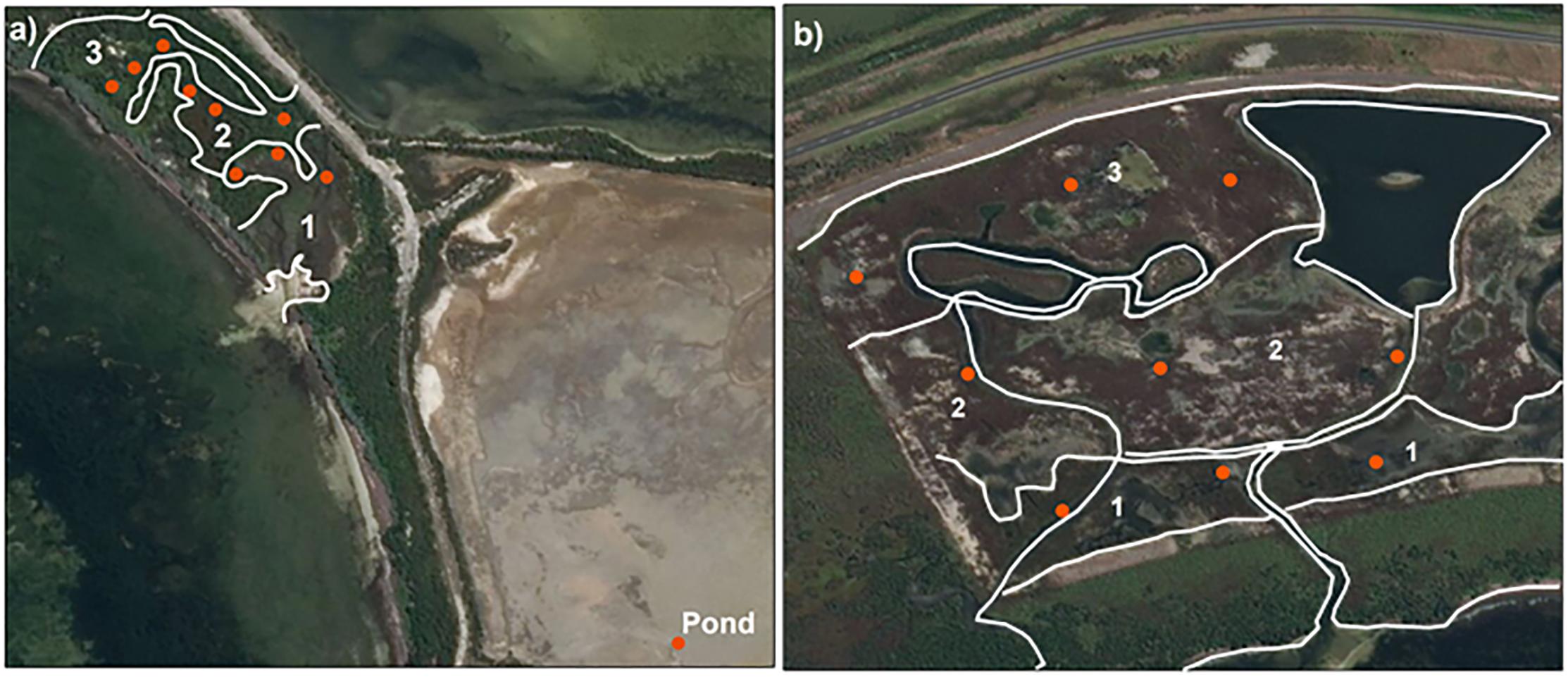
Figure 2. Location of the cores collected from (a) the recovered tidal marsh area and salt pond at Snake Island and (b) the rehabilitated site at the WTP. Given the difference in age of the tidal marsh sites (65 and 7 years old), the saltmarsh species present are different despite inhabiting similar tidal elevations. The recovered saltmarsh site is classified as a wet saltmarsh shrubland tidal marsh community and was split into three categories: (1) Sarcocornia (closest to seaward edge, located adjacent to intertidal seagrass and dominated by Sarcocornia), (2) Sarcocornia/Tecticornia (located at the transition zone between dominance by Sarcocornia and Tecticornia, which is a large shrub), and (3) Tecticornia (furthest from seaward edge, with little tidal inundation, dominated by Tecticornia). At the newly rehabilitated site, the locations were similarly split into three zones: (1) Sarcocornia (as above), (2) Sarcocornia/Suaeda (covered equally by Sarcocornia and Suaeda), and (3) Sarcocornia/Tecticornia/Suaeda an equal mix of the three species and furthest form seaward edge. Triplicate cores were collected from random locations within each of these three categories.
A petrol-powered hammer corer was used at the recovered and salt pond sites to hammer a 45 mm (internal diameter) × 1 m metal pipe into the sediment to a depth of at least 50 cm or until refusal was met. After removal, a foam disc was inserted through the top of each core to the level of the sediment to ensure that the sediment remained stable during transport. A similar procedure was taken at the rehabilitated site using 50 mm (internal diameter) × 1 m PVC pipes. The PVC cores were manually hammered into the sediment until refusal was reached. Before each core was removed a rubber-suction plug was used to create a vacuum seal, each end was then capped and taped. All cores were transported to Deakin University (Burwood, Australia). Geographic coordinates and elevations using a RTK-GPS were taken at each sampling location at all sites (Supplementary Table S1).
Sample Processing
All cores were extruded and sectioned in the laboratory. The cores taken from the recovered site and salt pond for carbon analysis were sectioned every 2 cm in the top 10 cm, then every 10 cm thereafter. Additional sections were also taken according to points of interest based on the appearance of the core. The rehabilitated site carbon analysis cores were sectioned every 1 cm in the top 20 cm, then 2 cm until 30 cm and every 5 cm, thereafter. Finer sections were taken as we expected this site to have less accumulated sediment following rehabilitation compared to the recovered site. The Sarcocornia sediment core, to be used for 210Pb analysis from all sites, were sectioned every 1 cm in the top 30 cm, then every 10 cm thereafter. All sediment sections were placed in pre-weighed plastic containers and dried at 60°C for 48–72 h until a constant weight was reached. The dry weight was recorded for each section and used to determine the dry bulk density (DBD) (g cm–3). All sections were then finely ground using a Retsch MM400 Mixer Mill for 3 min.
Age Models and Carbon Sequestration Rates
The Sarcocornia cores were selected from both the recovered and the rehabilitated sites for 210Pb analyses as these cores provided the largest sediment profile. Sections from the top 25 cm of each core were re-wetted using deionized water and sieved to 63 μm using a two-sieve method (i.e., 300 μm first to remove coarse sand, followed by 63 μm). Both fine and coarse fractions were oven dried at 60°C and the dry weights recorded. The fine fractions were sent to the Environmental Radioactivity Laboratory from the School of Science at Edith Cowan University to provide an estimate of the age of the sediment at different depths. Total 210Pb was determined every 1 or 2 cm along the cores via the analysis of its granddaughter 210Po (in equilibrium) by alpha spectrometry after acid digestion of the samples using an analytical microwave and in the presence of a known amount of 209Po added as a yield tracer (Sanchez-Cabeza et al., 1998). Excess 210Pb concentration was determined as the difference between total 210Pb and 226Ra (supported 210Pb). Ra-226 was determined for selected samples within each core by gamma spectrometry through the measurement of its decay product emission lines of 214Pb at 295 and 352 keV using calibrated geometries in an HPGe detector (CANBERRA, Mod. SAGe Well). The excess 210Pb concentration profiles were used to estimate the accretion rates where possible using the Constant Flux:Constant Sedimentation (CF:CS; Krishnaswamy et al., 1971) and the Constant Rate of Supply (CRS; Appleby and Oldfield, 1978) models following the recommendations in Arias-Ortiz et al. (2018).
The concentration profile of excess 210Pb in the recovered core suggests that the upper 4 cm were mixed, which is in accordance with the visual inspection of the sediments. Thereafter, the concentrations of 210Pb showed a decreasing trend with depth down to ∼14 cm, where a change in composition of the sediment to sand, shells, and gravel was observed (Supplementary Figure S1). The CF:CS model was applied below the mixed layer, obtaining an average sedimentation rate of 0.041 ± 0.003 g cm–2 year–1 (1.99 ± 0.15 mm year–1) (Supplementary Table S2), which allows to ascribe a date of 1952 ± 4 to the 14 cm layer. For the core from the rehabilitated site, the concentration profile of excess 210Pb showed two regions of decreasing activities with depth, from the surface to 11 cm and between 11 and 19 cm, coincidental with an apparent change in the texture of the sediment. The CF:CS and CRS models were applied and an average sedimentation rate of 0.14 ± 0.01 g cm–2 year–1 (2.2 ± 0.2 mm year–1) was obtained for the upper part of the core.
All cores collected from the recovered site appeared to show a distinct horizon (i.e., a visual marker indicating a change at the site) between an upper organic layer and deeper levels of predominantly sand, shell and gravel (Figures 3A–C). We observed this horizon at different depths in the cores relative to where the core was collected and its location to the shoreline (Figures 2, 3). A sand and shell horizon was also identified in the rehabilitated site cores (Figure 3E–G), so the depth for analysis of the lower rehabilitated site cores was determined from the 210Pb age dating, while the horizon marker approach was used for the rest of the Sarcocornia, Sarcocornia/Tecticornia, and Tecticornia cores (only top 1 cm of the high elevation core had organic matter, Figure 3G). The average accretion rate was calculated for the cores not analyzed by 210Pb by using the visual horizon marker depth (since re-establishment and dividing this by the age of that depth (year sampled – year at depth) (Adame et al., 2015b). The mean carbon accumulation rate (tons C ha–1 year–1) was calculated for each core by multiplying the accretion rate by the median carbon density for the dated sediment depth.
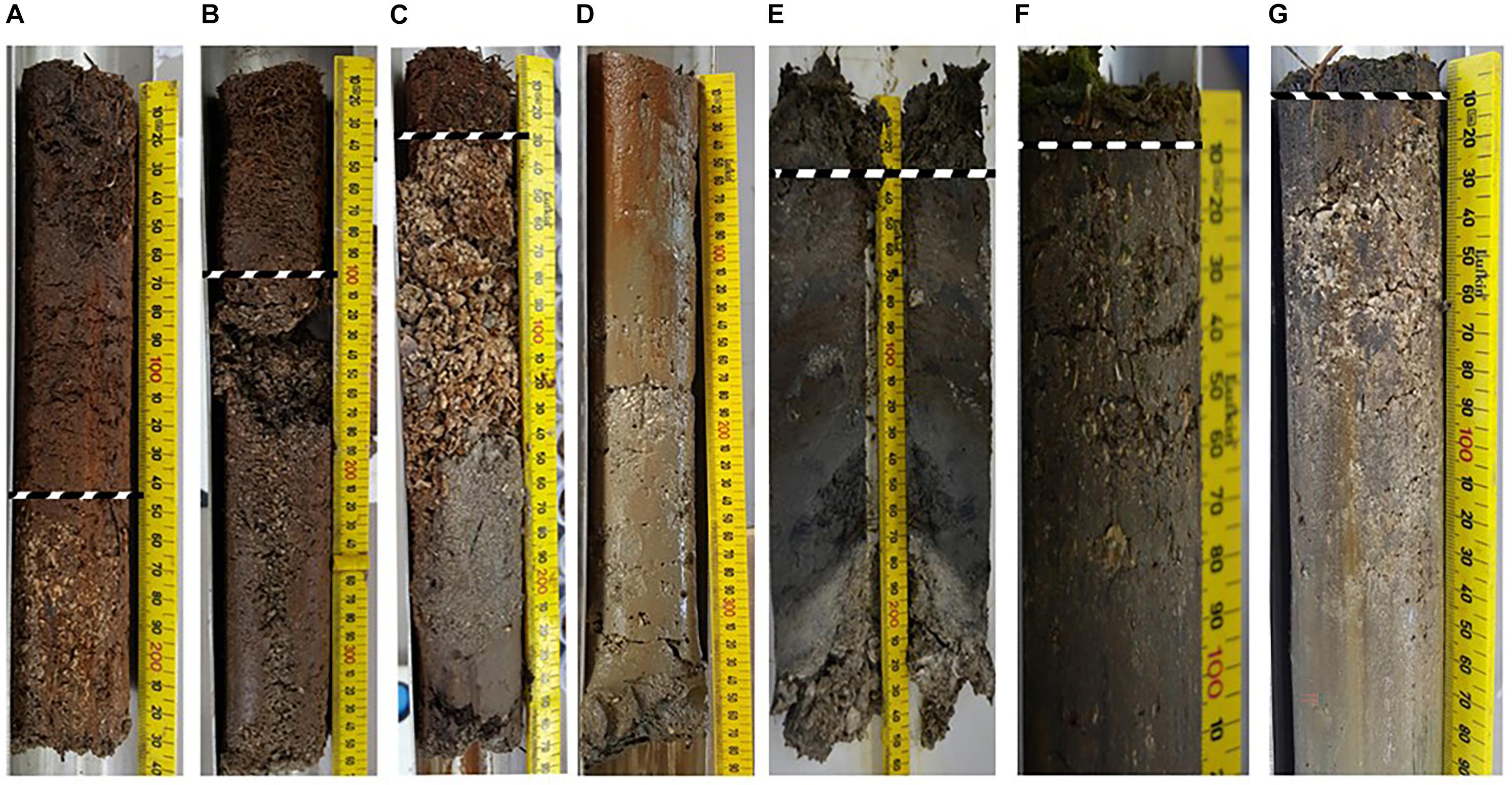
Figure 3. Sediment cores collected from the recovered, rehabilitated, and salt pond sites. The images show the horizons (dashed white line) between an upper organic layer and the deeper levels of shell and gravel. (A) Sarcocornia core 2L (recovered site); (B) Sarcocornia/Tecticornia core 2M (recovered site); (C) Tecticornia/Suaeda core 2H (recovered site); (D) Salt pond core; (E) Sarcocornia core 1L (rehabilitated site); (F) Sarcocornia/Tecticornia core 2M (rehabilitated site); and (G) Tecticornia/Suaeda core 2H (rehabilitated site).
The linear horizon marker accretion rate approach gave comparable results to the 210Pb method, with accretion rates at 1.9 ± 0.2 and 1.5 ± 0.3 mm year–1 for Sarcocornia and Sarcocornia/Tecticornia categories at the recovered zone, respectively, and 0.51 ± 0.05 mm year–1 for the Tecticornia category (Supplementary Table S1). According to the 210Pb dating, the rehabilitation occurred in 2010 would correspond to a depth of 3 cm (Supplementary Table S3). The linear method estimated 3.3 mm year–1 for the Sarcocornia category, and 1.1 and 0.56 mm year–1 for the Sarcocornia/Tecticornia and Tecticornia categories, respectively (Supplementary Table S1).
For the salt pond core, the concentrations of total 210Pb and 226Ra along the upper 24 cm indicated that there was negligible presence of excess 210Pb (Supplementary Table S4). Additionally, the pond site had no horizon marker (Figure 3D). This indicates that sediment is not being laid down in a consistent manner in the salt pond and therefore is not accreting sediment in the same way as the recovered or rehabilitated site.
Carbon Analyses
The sections selected for elemental carbon analysis were tested for the presence of inorganic carbon by adding concentrated hydrochloric acid (HCl) to a sub-sample and identifying calcium carbonate content when bubbles formed. The samples that contained inorganic carbon were then acidified by a wet acidification method (Harris et al., 2001) prior to carbon analysis to ensure that only organic carbon was detected by the elemental analyzer. Briefly, a ground sub-sample from each section was added to 50 ml centrifuge tubes together with 4 ml of 1 M HCl, which was slowly added to allow time for any rapid release of CO2. All samples were initially left for 24 h, then centrifuged for 5 min at maximum speed of 5,290 × g at 10°C using a Beckman Coulter Avanti J-26 XPI centrifuge. The supernatant was discarded and the samples repeatedly washed until no further effervesce or sand particles were observed. All acidified samples were oven dried at 40°C and re-ground using a manual mortar and pestle.
The elemental carbon content of the samples was determined in an automated elemental carbon and nitrogen (C/N) analyzer accompanied with Callidus software (v5.1) (EuroEA3000, EuroVector, Milan, Italy). Between 5 and 10 mg of ground sediment were enclosed in tin capsules. The C/N analyzer was calibrated using Acetanilide as a standard (71.09% C, 0.5–1.0 mg input mass). All run outputs in% C were checked for accuracy against the calibration curve produced by the standards, any standard that did not fit the curve were excluded and the output results adjusted (i.e., minimum 0.98% R-squared value). To determine the carbon stocks for each core, the carbon density (g cm–3) of each section was calculated by multiplying the DBD (g cm–3) by (% C/100). The sections above the natural horizon marker were then added together, to calculate the total amount of carbon accumulated since rehabilitation.
Statistical Analyses
The two response variables, carbon accumulation rates (tons C ha–1 year–1) and carbon stocks (tons C ha–1), since re-establishment, were compared between the recovered and rehabilitated sites using a one-way ANOVA. The salt pond was excluded from this comparison as negligible excess 210Pb was able to be determined, indicating that there had been no carbon accretion in the salt ponds. To meet the assumptions of normality and equal variances, the Kolmogorov–Smirnov test for normality and Levene’s test for equality of variances were performed. Carbon accumulation rates were log-10 transformed before statistical analysis to meet these assumptions. A one-way ANOVA was also used to compare the carbon accumulation rates and carbon stocks since re-establishment between the different categories of tidal marsh species presence within the wet saltmarsh shrubland tidal marsh community. A post hoc Tukey pairwise comparison was used to determine the differences in population means. To assist in making predictions about the potential gains in carbon accumulation and stocks for the salt ponds based on elevation, a linear regression analysis was used to determine the relationship between elevation and carbon accumulation rate and carbon stocks at both recovered and rehabilitated sites.
Spatial Analyses
We incorporated a range of data and spatial layers for the analysis (e.g., vegetation distribution and elevation) to estimate and map potential future restoration scenarios. As Saintilan et al. (2013) and Kelleway et al. (2017) have indicated in previous studies, elevation and tidal inundation are important factors when evaluating the rehabilitation potential and the ability of a site to accrete and build sediments. In this study, elevation data and predictive maps were derived from the 2017 Victorian Coastal Digital Elevation Model—Updated High Resolution 2.5 m DEM. Spatial layers were projected to the same coordinate system (Geocentric Datum of Australia 1994 VicGrid 94), and we used ArcGIS 10.5.1 for all spatial analyses included in this study.
Due to the opaque nature of the salt ponds preventing accurate LiDAR signals being recorded, elevation points were collected in the ponds using a REACH RS + Real Time Kinematic GPS (RTK-GPS) unit. The RTK-GPS was connected to a 2.0 m GPS Survey/Prism Monopole, allowing for a 2.085 offset height correction, and the Networked Transport of RTCM via Internet Protocol (NTRIP) was used to provide base data from satellites. The RTK point data (WGS84 Ellipsoid) was converted to Australian Height Datum (AHD) using the AusGeoid program and the AUSGeoid09 V1.01 grid file (Geoscience Australia, 2018). Then, the RTK points were merged with the LiDAR elevation data after correcting for the different datum projections. An intertidal Ecological Vegetation Classes (EVC) map in combination with a habitat map for seagrass meadows (Ball and Blake, 2001) and mangrove forests (Boon et al., 2011) of Port Philip Bay were used as a baseline layer to extract elevation values of different tidal marsh EVCs at the recovered site, salt ponds, and surrounding environment.
To estimate the flooding extent for the restoration scenario (i.e., bund walls are removed), we buffered the earthen wall by 50 m and assumed that this area was inundated by the reintroduction of tidal flow. Since elevation was our main driver, we assumed that elevation would decrease by 1 m due to inundation within this buffer, and then combined this layer with the original elevation layer for the study area. This method is likely to produce an overestimate of the restored flooding extent; however, such estimation is intended to develop a first-pass assessment on potential benefits of a tidal marsh rehabilitation and are not meant to replace hydrological modeling at the site scale.
The Empirical Bayesian Kriging Interpolation tool was used to create DEMs showing current EVCs and potential EVCs suitable for re-colonizing the salt ponds following rehabilitation based on the elevations they currently inhabit. In addition, we have also estimated the effects of SLR by considering a moderate (0.4 m) and a high (0.8 m) SLR scenarios by 2100. All DEMs were classified using the lower mean elevation (±SD) of each EVC to determine its break point. The current area (hectares) of each EVC and potential area occupied following rehabilitation was determined by multiplying the total number of cells in each category by the cell area (hectares). The difference between these areas was then used to estimate the sediment carbon gains per year following rehabilitation by multiplying the area by the mean carbon accumulation rate (tons C ha–1 year–1) calculated from the recovered site.
Using a conversion factor of 3.67 (the molecular weight ratio of CO2 to C), the mean carbon accumulation rate was then converted to CO2 equivalents. This enabled us to calculate the potential carbon offset value to be gained following rehabilitation using the weighted average price for Australian carbon credit units purchased at auction of AUD$13.52 metric tons–1 CO2 equivalents (Australian Emission Reduction Fund carbon price, June 2018). Our SLR scenarios were corrected for sediment accumulation over this period by using the mean linear accretion rate of 1.30 mm year–1 determined for the recovered site (Supplementary Table S1 for detailed information on accretion rates). All EVC distributions were based on changes to elevation due to SLR and did not account for any change in temperature or rainfall patterns that may affect plant survival.
Results
Carbon Analysis
The percentage carbon values at the recovered site to the depth sampled ranged from 7.22 to 33.96% with a mean of 19.58 ± 0.90%. The percentage carbon values at the rehabilitated site to the depth sampled were lower and ranged from 1.09 to 26.12% with mean of 10.58 ± 0.75%. The salt pond had the lowest percentage carbon values ranging from 0.89 to 1.71% with a mean of 1.42 ± 0.09% (Figure 4B).
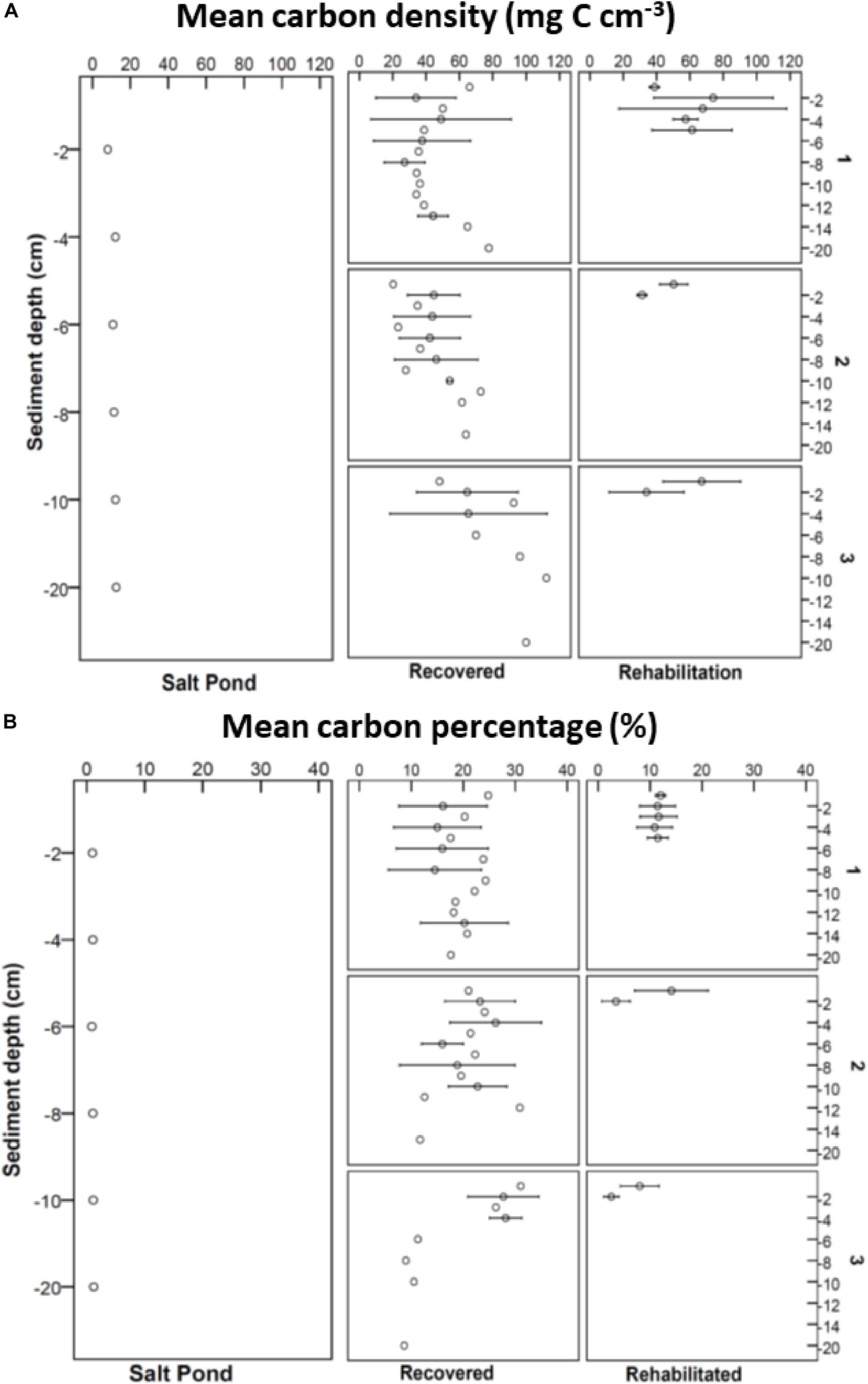
Figure 4. Mean carbon density (mg C cm– 3) over depth sampled (cm) (A) and mean carbon percentage (%) over depth samples (cm) (B) at the salt pond, recovered, and rehabilitated sites.
The carbon density over the depth sampled from the recovered site ranged from 12.7 to 112 mg C cm–3, with a mean of 51.5 ± 3.2 mg C cm–3. Relatively high carbon densities were observed in one Tecticornia replicate compared to the seaward edge cores. The carbon density over the depth sampled at the rehabilitated site ranged from 11.4 to 112.6 mg C cm–3 with a mean of 48.4 ± 2.8 mg C cm–3 (Figure 4A). Many of the high C density values were observed in the upper 0–2 cm sections, particularly in the Sarcocornia/Tecticornia/Sueda cores. The carbon density in the salt pond ranged from 8 to 20.1 mg C cm–3 with a mean of 13.6 ± 1.4 mg C cm–3 (Figure 4A).
As there was no carbon accumulation in the salt ponds, the mean carbon accumulation rates (log transformed) were determined and compared between the recovered and rehabilitated sites (Supplementary Table S1). Carbon stocks were evaluated since re-establishment, therefore only the recovered and rehabilitated sites were compared. A one-way ANOVA showed that the carbon accumulation rates were not significantly different between these sites (F1,16 = 1.756, p = 0.204); the mean carbon stocks since re-establishment were significantly different between the two sites (ANOVA, F1,16 = 23.179, p < 0.001). The mean carbon stocks since re-establishment at the rehabilitated site was lower (9.2 tons C ha–1) compared to the recovered site (37.7 tons C ha–1); however, this difference may be due to the difference in time since of re-establishment between the two sites (8 years at the rehabilitated site compared to 65 years at the recovered site, as determined by the 210Pb age dating analysis).
When comparing the mean carbon accumulation rate (log transformed) between the three different categories of tidal marsh species, there was no significant difference at the recovered site (ANOVA, F2,6 = 2.623, p = 0.152). However, the mean carbon accumulation rate (log transformed) at the rehabilitated site was significantly different across the transect (ANOVA, F2,6 = 25.361 p = 0.001), with the Sarcocornia category having the highest carbon accumulation rate (1.4 tons C ha–1 year–1) (Tukey p < 0.05) compared to the Sarcocornia/Sueda (0.8 tons C ha–1 year–1) and Sarcocornia/Tecticornia/Suaeda (0.6 tons C ha–1 year–1) categories (Supplementary Figure S2a). Likewise, the mean carbon stocks at the recovered site did not show any significant difference among the different categories of tidal marsh species (ANOVA, F2,6 = 1.570, p = 0.283, Supplementary Figure S2b), while the rehabilitated site was significantly different across the transect (ANOVA, F2,6 = 10.683, p = 0.011), with the Sarcocornia having the highest mean carbon stocks (15.8 tons C ha–1) since re-establishment compared to the Sarcocornia/Sueda (5 tons C ha–1) and Sarcocornia/Tecticornia/Suaeda (6.7 tons C ha–1) (Tukey p < 0.05) categories (Supplementary Figure S2b).
The linear regression analysis of the effect of elevation on carbon accumulation rate at the recovered site showed no effect (r2 = 0.312, F1,7 = 3.180, p = 0.118, n = 9). The model was reasonably weak with only 31.2% of the variation in carbon accumulation rate that could be explained by elevation (Supplementary Figure S2c). Similarly, there was no effect on carbon stocks due to elevation at the recovered site (r2 = 0.302, F1,7 = 3.022, p = 0.126, n = 9) or the rehabilitated site (Figure 5D).
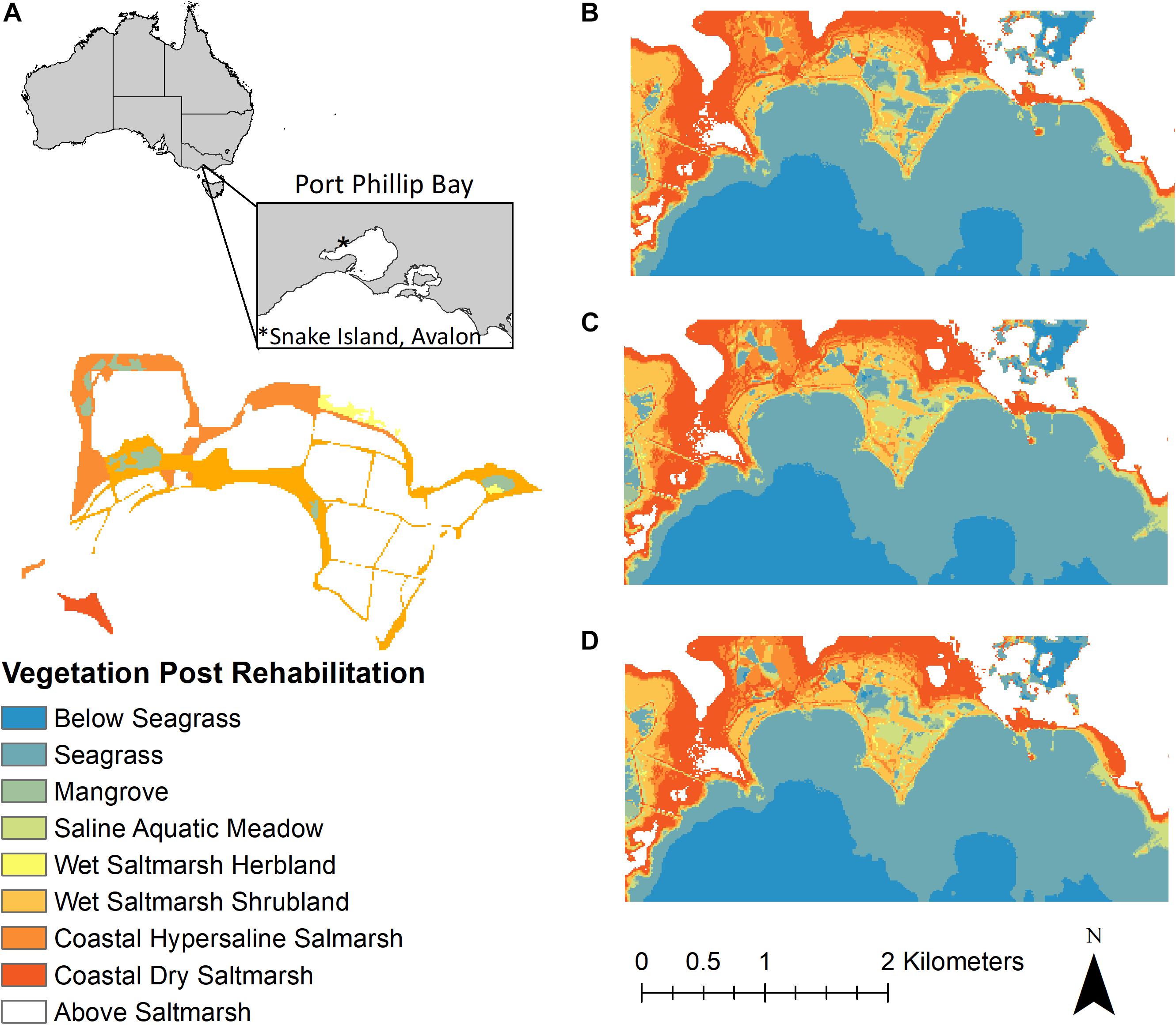
Figure 5. (A) Current EVCs at the recovered site and salt ponds, the bare areas shown relate to the salt ponds. (B) DEM predicting the different tidal marsh EVCs, seagrass, and mangrove habitats following rehabilitation. (C) The DEM of the possible plant communities following an SLR of 0.4 m by 2100 (adjusted to 0.3 m allowing for sediment accretion). (D) Following an SLR of 0.8 m (adjusted to 0.7 m).
Spatial Analyses
The current EVC map shows the salt ponds as bare areas surrounded by a narrow margin of Wet Saltmarsh Shrubland with the back, higher elevation ponds surrounded by Coastal Dry Saltmarsh vegetation (Figure 5A). If natural tidal flow was restored to the salt ponds, many of the lower ponds could support the re-establishment of Saline Aquatic Meadow species with increased areas of Wet Saltmarsh Shrubland. The higher salt ponds and elevations could support survival of increased areas of both Coastal Hypersaline and Coastal Dry Saltmarsh (Figure 5A). Some of the salt ponds, furthest from the seaward edge, remain too deep for tidal marsh species, but suitable for seagrass. Using the Port Phillip Bay mangrove habitat map (Boon et al., 2011), the areas of mangroves were estimated indicating suitable elevations for their survival; however, factors other than elevation may be limiting their survival as they are not commonly found in the area and were not evident in the historical maps (Figure 5B).
The sediment carbon gains following rehabilitation were calculated with a net increase of 177.5 ± 39.4 tons C year–1. Coastal Dry Saltmarsh EVC showed the greatest carbon gains following rehabilitation with gains of 85.2 tons C year–1. Wet Saltmarsh EVC and Coast Hypersaline Saltmarsh EVC showed carbon gains of 43.1 and 19 tons C year–1, respectively. Based on changes in elevation, the moderate SLR scenario showed an increase in seagrass in most ponds with reduced areas of Wet Saltmarsh Shrubland. Much of this EVC that surrounded the original salt ponds was replaced with Saline Aquatic Meadow; however, Wet Saltmarsh Shrubland EVC can still survive further inland (Figure 5C). The higher SLR scenario showed the original salt ponds almost completely submerged with an outline remaining of Saline Aquatic Meadow. Wet Saltmarsh Shrubland was further reduced and remains at the higher elevations. Coastal Dry Saltmarsh was present in the surrounding environment extending into the adjacent land that was previously at elevations unsuitable for tidal marsh species and used for farming (Figure 5D).
Discussion
Carbon Accumulation Rate and Carbon Stocks
This study estimated the carbon-gain potential of rehabilitating a salt pond back into a tidal marsh after more than a half century of tidal exchange restriction. The low mean carbon density (13.7 ± 1.4 mg C cm–3) and lack of sediment accretion measured in the salt pond is an indication that either the ponds are not accumulating carbon or accumulation is undetectable. The site would have stopped accumulating carbon since 1952, when Cheetham Salt (2013) was constructing the salt ponds at Avalon. The Cheetham Salt historical timeline indicated that the site was purchased in 1950 with the first salt harvest produced in 1954. This is contrasted by the adjacent recovered tidal marsh site where the mean carbon density (51.5 ± 3.2 mg C cm–3) was four times higher than the salt pond, with a mean carbon accumulation rate of 0.5 ± 0.3 tons C ha–1 year–1. The findings were consistent with the expectation that by rehabilitating the salt ponds back to coastal wetlands we would see an increase in carbon accumulation.
We found that there was no significant difference in carbon accumulation among the three different categories of tidal marsh species presence within the wet saltmarsh shrubland tidal marsh community at the recovered site. This contrasts with previous studies that found differences in accumulation rates based on tidal range, distribution of resident marsh species along the elevation gradient, position in the tidal frame and geomorphic origins, either marine or fluvial (Saintilan et al., 2013; Rogers et al., 2014; Contreras-Cruzado et al., 2017). However, Saintilan et al. (2013) compared carbon accretion rates at different locations extending over 2,000 km of south-eastern Australian coastline, while Rogers et al. (2014) compared carbon accretion rates across different habitat types (tidal marsh and mangrove). In contrast, our study compared accumulation rates at a single tidal marsh site within a small spatial scale (approximately 1,524 ha). The lack of influence of the species composition across the three zones suggests that relative differences in plant productivity may be less of a driver of long-term carbon accumulation estimates within this single recovered site. Possibly, a larger spatial scale is required to tease apart the effects of tidal range, plant composition, and position in the tidal frame on carbon accretion rates.
Previous studies and reviews comparing rehabilitated to reference (natural) sites note that it can take considerable time for newly rehabilitated sites to reach similar levels of carbon accumulation (Moreno-Mateos et al., 2012; Osland et al., 2012; Burden et al., 2019; and others), with above-ground biomass evolving more rapidly than below-ground biomass and organic matter in the sediments (Curado et al., 2013). For the purposes of this study, comparing a newly rehabilitated and a recovered tidal marsh site was to gain an indication of any differences that may occur in carbon density and accretion due to a newly developing ecosystem. We envisaged that the recovered site’s history was less complex than the rehabilitation site, such that its underlying strata had not been substantially altered. That been said, significant work was undertaken at the rehabilitated site to ensure that the top silt layer, which contained high levels of phosphorus and nitrogen, was completely removed before rehabilitation works commenced (Sonneman et al., 2008). We recommend that future studies, where possible, compare multiple recovered and rehabilitated sites in close proximity in order to reduce such extraneous inter-site variability. Nonetheless, comparison between these two sites is informative and important, as they are one of the rare rehabilitated tidal marsh ecosystems along the Victorian coast.
This study did not find a strong relationship between elevation and carbon accumulation rate at either the recovered or rehabilitation sites. Many studies have reported the importance of elevation in sedimentation rates due to its link to tidal inundation (Saintilan et al., 2013; Kelleway et al., 2017). However, other factors such as tidal range, location within the tidal frame, length of time of tidal inundation and differing plant communities, influence accretion rates variability, and therefore carbon accumulation (Saintilan et al., 2013). In our study, the change in elevation, particularly between the Sarcocornia and Sarcocornia/Tecticornia cores at the recovered site (0.18 m) and Sarcocornia and Sarcocornia/Tecticornia/Sueda at the rehabilitated site (0.31 m), was small, suggesting a small tidal range may be confounding the expected elevation-carbon relationship. However, we did show that the Tecticornia and Sarcocornia/Tecticornia/Suaeda categories, i.e., furthest from the seaward edge, cores at both sites all reported lower accretion and carbon accumulation rates. These results suggest that three different categories of tidal marsh species and tidal range were more important factors than elevation. Additional work is needed to decouple the effect of elevation, tidal range, and tidal marsh species on sediment and carbon accumulation.
Restoration Potential of Snake Island
Elevation is one of the main factors affecting the distribution of tidal marsh plant species and is an important consideration when determining rehabilitation success (Laegdsgaard, 2006). If this site were to be rehabilitated by removing the bund walls, an increase of 328 ha of coastal wetlands was estimated (Table 1). Currently, the bund walls surrounding the salt ponds support Wet Saltmarsh Shrubland species such as A. cinerea and T. arbuscula. This plant community has the potential to increase in area by a factor of three, as elevations within the salt ponds are suitable for these species’ establishment and survival, allowing them to spread into and further around any remaining the salt pond perimeters. Some of the more elevated salt pond areas would be able to support increased areas of Coastal Hypersaline and Coastal Dry Saltmarsh. However, some caution is required when evaluating the potential 158 ha increase in Coastal Dry Saltmarsh, as some of this area includes adjoining pastoral land (privately owned) that is not currently managed by Parks Victoria.
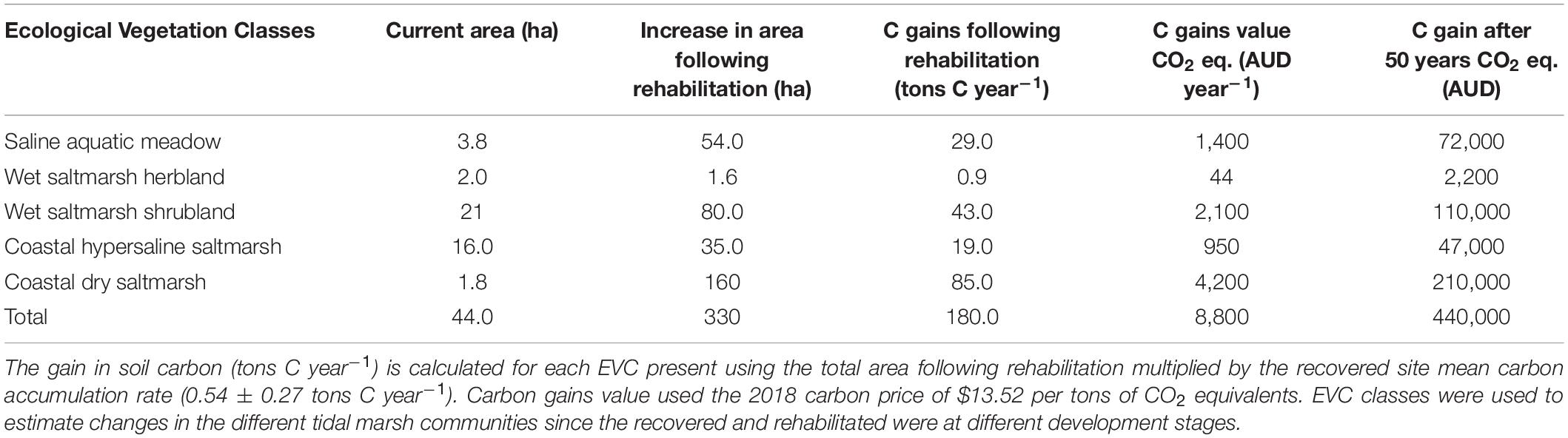
Table 1. Current EVC area (hectares) at the recovered site and salt ponds and the potential area (hectares) following rehabilitation.
The remaining salt ponds are at elevations more suitable for seagrass and Saline Aquatic Meadow plant communities rather than tidal marsh. These deeper areas offer a diversity of habitats important to the migratory and resident shorebirds and water birds that frequent the ponds (Brett Lane and Associates Pty Ltd, 2009). This also potentially allows for the inclusion of small islands in the rehabilitation plan, that have increased bird foraging and nesting opportunities at the rehabilitation site (Sonneman et al., 2008) and in other salt pond rehabilitation projects such as in South San Francisco Bay, California (Ackerman et al., 2014).
Organic Carbon Gains and Carbon Credits
We estimated that the rehabilitation of 328 hectares of tidal marsh would result in the accumulation of 180 ± 40 tons C year–1 annually. Using the current value for carbon, in 50 years the accumulated carbon of 9,000 tons C would be worth AUD$450,000 (AUD$13.52 metric ton–1 CO2 equivalents; Australian Emission Reduction Fund, December 2018). This carbon gains estimate could be an underestimate since we have not accounted for (a) the additional ecosystem potential and values of both seagrass and mangrove establishment at the site, (b) the value of the carbon bound within living plant biomass, and (c) the possibility of an increase/decrease in the price of carbon. An assessment of the cost of rehabilitating a degraded coastal site in Australia is required to more accurately understand the financial benefits of rehabilitation combined with the ability to put a “dollar value” on the additional ecosystem services (e.g., fisheries habitat, nutrient cycling, water purification, recreation, and protection from coastal erosion) that restoration could also provide to the surrounding environment and community (Barbier et al., 2011; Adame et al., 2015a).
Sea Level Rise
The predictive maps of the extended study area, displaying the effects of rising sea levels by 2100, were created using a modeling approach adjusted for tidal marsh accretion over this time (recovered site’s mean accretion rate = 1.30 mm year–1). This mean accretion rate is 1.6- to 2.6-fold lower than the SLR rates estimated for south-eastern Australia (2.1–2.8 mm year–1) and the global (3.4 mm year–1), respectively (Boon, 2012). As such, the predictive maps indicate that much of the tidal marsh area in the lower salt ponds will not keep pace with SLR. Both the moderate 0.4 m and the higher 0.8 m SLR scenario models predicted increased areas of seagrass and Saline Aquatic Meadow habitat in these lower salt ponds, with the other tidal marsh EVCs existing along the higher, landward margins. Coastal Dry Saltmarsh is the only habitat which will remain close to its current distribution, although is reliant on its ability to encroach into the surrounding pastoral landholdings.
Our study used a similar approach to model the current area suitable for tidal marsh distribution based on the mean elevation range as Rogers et al. (2012). Both studies also evaluated tidal marsh distribution area allowing for the effects of SLR. Rogers et al. (2012) study also applied an accretion model to the DEM suggesting that their approach did not overestimate the effects of SLR compared to a straightforward “bathtub” model that does not accounted for vertical sediment accretion over time. This same study (Rogers et al., 2012) used accretion data determined by feldspar marker horizons over an 8-year period, while our study was able to determine accretion rates from 210Pb age dating. It should be noted that the accretion rate was determined from the recovered tidal marsh site, thus the modeling assumed that all EVCs, seagrass, and mangrove habitats accrete sediments at the same rate. However, Serrano et al. (2019) showed in a recent study across Australian coastal wetlands that although tidal marsh and seagrass mean sequestration rates are similar, mangrove sequestration rates are three times higher than both tidal marsh and seagrass. Consequently, the vertical accretion rate may have been underestimated and therefore the effects of SLR overstated.
Further research is recommended to better understand how the bathymetry at this site will change following removal of the bund walls together with research into the factors that affect plant survival and re-establishment such as tidal inundation, wave action, and salinity at this site. As our approach suggested that elevations suitable for mangrove survival occur at the site and taking into consideration the additional carbon accumulation capabilities that this species provides, further research into the appropriateness of the site as mangrove habitat is also recommended.
Overall, by combing local carbon sequestration data with habitat distribution, this study provides a case for the blue carbon benefits of restoring tidal marsh via reinstating tidal exchange and allowing for passive re-vegetation to a degraded coastal area. This approach qualifies as a Tier 3 assessment under the IPCC Wetlands supplement, and is one of few case studies to apply this approach prior to rehabilitation. By scientifically measuring the potential carbon gains before rehabilitation, this research may assist land-managers and governments to make informed decisions regarding rehabilitation of these important ecosystems and provides a potential mechanism to fund future rehabilitation projects.
Data Availability Statement
The datasets generated for this study are available on request to the corresponding author.
Author Contributions
PIM and PC conceived the idea. AG and PC carried out the field work and statistical analyses. AG, ST-T, and PM carried out the laboratory analysis. AG and MD carried out the spatial analysis. All authors contributed to the interpretation and writing of the manuscript.
Funding
We acknowledge the support of the Australian Communities Foundation (Wanless Family Fund) and from an Australian Research Council Linkage Project (LP160100242). Funding was provided to PM and PIM through an Australian Research Council LIEF Project (LE170100219) and by the Generalitat de Catalunya (Grant 2017 SGR-1588). This work is contributing to the ICTA “Unit of Excellence” (MinECo, MDM2015-0552). The IAEA is grateful for the support provided to its Environment Laboratories by the Government of the Principality of Monaco.
Conflict of Interest
The authors declare that the research was conducted in the absence of any commercial or financial relationships that could be construed as a potential conflict of interest.
Acknowledgments
We thank Jami Butler and Clare Duncan for their contribution in the field.
Supplementary Material
The Supplementary Material for this article can be found online at: https://www.frontiersin.org/articles/10.3389/fmars.2020.00403/full#supplementary-material
References
Ackerman, J. T., Hartman, C. A., Herzog, M. P., Smith, L. M., Moskal, S. M., De La Cruz, S. E. W., et al. (2014). The Critical Role of Islands for Waterbird Breeding and Foraging Habitat in Managed Ponds of the South Bay Salt Pond Restoration Project, South San Francisco Bay, California. U.S. Geological Survey Open-File Report 2014-1263. Reston, VA: U.S. Geological Survey.
Adam, P. (2002). Saltmarshes in a time of change. Environ. Conserv. 29, 39–61. doi: 10.1111/gcb.14946
Adame, M. F., Hermoso, V., Perhans, K., Lovelock, C. E., and Herrera-Silveira, J. A. (2015a). Selecting cost-effective areas for restoration of ecosystem services. Conserv. Biol. 29, 493–502. doi: 10.1111/cobi.12391
Adame, M. F., Santini, N. S., Tovilla, C. A., Vázquez-Lule, C. L., and Guevara, M. (2015b). Carbon stocks and soil sequestration rates of tropical riverine wetlands. Biogeosciences 12, 3805–3818.
Appleby, P. G., and Oldfield, F. (1978). The calculation of lead-210 dates assuming a constant rate of supply of unsupported 210Pb to the sediment. CATENA 5, 1–8.
Arias-Ortiz, A., Masqué, P., Garcia-Orellana, J., Serrano, O., Mazarrasa, I., Marbà, N., et al. (2018). Reviews and syntheses: 210Pb-derived sediment and carbon accumulation rates in vegetated coastal ecosystems – setting the record straight. Biogeosciences 15, 6791–6818.
Ball, D., and Blake, S. (2001). Seagrass Mapping of Port Phillip Bay. Marine and Freshwater Resources Institute Report N39. Queenscliff: Victoria.
Barbier, E. B., Hacker, S. D., Kennedy, C., Koch, E. W., Stier, A. C., and Silliman, B. R. (2011). The value of estuarine and coastal ecosystem services. Ecol. Monogr. 81, 169–193.
Boon, P. I. (2012). Coastal wetlands of temperate eastern Australia: will Cinderella ever go to the ball? Mar. Freshw. Res. 63, 845–855.
Boon, P. I., Allen, T., Brook, J., Carr, G., Frood, D., Hoye, J., et al. (2011). Mangroves and Coastal Saltmarsh of Victoria: Distribution, Condition, Threats and Management. Bendigo, VIC: Department of Sustainability and Environment.
Brett Lane and Associates Pty Ltd (2009). Operational Manual Cheetham Wetlands, Volume 1: Ecological Values Report No. 9025. Hawthorn East, VIC: Brett Lane and Associates Pty Ltd.
Burden, A., Garbutt, A., and Evans, C. D. (2019). Effect of restoration on saltmarsh carbon accumulation in Eastern England. Biol. Lett. 15:20180773. doi: 10.1098/rsbl.2018.0773
Contreras-Cruzado, I., Infante-Izquierdo, M. D., Márquez-García, B., Hermoso-López, V., Polo, A., Nieva, F. J. J., et al. (2017). Relationships between spatio-temporal changes in the sedimentary environment and halophytes zonation in salt marshes. Geoderma 305, 173–187.
Curado, G., Grewell, B. J., Figueroa, E., and Castillo, J. M. (2014). Effectiveness of the aquatic halophyte Sarcocornia perennis spp. perennis as a biotool for ecological restoration of salt marshes. Water AirSoil Pollut. 225:2108.
Curado, G., Rubio-Casal, A. E., Figueroa, E., Grewell, B. J., and Castillo, J. M. (2013). Native plant restoration combats environmental change: development of carbon and nitrogen sequestration capacity using small cordgrass in European salt marshes. Environ. Monit. Assess. 185, 8439–8449. doi: 10.1007/s10661-013-3185-4
Davidson, N. C. (2014). How much wetland has the world lost? Long-term and recent trends in global wetland area. Mar. Freshw. Res. 65, 934–941. doi: 10.1016/j.marpolbul.2008.12.008
Duarte, C. M., Losada, I. J., Hendriks, I. E., Mazarrasa, I., and Marbà, N. (2013). The role of coastal plant communities for climate change mitigation and adaptation. Nat. Clim. Change 3, 961–968. doi: 10.1371/journal.pone.0044727
Ecology Australia (2012). Western Treatment Plan: Results of Monitoring of Saltmarsh Colonisation of the Western Lagoon to Mid 2012. Fairfield VIC: Ecology Australia PTY Ltd.
Gallego-Tévar, B., Grewell, B. J., Futrell, C. J., Drenovsky, R. E., and Castillo, J. M. (2019). Interactive effects of salinity and inundation on native Spartina foliosa, invasive S. densiflora and their hybrid from san francisco estuary. California. Ann. Bot. 125, 377–389. doi: 10.1093/aob/mcz170
Ghent, M. (2004). The Saltmarshes of the Southern Coast of Victoria: Floristic Composition, Variation and Distribution. Parkville VIC: University of Melbourne.
Harris, D., Horwath, W., and Kessel, C. (2001). Acid fumigation of soils to remove carbonates prior to total organic carbon or carbon-13 isotopic analysis. Soil Sci. Soc. Am. J. 65, 1853–1856.
Howard, J., Hoyt, S., Isensee, K., Telszewski, M., and Pidgeon, E. (2014). “Coastal blue carbon: methods for assessing carbon stocks and emissions factors in mangroves, tidal salt marshes, and seagrasses,” in Conservation International, Intergovernmental Oceanographic Comission of UNESCO, (Arlington, VA: International Union for Conservation of Nature).
Kelleway, J., Saintilan, N., Macreadie, P., and Ralph, P. (2016). Sedimentary factors are key predictors of carbon storage in SE Australian Saltmarshes. Ecosystems 19, 865–880.
Kelleway, J. J., Saintilan, N., Macreadie, P. I., Baldock, J. A., and Ralph, P. J. (2017). Sediment and carbon deposition vary among vegetation assemblages in a coastal salt marsh. Biogeosciences 14, 3763–3779.
Krishnaswamy, S., Lal, D., Martin, J. M., and Meybeck, M. (1971). Geochronology of lake sediments. Earth Planet. Sci. Lett. 11, 407–414.
Laegdsgaard, P. (2006). Ecology, disturbance and restoration of coastal saltmarsh in Australia: a review. Wetlands Ecol. Manag. 14, 379–399.
Macreadie, P. I., Ollivier, Q. R., Kelleway, J. J., Serrano, O., Carnell, P. E., Ewers Lewis, C. J., et al. (2017). Carbon sequestration by Australian tidal marshes. Sci. Rep. 7:44071. doi: 10.1038/srep44071
Mcleod, E., Chmura, G. L., Bouillon, S., Salm, R., Björk, M., Duarte, C. M., et al. (2011). A blueprint for blue carbon: toward an improved understanding of the role of vegetated coastal habitats in sequestering CO2. Front. Ecol. Environ. 9:552–560.
Moreno-Mateos, D., Power, M. E., Comín, F. A., and Yockteng, R. (2012). Structural and functional loss in restored wetland ecosystems. PLoS Biol. 10:e1001247. doi: 10.1371/journal.pbio.1001247
Osland, M. J., Spivak, A. C., Nestlerode, J. A., Lessmann, J. M., Almario, A. E., Heitmuller, P. T., et al. (2012). Ecosystem development after mangrove wetland creation: plant-soil change across a 20-year chronosequence. Ecosystems 15, 848–866.
Pendleton, L., Donato, D. C., Murray, B. C., Crooks, S., Jenkins, W. A., Sifleet, S., et al. (2012). Estimating global “Blue Carbon” emissions from conversion and degradation of vegetated coastal ecosystems. PLoS One 7:e43542. doi: 10.1371/journal.pone.0043542
Purnell, C., Peter, J., and Clemens, R. (2015). Shorebird Population Monitoring within Gulf St Vincent: July 2014 to June 2015 Annual Report. Melbourne: BirdLife Australia.
Rogers, K., Knoll, E. J., Copeland, C., and Walsh, S. (2016). Quantifying changes to historic fish habitat extent on north coast NSW floodplains. Australia. Regional Environ. Change 16, 1469–1479.
Rogers, K., Saintilan, N., and Copeland, C. (2012). Modelling wetland surface elevation dynamics and its application to forecasting the effects of sea-level rise on estuarine wetlands. Ecol. Model. 244, 148–157.
Rogers, K., Saintilan, N., and Woodroffe, C. D. (2014). Surface elevation change and vegetation distribution dynamics in a subtropical coastal wetland: implications for coastal wetland response to climate change. Estuar. Coast. Shelf Sci. 149, 46–56.
Saintilan, N., Rogers, K., Mazumder, D., and Woodroffe, C. (2013). Allochthonous and autochthonous contributions to carbon accumulation and carbon store in southeastern Australian coastal wetlands. Estuar. Coast. Shelf Sci. 128, 84–92.
Sanchez-Cabeza, J. A., Masqué, P., and Ani-Ragolta, I. (1998). 210Pb and210Po analysis in sediments and soils by microwave acid digestion. J. Radioanal. Nucl. Chem. 227, 19–22.
Serrano, O., Lovelock, C. E., Atwood, T. B., Macreadie, P. I., Canto, R., Phinn, S., et al. (2019). Australian vegetated coastal ecosystems as global hotspots for climate change mitigation. Nat. Commun. 10:4313. doi: 10.1038/s41467-019-12176-8
Sonneman, J., Jordan, B., Breen, P., and Daly, C. (2008). Melbourne: Habitat Enhancement Function Design Report.
Ullman, R., Bilbao-Bastida, V., and Grimsditch, G. (2013). Including Blue Carbon in climate market mechanisms. Ocean Coast. Manag. 83, 15–18.
Wegscheidl, C. J., Sheaves, M., McLeod, I. M., Hedge, P. T., Gillies, C. L., and Creighton, C. (2017). Sustainable management of Australia’s coastal seascapes: a case for collecting and communicating quantitative evidence to inform decision-making. Wetlands Ecol. Manag. 25, 3–22.
Wylie, L., Sutton-Grier, A. E., and Moore, A. (2016). Keys to successful blue carbon projects: lessons learned from global case studies. Mar. Policy 65, 76–84.
Keywords: additionality, carbon accumulation, coastal, restoration, salt ponds, saltmarsh, wetland ecosystems
Citation: Gulliver A, Carnell PE, Trevathan-Tackett SM, Duarte de Paula Costa M, Masqué P and Macreadie PI (2020) Estimating the Potential Blue Carbon Gains From Tidal Marsh Rehabilitation: A Case Study From South Eastern Australia. Front. Mar. Sci. 7:403. doi: 10.3389/fmars.2020.00403
Received: 09 March 2020; Accepted: 11 May 2020;
Published: 29 May 2020.
Edited by:
Michelle Jillian Devlin, Centre for Environment, Fisheries and Aquaculture Science (CEFAS), United KingdomReviewed by:
Jesús Manuel Castillo, University of Seville, SpainTracy Quirk, Louisiana State University, United States
Copyright © 2020 Gulliver, Carnell, Trevathan-Tackett, Duarte de Paula Costa, Masqué and Macreadie. This is an open-access article distributed under the terms of the Creative Commons Attribution License (CC BY). The use, distribution or reproduction in other forums is permitted, provided the original author(s) and the copyright owner(s) are credited and that the original publication in this journal is cited, in accordance with accepted academic practice. No use, distribution or reproduction is permitted which does not comply with these terms.
*Correspondence: Peter I. Macreadie, cC5tYWNyZWFkaWVAZGVha2luLmVkdS5hdQ==