- 1Tvärminne Zoological Station, Faculty of Biological and Environmental Sciences, University of Helsinki, Helsinki, Finland
- 2Baltic Sea Centre, Stockholm University, Stockholm, Sweden
- 3Novia University of Applied Sciences, Vaasa, Finland
The exchange between the water column and the seafloor is a complex process, and is particularly intensive in the shallow waters of highly productive coastal areas, where the temporal variability in the inputs of pelagic organic matter will determine many aspects of the benthic community structure. However, few studies have focused on the seasonality of inputs of organic matter to the seafloor, and on the consequent dynamics and time scales of response of benthic consumers. We conducted a 1-year study where we repeatedly sampled multiple organic compounds traditionally used as markers to study the link between the pelagic organic matter inputs and the seafloor, and the potential response of benthic macrofauna to seasonal trends in phytoplankton biomass. We simultaneously quantified the particulate organic matter in the water column, the sinking material and different seafloor compartments, and analyzed it for pigments, organic carbon and nitrogen content, C/N ratio, and stable isotopes. Seafloor sediment was also analyzed for total lipids, and the dominant macrobenthic species for isotopic signatures. Results showed a major deposition of fresh organic matter during the spring bloom followed by more degraded organic matter inputs during the late summer bloom and even lower quality of the organic matter reaching the seafloor during winter. Strong positive relationships between water column and sedimentary pigments suggest that phytoplankton was the main source of carbon to the seafloor. The isotopic signatures of the dominant macrobenthic species suggest a fast response to the organic matter inputs from the water column. However, different species responded differently to the deposition of organic matter. Macoma balthica and Marenzelleria spp. fed on more reworked and degraded sedimentary material, while Monoporeia affinis showed a shift in the feeding habits according to its life stage, with adult individuals feeding on fresher material than juveniles did. Our study highlights the seasonal variability of the benthic–pelagic coupling and the utility of a multi-marker approach to follow the temporal inputs of organic matter from the water column to the seafloor and benthic organisms.
Introduction
Nearshore pelagic conditions influence benthic coastal processes through an active and efficient transfer of organic matter from the water column to the seafloor (Kopp et al., 2015). Benthic–pelagic coupling plays a major role in determining the production, biological structure, and food web stability of both systems (Graf, 1992; Marcus and Boero, 1998; Griffiths et al., 2017). Phytoplankton production is the largest source of particulate organic material sinking to the seafloor and the most important driver of benthic communities, which are fuelled by this input. During phytoplankton blooms, a large part of the algal biomass is deposited on the seafloor providing an input of fresh organic matter to the benthos (Grebmeier et al., 1988; Tamelander et al., 2008). In coastal environments of the Baltic Sea, the euphotic zone can export up to 70% of the primary production to the benthic systems (Lignell et al., 1993). These organic matter depositions play a major role in the seasonal variation of seafloor biogeochemical cycles and food-web dynamics, which constitute important coastal ecosystem services (Kopp et al., 2015; Griffiths et al., 2017; Kauppi et al., 2017; Ehrnsten et al., 2019). Macrobenthic fauna often rapidly consume the organic matter inputs (Jumars and Wheatcroft, 1989; Witte et al., 2003) but our quantitative understanding remains limited.
The quantity and quality of the pelagic inputs on the seafloor largely depend on temporal variations in phytoplankton stocks and community composition, which can influence the diet patterns and growth of the benthic community (Nascimento et al., 2009; Zhang et al., 2015). In the Baltic Sea, the spring bloom is a well-documented event (Groetsch et al., 2016) that accounts for a large flux of matter from the pelagic habitat to benthic communities (Ólafsson and Elmgren, 1997) with a marked influence on the macrofauna activity and nutrient remineralization (Kauppi et al., 2017; Middelburg, 2018). In addition, the less regular and smaller late summer bloom entails an important secondary input to the benthos, although with smaller extent and lower nutritional quality than the spring bloom (Nascimento et al., 2009; Gustafsson et al., 2013).
Benthic macrofauna communities are particularly important contributors to the coastal filter and retention of the land-derived nutrients that reach the sea, and several studies have demonstrated the influence of benthic invertebrates on benthic–pelagic coupling and nutrient cycling in the Baltic Sea (Norkko et al., 2015; Gammal et al., 2017, 2019; Kauppi et al., 2017). However, coastal areas are under increasing pressure from multiple stressors, such as eutrophication and climate change that are affecting the timing, quality, and intensity of phytoplankton blooms (Griffiths et al., 2017; Tamelander et al., 2017). Changes in the occurrence and nature of the pelagic blooms will alter the duration, quality and quantity of organic material transfer to the benthos, and consequently the function of benthic macrofauna communities (Griffiths et al., 2017; Ehrnsten et al., 2020). Hence, there is a need to gain insights into the seasonal variations in organic matter inputs to understand the role of seafloor sediments in the coastal ecosystem functioning.
Specific organic compounds (e.g., pigments, lipids, carbon, and nitrogen content), have traditionally been used as markers for describing the quality and transformation pathways of organic matter (Le Guitton et al., 2015). Chlorophyll a can be used as indicator of “freshness” of the inputs of organic matter to the seafloor (Boon and Duineveld, 1996), and lipids constitute an important fraction of the total sedimentary organic matter that can be used to determine the quality of particulate organic matter (Christodoulou et al., 2009). Furthermore, stable isotopes (δ13C and δ15N) have typically been used to estimate the inputs of terrestrial and marine organic carbon to the sediment (Goñi et al., 2000; Fry, 2006), and to track the energy pathways from different sources of primary production through the pelagic and benthic food web (Tamelander et al., 2008; Morata et al., 2011; Karlson et al., 2014). A multiple marker approach can thus help to increase our understanding of the seasonal variation in benthic–pelagic coupling as these markers reflect inherent differences in the time scales over which they are integrated (Morata et al., 2008; Le Guitton et al., 2015).
It is well known that primary production varies greatly throughout the year with seasonality. Consequently, it is expected that the fresh inputs of organic matter to the seafloor will vary seasonally, thus affecting the uptake of the benthic fauna. In contrast to a relatively large number of microbial related studies, few studies have focused specifically on the dynamics and time scales of response of benthic macrofauna to pelagic production (Lessin et al., 2019). In fact, most of the studies on benthic–pelagic coupling, particularly in boreal environments such as the Baltic Sea, have been temporally limited to one or two seasons (Tamelander and Heiskanen, 2004; Morata et al., 2011; McTigue et al., 2015) or limited to only quantifying the inputs and not the actual potential uptake in the benthic system. Here, we study how the seasonal water column blooms will affect the sedimentation process by repeatedly sampling (i.e., 1–4 times per month) over almost an entire year to provide a better understanding of the nature and extent of benthic–pelagic coupling in coastal areas. We quantified the particulate organic matter in the water column, the sinking particulate matter and different seafloor compartments, and analyzed it for pigments, carbon and nitrogen content, C/N ratio, and stable isotopes. Seafloor sediment was also analyzed for total lipids, and the dominant macrobenthic species for isotopic signatures. We applied this multi-marker approach to quantify temporal variations in the deposition of pelagic organic matter and the potential benthic uptake of organic matter on the seafloor of the Baltic Sea.
Materials and Methods
Study Area
Storfjärden is situated on the Hanko Peninsula, SW coast of Finland (59° 51.331′ N, 23° 15.794′ E), and located at the entrance to the Gulf of Finland (Supplementary Figure S1). Storfjärden is an approximately 33-m-deep bay characterized by muddy sediments with direct connection to the open sea and can therefore experience large variation in hydrography due to upwelling events. Storfjärden can also be influenced by significant freshwater runoff (Tamelander and Heiskanen, 2004). All sampling was conducted from the hovercraft R/V Saga (February and March 2016, during conditions with sea ice) and from the R/V Saduria (from April to November 2016) (Table 1). We sampled once per month during autumn and winter (i.e., February, September, October, and November) and repeatedly (2–4 times per month) during spring and summer (i.e., from March to August) across the water column, the sinking particulate matter and the seafloor compartments (Table 1). All samples were frozen upon arrival in the laboratory and stored at −20°C until further analyses.
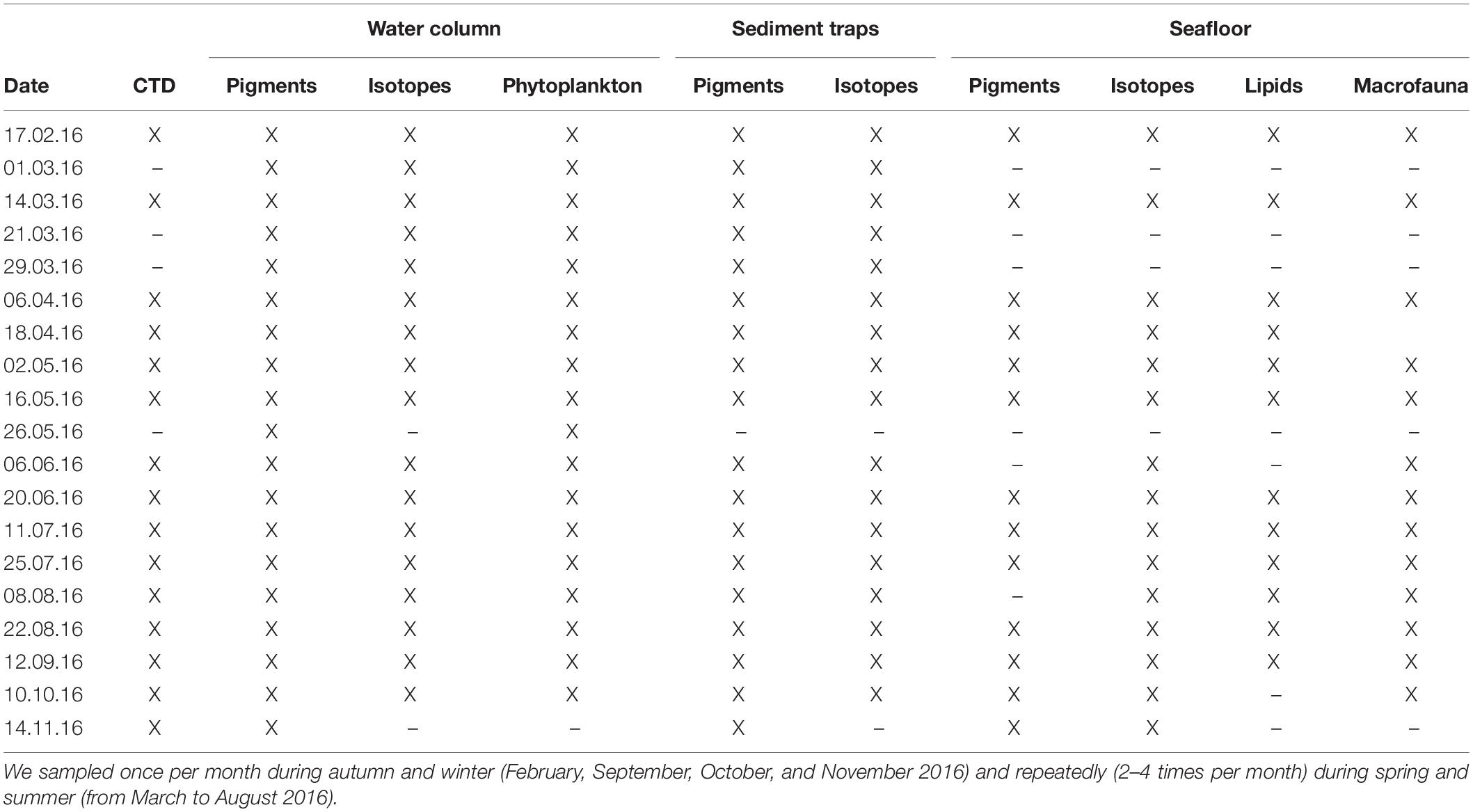
Table 1. Summary of the samples collected across the water column, the sinking particulate matter, and the seafloor compartments on the different sampling dates (X).
Water Column Sampling and Characterization
Vertical profiles of water column temperature and salinity were obtained at each sampling occasion by CTD casts (miniCTD Valeport®) from the surface to the seafloor (∼33 m). Samples for water column pigments and suspended particulate organic matter (SPOM) were collected intensively from February to November 2016 (see Table 1) at 0.5, 2, 4, and 7 m depths using a Ruttner-type sampler. The vertical export of pigments and POM was determined by means of short-term (24 h) deployment of sediment traps positioned at 20 m depth (Table 1). The traps (KC Maskiner og laboratorieudustyr) consisted of parallel cylinders with an inner diameter of 7.2 cm (height: diameter ratio of 6.25) mounted in a gimballed frame equipped with a vane. For deployment, the cylinders were filled with GF/F filtered seawater with salinity adjusted to 10 (ambient salinity ca. 6) by addition of NaCl. No preservatives were added to the traps. Degradation of OM inside the traps is considered negligible given the short exposure time.
Sediment Sampling
Seafloor sediment samples (n = 4) for pigments, POM, and lipids were collected randomly and regularly from February to November 2016 (Table 1). We used either a LIMNOS-Sediment Sampler (February and March) or a GEMAX corer for soft sediment (April to November) (Supplementary Figure S2), depending on the weather conditions. First, dissolved oxygen (DO, mg l–1) and temperature of the overlaying water retained in the sediment corers were measured (YSI ProOdo®) (Supplementary Figure S2). Then, each core was sliced in three layers to quantify the organic matter at different depths in the sediment (Supplementary Figure S2): layer 1 corresponding to the first top millimeters containing an organic fluff layer (∼0.4 cm), layer 2 corresponding to the next 1 cm (∼0.5–1.5 cm), and the deepest layer 3 (corresponding to ∼5–6 cm) as a contrasting comparison with the organic matter rich surface layers. Sediment from each layer was split into three similar fractions: for pigments (i.e., chlorophyll a and phaeopigments), for total lipids, and for C/N analyses. Fractions for pigment analyses were wrapped in foil immediately after slicing to avoid pigment degradation, and all samples were stored (−80°C) and then freeze-dried.
Benthic Macrofauna Sampling
Benthic macrofauna was collected from February to March using a LIMNOS-Sediment sampler (n = 3) and from April to October 2016 using a Van Veen grab sampler (n = 3) (Table 1). We sieved the sediment through a 0.5 mm sieve and the most common fauna, i.e., Macoma balthica (mollusc bivalve), Monoporeia affinis (amphipod crustacean), and Marenzelleria spp. (polychaete) were collected. These species are long-living motile or semi-motile suspension and deposit feeders inhabiting the uppermost 5 cm of the sediment with a key role on sediment bioturbation, organic matter burial, and nutrient recycling (Byrén et al., 2002; Josefson et al., 2012; Norkko et al., 2012; Kauppi et al., 2017). Adult and juvenile individuals of M. affinis were captured in different life stages during the sampling and separated accordingly (by visual size differences). The very abundant bottom-living midge larvae Chironomus spp. was also collected from the same sediment samples. The midge larvae constitute a large invertebrate biomass of the Baltic Sea and are considered a good indicator of eutrophication, organic rich sediments and oxygen depletion (Johnson et al., 1993).
Pigment Analysis: Water Column, Sediment Traps, and Sediment
Aliquots of the water column and sediment trap samples were filtered in triplicate on GF/F filters for determination of chlorophyll a (chl a) and phaeopigments (phaeo) from February to October 2016 (Table 1). Pigments were extracted in 96% ethanol over night at room temperature. The extracts were stored at −20°C for maximum 7 days after which fluorescence was measured on a Cary Eclipse Varian spectrofluorometer before and after acidification of the sample with 1N HCl (Parsons et al., 1984). Fluorescence readings were related to a standard of known concentration (Sigma C-6144). An integrated value of the total chlorophyll a and total phaeopigments (i.e., Tchl a, and Tphaeo; mg/m2) over the surface (0.5–7 m) water column was estimated to facilitate the interpretation of results and comparisons with the seafloor sediment. Numerically dominant phytoplankton species/groups were determined by microscopy of live samples using 200× magnification. The phytoplankton data are not quantitative, but it is expected that the numerically dominant groups identified will account for the highest biomass proportion.
Sediment subsamples (∼0.5 g) were placed into centrifuge tubes and 10 ml of 90% acetone was added to extract chl a and phaeo from the sediment. Tubes were stored at 4°C in darkness for 24 h and shaken periodically. Prior to analyses, the tubes were centrifuged at 3,000 rpm for 10 min at 20°C to ensure that absorption at 750 mm was <0.005. The supernatant (3 ml) was analyzed in a spectrophotometer (Shimadzu UV-VIS model) before and after acidification with two drops (100 μl) of 1N HCl to determine chl a and phaeo, respectively.
Lipid Extraction
Total lipids were extracted from sediment subsamples (∼0.2–0.5 g) by direct elution with a chloroform-methanol solution (2:1, v/v) and analyzed according to Zöllner and Kirsch (1962). This method is based on a spectrophotometric assay that allows analysis of small samples. It is based on the reaction of lipid degradation products with aromatic aldehydes, which results in a red coloration that can be quantified at 520 nm. In the case of POM, it is essential to extract the lipids from the bulk sample before performing the assay. This is because interference by non-lipid compounds (e.g., carbohydrates) results in high nonspecific absorbance after heating the sample in sulfuric acid and addition of the vanillin reagent, and thus in unreliable results (Gessner and Neumann, 2005). Quantification of total lipids was made using linear concentration-response regressions from known concentrations of standards solutions of pure cholesterol (SIGMA, C-8667) ranging from 0 to 15 μg ml–1.
Stable Isotope Analysis: δ13C and δ15N
Samples of water column SPOM and sediment trap samples were filtered in triplicate through pre-combusted (450°C, 4 h), HCl-washed, and pre-weighed GF/F filters. Filter samples were dried at 60°C over night, weighed for total mass (total particulate matter, TPM) and packed in tin capsules. Analyses of C and N content and stable isotope ratios were performed on a Europa Scientific ANCA-MS 20-20 15N/13C mass spectrometer.
Sediment samples were ground to fine powder with a mixer-mill (Retsch MM400) and subsamples (∼12 mg) were encapsulated in tin-cups. Total carbon (%TC) and δ13C determination, and total nitrogen (%TN) and δ15N analyses were performed by the Stable Isotope Facility from the University of California (UC Davis), using an elemental analyzer interfaced to a continuous flow isotope ratio mass spectrometer (IRMS). All stable carbon and nitrogen isotope values are reported in delta (δ) notation, in units of per mil (‰), where δ = [(Rsample/Rstandard) − 1] × 1,000. R = 13C/12C and 15N/14N, and the internationally accepted standards are Vienna Peedee belemnite (11,179 × 10–6 ± 20) and air (3,670 × 10–6 ± 40) for carbon and nitrogen, respectively (Sharp, 2015). The reproducibility on the bulk sediment samples was ±0.2‰, as determined by the standard deviation of multiple analyses. The presence of carbonates in this area is negligible, so acid was not added to remove the inorganic carbon of the samples. Immediately after the benthic sampling (see below), macrofauna individuals were kept alive overnight in filtered and aerated seawater (∼5°C) for evacuation of gut contents. Several macrofauna individuals of the same size were pooled into three replicate samples (when possible) to obtain sufficient material for stable isotopes analyses. Samples were dried at 60°C for 48 h, ground to fine powder and subsamples (∼1 mg) were encapsulated in tin-cups. Same procedure as for the sediment samples was used for the macrofauna samples. Note that acid was not added to remove the inorganic carbon of the samples, since the presence of carbonates in this area is negligible. In previous studies, we analyzed acidified and non-acidified samples, and we verified that differences were not significant (unpublished data).
Data Analysis
We explored POM quality in terms of organic matter degradation and microbial reworking based on the timescales of molecule degradation rates (Le Guitton et al., 2015): (1) Short time scales: pigments have higher degradation rates compared to lipids. Therefore, pigment derived parameters, such as the chl a/phaeo ratio can be used to assess the freshness of the organic matter (e.g., ratios >0.5 indicate fresh organic matter). (2) Intermediate time scales: lipids have a lower degradation rate compared to pigments, but a higher one compared to the bulk organic matter and amino acids (Veuger et al., 2012). (3) Long time scales: the C/N ratio can be used to represent POM quality on the long term. The C/N ratio increases from 6 to 7 for marine phytoplankton up to values as high as 10 with ongoing organic matter decomposition (Henrichs, 2005). Finally, sedimentary stable isotopes integrate signals at a longer time scale than other organic compounds (e.g., pigments and lipids). Stable isotopes provide time-integrated information on diet and habitat use. For instance, higher (or less negative) δ13C values (i.e., C-enriched) indicate that the organic material reaching the benthos is degraded (of marine origin) compared to lower δ13C values (i.e., C-depleted) that are indicative of fresher material (and more terrestrial origin) (Fry, 2006).
We examined potential correlations either for the same markers between different compartments (e.g., chl a in the water column vs chl a in the traps) or for different markers but in the same compartment [e.g., particulate organic carbon (POC) vs particulate organic nitrogen (PON) in the water column]. Normality was tested, and in case of compliance, parametric correlations (Pearson) were applied; otherwise, non-parametric correlations were used (Spearman). We applied generalized linear models (GzLM) to explore the temporal trend of the variable responses (pigments, carbon and nitrogen, total lipids, and stable isotopes) across the sedimentary layers. Time was considered as a continuous predictor co-variable and sediment layer was considered as a fixed factor (three levels) in the model. Model assumptions (i.e., homogeneity, normality, and independence) for the residuals were visually checked. Analyses were carried out in R 3.5.1 (R Development Core Team, 2019). All mean average values in the results section are presented as mean ± standard deviation (mean ± sd).
Results
Water Characterization
Salinity values were reasonably constant throughout the sampling dates (Supplementary Figure S3), with an average salinity of 6.04 ± 0.43 at ∼32 m, and 5.39 ± 0.41 at the sea surface (∼0.3 m). There was large seasonal variability in temperature, and an abrupt change in temperature (ΔT = 6.6°C between 0.3 and 32 m) was already recorded by mid-May (Supplementary Figure S3). This temperature change was maintained and increased in magnitude throughout the summer, reaching the maximum difference (ΔT = 13.3°C) at the end of July (Supplementary Figure S3). Temperature and DO in the bottom water immediately above the seafloor (measured in the cores) were negatively related (R = −0.59; p < 0.01) and showed a seasonal trend (Supplementary Figure S4). The lowest DO (6.3 ± 0.1 mg/l) and the highest temperature (13.1 ± 0.3°C) were recorded in late summer (Supplementary Figure S4).
Pigment Analysis: Chlorophyll a, Phaeopigments, and chl a/phaeo Ratio
Minimum values of the depth-integrated chlorophyll a (i.e., Tchl a) from the water column measurements were detected early in the year (Figure 1A). The first phytoplankton peak was recorded in spring (6 April) with a Tchl a concentration of 97.9 mg m–2 (Figure 1A and Supplementary Table S1), dominated by diatoms (Supplementary Table S2). A second peak (76.9 mg m–2) was recorded on 16 May (Figure 1A and Supplementary Table S1), and it was equally dominated by flagellates, diatoms, and dinoflagellates (Supplementary Table S2). The surface water Tchl a in June and July was on average 23 ± 8.6 mg m–2 (Supplementary Table S1). A third peak (57.6 mg m–2) dominated by dinoflagellates (Supplementary Table S2) was recorded in late summer (Figure 1A and Supplementary Table S1). There was a decrease in the pigment concentrations right after the late summer bloom (8 August), followed by a phytoplankton biomass increase that remained stable from September to November (Figure 1A). Total phaeopigments (Tphaeo) showed a similar pattern to Tchl a (Figure 1B), indicating that the blooms consisted of both fresh, but also degraded phytoplankton. However, the ratio values were >1 throughout the study (Figure 1C and Supplementary Table S1), indicating the “freshness” of the sinking material. The water column phytoplankton peaks were also captured in the traps during the same days except for the water column peak on 6 April that it was approximately 12 days later (i.e., 18 April in the traps) (Figure 1D and Supplementary Table S1). In the traps, phaeopigments showed a similar pattern to chl a (Figure 1E), and the ratio was always >1 (Figure 1F). We found a positive and significant correlation for chl a concentration between the water column and the traps (Table 2).
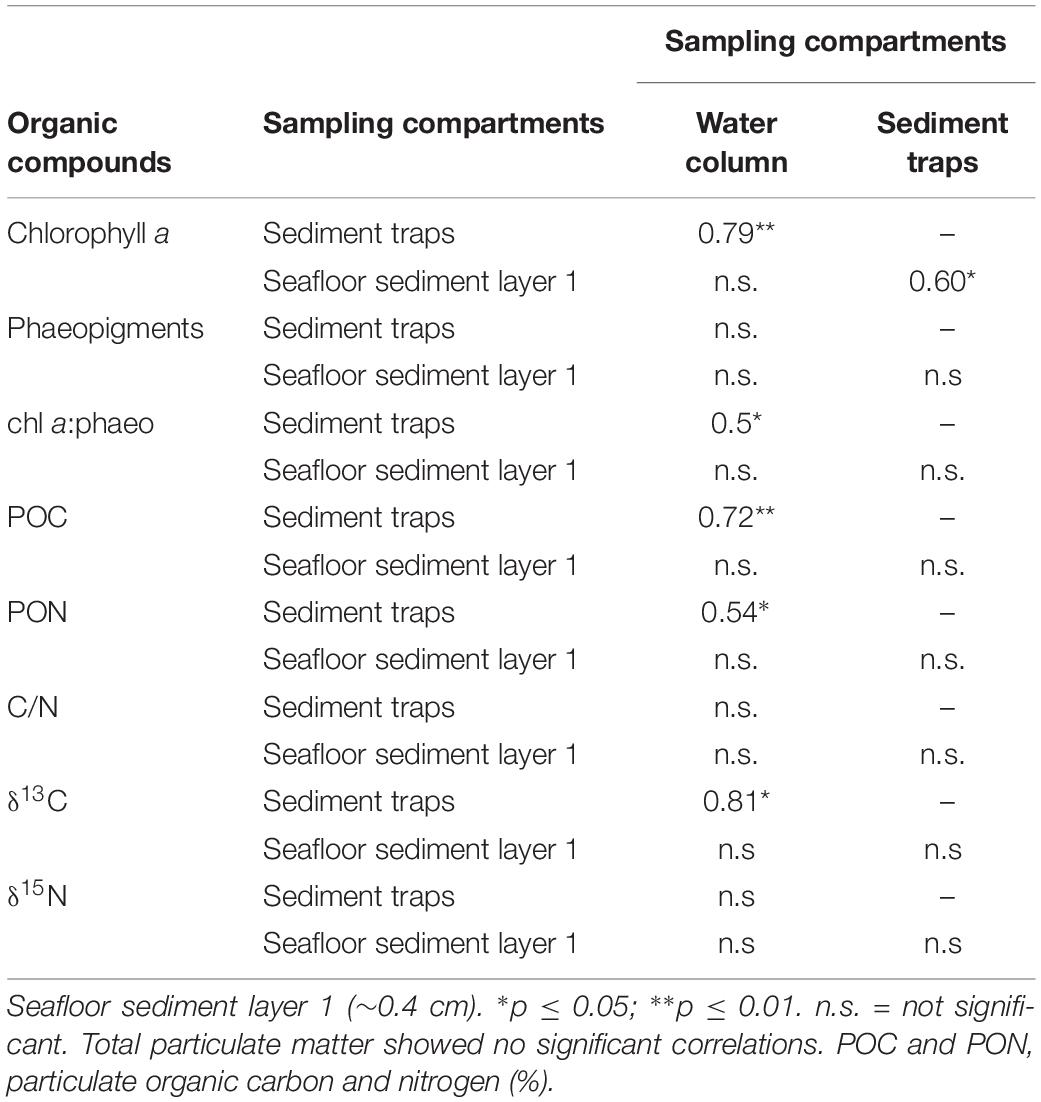
Table 2. Correlations (R) between the same organic compounds across the three sampling compartments (i.e., water column, sediment traps, and seafloor sediment surface) from February to November 2016 (see Table 1).
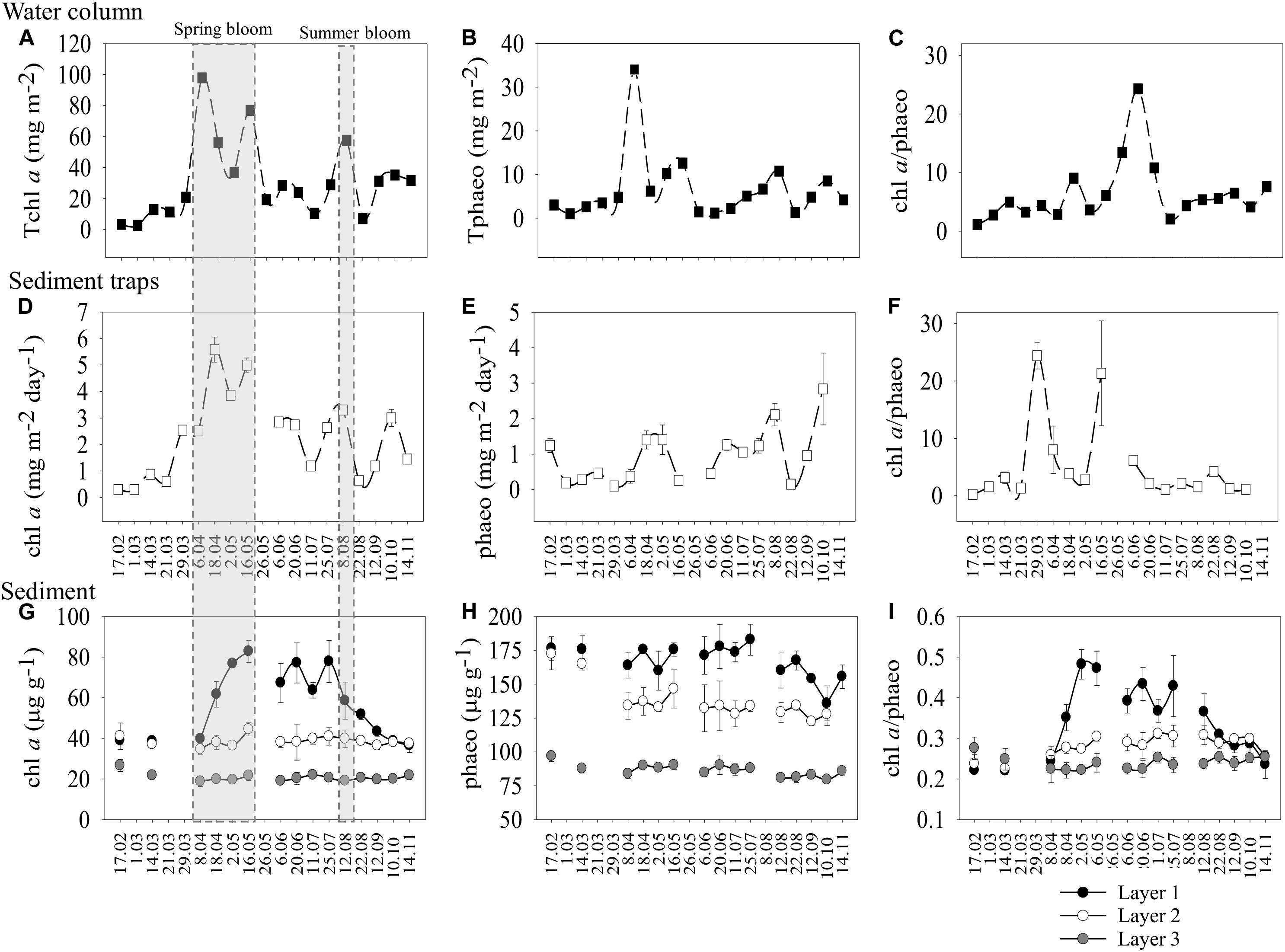
Figure 1. Temporal variations (from February to November 2016) of chlorophyll a and phaeopigment concentrations and the chlorophyll a/phaeopigment ratio in the depth-integrated water column particulate organic matter (0.5–7 m) (A–C), sediment traps (at 20 m) (D–F) and seafloor sediment (three different layers) (G–I) in Storfjärden bay (∼33 m deep). Gray shadow indicates bloom periods and a possible time lag between compartments. Mean values ± SD are shown for the traps and the sediment layers (chl a) and depth integrated values for the water column (Tchl a).
The surface sediment layer 1 showed significantly higher chl a concentrations (estimate = 65.90, t = 18.36; p < 0.01) than the deepest layers (Figure 1G). The maximum chl a in layer 1 was detected on 16 May (Figure 1G and Supplementary Table S3), following the pigment peaks detected in the water column (6 April; Figure 1A) and the traps (18 April; Figure 1D). The pigment concentration in layer 1 doubled from early April (39.75 μg g–1) to May (82.80 μg g–1), and then it was continuously high from May to the end of July, decreasing rapidly from August to November to values comparable to the previous winter (Figure 1G and Supplementary Table S3). Significant positive correlation was found between the chl a in layer 1 and the traps (Table 2). Unlike the water column and the traps, phaeo concentrations in the seafloor were higher than chl a concentrations, occasionally up to five times higher (Figure 1H), and the chl a/phaeo ratio was always lower than 0.5 (Figure 1I). However, the doubling of both chl a and chl a/phaeo ratio during spring suggests a large input of new organic material to the sediment (Figures 1G,H).
Particulate Organic Matter: POC, PON, and C/N Ratio
The highest value of TPM in the water column was recorded on 6 April (Figure 2A and Supplementary Table S4), concurrent with the peak of chl a (Figure 1A). The POC estimated from the TPM in the water column ranged between 2.5 and 13.9%, with maximum values reached on 6 April and 16 May (Figure 2A and Supplementary Table S4), correlating with chl a (Table 3). The PON correlated highly with the POC (Table 3), and we detected the highest values during spring (Supplementary Figure S5 and Supplementary Table S4). The lowest C/N ratio was recorded on 6 April (Figure 2B) coinciding with the spring diatom-dominated bloom (Figure 1A), and reflecting the input of nutritious matter rich in nitrogen. We found the highest C/N ratios on 17 February and 16 May (coinciding with the second spring bloom dominated by flagellates, dinoflagellates, and diatoms) (Figure 2B and Supplementary Table S4).
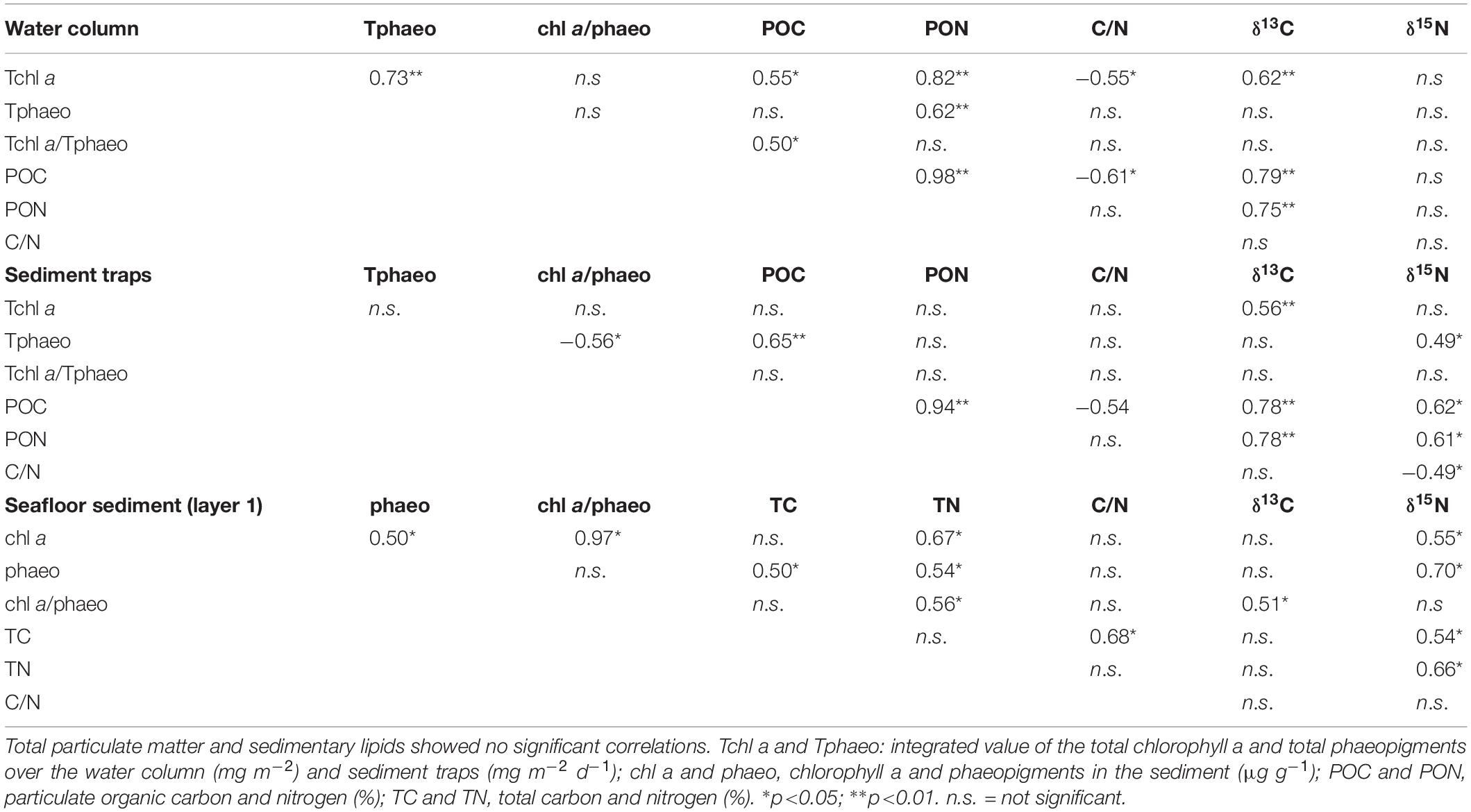
Table 3. Correlations (R) between the different organic compounds within the same sampling compartment (i.e., water column, sediment traps, and seafloor sediment surface).
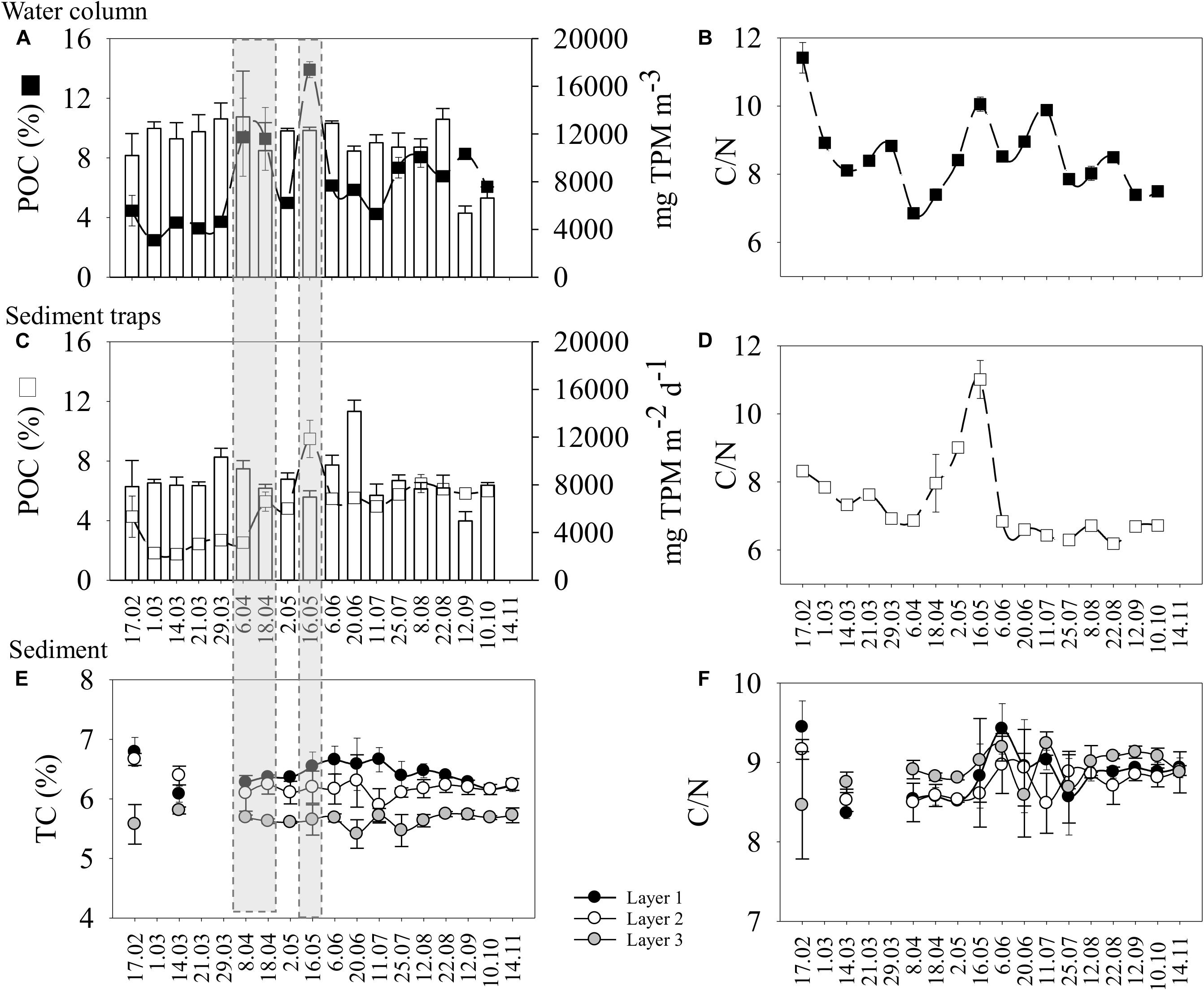
Figure 2. Temporal variation (from February to November 2016) of the particulate organic carbon content (% of the total particulate matter, TPM), and the molar C/N ratio in the water column (A,B) and sediment traps (C,D), and total carbon (%) and the molar C/N ratio from the seafloor sediment layers (E,F). Additionally, sedimentation of TPM in the water column (A) and sediment traps (C) was bar-plotted. Mean values ± SD are shown. Note that for the water column and sediment traps most dates only have one replicate (see Supplementary Table S4), and samples were not taken in November 2016.
The highest value of TPM in the traps was recorded on 20 June (Figure 2C and Supplementary Table S4). The POC% estimated from the TPM in the traps followed a similar pattern to that described for the water column (Figures 2A,C and Table 2). The POC% in the material collected by the traps (on average 5.3 ± 2.2%) started increasing in April and we registered the maximum POC%, and C/N, on 16 May (Figures 2C,D and Supplementary Table S4). There was a time lag between the water column and the sediment traps regarding the POC (Figures 2A,C) similar to the time lag for chl a concentrations (Figures 1A,D). A spring peak of POC was recorded in the traps on 18 April (Figure 2C), 12 days after the first POC% peak was recorded in the water column (Figure 2A). The maximum POC% was simultaneously detected in the water samples and sediment traps on 16 May (Figures 2A,C). The PON% from the traps showed as similar trend as the POC% (Table 3), with the highest value detected on 16 May (Supplementary Figure S5 and Supplementary Table S4). Most of the sampling dates showed a C/N < 8 (i.e., relatively fresh material), particularly from June onward (Figure 2D). The highest C/N ratio was recorded on 16 May, and it was comparable to the peak recorded in the water column on that date (Figures 2B,D).
The total sedimentary carbon (TC) and nitrogen (TN) sedimentary values showed a narrow range of fluctuation over time compared to the water column or the sediment trap ranges (Figure 2E and Supplementary Figure S5). The highest sedimentary TC (estimate = 6.51, t = 103.2; p < 0.001) and TN (estimate = 0.87, t = 145.5; p < 0.001) were always recorded in layer 1 (Figure 2E and Supplementary Figure S5). A decrease in TC and TN with sediment depth is expected because of the remineralization of the organic matter. Sedimentary TC increased (estimate = 0.02, t = 4.13; p < 0.001) and TN decreased (estimate = −0.02, t = −5.63; p < 0.001) over time in layer 1 (Figure 2E and Supplementary Figure S5). Positive correlations were observed between TN and the pigments (i.e., chl a and phaeo), and between TC and phaeo (Table 3). We detected the maximum value in the seafloor C/N ratio (on average 8.8 ± 0.4; Supplementary Table S5) on 6 June (Figure 2F), while the peak in the water column and the traps occurred on 16 May (Figures 2B,D).
Total Lipids in the Seafloor
There were no significant differences in the total lipid content between sediment layers (Figure 3; p > 0.05, GzLM). However, the lipid content increased over time for all sediment layers (i.e., layer 1: estimate = 3.60, t = 2.37; p < 0.05; layer 2: estimate = 2.85, t = 1.44; p < 0.05; layer 3: estimate = 5.1, t = 1.44; p < 0.001) with several peaks registered in summer (Figure 3 and Supplementary Table S6). After summer, there was a general decrease in the lipid content with a (highly variable) maximum at the end of the sampling period across layers (Figure 3 and Supplementary Table S6).
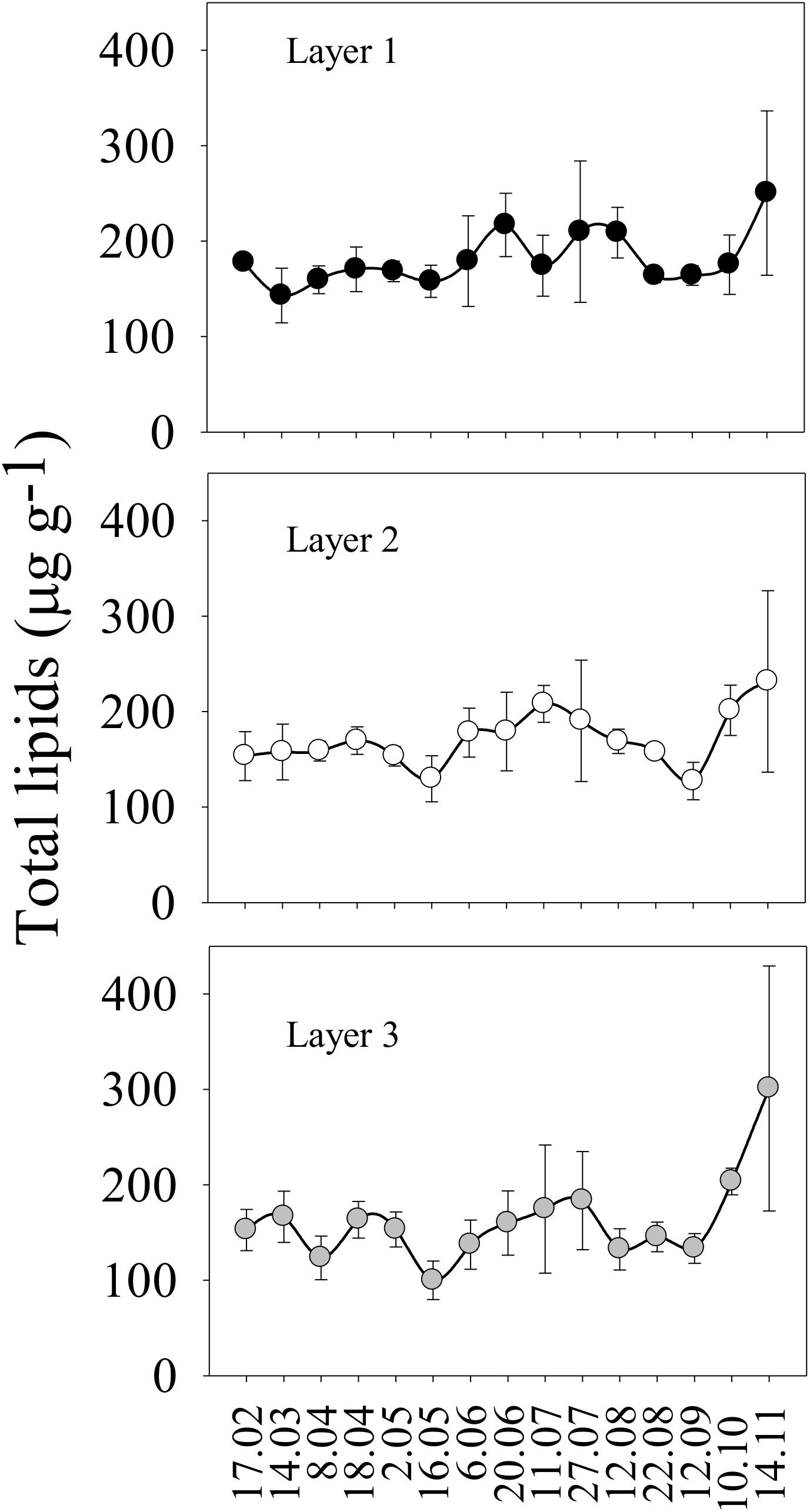
Figure 3. Temporal dynamic (from February to November 2016) of total lipids concentration (μg/g of sediment) in the seafloor sediment per layer (layer 1: 0–0.4 cm, layer 2: 0.5–1.5 cm, and layer 3: 5–6 cm). Mean ± SD are shown.
Stable Isotopes in the Water Column, Sediment Traps, and Sediment
We observed a seasonal shift of the δ13C values of water column SPOM, increasing from the lowest winter values to the highest spring peak on 16 May (Figure 4A and Supplementary Table S7). The maximum variation of the carbon isotope (Δδ13C) was approximately 10‰ (Figure 4A), indicating a large enrichment of carbon from winter to spring. We also detected two small peaks in April and August (Figure 4A) corresponding to peaks in pigment concentrations (Figure 1A). There were significant correlations between δ13C and Tchl a, POC and PON within the water column (Table 3). The δ15N of SPOM showed a variable range of values over the year (i.e., 0.40–6.09‰), with the maximum value (6.09 ± 2.48‰) registered on 2 May (Figure 4A and Supplementary Table S7).
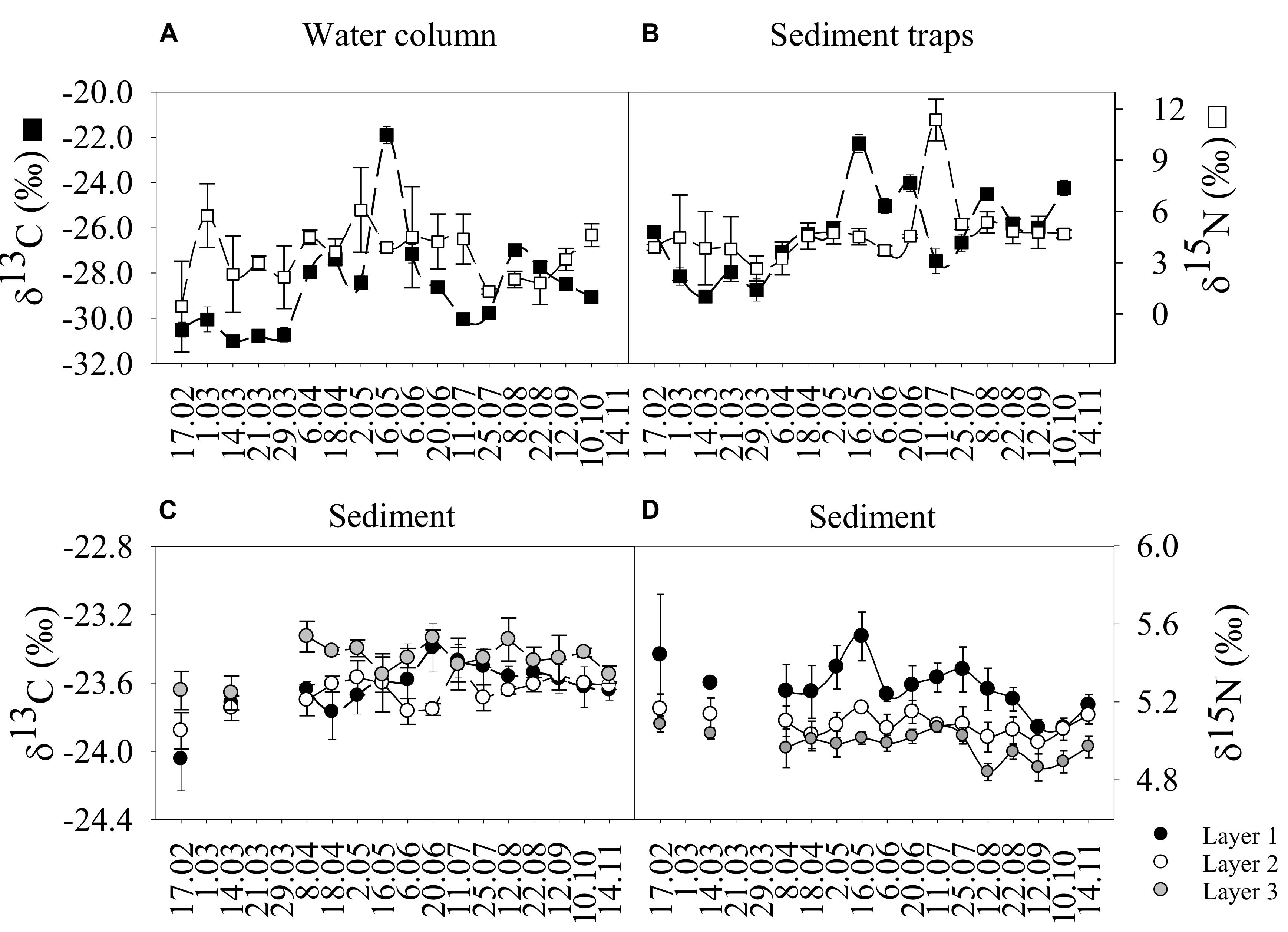
Figure 4. Temporal variation (from February to November 2016) of the δ13C and δ15N in the water column (A), sediment traps (B), and seafloor sediment layers (C,D). Mean values ± SD are shown. Note that samples for the water column and sediment traps were not taken in November 2016.
The δ13C values of the sediment traps showed a similar pattern to that described for the water column (Figures 4A,B), reflecting a coupling between compartments (Figure 5 and Table 2). Similarly, δ13C values were significantly correlated with chl a, POC and PON in the traps (Table 3). The maximum δ13C of sediment trap material was recorded on 16 May (Figure 4B and Supplementary Table S7). In addition, we detected a second δ13C peak on 20 June (Figure 4B). The maximum variation of carbon (Δδ13C) in the traps was ∼7‰ with a minimum recorded on 29 March (Figure 4B and Supplementary Table S7). The δ15N values of the traps showed a narrow temporal fluctuation, with a maximum peak (11.36 ± 1.22‰) registered on 11 July (Figure 4B and Supplementary Table S7).
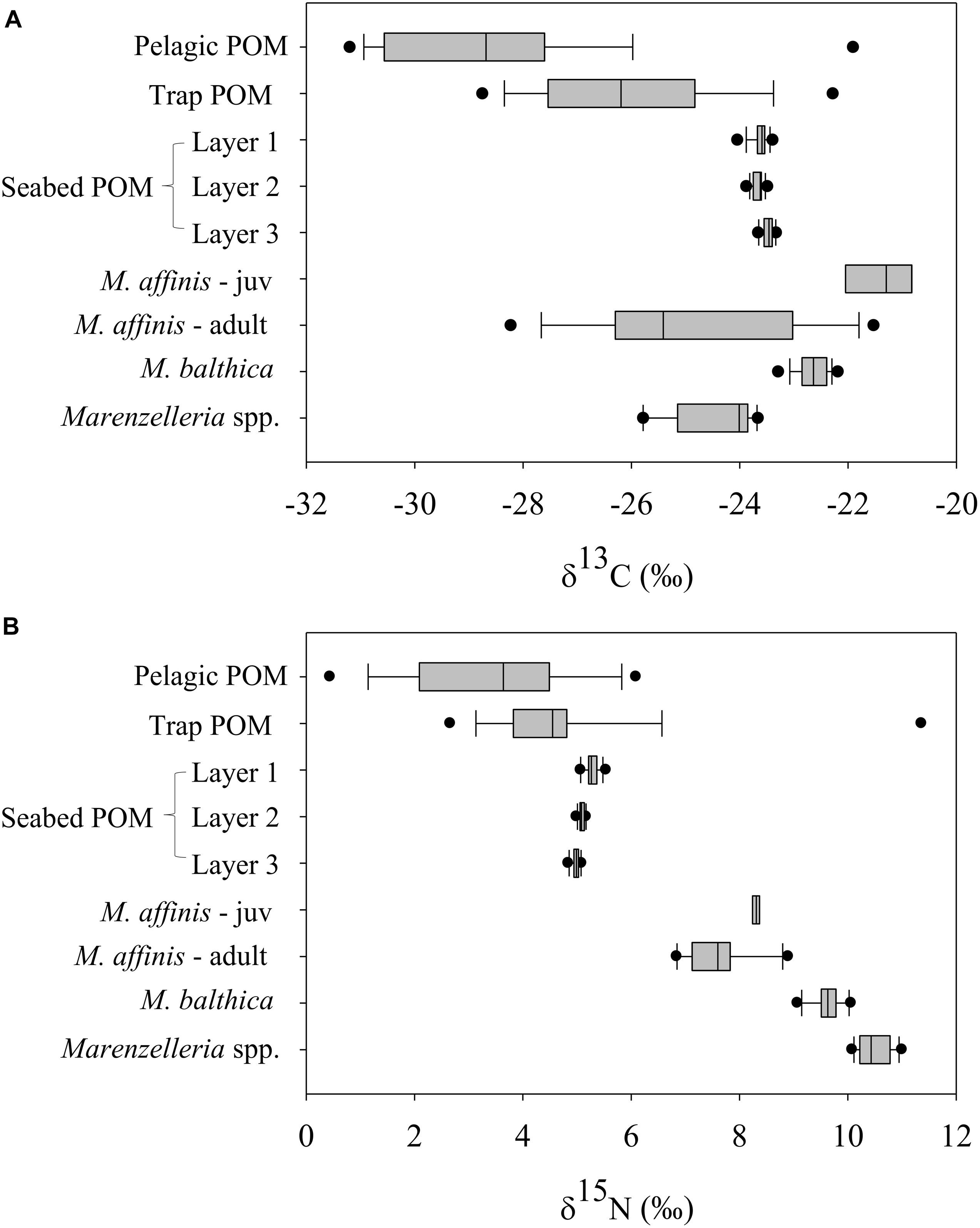
Figure 5. Median and percentiles (10th, 25th, 75th, and 90th) with error bars of the stable isotopes δ13C (A) and δ15N (B) for the main particulate organic sources collected from the different compartments (SPOM from the water column, POM from the sediment traps, and seafloor POM from the different sediment layers) and the main benthic consumers (Macoma balthica, Marenzelleria spp., and Monoporeia affinis in both adult and juvenile stages) over time (from February to November 2016).
The δ13C sedimentary values showed significant differences between layers (layer 1 = layer 2 > layer 3; p < 0.001) (Figure 4C). The δ13C values in layer 1 (estimate = 0.02, t = 4.13; p < 0.001) and layer 2 (estimate = 0.01, t = 2.71; p < 0.001) increased significantly from winter to early summer (20 June) tracking the organic matter inputs (Figure 4C and Supplementary Table S8). The maximum temporal variation (Δδ13C) in the superficial layer was low ∼1 ‰ (Figure 4C). Similar to the traps (Figure 4B), we detected a δ13C peak on 20 June (Figure 4C and Supplementary Table S8). Therefore, the sedimentary δ13C was more enriched in spring (of the organic matter that reaches the seafloor over time concurrent with the bloom-onset) than in winter. In contrast, δ15N showed a very narrow (Δδ15N = 0.47‰) fluctuation over time (Figure 4D and Supplementary Table S8). There were significant differences between layers (layer 1 > layer 2 > layer 3; p < 0.001) with a maximum value (5.54 ± 0.12‰) registered on 16 May for layer 1. The sedimentary δ15N values decreased over time from higher values in winter/spring toward lighter values in summer/autumn for layer 1 (estimate = −0.02, t = −5.63; p < 0.001) and layer 3 (estimate = −0.01, t = −3.1; p < 0.01) (Figure 4D). Layer 1 showed significant correlations between δ15N and chl a, phaeo, TC, and TN (Table 3).
Benthic Macrofauna Uptake: Stable Isotope Analysis
In general, benthic species tracked the changes in the sedimentary δ13C signal (p ≤ 0.05), particularly M. balthica (R = 0.52), Marenzelleria spp. (R = 0.56), and M. affinis (R > 0.7) (Figures 5, 6). Chironomus spp. did not reflect the change in δ13C of any of the potential sources and its isotopic composition was on average much lower (i.e., −42.1 ± 3.3‰) than any other species or source (Figure 6 and Supplementary Tables S7–S9), likely due to a diet that includes carbon derived from biogenic methane (Grey and Deines, 2005; Hershey et al., 2006). Both, M. balthica and Marenzelleria spp. experienced a gradual increase in δ13C over time comparable to the increase on the seafloor (Figures 4C, 6). Selected sampling dates spanning specific pre-bloom, peak-bloom, and post-bloom periods in the water column show a clear variation in the δ13C values of M. balthica and Marenzelleria spp. (Figure 7). High δ13C values (i.e., less negative) of these two species coincided with the highest δ13C values detected on 20 June in the seafloor (Figure 7 and Supplementary Tables S8, S9), and with their δ13C signatures very close to each other on that date (Δδ13C: layer 1 vs. M. balthica = 1‰ and layer 1 vs. Marenzelleria spp. = 0.28 ‰) (Figure 7 and Supplementary Figure S6). The maximum Δδ13C recorded was less than ∼1‰ in the case of M. balthica and ∼2‰ in the case of Marenzelleria spp. for the whole study period (Figures 6, 7). The isotopic composition of M. affinis was masked by ontogenetic differences in the diet of this species. Thus, adult individuals of M. affinis fed on lower δ13C values (i.e., more negative) than the juveniles (Figures 5–7). The average δ13C value of the adults (−24.88 ± 1.96‰) was closer to the average isotopic signature in the traps (−26.2 ± 1.71‰) than to other sources (Figure 5), and increased slightly over time in parallel to the sinking of organic matter (Figures 6, 7 and Supplementary Table S9). The juvenile individuals, present during summer, consumed more reworked material (i.e., higher δ13C) (Figures 6, 7 and Supplementary Table S9). The δ13C value (−21.39 ± 0.62‰) of the juveniles was closer to the seafloor sediment signature (−23.62 ± 0.15‰) than to any other source (Figures 5–7), although slightly more enriched (i.e., direct feeding). Both, M. balthica and Marenzelleria spp. showed higher δ15N values across all sampling dates compared to M. affinis (Figure 7 and Supplementary Table S9). However, the highest δ15N value registered in the traps on 11 July (Figure 4D and Supplementary Table S7) was also detected in the juvenile forms of M. affinis (Figures 6, 7 and Supplementary Table S9).
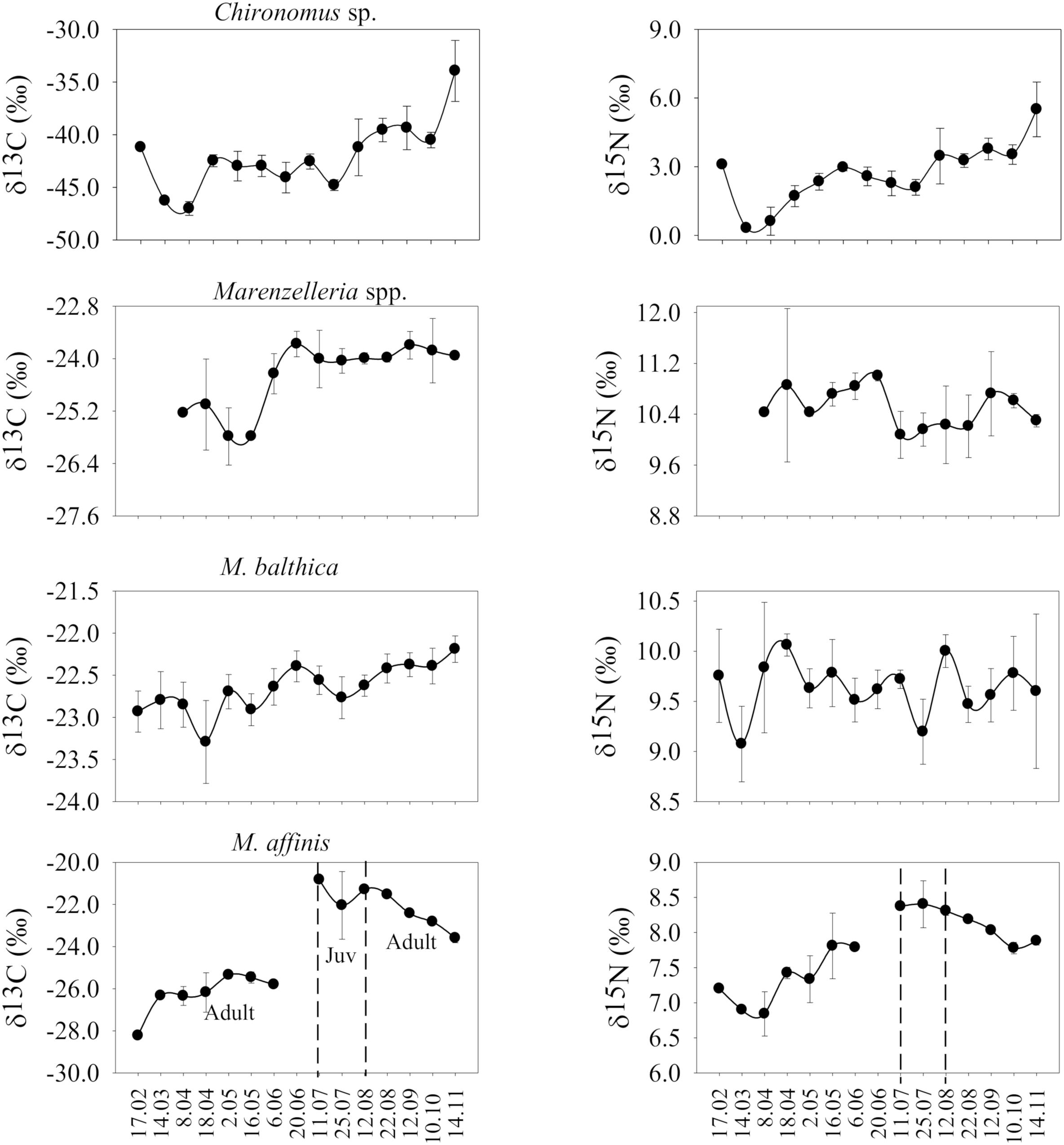
Figure 6. Mean (±sd) values of the stable isotope δ13C signals estimated from the four benthic macroinvertebrate species (deposit feeders: Chironomus spp., Marenzelleria spp., Macoma balthica, and Monoporeia affinis in adult and juvenile stages) sampled over time (February to November 2016).
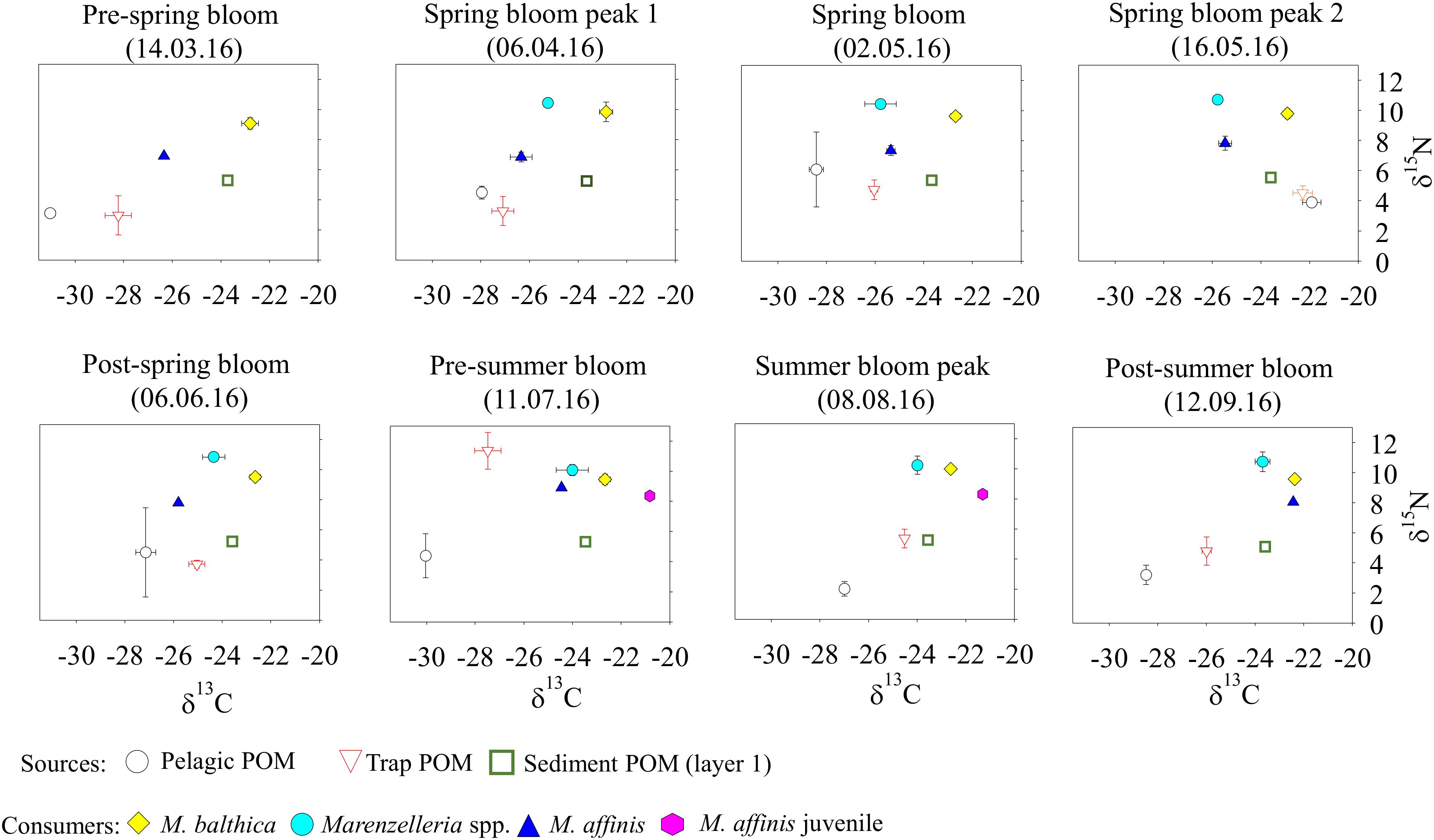
Figure 7. Isotopic distribution of the organic sources collected from the different compartments (POM from the water column, POM from the sediment traps, and seafloor POM from surface layer 1) and the main benthic consumers (Macoma balthica, Marenzelleria spp., and Monoporeia affinis in both adult and juvenile stages) over selected sampling dates encompassing specific periods of pre-bloom, peak-bloom, and post-bloom. Mean values ± SD are shown.
Discussion
We studied the seasonal variability in the pelagic food supply to the benthos by identifying the quantity and quality of the organic matter deposition occurring during a year in Storfjärden bay (Finland). Here, we measured simultaneously a combination of organic compounds traditionally used as markers across the water column, the sinking particulate matter, the sediment layers and the dominant macrobenthic species. The use of multiple markers, covering multiple characteristic time scales, was key to understanding how seasonal changes in organic matter inputs can affect the benthic community. An understanding of the origin and fate of the organic matter deposited on the seafloor is critical for unraveling the dynamics of the benthic–pelagic coupling in such a highly productive coastal ecosystem.
Organic Matter Inputs Over Short-Time Scales: Chlorophyll a and Phaeopigments
We observed that the largest water column increases in the pigment concentrations occurred three times during the year; i.e., twice in spring (one peak in April and a second peak in May) and once in summer (a lower peak in August). The positive correlations between the SPOM (i.e., Tchl a) and the chl a concentrations collected by the traps as sinking particulate matter, and also between the sinking material and the sedimentary chl a, suggest that local primary production contributes significantly to the inputs of fresh organic matter to the benthic compartment. We estimated that the chl a concentrations in spring were ∼35 times (water column), 2.5 times (sinking matter), and ∼2 times (seafloor) higher than in winter (maximum values). The increase in the sedimentary chl a/phaeo ratio from winter to summer indicates both an increase in the quantity (i.e., higher biomass) and in the quality (i.e., fresher material) of the matter reaching the seafloor. Therefore, organic matter inputs were lower in autumn and winter, and the composition was more degraded compared to spring, indicated by the lower chl a/phaeo ratio of the traps. These results support the tight nature of benthic–pelagic coupling in seasonally variable coastal areas from high latitudinal locations (Oliver and Dayton, 1977; Morata et al., 2008; McTigue et al., 2015) such as the Baltic Sea (Tamelander et al., 2017).
Sedimentary pigments not only give an estimate of the amount of pelagic production exported to the benthos, but can also indicate how this organic matter degrades on the seafloor (McTigue et al., 2015). The sedimentary chl a/phaeo ratio did not exceed 0.5 during the year, indicating that despite fresher material arriving from the water column, the bulk sediment matter was still degraded compared to the high chl a/phaeo ratios (>0.5) in the water column and sinking material. We identified a time lag of about 12–15 days (based on our sampling frequency) between the pelagic phytoplankton peak and the subsequent seafloor deposition. We suggest that fresh material arrives at the seafloor during spring, which improves the quality of the organic matter, and that is reflected in the increase of the sedimentary chl a/phaeo ratio (∼twofold) from winter to spring. This is supported by observations of phytoplankton viability showing that a large proportion of the sinking phytoplankton (collected by sediment traps) consists of intact and living cells (Elovaara et al., 2020). Despite this fresh input in chl a from the water column, the amount of phaeopigments accumulated in the seafloor was much higher than the chl a concentration (up to five times). We expect that Storfjärden, a typical muddy accumulation bay, can accumulate large amounts of degraded matter (including degradation products of chlorophyll) for long periods in the sediments (Morata et al., 2008). Therefore, fresh inputs of chl a are quickly metabolized either by bacterial or macrofaunal activity and/or diluted in the large bulk of sedimentary phaeopigments once they reach the seafloor (Boon and Duineveld, 1996; Boon et al., 1998; Morata et al., 2011). The chl a in the sediment surface layer (i.e., layer 1) was higher and more variable than in the other layers (i.e., layers 2 and 3), suggesting a limited sedimentary transport of pigments between layers and a rapid consumption of the sedimentary matter (Renaud et al., 2007). Recent evidence of food-limitation in shallow coastal areas highlights the importance of sedimentary organic matter as a key food source for the benthic system that may buffer the dependence on seasonal inputs from the water column (Ehrnsten et al., 2019).
Organic Matter Inputs Over Intermediate Time Scales: Lipids as Sedimentary Markers
The positive correlation between sedimentary chl a and sedimentary lipids suggests a phytodetritus origin of the organic matter supporting a benthic detritus-based food web (Kürten et al., 2013). Based on our results, fresh matter arrived at the seafloor, readily available to be exploited by the benthic fauna relatively soon after the two spring blooms (April and May). Pigments have higher degradation rates compared to lipids (Le Guitton et al., 2015), and they were quickly consumed in the first sediment layer. Sedimentary lipids across the three sediment layers changed nearly simultaneously through time, indicating a strong bioturbation effect and longer degradation rates compared to pigments. Note that on 16 May a fresh input of matter in the form of chl a occurred, and it was transmitted almost simultaneously through the water column, the sinking particulate matter and the seafloor. However, the total lipid concentration showed low values at that specific date, and it was only several days later that an increase for total lipids was detected in the sediment. These results are in line with our expectations, since lipids are used as an intermediate temporal scale marker (Le Guitton et al., 2015).
Organic Matter Inputs Over Long-Time Scales: C/N Ratio and Stable Isotopes
We detected two peaks of POC and PON in both the water column and the sediment traps during the spring blooms (i.e., April and May). Sedimentation of TPM was quite stable during most of the year, and a clear peak was observed in the sediment traps after the spring blooms (20 June), likely related to a resuspension event caused by vertical mixing during summer, when a salinity increase between ∼20 m and the seafloor, and a decreasing surface temperature were simultaneously recorded (Supplementary Figure S3). The low POC content of the trapped material captured in June also indicates that the high early summer TPM was likely caused by resuspension of material from the bottom (Tamelander and Heiskanen, 2004). The strong positive relationship between TN and chl a in the seafloor could be reflecting the sinking of matter in the form of fresh biomass that increases the nitrogen content in the sediment. Similarly, a positive relationship between TC and phaeo could be indicative of the degradation of the organic matter in the seafloor.
Our results show C/N ratio values ranging from 6 to 8 for most of the study period (water column, sinking particulate matter, and seafloor). These values indicate local inputs of organic matter typically associated with marine phytoplankton (Tamelander and Heiskanen, 2004; Morata et al., 2008). Our results also indicate the relative freshness of that material, since C/N ratio values ∼8 are still considered “fresh” material (Tahey et al., 1994), while ratio values as high as 10 are interpreted as decaying marine organic matter (Henrichs, 2005). The C/N ratios of the traps indicate that the major phytoplankton deposition events differed in terms of nutritional quality. Thus, deposition during the first part of the spring bloom (April) was characterized by low C/N ratios (<8) characteristic of growing phytoplankton, and diatom dominated blooms that provide fresh supply of matter (Tamelander and Heiskanen, 2004). The second deposition in May was related to a bloom shared by diatoms and cyst-forming dinoflagellates such as Peridiniella catenata (Elovaara et al., 2020) with higher C/N (∼11). The input of cysts and dinoflagellate-derived detritus possibly lowered the quality of the sinking material in May. Although the C/N ratio indicates phytoplankton as the main source of food during summer, the lower chl a/phaeo ratio suggests a more degraded composition of the input from the dinoflagellate-dominated summer bloom.
The δ13C signal provides a long-term indication of potential food quality that is complementary to the C/N ratio. High δ13C values of the water column during the productive period are related to the accumulation of phytoplankton biomass in the water column reflected in a positive relationship between δ13C and chl a concentration (Tamelander et al., 2009). In our study, δ13C values for the water column suspended biomass suggest a trimodal pattern with a small peak in April followed by a maximum in May, both in connection with the spring bloom, and a third small peak in late summer (Figure 4). This pattern agrees well with the pattern observed for the sinking organic matter (i.e., sediment traps). However, these peaks were not so obvious in the seafloor, where a δ13C maximum was detected on 20 June in the surface layer. Our study shows that the organic matter was more 13C-enriched near the seafloor (i.e., traps or seafloor) than in the water column (Figure 8). Higher δ13C values (on average) in the sediment compared to the water column (∼4‰) indicates a more degraded composition of the sedimentary material as POM sinks toward the seafloor (McTigue et al., 2015). Several reasons can be responsible for the organic matter enrichment in the seafloor. For instance, microbial degradation can cause δ13C-enrichment of the organic matter, but the extent that the microbial community processes organic matter prior to macrofaunal assimilation is poorly understood despite its previously postulated role in benthic food web dynamics (Lovvorn et al., 2005). The slightly more 13C-enriched sedimentary matter might also be related to an occasional resuspension event by bottom currents that become part of the near-bottom SPOM (McTigue et al., 2015). The resuspension of sedimentary material, which commonly occurs in the shallow coastal areas of the Baltic Sea (Blomqvist and Larsson, 1994), can also contribute to the sedimentary enrichment as it provides material that has been previously remineralized and reworked. Remineralized organic matter is known to be a main energy pathway, and together with primary producer-derived pelagic food, it is a fundamental food supply for some species of the macrobenthic community (Kürten et al., 2013). We hypothesize that the degradation of organic matter, causing increased base-line δ13C values occurs before benthic-faunal food web assimilation. Therefore, the peak of δ13C detected in the seafloor on 20 June, likely caused by a resuspension event as indicated by a vertical mixing flux (Supplementary Figure S3), is consistent with the simultaneous δ13C peaks in both the traps and in the seafloor during that date, and supported by the increase in TPM in the sediment traps (see above). Our results indicate that resuspension events are potentially important factors in seasonal benthic–pelagic organic matter cycling (Joensuu et al., 2020). However, we have not measured these events directly. We suggest that future studies need to include analysis of these events to obtain a better understanding of the seasonal variation in the organic matter transfer from the water column to the seafloor.
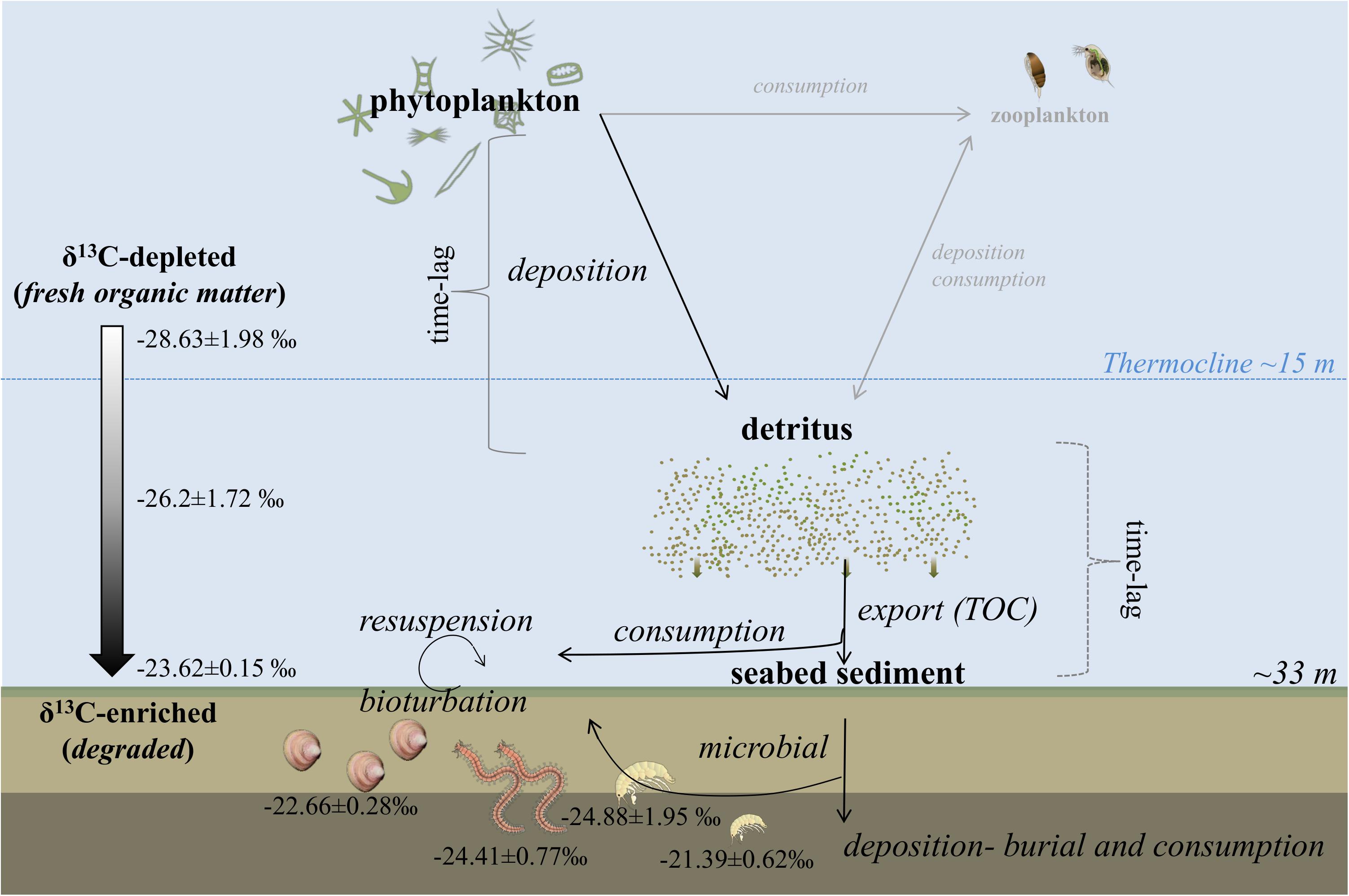
Figure 8. Conceptual diagram depicting the major pathways of organic matter assimilation from primary producers (i.e., phytoplankton) in the water column to higher trophic levels (i.e., deposit feeders) proposed for Storfjärden bay (Finland). The δ13C values are given as the temporal average obtained in this study (Supplementary Tables S7–S9). In light gray, we show those compartments not measured in this study (e.g., zooplankton). Images are courtesy of the Integration and Application Network, University of Maryland Center for Environmental Science (ian.umces.edu/symbols/).
The maxima seen in δ15N coincided, with some time lags, with the minima of δ13C across the three compartments (i.e., water column, sinking particulate matter, and seafloor) and vice versa (Rolff, 2000). In the water column, although several peaks of δ15N were registered from late winter to the end of the spring bloom period, the range of change was less defined and more variable than that seen in δ13C. The δ15N values of the traps showed a steady seasonal increase to a maximum peak on 11 July followed by a rapid decline, reflecting temporal shifts in surface water productivity. Low seston δ15N values have typically been attributed to isotopic fractionation during the assimilation of nitrate by phytoplankton, while dramatic increases of δ15N values have been associated with microbial degradation activity (Ostrom et al., 1997; Morata et al., 2008). In this context, lighter δ15N values would indicate a higher portion of “fresh” algae in the sedimenting material, whereas heavier values would indicate a higher portion of aged or biologically processed organic matter (Fuentes et al., 2013). Sedimentary δ15N values showed a steady seasonal decrease from winter toward summer explained by the degradation of the increasing contribution of fresh sedimenting organic matter.
Benthic Macrofauna Uptake Using Stable Isotopes as Long-Term Footprints
Long-term temporal studies on benthic response to food inputs have showed that the benthic community is capable of rapid increases in activity (i.e., days to weeks) as an immediate response to bloom sedimentation (Graf, 1992; Renaud et al., 2008; Kauppi et al., 2017). In the Baltic Sea, the isotopic signal of the blooms have been successfully used as tracers of the dietary origin of benthic consumers (Karlson et al., 2014), and no time lag in the response between producers and invertebrate consumers have been observed on a monthly basis (Nordström et al., 2009). In our study, temporal variation of the δ13C in the benthic fauna reproduced well the isotopic pattern of the seafloor, suggesting a tight seasonal coupling within the benthic compartment. Macrobenthic species clearly responded fast to the organic matter inputs from the water column in accordance with previous studies (Witte et al., 2003). However, there was a species-specific response to the deposition of organic matter, showing a different dependency on fresh inputs from phytoplankton blooms. Previously, a number of isotope tracer experiments suggested potential intra- and interspecific trophic resource selectivity among the benthos (Ólafsson et al., 1999; Rossi et al., 2004; Byrén et al., 2006; Karlson et al., 2014). Our field based study showed a similarity in δ13C between the seafloor sediment and the macrobenthic deposit feeders (on average), particularly M. balthica and Marenzelleria spp. (Figure 8). The isotopic signature of the sediment traps was also closer to these benthic species compared to the δ13C signature of the water column (Figure 8). The organic matter that reaches the seafloor can be resuspended and reworked, and efficiently exploited by the benthic fauna. Thus, M. balthica expressed δ13C values closer to the sediment surface values (offset by 0.5–1‰), suggesting a constant uptake of this type of material, and hence lower dependency on freshly deposited material. Deposit-feeding invertebrates such as M. balthica can switch feeding behavior (i.e., from deposit to suspension feeding) according to the availability of food (Kamermans, 1994; Rossi et al., 2004). Storfjärden is a deep aphotic muddy basin where microphytobenthos, a natural fresh food source for M. balthica with high δ13C values (Rossi et al., 2004), is not readily available due to the lack of light. However, there is a large microbial community to feed on. In addition, M. balthica is known to stimulate the growth of its own food source (i.e., bacteria and diatoms) by “gardening” (Reise, 1983). Moreover, M. balthica is an efficient bioturbator facilitating rapid degradation of organic matter by reworking the surface extensively, and potentially stimulating microbial communities (Volkenborn et al., 2012) with strong effects on the exchange of inorganic nutrient fluxes (Norkko et al., 2013). Marenzelleria spp. possibly exploit fresh inputs during the early stage of the phytoplankton bloom, indicated by a small (∼1‰) offset to the trap material in April, but likely shifting to deposit feeding from the sediment surface following the spring bloom (Figure 7 and Supplementary Figure S6). These polychaete species are known to dominate the benthic community after the spring bloom, and their high biological activity can explain a significant high proportion of the seafloor nutrient regeneration of this study area (Kauppi et al., 2017). Moreover, Marenzelleria spp. have also been shown to efficiently subduct deposited spring bloom organic matter to deeper sediment layers due to their bioturbation activities (Josefson et al., 2012), hence potentially contributing to building a fresh food bank in the sediment for other species. Some studies have indicated that benthic fauna assimilate a carbon source that is 13C-enriched relative to phytoplankton (Lovvorn et al., 2005; McTigue et al., 2015). It has been proposed that resuspended material often dominates the diet of benthic suspension feeders in the Baltic Sea, as opposed to the traditional view that phytoplankton are their primary food source (Lauringson et al., 2014).
It has been suggested that local primary production, despite having a striking effect on the growth of the M. affinis individuals, is not enough to subsidize populations of M. affinis in the Baltic Sea, and that resuspension and lateral transport of organic material have a key role in supporting the amphipod population. In our study, the isotopic signature of M. affinis was dependent on the life stage of this species and the sampling period. Thus, juvenile individuals of this crustacean species, mainly present during summer, showed a more C-enriched signal compared to the seafloor sediment (on average) or to the other consumers (Figure 8). This suggests that juveniles of this species could be consuming more reworked material than the rest of the benthic species, likely after it has been reworked by the microbial community. Adult individuals of M. affinis expressed the most variable isotopic signal among all the species over time (Δδ13C ∼2‰). Mature individuals of M. affinis are known to have circadian migrations in the water column, while juveniles are more likely bound to the sediments (Donner and Lindström, 1980), which can explain their specific isotopic signatures. Thus, the δ13C signal of the adult amphipods can be allocated somewhere in between the sinking material and the seafloor signals (Figure 8). This result indicates that a mixture of both sources could be simultaneously exploited by this species at the adult stage. In general, adult individuals of M. affinis had lower δ13C values (i.e., more negative) than juveniles, suggesting that adults fed on fresher material than juveniles did. The smallest offset in δ13C values between M. affinis and the traps are coincident with the main spring bloom event and subsequent deposition, suggesting a fast uptake of newly deposited material from the diatom-dominated bloom. However, after the summer bloom and during autumn, early stage adults showed a similar isotope signal as juveniles, most likely due to the more degraded or reworked sedimentary material occurring later in the year. Our study monitored for a full year and with a very high frequency the seasonal variation of the transfer of organic matter from the water column to the seafloor using a range of tracers. However, we did not measure all the processes and components involved with the benthic–pelagic coupling, such as the significant contribution of zooplankton (Figure 8) to the organic matter cycling and vertical export (Lovvorn et al., 2005; Tamelander et al., 2008). Hence, there are many aspects related to benthic–pelagic coupling, including also, for example, food web interactions, resuspension dynamics, and microbial processes that need resolving for a more complete picture of these critically important processes.
Conclusion
Very few seasonal benthic–pelagic coupling studies have examined the temporal variability in the quantity and quality of the organic matter inputs from the water column to the surface coastal sediments. We sampled intensively a combination of organic compounds typically used as markers to track the response and carbon uptake of the dominant benthic macrofauna to the seasonal variability of the pelagic contribution to the shallow sediments of the Baltic Sea. We showed the importance of the spring blooms in the benthic–pelagic coupling and the secondary role of the summer bloom as a more degraded input to the benthos. In general, there was a direct link between the local pelagic phytoplankton inputs and the deposition of organic matter to the benthos. However, it was only several days later (i.e., 12–15 days based on our sampling frequency) that the spring bloom of fresh organic matter was detected in the sediment. Macrobenthic species responded fast to the organic matter inputs from the water column, but differed in terms of uptake of freshly deposited and reworked sedimentary material. Thus, M. balthica and Marenzelleria spp. exploited a larger proportion of more reworked and degraded sedimentary material as part of their diet, while changes in the feeding habits between the different life stages of M. affinis indicated that adults fed on fresher material than juveniles did. The occurrence and nature of the pelagic blooms are sensitive to human activities that will alter the duration, quality and quantity of organic material transfer to the benthos (Griffiths et al., 2017). The consequences of such changes for the seafloor communities are unknown, although we can expect that many benthic biological processes will be affected. It has recently been suggested that climate change can potentially weaken benthic–pelagic coupling in the future due to shifts in the composition of pelagic production and the reduction in POC export to the seafloor (Ehrnsten et al., 2020). These changes in the pelagic system will have direct consequences for benthic macrofaunal biomass and coastal ecosystem functioning. The multiple marker approach is a useful tool to track natural and human-induced changes in the seasonal pelagic organic matter supply to the seafloor, and to understand the ability of seafloor sediments and associated benthic consumers to adjust to such changes.
Data Availability Statement
All datasets generated for this study are included in the article/Supplementary Material.
Author Contributions
JN and AN designed the study. IR, PL-M, TT, JN, and AN collected the data. IR and PL-M analyzed data. IR and PL-M wrote a draft of the manuscript. All authors contributed to the revision of the work.
Funding
This study was funded by the BONUS COCOA project, which was supported by BONUS (Art 185), funded jointly by the EU and the Academy of Finland. Further funding was provided by research grants from The Academy of Finland (Project ID 294853) and the University of Helsinki and Stockholm University strategic fund for collaborative research (the Baltic Bridge initiative). TT was supported by a grant from the Swedish cultural heritage foundation and the Walter and Andrée de Nottbeck Foundation. This study has utilized research infrastructure facilities provided by FINMARI (Finnish Marine Research Infrastructure network, the Academy of Finland, Project ID 283417).
Conflict of Interest
The authors declare that the research was conducted in the absence of any commercial or financial relationships that could be construed as a potential conflict of interest.
Acknowledgments
We are grateful for the constructive comments made by the reviewers, which helped to improve the manuscript. We would like to thank Maria Degerlund for providing phytoplankton species data, the laboratory staff at Tvärminne Zoological Station, and the crew of R/V Saduria for assistance.
Supplementary Material
The Supplementary Material for this article can be found online at: https://www.frontiersin.org/articles/10.3389/fmars.2020.00404/full#supplementary-material
References
Blomqvist, S., and Larsson, U. (1994). Detrital bedrock elements as tracers of settling resuspended particulate matter in a coastal area of the Baltic Sea. Limnol. Oceanogr. 39, 880–896. doi: 10.4319/lo.1994.39.4.0880
Boon, A. R., and Duineveld, G. C. A. (1996). Phytopigments and fatty acids as molecular markers for the quality of near-bottom particulate organic matter in the North Sea. J. Sea Res. 35, 279–291. doi: 10.1016/s0077-7579(96)90084-8
Boon, A. R., Duineveld, G. C. A., Berghuis, E. M., and Van der Weele, J. A. (1998). Relationships between benthic activity and the annual phytopigment cycle in nearbottom water and sediments in the southern North Sea. Estuar. Coast. Shelf Sci. 46, 1–13. doi: 10.1006/ecss.1997.0264
Byrén, L., Ejdung, G., and Elmgren, R. (2002). Comparing rate and depth of feeding in benthic deposit-feeders: a test on two amphipods, Monoporeia affinis (Lindström) and Pontoporeia femorata Kröyer. J. Exp. Mar. Biol. Ecol. 281, 109–121. doi: 10.1016/s0022-0981(02)00441-0
Byrén, L., Ejdung, G., and Elmgren, R. (2006). Uptake of sedimentary organic matter by the deposit-feeding Baltic amphipods Monoporeia affinis and Pontoporeia femorata. Mar. Ecol. Prog. Ser. 313, 135–143. doi: 10.3354/meps313135
Christodoulou, S., Marty, J. C., Miquel, J. C., Volkman, J. K., and Rontani, J. F. (2009). Use of lipids and their degradation products as biomarkers for carbon cycling in the northwestern Mediterranean Sea. Mar. Chem. 113, 25–40. doi: 10.1016/j.marchem.2008.11.003
Donner, K. I., and Lindström, M. (1980). Sensitivity to light and circadian activity of Pontoporeia affinis (Crustacea. Amphipoda). Ann. Zool. Fennici. 17, 203–212. doi: 10.1007/bf00413923
Ehrnsten, E., Norkko, A., Müller-Karulis, B., Gustafsson, E., and Gustafsson, B. G. (2020). The meagre future of benthic fauna in a coastal sea – Benthic responses to recovery from eutrophication in a changing climate. Glob. Chang. Biol. 26, 2235–2250. doi: 10.1111/gcb.15014
Ehrnsten, E., Norkko, A., Timmermann, K., and Gustafsson, B. G. (2019). Benthic-pelagic coupling in coastal seas – Modelling macrofaunal biomass and carbon processing in response to organic matter supply. J. Mar. Syst. 196, 36–47. doi: 10.1016/j.jmarsys.2019.04.003
Elovaara, S., Degerlund, M., Franklin, D. J., Kaartokallio, H., and Tamelander, T. (2020). Seasonal variation in estuarine phytoplankton viability and its relationship with carbon dynamics in the Baltic Sea. Hydrobiologia 847, 2485–2501. doi: 10.1007/s10750-020-04267-1
Fuentes, N., Güde, H., Wessels, M., and Straile, D. (2013). Allochthonous contribution to seasonal and spatial variability of organic matter sedimentation in a deep oligotrophic lake (Lake Constance). Limnologica. 43, 122–130. doi: 10.1016/j.limno.2012.06.003
Gammal, J., Järnström, M., Bernard, G., Norkko, J., and Norkko, A. (2019). Environmental context mediates biodiversity–ecosystem functioning relationships in coastal soft-sediment habitats. Ecosystems. 22, 137–151. doi: 10.1007/s10021-018-0258-9
Gammal, J., Norkko, J., Pilditch, C. A., and Norkko, A. (2017). Coastal hypoxia and the importance of benthic macrofauna communities for ecosystem functioning. Estuar. Coast. 40, 457–468. doi: 10.1371/journal.pone.0049795
Gessner, M. O., and Neumann, P. T. M. (2005). “Total lipids,” in Methods to study Litter decomposition: A Practical Guide, eds M. A. S. Graça, F. Bärlocher, and M. O. Gessner (Berlin: Springer).
Goñi, M. A., Yunker, M. B., Macdonald, R. W., and Eglinton, T. I. (2000). Distribution and sources of organic biomarkers in arctic sediments from the mackenzie river and beaufort shelf. Marin. Chem. 71, 23–51. doi: 10.1016/s0304-4203(00)00037-2
Graf, G. (1992). Benthic-pelagic coupling: a benthic view. Oceanogr. Mar. Biol. 30, 149–190. doi: 10.1007/s11356-015-4997-2
Grebmeier, J., Feder, H., and McRoy, C. (1988). Pelagic-benthic coupling on the shelf of the northern bering and chukchi seas. I. Food supply source and benthic biomass. Mar. Ecol. Prog. Ser. 48, 57–67. doi: 10.3354/meps048057
Grey, J., and Deines, P. (2005). Differential assimilation of methanotrophic and chemoautotrophic bacteria by lake chironomid larvae. Aquat. Microb. Ecol. 40, 61–66. doi: 10.3354/ame040061
Griffiths, J. R., Kadin, M., Nascimento, F. J. A., Tamelander, T., Törnroos, A., Bonaglia, S., et al. (2017). The importance of benthic-pelagic coupling for marine ecosystem functioning in a changing world. Glob. Chang. Biol. 23, 2179–2196. doi: 10.1111/gcb.13642
Groetsch, P. M. M., Simis, S. G. H., Eleveld, M. A., and Steef, W. M. P. (2016). Spring blooms in the Baltic Sea have weakened but lengthened from 2000 to 2014. Biogeosciences 17, 4959–4973. doi: 10.5194/bg-13-4959-2016
Gustafsson, O., Gelting, J., Andersson, P., Larsson, U., and Roos, P. (2013). An assessment of upper ocean carbon and nitrogen export fluxes on the boreal continental shelf: a 3-year study in the open Baltic Sea comparing sediment traps, 234Th proxy, nutrient, and oxygen budgets. Limnol. Oceanogr Meth. 11, 495–510. doi: 10.4319/lom.2013.11.495
Henrichs, S. M. (2005). “Organic matter in coastal marine sediments,” in The Global Coastal Ocean: Multiscale Interdisciplinary Processes, Vol. 13, eds A. R. Robinson and K. H. Brink (Cambridge, MA: Harvard University Press), 129–163.
Hershey, A. E., Beaty, S., Fortino, K., Kelly, S., Keyse, M., and Luecke, C. (2006). Stable isotope signatures of benthic invertebrates in arctic lakes indicate limited coupling to pelagic production. Limnol. Oceanogr. 51, 177–188. doi: 10.4319/lo.2006.51.1.0177
Joensuu, M., Pilditch, C. A., and Norkko, A. (2020). Temporal variation in resuspension potential and associated nutrient dynamics in shallow coastal environments. Estuar. Coast. (in press). doi: 10.1007/s12237-020-00726-z
Johnson, R. K., Wiederholm, T., and Rosenberg, D. M. (1993). “Freshwater biomonitoring using individual organisms, populations and species assemblages of benthic macroinvertebrates,” in Freshwater Biomonitoring and Benthic Macroinvertebrates, eds D. M. Rosenberg and V. H. Resh (Atlanta, GA: Chapman Hall), 488.
Josefson, A. B., Norkko, J., and Norkko, A. (2012). Burial and decomposition of plant pigments in surface sediments of the Baltic Sea: role of oxygen and benthic fauna. Mar. Ecol. Prog Ser. 455, 33–49. doi: 10.3354/meps09661
Jumars, P. A., and Wheatcroft, R. A. (1989). “Response of benthos to changing food quality and quantity, with a focus on deposit feeding and bioturbation,” in Productivity of the Ocean: Present and Past, eds W. H. Berger, V. S. Smetacck, and G. Wefer (Hoboken, NJ: John Wiley & Sons Limited).
Kamermans, P. (1994). Similarity in food source and timing of feeding in deposit- and suspension-feeding bivalves. Mar. Ecol. Prog. Ser. 104, 63–75. doi: 10.3354/meps104063
Karlson, A. M. L., Gorokhova, E., and Elmgren, R. (2014). Nitrogen fixed by cyanobacteria is utilized by deposit-feeders. PLoS One 9:e104460. doi: 10.1371/journal.pone.0104460
Kauppi, L., Norkko, J., Ikonen, J., and Norkko, A. (2017). Seasonal variability in ecosystem functions: quantifying the contribution of invasive species to nutrient cycling in coastal ecosystems. Mar. Ecol. Prog. Ser. 572, 193–207. doi: 10.3354/meps12171
Kopp, D., Lefebvre, S., Cachera, M., Villanueva, M. C., and Ernande, B. (2015). Reorganization of a marine trophic network along an inshore–offshore gradient due to stronger pelagic– benthic coupling in coastal areas. Prog. Oceanogr. 130, 157–171. doi: 10.1016/j.pocean.2014.11.001
Kürten, B., Frutos, I., Struck, U., Painting, S. J., Polunin, N. V. C., and Middelburg, J. J. (2013). Trophodynamics and functional feeding groups of North Sea fauna: a combined stable isotope and fatty acid approach. Biogeochemistry 113, 189–212. doi: 10.1007/s10533-012-9701-8
Lauringson, V., Kotta, J., Orav-Kotta, H., and Kaljurand, K. (2014). Diet of mussels mytilus trossulus and dreissena polymorpha in a brackish nontidal environment. Mar. Ecol. 35(Suppl.1), 56–66.
Le Guitton, M., Soetaert, K., Sinninghe Damsté, J. S., and Middelburg, J. J. (2015). Biogeochemical consequences of vertical and lateral transport of particulate organic matter in the southern North Sea: a multiproxy approach. Estuar. Coast. Mar. Sci. 165, 117–127. doi: 10.1016/j.ecss.2015.09.010
Lessin, G., Bruggeman, J., McNeill, C. L., and Widdicombe, S. (2019). Time scales of benthic macrofaunal response to pelagic production differ between major feeding groups. Front. Mar. Sci. 6:15. doi: 10.3389/fmars.2019.00015
Lignell, R., Heiskanen, A. S., Kuosa, H., Gundersen, K., Kuuppo-Leinikki, P., Pajuniemi, R., et al. (1993). Fate of a phytoplankton spring bloom: sedimentation and carbon flow in the planktonic food web in the northern Baltic. Mar. Ecol. Prog Ser. 94, 239–239.
Lovvorn, J. R., Cooper, L. W., Brooks, M. L., De Ruyck, C. C., Bump, J. K., and Grebmeier, J. M. (2005). Organic matter pathways to zooplankton and benthos under pack ice in late winter and open water in late summer in the north-central Bering Sea. Mar. Ecol. Prog Ser. 291, 135–150. doi: 10.3354/meps291135
Marcus, N. H., and Boero, F. (1998). Minireview: the importance of benthic-pelagic coupling and the forgotten role of life cycles in coastal aquatic systems. Limnol. Oceanogr. 43, 763–768. doi: 10.4319/lo.1998.43.5.0763
McTigue, N. D., Bucolo, P., Liu, Z., and Dunton, K. H. (2015). Pelagic-benthic coupling, food webs, and organic matter degradation in the Chukchi Sea: insights from sedimentary pigments and stable carbon isotopes. Limnol. Oceanogr. 60, 429–445. doi: 10.1002/lno.10038
Middelburg, J. J. (2018). Reviews and syntheses: to the bottom of carbon processing at the seafloor. Biogeosciences 15, 413–427. doi: 10.5194/bg-15-413-2018
Morata, N., Poulin, M., and Renaud, P. E. (2011). A multiple biomarker approach to tracking the fate of an ice algal bloom to the sea floor. Polar biol. 34, 101–112. doi: 10.1007/s00300-010-0863-3
Morata, N., Renaud, P. E., Brugel, S., Hobson, K. A., and Johnson, B. J. (2008). Spatial and seasonal variations in the pelagic–benthic coupling of the southeastern Beaufort Sea revealed by sedimentary biomarkers. Mar. Ecol. Prog Ser. 371, 47–63. doi: 10.3354/meps07677
Nascimento, F. J. A., Karlson, A. M. L., Näslund, J., and Gorokhova, E. (2009). Settling cyanobacterial blooms do not improve growth conditions for soft bottom meiofauna. J. Exp. Mar. Biol. Ecol. 368, 138–146. doi: 10.1016/j.jembe.2008.09.014
Nordström, M., Aarnio, K., and Bonsdorff, E. (2009). Temporal variability of a benthic food web: patterns and processes in a low-diversity system. Mar. Ecol. Prog. Ser. 378, 13–26. doi: 10.3354/meps07872
Norkko, A., Villnäs, A., Norkko, J., Valanko, S., and Pilditch, C. A. (2013). Size matters: implications of the loss of large individuals for ecosystem function. Sci. Rep. 3:2646. doi: 10.1038/srep02646
Norkko, J., Gammal, J., Hewitt, J. E., Josefson, A. B., Carstensen, J., and Norkko, A. (2015). Seafloor ecosystem function relationships: in situ patterns of change across gradients of increasing hypoxic stress. Ecosystems 18, 1424–1439. doi: 10.1007/s10021-015-9909-2
Norkko, J., Reed, D. C., Timmermann, K., Norkko, A., Gustafsson, B. G., Bonsdorff, E., et al. (2012). A welcome can of worms? Hypoxia mitigation by an invasive species. Glob. Change Biol. 18, 422–434. doi: 10.1111/j.1365-2486.2011.02513.x
Ólafsson, E., and Elmgren, R. (1997). Seasonal dynamics of sublittoral meiobenthos in relation to phytoplankton sedimentation in the Baltic Sea. Estuar. Coast. Mar. Sci. 45, 149–164. doi: 10.1006/ecss.1996.0195
Ólafsson, E., Modig, H., and van de Bund, W. J. (1999). Species-specific uptake of radio-labelled phytodetritus by benthic meiofauna from the Baltic Sea. Mar. Ecol. Prog. Ser. 177, 63–72. doi: 10.3354/meps177063
Oliver, J. S., and Dayton, P. K. (1977). Antarctic soft-bottom benthos in oligotrophic and eutrophic environments. Science 197, 55–58. doi: 10.1126/science.197.4298.55
Ostrom, N. E., Macko, S. A., Deibel, D., and Thompson, R. J. (1997). Seasonal variation in the stable carbon and nitrogen isotope biogeochemistry of a coastal cold ocean environment. Geochim. Cosmochim. Act. 61, 2929–2942. doi: 10.1016/s0016-7037(97)00131-2
Parsons, T. R., Maita, Y., and Lalli, C. M. (1984). A Manual of Chemical and Biological Methods for Seawater Analysis. Oxford: Pergamon Press, 173.
R Development Core Team (2019). R: a Language and Environment for Statistical Computing. Vienna: R Foundation for Statistical Computing.
Reise, K. (1983). Biotic enrichment of intertidal sediments by experimental aggregates of the deposit-feeding bivalve Macoma balthica. Mar. Ecol. Prog. Ser. 12, 229–236. doi: 10.3354/meps012229
Renaud, P. E., Morata, N., Carroll, M. L., Denisenko, S. G., and Reigstad, M. (2008). Pelagic-benthic coupling in the western Barents Sea: processes and time scales. Deep-Sea Res. II 55, 2372–2380. doi: 10.1016/j.dsr2.2008.05.017
Renaud, P. E., Riedel, A., Michel, C., Morata, N., Gosselin, M., Juul-Pedersen, T., et al. (2007). Seasonal variation in benthic community oxygen demand: a response to an ice algal bloom in the Beaufort Sea. Canadian Arctic. J. Mar. Syst. 67, 1–12. doi: 10.1016/j.jmarsys.2006.07.006
Rolff, C. (2000). Seasonal variation in δ13C and δ15N of size-fractionated plankton at a coastal station in the northern Baltic proper. Mar. Ecol. Prog. Ser. 203, 47–65. doi: 10.3354/meps203047
Rossi, F., Herman, P. M. J., and Middelburg, J. J. (2004). Interspecific and intraspecific variation of δ13C and δ15N in deposit- and suspension-feeding bivalves (Macoma balthica and Cerastoderma edule): evidence of ontogenetic changes in feeding mode of Macoma balthica. Limnol. Oceanogr. 49, 408–414. doi: 10.4319/lo.2004.49.2.0408
Tahey, T. M., Duineveld, G. C. A., Berghuis, E. M., and Helder, W. (1994). Relation between sediment-water fluxes of oxygen and silicate and faunal abundance at continental shelf, slope and deep-water stations in the northwest Mediterranean. Mar. Ecol. Prog. Ser. 104, 119–130. doi: 10.3354/meps104119
Tamelander, T., and Heiskanen, A. S. (2004). Effects of spring bloom phytoplankton dynamics and hydrography on the composition of settling material in the coastal northern Baltic Sea. J. Mar. Syst. 52, 217–234. doi: 10.1016/j.jmarsys.2004.02.001
Tamelander, T., Kivimäe, C., Bellerby, R. G., Renaud, P. E., and Kristiansen, S. (2009). Base-line variations in stable isotope values in an Arctic marine ecosystem: effects of carbon and nitrogen uptake by phytoplankton. Hydrobiologia 630, 63–73. doi: 10.1007/s10750-009-9780-2
Tamelander, T., Reigstad, M., Hop, H., Carroll, M. L., and Wassmann, P. (2008). Pelagic and sympagic contribution of organic matter to zooplankton and vertical export in the Barents Sea marginal ice zone. Deep-Sea Res. Pt. II Top. Stud. Oceanogr. 55, 2330–2339. doi: 10.1016/j.dsr2.2008.05.019
Tamelander, T., Spilling, K., and Winder, M. (2017). Organic matter export to the seafloor in the Baltic Sea: drivers of change and future projections. Ambio 46, 842–851. doi: 10.1007/s13280-017-0930-x
Veuger, B., van Oevelen, D., and Middelburg, J. J. (2012). Fate of microbial nitrogen, carbon, hydrolysable amino acids, monosaccharides, and fatty acids in sediment. Geochim. Cosmochim. Act. 83, 217–233. doi: 10.1016/j.gca.2011.12.016
Volkenborn, N., Meile, C., Polerecky, L., Pilditch, C. A., Norkko, A., Norkko, J., et al. (2012). Intermittent bioirrigation and oxygen dynamics in permeable sediments: an experimental and modeling study of three tellinid bivalves. J. Mar. Res. 70, 794–823. doi: 10.1357/002224012806770955
Witte, U., Aberle, N., Sand, M., and Wenzhöfer, F. (2003). Rapid response of a deep-sea benthic community to POM enrichment: an in situ experimental study. Mar. Ecol. Prog. Ser. 251, 27–36. doi: 10.3354/meps251027
Zhang, Q., Warwick, R. M., McNeill, C. L., Widdicombe, C. E., Sheehan, A., and Widdicombe, S. (2015). An unusually large phytoplankton spring bloom drives rapid changes in benthic diversity and ecosystem function. Prog. Oceanogr. 137(Pt B), 533–545. doi: 10.1016/j.pocean.2015.04.029
Keywords: benthic–pelagic coupling, seasonal patterns, spring and summer blooms, life stage, Baltic Sea
Citation: Rodil IF, Lucena-Moya P, Tamelander T, Norkko J and Norkko A (2020) Seasonal Variability in Benthic–Pelagic Coupling: Quantifying Organic Matter Inputs to the Seafloor and Benthic Macrofauna Using a Multi-Marker Approach. Front. Mar. Sci. 7:404. doi: 10.3389/fmars.2020.00404
Received: 27 February 2020; Accepted: 11 May 2020;
Published: 05 June 2020.
Edited by:
Xavier Pochon, Cawthron Institute, New ZealandReviewed by:
Dick Van Oevelen, Royal Netherlands Institute for Sea Research (NIOZ), NetherlandsSaskia Rühl, Plymouth Marine Laboratory, United Kingdom
Leon Moodley, Norwegian Research Institute (NORCE), Norway
Copyright © 2020 Rodil, Lucena-Moya, Tamelander, Norkko and Norkko. This is an open-access article distributed under the terms of the Creative Commons Attribution License (CC BY). The use, distribution or reproduction in other forums is permitted, provided the original author(s) and the copyright owner(s) are credited and that the original publication in this journal is cited, in accordance with accepted academic practice. No use, distribution or reproduction is permitted which does not comply with these terms.
*Correspondence: Iván F. Rodil, aXZhbi5yb2RpbEBoZWxzaW5raS5maQ==