- 1Alfred Wegener Institute, Helmholtz Centre for Polar and Marine Research, Bremerhaven, Germany
- 2Coastal Ecology Section, Alfred Wegener Institute, Helmholtz Centre for Polar and Marine Research, Wadden Sea Station Sylt, List, Germany
- 3Marine Botany, Institute of Biology and Chemistry, University of Bremen, Bremen, Germany
Phenotypic plasticity (genotype × environment interaction) is an especially important means for sessile organisms to cope with environmental variation. While kelps, the globally most productive group of seaweeds, generally possess a wide thermal performance range, kelp populations at their warm distribution limits are threatened by ocean warming. Here, we investigated effects of temperature during ontogeny of the kelp Laminaria digitata across haploid gametophyte and diploid sporophyte life cycle stages in five distinct genetic lines. We hypothesized that thermal plasticity increases trait performance of juvenile sporophytes in experimental temperatures that match the temperature experienced during gametogenesis and recruitment, and that plasticity differs among genetic lines (genetic variation for plasticity). We applied a full-factorial experimental design to generate different temperature histories by applying 5 and 15°C during meiospore germination, gametogenesis of parental gametophytes and recruitment of offspring sporophytes (19–26 days), and juvenile sporophyte rearing (91–122 days). We then tested for thermal plasticity among temperature history treatments at 5 and 15°C in a final 12-day experiment assessing growth, the storage compound mannitol, carbon and nitrogen contents, and fluorometric responses in 3–4 month old sporophytes for five genetic lines. Our study provides evidence for the importance of cold temperatures at early development on later sporophyte performance of L. digitata. Gametogenesis and recruitment at 5°C promoted higher growth of offspring sporophytes across experimental temperatures. While photosynthetic capacity was higher at 15°C, carbon and nitrogen storage were higher at 5°C, both showing fast acclimation responses. We identified an important role of genetic variation for plasticity in shaping L. digitata’s thermal plasticity. Trait performance at 5 or 15°C (reaction norm slopes) differed among genetic lines, even showing opposite response patterns. Interestingly, genetic variation for plasticity was only significant when sporophytes were reared at 5°C. Thus, we provide evidence that the cold-temperate to Arctic kelp species, L. digitata, which possesses a wide temperature tolerance between 0 and 23°C, is impaired by warm temperature during gametogenesis and recruitment, reducing growth of juvenile sporophytes and expression of variable thermal plasticity in the wild.
Introduction
In a changing environment, organisms have few mechanisms to cope with temporal habitat heterogeneity. In order to prevent range contractions, populations can move according to their environmental requirements (i.e., range shifts) or they can acclimate and/or adapt to the new conditions, all of which are interactive processes within a complex response framework (Donelson et al., 2019). Especially for sessile species, phenotypic plasticity is an important means of quick response to environmental change. Phenotypic plasticity has been studied for decades (Bradshaw, 1965; Sultan, 1995; Chevin et al., 2010; Fox et al., 2019), and describes phenotypic changes in an individual in response to its environment (genotype × environment interaction). This includes fast and reversible acclimation responses, but also carry-over effects due to exposure during early ontogeny which persist during development (COE, also developmental plasticity; Palmer et al., 2012; Byrne et al., 2020). Additionally, the environment experienced by parents can influence offspring traits regardless of offspring environment (parental effects sensu Salinas et al., 2013). Importantly, environmental change cues may elicit different plastic responses among genotypes (genetic variation for plasticity; Newman, 1994; Nicotra et al., 2010), thereby increasing trait variability within a population that selection can act on. These concepts highlight the importance of taking into account environmental history and investigating multiple genotypes when assessing thermal plasticity of populations and species.
Brown seaweeds of the order Laminariales (i.e., kelps sensu stricto) are especially important habitat builders along warm-temperate to polar rocky shorelines (Lüning, 1990). They provide a three-dimensional, species-rich and highly productive habitat, the marine forest (Wernberg and Filbee-Dexter, 2019), which is globally under threat by rising sea temperatures especially at their equatorward margins (Wernberg et al., 2016; Vergés et al., 2019). Many kelps have a broad thermal performance range spanning 20°C or more (e.g., Lüning, 1984; tom Dieck, 1993; Wiencke et al., 1994), within which their metabolism can quickly adjust (i.e., acclimate) and prevent irreversible stress responses. This high acclimation capacity allows kelps to persist along environmental gradients by fast adjustment of e.g., photosynthesis (Davison and Davison, 1987; Davison et al., 1991; Rothäusler et al., 2011), carbon metabolism (Scheschonk et al., 2019), or pigment contents (Li et al., 2019; Mabin et al., 2019b) in response to a variety of factors. All laminarian kelps alternate between independent generations of haploid gametophytes and diploid sporophytes in their life cycle. Therefore, kelps provide a useful experimental system to investigate thermal plasticity across ontogeny. We consider the kelp germline to encompass the whole process between meiosis and production of eggs and sperm (see also Grossniklaus, 2011; Schmidt et al., 2015). Therefore, the majority of the kelp germline is contained in the autonomous gametophyte generation, which allows for experimental control of the germline environment from meiospore release to zygote formation. Further, this facilitates breeding of gametophytes obtained from one sporophyte, allowing comparisons among genetic lines and tests of genetic variation for plasticity.
In this study, we investigated thermal plasticity of the kelp Laminaria digitata (Hudson) J.V. Lamouroux, which is a key habitat-former in the upper sublittoral and infralittoral fringe of cold-temperate and Arctic rocky coasts (Lüning, 1990; Dankworth et al., 2020). In the North Atlantic, L. digitata is one of four kelp species of the genus Laminaria (among other kelp genera), which occur across marine biogeographical regions (sensu Lüning, 1990): L. solidungula is an Arctic species; L. digitata occurs in Arctic and cold-temperate regions; L. hyperborea is a cold-temperate species and co-occurs along most of its distribution with L. digitata in the sublittoral; and L. ochroleuca is a warm-temperate species and co-occurs with L. digitata only in Brittany and South England (Smale et al., 2015). Along the European coast, L. digitata occurs between the 0°C February isotherm on the archipelago of Spitsbergen and the 18°C August isotherm in southern Brittany, France (for isotherms, see Müller et al., 2009). In Brittany, the species marginally extends into the warm-temperate region (February isotherm > 10°C sensu Lüning, 1990). Despite a high gametophyte temperature tolerance of 23°C over several weeks (tom Dieck, 1993), models predict the loss of the southernmost L. digitata populations due to ocean warming (Raybaud et al., 2013; Assis et al., 2018).
At our study location on the North Sea island of Helgoland (Germany), L. digitata meiospore release occurs mainly between May and November (Bartsch et al., 2013), but it is not known when gametogenesis and recruitment of juvenile sporophytes are most prevalent, especially as vegetative gametophytes might provide a perennial “seed bank” (tom Dieck, 1993; Edwards, 2000). Therefore, temperatures might vary greatly during formation of primordial germ cells in gametophytes and during subsequent recruitment and growth of juvenile offspring sporophytes. Despite L. digitata’s potentially broad thermal performance spectrum, a relatively warm temperature of 15°C was shown to enhance gametogenesis, sporophyte growth and sporogenesis (tom Dieck, 1992; Bartsch et al., 2013; Martins et al., 2017; Franke, 2019) compared to a cool temperature of 5°C, whereas sporophyte recruitment was most successful under cool conditions (Martins et al., 2017). Importantly, it is unknown whether and how temperature variation during early life stages of gametogenesis and recruitment influences thermal plasticity of juvenile sporophytes.
Generally, temperature responses of L. digitata are not static, but may vary across seasons (Lüning, 1984) and are shaped by endogenous and annual abiotic changes. For example, the endogenous growth rhythmicity present in laminarian kelps is modulated and synchronized by changes in daylength (Lüning, 1991; tom Dieck, 1991; Schaffelke and Lüning, 1994), but the influence of rhythmicity and daylengths on the temperature performance of kelps is unknown. Daylength and temperature may control the seasonal accumulation of the carbon storage compounds mannitol and laminarin in Laminariales (Schaffelke, 1995), which naturally peak in late summer (Black, 1954; Schiener et al., 2015; Manns et al., 2017). Additionally, few indications for carry-over and parental effects have been shown in kelp and fucoid seaweeds. Li and Brawley (2004) demonstrated a positive parental effect of warm receptacle environment on Fucus vesiculosus embryo survival during heat stress. Mabin et al. (2019a) demonstrated significant variation in morphology between lineages of Ecklonia radiata gametophytes at different temperatures and irradiances, which they attributed to potential parental and genetic effects. The latter further indicates that individual phenotypic variation may play a substantial role in shaping response variability of kelp populations. Phenotypic differences among genotypes have been shown to modulate plasticity in terrestrial plants (Galloway, 2001; Suter and Widmer, 2013), but similar research in kelps does not exist to our knowledge.
The main objective of this study was to investigate thermal plasticity of juvenile Laminaria digitata sporophytes in the context of their temperature history across ontogeny and among genotypes. Our approach tested for effects of temperature treatments of 5 and 15°C over the kelp life cycle on thermal plasticity of juvenile L. digitata sporophytes. First, we assessed growth at 5 and 15°C across multiple seasons using wild adult sporophytes to relate our experimental results to adult sporophyte responses in the wild. We then released meiospores from five wild sporophytes, cultivated them separately as genetic lines and produced four temperature history treatments by applying 5 and 15°C during meiospore germination, gametogenesis and sporophyte recruitment, and during growth of juvenile sporophytes. In a final 12-day experiment, we split 3–4 month old centimeter-sized sporophytes again between 5 and 15°C, and assessed thermal plasticity of growth, the storage compound mannitol, carbon and nitrogen contents, and fluorometric parameters. We hypothesized that trait performance of juvenile sporophyte offspring would increase in experimental temperatures that matched the temperature experienced during gametogenesis and recruitment (COE or developmental plasticity) in comparison to mismatching temperature history treatments (match-mismatch approach; Engqvist and Reinhold, 2016). We further hypothesized that genetic lines would differ in their capacity for thermal plasticity (genetic variation for plasticity), potentially contributing to thermal response variability of L. digitata populations under ocean warming.
Materials and Methods
Experimental Design
To assess seasonal thermal plasticity of adult sporophytes in the wild, we first followed growth of field-collected sporophyte meristems at 5 and 15°C at three time points over the year (experiment 1, Figure 1). At these temperatures, sporophyte growth is in its optimum range (Bolton and Lüning, 1982) and gametogenesis is not inhibited (tom Dieck, 1992; Martins et al., 2017).
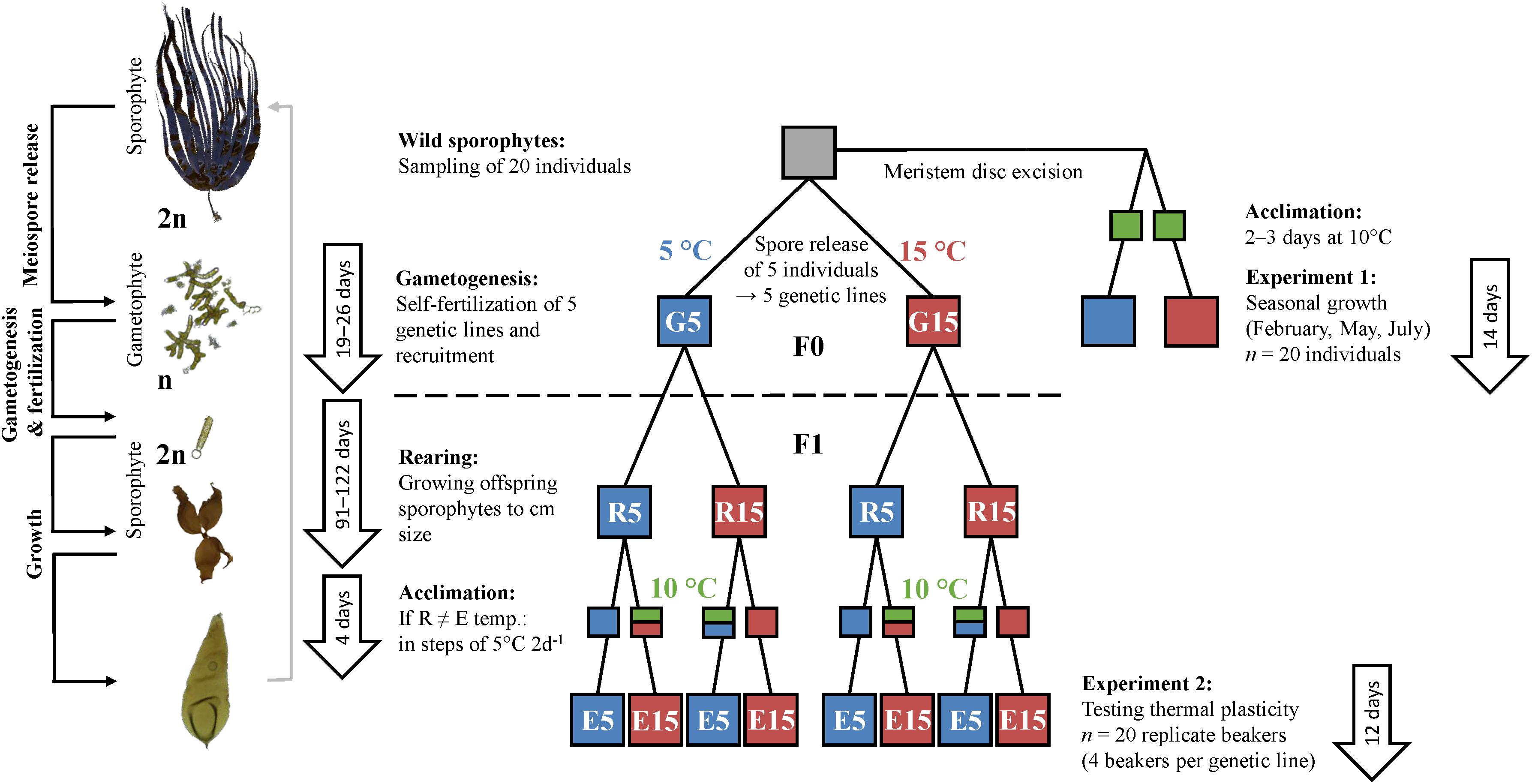
Figure 1. Experimental design to test for thermal plasticity of wild adult sporophytes (experiment 1) and juvenile sporophytes (experiment 2) of Laminaria digitata. Twenty fertile L. digitata sporophytes were sampled in the field (Helgoland, North Sea). Excised meristems from 20 individuals sampled in May, July 2017 and February 2018 were used for experiment 1. Meiospores of five sporophytes were released and genetic lines were maintained by self-fertilization in 5 and 15°C (gametogenesis, 19–26 days). Throughout the flowchart for experiment 2, each colored box represents five separate genetic lines. Following recruitment, each gametogenesis treatment was divided to rear juvenile microscopic sporophytes at 5 and 15°C (rearing, 91–122 days). An acclimation period of 4 days was applied for all treatments, with stepwise temperature change of 2°C d–1 for treatments in which rearing and experimental temperatures differed. Experiment 2 was conducted with 7–11 cm long sporophytes from five genetic lines at 5 and 15°C for 12 days. The schematic life cycle represents life stages at the different treatment steps of the experiment.
Following this, we tested for effects of temperature during early ontogeny on trait plasticity of juvenile offspring L. digitata sporophytes. Here, we employed a full-factorial experimental design using five genetic lines of L. digitata at two experimental temperatures (5 and 15°C), and encompassing parent (F0) and offspring (F1) generations at three time periods: during gametogenesis and recruitment (F0/F1), early sporophyte (F1) rearing, and growth of F1 juvenile sporophytes (experiment 2; Figure 1). This full-factorial experimental approach on distinct genetic lines allowed disentanglement of the roles of genetic background and environmental effects, as well as assessments of genetic variation for plasticity (Herman and Sultan, 2016; Donelson et al., 2018).
Sampling and Cultivation of Laminaria digitata
We collected 20 fertile L. digitata adult sporophytes from the low intertidal zone on the island of Helgoland (North Sea, Germany; 54.1779 N, 7.8926 E) on 10 May 2017, 10 July 2017 and 04 February 2018. For the seasonal growth experiment (experiment 1), two discs (Ø 24 mm) were cut from each sporophyte meristem at a distance of 5 cm from the stipe-blade transition zone and transported to the laboratory wet and cool. In July, we also sampled fertile blade parts to be used for meiospore release in the main experiment on thermal plasticity of juvenile sporophytes (experiment 2). The use of blade tissue samples is an established experimental method to gain approximations of whole-organism responses (Graiff et al., 2015; Hargrave et al., 2017; King et al., 2019). For example, excised meristematic tissue can be used to investigate growth activity and photosynthesis (Buchholz and Lüning, 1999; Scheschonk et al., 2019), and vegetative blade tissue can be used to induce sporogenesis (Bartsch et al., 2013).
All samples were cultivated in 10 mL L–1 Provasoli-enriched sterilized natural seawater (PES; Provasoli, 1968; modifications: HEPES-buffer instead of TRIS, double concentration of Na2glycerophosphate, iodine enrichment after Tatewaki, 1966) in temperature-controlled chambers (5, 10, 15°C, variation ± 1°C). The light:dark photoperiod of 16 h:8 h applied in this study induces constant, year-round growth in L. digitata (Schaffelke and Lüning, 1994). Irradiance varied depending on the life cycle stage to accommodate the irradiance requirements of each stage (Lüning, 1980; tom Dieck, 1992; Han and Kain, 1996): 30–40 μmol photons m–2 s–1 for meristem discs and juvenile sporophytes, and 16–18 μmol photons m–2 s–1 for gametophytes (ProfiLux 3 with LED Mitras daylight 150, GHL Advanced Technology, Kaiserslautern, Germany). Sporophytes and meristem discs were cultivated in aerated one liter glass beakers filled with 800 mL PES.
Experiment 1: Seasonal Growth of Wild Laminaria digitata Sporophytes
Growth of meristem discs at 5 and 15°C was assessed in February, May and July (Figure 1, experiment 1). Discs were first acclimated to laboratory conditions for two to three days at 10°C (n = 20 individuals each held separately). Subsequently, two discs per individual were divided between 5 and 15°C in a paired design (Figure 1). Medium in the beakers was changed weekly. After 14 days, we assessed area growth via image analysis (WinFolia Pro 2006a software; Regent Instruments Inc., Quebec, Canada). Absolute area growth rates were calculated with a linear formula:
where x1 = area at time 1, x2 = area at time 2, t1 = time 1 in days (d), t2 = time 2 in days. We also measured quantum yield of photosystem II (Fv/Fm) as a stress parameter in the center of each disc using pulse-amplitude modulation fluorometry (PAM-2100, Walz, Effeltrich, Germany) after 5 min dark-acclimation.
Meiospore Release, Gametogenesis, Recruitment, and Rearing
Sori sampled in July were stored for 1–2 days in plastic bags at <5 μmol photons m–2 s–1 in sterilized natural seawater (SW) prior to meiospore release. Meiospores were released from sori following the method of Bartsch (2018). Meiospores from five adult individuals were sowed separately into plastic dishes. These five genetic lines were followed separately throughout the experiment. Each set of meiospores was immediately split to germinate, grow, complete gametogenesis and recruit microscopic sporophytes at 5 and 15°C (gametogenesis treatment: G5 and G15; Figure 1).
Gametogenesis in L. digitata is a gradual process stretching over one to several weeks depending on environmental conditions (Lüning, 1980). In our experiment, following settlement overnight, meiospores germinated within four days and further developed into one- to few-celled male and female free-living gametophytic filaments, which subsequently became fertile. Fertile female gametophytes extrude single eggs which remain loosely attached to the discharged oogonium (Schreiber, 1930). Free-swimming spermatozoids produced by male gametophytes subsequently fertilize the eggs (Lüning, 1980). The resulting zygote is the initial cell of the next generation, the diploid F1-offspring sporophyte, which is physically separated from the parental gametophyte generation (Schreiber, 1930).
Because not all gametophyte cells undergo gametogenesis simultaneously, we ensured saturation of sporophyte recruitment within each gametogenesis temperature to impede sporophyte recruitment from remaining vegetative gametophytes in the following F1 rearing step. In our experiment, most female gametophytes had released their eggs after 19 days at 15°C and after 26 days at 5°C. At this time, the recruited sporophytes were between one and seven days old. Following saturation, recruited microscopic F1 sporophytes were again divided into 5 and 15°C to enable growth to a sufficient length of several cm for experiment 2 (rearing treatment: R5 and R15; Figure 1). The rearing phase took between 91 and 122 days, and produced four temperature history pre-treatments (G5–R5, G5–R15, G15–R5, G15–R15).
Experiment 2: Thermal Plasticity in Juvenile Laminaria digitata Sporophytes
Experiment 2 was conducted in two consecutive runs to reduce the workload at one time and to accommodate slower sporophyte growth at 5°C. The first run included all sporophytes reared at 15°C and the second run, which started 24 days later, included all sporophytes reared at 5°C. Sporophytes reared at 5°C had a mean length of 11 cm and sporophytes reared at 15°C had a mean length of 7 cm at the start of the experiment. We accounted for differences in rearing time and initial length in the statistical analyses (see below). At this point, the five genetic lines from four temperature histories were again divided into 5 and 15°C (E5 and E15, resulting in eight experimental treatment groups; Figure 1, experiment 2) to assess their thermal plasticity in contrasting temperature environments (Sultan, 2004). When the experimental temperature differed from the rearing temperature, we first acclimated sporophytes for two days at 10°C followed by two days at the target temperature to reduce acute temperature stress responses in the experiment. When rearing and experimental temperatures matched, sporophytes were transferred to the experimental setup without a change of temperature and held for four days prior to the start of the experiment (Figure 1, acclimation). For each genetic line in the eight temperature history groups, four replicate beakers each containing five sporophytes (n = 20 replicates per treatment group, N = 160 experimental units in total) were held in 1.7 L PES in aerated plastic (PETG) containers. The medium was exchanged every three days over the course of acclimation and the 12-day experiment.
Growth
For experiment 2, photos were taken after acclimation (day 0) and at the end of the experiment (day 12), and lengths of sporophytes were measured by tracing a central line along each sporophyte using the segmented line tool in ImageJ 1.51j8 (Schneider et al., 2012). Length sums of the five sporophytes per beaker were used to assess growth (n = 4 replicate beakers per experimental treatment and genetic line).
Biochemistry
We assessed mannitol content (the primary photosynthetic product and compatible solute; Davison and Reed, 1985; Groisillier et al., 2014) to estimate carbon assimilation by sporophytes. Analyses of elemental carbon and nitrogen provided insight into assimilation and nutrient storage, while C:N ratios allowed interpretations of nutrient sufficiency (Hurd et al., 1996; Rosell and Srivastava, 2004). Before acclimation (day -4), 3–22 sporophytes from each genetic line and temperature history were pooled to reach sufficient dry weight (> 200 mg), deep-frozen in liquid nitrogen and stored at −80°C (n = 5 genetic lines per treatment; N = 20 samples in total). After the experiment (day 12), two sporophytes from each experimental replicate (n = 20 replicates per experimental treatment; N = 160 samples in total) were deep-frozen. All samples were lyophilized and ground to a fine powder. Mannitol was extracted in 70% ethanol from three subsamples (10–15 mg) of each experimental replicate and analyzed following the HPLC method described by Karsten et al. (1991). Means of the three subsamples of each mannitol replicate were used in the statistical analysis. For carbon and nitrogen analysis, samples of 2–3 mg for carbon and nitrogen were combusted at 1000°C in an elemental analyzer (EURO EA, HEKAtech GmbH, Wegberg, Germany) with acetanilide as standard.
Fluorometry
Temperature can influence photosynthesis by mediating pigment, enzyme or photosystem contents (Gerard and Du Bois, 1988; Davison et al., 1991; Machalek et al., 1996; Li et al., 2019). Therefore, we tested for variation of fluorometric parameters in response to temperature treatments as a measure of photo-acclimation. One sporophyte per replicate was randomly chosen for fluorometric measurements (using a PAM-2100 device) before temperature acclimation (day -4) and at the end of experiment 2 (day 12). After 5 min dark acclimation, optimum quantum yield of photosystem II (Fv/Fm) was measured in the basal meristematic region of the sporophytes, directly followed by rapid light curves (RLC) with irradiance steps between 0–511 μmol photons m–2 s–1. Based on the photon flux density (PFD) and the effective quantum yield, relative electron transport rates (rETR) in photosystem II were calculated as rETR = PFD ∗ Yield (Maxwell and Johnson, 2000; Hanelt, 2018). rETR was plotted against PFD, and the resulting rETR vs. irradiance curves were fit following the model of Jassby and Platt (1976) to calculate maximum relative electron transport rate rETRmax, saturation irradiance Ik, and photosynthetic efficiency α of each curve.
Statistics
Because of bleaching of three thalli during experiment 2, we removed three replicates from all analyses (leading to total N = 157, and n = 18 and n = 19 in one treatment group each). For the growth analysis, one more replicate was removed after the loss of two sporophytes from a replicate beaker (N = 156). All analyses were performed in the R statistical environment (R version 3.6.0; R Core Team, 2019). Linear mixed effects models were fit using the lme function within the “nlme” package (Pinheiro et al., 2019) including a weights argument to incorporate the variance structure (Zuur et al., 2009). Normal distribution of standardized residuals was assessed visually via Q-Q plots and histograms, and non-normality was treated by log-transformation (Underwood, 1997). Factor significance was assessed via analyses of covariance (ANCOVA) and p-values were corrected for multiple testing following the false discovery rate approach (FDR; Benjamini and Hochberg, 1995).
In experiment 1, meristem disc growth rates and Fv/Fm were modeled using temperature treatment, month, and their interaction as fixed effects, with initial values of area and Fv/Fm as respective covariates, random intercepts for individuals (area and Fv/Fm models), and random slopes for experimental temperature (area model only). Post hoc pairwise comparisons of least-squares means (Tukey adjusted) between treatments were performed using the “emmeans” package (Lenth, 2019).
In experiment 2, we fit separate linear mixed effects models for each rearing temperature (i.e., experimental run) to account for any potentially confounding effects of assaying the two rearing temperatures at different times. Response parameters were modeled using initial values of each parameter as covariates, temperature treatment steps and their interaction (gametogenesis ∗ experimental temperature) as fixed effects, random intercepts for genetic line, and random slopes for experimental temperature. We tested for significance of gametogenesis temperature (temperature effects during early ontogeny), experimental temperature (temperature acclimation within the sporophyte stage), and their interaction (modulation of sporophyte thermal plasticity by gametogenesis temperature) using two-way ANCOVA. Significance of random factors was assessed using likelihood ratio tests between full (random slope for experimental temperature and random intercept for genetic line) and stepwise reduced models (random intercept for genetic line, and no random argument).
Results
Experiment 1: Seasonal Growth of Wild L. digitata Sporophytes
Seasonal growth (Figure 2A) and optimum quantum yield (Fv/Fm; Figure 2B) of wild L. digitata meristem tissue were significantly influenced by experimental temperature, sampling month, and their interaction (Table 1). Mean initial absolute growth rates between days 0 and 3 (data not shown) decreased over seasons from 0.39 cm2 d–1 in February to 0.16 cm2 d–1 in May to 0.09 cm2 d–1 in July, and thereby reflected known seasonal growth responses of L. digitata (Kain, 1979; Lüning, 1979). While in February and July growth rates over 14 days were significantly higher at 15°C than at 5°C (Tukey tests, p < 0.001), there were no significant differences between growth at 5°C and 15°C in May (Tukey test, p = 0.996; Figure 2A; for individual growth rates see Supplementary Table S1). Mean daily sea surface temperatures (SST) over 14 days before sampling in February, May and July were 5.8°C ± 1.2°C, 8.6°C ± 0.8°C, and 16.1°C ± 1.3°C, respectively (Helgoland Roads data; Wiltshire et al., 2008). Mean optimum quantum yield (Fv/Fm; Figure 2B) ranged between 0.7 and 0.8 across seasons, and was always significantly higher at 15°C compared to 5°C (p < 0.0001; Table 1), while mean Fv/Fm was lowest at 5°C in July.
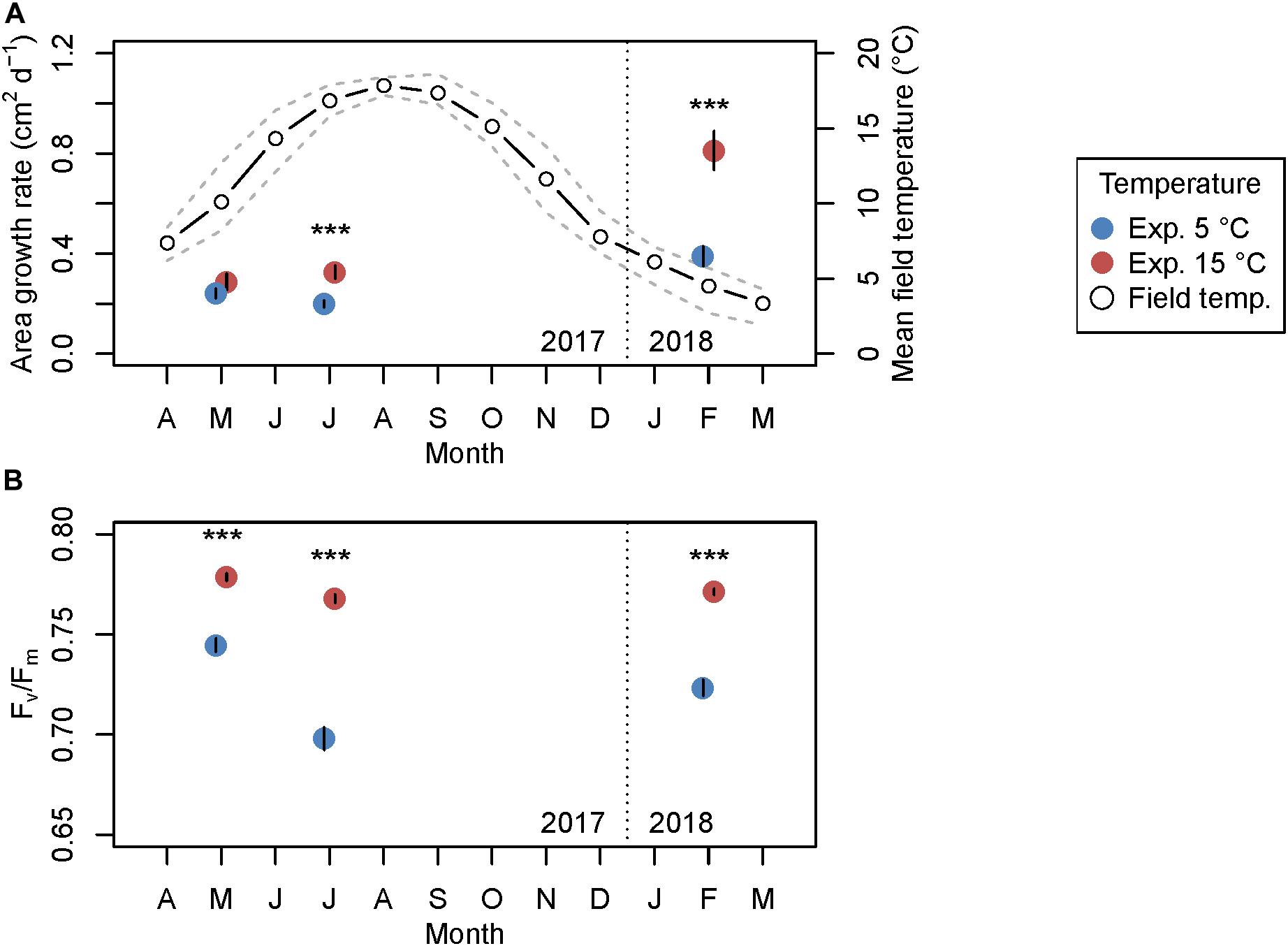
Figure 2. Seasonal growth of wild adult Laminaria digitata meristem discs sampled in May and July 2017, and February 2018 cultivated at 5 and 15°C for 14 days (experiment 1). (A) Absolute area growth rate over 14 days (primary y-axis, mean values ± SE, n = 20) and field sea-surface temperature around Helgoland (secondary y-axis). Open circles represent mean temperatures per month and dashed gray lines delimit minimum and maximum monthly temperatures for the year 2017/2018 (Helgoland Roads data, Wiltshire et al., 2008). (B) Quantum yield Fv/Fm at the end of the 14-day experiment (mean values ± SE, n = 20). ***p < 0.001 (Tukey test).
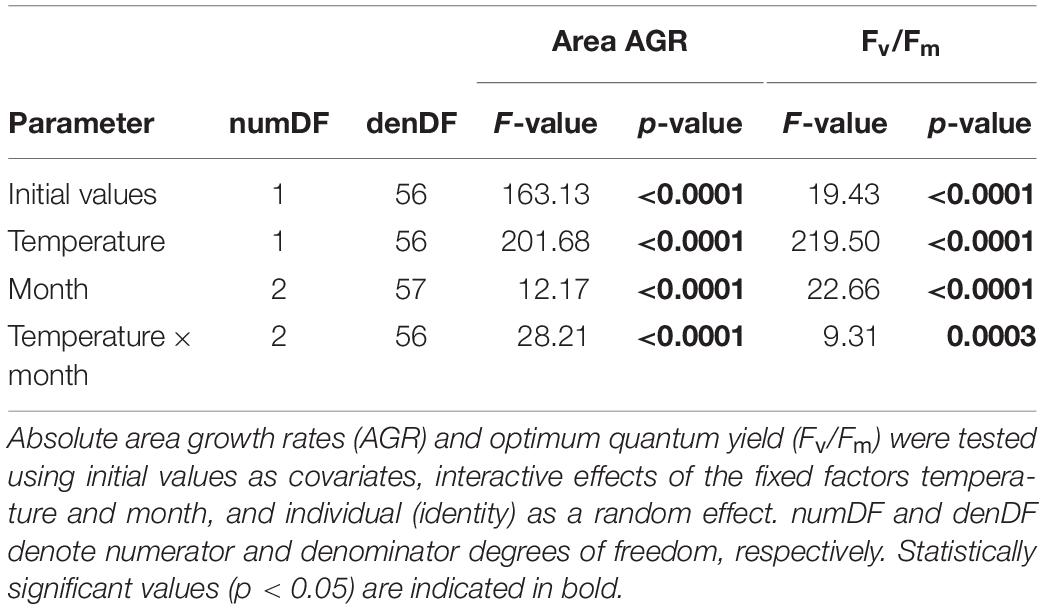
Table 1. Results of linear mixed effects models to examine thermal plasticity of wild adult Laminaria digitata meristem discs in the seasonal growth experiment 1.
Experiment 2: Thermal Plasticity in Juvenile L. digitata Sporophytes
Due to the different rearing temperatures, initial values for most parameters differed at the start of experiment 2 (Supplementary Table S2), which we accounted for by including them as model covariates and by separating the statistical analyses by rearing temperature. We show residual growth based on linear models of final length as a function of initial length for each rearing temperature in Figure 3 (absolute growth rates are given in the text and Supplementary Figure S1). In Figure 3, the dotted zero-line represents predicted values based on initial length and deviations from this line represent temperature effects. All other response parameters are shown as absolute values. For all parameters in experiment 2, we show reaction norms of traits across experimental temperatures (E5 or E15) color-coded by gametogenesis temperatures (G5 or G15) in two rearing temperature panels (R5 or R15). In this context, we define reaction norms as the relationship (slope) between trait responses at the two experimental treatments E5 and E15.
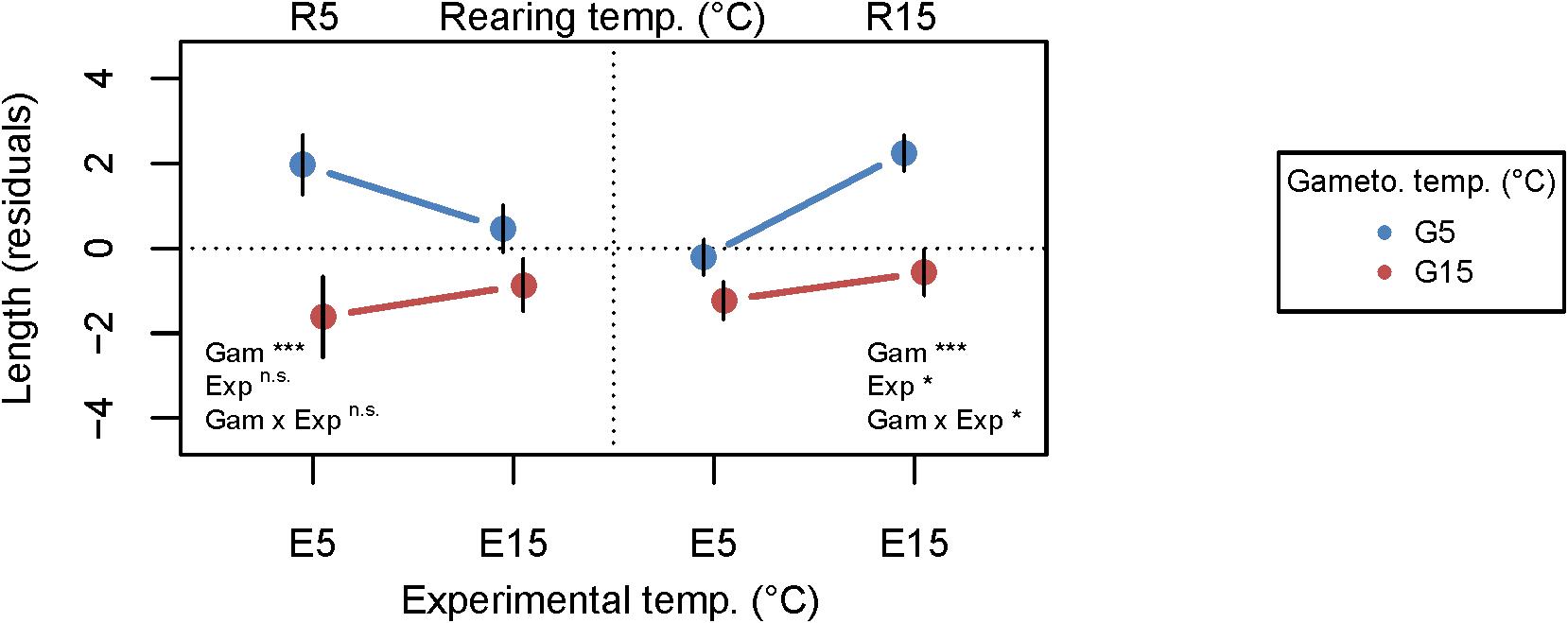
Figure 3. Growth reaction norms of juvenile Laminaria digitata sporophytes in experiment 2 to visualize effects of gametogenesis temperature on thermal plasticity of sporophytes. Primary x-axis: experimental temperature, secondary x-axis: rearing temperature, symbol colors: gametogenesis temperature. Residuals of simple linear models of final length as a function of initial length are shown as a growth parameter. The zero-line represents the final length modeled based on initial length, and deviations from the zero-line are interpreted as treatment effects. Mean over all genetic lines ± SE, n = 20. Statistical significance of the fixed factors gametogenesis temperature (Gam), experimental temperature (Exp) and their interaction (Gam × Exp) is summarized in the figure; ***p < 0.001; *p < 0.05; n.s., not significant. For full statistical report see Table 2.
Growth
Despite the long rearing period (91–122 days), we found significant, persistent effects of gametogenesis and recruitment temperature on growth of 3–4 month old sporophytes (Figure 3 and Table 2). In both rearing temperatures, mean sporophyte growth was significantly higher following G5 (R5: p = 0.0003; R15: p < 0.0001; Table 2), whereas mean growth of sporophytes following G15 remained below the zero prediction across experimental temperatures. Effects of experimental temperature and interactive gametogenesis × experimental temperature effects were only significant in R15 (Table 2). The significant main effect of experimental temperature in R15 (p = 0.0273; Table 2) led to higher overall growth at E15 compared to E5. This indicates an acclimation response, in which growth rates were adjusted to the experimental temperature within 12 days. In R15, the significant gametogenesis × experimental temperature interaction (p = 0.0264; Table 2) modified sporophyte growth responses. This is shown as higher growth in the G5–R15–E15 treatment (0.30 ± 0.04 cm sporophyte–1 d–1, treatment mean ± SD) compared to the other treatments (G5–R15–E5: 0.27 ± 0.04 cm d–1; G15–R15–E5: 0.25 ± 0.04 cm d–1; G15–R15–E15: 0.26 ± 0.04 cm d–1). Within R5, growth was highest in the matching gametophyte-sporophyte (gametogenesis-experimental) temperature treatment (G5–R5–E5: 0.38 ± 0.06 cm d–1) compared to the mismatched treatments (G5–R5–E15: 0.36 ± 0.04 cm d–1; G15–R5–E15: 0.34 ± 0.05 cm d–1; G15–R5–E5: 0.31 ± 0.07 cm d–1). Here, the gametogenesis × experimental temperature interaction was marginally non-significant (p = 0.0859 after correcting for multiple testing; Table 2).
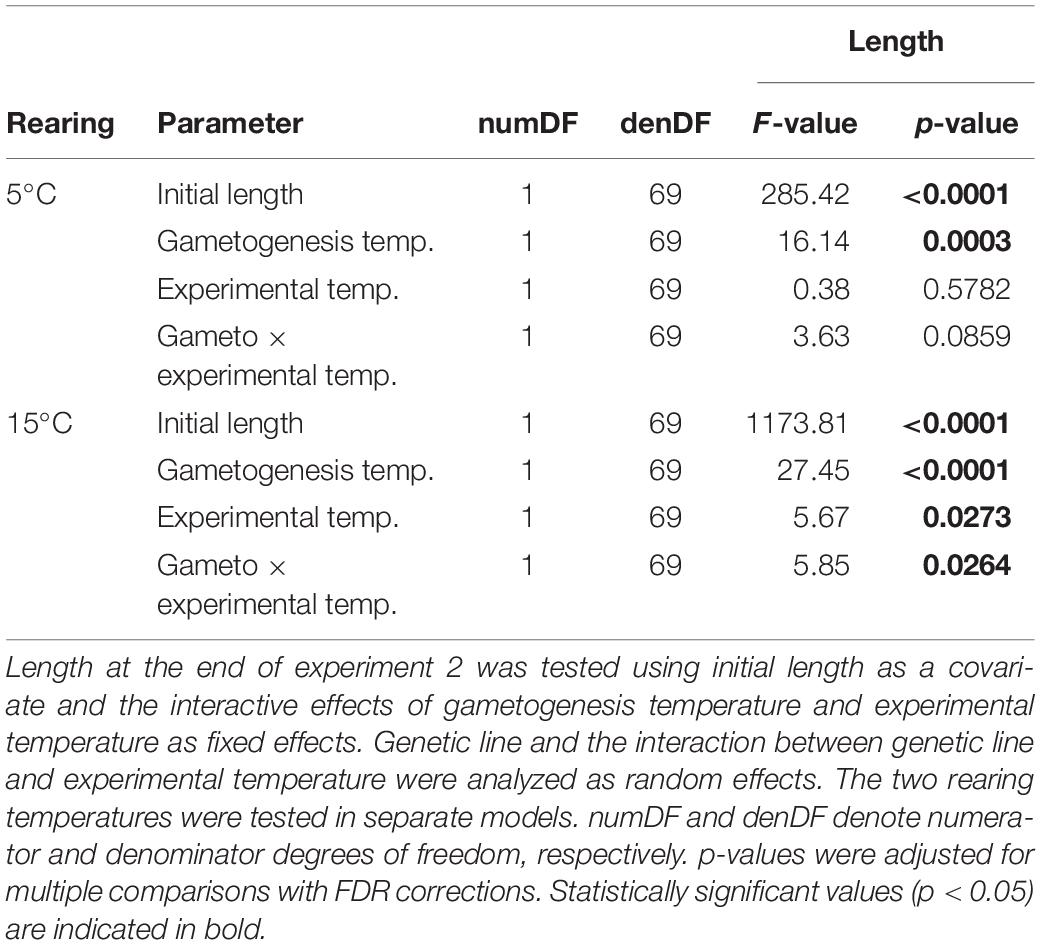
Table 2. Results of linear mixed effects models to examine thermal plasticity of length growth of juvenile Laminaria digitata sporophytes in experiment 2.
We then tested if genetic lines differed in their thermal plasticity (genetic variation for plasticity) by investigating if model fits significantly improved when including random intercept and slope arguments (Figure 4 and Table 3). A significant random intercept indicates that the magnitude of response to the experimental temperatures differed among genetic lines, whereas a significant random slope indicates that genetic lines differed in the direction and/or expression of their thermal plasticity (genotype × environment interaction; GxE; Saltz et al., 2018). For growth, genetic line (random intercept) was significant at both rearing temperatures (R5: p = 0.0013; R15: p = 0.0002; Table 3), indicating differing magnitudes of response among the five genetic lines within each rearing temperature. Within R5, GxE (random slope) was significant (p = 0.0001; Table 3), indicating that the genetic lines differed in their plastic growth response to temperature, but only when reared at 5°C. This genetic variation for plasticity is visible as more variance among reaction norms within R5, whereas reaction norm slopes were more similar within R15 (but still differed in magnitude). This increased variance among individuals in R5 might in turn explain the marginally non-significant interaction of gametogenesis and experimental temperature in the mean growth response in R5 (Figure 3).
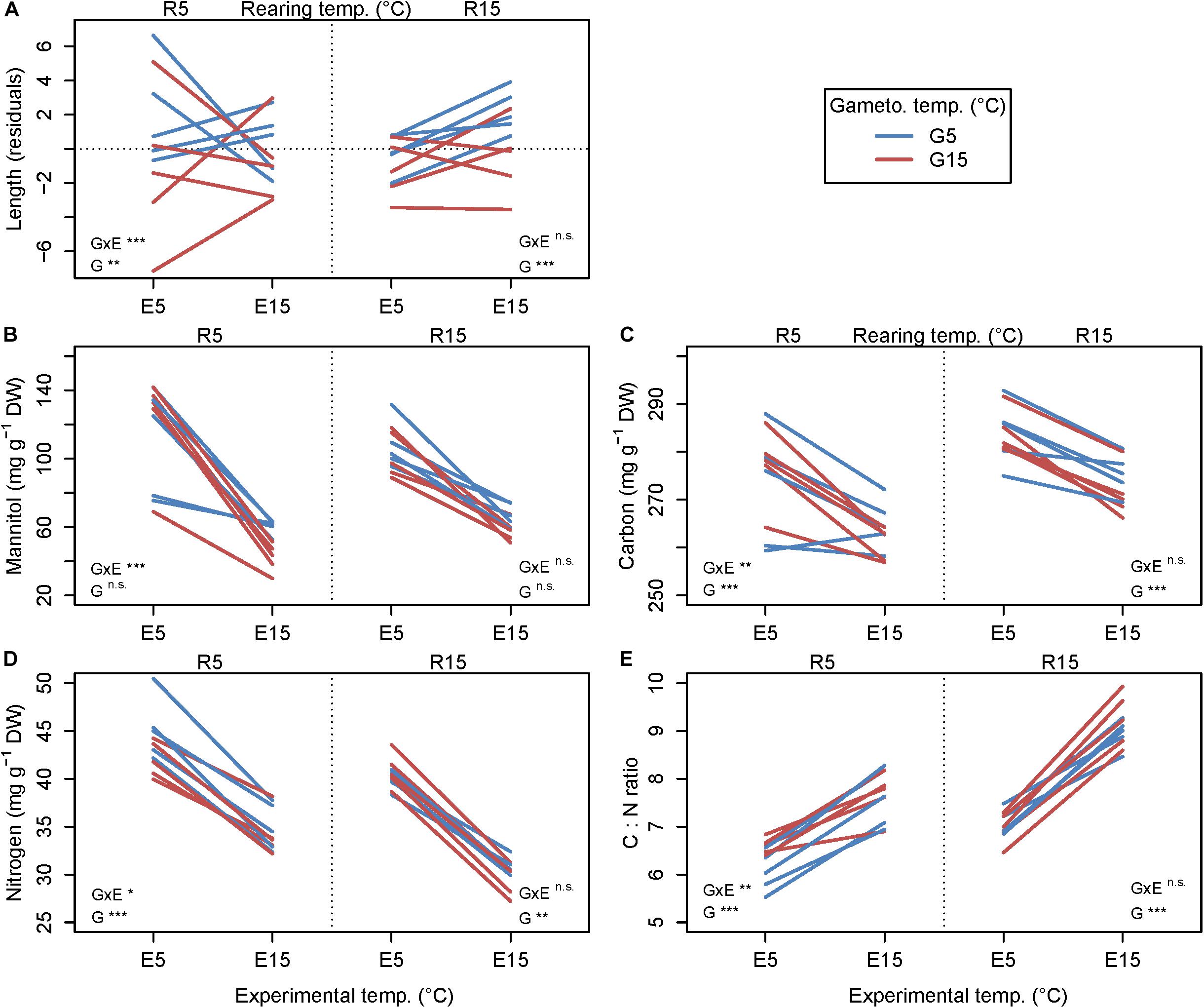
Figure 4. Reaction norms of growth and biochemical parameters for each genetic line of Laminaria digitata in experiment 2 to visualize genetic variation for plasticity. Primary x-axis: experimental temperature, secondary x-axis: rearing temperature, slope colors: gametogenesis temperature. All plots show values obtained at the end of the 12-day experiment. (A) residual length growth, (B) mannitol contents in dry mass, (C) total carbon contents in dry mass, (D) total nitrogen contents in dry mass, (E) carbon to nitrogen mass ratio (C:N ratio). Means over single genetic lines, except for (B) means of mean values due to extraction in triplicates, n = 4. Statistical significance of the random factors for genetic line × experimental temperature interaction (GxE; random slope) and genetic line (G; random intercept) is summarized in the figure; ***p < 0.001; **p < 0.01; *p < 0.05; n.s., not significant. For full statistical report see Table 3.
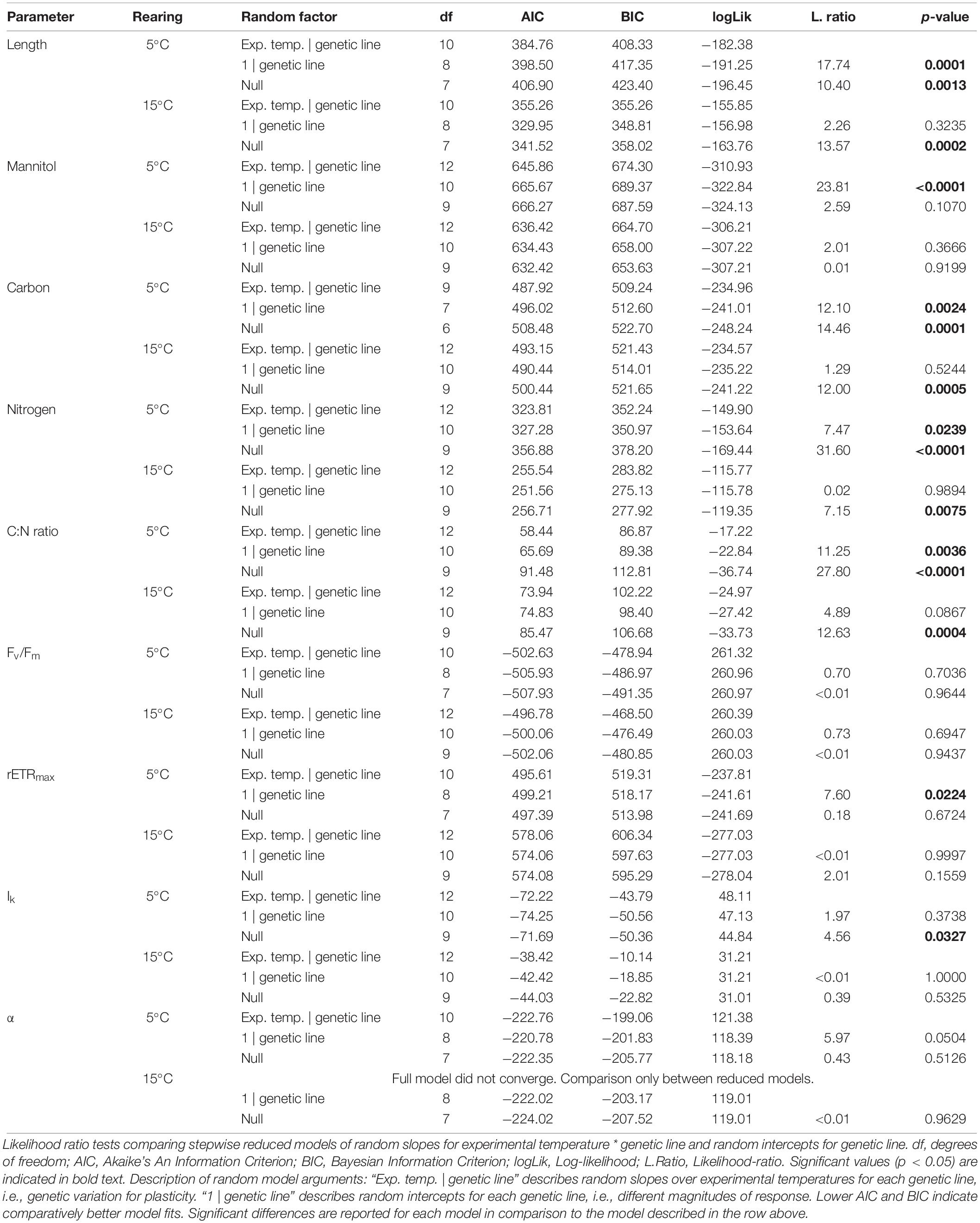
Table 3. Results of likelihood ratio tests to determine significance of the random effects “experimental temperature | genetic line” (GxE; random slope) and “1 | genetic line” (G; random intercept) on tested parameters in juvenile Laminaria digitata sporophytes in experiment 2.
Absolute growth rates show the same pattern of genetic variation for plasticity in R5 (Supplementary Figure S1; mind the effect of initial size on growth rates between R5 and R15). Higher growth following G5 was evident in three out of five genetic lines (Supplementary Figures S1A,C,D) and in the overall mean response (Supplementary Figure S1F). Growth following R5 was either better at 15°C (Supplementary Figure S1A) or 5°C (Supplementary Figure S1B), and in one case, growth was higher in matching gametogenesis and experimental temperatures (Supplementary Figure S1C).
Biochemistry
All biochemical parameters were significantly influenced by gametogenesis temperature, experimental temperature, and interactive effects of gametogenesis × experimental temperature (Figures 4B–E and Table 4; for means over all genetic lines see Supplementary Figure S2). For all parameters, the main effect of experimental temperature was most distinct, indicating fast thermal acclimation capacity of biochemical pathways. Effects of gametogenesis temperature were weak. Overall, there were higher carbon, nitrogen and mannitol contents at E5 than at E15 (Figures 4B–E and Table 4). Additionally, genetic variation for plasticity (i.e., variation in reaction norm slopes) was significant for all biochemical parameters, but only following rearing at 5°C (Table 3).
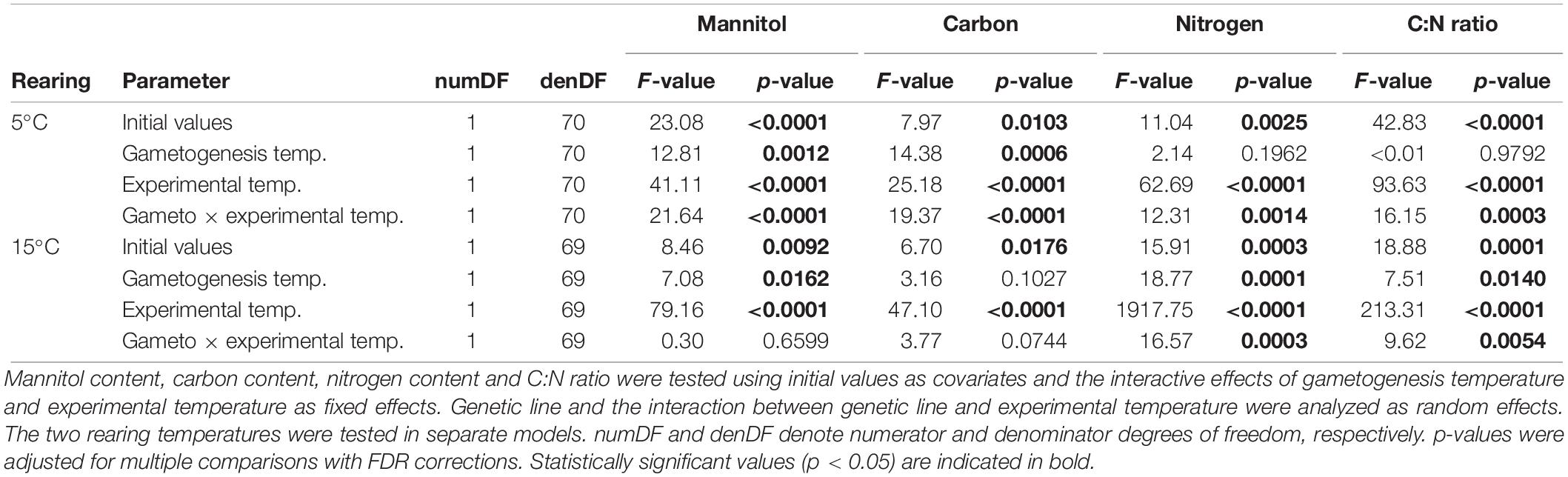
Table 4. Results of linear mixed effects models to examine thermal plasticity of biochemical parameters of juvenile Laminaria digitata sporophytes in experiment 2.
Mean mannitol content (Figure 4B) was significantly higher at E5 than at E15 for both rearing temperatures (R5: 128% higher at E5, p < 0.0001; R15: 68% higher, p < 0.0001; Table 4) irrespective of gametogenesis temperature. For mannitol, response magnitude (random intercept) did not differ significantly among genetic lines in either rearing temperature, but response plasticity (random slope) differed significantly among genetic lines in R5 (p < 0.0001; Table 3). The significant interaction of gametogenesis and experimental temperatures in R5 (p < 0.0001; Table 4) was likely driven by the reaction norms of two genetic lines in G5–R5 that had shallower slopes than the rest (Figure 4B; see also the crossing mean reaction norms in Supplementary Figure S2A).
A similar pattern arose for carbon content (Figure 4C), where values were significantly higher at E5 than at E15 for both rearing temperatures (R5: 4.5% higher at E5, p < 0.0001; R15: 4.0% higher, p < 0.0001; Table 4) irrespective of gametogenesis temperature. For carbon, response magnitude differed significantly among genetic lines in both rearing temperatures (R5: p = 0.0001; R15: p = 0.0005; Table 3), but response plasticity was only significant within R5 (p = 0.0024; Table 3). This again indicates genetic variation for plasticity only following rearing at 5°C, with two genetic lines in G5–R5 having comparatively shallow (and even opposite) slopes compared to the other lines (Figure 4C). The significant interaction of gametogenesis × experimental temperature in R5 (p < 0.0001; Table 4) is visible as crossing mean reaction norms of G5–R5 and G15–R5, due to lower mean carbon contents in G5–R5–E5 (Figure 4C; see also Supplementary Figure S2B).
Nitrogen contents (Figure 4D) were also primarily influenced by experimental temperature, with significantly higher values at E5 than at E15 for both rearing temperatures (R5: 26% higher at E5, p < 0.0001; R15: 34% higher, p < 0.0001; Table 4) irrespective of gametogenesis temperature. Again, response magnitude differed significantly among genetic lines in both rearing temperatures (R5: p < 0.0001; R15: p = 0.0075; Table 3), but response plasticity was only significant within R5 (p = 0.0239; Table 3). The interaction between gametogenesis and experimental temperatures was significant for both rearing temperatures (R5: p = 0.0014; R15: p = 0.0003; Table 4), seen as slight differences in overall reaction norm slopes between G5 and G15 (Supplementary Figure S2C).
C:N ratios (Figure 4E) generally followed a reverse pattern to that of nitrogen content because relative differences in carbon content among treatments were of much smaller magnitude than differences in nitrogen contents. C:N ratios were primarily influenced by experimental temperature, with significantly higher values at E15 than at E5 for both rearing temperatures (R5: 21% higher at E15, p < 0.0001; R15: 29% higher, p < 0.0001; Table 4) irrespective of gametogenesis temperature. Response magnitude differed significantly among genetic lines in both rearing temperatures (R5: p < 0.0001; R15: p = 0.0004; Table 3), but response plasticity was only significant within R5 (p = 0.0036; Table 3). The significant two-way interactions of gametogenesis × experimental temperature in both rearing temperatures (R5: p = 0.0003; R15: p = 0.0054; Table 4) are visible as slightly different (non-parallel) overall reaction norm slopes between G5 and G15 (Supplementary Figure S2D).
Fluorometry
Fluorometry results are presented as mean reaction norms over all genetic lines (Figure 5), as genetic variation for plasticity among genetic lines was not significant for most fluorometric parameters (Table 3; reaction norms of single genetic lines are presented in Supplementary Figure S3). Similarly to biochemistry, fluorometric characteristics were predominantly influenced by experimental temperature, with only occasional significant effects of gametogenesis temperature or interactive effects (Figure 5 and Table 5). For optimum quantum yield (Fv/Fm; Figure 5A), only experimental temperature had a significant effect within R15 (p = 0.0169; Table 5), in which Fv/Fm was significantly higher at E5 (0.775 ± 0.007, mean ± SD) than at E15 (0.767 ± 0.011, mean ± SD). Fv/Fm in juvenile sporophytes thereby showed a different response than the field material (experiment 1, Figure 2), in which values were significantly higher at 15°C across seasons. All samples expressed high Fv/Fm values (> 0.7), indicating good sample health over the course of the experiment. For Fv/Fm, response magnitude and plasticity did not differ significantly among genetic lines (Supplementary Figure S3, Table 3). Maximum relative electron transport rate (rETRmax; Figure 5B) and saturation irradiance (Ik; Figure 5C) responded similarly. Both were most strongly influenced by experimental temperature (p < 0.0001 for both parameters in R5 and R15; Table 5), with values at E15 about 30–70% higher than at E5. The significant gametogenesis × experimental temperature interaction within R15 for both rETRmax (p = 0.0169; Table 5) and Ik (p = 0.0162; Table 5) is shown by the steeper slopes of G5 compared to G15, leading to the highest values in G5–R15–E15 (Figures 5B,C). For rETRmax, response plasticity (random slope) significantly differed among genetic lines at R5 (p = 0.0224, Table 3, Supplementary Figure S3B), while for Ik, response magnitude (random intercept) significantly differed at R5 (p = 0.0327, Table 3 and Supplementary Figure S3C). Photosynthetic efficiency (α; Figure 5D) was not significantly affected by any of the tested factors (Tables 3, 5). For all fluorometric parameters, standard deviations among the four replicates within the genetic lines were comparable to the extent of variation among genetic lines (data not shown), which likely caused non-significance of random slopes and random intercepts, despite visual differences among mean reaction norms (Supplementary Figure S3).
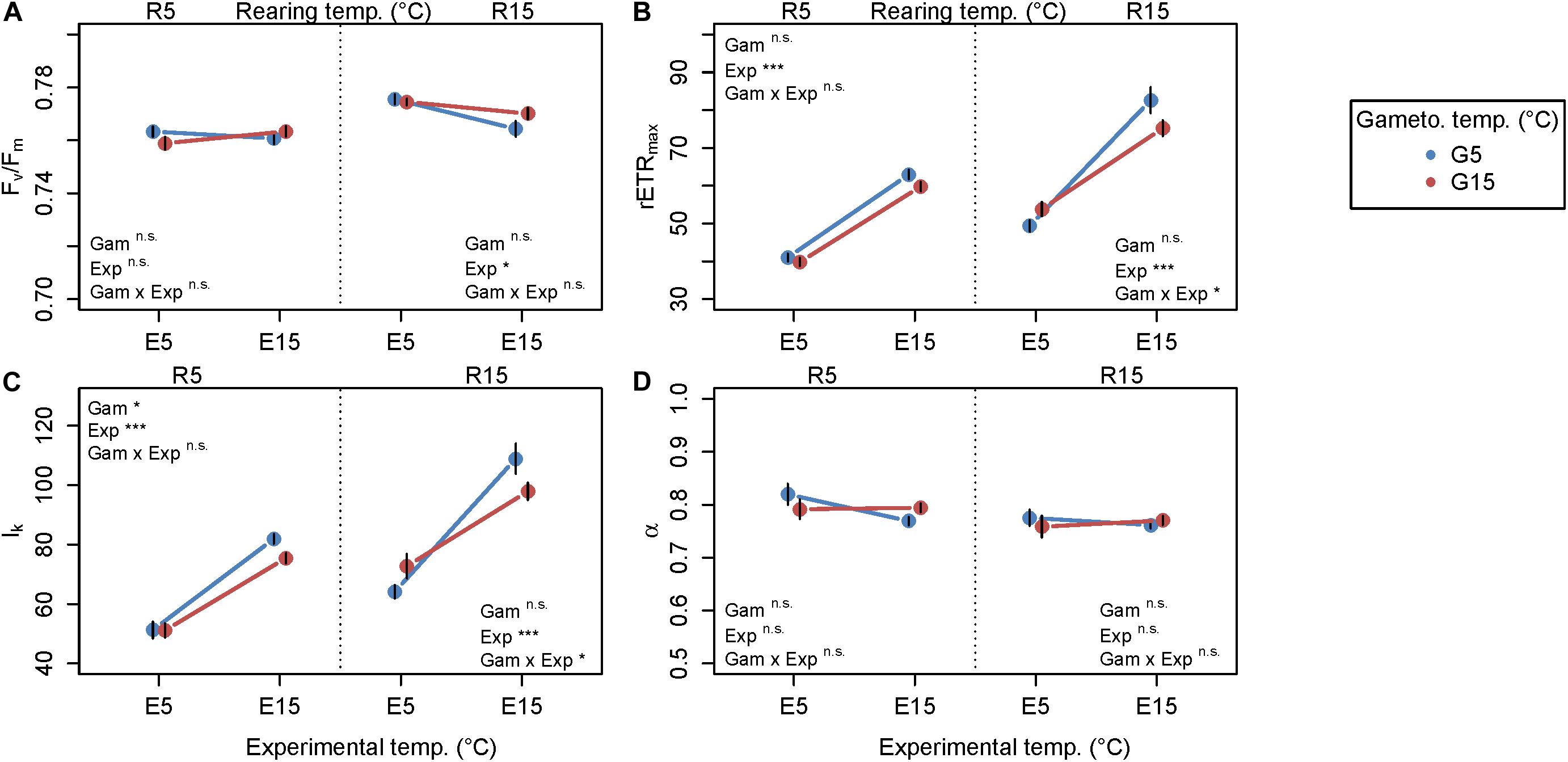
Figure 5. Reaction norms of fluorometric parameters averaged over all five genetic lines of juvenile Laminaria digitata sporophytes in experiment 2. Primary x-axis: experimental temperature, secondary x-axis: rearing temperature, symbol colors: gametogenesis temperature. All plots show values obtained at the end of the 12-day experiment. (A) Optimum quantum yield Fv/Fm. (B) Maximum relative electron transport rate rETRmax. (C) Saturation irradiance Ik (μmol photons m−2 s−1). (D) Photosynthetic efficiency α (rETR/μmol photons m−2 s−1). Mean values ± SE over all genetic lines, n = 20. Statistical significance of the fixed factors gametogenesis temperature (Gam), experimental temperature (Exp), and their interaction (Gam × Exp) is summarized in the figure; ***p < 0.001; *p < 0.05; n.s., not significant. For full statistical report see Table 5.
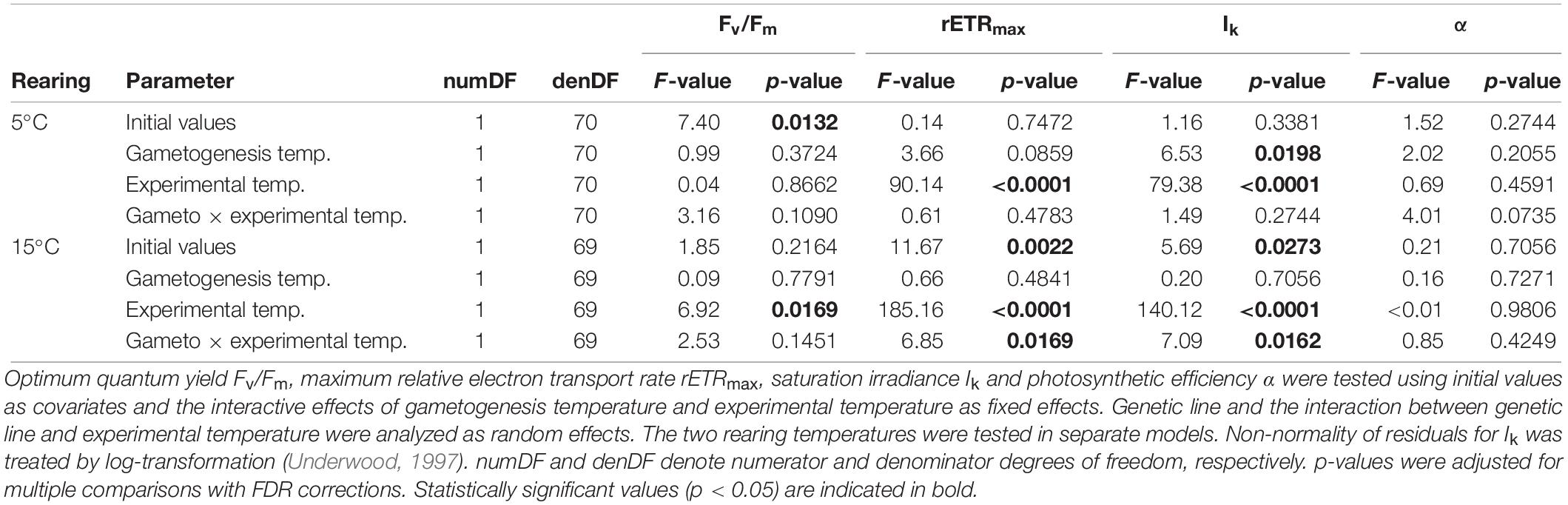
Table 5. Results of linear mixed effects models to examine thermal plasticity of fluorometric parameters of juvenile Laminaria digitata sporophytes in experiment 2.
Discussion
Our study was designed to test for effects of temperature during germline development and ontogeny on thermal plasticity of juvenile kelp sporophytes. Our major findings suggest that early exposure to cold temperature promotes the development of juvenile Laminaria digitata sporophytes. This contrasts our hypothesis that matching temperatures across ontogeny, specifically in the context of climate warming, should benefit kelp trait performance. Most importantly, gametogenesis and recruitment at 5°C promoted faster growth of 3–4 month old offspring sporophytes across all thermal environments, indicating persistent carry-over effects across ontogeny. Interestingly, reaction norm slopes (i.e., thermal plasticity) differed significantly among five genetic lines, but this variation for plasticity only became apparent in sporophytes reared at 5°C. Following rearing at cold temperature, there was no overall growth benefit of either 5 or 15°C among genetic lines due to high variation in plasticity. Such effects of temperature history and genotype may explain slight differences in previous reports of L. digitata’s temperature characteristics, where growth was either the same at 5 and 15°C (Bolton and Lüning, 1982) or better at 15°C (tom Dieck, 1992; Franke, 2019). We also detected interactive effects of gametogenesis and experimental temperatures on thermal plasticity of growth, biochemical and fluorometric characteristics of juvenile sporophytes, but these effects were weak, providing further evidence that potential cross-generational effects may not be a panacea in the face of climate change (Byrne et al., 2020). Biochemical and fluorometric parameters were generally highly plastic and mainly responded to experimental temperatures, indicating fast acclimation responses. For example, mannitol, carbon and nitrogen storage were significantly higher at 5°C compared to 15°C, while photosynthetic capacity increased at 15°C. Genetic variation for plasticity was also only evident following cold rearing for biochemical parameters. Taken together, trait performance was highest following either cold gametogenesis and recruitment, cold offspring rearing, or cold experimental temperatures, suggesting potential consequences for the persistence of cold-temperate kelp populations under climate warming.
Seasonal Growth of Wild L. digitata Sporophytes
In experiment 1, meristem tissue from wild sporophytes generally grew faster at 15°C compared to 5°C or at the same rate (May). Thus, our field-collected sporophytes responded similarly to published evidence from juvenile laboratory-grown L. digitata sporophytes, with either no difference in growth at 5 and 15°C (Bolton and Lüning, 1982) or a clear benefit of 15°C (tom Dieck, 1992; Franke, 2019). Higher growth at 15°C was especially striking in late winter following cold in situ temperatures. High growth rates in late winter can be explained by the start of the fast growing season for L. digitata (Perez, 1971; Kain, 1979; Gomez and Lüning, 2001), which is mediated by an endogenous circannual rhythmicity and the transition from short to long daylengths (Schaffelke and Lüning, 1994). Therefore, although conditions in the field modified the magnitude of temperature plasticity of Laminaria digitata adult sporophytes throughout the year, the general response pattern was stable, with warm temperatures generally favoring growth. However, the observation of higher growth of a few individuals at 5°C in May indicates that this pattern might not be true for all genotypes, especially when taking into account their environmental history.
Gametogenesis at Cold Temperature Promotes Offspring Sporophyte Growth
Growth responses of 3–4 month old juvenile sporophytes were not uniform, but differed depending on genetic line and their temperature history across generations and ontogeny (experiment 2). When comparing mean growth over G5 and G15, an experimental temperature of 15°C was beneficial (following R15) or the same (following R5) as 5°C for growth over a 12–day period, as shown in other studies (Bolton and Lüning, 1982; tom Dieck, 1992; Franke, 2019). However, in our study, growth responses differed significantly among temperature history treatments. Our initial hypothesis that matching gametogenesis/recruitment and experimental temperatures would improve trait performance was not confirmed. Rather, a gametogenesis temperature of 5°C increased sporophyte growth in the mismatching G5–R15–E15 treatment.
Most importantly, the gametogenesis treatment of 5°C generally improved growth of juvenile sporophytes in both rearing temperatures (Figure 3), and this was most strongly expressed in the matching rearing-experimental environments (G5–R5–E5 and G5–R15–E15). This indicates a beneficial effect of a cold environment during gametogenesis and/or recruitment which persisted in juvenile sporophyte offspring. Several potential underlying mechanisms may be involved: exposure of newly recruited sporophytes to the cold parental environment might have induced beneficial carry-over effects within the sporophyte generation (Palmer et al., 2012; Byrne et al., 2020). For instance, a stimulating effect of 5°C on assimilative processes (see carbon and nitrogen storage, Figure 4) of newly recruited sporophytes might have mediated faster growth in 3–4 month-old sporophytes. Potentially, epigenetic mechanisms such as DNA methylation (Qu et al., 2013; Fan et al., 2020) or histone modification (Bourdareau, 2018; Pearson et al., 2019) initiated during early ontogeny may facilitate changes in gene transcription during later life. However, this emerging field of research does not yet provide substantial evidence for these processes in kelps. Alternatively, the mediating factor could be exposure of the parental germline to cold temperature. In this respect, our results could reflect a “silver spoon” parental effect (Uller et al., 2013; Baker et al., 2019; Germain et al., 2019). “Silver spoon” effects describe an advantage for individuals born to parents with access to abundant resources, which benefits offspring through, e.g., maternal provisioning. Mechanisms facilitating resource-based “silver spoon” effects in kelps could potentially include energy transfer between haploid and diploid generations, for example via gamete lipid content (Brzezinski et al., 1993). Neutral lipids are the major form of carbon storage in kelp meiospores and gametes (eggs and sperm), and are therefore crucial for kelp reproduction (Brzezinski et al., 1993; Reed et al., 1999). A higher lipid concentration in germ cells could give zygotes an energetic advantage across generations, but temperature effects on lipid storage are as yet unknown.
Interestingly, in L. digitata, gametogenesis is faster at 15°C than 5°C, but recruitment is twice as high at 5°C (Martins et al., 2017). This might be due to the prolonged vegetative growth of gametophytes at 5°C compared to 15°C (pers. obs.), which may result in more female cells per gametophyte. As all female cells may develop into oogonia, this was previously discussed as a potentially adaptive response increasing recruitment at non-optimal reproductive conditions (Bolton and Levitt, 1985). Our study reveals that in addition to higher recruitment at cold temperature (Martins et al., 2017), gametogenesis and recruitment at cold temperature seems to be a prerequisite for enhanced juvenile sporophyte growth of L. digitata. This effect was evident under constant long daylengths, which suppress the free-running growth rhythm of L. digitata (Schaffelke and Lüning, 1994). Whether photoperiod or endogenous circannual rhythms also affect thermal plasticity of L. digitata is yet unknown.
High Acclimation Potential of Biochemical and Fluorometric Parameters
Biochemical contents (mannitol, carbon, nitrogen) of juvenile sporophytes were mostly influenced by acclimation responses to experimental temperatures, favoring 5 over 15°C (Figure 4 and Table 4), and only showed weak interactive effects between gametogenesis and experimental temperature. Therefore, effects of temperature during gametogenesis and recruitment on biochemical trait plasticity might be negligible in the wild compared to the high and fast acclimation capacity in response to the immediate environment. Increased carbon and mannitol contents at 5°C indicated more efficient carbon assimilation processes at cold temperature, despite possibly lower photosynthetic capacity (Figure 5). This contrasts the naturally occurring peaks in carbon storage in late summer when growth is minimal (Haug and Jensen, 1954; Schiener et al., 2015). Naturally, a temperature of 5°C would rather co-occur with short daylengths at our study location of Helgoland. Therefore, the pattern of high growth and high carbon storage at 5°C might be a product of experimental long days in our experiment, which may stimulate carbon storage accumulation (in L. hyperborea; Schaffelke, 1995).
Fluorometric results indicated photoacclimative responses to experimental temperature (Figure 5 and Table 5), but in contrast to biochemical contents, the maximum relative electron transport rate (rETRmax) and saturation irradiance (Ik) were promoted at warm temperature. Photosynthetic rates usually increase at higher, sublethal temperatures (Delebecq et al., 2016; Burdett et al., 2019), with photosynthetic thermal optima often exceeding thermal growth optima (Eggert and Wiencke, 2000; Graiff et al., 2015; Fernández et al., 2020). In E5, maximum relative electron transport rate rETRmax was close to the irradiance conditions in our experiment (30–40 μmol photons m–2 s–1), while rETRmax was higher at E15. Therefore, faster metabolism and higher energy demands at 15°C might have reduced carbon and mannitol storage compared to 5°C.
Nitrogen contents were highest in the matching G5–R5–E5 thermal history and lowest in G15–R15–E15 (Figure 4 and Table 4). Due to the high amount of nitrogen storage in all E5 treatments, C:N ratios were approximating the Redfield ratio of 106C:16N (= 6.625). Atkinson and Smith (1983) described this value as the lower limit, which is only attainable in strongly nitrogen-enriched environments, such as present in our PES cultivation medium (Sarker et al., 2013), and which is characteristic of rapid growth phases in seaweeds (Niell, 1976). As all samples were treated with the same nutrient enrichment and all grew well, the high nitrogen contents only in E5 were probably not the cause of fast growth. From an ecological standpoint, high internal nitrogen concentrations at cold temperature might indicate a seasonal pattern, as L. digitata’s growth period starts in late winter to spring, when the nutrient content of seawater is high (Davison et al., 1984; Wiltshire et al., 2015). Nitrate reductase (NR) activity peaks in spring in a Scottish L. digitata population (Davison et al., 1984), despite a potentially wide temperature range for kelp NR activity (Fernández et al., 2020), suggesting that the year-round uptake potential might be controlled by interactive seasonal patterns of nitrate availability, NR activity, temperature and irradiance, among others (Young et al., 2007a, b). However, nutrient uptake across temperature or seasonal gradients is rarely studied for seaweeds (Roleda and Hurd, 2019).
Genetic Variation for Plasticity
Variation for plasticity among genotypes offers a target for selection in a warming environment if the variation of traits is heritable (Chevin et al., 2010; Munday et al., 2017, 2019), and is an important component of the adaptive capacity of populations and species. Already during recruitment of juvenile sporophytes for experiment 2, we observed that one out of ten genetic lines recruited more sporophytes at 15°C than at 5°C (Supplementary Figure S4 and Supplementary Table S3). This was a first indication that the tested genetic lines might express differential plasticity and differing temperature preferences. This was also indicated in experiment 1, where in May, a few individuals had higher growth at 5°C than 15°C (Supplementary Table S1), whereas mean growth of all 20 individuals did not differ between 5 and 15°C (Figure 2).
In experiment 2, thermal reaction norms of growth and biochemical characteristics varied in magnitude (intercept) and plasticity (slope) among the five genetic lines (Figure 4 and Table 3), indicating that genetic lines differed in their expression of genotype × environment interactions (see also Galloway, 2001; Suter and Widmer, 2013). Interestingly, while the magnitude of response varied significantly in both rearing temperatures, only the 5°C rearing environment was associated with significant variation for plasticity among the five genetic lines (Figure 4 and Table 3). Therefore, temperature history modulated the extent of plastic responses, in that cold rearing generally induced higher thermal plasticity in growth and biochemical responses than warm rearing. Variation for thermal plasticity was most obvious for growth, where each genetic line responded differently (Figure 4 and Supplementary Figure S1). This variation in thermal responses across genetic lines seemed to outweigh the effects of temperature history treatments (gametogenesis/recruitment and rearing) on response plasticity toward the experimental temperatures (Figures 3, 4).
Our results show that thermal responses were highly plastic, and that better growth of L. digitata at 15°C might not be universal, but differs depending on genetic background and thermal history. Furthermore, thermal carry-over effects from the rearing treatment possibly modulated the extent of genotype × environment interactions on trait responses. As phenotypic trait variation is an important target for natural selection (Chevin et al., 2010; Kelly, 2019), this could have important consequences for the adaptive capacity of Laminaria digitata populations during ocean warming. For instance, if phenotypic variation of adaptive traits among genotypes is lower in sporophytes recruited at warmer temperature, then selection is less able to remove maladaptations from a population. This in turn would decrease the strength of directional selection (Ghalambor et al., 2015), and therefore could reduce the adaptive capacity of the population in a negative feedback loop under increasingly warm temperature.
The Importance of Cold Seasons
We hypothesized that trait performance should increase in matching parent gametophyte and offspring sporophyte environments. However, interactive temperature effects played a minor role in shaping the reaction norms of juvenile L. digitata sporophytes. Rather, gametogenesis and recruitment at 5°C benefited mean growth of juvenile sporophytes, sporophyte rearing at 5°C enabled high thermal plasticity, and carbon and nitrogen contents significantly increased in the 5°C experimental treatment. In this study, we add to previous evidence of higher recruitment at a cold temperature (5°C) compared to a warm temperature (15°C) for L. digitata (Martins et al., 2017), and show a general disadvantage of early ontogeny at 15°C compared to 5°C for juvenile L. digitata sporophytes.
Across L. digitata’s European distribution, temperature regimes differ substantially. While mean monthly SST vary between 0 and 7°C over the year in Kongsfjorden, Spitsbergen (Müller et al., 2009; Bartsch et al., 2016), mean monthly SST range between 7 and 18°C at the southern distribution limit in Quiberon, France (Oppliger et al., 2014). L. digitata sporophytes become fertile mostly from early summer to late autumn along their latitudinal distribution (Sjøtun and Schoschina, 2002; Bartsch et al., 2008, 2013; Olischläger and Wiencke, 2013). According to our results, northern to Arctic populations may be favored by gametogenesis, recruitment and growth of early sporophytes during summer to autumn conditions (Sjøtun and Schoschina, 2002) at cold temperature (5°C) and long daylength. Further, these conditions increase sporophyte recruitment compared to warmer temperatures and/or a short photoperiod (Martins et al., 2017). Conversely, summer temperatures at the warm distribution limit (≥18°C) such as at our study location Helgoland (Figure 2; Wiltshire et al., 2008; Bartsch et al., 2013) or Quiberon (Oppliger et al., 2014), may induce vegetative persistence of gametophytes after summer spore release (tom Dieck, 1992; Martins et al., 2017). We provide evidence that recruitment at the end of southern summers, when decreasing temperatures allow gametogenesis (e.g., 15°C; Martins et al., 2017), might lead to reduced growth and reduced thermal plasticity of juvenile sporophytes. The comparative benefit of early ontogeny at 5°C demonstrated in this study will be achievable throughout the year at northern locations, but only in winter to spring at southern locations. Subsequent investigations into optimal conditions for recruitment and thermal plasticity are needed, as temperature and daylength may shape responses interactively (Schaffelke and Lüning, 1994; Martins et al., 2017), and as populations might differ in their thermal plasticity (King et al., 2018, 2019).
With a predicted warming of the North Atlantic by 1–3°C (Müller et al., 2009; Schrum et al., 2016), southern L. digitata populations are threatened by rising maximum temperatures and marine heatwaves (Raybaud et al., 2013; Assis et al., 2018). This is especially true for populations where sporophytes already encounter temperatures at their upper tolerance limit (e.g., Helgoland, North Sea, Bartsch et al., 2013; Quiberon, Brittany, Oppliger et al., 2014), which affect traits such as reproduction, photosynthesis, growth and mortality (Bartsch et al., 2013; Burdett et al., 2019; Nepper-Davidsen et al., 2019). As suggested by our data, rising temperatures might not only have immediate detrimental effects such as local extinctions of populations due to marine heatwaves (reviews by Straub et al., 2019; Smale, 2020). Reduced growth and thermal plasticity induced by warming during early ontogeny might additionally lead to an overall weakened performance of southern L. digitata populations.
Data Availability Statement
The raw data and R code generated during this study are available in the PANGAEA database (https://doi.org/10.1594/PANGAEA.917810).
Author Contributions
DL, LS, and IB planned and designed the experiments. DL conducted the experiments. ND performed PAM measurements and rapid light curve fits. DL and LS analyzed the data. IB supervised the project. DL, IB, LS, ND, and KV contributed to the data interpretation and discussion. DL wrote the manuscript, which was reviewed, revised, and approved by all authors. All authors contributed to the article and approved the submitted version.
Funding
This research was funded through the 2015–2016 BiodivERsA COFUND call for research proposals (program MARFOR), with the national funder German Research Foundation (DFG; grant no. VA 105/25-1).
Conflict of Interest
The authors declare that the research was conducted in the absence of any commercial or financial relationships that could be construed as a potential conflict of interest.
Acknowledgments
We thank A. Wagner, C. Daniel, and N. Martins for technical support in sampling, cultivation, and sample processing; P. Pickenbrock for conducting the seasonal growth experiment in February; U. Karsten, A. Graiff, and J. Müller for support and infrastructure in the mannitol analysis; K. M. Wegner for statistical support, K. Bischof for supervision in this Ph.D. project; and two reviewers who provided valuable comments on an earlier version of the manuscript.
Supplementary Material
The Supplementary Material for this article can be found online at: https://www.frontiersin.org/articles/10.3389/fmars.2020.00456/full#supplementary-material
References
Assis, J., Araújo, M. B., and Serrão, E. A. (2018). Projected climate changes threaten ancient refugia of kelp forests in the North Atlantic. Glob. Chang. Biol. 24, e55–e66. doi: 10.1111/gcb.13818
Atkinson, M. J., and Smith, S. V. (1983). C:N:P ratios of benthic marine plants. Limnol. Oceanogr. 28, 568–574. doi: 10.4319/lo.1983.28.3.0568
Baker, B. H., Sultan, S. E., Lopez-Ichikawa, M., and Waterman, R. (2019). Transgenerational effects of parental light environment on progeny competitive performance and lifetime fitness. Philos. Trans. R. Soc. B Biol. Sci. 374:20180182. doi: 10.1098/rstb.2018.0182
Bartsch, I. (2018). “Derivation of clonal stock cultures and hybridization of kelps,” in Protocols for Macroalgae Research, eds B. Charrier, T. Wichard, and C. R. K. Reddy (Boca Raton, FL: CRC Press), 61–78. doi: 10.1201/b21460-3
Bartsch, I., Paar, M., Fredriksen, S., Schwanitz, M., Daniel, C., Hop, H., et al. (2016). Changes in kelp forest biomass and depth distribution in Kongsfjorden, Svalbard, between 1996–1998 and 2012–2014 reflect Arctic warming. Polar Biol. 39, 2021–2036. doi: 10.1007/s00300-015-1870-1
Bartsch, I., Vogt, J., Pehlke, C., and Hanelt, D. (2013). Prevailing sea surface temperatures inhibit summer reproduction of the kelp Laminaria digitata at Helgoland (North Sea). J. Phycol. 49, 1061–1073. doi: 10.1111/jpy.12125
Bartsch, I., Wiencke, C., Bischof, K., Buchholz, C. M., Buck, B. H., Eggert, A., et al. (2008). The genus Laminaria sensu lato: recent insights and developments. Eur. J. Phycol. 43, 1–86. doi: 10.1080/09670260701711376
Benjamini, Y., and Hochberg, Y. (1995). Controlling the false discovery rate: a practical and powerful approach to multiple testing. J. R. Stat. Soc. Ser. B 57, 289–300. doi: 10.2307/2346101
Black, W. A. P. (1954). Concentration gradients and their significance in Laminaria saccharina (L.) Lamour. J. Mar. Biol. Assoc. U.K. 33, 49–60. doi: 10.1017/S0025315400003465
Bolton, J. J., and Levitt, G. J. (1985). Light and temperature requirements for growth and reproduction in gametophytes of Ecklonia maxima (Alariaceae: Laminariales). Mar. Biol. 87, 131–135. doi: 10.1007/BF00539420
Bolton, J. J., and Lüning, K. (1982). Optimal growth and maximal survival temperatures of Atlantic Laminaria species (Phaeophyta) in culture. Mar. Biol. 66, 89–94. doi: 10.1007/BF00397259
Bourdareau, S. (2018). Genetic and Epigenetic Control of Life Cycle Transitions in the Brown alga Ectocarpus sp. Ph.D. thesis, Sorbonne Université, Paris.
Bradshaw, A. D. (1965). “Evolutionary significance of phenotypic plasticity in plants,” in Advances in Genetics, Vol. 13, eds E. W. Caspari and L. M. Thoday (New York, NY: Academic Press), 115–155. doi: 10.1016/S0065-2660(08)60048-6
Brzezinski, M. A., Reed, D. C., and Amsler, C. D. (1993). Neutral lipids as major storage products in zoospores of the giant kelp Macrocystis pyrifera (Phaeophyceae). J. Phycol. 29, 16–23. doi: 10.1111/j.1529-8817.1993.tb00275.x
Buchholz, C., and Lüning, K. (1999). Isolated, distal blade discs of the brown alga Laminaria digitata form sorus, but not discs, near to the meristematic transition zone. J. Appl. Phycol. 11, 579–584. doi: 10.1023/A:1008116828263
Burdett, H. L., Wright, H., and Smale, D. A. (2019). Photophysiological responses of canopy-forming kelp species to short-term acute warming. Front. Mar. Sci. 6:516. doi: 10.3389/fmars.2019.00516
Byrne, M., Foo, S. A., Ross, P. M., and Putnam, H. M. (2020). Limitations of cross- and multigenerational plasticity for marine invertebrates faced with global climate change. Glob. Chang. Biol. 26, 80–102. doi: 10.1111/gcb.14882
Chevin, L.-M., Lande, R., and Mace, G. M. (2010). Adaptation, plasticity, and extinction in a changing environment: towards a predictive theory. PLoS Biol. 8:e1000357. doi: 10.1371/journal.pbio.1000357
Dankworth, M., Heinrich, S., Fredriksen, S., and Bartsch, I. (2020). DNA barcoding and mucilage ducts in the stipe reveal the presence of Hedophyllum nigripes (Laminariales, Phaeophyceae) in Kongsfjorden (Spitsbergen). J. Phycol. doi: 10.1111/jpy.13012 [Epub ahead of print].
Davison, I. R., Andrews, M., and Stewart, W. D. P. (1984). Regulation of growth in Laminaria digitata: use of in-vivo nitrate reductase activities as an indicator of nitrogen limitation in field populations of Laminaria spp. Mar. Biol. 84, 207–217. doi: 10.1007/BF00393006
Davison, I. R., and Davison, J. O. (1987). The effect of growth temperature on enzyme activities in the brown alga Laminaria saccharina. Br. Phycol. J. 22, 77–87. doi: 10.1080/00071618700650101
Davison, I. R., Greene, R. M., and Podolak, E. J. (1991). Temperature acclimation of respiration and photosynthesis in the brown alga Laminaria saccharina. Mar. Biol. 110, 449–454. doi: 10.1007/BF01344363
Davison, I. R., and Reed, R. H. (1985). The physiological significance of mannitol accumulation in brown algae: the role of mannitol as a compatible cytoplasmic solute. Phycologia 24, 449–457. doi: 10.2216/i0031-8884-24-4-449.1
Delebecq, G., Davoult, D., Janquin, M. A., Oppliger, L. V., Menu, D., Dauvin, J. C., et al. (2016). Photosynthetic response to light and temperature in Laminaria digitata gametophytes from two French populations. Eur. J. Phycol. 51, 71–82. doi: 10.1080/09670262.2015.1104556
Donelson, J. M., Salinas, S., Munday, P. L., and Shama, L. N. S. (2018). Transgenerational plasticity and climate change experiments: where do we go from here? Glob. Chang. Biol. 24, 13–34. doi: 10.1111/gcb.13903
Donelson, J. M., Sunday, J. M., Figueira, W. F., Gaitán-Espitia, J. D., Hobday, A. J., Johnson, C. R., et al. (2019). Understanding interactions between plasticity, adaptation and range shifts in response to marine environmental change. Philos. Trans. R. Soc. B Biol. Sci. 374:20180186. doi: 10.1098/rstb.2018.0186
Edwards, M. S. (2000). The role of alternate life-history stages of a marine macroalga: a seed bank analogue? Ecology 81, 2404–2415. doi: 10.2307/177463
Eggert, A., and Wiencke, C. (2000). Adaptation and acclimation of growth and photosynthesis of five Antarctic red algae to low temperatures. Polar Biol. 23, 609–618. doi: 10.1007/s003000000130
Engqvist, L., and Reinhold, K. (2016). Adaptive trans-generational phenotypic plasticity and the lack of an experimental control in reciprocal match/mismatch experiments. Methods Ecol. Evol. 7, 1482–1488. doi: 10.1111/2041-210X.12618
Fan, X., Han, W., Teng, L., Jiang, P., Zhang, X., Xu, D., et al. (2020). Single-base methylome profiling of the giant kelp Saccharina japonica reveals significant differences in DNA methylation to microalgae and plants. New Phytol. 225, 234–249. doi: 10.1111/nph.16125
Fernández, P. A., Gaitán-Espitia, J. D., Leal, P. P., Schmid, M., Revill, A. T., and Hurd, C. L. (2020). Nitrogen sufficiency enhances thermal tolerance in habitat-forming kelp: implications for acclimation under thermal stress. Sci. Rep. 10:3186. doi: 10.1038/s41598-020-60104-4
Fox, R. J., Donelson, J. M., Schunter, C., Ravasi, T., and Gaitán-Espitia, J. D. (2019). Beyond buying time: the role of plasticity in phenotypic adaptation to rapid environmental change. Philos. Trans. R. Soc. B Biol. Sci. 374:20180174. doi: 10.1098/rstb.2018.0174
Franke, K. (2019). Performance of Different life Cycle Stages of the Arctic Kelps Laminaria digitata and Saccharina nigripes along Temperature Gradients. Master’s thesis, University of Bremen, Bremen.
Galloway, L. F. (2001). Parental environmental effects on life history in the herbaceous plant Campanula americana. Ecology 82, 2781–2789. doi: 10.1890/0012-9658(2001)082[2781:peeolh]2.0.co;2
Gerard, V. A., and Du Bois, K. R. (1988). Temperature ecotypes near the southern boundary of the kelp Laminaria saccharina. Mar. Biol. 97, 575–580. doi: 10.1007/BF00391054
Germain, R. M., Grainger, T. N., Jones, N. T., and Gilbert, B. (2019). Maternal provisioning is structured by species’ competitive neighborhoods. Oikos 128, 45–53. doi: 10.1111/oik.05530
Ghalambor, C. K., Hoke, K. L., Ruell, E. W., Fischer, E. K., Reznick, D. N., and Hughes, K. A. (2015). Non-adaptive plasticity potentiates rapid adaptive evolution of gene expression in nature. Nature 525, 372–375. doi: 10.1038/nature15256
Gomez, I., and Lüning, K. (2001). Constant short-day treatment of outdoor-cultivated Laminaria digitata prevents summer drop in growth rate. Eur. J. Phycol. 36, 391–395. doi: 10.1080/09670260110001735548
Graiff, A., Liesner, D., Karsten, U., and Bartsch, I. (2015). Temperature tolerance of western Baltic Sea Fucus vesiculosus - growth, photosynthesis and survival. J. Exp. Mar. Biol. Ecol. 471, 8–16. doi: 10.1016/j.jembe.2015.05.009
Groisillier, A., Shao, Z., Michel, G., Goulitquer, S., Bonin, P., Krahulec, S., et al. (2014). Mannitol metabolism in brown algae involves a new phosphatase family. J. Exp. Bot. 65, 559–570. doi: 10.1093/jxb/ert405
Grossniklaus, U. (2011). Plant germline development: a tale of cross-talk, signaling, and cellular interactions. Sex. Plant Reprod. 24, 91–95. doi: 10.1007/s00497-011-0170-3
Han, T., and Kain, J. M. (1996). Effect of photon irradiance and photoperiod on young sporophytes of four species of the Laminariales. Eur. J. Phycol. 31, 233–240. doi: 10.1080/09670269600651431
Hanelt, D. (2018). “Photosynthesis assessed by chlorophyll fluorescence,” in Bioassays, eds D.-P. Häder and G. S. Erzinger (Amsterdam: Elsevier), 169–198. doi: 10.1016/B978-0-12-811861-0.00009-7
Hargrave, M. S., Foggo, A., Pessarrodona, A., and Smale, D. A. (2017). The effects of warming on the ecophysiology of two co-existing kelp species with contrasting distributions. Oecologia 183, 531–543. doi: 10.1007/s00442-016-3776-1
Haug, A., and Jensen, A. (1954). Seasonal Variations in the Chemical Composition of Alaria esculenta, Laminaria saccharina, Laminaria hyperborea and Laminaria digitata from Northern Norway. Nor. Inst. tang- og Tareforsk., Report No. 4. Oslo: Akademisk Trykningssentral.
Herman, J. J., and Sultan, S. E. (2016). DNA methylation mediates genetic variation for adaptive transgenerational plasticity. Proc. R. Soc. B Biol. Sci. 283:20160988. doi: 10.1098/rspb.2016.0988
Hurd, C. L., Harrison, P. J., and Druehl, L. D. (1996). Effect of seawater velocity on inorganic nitrogen uptake by morphologically distinct forms of Macrocystis integrifolia from wave-sheltered and exposed sites. Mar. Biol. 126, 205–214. doi: 10.1007/BF00347445
Jassby, A. D., and Platt, T. (1976). Mathematical formulation of the relationship between photosynthesis and light for phytoplankton. Limnol. Oceanogr. 21, 540–547. doi: 10.4319/lo.1976.21.4.0540
Karsten, U., Thomas, D. N., Weykam, G., Daniel, C., and Kirst, G. O. (1991). A simple and rapid method for extraction and separation of low molecular weight carbohydrates from macroalgae using high-performance liquid chromatography. Plant Physiol. Biochem. 29, 373–378.
Kelly, M. (2019). Adaptation to climate change through genetic accommodation and assimilation of plastic phenotypes. Philos. Trans. R. Soc. B Biol. Sci. 374:20180176. doi: 10.1098/rstb.2018.0176
King, N. G., McKeown, N. J., Smale, D. A., and Moore, P. J. (2018). The importance of phenotypic plasticity and local adaptation in driving intraspecific variability in thermal niches of marine macrophytes. Ecography 41, 1469–1484. doi: 10.1111/ecog.03186
King, N. G., McKeown, N. J., Smale, D. A., Wilcockson, D. C., Hoelters, L., Groves, E. A., et al. (2019). Evidence for different thermal ecotypes in range centre and trailing edge kelp populations. J. Exp. Mar. Biol. Ecol. 514–515, 10–17. doi: 10.1016/j.jembe.2019.03.004
Lenth, R. (2019). emmeans: Estimated Marginal Means, aka Least-Squares Means. Available online at: https://cran.r-project.org/package=emmeans (accessed April 3, 2019).
Li, H., Monteiro, C., Heinrich, S., Bartsch, I., Valentin, K., Harms, L., et al. (2019). Responses of the kelp Saccharina latissima (Phaeophyceae) to the warming Arctic: from physiology to transcriptomics. Physiol. Plant. 168, 5–26. doi: 10.1111/ppl.13009
Li, R., and Brawley, S. H. (2004). Improved survival under heat stress in intertidal embryos (Fucus spp.) simultaneously exposed to hypersalinity and the effect of parental thermal history. Mar. Biol. 144, 205–213. doi: 10.1007/s00227-003-1190-9
Lüning, K. (1979). Growth strategies of three Laminaria species (Phaeophyceae) inhabiting different depth zones in the sublittoral region of Helgoland (North Sea). Mar. Ecol. Prog. Ser. 1, 195–207. doi: 10.3354/meps001195
Lüning, K. (1980). Critical levels of light and temperature regulating the gametogenesis of three Laminaria species (Phaeophyceae). J. Phycol. 16, 1–15. doi: 10.1111/j.1529-8817.1980.tb02992.x
Lüning, K. (1984). Temperature tolerance and biogeography of seaweeds: the marine algal flora of Helgoland (North Sea) as an example. Helgol. Meeresunters. 38, 305–317. doi: 10.1007/BF01997486
Lüning, K. (1990). Seaweeds: Their Environment, Biogeography, and Ecophysiology. New York, NY: John Wiley & Sons.
Lüning, K. (1991). Circannual growth rhythm in a brown alga, Pterygophora californica. Bot. Acta 104, 157–162. doi: 10.1111/j.1438-8677.1991.tb00211.x
Mabin, C. J. T., Johnson, C. R., and Wright, J. T. (2019a). Family-level variation in early life-cycle traits of kelp. J. Phycol. 55, 380–392. doi: 10.1111/jpy.12820
Mabin, C. J. T., Johnson, C. R., and Wright, J. T. (2019b). Physiological response to temperature, light, and nitrates in the giant kelp Macrocystis pyrifera from Tasmania. Australia. Mar. Ecol. Prog. Ser. 614, 1–19. doi: 10.3354/meps12900
Machalek, K. M., Davison, I. R., and Falkowski, P. G. (1996). Thermal acclimation and photoacclimation of photosynthesis in the brown alga Laminaria saccharina. Plant Cell Environ. 19, 1005–1016. doi: 10.1111/j.1365-3040.1996.tb00207.x
Manns, D., Nielsen, M. M., Bruhn, A., Saake, B., and Meyer, A. S. (2017). Compositional variations of brown seaweeds Laminaria digitata and Saccharina latissima in Danish waters. J. Appl. Phycol. 29, 1493–1506. doi: 10.1007/s10811-017-1056-z
Martins, N., Tanttu, H., Pearson, G. A., Serrão, E. A., and Bartsch, I. (2017). Interactions of daylength, temperature and nutrients affect thresholds for life stage transitions in the kelp Laminaria digitata (Phaeophyceae). Bot. Mar. 60, 109–121. doi: 10.1515/bot-2016-0094
Maxwell, K., and Johnson, G. N. (2000). Chlorophyll fluorescence – a practical guide. J. Exp. Bot. 51, 659–668. doi: 10.1093/jexbot/51.345.659
Müller, R., Laepple, T., Bartsch, I., and Wiencke, C. (2009). Impact of oceanic warming on the distribution of seaweeds in polar and cold-temperate waters. Bot. Mar. 52, 617–638. doi: 10.1515/BOT.2009.080
Munday, P. L., Donelson, J. M., and Domingos, J. A. (2017). Potential for adaptation to climate change in a coral reef fish. Glob. Chang. Biol. 23, 307–317. doi: 10.1111/gcb.13419
Munday, P. L., Schunter, C., Allan, B. J. M., Nicol, S., Parsons, D. M., Pether, S. M. J., et al. (2019). Testing the adaptive potential of yellowtail kingfish to ocean warming and acidification. Front. Ecol. Evol. 7:253. doi: 10.3389/fevo.2019.00253
Nepper-Davidsen, J., Andersen, D., and Pedersen, M. (2019). Exposure to simulated heatwave scenarios causes long-term reductions in performance in Saccharina latissima. Mar. Ecol. Prog. Ser. 630, 25–39. doi: 10.3354/meps13133
Newman, R. A. (1994). Genetic variation for phenotypic plasticity in the larval life history of Spadefoot Toads (Scaphiopus couchii). Evolution 48, 1773–1785. doi: 10.2307/2410507
Nicotra, A. B., Atkin, O. K., Bonser, S. P., Davidson, A. M., Finnegan, E. J., Mathesius, U., et al. (2010). Plant phenotypic plasticity in a changing climate. Trends Plant Sci. 15, 684–692. doi: 10.1016/j.tplants.2010.09.008
Niell, F. X. (1976). C:N ratio in some marine macrophytes and its possible ecological significance. Bot. Mar. 19, 347–350. doi: 10.1515/botm.1976.19.6.347
Olischläger, M., and Wiencke, C. (2013). Seasonal fertility and combined effects of temperature and UV-radiation on Alaria esculenta and Laminaria digitata (Phaeophyceae) from Spitsbergen. Polar Biol. 36, 1019–1029. doi: 10.1007/s00300-013-1325-5
Oppliger, L. V., von Dassow, P., Bouchemousse, S., Robuchon, M., Valero, M., Correa, J. A., et al. (2014). Alteration of sexual reproduction and genetic diversity in the kelp species Laminaria digitata at the southern limit of its range. PLoS One 9:e102518. doi: 10.1371/journal.pone.0102518
Palmer, C. M., Bush, S. M., and Maloof, J. N. (2012). “Phenotypic and developmental plasticity in plants,” in eLS (Chichester: John Wiley & Sons Ltd), 1–19. doi: 10.1002/9780470015902.a0002092.pub2
Pearson, G. A., Martins, N., Madeira, P., Serrão, E. A., and Bartsch, I. (2019). Sex-dependent and -independent transcriptional changes during haploid phase gametogenesis in the sugar kelp Saccharina latissima. PLoS One 14:e0219723. doi: 10.1371/journal.pone.0219723
Perez, R. (1971). Ecologie, croissance et régénération, teneurs en acide alginique de Laminaria digitata sur les côtes françaises de la Manche. Rev. Trav. Pêches Marit. 35, 287–346.
Pinheiro, J., Bates, D., DebRoy, S., Sarkar, D., and R Core Team. (2019). {nlme}: Linear and Nonlinear Mixed Effects Models. Available online at: https://cran.r-project.org/package=nlme (accessed April 3, 2019).
Provasoli, L. (1968). “Media and prospects for the cultivation of marine algae. in Cultures and Collections of Algae,” in Proceedings of the US-Japan Conference, Hakone, September 1966, eds A. Watanabe and A. Hattori (Tokyo: Japan Society of Plant Physiology), 63–75.
Qu, J.-Q., Wang, X., Liu, C., Li, X.-L., Zheng, X.-L., and Liu, T. (2013). Preliminary analysis of DNA methylationin of Saccharina japonica with MSAP. Period. Ocean Univ. China 43, 54–59.
R Core Team (2019). R: A Language and Environment for Statistical Computing. Available online at: https://www.r-project.org/ (accessed June 18, 2019).
Raybaud, V., Beaugrand, G., Goberville, E., Delebecq, G., Destombe, C., Valero, M., et al. (2013). Decline in kelp in West Europe and climate. PLoS One 8:e66044. doi: 10.1371/journal.pone.0066044
Reed, D. C., Brzezinski, M. A., Coury, D. A., Graham, W. M., and Petty, R. L. (1999). Neutral lipids in macroalgal spores and their role in swimming. Mar. Biol. 133, 737–744. doi: 10.1007/s002270050515
Roleda, M. Y., and Hurd, C. L. (2019). Seaweed nutrient physiology: application of concepts to aquaculture and bioremediation. Phycologia 58, 552–562. doi: 10.1080/00318884.2019.1622920
Rosell, K.-G., and Srivastava, L. M. (2004). Seasonal variations in total nitrogen, carbon and amino-acids in Macrocystis integrifolia and Nereocystis luetkeana (Phaeophyta). J. Phycol. 21, 304–309. doi: 10.1111/j.0022-3646.1985.00304.x
Rothäusler, E., Gómez, I., Karsten, U., Tala, F., and Thiel, M. (2011). Physiological acclimation of floating Macrocystis pyrifera to temperature and irradiance ensures long-term persistence at the sea surface at mid-latitudes. J. Exp. Mar. Biol. Ecol. 405, 33–41. doi: 10.1016/j.jembe.2011.05.018
Salinas, S., Brown, S. C., Mangel, M., and Munch, S. B. (2013). Non-genetic inheritance and changing environments. Non Genet. Inherit. 1, 38–50. doi: 10.2478/ngi-2013-0005
Saltz, J. B., Bell, A. M., Flint, J., Gomulkiewicz, R., Hughes, K. A., and Keagy, J. (2018). Why does the magnitude of genotype-by-environment interaction vary? Ecol. Evol. 8, 6342–6353. doi: 10.1002/ece3.4128
Sarker, M. Y., Bartsch, I., Olischläger, M., Gutow, L., and Wiencke, C. (2013). Combined effects of CO2, temperature, irradiance and time on the physiological performance of Chondrus crispus (Rhodophyta). Bot. Mar. 56, 63–74. doi: 10.1515/bot-2012-0143
Schaffelke, B. (1995). Storage carbohydrates and abscisic acid contents in Laminaria hyperborea are entrained by experimental daylengths. Eur. J. Phycol. 30, 313–317. doi: 10.1080/09670269500651101
Schaffelke, B., and Lüning, K. (1994). A circannual rhythm controls seasonal growth in the kelps Laminaria hyperborea and L. digitata from Helgoland (North Sea). Eur. J. Phycol. 29, 49–56. doi: 10.1080/09670269400650471
Scheschonk, L., Becker, S., Hehemann, J. H., Diehl, N., Karsten, U., and Bischof, K. (2019). Arctic kelp eco-physiology during the polar night in the face of global warming: a crucial role for laminarin. Mar. Ecol. Prog. Ser. 611, 59–74. doi: 10.3354/meps12860
Schiener, P., Black, K. D., Stanley, M. S., and Green, D. H. (2015). The seasonal variation in the chemical composition of the kelp species Laminaria digitata, Laminaria hyperborea, Saccharina latissima and Alaria esculenta. J. Appl. Phycol. 27, 363–373. doi: 10.1007/s10811-014-0327-1
Schmidt, A., Schmid, M. W., and Grossniklaus, U. (2015). Plant germline formation: common concepts and developmental flexibility in sexual and asexual reproduction. Development 142, 229–241. doi: 10.1242/dev.102103
Schneider, C. A., Rasband, W. S., and Eliceiri, K. W. (2012). NIH Image to ImageJ: 25 years of image analysis. Nat. Methods 9, 671–675. doi: 10.1038/nmeth.2089
Schreiber, E. (1930). Untersuchungen über Parthenogenesis, Geschlechtsbestimmung und Bastardisierungsvermögen bei Laminarien. Planta 12, 331–353. doi: 10.1007/bf01948810
Schrum, C., Lowe, J., Meier, H. E. M., Grabemann, I., Holt, J., Mathis, M., et al. (2016). “Projected Change – North Sea,” in North Sea Region Climate Change Assessment, eds M. Quante and F. Colijn (Cham: Springer), 175–217. doi: 10.1007/978-3-319-39745-0_6
Sjøtun, K., and Schoschina, E. V. (2002). Gametophytic development of Laminaria spp. (Laminariales, Phaeophyta) at low temperature. Phycologia 41, 147–152. doi: 10.2216/i0031-8884-41-2-147.1
Smale, D. A. (2020). Impacts of ocean warming on kelp forest ecosystems. New Phytol. 225, 1447–1454. doi: 10.1111/nph.16107
Smale, D. A., Wernberg, T., Yunnie, A. L. E., and Vance, T. (2015). The rise of Laminaria ochroleuca in the Western English Channel (UK) and comparisons with its competitor and assemblage dominant Laminaria hyperborea. Mar. Ecol. 36, 1033–1044. doi: 10.1111/maec.12199
Straub, S. C., Wernberg, T., Thomsen, M. S., Moore, P. J., Burrows, M. T., Harvey, B. P., et al. (2019). Resistance, extinction, and everything in between – The diverse responses of seaweeds to marine heatwaves. Front. Mar. Sci. 6:763. doi: 10.3389/fmars.2019.00763
Sultan, S. E. (1995). Phenotypic plasticity and plant adaptation. Acta Bot. Neerl. 44, 363–383. doi: 10.1111/j.1438-8677.1995.tb00793.x
Sultan, S. E. (2004). Promising directions in plant phenotypic plasticity. Perspect. Plant Ecol. Evol. Syst. 6, 227–233. doi: 10.1078/1433-8319-00082
Suter, L., and Widmer, A. (2013). Environmental heat and salt stress induce transgenerational phenotypic changes in Arabidopsis thaliana. PLoS One 8:e60364. doi: 10.1371/journal.pone.0060364
Tatewaki, M. (1966). Formation of a crustaceous sporophyte with unilocular sporangia in Scytosiphon lomentaria. Phycologia 6, 62–66. doi: 10.2216/i0031-8884-6-1-62.1
tom Dieck, I. (1991). Circannual growth rhythm and photoperiodic sorus induction in the kelp Laminaria setchellii (Phaeophyta). J. Phycol 27, 341–350. doi: 10.1111/j.0022-3646.1991.00341.x
tom Dieck, I. (1992). North Pacific and North Atlantic digitate Laminaria species (Phaeophyta): hybridization experiments and temperature responses. Phycologia 31, 147–163. doi: 10.2216/i0031-8884-31-2-147.1
tom Dieck, I. (1993). Temperature tolerance and survival in darkness of kelp gametophytes (Laminariales, Phaeophyta) - Ecological and biogeographical implications. Mar. Ecol. Prog. Ser. 100, 253–264. doi: 10.3354/meps100253
Uller, T., Nakagawa, S., and English, S. (2013). Weak evidence for anticipatory parental effects in plants and animals. J. Evol. Biol. 26, 2161–2170. doi: 10.1111/jeb.12212
Underwood, A. J. (1997). Experiments in Ecology: Their Logical Design and Interpretation Using Analysis of Variance. Cambridge: Cambridge University Press.
Vergés, A., McCosker, E., Mayer-Pinto, M., Coleman, M. A., Wernberg, T., Ainsworth, T., et al. (2019). Tropicalisation of temperate reefs: implications for ecosystem functions and management actions. Funct. Ecol. 33, 1000–1013. doi: 10.1111/1365-2435.13310
Wernberg, T., Bennett, S., Babcock, R. C., de Bettignies, T., Cure, K., Depczynski, M., et al. (2016). Climate-driven regime shift of a temperate marine ecosystem. Science 353, 169–172. doi: 10.1126/science.aad8745
Wernberg, T., and Filbee-Dexter, K. (2019). Missing the marine forest for the trees. Mar. Ecol. Prog. Ser. 612, 209–215. doi: 10.3354/meps12867
Wiencke, C., Bartsch, I., Bischoff, B., Peters, A. R., and Breeman, A. M. (1994). Temperature requirements and biogeography of Antarctic, Arctic and amphiequatorial seaweeds. Bot. Mar. 37, 247–259. doi: 10.1515/botm.1994.37.3.247
Wiltshire, K. H., Boersma, M., Carstens, K., Kraberg, A. C., Peters, S., and Scharfe, M. (2015). Control of phytoplankton in a shelf sea: determination of the main drivers based on the Helgoland Roads Time Series. J. Sea Res. 105, 42–52. doi: 10.1016/j.seares.2015.06.022
Wiltshire, K. H., Malzahn, A. M., Wirtz, K., Greve, W., Janisch, S., Mangelsdorf, P., et al. (2008). Resilience of North Sea phytoplankton spring bloom dynamics: an analysis of long-term data at Helgoland Roads. Limnol. Oceanogr. 53, 1294–1302. doi: 10.2307/40058252
Young, E. B., Dring, M. J., and Berges, J. A. (2007a). Distinct patterns of nitrate reductase activity in brown algae: light and ammonium sensitivity in Laminaria digitata is absent in Fucus species. J. Phycol. 43, 1200–1208. doi: 10.1111/j.1529-8817.2007.00403.x
Young, E. B., Dring, M. J., Savidge, G., Birkett, D. A., and Berges, J. A. (2007b). Seasonal variations in nitrate reductase activity and internal N pools in intertidal brown algae are correlated with ambient nitrate concentrations. Plant Cell Environ. 30, 764–774. doi: 10.1111/j.1365-3040.2007.01666.x
Keywords: phenotypic plasticity, temperature acclimation, developmental plasticity, carry-over effect, biphasic life cycle, gametogenesis, ontogeny, genetic variation
Citation: Liesner D, Shama LNS, Diehl N, Valentin K and Bartsch I (2020) Thermal Plasticity of the Kelp Laminaria digitata (Phaeophyceae) Across Life Cycle Stages Reveals the Importance of Cold Seasons for Marine Forests. Front. Mar. Sci. 7:456. doi: 10.3389/fmars.2020.00456
Received: 26 March 2020; Accepted: 22 May 2020;
Published: 17 June 2020.
Edited by:
Thomas Wernberg, The University of Western Australia, AustraliaReviewed by:
John Bolton, University of Cape Town, South AfricaSamuel Starko, University of Victoria, Canada
Copyright © 2020 Liesner, Shama, Diehl, Valentin and Bartsch. This is an open-access article distributed under the terms of the Creative Commons Attribution License (CC BY). The use, distribution or reproduction in other forums is permitted, provided the original author(s) and the copyright owner(s) are credited and that the original publication in this journal is cited, in accordance with accepted academic practice. No use, distribution or reproduction is permitted which does not comply with these terms.
*Correspondence: Daniel Liesner, ZGFuaWVsLmxpZXNuZXJAYXdpLmRl