Simulated Heatwaves Lead to Upregulated Chemical Defense of a Marine Foundation Macrophyte Against Microbial Colonizers
- 1GEOMAR Helmholtz Centre for Ocean Research Kiel, Benthic Ecology, Kiel, Germany
- 2Plymouth Marine Laboratory, Marine Ecology and Biodiversity, Plymouth, United Kingdom
Climate change is characterized not only by an increase in mean temperature, but also an increase in the variability around the means causing extreme events like marine heatwaves. These events are expected to have strong influence on the ecology of marine foundation species such as the eelgrass Zostera marina. Bacterial and macroscopic foulers are ubiquitous in the marine environment; they can have detrimental impacts on macrophytes and warming is known to enhance bacterial fouling. Thus, to investigate the consequence of heatwaves on the chemical defense of eelgrass against microbial colonizers, we incubated Z. marina plants in the Kiel Outdoor Benthocosm system under ambient control conditions and two different heatwave treatments: a treatment experiencing two spring heatwaves followed by a summer heatwave, and a treatment only experiencing just the summer heatwave. The capacity to deter microbial colonizers was found to be significantly up-regulated in Z. marina from both heatwave treatments in comparison to Z. marina under control conditions, suggesting defense regulation of Z. marina in response to marine heatwaves. We conclude climate extremes such as heatwaves can trigger a regulation in the defense capacity, which could be necessary for resilience against climate change scenarios. Such dynamics in rapid regulation of defense capacity as found in this study could also apply to other host plant – microbe interactions under scenarios of ongoing climate change or extreme climate events like heatwaves.
Introduction
Climate change is predicted to affect life on earth. Many species have already shifted their geographical range in response to global warming and a poleward redistribution of species has been documented in both terrestrial and marine environments (Sunday et al., 2012). Global and regional climate models predict not only an increase of mean temperatures but also increased duration and frequency of severe heatwave events by 17 and 34%, respectively (Oliver et al., 2018). Marine species are often less adapted to fluctuating temperatures than terrestrial organisms, as the general buffering capacity of the ocean often prevents abrupt temperature change (Hoegh-Guldberg and Bruno, 2010), thus making them more vulnerable toward abnormally high temperature.
Macrophyte based coastal ecosystems – and in particular seagrass meadows – often lack species redundancy, which can make them particularly vulnerable to warming stress (Ehlers et al., 2008). Temperature stress effects on such habitat forming species may therefore result in changes of whole coastal environments (Holling, 1973; Hughes et al., 2003). In many parts of the world seagrasses are of great importance for the near shore marine ecosystems, and Zostera marina, also known as eelgrass, is one of the most dominant seagrass species in temperate areas (Den Hartog and Kuo, 2006). The eelgrass ecosystem is very productive and supports a diverse assemblage of organisms (Hemminga and Duarte, 2000; Den Hartog and Kuo, 2006). Within this system, eelgrass together with seaweeds provides a supportive nursery habitat for several fish and shellfish species that are of economic importance (Orth et al., 2006; Plummer et al., 2013) and the basis of a complex food web that supports all other organisms. Eelgrass meadows have numerous ecological functions that are important for the stability of the ecosystem and at the same time for fisheries (Duarte, 2002). Hence, loss of eelgrass on a larger scale can lead to serious consequences and devastation of the seagrass ecosystem (Hemminga and Duarte, 2000; Waycott et al., 2009). The currently observed decline of eelgrass in most coastal environments (Waycott et al., 2009) often appears accelerated by warming. For example, a heatwave of 3 weeks during the summer of 2003 caused major losses and die-offs of eelgrass in Europe (Greve et al., 2003; Reusch et al., 2005). High temperature (30°C) was also found to have severe negative impacts on eelgrass in the lower Chesapeake Bay, affecting growth, tissue integrity, nitrogen metabolism, and protein/enzyme synthesis (Hammer et al., 2018). Also in the future such heatwaves are likely to cause further losses of eelgrass through direct physiological stress due to damage to photosystems or negative carbon balance (Winters et al., 2011). At the same time incidences of infectious diseases often increase with high temperatures, including wasting disease of Z. marina (Sullivan et al., 2018; Brakel et al., 2019), which might increase the sensitivity of eelgrass further.
The optimum water temperature for growth of Z. marina is between 10 and 20°C. Temperatures of 25°C or more can largely decrease photosynthetic rates and survival of Z. marina (Nejrup and Pedersen, 2008). While current maximum summer temperatures (around 24°C) in the southern Baltic Sea are still tolerated by eelgrass, additional temperature increase in the coming decades may threaten the fitness of eelgrass plants in this brackish inland sea (Salo and Pedersen, 2014). In the Kiel Fjord, summer water temperature has been predicted to increase between 3 and 5°C and winter temperature could increase even by 5–10°C before the end of the century (BACC 2006). Communities and ecosystems in the western Baltic Sea have already suffered from climate change and knowledge of the vulnerability of key coastal habitat forming species such as Z. marina to temperature change is important for the development of management concepts for this environment (Ehlers et al., 2008).
Being a significant source of dissolved organic carbon for heterotrophic bacteria (Asmala et al., 2019), eelgrass – like other aquatic macrophytes – is continuously subject to colonization by epibacteria (Fahimipour et al., 2017). Many of the consequences of this microbial settlement are detrimental, for example microbial settlers often attract macrofoulers (Wahl et al., 2012), and seagrass is not an exception (Hughes et al., 2009; Waycott et al., 2009). The microbiota of eelgrass roots and leaves comprise specific symbiotic components that provide important metabolic functions (Cucio et al., 2016; Ettinger et al., 2017). Instead of a specific core microbiome eelgrass leaves bear a fraction of the bacterial community that is present in the surrounding water (Bengtsson et al., 2017; Fahimipour et al., 2017). This strongly suggests that microbes settle on eelgrass leaves more or less randomly and they then undergo a filtering community assembly mechanism (Fahimipour et al., 2017). The later was shown to be driven at least in part by chemical defense compounds that are present on the host surface (Guan et al., 2017). Seagrasses defend themselves against excessive colonization; one of the strategies is the production (Fusetani, 2011; Marhaeni et al., 2011; Zidorn, 2016) and release of functional compounds that repel bacteria (Guan et al., 2017). In particular, phenolic secondary metabolites from seagrasses have repeatedly been identified as bioactive compounds that may affect bacterial settlers (Iyapparaj et al., 2014) and macrofoulers (Davis et al., 1989). Recently Rosmarinic acid was identified as a main defense compound against bacterial settlers that colonize the surface of Z. marina (Guan et al., 2017).
Although temperature has been reported to be an important factor influencing the production of phenolic compounds in terrestrial plants (Løvdal et al., 2010), equivalent research with marine plants has rarely been conducted, except by Vergeer et al. (1995), who suggested that a negative relationship between temperature and the content of phenolic defense compounds (that are active against the wasting disease pathogen Labyrinthula zosterae) may exist in Z. marina. Further, the concentrations of phenolics in eelgrass can be subject to seasonal variation. Ravn et al. (1994) detected the highest tissue concentrations of Rosmarinic acid in eelgrass during the warm season. The same was also observed with surface concentrations of this compound, which were repeatedly observed to be high in summer (Guan et al., 2017, 2019) and low during the cold season (Guan et al., 2019; Papazian et al., 2019).
The environmental stress hypothesis (ESH) predicts unfavorable environmental conditions could generally limit resources or the physiological capacity for chemical defenses in host macrophytes (Menge et al., 2002; Weinberger et al., 2011). For example, in the red seaweed Laurencia dendroidea severe conditions of temperature and salinity promoted a decrease in concentrations of the surface associated anti-fouling compound elatol, as predicted by the ESH (Sudatti et al., 2011). In other cases, chemical defense of marine macrophytes has been found to contradict the ESH. For example, surface associated defense is unaffected in the brown seaweed Fucus vesiculosus at a relatively high temperature of 25°C (Saha et al., 2014).
The first goal of the present study was to investigate whether Z. marina, under heatwave treatments, undergoes upregulation or downregulation of its chemical defense against microsettlers when compared to Z. marina exposed to control conditions. A second goal was to investigate whether repeated heatwaves would result in a weaker or stronger defense than one single heatwave.
To test this eelgrass was incubated in outdoor benthocosms under the same conditions as in natural assemblages, except for heatwave treatments. Control treatments (tanks under control conditions without heatwaves; 0HW) were compared to treatments with one simulated summer heatwave (1HW) and treatments which experienced two simulated spring heatwaves along with the simulated summer heatwave (3HWs). Response variables studied were (a) anti-settlement activity of surface extract of Zostera against bacterial colonizers, (b) abundance of bacterial colonizers on eelgrass and (c) concentrations of the defense compound Rosmarinic acid on the surface of eelgrass leaves.
Materials and Methods
Z. marina and Sediment Collection
Z. marina was collected by scuba diving at a water depth of 3–4 m from Falkenstein, Kiel, Germany (54°38’ N/10°17’ E) in May 2015. Sediment was collected from the same site and on the same day, using a Van Veen grab sampler that collected ∼15–20 cm of the top sediment. The sampling site is located in a distance of 7 km from the experimental site in the Kiel Fjord (southwest Baltic Sea), a brackish water environment, where the average surface water salinity is around 16, with a possible variation between 10 and 24. Z. marina was transported in cooler boxes to the lab and maintained for one night in 300 L tanks containing Baltic Sea water (waterflow at 1800 L/d) in a climate room at 15°C, under a light intensity of 20–30 μmol photons m–2 s–1, before they were stocked into the outdoor mesocosms as described below.
Experimental Setup
Benthocosm System and Temperature Treatment
The experiment was conducted in the Kiel outdoor benthocosms facility (KOB). The KOB is a mesocosm system located on aluminum floats in the inner Kiel Fjord, at ~7.5 km from the collection site. The KOB allows for the control and manipulation of various environmental parameters to obtain target treatments (Supplementary Figure S1; for details about and images of the KOB see Wahl et al., 2015). Six benthocosm tanks – each with a volume of 3,000 L (inner size: 2 m × 2 m × 0.9 m) – were used in this experiment. Each tank was evenly divided into two independent subunits by implementing a PVC wall. The water temperature in each subunit was adjusted with an accuracy of close to 100% (see below) by aquarium heaters and coolers. These were controlled by designed software (GHL Profilux Control or GHL Control Center) that allows for the realization of complex temperature treatments. In total 12 controllers were applied to permanently control and record the temperature in each of the subunits and an additional sensor was used to measure the temperature in the Kiel Fjord. Seawater was constantly pumped from the Fjord (water depth: 1–1.2 meter) into the tanks at an exchange rate of 1,800 L per day, while wave generators prevented stratification within the tanks and simulated near-natural conditions. As a consequence, salinity in the benthocosms experienced the same fluctuations as salinity in Kiel Fjord surface water (Wahl et al., 2015). During the experiment described here, salinity varied between 13.9 and 16.4 with a mean of 15.4 (Pansch et al., 2018).
To set up the temperature regimes in the tanks we analyzed temperature fluctuations in the Kiel Fjord from May to September in 15 preceding years (2000–2014) and in time intervals of 5 min (Pansch et al., 2018). The temperature regime of 2009 was identified as the least divergent from the mean, i.e., as the regime exhibiting the least extremes. This temperature fluctuation regime was therefore realized in two tanks (four subunits) as the control treatment. The remaining four tanks received the same temperature treatment, but with an intermittent simulation of heatwaves. Three heatwaves (3HWs) were applied in two tanks (four subunits) in May, July and August, each lasting 7 day, while only the last of these heatwaves, i.e., 1HW, was applied in two other tanks (four subunits) in August only, when the seasonal temperature increase had reached its annual maximum.
To identify realistic heatwave treatments, we analyzed temperature fluctuations in the Kiel Fjord between 2000 and 2014. We considered heat waves as events during which the daily temperature increases over two or more consecutive days exceed a given threshold; and we defined this threshold as the highest daily increase that allowed finding, at least, one heat wave in each year of the time series. In the period 2000–2014, this threshold corresponded to 0.7°C/day. This threshold was then used to identify heat waves and to further analyze them. Increases up to 1.2°C d–1 and a maximum temperature anomaly of +5.2°C above average occurred twice in these years (for further details see Pansch et al., 2018). Hence, the 1st (May) and 2nd (July) heatwave of the 3HW treatment followed a temperature increase of 1.2°C d–1 over 3 days, remained at the elevated temperature (+3.6°C) for 4 days and dropped to control conditions over a period of 2 days. The heatwave in August in both treatments (3HWs and 1HW) increased by 1.7°C day–1 over 3 days, remained at the elevated temperature (+5.2°C) for 4 days and dropped to control conditions within 2 days. These temperature increases applied during the heatwave treatments represent the maximal increases observed within two consecutive days during the years 2000–2014. Maximum temperatures obtained were 20.4°C for the control treatments and 25.2°C for 1HW and 3HW treatments (see Figure 1 for the treatment applied). This maximum temperature of 25.2°C exceeds maximum temperatures that occurred 2000–2014 in shallow, open waters of the Kiel Fjord by about 1°C (Pansch et al., 2018). The achieved temperature treatments were largely identical with programmed daily and seasonal fluctuations. Deviations from programmed values were only recorded during some exceptionally warm days, mostly during late afternoon.
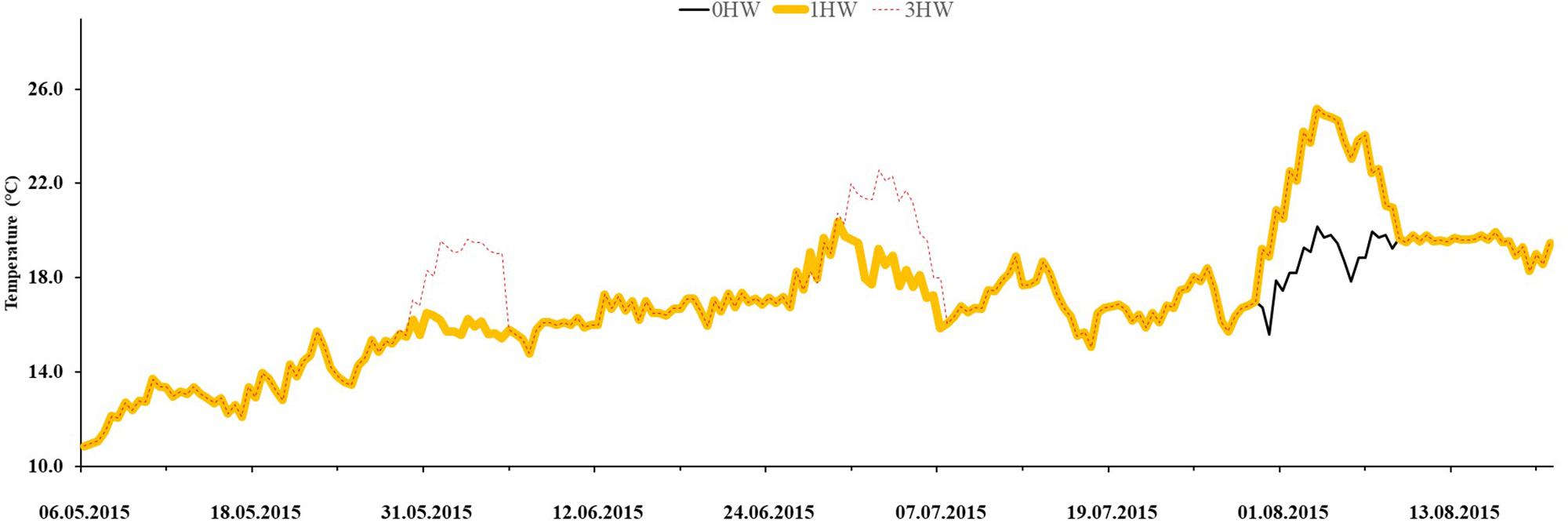
Figure 1. Implemented temperature regime within the experimental units over the experimental period (May to August 2015). Control (0HW), one summer heatwave (1HW) and two late spring/early summer heatwaves followed by a summer heatwave (3HW). Figure has been adapted from Saha et al. (2019). The dates on X-axis are given as dd-mm-yyyy.
Set-Up With Eelgrass
Eelgrass was planted into individual 5 L aquaria made from polystyrene (26 cm × 16 cm × 15 cm) that contained sediment to a depth of 10 ± 1 cm. Six individual plants were planted in each aquarium to obtain an initial density of 144 shoots m–2, corresponding with eelgrass meadows of low density (Holmer and Nielsen, 1997). Eleven aquaria – together containing 66 plants – were placed at the bottom (70 cm water depth) of each benthocosm subunit. In addition, various benthic invertebrates and macroalgae that are common in the Kiel Fjord (e.g., Idotea baltica, Littorina littorea, Gammarus spp., Mytilus edulis, Balanus improvisus, Fucus vesiculosus, Agarophyton vermiculophyllum) were inoculated into all benthocosm subunits at identical densities and natural abundances (Werner et al., 2016), in order to create a diverse benthic ecosystem. The performance of benthic and free living animals and macrophytes has been presented in Pansch et al. (2018) and Saha et al. (2019). Briefly, the 3HW treatments resulted in a reduction of longitudinal growth by 40% and increased formation of side branches in Z. marina, whereas photosynthesis and respiration rates were not significantly affected by both treatments. Symptoms of wasting disease throughout the experiment were generally low and not affected by the treatments and also the defense capacity of Z. marina against the wasting disease pathogen Labyrinthula zosterae was not measurably compromised (Saha et al., 2019).
Sampling for Z. marina Surface Extract Generation, Rosmarinic Acid, and Epibacteria Quantification
Samples for Z. marina surface extract generation, Rosmarinic acid quantification and quantification of epibacteria on the surface of Z. marina were taken immediately prior to the start of the experiment in May, after application of two spring heatwaves in July and after the end of the experiment, i.e., after the summer heatwave in August. At each of these occasions, two plants were sampled from each subunit and processed as described below. The replication level was four in each case, with each subunit acting as a true replicate.
Surface Extract Generation
The “dipping method” (de Nys et al., 1998) was modified to obtain compounds from the surface of sampled eelgrass leaves as described in Guan et al. (2017). Briefly, spin-dried leaves (entire leaves) were dipped into 2-propanol for 5 s, which allowed to extract surface compounds without disruption of epidermal cells (see Guan et al., 2017 for details). This method is benign and does not extract intracellular compounds. The concentration of compounds was related to the extracted surface volume, which was determined by a product of the leaf surface area and the mean thickness of 30 μm of the leaf surface boundary layer of marine plants (Wahl et al., 2010). The leaf surface was calculated from the leaf wet weight, using the conversion factor of 78.9903 cm2 g–1 (Guan et al., 2017). The obtained surface extracts were filtered through glass fiber-filters with a mesh size of 0.2 μm (Carl Roth, Karlsruhe, Germany), evaporated in vacuo and stored at −20°C. For all subsequent steps the extracts were redissolved in methanol to yield stock solutions at the same 3.33-fold natural concentration (for example, extract originating from a surface volume of 1,000 μl dissolved in 300 μl of methanol).
Defense Capacity Test
Inhibition or reduction in bacterial settlement and attachment represents the first line of defense against microbial challenge (Lane et al., 2009). Thus, an anti−settlement assay was employed as the most relevant criterion for determining anti-microfouling defense, as it quantifies both repellent and toxic effects (Wahl et al., 1994). Anti-settlement assays were done to quantify the defense capacity of Z. marina surface extracts against fouling bacterial strains isolated from various substrates as described previously (Saha et al., 2011, 2012, Guan et al., 2017). Bioassays were conducted with five bacterial isolates originating from non-living substrates on North Sea and Baltic Sea coasts (Saha et al., 2016) that had been cryopreserved at −80°C. The isolates were Alteromonas stellipolaris, Pseudoalteromonas carrageenivora, Marivita litorea, Polaribacter dokdonensis, and Vibrio cyclitrophicus. All bacteria were grown in sterile liquid nutrient medium [5 g peptone (Carl Roth, Karlsruhe, Germany), 3 g yeast extract (Carl Roth, Karlsruhe, Germany), 16 g artificial sea salt and 1 L deionized water] at 25°C for 1 or 2 days before they were tested individually in the assays.
To test 1-fold natural concentrations of extracted compounds, 30 μl of each extract dissolved in methanol at 3.33-fold-natural concentration were added into eight wells (to account for the variability in the bacterial settlement rates) on 96 well microtiter plates and diluted with 70 μl of pure methanol. Control wells received 100 μl of pure methanol instead. The solvent was then evaporated in a freeze-dryer and 100 μl of bacterial suspension (with O.D. between 0.5 and 0.8) were added into four of each eight wells, while sterile seawater was added into the remaining four wells. After 2 h of settlement at 25°C in darkness, the wells were emptied and immediately rinsed with 100 μl of sterile seawater. Syto 9 (Invitrogen, Waltham, MA, United States) dissolved in sterile sea water was then applied at a final concentration of 5 μM to stain the DNA of attached bacterial cells (as described in Saha et al., 2011, 2012; Guan et al., 2017). After the staining procedure excess dye was rinsed away with sterile sea water and the fluorescence intensity was measured with a Hidex Chameleon IV plate reader (excitation: 477–491 nm, emission: 540 nm). Extract replication was four times for each of the treatment tanks and control tanks.
Quantification of Rosmarinic Acid
Rosmarinic acid was quantified by HPLC on a semi-preparative LichroCart 250/10 Purospher STAR-rp 18 ec column (VWR, Radnor, United States) using a Varian 940-LC with diode array detection (Varian 940-LC, Agilent Technologies, Palo Alto, CA, United States). The solvent system ranged from 0.1% formic acid dissolved in Acetonitrile to 0.1% formic acid dissolved in water, as described previously (Guan et al., 2017). The detection of Rosmarinic acid was based on its light absorption at 350 nm and concentrations were derived from peak areas after calibration of the system with purchased Rosmarinic acid.
Epibacterial Abundance on Z. marina
Biofilms from 1 cm2 of Z. marina surface (1 cm from the tips) were collected by swabbing with a sterile cotton tip, followed by vortexing the cotton tip for 30 s in a sterile Eppendorf vial containing 1 ml of sterile-filtered seawater (SSW, 16 ‰). The relative abundance of diatoms (and any other possible photoautotrophs) in 100 μL of subsample was determined by measuring the fluorescence of chlorophyll a at 477–491 nm (excitation) and 677 nm (emission), using a plate reader (Hidex Chameleon IV, Turku, Finland) and 96-well microtiter well plates (Greiner). Subsequently, the relative density of all microfoulers (including bacteria and diatoms) was determined by staining all the particles in the same 100 μL subsample with the fluorescent DNA–binding dye Syto 9 at 5 μM (Invitrogen GmbH). Following an incubation time of 10 min in darkness, fluorescence was subsequently measured (excitation 477–491 nm, emission 540 nm), using the same plate reader. Replication was four for each treatment. The first measurements provided data on treatment effects on the relative microalgal density at the algal surface, while the second measurement provided similar information on all epibiotic cells. Thereafter, the bacterial abundance was determined in relative units (RU) by subtracting the first measurement from the second one.
Statistical Analysis
A log effect ratio was used to express the relative activity strength of extracts (i.e., defense capacity) from each plant in RU. It is calculated as the logarithm of the ratio of fluorescence intensity of target microbes settled in presence of extract and fluorescence intensity of target microbes settled on solvent controls. Thus, negative values indicate an inhibitory effect of an extract against a target bacterial strain, while “0” would indicate the absence of such an effect. Mean log effect ratios of the activity strength against all tested bacterial strains are presented here.
Subreplicates – data obtained within the same tank at the same time point – were averaged and tanks were treated as replicated experimental units. Data obtained within the same unit at different time points were treated as repeated measures. Two-way-repeated-measures-ANOVA – with the factor sampling month as within-measures factor and the factor heatwave treatment as between-measures factor – was used to investigate the interactive and single effects of both factors on the activity strength against bacteria, the production of Rosmarinic acid in eelgrass, and the log-transformed densities of bacteria colonizing eelgrass plants. Shapiro-Wilk’s test was used to test whether data within groups were normally distributed (p < 0.05) and Levene’s test was used to test for homogeneity of variances (p < 0.05). Tukey’s HSD test was used to compare the means of single groups. The relationships between the abundance of bacteria settled on Z. marina, activity strength of surface extracts and concentrations of the defense compound Rosmarinic acid were analyzed with simple linear regression (p < 0.05). All statistical analyses were conducted with the Statistica 8.0 software package (StatSoft, Tulsa, OK, United States).
Results
Variation of Bacterial Density on Z. marina Surface
Bacteria that settled on eelgrass differed significantly between sampling times (Figure 2 and Table 1). They peaked in July (0HW: 4.71 RU; 1HW: 4.93 RU, 3HWs 4.77 RU) and had lower and similar densities in May and August, but were unaffected by the heatwave treatments (p = 0.2714, Figure 2 and Table 1).
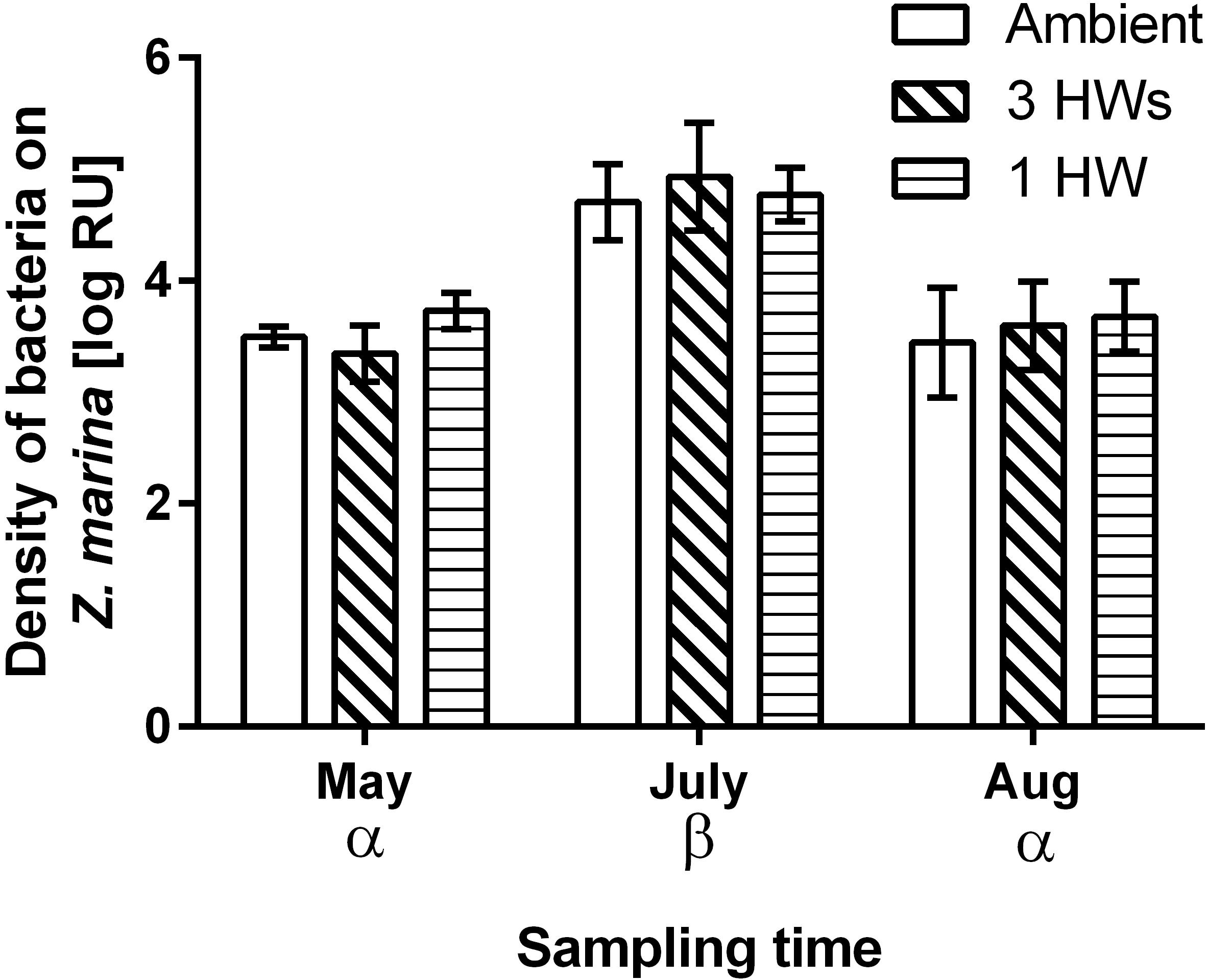
Figure 2. Epibacterial abundance on surface of Z. marina, as observed in three different months in mesocosms without heatwaves (unshaded bars), with 3HWs (diagonally shaded bars) and with 1HW (horizontally shaded bars). Different Greek letters indicate sampling times that differed significantly in a Tukey post hoc-test (p < 0.05).
Defense Capacity and Concentration of Rosmarinic Acid
All five tested bacterial isolates were deterred by Z. marina surface extracts (Supplementary Figure S2). However, sensitivities of bacterial isolates differed, and the sensitivities of single isolates varied in most cases with sampling time and – to a lesser degree – with heatwave treatment (Supplementary Figure S2 and Supplementary Table S1). Four of the five isolates exhibited significantly lesser sensitivity toward extracts sampled at the end of the experiment than toward extracts sampled earlier (Supplementary Figure S2 and Supplementary Table S1). Correspondingly the mean sensitivity of all five isolates was significantly lower at the end of the experiment, while the highest mean sensitivity was detected in samples from July (Figure 3 and Table 2). At the end of the experiment both heatwave treatments had a significant influence (p = 0.0470, Figure 3 and Table 2) on the defense capacity of Z. marina (mean activity strength against all five tested strains) and samples from eelgrass experiencing those treatments were more deterrent toward bacterial settlement than samples from control tanks.
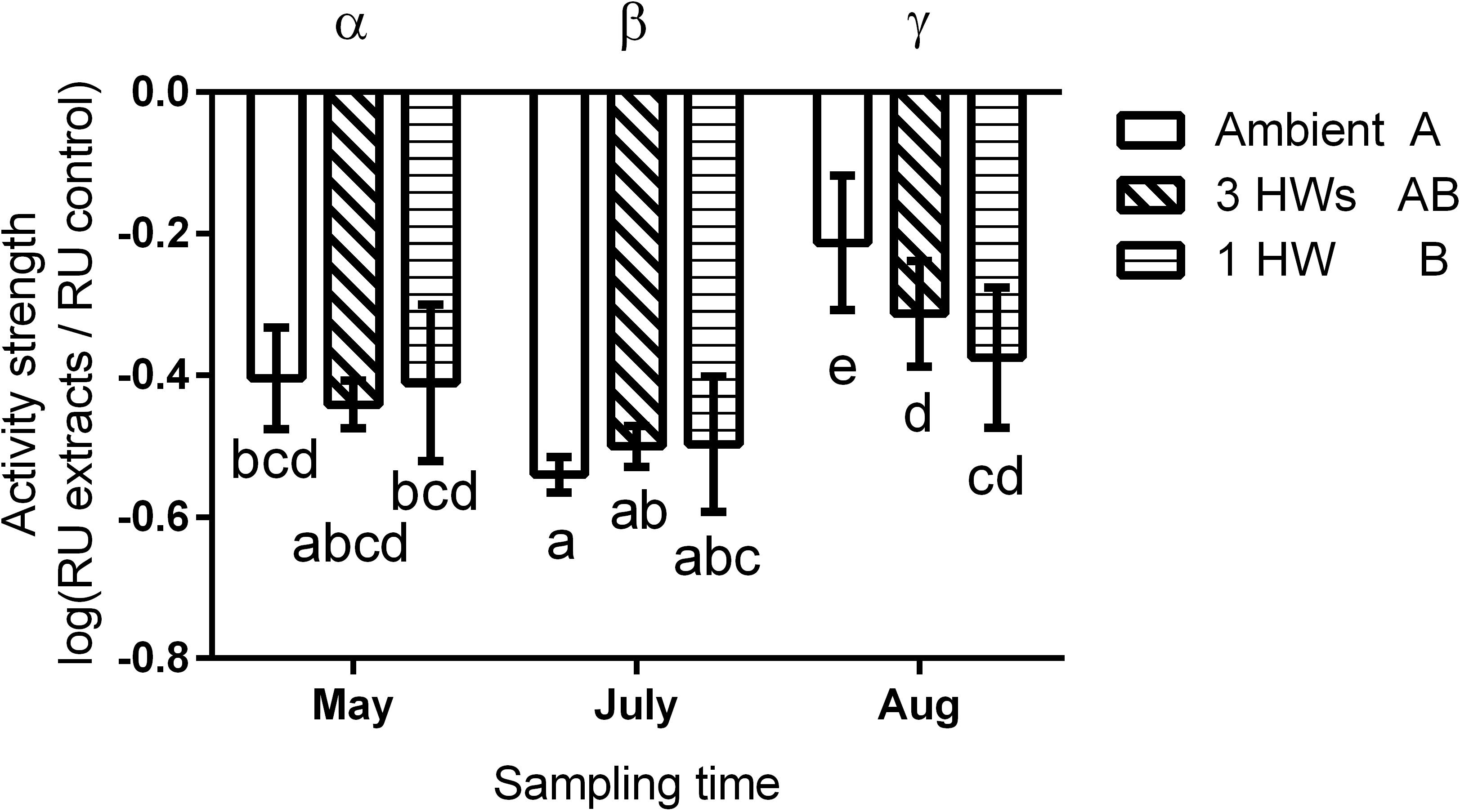
Figure 3. Mean anti-settlement activity of propan-2-ol surface extract from Z. marina at 1-fold natural concentration, at three sampling time points and in response to without heatwaves (unshaded bars), with 3HWs (diagonally shaded bars) and with 1HW (horizontally shaded bars) (mean ± 95% CI, n = 5 bacterial isolates). Anti-settlement activity was determined as the fluorescence of cells settled on extracts relative to the fluorescence of cells settled on solvent controls, therefore increasingly negative values indicate increasing activity strength. Sampling times and treatments at individual sampling times that are significantly different (Tukey post hoc test, p < 0.05) are marked by Greek letters and small Latin letters, respectively. Treatments that are overall significantly different are marked by capital Latin letters.
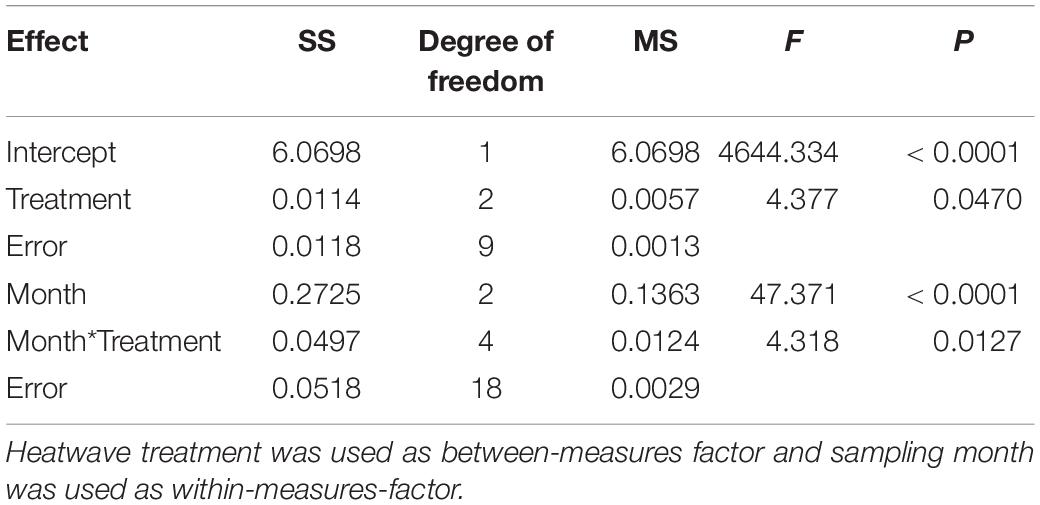
Table 2. Two-way-repeated measures ANOVA of mean antifouling defense strength of Z. marina against five bacterial strains at 1-fold natural concentration.
The concentration of Rosmarinic acid on Z. marina surfaces from different heatwave treatments exhibited no significant differences (p = 0.358, Figure 4 and Table 3). However, the mean Rosmarinic acid concentration (pooled over the three treatments for each month) differed among sampling times and it decreased significantly from May to August.
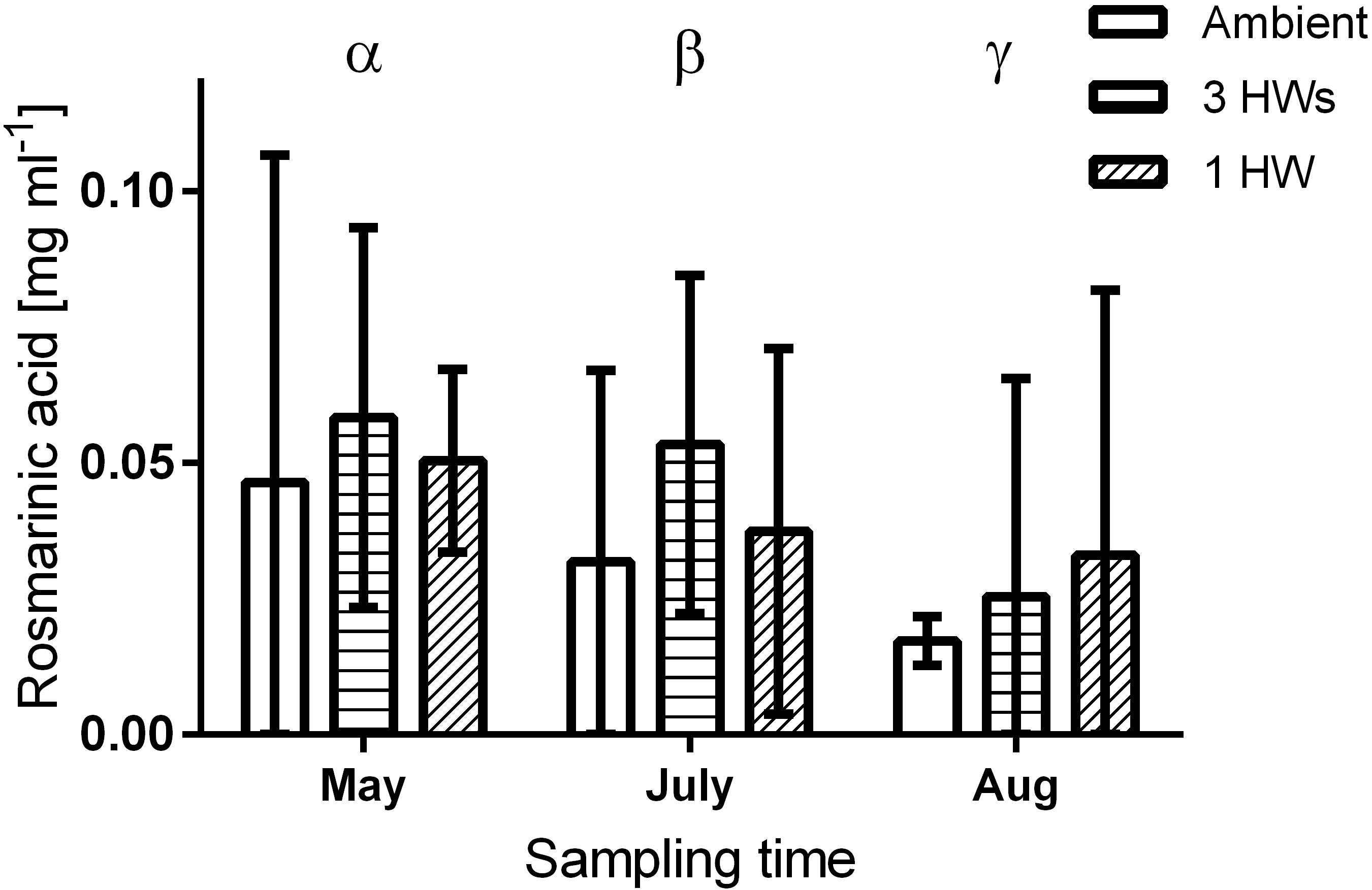
Figure 4. Concentration of Rosmarinic acid in the surface volume of eelgrass plants, at three sampling time points and in response to without heatwaves (unshaded bars), with 3HWs (horizontally shaded bars) and with 1HW (diagonally shaded bars) (mean ± 95% CI). Greek letters indicate months with significantly different concentrations (Tukey post hoc test, p < 0.05).
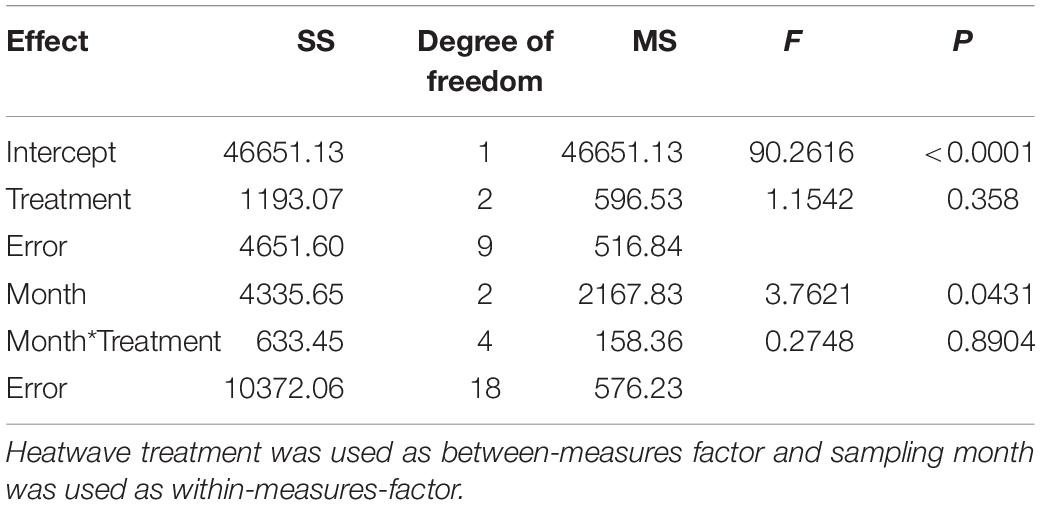
Table 3. Two-way-repeated measures ANOVA of Rosmarinic acid concentration on the surface of Z. marina.
Correlations Among Factors
Both antifouling activity and Rosmarinic acid concentration varied between months, but were not significantly correlated for May or July (Figures 5A,B). However, a positive correlation of the two variables existed in August (p < 0.0176, Figure 5C). Bacterial abundance on eelgrass correlated with antifouling defense activity within the August samples (p < 0.0537, Figure 5F), but not for May or July samples (Figures 5D,E). Rosmarinic acid concentrations also correlated with the number of settled bacteria on eelgrass in August (p < 0.014, Figure 5I), but not in May or July (Figures 5G,H).
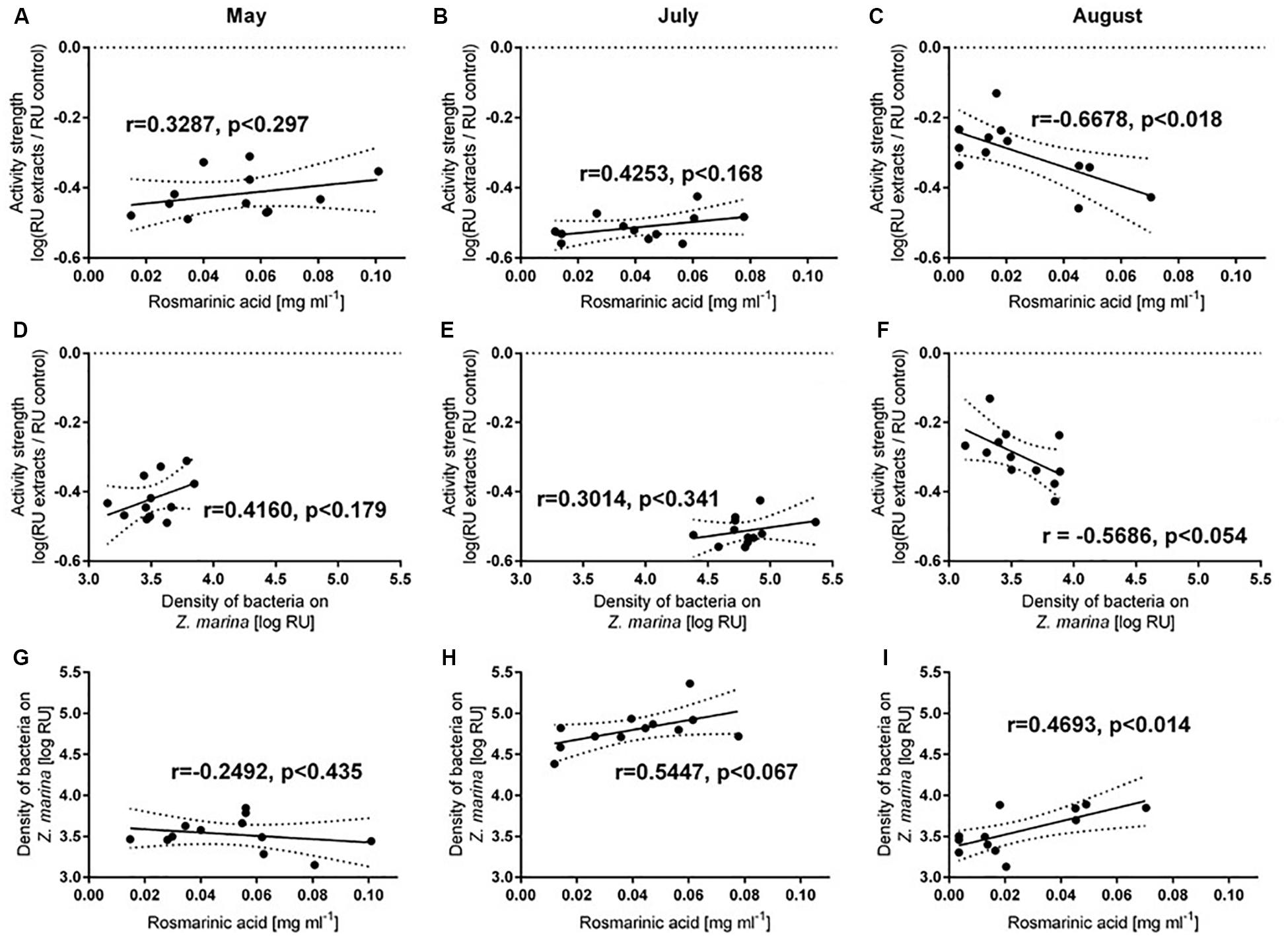
Figure 5. Pearson correlations (± 95% CI) of Z.marina defense capacity and surface concentration of Rosmarinic acid on eelgrass (A–C), of Z.marina defense capacity and density of bacteria settled on Z.marina (D–F) and of density of bacteria settled on Z.marina and surface concentration of Rosmarinic acid on Z.marina (H,I). Samples were collected in May (A,D,G), July (B,E,H), and August (C,D,I).
Discussion
The general design of our experiment allows for a comparison of heatwave effects and the effects of seasonal warming. During incubation in the benthocosms under control conditions with no heatwave, the Z. marina plants experienced a relatively rapid temperature increase from 9.5°C in May to 18.3°C in July, followed by a moderate increase to 19.4°C in August. The applied heatwaves, in contrast, were characterized by a much more rapid increase of 5.2°C within only a few days and they were therefore generally expected to cause more pronounced effects than seasonal warming. However, the seasonal effects were overall more pronounced and marked by a peak in bacterial settlers on Z. marina in July. A similar pattern with higher numbers of bacterial settlers on eelgrass in summer than spring and autumn was also observed in a field study conducted in the Kiel Fjord, where it reflected increased pressure by microbial settlers (Guan et al., 2019). In general bacteria tend to colonize aquatic plants in higher numbers at high temperature, either because the bacteria are more active under such condition (Case et al., 2011) or because the hosts become more susceptible when they are weakened by heat stress (Egan et al., 2013). The July peak in our experiment corresponded with a peak in defense activity strength against microbial settlers. This – together with the limited effects of different temperature treatments on the overall performance of Z. marina in our experiment (Saha et al., 2019) and the circumstance that settler densities peaked before autumn – strongly suggests that seasonal fluctuations in settlement pressure rather than heat stress effects on eelgrass were the primary reason for fluctuating settler densities. The peak in defense activity strength in July also suggests that Z. marina has a general capacity to regulate its defense activity against settlers according to demand, similar to certain seaweeds (Saha and Wahl, 2013; Wang et al., 2018). The chemical defense of plants is in most cases dynamic and selective (Lankau, 2007) and only microbes that are resistant to the fluctuating chemical defense of Z. marina can be expected to colonize the plant.
Despite their more rapid impact heatwaves affected the defense capacity of eelgrass less than seasonal warming. There was no significant difference in defense capacity of Z. marina against bacteria among the HW treatments and the controls in May and July, but in August this capacity was relatively increased in samples that experienced heatwave treatments, i.e., 1HW and 3HW. Under control conditions the defense capacity of Z. marina significantly decreased from July to August by 65.6%, but this reduction was less pronounced when heatwaves were applied. Application of three heatwaves resulted in reduction of the defense activity strength by 37%, while the 1HW treatment prevented/had a significant decrease of the defense capacity from July to August. Thus, although not significantly different from the 3HW treated samples at the last sampling in August, plants that experienced their first heatwave directly prior to this last sampling exhibited a tendency toward stronger maintenance of defense capacity. This indicates that a single HW treatment causes certainly not a weakened, but rather an increased defense capacity against microfoulers as compared to repeated heatwaves. Thus, preconditioning by repeated heatwaves may not result in a stronger, but in tendency in a weaker defense of Z.marina against bacterial colonizers than one single heatwave at the warmest time of the year. This could also explain the fact that no difference in the defense capacities of differently treated Z. marina plants was observed in July, when plants from the 3HW treatment had just been exposed to the second (and thus to a repeated) HW, while the other two groups had received no HW yet.
The differences in defense activity strength in August were not reflected in differences in the densities of microbial settlers on Z. marina, which can be due to various reasons. One possible explanation can be direct heatwave effects on the microbial settler community. For example, an increase in bacterial settlement pressure may have compensated the decrease in settlers resulting from increased defense capacity (or vice versa). Alternatively, our sampling in August possibly happened too early or too late after the upregulation. In the first case (i.e., sampling directly after the upregulation) its effects on the microbiota had possibly not yet happened. In the second case (i.e., sampling too late) settler communities resistant to the new defense activity may already have been selected after the effects occured.
Promoting effects of temperature increase on the antifouling defenses of marine macrophytes have been described before. For instance, surface concentrations of the deterrents Fucoxanthin and DMSP on the brown seaweed Fucus vesculosus were higher at an increased temperature of 25°C (Saha et al., 2014). Thus, increased defense activity for heatwave treated samples when compared to control samples could be a result of increased production of other (unidentified, Guan et al., 2017) defense chemicals for Z. marina at higher temperature.
Concentrations of the identified deterrent compound Rosmarinic acid changed steadily with the seasonal temperature increase, however, there was no significant change in Rosmarinic acid concentrations among different treatments. Rosmarinic acid concentration only differed among different months and showed a decreasing trend with the seasonal increase in water temperature from May through July to August. This strongly suggests that additional (unidentifed) deterrent compounds that underwent other seasonal concentration dynamics must have been active and it confirms our previous conclusion that Rosmarinic acid cannot be the only compound that deters microsettlers from the surface of Z. marina (Guan et al., 2017; Papazian et al., 2019). Likewise, the differences in deterrence observed after different heatwave treatments cannot be fully explained with changes in Rosmarinic acid concentrations. In August, antifouling defense was strongest after the application of 1HW and 3HWs and the weakest under control conditions. Mean Rosmarinic acid concentrations also decreased in this order, although there was no significant difference among different treatments and controls. However, heatwave treatments in August had no significant effect on Rosmarinic acid concentrations and correlations between Rosmarinic acid concentration and antifouling activity were not detected in May and July. A positive correlation was detected in August between the abundance of bacteria settled on Z. marina plants and the Rosmarinic acid surface concentration, suggesting that the increase in antifouling defense in the presence of high numbers of settlers in August could be possibly due to an increase in Rosmarinic acid. It remains unclear whether absence of a significant effect in July after the application of two heatwaves was due (i) to the generally increased defense capacity of Z. marina in July, or (ii) due to the fact that the heatwave applied in July was a second heatwave (perhaps resulting in a less pronounced effect) or (iii) to the fact that other compounds than Rosmarinic acid (that were, perhaps, simply not affected by the heatwave treatment) apparently dominated the deterrent surface chemistry of Z. marina.
Conclusion
In conclusion, the application of a single heatwave in August during the warmest period caused a significant increase of anti-settlement activity when compared to control treatments, while this increase was less pronounced and non-significant in plants that had experienced preceding heatwaves. Since both, the 1HW and 3HWs treated plants had significantly increased defense capacity at the end of the experiment we conclude that a regulation of chemical defense capacity to heat stimulation must have taken place. Thus, even after transient exposure to water temperatures of 25°C, Z. marina from the Baltic Sea seems capable of a dynamic management of its surface defense chemistry; even though increased mortality and reduced growth were previously observed with plants from the same region that were maintained for 6 weeks at this temperature (Nejrup and Pedersen, 2008). In this light a collapse of Z. marina resistance to epibionts will hardly be weakened by such extreme heat events, provided the heatbursts donot occur over prolonged time periods.
Data Availability Statement
The content of this publication is part of the doctoral thesis of CG (Guan, 2017). The datasets generated for this study are available at PANGAEA, 10.1594/PANGAEA.918682.
Author Contributions
CG and MS performed the experiments. CG and FW analyzed the data. CG, MS, and FW conceived and designed the experiments and wrote the manuscript. All authors contributed to the article and approved the submitted version.
Funding
This research was funded by the Chinese Scholarship Council (CSC), grant number 201206330077 provided to CG and by the DFG excellence cluster “Future Ocean”, grant number CP1215 awarded to MS as the PI.
Conflict of Interest
The authors declare that the research was conducted in the absence of any commercial or financial relationships that could be construed as a potential conflict of interest.
Acknowledgments
The authors thank Thomas Meser from Plymouth Marine Laboratory for proofreading the manuscript.
Supplementary Material
The Supplementary Material for this article can be found online at: https://www.frontiersin.org/articles/10.3389/fmars.2020.00463/full#supplementary-material
References
Asmala, E., Gustafsson, C., Krause-Jensen, D., Norkko, A., Reader, H., Staehr, P. A., et al. (2019). Role of eelgrass in the coastal filter of contrasting Baltic Sea environments. Estuar. Coast. 42, 1882–1892. doi: 10.1007/s12237-019-00615-0
Bengtsson, M. M., Buhler, A., Brauer, A., Dahlke, S., Schubert, H., and Blindow, I. (2017). Eelgrass leaf surface microbiomes are locally variable and highly correlated with epibiotic eukaryotes. Front. Microbiol. 8:1312. doi: 10.3389/fmicb.2017.01312
Brakel, J., Jakobsson-thor, S., Bockelmann, A. C., and Reusch, T. (2019). Modulation of the eelgrass – labyrinthula zosterae interaction under predicted ocean warming. Salinity change and light limitation. Front. Mar. Sci. 6:268. doi: 10.3389/fmars.2019.00268
Case, R. J., Longford, S. R., Campbell, A. H., Low, A., Tujula, N., Steinberg, P. D., et al. (2011). Temperature induced bacterial virulence and bleaching disease in a chemically defended marine macroalga. Environ. Microbiol. 13, 529–537. doi: 10.1111/j.1462-2920.2010.02356.x
Cucio, C., Engelen, A. H., Costa, R., and Muyzer, G. (2016). Rhizosphere microbiomes of european seagrasses are selected by the plant, but are not species specific. Front. Microbiol. 7:440. doi: 10.3389/fmicb.2016.00440
Davis, A. R., Targett, N. M., McConnell, O. J., and Young, C. M. (1989). “Epibiosis of marine algae and benthic invertebrates: natural products chemistry and other mechanisms inhibiting settlement and overgrowth,” in Bioorganic Marine Chemistry, Vol. 3, ed. P. J. Scheuer (Berlin: Springer), 85–114. doi: 10.1007/978-3-642-74560-7_4
de Nys, R., Dworjanyn, S. A., and Steinberg, P. D. (1998). A new method for determining surface concentrations of marine natural products on seaweeds. Mar. Ecol. Prog. Ser. 162, 79–87. doi: 10.3354/meps162079
Den Hartog, C., and Kuo, J. (2006). “Taxonomy and biogeography of Sea grasses,” in Sea Grasses: Biology, Ecology and Conservation, eds A. Larkum, R. J. Orth, and C. Duarte (Berlin: Springer), 1–23.
Duarte, C. M. (2002). The future of seagrass meadows. Environ. Conserv. 29, 192–206. doi: 10.1017/s0376892902000127
Egan, S., Harder, T., Burke, C., Steinberg, P., Kjelleberg, S., and Thomas, T. (2013). The seaweed holobiont: understanding seaweed-bacteria interactions. FEMS Microbiol. Rev. 37, 462–476. doi: 10.1111/1574-6976.12011
Ehlers, A., Worm, B., and Reusch, T. B. H. (2008). Importance of genetic diversity in eelgrass Zostera marina for its resilience to global warming. Mar. Ecol. Prog. Ser. 355, 1–7. doi: 10.3354/meps07369
Ettinger, C. L., Voerman, S. E., Lang, J. M., Stachowicz, J. J., and Eisen, J. A. (2017). Microbial communities in sediment from Zostera marina patches, but not the Z. marina leaf or root microbiomes, vary in relation to distance from patch edge. PeerJ 5:e3246. doi: 10.7717/peerj.3246
Fahimipour, A. K., Kardish, M. R., Lang, J. M., Green, J. L., Eisen, J. A., and Stachowicz, J. J. (2017). Global-scale structure of the eelgrass microbiome. Appl. Environ. Microbiol. 83:e03391-16. doi: 10.1128/AEM.03391-16
Fusetani, N. (2011). Antifouling marine natural products. Nat. Prod. Rep. 28, 400–410. doi: 10.1039/c0np00034e
Greve, T. M., Borum, J., and Pedersen, O. (2003). Meristematic oxygen variability in eelgrass (Zostera marina). Limnol. Oceanogr. 48, 210–216. doi: 10.4319/lo.2003.48.1.0210
Guan, C. (2017). Zostera Marina and Epibionts: The Dynamics of Chemical Defenses. Doctoral Dissertation, Christian-Albrechts-Universität Kiel, Germany, 159.
Guan, C., Parrot, D., Wiese, J., Sönnichsen, F. D., Saha, M., Tasdemir, D., et al. (2017). Identification of rosmarinic acid and sulfated flavonoids as inhibitors of microfouling on the surface of eelgrass Zostera marina. Biofouling 33, 867–880. doi: 10.1080/08927014.2017.1383399
Guan, C., Saha, M., and Weinberger, F. (2019). Chemical defence of a seagrass against microfoulers and its seasonal dynamics. Appl. Sci. 9:1258. doi: 10.3390/app9061258
Hammer, K. J., Borum, J., Hasler-Sheetal, H., Shields, E. C., Sand-Jensen, K., and Moore, K. A. (2018). High temperatures cause reduced growth, plant death and metabolic changes in eelgrass Zostera marina. Mar. Ecol. Prog. Ser. 604, 121–132. doi: 10.3354/meps12740
Hoegh-Guldberg, O., and Bruno, J. F. (2010). The impact of climate change on the world’s marine ecosystems. Science 328, 1523–1528. doi: 10.1126/science.1189930
Holling, C. S. (1973). Resilience and stability of ecological systems. Annu. Rev. Ecol. Syst. 4, 1–23. doi: 10.1146/annurev.es.04.110173.000245
Holmer, M., and Nielsen, S. L. (1997). Sediment sulfur patterns related to biomass-density patterns in Zostera marina (eelgrass) beds. Mar. Ecol. Prog. Ser. 146, 163–171. doi: 10.3354/meps146163
Hughes, A. R., Williams, S. L., Duarte, C. M., Heck, K. L., and Waycott, M. (2009). Associations of concern: declining seagrasses and threatened dependent species. Front. Ecol. Environ. 7:242. doi: 10.1890/080041
Hughes, T. P., Baird, A. H., Bellwood, D. R., Card, M., Connolly, S. R., Folke, C., et al. (2003). Climate change, human impacts, and the resilience of coral reefs. Science 301, 929–933. doi: 10.1126/science.1085046
Iyapparaj, P., Revathi, P., Ramasubburayan, R., Prakash, S., Palavesam, A., Immanuel, G., et al. (2014). Antifouling and toxic properties of the bioactive metabolites from the seagrasses Syringodium isoetifolium and Cymodocea serrulata. Ecotoxicol. Environ. Saf. 103, 54–60. doi: 10.1016/j.ecoenv.2014.02.009
Lane, A. L., Nyadong, L., Galhena, A. S., Shearer, T. L., Stout, E. P., Parry, R. M., et al. (2009). Desorption electrospray ionization mass spectrometry reveals surface-mediated antifungal chemical defense of a tropical seaweed. Proc. Natl. Acad. Sci. U. S. A. 106, 7314–7319. doi: 10.1073/pnas.0812020106
Lankau, R. A. (2007). Specialist and generalist herbivores exert opposing selection on a chemical defense. New Phytol. 175, 176–184. doi: 10.1111/j.1469-8137.2007.02090.x
Løvdal, T., Olsen, K. M., Slimestad, R., Verheul, M., and Lillo, C. (2010). Synergetic effects of nitrogen depletion, temperature, and light on the content of phenolic compounds and gene expression in leaves of tomato. Phytochemistry 71, 605–613. doi: 10.1016/j.phytochem.2009.12.014
Marhaeni, B., Radjasa, O. K., Khoeri, M. M., Sabdono, A., Bengen, D. G., and Sudoyo, H. (2011). Antifouling activity of bacterial symbionts of seagrasses against marine biofilm-forming bacteria. J. Environ. Protect. 02, 1245–1249. doi: 10.4236/jep.2011.29143
Menge, B. A., Sanford, E., Daley, B. A., Freidenburg, T. L., Hudson, G., and Lubchenco, J. (2002). Inter-hemispheric comparison of bottom-up effects on community structure: insights revealed using the comparative-experimental approach. Ecol. Res. 17, 1–16. doi: 10.1046/j.1440-1703.2002.00458.x
Nejrup, L. B., and Pedersen, M. F. (2008). Effects of salinity and water temperature on the ecological performance of Zostera marina. Aquat. Bot. 88, 239–246. doi: 10.1016/j.aquabot.2007.10.006
Oliver, E. C. J., Donat, M. G., Burrows, M. T., Moore, P. J., Smale, D. A., Alexander, L. V., et al. (2018). Longer and more frequent marine heatwaves over the past century. Nat. Commun. 9:1324.
Orth, R. J., Carruthers, T. J. B., Dennison, W. C., Duarte, C. M., Fourqurean, J. W., Heck, K. L., et al. (2006). A global crisis for seagrass ecosystems. Bioscience 56, 987–996.
Pansch, C., Scotti, M., Barboza, F. R., Al-Janabi, B., Brakel, J., Briski, E., et al. (2018). Heat waves and their significance for a temperate benthic community: a near-natural experimental approach. Glob. Change Biol. 24, 4357–4367. doi: 10.1111/gcb.14282
Papazian, S., Parrot, D., Burýšková, B., Weinberger, F., and Tasdemir, D. (2019). Surface chemical defence of the eelgrass Zostera marina against microbial foulers. Sci. Rep. 9:3323. doi: 10.1038/s41598-019-39212-3
Plummer, M. L., Harvey, C. J., Anderson, L. E., Guerry, A. D., and Ruckelshaus, M. H. (2013). The role of eelgrass in marine community interactions and ecosystem services: results from ecosystem-scale food web models. Ecosystems 16, 237–251. doi: 10.1007/s10021-012-9609-0
Ravn, H., Pedersen, M. F., Borum, J., Andary, C., Anthoni, U., Christophersen, C., et al. (1994). Seasonal variation and distribution of two phenolic compounds, rosmarinic acid and caffeic acid, in leaves and roots-rhizomes of eelgrass (Zostera marina L.). Ophelia 40, 51–61. doi: 10.1080/00785326.1994.10429550
Reusch, T. B., Ehlers, A., Hammerli, A., and Worm, B. (2005). Ecosystem recovery after climatic extremes enhanced by genotypic diversity. Proc. Natl. Acad. Sci. U.S.A. 102, 2826–2831. doi: 10.1073/pnas.0500008102
Saha, M., Barboza, F. R., Somerfield, P. J., Al-Janabi, B., Beck, M., Brakel, J., et al. (2019). Response of foundation macrophytes to near-natural simulated marine heatwaves. Glob Change Biol. 26, 417–430. doi: 10.1111/gcb.14801
Saha, M., Rempt, M., Gebser, B., Grueneberg, J., Pohnert, G., and Weinberger, F. (2012). Dimethylsulphopropionate (DMSP) and proline from the surface of the brown alga Fucus vesiculosus inhibit bacterial attachment. Biofouling 28, 593–604. doi: 10.1080/08927014.2012.698615
Saha, M., Rempt, M., Grosser, K., Pohnert, G., and Weinberger, F. (2011). Surface-associated fucoxanthin mediates settlement of bacterial epiphytes on the rockweed Fucus vesiculosus. Biofouling 27, 423–433. doi: 10.1080/08927014.2011.580841
Saha, M., Rempt, M., Stratil, S. B., Wahl, M., Pohnert, G., and Weinberger, F. (2014). Defence chemistry modulation by light and temperature shifts and the resulting effects on associated epibacteria of Fucus vesiculosus. PLoS One 9:e105333. doi: 10.1371/journal.pone.0105333
Saha, M., and Wahl, M. (2013). Biofouling : the journal of bioadhesion and biofilm seasonal variation in the antifouling defence of the temperate brown alga fucus vesiculosus. Biofouling 29, 661–668. doi: 10.1080/08927014.2013.795953
Saha, M., Wiese, J., Weinberger, F., and Wahl, M. (2016). Rapid adaptation to controlling new microbial epibionts in the invaded range promotes invasiveness of an exotic seaweed. J. Ecol. 104, 969–978. doi: 10.1111/1365-2745.12590
Salo, T., and Pedersen, M. F. (2014). Synergistic effects of altered salinity and temperature on estuarine eelgrass (Zostera marina) seedlings and clonal shoots. J. Exp. Mar. Biol. Ecol. 457, 143–150. doi: 10.1016/j.jembe.2014.04.008
Sudatti, D. B., Fujii, M. T., Rodrigues, S. V., Turra, A., and Pereira, R. C. (2011). Effects of abiotic factors on growth and chemical defenses in cultivated clones of laurencia dendroidea J. Agardh (Ceramiales, Rhodophyta). Mar. Biol. 158, 1439–1446. doi: 10.1007/s00227-011-1660-4
Sullivan, B. K., Trevathan-Tackett, S. M., Neuhauser, S., and Govers, L. L. (2018). Review: host-pathogen dynamics of seagrass diseases under future global change. Mar. Pollut. Bull. 134, 75–88. doi: 10.1016/j.marpolbul.2017.09.030
Sunday, J. M., Bates, A. E., and Dulvy, N. K. (2012). Thermal tolerance and the global redistribution of animals. Nat. Clim. Change 2, 686–690. doi: 10.1038/nclimate1539
Vergeer, L. H. T., Aarts, T. L., and Degroot, J. D. (1995). The wasting disease and the effect of abiotic factors (light-Intensity, temperature, salinity) and infection with Labyrinthula zosterae on the phenolic content of Zostera marina shoots. Aquat. Bot. 52, 35–44. doi: 10.1016/0304-3770(95)00480-N
Wahl, M., Buchholz, B., Winde, V., Golomb, D., Guy-Haim, T., Müller, J., et al. (2015). A mesocosm concept for the simulation of near-natural shallow underwater climates: the kiel outdoor benthocosms (KOB). Limnol. Oceanogr. Methods 13, 651–663. doi: 10.1002/lom3.10055
Wahl, M., Jensen, P. R., and Fenical, W. (1994). Chemical control of bacterial epibiosis on ascidians. Mar. Ecol. Prog. Ser. 110, 45–57.
Wahl, M., Goecke, F., Labes, A., Dobretsov, S., and Weinberger, F. (2012). The second skin: ecological role of epibiotic biofilms on marine organisms. Front. Microbiol. 3:292. doi: 10.3389/fmicb.2012.00292
Wahl, M., Shahnaz, L., Dobretsov, S., Saha, M., Symanowski, F., David, K., et al. (2010). Ecology of antifouling resistance in the bladder wrack Fucus vesiculosus: patterns of microfouling and antimicrobial protection. Mar. Ecol. Prog. Ser. 411, 33–48. doi: 10.3354/meps08644
Wang, S., Weinberger, F., and Lenz, M. (2018). Fluctuations in the strength of chemical antifouling defenses in a red macroalga in response to variations in epibiont colonization pressure. Mar. Biol. 165:107. doi: 10.1007/s00227-018-3365-4
Waycott, M., Duarte, C. M., Carruthers, T. J. B., Orth, R. J., Dennison, W. C., Olyarnik, S., et al. (2009). Accelerating loss of seagrasses across the globe threatens coastal ecosystems. Proc. Natl. Acad. Sci. U.S.A. 106, 12377–12381. doi: 10.1073/pnas.0905620106
Weinberger, F., Rohde, S., Oschmann, Y., Shahnaz, L., Dobretsov, S., and Wahl, M. (2011). Effects of limitation stress and of disruptive stress on induced antigrazing defense in the bladder wrack Fucus vesiculosus. Mar. Ecol. Prog. Ser. 427, 83–94. doi: 10.3354/meps09044
Werner, F. J., Graiff, A., and Matthiessen, B. (2016). Temperature effects on seaweed-sustaining top-down control vary with season. Oecologia 180, 889–901. doi: 10.1007/s00442-015-3489-x
Winters, G., Nelle, P., Fricke, B., Rauch, G., and Reusch, T. B. H. (2011). Effects of a simulated heat wave on photophysiology and gene expression of high- and low-latitude populations of Zostera marina. Mar. Ecol. Prog. Ser. 435, 83–95. doi: 10.3354/meps09213
Keywords: chemical defense, Zostera marina, heatwaves, fouling, climate extremes, plant–climate interactions, climate change
Citation: Guan C, Saha M and Weinberger F (2020) Simulated Heatwaves Lead to Upregulated Chemical Defense of a Marine Foundation Macrophyte Against Microbial Colonizers. Front. Mar. Sci. 7:463. doi: 10.3389/fmars.2020.00463
Received: 01 November 2019; Accepted: 25 May 2020;
Published: 14 July 2020.
Edited by:
Michelle Jillian Devlin, Centre for Environment, Fisheries and Aquaculture Science (Cefas), United KingdomReviewed by:
Ryan Mueller, Oregon State University, United StatesJessie Campbell Jarvis, University of North Carolina Wilmington, United States
Copyright © 2020 Guan, Saha and Weinberger. This is an open-access article distributed under the terms of the Creative Commons Attribution License (CC BY). The use, distribution or reproduction in other forums is permitted, provided the original author(s) and the copyright owner(s) are credited and that the original publication in this journal is cited, in accordance with accepted academic practice. No use, distribution or reproduction is permitted which does not comply with these terms.
*Correspondence: Mahasweta Saha, sahamahasweta@gmail.com