- 1ArcticNet, Québec-Océan, Takuvik, Département de Biologie, Université Laval, Quebec City, QC, Canada
- 2Department of Fisheries and Oceans Canada, St. John’s, NL, Canada
- 3Québec Océan, Institut des Sciences de la Mer de Rimouski, Université du Québec à Rimouski, Rimouski, QC, Canada
- 4Departments of Geography, Biology, Earth Sciences, Memorial University, St. John’s, NL, Canada
- 5Département de Biologie, Université de Sherbrooke, Sherbrooke, QC, Canada
- 6Québec Océan, Département de Biologie, Chimie et Géographie, Université du Québec à Rimouski, Rimouski, QC, Canada
The relationships between infaunal diversity and ecosystem function of biogenic structures in the Eastern Canadian Arctic remain poorly documented. Our study investigated the influence of sponge gardens at the Frobisher Bay site (137 m) and bamboo corals at the Baffin Bay site (1007 m) on the infaunal community structure and benthic ecosystem functioning. The occurrence of both types of biogenic structure type enhanced particular taxa and/or feeding guilds. A large density of suspension filter feeders was observed in bamboo coral sediment, whereas bare sediment exhibited a large proportion of nematodes and deposit-detritus feeders. Sponge gardens’ sediment showed a high proportion of isopods, Paraonidae polychaetes and up/down conveyors whereas bare sediment exhibited a large density of filter feeders. Through incubation cores, we measured ex situ benthic nutrient and oxygen fluxes at the sediment-water interface in each habitat and site. Biogeochemical fluxes varied significantly between habitats in the Baffin Bay site with a significant impact of bamboo coral habitat on nutrient fluxes (nitrate, ammonium, and silicate). Surprisingly, the sediment hosting bamboo corals acted as a source of nitrate and ammonium reaching values similar or higher to the Frobisher site despite the difference in water depth, and thus food supply between the two sites. These significant releases could derive from (i) a high organic matter deposition in bamboo coral habitat, allowed by their erected structure, (ii) a high efficiency of bioturbators (surficial modifiers and burrowers) mixing the surface layer of the sediment, and (iii) the difference in sediment type. Our study highlighted that, compared to its adjacent habitat, the presence of bamboo corals appeared to enhance the infaunal density and nutrient release of its sediment. In contrast, the impact of sponge gardens was not as clear as for bamboo coral habitat, likely due to the relatively significant presence of megabiota in the sponge garden adjacent habitat. Thus, our results based on a relatively small sample size, indicate that the bamboo coral habitat seems to increase the efficiency of deep-benthic ecosystem functioning, while that of sponge garden on the shallow ecosystem functioning remains uncertain.
Introduction
Three-dimensional biogenic structures, designated as marine animal forests, are composed primarily of ecosystem engineering species such as sponges, cnidarians, bryozoans, ascidians and other sessile benthic organisms (Gili and Coma, 1998; Rossi et al., 2012). Ecosystem engineering species shape their habitat and the resources therein, which directly or indirectly benefits the entire ecosystem (Jones et al., 1994). Habitat heterogeneity resulting from these structures represents one of the main factors that influences the distribution of biodiversity at both local and regional spatial scales (Levin et al., 2001; Buhl-Mortensen et al., 2010). Biogenic structures provide microhabitats and shelters for a large assembly of fish species, mobile crustaceans and mollusks (Fosså et al., 2002; Cerrano et al., 2010; Baillon et al., 2012; Buhl-Mortensen and Buhl-Mortensen, 2014), and usually harbor more biodiversity than bare sediment (i.e., habitat without biogenic structures; Cerrano et al., 2010) at comparable depths. The main factor driving this biodiversity gain in biogenic habitats is derived, in part, from their erected structure. Indeed, by decreasing the current flow velocity, biogenic structures stabilize soft substrates and increase organic matter sedimentation (Bruno and Kennedy, 2000; Cerrano et al., 2010). Previous studies have shown that hotspots of organic matter processing formed by scleractinian cold-water corals contribute to an enhancement of the microbial activity altering nutrient cycling (e.g., nitrogen), and thus inducing a higher oxygen consumption and nutrient releases (Khripounoff et al., 2014; Cathalot et al., 2015). Similarly, to biogenic structures, sponges help to consolidate and stabilize the sediment (Bell, 2008). Sponges may also influence the water column by consuming dissolved oxygen by respiration (Bell, 2008) filtering and catching particles for food supply (e.g., dissolved organic carbon; Yahel et al., 2003) and altering nutrient cycling (i.e., silicate and nitrogen; Bell, 2008).
Resource availability, water depth, biodiversity, and climate change strongly influence ecosystem functioning (Bourgeois et al., 2017). The remineralization of sediment organic matter is considered to be an important ecosystem process and is measured as oxygen consumption and benthic nutrient fluxes (e.g., ammonium, nitrate, nitrite, phosphate and silicate). The quantity and the quality of food supply (i.e., organic matter and pigments) reaching the seafloor are generally related to the mineralizing activity of bacteria in the water column. Consequently, a decrease in organic matter availability and quality is often observed with increasing water depth. Water depth and resource availability (i.e., quality and quantity) have a great influence on sediment oxygen demand (SOD) (Mouret et al., 2010; Bourgeois et al., 2017). Functional diversity has profound implications for ecosystems and their functioning (Mermillod-Blondin et al., 2004; Michaud et al., 2006, 2009; Link et al., 2013a; Snelgrove et al., 2014) and seems to be more important than the diversity per se (Bolam et al., 2002; Belley et al., 2016; Belley and Snelgrove, 2017). As a result of their bioturbation activities (e.g., reworking sediment and bio-irrigation) and their behavior (e.g., feeding, mobility), which generate different levels of disturbance (Biles et al., 2002) infaunal organisms may cyclically enhance oxygen penetration within the sediment, increase oxic respiration, stimulate organic matter degradation and change inorganic nutrient fluxes at the sediment-water interface (Vopel et al., 2003; Belley and Snelgrove, 2016). By transporting fresh organic matter into the deeper sediment layers, gallery diffuser organisms (i.e., digging galleries in the sediment) stimulate microbial processes inducing an influx of oxygen and nitrate from the water into the anoxic parts of the sediment and a release of ammonium and phosphate (Aller, 1988; Michaud et al., 2006; Piot et al., 2013; Belley and Snelgrove, 2017).
Climate change is also an important factor in driving the Arctic ecosystem functioning (Hoegh-Guldberg et al., 2018). With global warming, a significant decrease in the extent and duration of ice cover is already observed (Hoegh-Guldberg and Bruno, 2010; Barnhart et al., 2016). These changes will (i) affect the duration and magnitude of phytoplankton blooms (Wassmann and Reigstad, 2011) and consequently the resources availability for benthic communities as well as (ii) open more shipping routes in the Arctic. The latter would intensify maritime activities such as fishing and transport (Melia et al., 2016) and increase the risk of introducing invasive species (Goldsmit et al., 2019). Although biogenic structures may adapt to naturally changing environmental variables (e.g., water mass temperature anomaly; Rossi et al., 2011) direct disturbances such as bottom trawling, overfishing, the introduction of invasive species and oil drilling represent a significant threat to habitat heterogeneity, food availability, biodiversity, sediment dynamics and ecosystem functioning (Thrush and Dayton, 2002; Rossi, 2013; Pusceddu et al., 2014). Biogenic structures have been defined as vulnerable marine ecosystems due to their scarcity, fragility, and the high density and diversity of organisms associated with them (FAO, 2009). In order to provide for the consequences of increased human activity (e.g., bottom trawling, oil drilling), baselines must be established with respect to biodiversity within vulnerable marine ecosystems, interspecies relationships and their impact on ecosystem functioning. In 2010, a strategic plan for biodiversity (Aichi Biodiversity Targets) was adopted to increase, inter alia, the conservation areas of particular importance for biodiversity and ecosystem services (Target 11, Strategic Goal C) (Secretariat of the Convention on Biological Diversity, 2014). In Eastern Canadian Arctic areas of high coral and sponge density have been identified using scientific trawl surveys data (Kenchington et al., 2010). More recently, remotely operated vehicle (ROV) video surveys in this region led to the identification of two areas holding high concentrations of biogenic structures. Neves et al. (2015) reported dense aggregations of bamboo coral colonies (Keratoisis sp.) living on a soft substrate in the Southeast of Baffin Bay (873 m). Furthermore, Dinn et al. (2019) reported the presence of dense aggregations of sponges (Iophon koltuni) on soft substrate in Frobisher Bay (137 m).
Our study aimed at assessing the influence of biogenic structures (i.e., bamboo corals and sponges) on the infaunal community structure and ecosystem functioning. We addressed our objectives by exploring the following research questions: (i) are the taxonomic and functional diversities of infaunal communities similar in bare sediment and biogenic structure sediment?; (ii) do biogeochemical fluxes at the sediment-water interface vary spatially according to the nature of the habitat (biogenic structures vs. bare sediment)?; and (iii) which environmental and biological variables explain biogeochemical fluxes in these habitats?
We formulated the following hypotheses: (i) infauna are more abundant and diverse in biogenic structures compared to bare sediment regardless of water depth and the nature of the biogenic structure; (ii) sediment in biogenic structures exhibits a higher oxygen demand relative to bare sediment due to the higher levels of organic matter deposition and larger biogeochemical fluxes; and (iii) resource availability (i.e., sedimentary organic matter content and chlorophyll a concentration), infauna density and bioturbation activities are the main variables explaining biogeochemical fluxes.
Materials and Methods
Study Area and Field Sampling
Samples were collected at two sites in the Eastern Canadian Arctic (Figure 1) aboard the scientific icebreaker CCGS Amundsen during the summer of 2017 (July 15–27). In Frobisher Bay (FB), the sampled sites are in the shallowest enclosed portion of the Bay at depths of 135–137 m within an area of submarine slope failures <1 km from Hill Island (Figure 1). Although the sampled locations were in areas mainly composed of soft substrate, a Super Mohawk ROV (Amundsen Science) video survey revealed the presence of bedrock walls near the targeted stations. In Baffin Bay (BB), the sampled site is located offshore in the Southeast portion of the Bay, at ∼140 km from Baffin Island, Canada (Figure 1). Multibeam bathymetry of the area indicated an overall flat slope, and ROV video surveys did not indicate the presence of rocky environments near the study area. BB samples were collected at depths of 874–1007 m. The sites were characterized by areas of bare sediment and biogenic habitats comprised of sponge gardens Iophon koltuni (Dinn et al., 2019) and bamboo corals Keratoisis sp. (Neves et al., 2015) in FB and BB, respectively. I. koltuni was the most abundant and dominant sponges in FB (Figure 2a); unfortunately, it was not possible to count individuals or estimate the covering percentage from the ROV videos because the camera was positioned in a forward-looking angle, rather than downward. Colonies of Keratoisis sp. were found in very high densities in this region (e.g., a 2000 kg trawl bycatch has been reported for this location; Kenchington et al., 2010) and grew from “root-like” branches, instead of from a single point of attachment (Figure 2c; Neves et al., 2015). Colonies could not be distinguished from one another based on our video data, and therefore the estimation of colony density was not possible. However, colonies are estimated to measure <1 m in height.
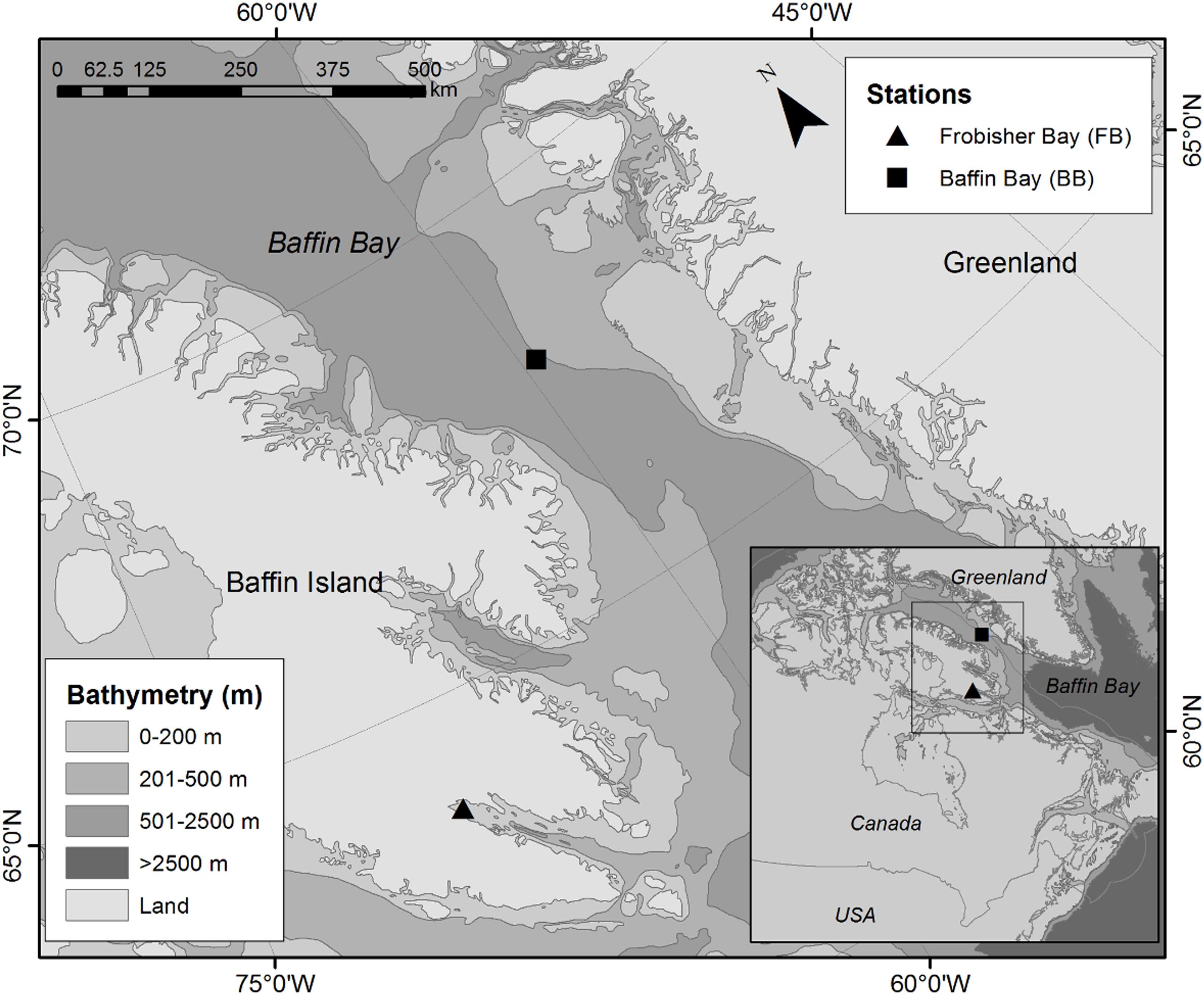
Figure 1. Sampling sites in Frobisher Bay (FB, 137 m depth) and in Baffin Bay (BB, 1007 m depth) in July 2017.
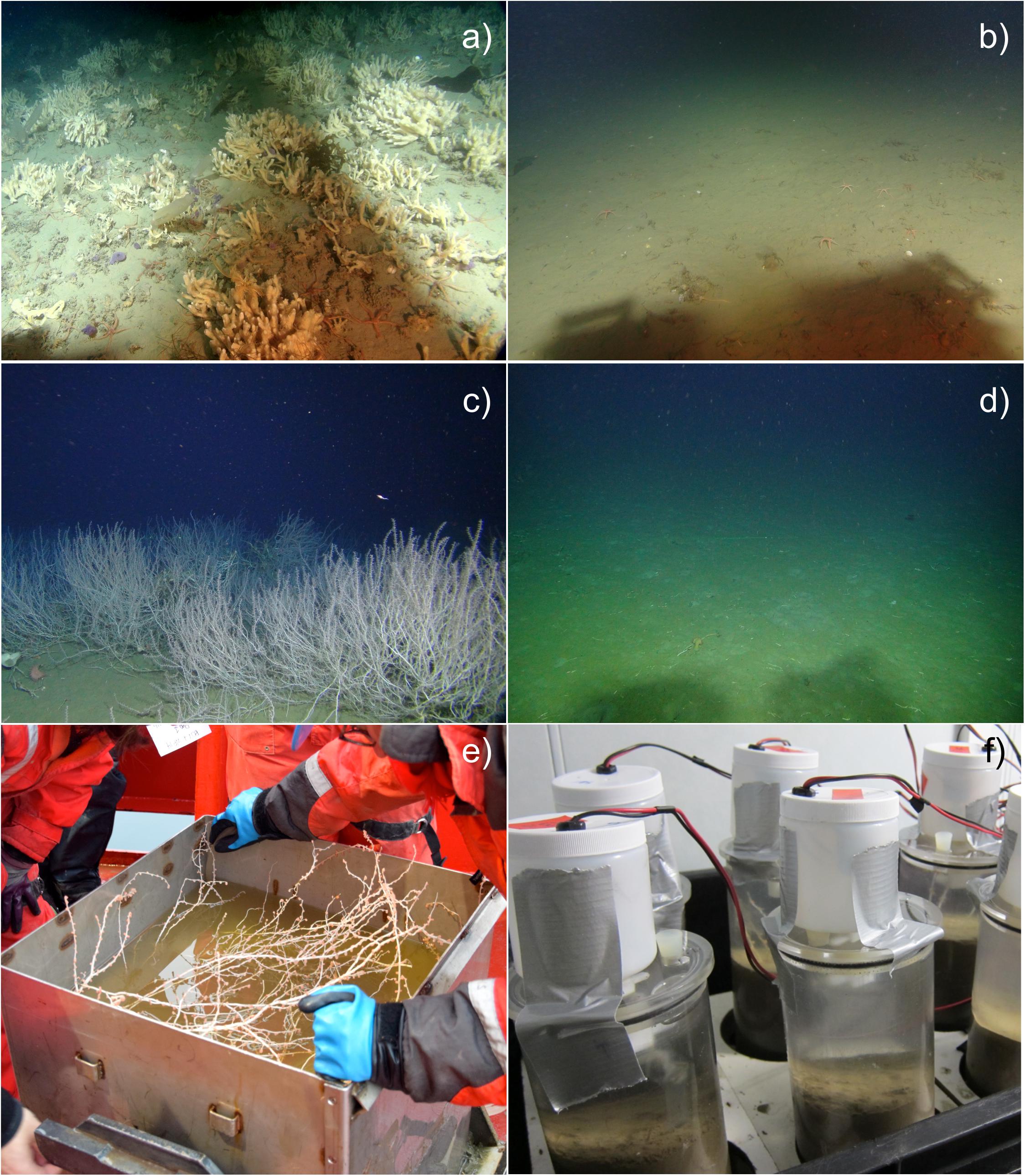
Figure 2. Photo-plate of the sampling sites and incubations experiments: (a) Sponge gardens (Iophon koltuni) in the FB site, (b) Bare sediment in the FB site, (c) Bamboo coral habitat (Keratoisis sp.) in the BB site, (d) Bare sediment in the BB site, (e) sample of the box-core deployed in bamboo corals, (f) Incubations experiments of the sediment cores. Credits (a–d): ArcticNet-CSSF-DFO.
The sampling positions at biogenic and non-biogenic habitats were defined during ROV video surveys at the two sites (Figures 2a–d). Positions were carefully selected to avoid overlap between the two types of habitats (i.e., when most of the camera’s field of view was dominated by a single habitat over the course of approximately 5 m). At each site, we deployed two box cores (0.5 × 0.5 m) per habitat (i.e., inside the biogenic structures and in the bare sediment; Figure 2e) approximately 200 m apart in FB site and 500 m apart in BB site. From each box core, we collected three sediment cores (i.d = 9.8 cm, H = 30 cm) for a total of six cores per habitat and 12 cores per site. Sediment cores sampled in biogenic structures habitats were visually exempted from biogenic structures. Bottom (10 m above the seafloor) temperature, salinity and oxygen saturation at each site were recorded with a conductivity-temperature-depth (CTD) probe. Sediment samples for chlorophyll a, phaeopigments and sediment properties were also collected from each box core (section “Environmental Variables”).
Incubations
Incubation cores, enclosing a 10 cm water layer on top of the 20 cm sediment layer, were carried out using bottom water collected from the same depth (Figure 2f). Sediment cores were acclimated for 6 h to allow suspended sediment particles to settle to the sediment surface. During this acclimation period, the water supernatant in cores was supplied with air using aquarium air pumps to avoid sub-oxic conditions during incubations. The cores were kept in the dark. After the acclimation period, each sediment core was sealed with a lid equipped with a magnetic stirrer and a hole open only for water sampling. Sediment cores were incubated in the dark at a temperature-controlled room (2–4°C) until a maximum of 20% of available oxygen was consumed. This procedure was carried out to avoid suboxic conditions and biogeochemical transformations (Renaud et al., 2007; Link et al., 2011). The duration of the incubation periods was 28 and 48 h for FB and BB sites, respectively. Three supplementary cores, incubated with bottom water only, served as controls, to measure oxygen consumption and nutrient concentrations in the water layer.
Benthic Fluxes Measurements
The sediment (community) oxygen demand was measured periodically (at 3 h intervals) by introducing an oxygen dipping probe through a hole in the lid (Fibox, PreSens, Regensburg, Germany). To determine the nutrient concentrations (NH4+, NO3–, NO2–, Si(OH)4, PO43–), water samples were collected at the sediment-water interface at the beginning, midpoint and end of incubations (Renaud et al., 2007; Link et al., 2011). The sampled water was immediately replaced by an equivalent volume of bottom water, with a known solute concentration. To prevent spurious measures, the volume of water withdrawn and replaced during each sampling never exceeded 10% of the total volume of the core. Water samples were filtered through GF/F filters (nominal porosity 0.7 μm) using acid-rinsed plastic syringes. Each sample was stored at −20°C until analysis. Measurements of nitrate, nitrite, phosphate and silicate were performed using a Bran and Luebbe Autoanalyzer 3 applying colorimetric methods adapted from Grasshoff et al. (1999). For ammonium measurements, water samples were analyzed using a Turner Design fluorimeter following the method proposed by Holmes et al. (1999). The detection limit was 0.100 μmol L–1 for ammonium, 0.010 μmol L–1 for nitrate and nitrite, 0.020 μmol L–1 for phosphate and 0.016 μmol L–1 for silicate.
Oxygen and nutrient fluxes were assessed from the slope of the linear regression of oxygen and nutrient concentration versus time of incubation and corrected for solute concentration in the replacement water measured at each filling. Median flux determined in the control cores was subtracted from each sediment core measurement. A positive nutrient flux indicates a release of nutrients from the sediment into the water column, while a negative flux signifies an uptake of nutrients from the water column into the sediment.
Infauna Identification and Taxonomic Diversity
Following incubation, sediment cores were sieved through a 500 μm mesh sieve to collect infauna. Organisms were fixed with 4% formaldehyde solution. They were sorted under a dissecting microscope in the laboratory and identified to the lowest possible taxonomic level. We determined density (N; mean ± SE individuals m–2) for each taxon, wet biomass (mean ± SE g m–2) and taxonomic richness as the number of taxa present in each sediment core (mean ± SE). We also calculated the Shannon-Wiener (H’) and Pielou’s evenness (J’) diversity indices for each sediment core using the “vegan” R package (R Core Team, 2018).
Biological Traits and Functional Diversity
We selected four biological trait categories, and 15 modalities based on availability for all taxa (Table 1) to reflect ecosystem functioning in terms of sediment oxygen consumption and nutrient cycling (Macdonald et al., 2010; Link et al., 2013b; Queirós et al., 2013; Degen et al., 2018; WoRMS Editorial Board, 2018). When a biological trait was not available for a specific taxon, we obtained information from one higher taxonomic level (Supplementary Table S1). We assigned a code, from 0 to 1 to each taxon for each modality. Hence, a value of 0 means complete absence of the modality and a value of 1 means complete dominance of the modality. For example, the Sedentaria Annelida Aricidea suecica can adjust its feeding type according to the environmental conditions, between filter/suspension feeder and deposit feeder, so we assigned 0.5 for its feeding type modality. Functional and taxa abundance matrices were used to measure multidimensional functional diversity indices (see below) using the “FD” package (Laliberté et al., 2014) in R (R Core Team, 2018): functional richness (FRic), functional evenness (FEve) (Villéger et al., 2008) and a single-trait-based index [i.e., the community-level weighted means of trait values (CWM)]. CWM is weighted by the relative abundance of organisms identified by the trait’s modality and represents functional composition (Lavorel et al., 2008; Laliberté and Legendre, 2010).
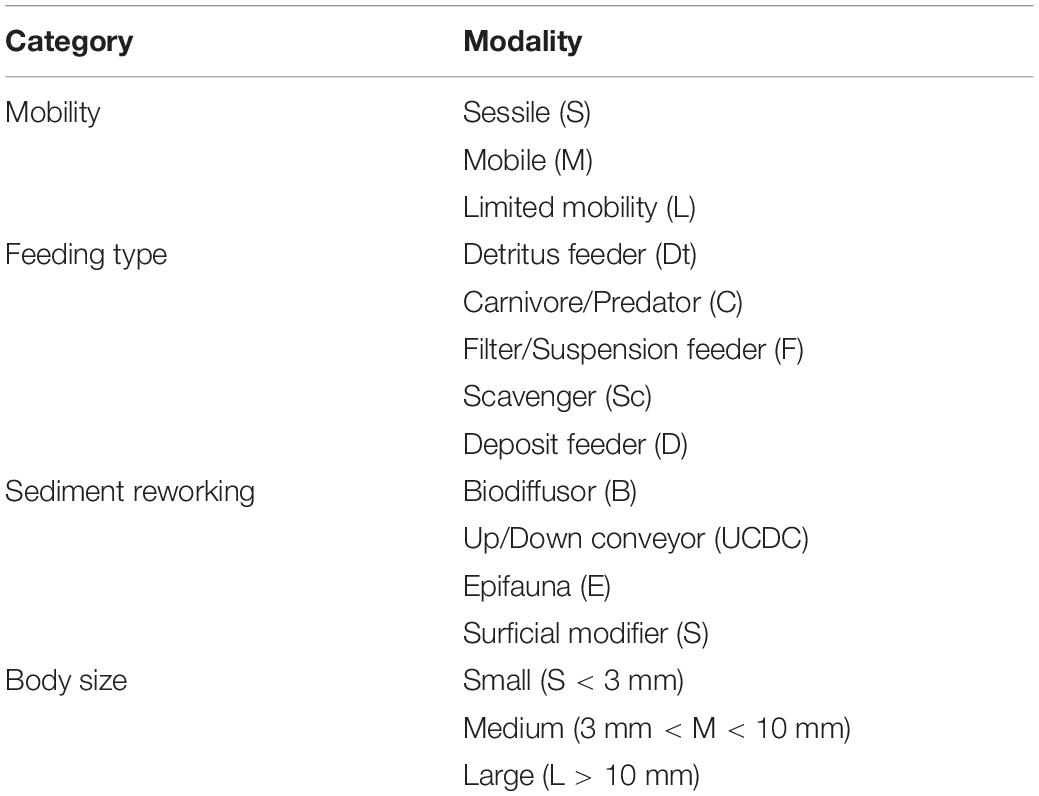
Table 1. Biological traits used in the functional diversity analysis according the categories and the modalities.
Environmental Variables
Three sub-cores of 2.4 cm in diameter were sampled within each box-core, for a total of six replicates per habitat, to characterize sediment surface properties: chlorophyll a (Chl a) and phaeopigment (phaeo) concentrations (mg m–2), organic matter content (% of dry sediment), porosity (%) and grain size (%).
Chlorophyll a and phaeopigment concentrations were quantified using a modified protocol of Riaux-Gobin and Klein (1993) and Link et al. (2011). We placed 2 g of wet sediment (the top centimeter of the sediment) in 10 mL of 90% acetone (v/v) for 24 h at 4°C and then analyzed the supernatant before and after acidification (HCL 5%) using a TURNER Design 10AU fluorometer. Then, the sediment was dried and weighed to standardize pigment concentrations per gram of dry sediment. We sampled the top centimeter of the sediment sub-cores to quantify organic matter (OM) content by loss of ignition (500°C). The water content was determined by comparing the mass of wet and dried sediment. Porosity was then calculated using a dry sediment density of 2.65 g cm–3 (Berner, 1980). We sampled the first five centimeters of sediment to determine grain size with a Laser Diffraction Particle Size Analyzer LA-950 HORIBA. Sediment type classes were based on Wentworth (1922); Folk and Ward (1957): mud (<3.9 μm), silt (<3.9 μm × < 62.5 μm), sand (<62.5 μm × < 2 mm) and gravel (>2 mm). No sieving was performed prior to analysis because no large particles (>2 mm) occurred in our sediment samples. Sediment properties are presented in Table 2.
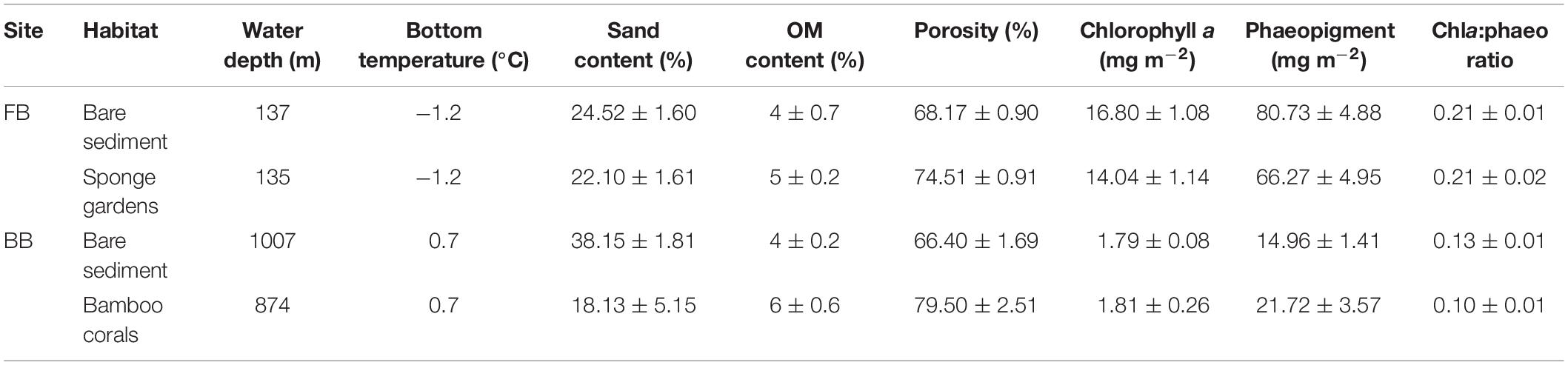
Table 2. Environmental conditions (mean ± SE) corresponding to the site (Frobisher Bay FB; and Baffin Bay BB) and the habitat.
Statistical Analyses
Spatial Variation of Environmental Variables
Principal Component Analysis (PCA; Hotelling, 1933) performed on a correlation matrix, was used to identify the combinations of environmental parameters that best explain habitat variability. Variables with a high Pearson’s correlation among themselves (>0.85) were excluded from the analysis. Chl a and phaeopigment concentrations were combined into a Chl a:phaeopigment ratio and only sand content was retained due to the negative correlation with mud. We carried out the PCA in R (R Core Team, 2018) using the “FactoMineR” package (Husson et al., 2020).
Spatial Variation of Benthic Communities and Benthic Fluxes
Frobisher Bay and Baffin Bay sites were analyzed separately. Density, univariate measures of biodiversity as well as benthic fluxes were analyzed using Student t-test with habitats (two levels: biogenic structures versus bare sediment) as factors and individual cores as replicates. All data were tested for normality and heteroscedasticity using Shapiro–Wilk and Bartlett’s test, respectively. When conditions were not satisfied, a non-parametric Wilcoxon-Mann-Whitney test was used instead. We carried out these analyses in R (R Core Team, 2018).
Benthic Biogeochemical Flux Drivers
Redundancy analysis (RDA; Rao, 1964) was used to identify the functional and taxonomic diversity indices as well as the environmental variables (explanatory variables) influencing biogeochemical flux variation (response variables) in biogenic structures and bare sediment. Predictor variables with a high Pearson’s correlation among themselves (>0.85) were excluded from the analyses (Shannon-Wiener index, bottom water dissolved oxygen, total pigments, Chl a, phaeo, mud content, and temperature). To obtain the model with the most parsimonious set of variables, a forward stepwise model selection was performed on the environmental variables (Chl a:phaeo ratio, organic matter content, porosity, sandy composition, water depth) and the diversity variables (taxa richness, density, Pielou’s evenness index, functional richness, functional evenness, CWM_modalities (Table 1; Blanchet et al., 2008). A permutation-based ANOVA was used to test the significance of axes and the term selected by the model. We performed the RDA using the “vegan” package (Oksanen et al., 2017) in R (R Core Team, 2018).
Results
Sediment Properties
Near-bottom water temperature at the study sites varied between −1.2 and 0.7°C, in the FB and BB sites, respectively (Table 2). Chl a concentration in the surficial seafloor sediments varied between 14.04 and 16.80 mg m–2 in the shallowest site FB and between 1.79 and 1.81 mg m–2 in the deeper site BB (Table 2). Phaeopigment concentrations in the surficial seafloor sediments ranged from 66.27 to 80.73 mg m–2 in the FB site and from 14.96 to 21.72 mg m–2 in the BB site (Table 2). The sediment had a sand content varying between 22.10 and 24.52% in the FB site and between 18.13 and 38.15% in the BB site (Table 2). The organic matter content ranged from 4 to 5% in the FB site and from 4 to 6% in the deeper site BB (Table 2).
The first and second principal components (PC1 and PC2, Figure 3) explained 62.3 and 24.8% of the variation within the data set, respectively. The sand content was negatively related to the OM content and porosity along PC1 (Figure 3). FB sites were separated from BB sites along the PC1 axis. Chlorophyll a to phaeopigment ratio was correlated positively along PC2. This shows that FB sites were correlated with a high Chl a:phaeo ratio, indicating a high concentration of Chl a and a low concentration of phaeopigment in the sediment (Table 2 and Figure 3). BB sites presented a lower concentration of those. Biogenic structure and bare sediment habitats in BB site were strongly separated across the PC2 axis. The sediment amongst biogenic structures at the BB site was muddy with high porosity and presented a high OM content. By contrast, bare sediment presented coarser sediment with low porosity and a lower OM content (Table 2 and Figure 3).
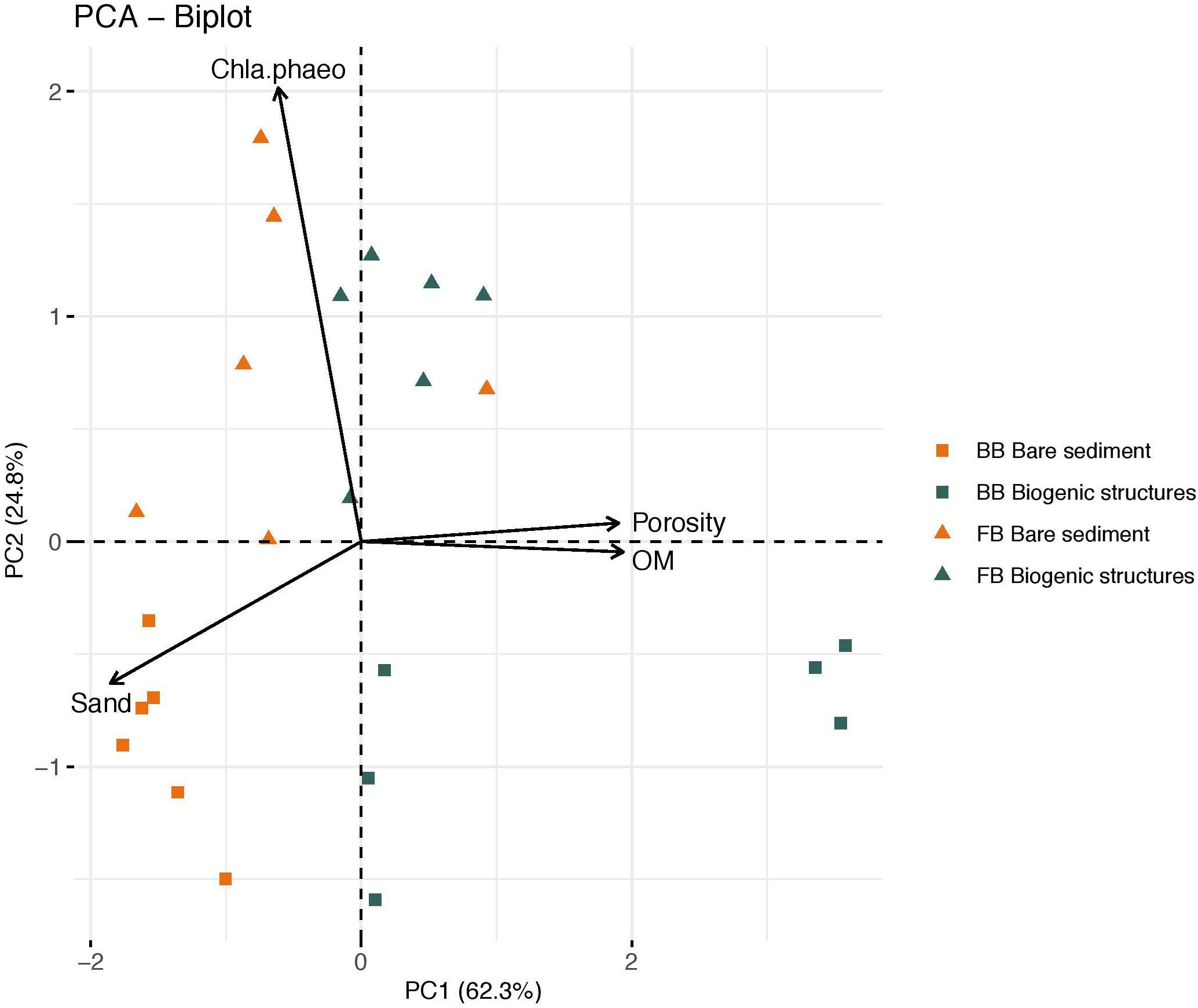
Figure 3. Principal component analysis (PCA) plot of environmental variables. Vectors indicate the direction and strength of each variables’ contribution to the overall distribution. Triangle and square labels define Frobisher Bay and Baffin Bay, respectively, while their colors (green and orange) represent the habitat: biogenic structures and bare sediment, respectively. The explicative variables are designated by Chla.phaeo (chlorophyll a:phaeopigment ratio), OM (% organic matter content), Sand (% sand content), and Porosity (%).
Infaunal Community Composition
A total of 115 taxa from 11 phyla were identified in this study. Fifty-three taxa were present in biogenic structure sediment and 60 taxa in bare sediment at the FB site. At the BB site, 39 taxa were present in biogenic structure sediment and 28 taxa in bare sediment.
At FB site, all polychaete families had similar proportions in each habitat, except for Paraonidae with a slightly higher proportion in biogenic structure than bare sediment (9 and 4% respectively), and Maldanidae with a lower proportion biogenic structure than bare sediment (4 and 8%, respectively, Figure 4A). Biogenic structure sediment and bare sediment had similar proportions of amphipods (4 and 6%, respectively) and cumaceans (7%). In contrast, biogenic structures had higher proportion of isopods (9%) dominated by Desmosoma lineare. Bare sediment had higher proportion of bivalves (7%) dominated by Ennucula tenuis and Macoma calcarea. Bioturbation type composition differed between habitats with higher proportion of surficial modifiers in biogenic structure sediment (e.g., Ennucula tenuis and Leucon nasicoides; 71%; Figure 4B). In contrast, bare sediment was distinguished from the biogenic structure sediment by higher proportion of up/down conveyors (e.g., Maldanidae; 23%). Deposit feeders showed similar densities in each habitat (Figure 4C). Feeding type composition differed between habitats with higher densities of filter feeders in bare sediment (e.g., Macoma calcarea; 14%; Figure 4C).
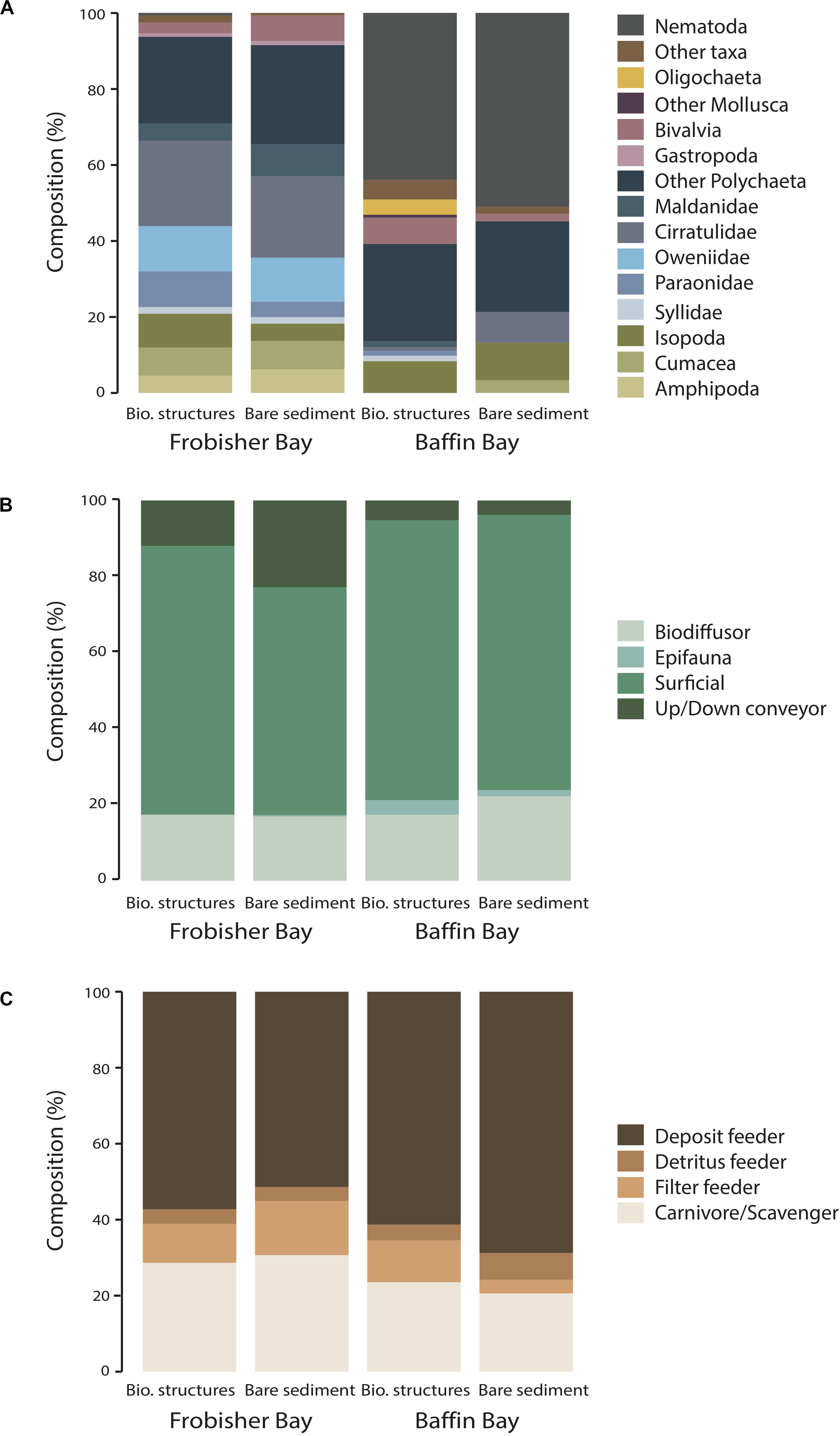
Figure 4. Taxonomic and functional trait composition in biogenic structure and its adjacent bare sediment habitat as a function of site (Baffin Bay and Frobisher Bay). (A) Taxonomic composition of dominant infauna. Other taxa include Cnidaria, Chordata, Echinodermata, Sipuncula, and Brachiopoda. (B) Bioturbation type and (C) Feeding type.
At BB site, nematodes were the most abundant group (47%) which had a slightly higher proportion in bare sediment (51%) than in biogenic structures (44%; Figure 4A). Polychaetes had similar proportions in both habitats. However, some taxa were only found in biogenic structure sediment such as Syllidae (e.g., Ancistrosyllis groenlandica), Paraonidae and Maldanidae. Moreover, bare sediment had the highest proportion of arthropods dominated by isopods (10%) and cumaceans (3%). In contrast, biogenic structure sediment showed a higher proportion of bivalves (7%) dominated by Thyasira sp. and Astarte sp. Biogenic structure sediment also had the highest proportion of “Other taxa” (2%) dominated by the hydrozoan Corymorpha sp. and the brachiopod Terebellinae. Surficial modifiers were the most abundant bioturbation group and showed similar proportions in each habitat (∼73%; Figure 4B). Bare sediment was distinguished from biogenic structure sediment by having slightly higher proportion of biodiffusors (e.g., Paraonidae; 22%), while biogenic structure sediment had a slightly higher proportion of epifauna (Figure 4B). Feeding type composition differed between habitats with higher proportion of filter feeders in biogenic structures (e.g., Thyasiridae; 11%) and higher densities of detritus/deposit feeders in bare sediment (e.g., Nematoda; 75%; Figure 4C).
Variation in Diversity and Functional Indices
At FB site, a higher average infaunal density trend was observed in bare sediment (1061 ± 69 ind m–2) compared to biogenic structure sediment (875 ± 67 ind m–2) but the difference was not statistically significant (p = 0.08; Table 3 and Figure 5A). Taxa richness presented similar values in biogenic structure sediment and bare sediment (19 ± 2 and 21 ± 1 taxa, respectively; Table 3 and Figure 5B). The same pattern was observed for biomass, which ranged from 24.3 ± 9.7 to 27.1 ± 9.9 g m–2 in biogenic structure sediment and bare sediment, respectively (p > 0.05; Table 3 and Figure 5C). Diversity indices (Shannon index and Pielou’s evenness) and functional indices (functional richness and functional evenness) did not differ between habitats (p > 0.05, Table 3).
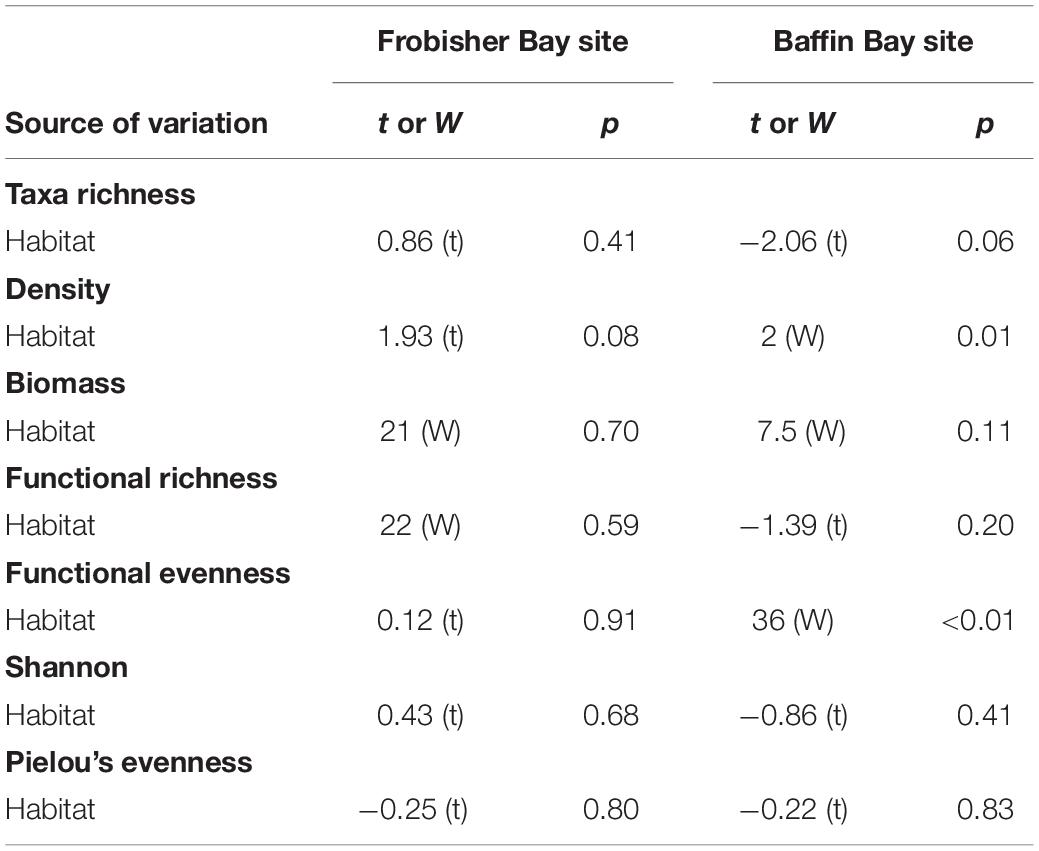
Table 3. Student’s t-test and Wilcoxon-Mann-Whitney (W) test results for density, diversity and functional indices to test the effect of habitat (biogenic structures and bare sediment) in Frobisher Bay and Baffin Bay sites.
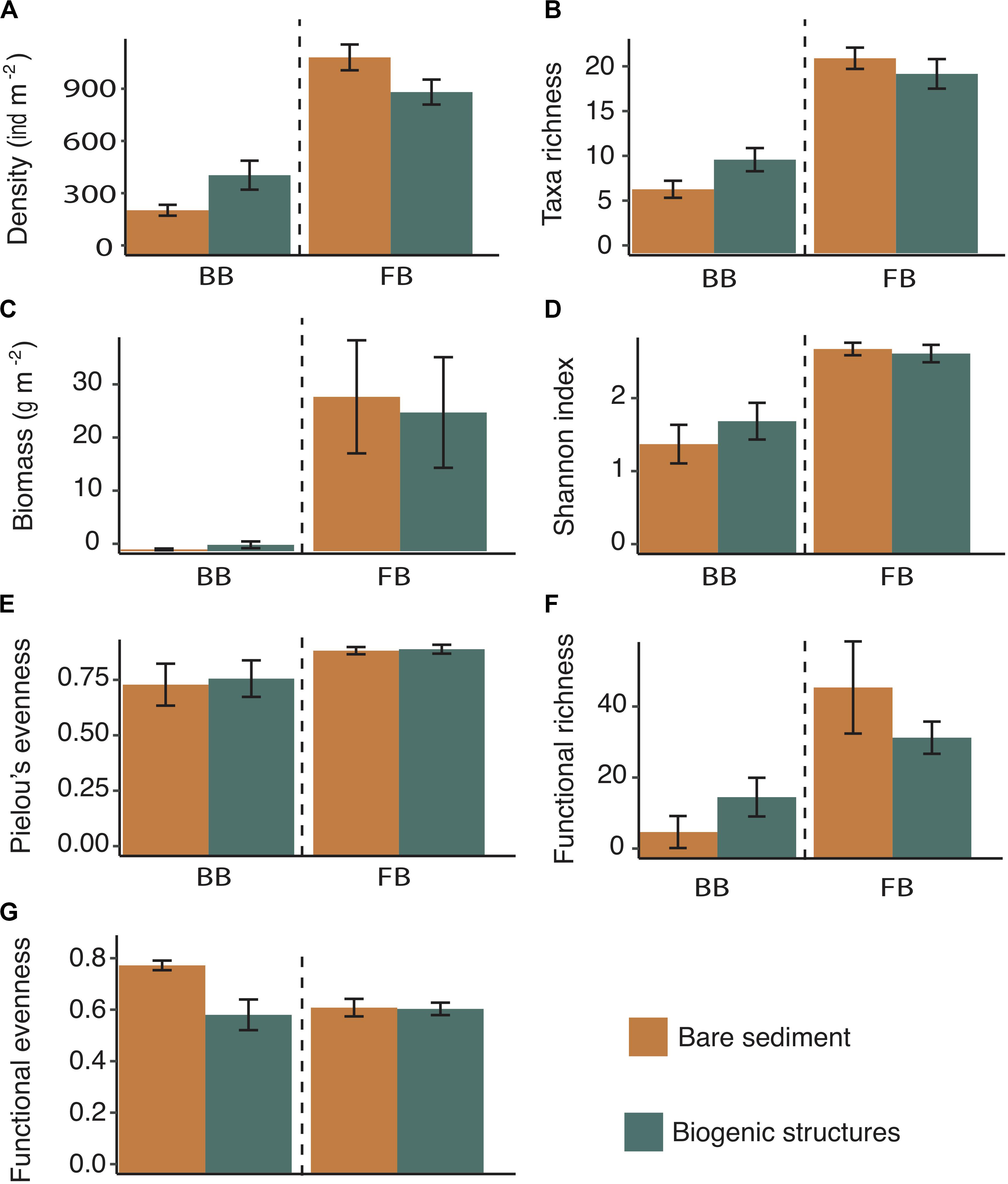
Figure 5. Mean functional and taxonomic diversity indices (±SE) in Baffin Bay (BB) and in Frobisher Bay (FB) within biogenic structures and bare sediment: (A) the density of benthic organisms (individuals m– 2), (B) taxa richness (number of taxa), (C) wet biomass (g m– 2), (D) Shannon index, (E) Pielou’s evenness index, (F) functional richness, and (G) functional evenness. Dash lines separate the site Baffin Bay and Frobisher Bay sites.
At BB site, infaunal density differed between habitats with a higher value in biogenic structure sediment (430 ± 78 ind m–2) compared to bare sediment (242 ± 29 ind m–2; p < 0.05; Figure 5A). Biogenic structure sediment showed a slightly higher number of taxa (10 ± 1 taxa) compared to bare sediment (7 ± 1 taxa; p = 0.06; Table 3 and Figure 5B). Biomass presented similar values in biogenic structure sediment and bare sediment (1.1 ± 0.6 and 0.3 ± 0.1 g m–2, respectively; p > 0.05; Figure 5C). Diversity indices (Shannon index and Pielou’s evenness) and functional richness did not differ between habitats (p > 0.05; Table 3 and Figures 5D–F). In contrast, functional evenness differed between habitats, with a higher evenness observed in bare sediment (p < 0.05; Figure 5G).
Variation in Benthic Biogeochemical Fluxes
At FB site, sediment oxygen uptake varied between 3242 ± 1014 μmol O2 m–2 d–1 in the biogenic structure sediment and 4291 ± 478 μmol O2 m–2 d–1 in the bare sediment (Figure 6A). The uptake of nitrite ranged from −2.7 ± 1 to −0.9 ± 1.4 μmol NO2– m–2 d–1 in bare sediment and biogenic structure sediment, respectively (Figure 6E). Nitrate was released from the sediment with values varying between 73 ± 19 and 90 ± 20 μmol NO3– m–2 d–1 in biogenic structure sediment and bare sediment, respectively (Figure 6F). The pattern for ammonium fluxes was more variable within the two habitats, with release values varying between 1.5 ± 35 and 36 ± 35 μmol NH4+ m–2 d–1 in biogenic structure sediment and bare sediment, respectively (Figure 6D). The release of silicic acid ranged from 1522 ± 178 to 2145 ± 242 μmol Si(OH)4 m–2 d–1 in biogenic structure sediment and bare sediment, respectively (Figure 6B). Phosphate was also released from the sediment with values ranging from 15 ± 5 to 29 ± 18 μmol PO43– m–2 d–1 (Figure 6C). Benthic nutrient fluxes did not differ between habitats (p > 0.10), except for a trend of higher silicate release in bare sediment (p = 0.06; Table 4 and Figure 6).
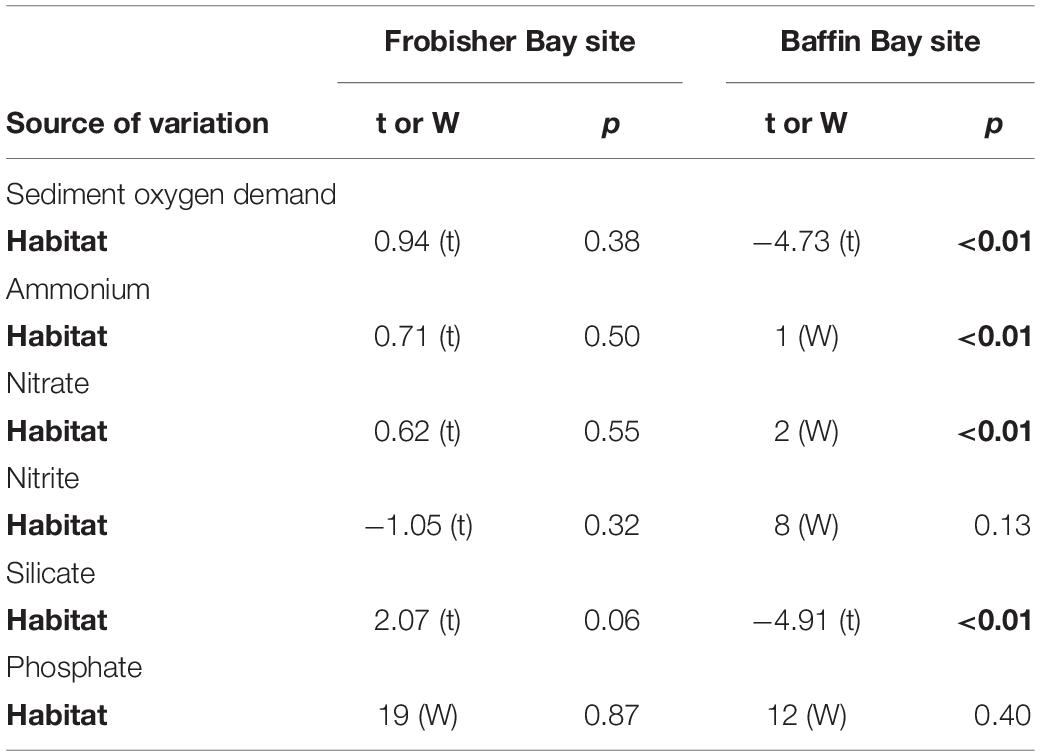
Table 4. Student’s t-test and Wilcoxon-Mann–Whitney (W) test results for individual biogeochemical fluxes to test the effect of habitat (biogenic structures and bare sediment) in Frobisher Bay and Baffin Bay sites.
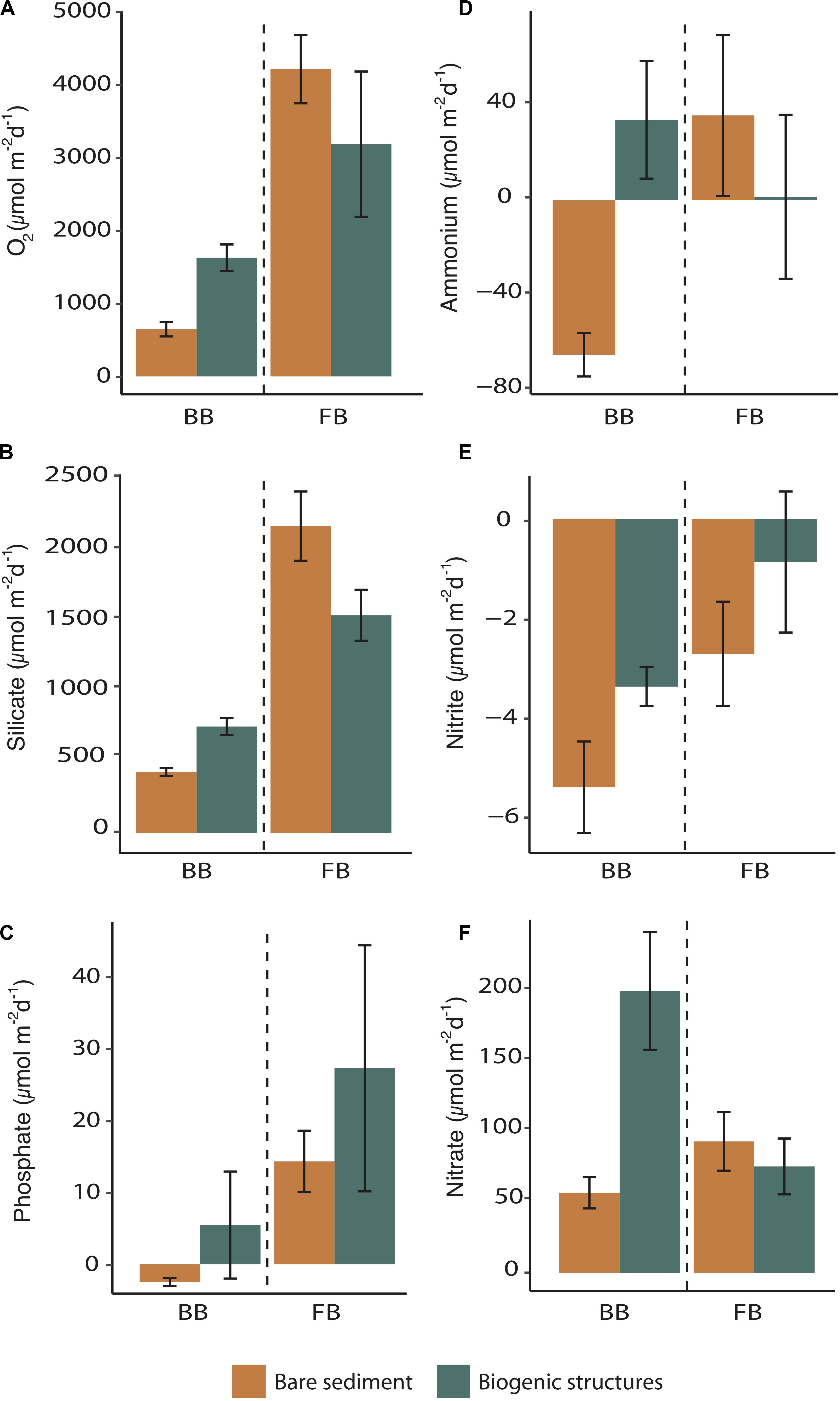
Figure 6. Mean biogeochemical flux (±SE) of (A) sediment oxygen demand, (B) silicate, (C) phosphate, (D) ammonium, (E) nitrite, and (F) nitrate measured within biogenic structures and bare sediment at each site (BB = Baffin Bay, FB = Frobisher Bay). Units are in μmol m– 2 d– 1. Green bars represent fluxes measured in biogenic structures and orange bars represent fluxes measured in bare sediment. Dash lines separate the site Baffin Bay and Frobisher Bay sites.
At BB site, sediment oxygen uptake was significantly (p < 0.01; Table 4) higher in biogenic structure sediment (1657 ± 186 μmol O2 m–2 d–1) than in bare sediment (659 ± 100 μmol O2 m–2 d–1; Figure 6A). Nitrite uptake did not differ between habitats (p = 0.13; Table 4), with values ranged from −5.4 ± 0.9 to −3.4 ± 0.4 μmol NO2– m–2 d–1 in bare sediment and biogenic structure sediment, respectively (Figure 6E). Nitrate was significantly (p < 0.01) higher in biogenic structure sediment (193 ± 40 μmol NO3– m–2 d–1) than in bare sediment (55 ± 11 μmol NO3– m–2 d–1; Figure 6F). The pattern for ammonium fluxes differed significantly between habitats (p < 0.01) with a release in biogenic structure sediment (34 ± 25 μmol NH4+ m–2 d–1) and an uptake in bare sediment (−66 ± 9 μmol NH4+ m–2 d–1; Figure 6D). The release of silicic acid was significantly higher (p < 0.01) in biogenic structure sediment (745 ± 59 μmol Si(OH)4 m–2 d–1) than in bare sediment (427 ± 27 μmol Si(OH)4 m–2 d–1; Figure 6B). Phosphate fluxes did not differ between habitats (p = 0.40; Table 4), with a trend of release (6 ± 8 μmol PO43– m–2 d–1) within the biogenic structure sediment, and a clear uptake in bare sediment (−3 ± 0.6 μmol PO43– m–2 d–1) (Figure 6C).
Benthic Biogeochemical Flux Drivers
Environmental Variables
From the five tested environmental parameters (i.e., Chl a:phaeo ratio, OM content, porosity, sandy composition and water depth), the stepwise model selected only water depth and sand content as the drivers of benthic fluxes. The model explained 38% (R2 = 0.43) of the total multivariate benthic flux variation. Permutational ANOVA test showed a significance of constraints of axes (p-value <0.05). The first and the second axis account for 28 and 15% of the total flux variation, respectively, (Supplementary Table S2 and Figure 7). Depth was positively correlated to the first axis, explaining 66% of the fitted flux variation (Supplementary Table S2 and Figure 7). The sand content was inversely correlated to the second axis, explaining 34% of the fitted flux variation.
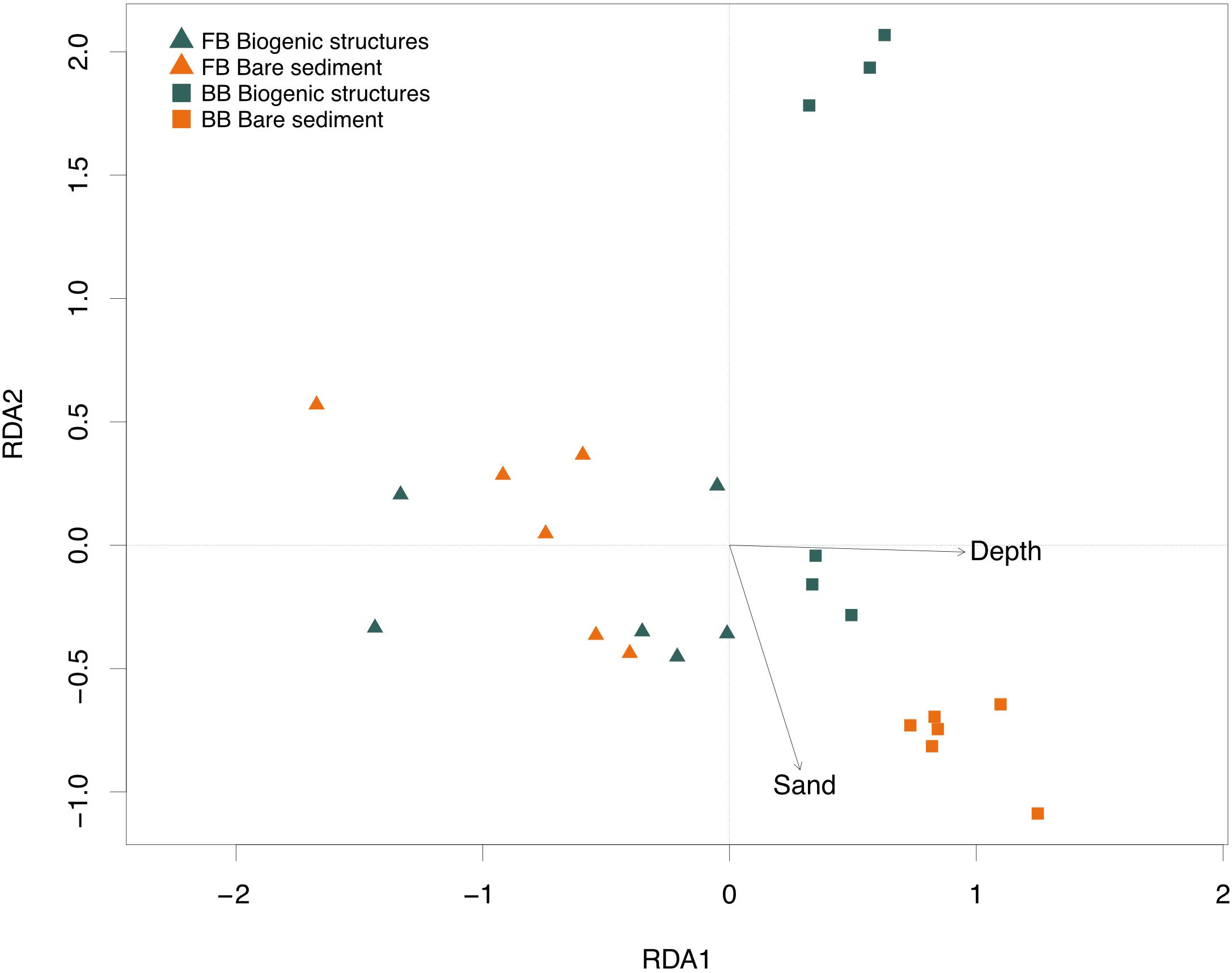
Figure 7. Plot of the redundancy analysis (RDA) models of environmental variables best explaining variation in Baffin Bay (BB) and Frobisher Bay (FB) biogenic structure sediment and bare sediment. Triangle and square labels define Frobisher Bay and Baffin Bay, respectively, while their colors (green and orange) represent the habitat: biogenic structures and bare sediment, respectively.
Diversity Indices
Of the 22 tested diversity indices (listed in section “Benthic Biogeochemical Flux Drivers”), the stepwise model selected only six variables: community weighted means of surficial modifiers (CWM.Surficial), up and down conveyors (CWM.UCDC), mobile organisms (CWM.Mobile), sessile organisms (CWM.Sessile), taxa richness (S) and functional evenness (FEve) as the drivers of benthic fluxes. The model explained 48% (R2 = 0.61) of the total multivariate benthic flux variation. The first and second axis of the RDA model accounted for 36 and 15% of total biogeochemical flux variation, respectively, (Supplementary Table S3 and Figure 8). The community weighted means of up and down conveyors (CWM.UCDC), sessile (CWM.Sessile) and taxa richness (S) contributed to the first axis explaining 58% of the fitted flux variation (Supplementary Table S3 and Figure 8). The second axis was correlated to functional evenness (Feve), explaining 25% of the fitted flux variation (Supplementary Table S3 and Figure 8). Benthic fluxes at the FB site were driven by high values of community weighted means of up and down conveyors and sessile organisms (CWM.UCDC and CWM.Sessile, e.g., Maldanidae) and higher taxa richness. Whereas, benthic fluxes at the BB site were primarily driven by high values of community weighted means of surficial modifiers (CWM.Surficial), mobile organisms (CWM.Mobile), and a high functional evenness (FEve).
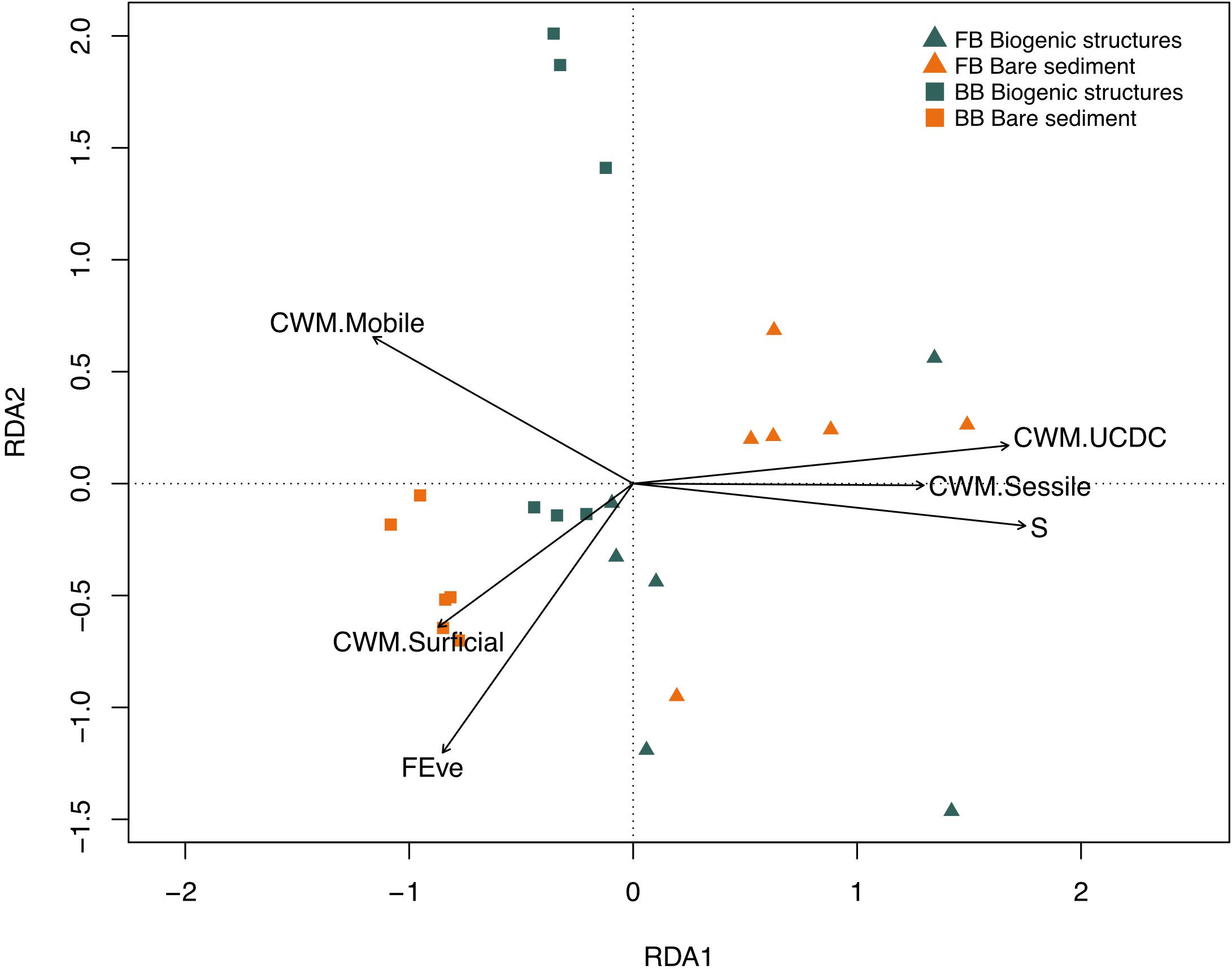
Figure 8. Plot of the redundancy analysis (RDA) models of functional and diversity indices best explaining variation in Baffin Bay (BB) and Frobisher (FB) Bay biogenic structure sediment and bare sediment. Triangle and square labels define Frobisher and Baffin Bay, respectively, while their colors (green and orange) represent the habitat: biogenic structures and bare sediment, respectively.
Discussion
Infaunal Communities
Habitat heterogeneity (grain size, substrate variability, and food supply) decreases with increasing water depth (Freiwald et al., 2004), inducing most generally a homogeneity in deep environments. At local scales, the occurrence of biogenic structures in a homogeneous habitat enhances its complexity by modifying the sedimentation of the particles and increasing the surface area available for settlement, shelter and niche space (Thrush and Dayton, 2002; Costello et al., 2005; Beazley et al., 2015). Effects of deep-water sponge gardens on infaunal assemblages have been shown previously in water depths above 245 m (Bett and Rice, 1992; Barrio Frojan et al., 2012; Beazley et al., 2015). In the current study, we highlighted some differences in the infaunal composition at a shallower depth (137 m; FB site). Sponge gardens provide a suitable habitat for reproduction, shelters, nurseries and constant food supply (Przeslawski et al., 2013; Kersken et al., 2014). Some infaunal organisms have been reported to live in association with sponge gardens (e.g., amphipods and polychaetes). For instance, we observed a higher proportion of isopods and the polychaete family Paraonidae in the FB sponge garden habitat where they could find shelters. On the contrary filter feeders like bivalves had a lower proportion in sponge garden habitat compared to bare sediment. Although biogenic structures generally increase organic particle sedimentation (Bruno and Kennedy, 2000; Cerrano et al., 2010), sponges are known to filter large volumes of water, reducing the detrital input to the surrounding seafloor due to their considerable filtration capacity (Vogel, 1977). Limiting the deposition of organic matter on the seafloor, sponge gardens at the FB site seem to result in a lower proportion of filter feeders and a lower infaunal density compared to its adjacent habitat. However, large ascidians, crinoids (Heliometra glacialis), sea anemones, drift/detrital kelp and other less represented sponges were abundant around sponge gardens, as previously reported by Dinn et al. (2019). Their patchy distributions in bare sediment might also contribute to infaunal density, inducing its higher heterogeneity in comparison to bare sediment found in the deep-sea. Therefore, in shallow habitats like FB (<245 m), we suggest that the effect of sponges on infauna might be virtually non-existent or harder to detect due to the apparent high heterogeneity of megabiota in its adjacent habitat and the high heterogeneity in the sponge gardens.
Contrary to sponges-associated infaunal communities, coral-associated infaunal communities exhibited differences from its adjacent bare sediment habitat. Cold-water corals have been reported to enhance biodiversity regardless of the coral taxa, although different levels of infaunal abundance or species richness have been noted (Demopoulos et al., 2014; Bourque and Demopoulos, 2018). Cold-water corals are frequently reported along continental margins with locally accelerated currents (Roberts and Cairns, 2014), and their occurrence directly modulates the availability of resources to their surrounding environment by enhancing the trapping of organic matter (Jones et al., 1994; Mienis et al., 2009; Van Oevelen et al., 2009; Cathalot et al., 2015). The high proportion of filter/suspension and deposit feeders, such as bivalves (e.g., Thyasira sp. and Astarte sp.), oligochaetes and “Other taxa” (e.g., Brachiopoda and Ascidiacea) in the bamboo coral habitat reflected its enrichment in suspended and particulate organic matter. Consistent with this finding, the high percentage of organic matter in bamboo coral sediment compared to its adjacent sediment (6 ± 0.6 and 4 ± 0.2%, respectively) attested to their positive effect on the trapping of organic matter. By improving food availability, bamboo coral habitat enhanced infaunal density. The distinction between BB habitats was also apparent in the functional trait analysis. The bare sediment habitat presented the highest functional evenness denoting less diverse functional traits. Thus, bamboo coral sediment appeared to be a more complex ecosystem compared to bare sediment habitat in the deep-sea.
Our results highlight the importance of octocorals as key ecosystem engineers in the deep-sea, which are critical to increase infauna density. For sponge gardens, the effect of this habitat seems to be more complex and some uncertainties could be underlined due to the heterogeneity of shallower site (FB).
Benthic Biogeochemical Flux Drivers
Sediment oxygen demand (i.e., SOD), measured in this study, exhibited comparable values to those reported in previous Arctic and Antarctic studies regarding continental shelves (10 460 ± 7 940 μmol O2 m–2 d–1) and slope/rise (2 230 ± 2 370 μmol O2 m–2 d–1; Piepenburg et al., 1995; Clough et al., 2005; Renaud et al., 2007; Hartnett et al., 2008; Link et al., 2011, 2013a, 2013b; Kim et al., 2016; Bourgeois et al., 2017). A high quantity of food supply reaching the sediment increases benthic mineralization processes and, therefore, oxygen consumption (Bianucci et al., 2012; Bourgeois et al., 2017). As previously mentioned, biogenic structures at the BB site showed a positive effect in the trapping of organic matter. Consistent with this observation, the highest SOD observed in biogenic structures at the BB site reflected its higher food supply to the benthos.
Regarding benthic nutrient fluxes (phosphate, silicate, nitrate, nitrite and ammonium), there are only a few reports for the Canadian Arctic sediment and data were mostly obtained from the Western Arctic and Northern Baffin Bay (Rysgaard et al., 2004; Kenchington et al., 2011; Link et al., 2011, 2013a, 2013b). Values in our study were in the range found in other regions of Arctic and Antarctic (Link et al., 2011, 2013a, 2013b; Kim et al., 2016; Bourgeois et al., 2017).
Bioturbation affects ecosystems and biogeochemical processes at the water-sediment interface at a varying degree depending on the community composition and the functional composition (Clough et al., 1997; Mermillod-Blondin et al., 2004; Michaud et al., 2006; Link et al., 2011, 2013b; Belley and Snelgrove, 2017). Bare sediment in the FB site presented a higher proportion of upward conveyors (e.g., polychaetes Maldanidae), feeding head-down at depth in the sediment. Thus, they could excrete components produced at depth to the surface (e.g., silicate, ammonium and nitrate; Kristensen et al., 2012) and explained the higher release of silicate in bare sediment habitat.
Low phosphate release was observed from the two habitats within the two sites. Since phosphate cannot be used as an electron acceptor (Hensen et al., 2006) its release to overlying water column was mostly explained by the aerobic organic matter mineralization and/or from the loss of phosphate adsorption capacity from the sediment (Sundby et al., 1992; Jensen et al., 1995; Anschutz et al., 2007). The phosphate release observed in the FB site was certainly due to an intensified oxic degradation of organic matter reflected by a higher SOD.
Fluxes involved in the nitrogen cycle (e.g., nitrate and ammonium) presented a complex pattern disturbed by (i) the water depth, (ii) the presence of bioturbators, and (iii) the sediment grain size. Shallow sediments (<500 m) usually present a high release of nitrate (∼100 μmol NO3– m–2 d–1) whereas at deep depth (>790 m), they present a low release of nitrate (<100 μmol NO3– m–2 d–1; Link et al., 2013b). Generally, highest nitrite uptake is linked to sediment with a low nitrate release and therefore indirectly correlated with water depth (Link et al., 2013a). Consistent with these findings, nitrate fluxes had values around 100 μmol NO3– m–2 d–1 and a low nitrite uptake in both habitats at the shallower site FB. As expected, we also observed a nitrate release below 100 μmol NO3– m–2 d–1 associated to a slightly higher nitrite uptake in bare sediment at the deeper BB site. Interestingly, we observed the highest value of nitrate release (192 ± 40 μmol NO3– m–2 d–1) in biogenic structure habitat at the deeper BB site. Likewise, BB biogenic structure sediment acted as a source of ammonium. This latter may suggest that much ammonium was produced, which could not wholly be turned over by nitrification to nitrate. These releases highlighted that the presence of cold-water corals stimulates ammonium release and nitrification rates of the underlying sediment (de Froe et al., 2019).
Burrower organisms, such as the bivalve Astarte sp. and the polychaete Ancistrosyllis groenlandica and Prionospio sp., ventilate their burrows which favors introducing fresh oxygenated water into the sediment. This input of oxygenated water in the nitrification zone (0 to 4 cm; Michaud et al., 2006) may stimulate nitrification reactions (Aller, 2001) and explain the release of nitrate and ammonium in biogenic structures sediment observed at the BB site. Moreover, bamboo coral sediment presented a high abundance of bivalves (e.g., Astarte sp.), mobile polychaetes (e.g., Pholoe sp., Ancistrosyllis groenlandica) and nematodes. All of these taxa, defined as surficial modifiers, mix the surface of the sediment (i.e., oxic layer where nitrate is produced) and despite their relatively low impact on bioturbation (Queirós et al., 2013) their high abundance positively affected nitrate and silicate fluxes (Aller, 1994; Soetaert et al., 1997; Ingels et al., 2009; Piot et al., 2013; Belley and Snelgrove, 2016). In our study, their presence might have stimulated organic matter degradation and hence the release of nutrients (e.g., nitrate and silicate).
On the contrary, bare sediment at the BB site acted as a sink of ammonium. This ammonium uptake could be related to (i) a sandier sediment and/or (ii) to a higher functional evenness. The permeable substrate supports the transport of solute advection that can affect sediment biogeochemistry and benthic exchange fluxes (Janssen et al., 2005; Rao et al., 2012; Huettel et al., 2014). For example, enhanced O2 supply can promote the reoxidation of reduced inorganic species produced under anaerobic conditions. This process would transport oxygen deeper in BB bare sediment, decreasing the SOD and deepening the different diagenetic redox fronts. The low phosphate uptake measured in BB bare sediment is in agreement with a deeper Fe-oxide rich horizon as discussed above. These conditions would favor the production of nitrate from ammonia and nitrite, by nitrification and chemioxidation (via metal-oxides oxidation). The exact mechanisms to form nitrate need further investigations and the net fluxes measured here do not allow to discriminate the diagenetic pathways involved. However, BB sediments are a source of benthic nitrate and its production requires the input of both ammonia and nitrite from the water column. Similarly, to Belley and Snelgrove (2017) we highlighted that a higher functional evenness was associated with lower benthic fluxes in bare sediment, despite a similar functional richness to biogenic structure’s sediment at the BB site. The difference in the density of some bioturbators (i.e., functional evenness) might be responsible for the differences in fluxes. For instance, the presence of micro-burrowers, built by nematodes at small-scale, is known to enhance sediment porosity which may facilitate fluid advection (Aller and Aller, 1992; Pike et al., 2001; Piot et al., 2013). The sandier sediment in bare sediment at the BB site (38.15 ± 1.81%) and the density of bioturbators (e.g., nematodes, biodiffusors) might affect the porosity (66.40 ± 1.69 and 79.5 ± 2.51% in bare sediment and biogenic structures sediment, respectively), the solutes transport processes and then the benthic fluxes. The latter might lead to an ammonium uptake and nitrate release in bare sediment habitat.
The benthic remineralization efficiency between the two sampling habitats at the BB site pointed to the importance of food supply and infaunal community bioturbators (e.g., up/down conveyors and surficial modifiers). Despite the lower infaunal density, taxonomic and functional diversity at the BB deep site, bamboo coral habitat seems to strongly influence deep-benthic ecosystem functioning by increasing its efficiency in the deep-sea and more particularly nitrate efflux, an element limiting for primary production in the Arctic sea (Tremblay et al., 2015).
Conclusion and Perspectives
Our study is the first to document and compare biodiversity and benthic fluxes within biogenic structures and bare sediment in the Canadian Arctic. Although the effects of sponge gardens on benthic biogeochemical fluxes and infaunal biodiversity have been previously highlighted in other studies, we could not demonstrate their effects, which might be related to the high heterogeneity of this shallower site. On the other hand, bamboo corals appeared to enhance the infaunal density and ecosystem functioning, despite their deep-water location, where food quality and supply are more limited. Nitrate efflux was the highest in this habitat, leading to a strong nutrient export toward surrounding waters. The latter was mostly derived from the impact of the physical structure of bamboo corals on particles deposition, associated to a high abundance of infaunal bioturbators (surficial modifiers). Our sample size was small due to the constraints of working in the Arctic and deep-water environments; therefore, our results should be interpreted in light of this limitation.
Bamboo corals, such as the ones found at the BB site, are vulnerable marine ecosystem indicators, and the surveyed area is part of a marine refuge (Disko Fan Conservation Area) where bottom-contact fishing activities are currently partly prohibited (Hiltz et al., 2018). A better understanding of their spatial distribution both inside and outside of the refuge boundaries, as well as of their capacity in the trapping of organic matter, and their role as habitat for epifauna is warranted. Arctic sponge gardens at the FB site, have only recently been discovered. However, although we were less successful at identifying a clear ecosystem functional role of these sponges in terms of their influence on infauna and biogeochemical fluxes, a better comprehension of sponge gardens distribution and ecological roles is still essential before they become exposed to physical damage from anthropogenic activities.
In view of a seafloor homogenization due to the anticipated increase in fisheries in the Arctic, it seems necessary to extend studies on biogenic structures to better understand their role in the Arctic ecosystem functioning.
Data Availability Statement
The datasets generated for this study are available on request to the corresponding author.
Author Contributions
MP designed the study, prepared the data, performed the analyses, and led the drafting of the manuscript. CG performed the infauna samples, benthic incubations and ammonium analyses, reviewed drafts of the manuscript, and approved the final draft. BN made figures, authored and reviewed drafts of the manuscript, and approved the final draft. GC, EE, and FM authored and reviewed drafts of the manuscript and approved the final draft. FB authored and reviewed analyses, drafts of the manuscript, and approved the final draft. CN and PA designed the study, authored and reviewed drafts of the manuscript, and approved the final draft. All authors contributed to the article and approved the submitted version.
Funding
This work was supported by the Natural Sciences and Engineering Research Council of Canada (NSERC) through the Network of Centres of Excellence of Canada, ArcticNet, Amundsen Science (grant numbers 33108, 2014–2017 and 35560, 2017–2023) and Québec Océan (grant number 186795, 2015–2021).
Conflict of Interest
The authors declare that the research was conducted in the absence of any commercial or financial relationships that could be construed as a potential conflict of interest.
Acknowledgments
We would like to thank CCGS Amundsen officers and crew, scientists, technicians, and ROV operators V. Auger and P. Lockhart (Canadian Scientific Submersible Facility) for their support on board. We also thank V. De Carufel for her assistance during the field campaign, J. Gagnon for nutrient analyses, L. de Montety and L. Tréau de Coeli for taxonomy expertise, V. Ouisse and R. Belley for their expertise in benthic fluxes and D. Christiansen-Stowe and R. Amiraux for their comments and corrections. We would also like to thank our reviewers for their helpful comments. This is a contribution to the research programs of Québec-Océan and Takuvik (UMI 3376). This project was part of the HiBio program funded by ArcticNet.
Supplementary Material
The Supplementary Material for this article can be found online at: https://www.frontiersin.org/articles/10.3389/fmars.2020.00495/full#supplementary-material
References
Aller, R. C. (1988). “Benthic fauna and biogeochemical processes in marine sediments: the role of burrow structures,” in Nitrogen Cycling in Coastal Marine Environments, eds T. H. Blackburn and J. Sorensen (New York, NY: Wiley, John & Sons Ltd), 301–341.
Aller, R. C. (1994). Bioturbation and remineralization of sedimentary organic matter: effects of redox oscillation. Chem. Geol. 114, 331–345. doi: 10.1016/0009-2541(94)90062-0
Aller, R. C. (2001). “Chapter 11 - Transport and reactions in the bioirrigated zone,” in The Benthic Boundary Layer: Transport Processes and Biogeocahmistry, eds B. Boudreau and B. B. Jorgensen (Oxford: Oxford University Press), 269–301.
Aller, R. C., and Aller, J. Y. (1992). Meiofauna and solute transport in marine muds. Limnol. Oceanogr. 37, 1018–1033. doi: 10.4319/lo.1992.37.5.1018
Anschutz, P., Chaillou, G., and Lecroart, P. (2007). Phosphorus diagenesis in sediment of the Thau Lagoon. Estuar. Coast. Shelf Sci. 72, 447–456. doi: 10.1016/j.ecss.2006.11.012
Baillon, S., Hamel, J.-F., Wareham, V. E., and Mercier, A. (2012). Deep cold-water corals as nurseries for fish larvae. Front. Ecol. Environ. 10, 351–356. doi: 10.1890/120022
Barnhart, K. R., Miller, C. R., Overeem, I., and Kay, J. E. (2016). Mapping the future expansion of Arctic open water. Nat. Clim. Chang. 6, 280–285. doi: 10.1038/nclimate2848
Barrio Frojan, C. R. S., MacIsaac, K. G., McMillan, A. K., del Mar, Sacau Cuadrado, M., Large, P. A., et al. (2012). An evaluation of benthic community structure in and around the Sackville Spur closed area (Northwest Atlantic) in relation to the protection of vulnerable marine ecosystems. ICES J. Mar. Sci. 69, 213–222. doi: 10.1093/icesjms/fss004
Beazley, L., Kenchington, E., Yashayaev, I., and Murillo, F. J. (2015). Drivers of epibenthic megafaunal composition in the sponge grounds of the Sackville Spur, northwest Atlantic. Deep Res. Part I 98, 102–114. doi: 10.1016/j.dsr.2014.11.016
Bell, J. J. (2008). The functional roles of marine sponges. Estuar. Coast. Shelf Sci. 79, 341–353. doi: 10.1016/j.ecss.2008.05.002
Belley, R., and Snelgrove, P. V. R. (2016). Relative contributions of biodiversity and environment to benthic ecosystem functioning. Front. Mar. Sci. 3:242. doi: 10.3389/fmars.2016.00242
Belley, R., and Snelgrove, P. V. R. (2017). The role of infaunal functional and species diversity in short-term response of contrasting benthic communities to an experimental food pulse. J. Exp. Mar. Biol. Ecol. 491, 38–50. doi: 10.1016/j.jembe.2017.03.005
Belley, R., Snelgrove, P. V. R., Archambault, P., and Juniper, S. K. (2016). Environmental drivers of benthic flux variation and ecosystem functioning in Salish Sea and Northeast Pacific Sediments. PLoS One 11:e0151110. doi: 10.1371/journal.pone.0151110
Berner, R. A. (1980). Early Diagenesis: A Theoretical Approach. Princeton, NJ: University Press Princeton.
Bett, B. J., and Rice, A. L. (1992). The influence of hexactinellid sponge (Pheronema carpenteri) spicules on the patchy distribution of macrobenthos in the Porcupine Seabight (Bathyal NE Atlantic) T. Ophelia 36, 217–226. doi: 10.1080/00785326.1992.10430372
Bianucci, L., Fennel, K., and Denman, K. L. (2012). Role of sediment denitrification in water column oxygen dynamics: comparison of the North American East and West Coasts. Biogeosciences 9, 2673–2682. doi: 10.5194/bg-9-2673-2012
Biles, C. L., Paterson, D. M., Ford, R. B., Solan, M., and Raffaelli, D. G. (2002). Bioturbation, ecosystem functioning and community structure. Hydrol. Earth Syst. Sci. 6, 999–1005. doi: 10.5194/hess-6-999-2002
Blanchet, F. G., Legendre, P., and Borcard, D. (2008). Forward selection of explanatory variables. Ecology 89, 2623–2632. doi: 10.1890/07-0986.1
Bolam, S. G., Fernandes, T. F., and Huxham, M. (2002). Diversity, biomass, and ecosystem processes in the marine benthos. Ecol. Monogr. 72, 599–615. doi: 10.2307/3100059
Bourgeois, S., Archambault, P., and Witte, U. (2017). Organic matter remineralization in marine sediments: a Pan-Arctic synthesis. Glob. Biogeochem. Cycles 31, 190–213. doi: 10.1002/2016GB005378
Bourque, J. R., and Demopoulos, A. W. J. (2018). The influence of different deep-sea coral habitats on sediment macrofaunal community structure and function. PeerJ 6, 1–32. doi: 10.7717/peerj.5276
Bruno, J. F., and Kennedy, C. W. (2000). Patch-size dependent habitat modification and facilitation on New England cobble beaches by Spartina alterniflora. Oecologia 122, 98–108. doi: 10.1007/pl00008841
Buhl-Mortensen, L., Vanreusel, A., Gooday, A. J., Levin, L. A., Priede, I. G., Buhl-Mortensen, P., et al. (2010). Biological structures as a source of habitat heterogeneity and biodiversity on the deep ocean margins. Mar. Ecol. 31, 21–50.
Buhl-Mortensen, P., and Buhl-Mortensen, L. (2014). Diverse and vulnerable deep-water biotopes in the Hardangerfjord. Mar. Ecol. Prog. Ser. 10, 253–267. doi: 10.1080/17451000.2013.810759
Cathalot, C., Van Oevelen, D., Cox, T. J. S., Kutti, T., Lavaleye, M., Duineveld, G., et al. (2015). Cold-water coral reefs and adjacent sponge grounds: hotspots of benthic respiration and organic carbon cycling in the deep sea. Front. Mar. Sci. 2:37. doi: 10.3389/fmars.2015.00037
Cerrano, C., Danovaro, R., Gambi, C., Pusceddu, A., Riva, A., and Schiaparelli, S. (2010). Gold coral (Savalia savaglia) and gorgonian forests enhance benthic biodiversity and ecosystem functioning in the mesophotic zone. Biodivers. Conserv. 19, 153–167. doi: 10.1007/s10531-009-9712-5
Clough, L. M., Ambrose, W. G., Cochran, K. J., Barnes, C., Renaud, P. E., and Aller, R. C. (1997). Infaunal density, biomass and bioturbation in the sediments of the Arctic Ocean. Deep Res. Part II 44, 1683–1704. doi: 10.1016/s0967-0645(97)00052-0
Clough, L. M., Renaud, P. E., and Ambrose, W. G. Jr. (2005). Impacts of water depth, sediment pigment concentration, and benthic macrofaunal biomass on sediment oxygen demand in the western Arctic Ocean. Can. J. Fish. Aquat. Sci. 62, 1756–1765. doi: 10.1139/f05-102
Costello, M. J., McCrea, M., Freiwald, A., Lundalv, T., Jonsson, L., Bett, B. J., et al. (2005). “Role of cold-water Lophelia pertusa coral reefs as fish habitat in the NE Atlantic,” in Cold-Water Corals and Ecosystems, eds A. Freiwald and J. M. Roberts (Berlin: Springer-Verlag), 771–805. doi: 10.1007/3-540-27673-4_41
de Froe, E., Rovelli, L., Glud, R. N., Maier, S. R., Duineveld, G., Mienis, F., et al. (2019). Benthic oxygen and nitrogen exchange on a cold-water coral reef in the North-East Atlantic ocean. Front. Mar. Sci. 6:65. doi: 10.3389/fmars.2019.00665
Degen, R., Aune, M., Bluhm, B. A., Cassidy, C., Kêdra, M., Kraan, C., et al. (2018). Trait-based approaches in rapidly changing ecosystems: a roadmap to the future polar oceans. Ecol. Indic. 91, 722–736. doi: 10.1016/j.ecolind.2018.04.050
Demopoulos, A. W. J., Bourque, J. R., and Frometa, J. (2014). Biodiversity and community composition of sediment macrofauna associated with deep-sea Lophelia pertusa habitats in the Gulf of Mexico. Deep Res. Part I Oceanogr. Res. Pap. 93, 91–103. doi: 10.1016/j.dsr.2014.07.014
Dinn, C., Edinger, E., and Leys, S. P. (2019). Sponge (Porifera) fauna of Frobisher Bay, Baffin Island, Canada with the description of an Iophon rich sponge garden. Zootaxa 4576, 301–325. doi: 10.11646/zootaxa.4576.2.5
FAO (2009). International Guidelines for the Management of Deep-sea Fisheries in the High Seas. Rome: FAO.
Folk, R. L., and Ward, W. C. (1957). Brazos river bar: a study in the significance of grain size parameters. J. Sediment. Petrol. 27, 3–26. doi: 10.1306/74d70646-2b21-11d7-8648000102c1865d
Fosså, J. H., Mortensen, P. B., and Furevik, D. M. (2002). The deep-water coral Lophelia pertusa in Norwegian waters: distribution and fishery impacts. Hydrobiologia 471, 1–12.
Freiwald, A., Fosså, J. H., Grehan, A., Koslow, T., and Roberts, J. M. (2004). Cold-water Coral Reefs. Cambridge: UNEP-WCMC. doi: 10.1111/j.1439-0485.2010.00359.x
Gili, J. M., and Coma, R. (1998). Benthic suspension feeders: their paramount role in littoral marine food webs. Trends Ecol. Evol. 13, 316–321. doi: 10.1016/S0169-5347(98)01365-2
Goldsmit, J., McKindsey, C., Archambault, P., and Howland, K. L. (2019). Ecological risk assessment of predicted marine invasions in the Canadian Arctic. PLoS One 14:e0211815. doi: 10.1371/journal.pone.0211815
Grasshoff, K., Kremling, K., and Ehrhardt, M. (1999). Methods of Seawater Analyses. Weinheim, NY: Wiley-VCH.
Hartnett, H., Boehme, S., Thomas, C., DeMaster, D., and Smith, C. (2008). Benthic oxygen fluxes and denitrification rates from high-resolution porewater profiles from the Western Antarctic Peninsula continental shelf. Deep Res. Part II Top. Stud. Oceanogr. 55, 2415–2424. doi: 10.1016/j.dsr2.2008.06.002
Hensen, C., Zabel, M., and Schulz, H. N. (2006). “Early diagenesis at the benthic boundary layer: oxygen, nitrogen, and phosphorus in marine sediments,” in Marine Geochemistry, eds H. D. Schulz M. Zabel (Berlin: Springer), 207–240. doi: 10.1007/3-540-32144-6_6
Hiltz, E., Fuller, S. D., and Mitchell, J. (2018). Disko fan conservation area: a Canadian case study. Parks 24, 17–30. doi: 10.2305/IUCN.CH.2018.PARKS-24-SIEH.en
Hoegh-Guldberg, O., and Bruno, J. (2010). The impact of climate change on the world’s marine ecosystems. Science 328, 1523–1528. doi: 10.1126/science.1189930
Hoegh-Guldberg, O., Jacob, D., Taylor, M., Bindi, M., Brown, S., Camilloni, I., et al. (2018). “Impacts of 1.5o C global warming on natural and human systems,” in Global Warming of 1.5°C. An IPCC Special Report on the Impacts of Global Warming of 1.5°C above Pre-Industrial Levels and Related Global Greenhouse Gas Emission Pathways, in the Context of Strengthening the Global Response to the Threat of Climate Change, eds V. Masson-Delmotte, P. Zhai, H.-O. Pörtner, D. Roberts, J. Skea, P. R. Shukla, et al. (Geneva: World Meteorological Organization), 175–311. doi: 10.1002/ejoc.201200111
Holmes, R. M., Aminot, A., Kérouel, R., Hooker, B. A., and Peterson, B. J. (1999). A simple and precise method for measuring ammonium and marine and freshwater. Can. J. Fish. Aquat. Sci. 56, 1801–1808. doi: 10.1139/f99-128
Hotelling, H. (1933). Analysis of a complex of statistical variables into principal components. J. Educ. Psychol. 24, 498–520. doi: 10.1037/h0070888
Huettel, M., Berg, P., and Kostka, J. E. (2014). Benthic exchange and biogeochemical cycling in permeable sediments. Ann. Rev. Mar. Sci. 6, 23–51. doi: 10.1146/annurev-marine-051413-012706
Husson, F., Josse, J., Le, S., and Mazet, J. (2020). Package ‘ FactoMineR.’. Available online at: https://cran.r-project.org/web/packages/FactoMineR/FactoMineR.pdf (accessed January 12, 2020).
Ingels, J., Kiriakoulakis, K., Wolff, G. A., and Vanreusel, A. (2009). Nematode diversity and its relation to the quantity and quality of sedimentary organic matter in the deep Nazaré Canyon, Western Iberian Margin. Deep Res. Part I 56, 1521–1539. doi: 10.1016/j.dsr.2009.04.010
Janssen, F., Huettel, M., and Witte, U. (2005). Pore-water advection and solute fluxes in permeable marine sediments (II): benthic respiration at three sandy sites with different permeabilities (German Bight, North Sea). Limnol. Oceanogr. 50, 779–792. doi: 10.4319/lo.2005.50.3.0779
Jensen, H. S., Mortensen, P. B., Andersen, F., Rasmussen, E., and Jensen, A. (1995). Phosphorus cycling in a coastal marine sediment, Aarhus Bay, Denmark. Limnol. Oceanogr. 40, 908–917. doi: 10.4319/lo.1995.40.5.0908
Jones, C. G., Lawton, J. H., and Shachak, M. (1994). Organisms as ecosystem engineers. Oikos 69, 373–386. doi: 10.2307/3545850
Kenchington, E., Lirette, C., Cogswell, A., Archambault, D., Archaumbault, P., Benoit, H., et al. (2010). Delineating coral and sponge concentrations in the biogeographic regions of the east coast of canada using spatial analyses. DFO Can. Sci. Advis. Sec. Res. Doc. 2010/041. vi + 202.
Kenchington, E. L., Link, H., Roy, V., Archambault, P., Siferd, T., Treble, M., et al. (2011). Identification of mega- and macrobenthic ecologically and biologically significant areas (EBSAs) in the hudson bay complex, the western and eastern canadian arctic. DFO Can. Sci. Advis. Sec. Res. Doc. 2011/071.vi + 52.
Kersken, D., Göcke, C., Brandt, A., Lejzerowicz, F., Schwabe, E., Seefeldt, M. A., et al. (2014). The infauna of three widely distributed sponge species (Hexactinellida and Demospongiae) from the deep Ekström Shelf in the Weddell Sea, Antarctica. Deep Res. Part II Top. Stud. Oceanogr. 108, 101–112. doi: 10.1016/j.dsr2.2014.06.005
Khripounoff, A., Caprais, J.-C., Le Bruchec, J., Rodier, P., Noel, P., and Cathalot, C. (2014). Deep cold-water coral ecosystems in the Brittany submarine canyons (Northeast Atlantic): hydrodynamics, particle supply, respiration, and carbon cycling. Limnol. Ocean 59, 87–98. doi: 10.4319/lo.2014.59.01.0087
Kim, S. H., Choi, A., Jin Yang, E., Lee, S. H., and Hyun, J. H. (2016). Low benthic respiration and nutrient flux at the highly productive Amundsen Sea Polynya, Antarctica. Deep Res. Part II Top. Stud. Oceanogr. 123, 92–101. doi: 10.1016/j.dsr2.2015.10.004
Kristensen, E., Penha-Lopes, G., Delefosse, M., Valdemarsen, T., Quintana, C. O., and Banta, G. T. (2012). What is bioturbation? the need for a precise definition for fauna in aquatic sciences. Mar. Ecol. Prog. Ser. 446, 285–302. doi: 10.3354/meps09506
Laliberté, E., and Legendre, P. (2010). A distance-based framework for measuring functional diversity from multiple traits. Ecology 91, 299–305. doi: 10.1890/08-2244.1
Laliberté, E., Legendre, P., and Shipley, B. (2014). MeasuringFunctional Diversity (FD) from Multiple Traits, and Other Tools for Functional Ecology. Package’FD’. Available online at: http://cran.r-project.org/web/packages/FD/FD.pdf (accessed February 17, 2019).
Lavorel, S., Grigulis, K., Mcintyre, S., Williams, N. S. G., Garden, D., Dorrough, J., et al. (2008). Assessing functional diversity in the field – methodology matters! Funct. Ecol. 22, 134–147. doi: 10.1111/j.1365-2435.2007.01339.x
Levin, L. A., Etter, R. J., Rex, M. A., Gooday, A. J., Smith, C. R., Pineda, J., et al. (2001). Environmental influences on regional deep-sea species diversity. Annu. Rev. Ecol. Evol. Syst. 32, 51–93. doi: 10.1146/annurev.ecolsys.32.081501.114002
Link, H., Archambault, P., Tamelander, T., Renaud, P. E., and Piepenburg, D. (2011). Spring-to-summer changes and regional variability of benthic processes in the western Canadian Arctic. Polar Biol. 34, 2025–2038. doi: 10.1007/s00300-011-1046-6
Link, H., Chaillou, G., Forest, A., Piepenburg, D., and Archambault, P. (2013a). Multivariate benthic ecosystem functioning in the Arctic – benthic fluxes explained by environmental parameters in the southeastern Beaufort Sea. Biogeosciences 10, 5911–5929. doi: 10.5194/bg-10-5911-2013
Link, H., Piepenburg, D., and Archambault, P. (2013b). Are hotspots always hotspots? The relationship between diversity, resource and ecosystem functions in the Arctic. PLoS One 8:e74077. doi: 10.1371/journal.pone.0074077
Macdonald, T. A., Burd, B. J., Macdonald, V. I., and van Roodselaar, A. (2010). Taxonomic and feeding guild classification for the marine benthic macroinvertebrates of the strait of Georgia, British Columbia. Can. Tech. Rep. Fish. Aquat. Sci. 2874, 4–63.
Melia, N., Haines, K., and Hawkins, E. (2016). Sea ice decline and 21st century trans-Arctic shipping routes. Geophys. Res. Lett. 48, 9720–9728. doi: 10.1002/2016GL069315
Mermillod-Blondin, F., Rosenberg, R., François-Carcaillet, F., Norling, K., and Mauclaire, L. (2004). Influence of bioturbation by three benthic infaunal species on microbial communities and biogeochemical processes in marine sediment. Aquat. Microb. Ecol. 36, 271–284. doi: 10.3354/ame036271
Michaud, E., Desrosiers, G., Aller, R. C., Mermillod-Blondin, F., Sundby, B., and Stora, G. (2009). Spatial interactions in the Macoma balthica community control biogeochemical fluxes at the sediment-water interface and microbial abundances. J. Mar. Res. 67, 43–70. doi: 10.1357/002224009788597926
Michaud, E., Desrosiers, G., Mermillod-Blondin, F., Sundby, B., and Stora, G. (2006). The functional group approach to bioturbation: II. The effects of the Macoma balthica community on fluxes of nutrients and dissolved organic carbon across the sediment-water interface. J. Exp. Mar. Biol. Ecol. 337, 178–189. doi: 10.1016/j.jembe.2006.06.025
Mienis, F., de Stigter, H. C., de Haas, H., and van Weering, T. C. E. (2009). Near-bed particle deposition and resuspension in a cold-water coral mound area at the Southwest Rockall Trough margin, NE Atlantic. Deep Res. Part I 56, 1026–1038. doi: 10.1016/j.dsr.2009.01.006
Mouret, A., Anschutz, P., Deflandre, B., Chaillou, G., Hyacinthe, C., Deborde, J., et al. (2010). Oxygen and organic carbon fluxes in sediments of the Bay of Biscay. Deep Res. Part I Oceanogr. Res. Pap. 57, 528–540. doi: 10.1016/j.dsr.2009.12.009
Neves, B. D. M., Edinger, E., Hillaire-Marcel, C., Saucier, E. H., France, S. C., Treble, M. A., et al. (2015). Deep-water bamboo coral forests in a muddy Arctic environment. Mar. Biodivers. 45, 867–871. doi: 10.1007/s12526-014-0291-7
Oksanen, J., Blanchet, F. G., Friendly, M., Kindt, R., Legendre, P., Mcglinn, D., et al. (2017). Vegan: Community Ecology Package. R Package Vegan, vers. 2.4-2. Available online at: https://CRAN.R-project.org/package=vegan (accessed January 11, 2019).
Piepenburg, D., Blackburn, T. H., VonDorrien, C. F., Gutt, J., Hall, P. O., Hulth, S., et al. (1995). Partitioning of benthic community respiration in the Arctic (northwestern Barents Sea). Mar. Ecol. Prog. Ser. 118, 199–214. doi: 10.3354/meps118199
Pike, J., Bernhard, J. M., Moreton, S. G., and Butler, I. B. (2001). Microbioirrigation of marine sediments in dysoxic environments: implications for early sediment fabric formation and diagenetic processes. Geology 29, 923–926.
Piot, A., Nozais, C., and Archambault, P. (2013). Meiofauna affect the macrobenthic biodiversity – ecosystem functioning relationship. Oikos 123, 203–213. doi: 10.1111/j.1600-0706.2013.00631.x
Przeslawski, R., McArthur, M. A., and Anderson, T. J. (2013). Infaunal biodiversity patterns from Carnarvon Shelf (Ningaloo Reef), Western Australia. Mar. Freshw. Res. 64:573. doi: 10.1071/mf12240
Pusceddu, A., Bianchelli, S., Martín, J., Puig, P., Palanques, A., Masqué, P., et al. (2014). Chronic and intensive bottom trawling impairs deep-sea biodiversity and ecosystem functioning. Proc. Natl. Acad. Sci. U.S.A. 111, 8861–8866. doi: 10.1073/pnas.1405454111
Queirós, A. M., Birchenough, S. N. R., Bremner, J., Godbold, J. A., Parker, R. E., Romero-Ramirez, A., et al. (2013). A bioturbation classification of European marine infaunal invertebrates. Ecol. Evol. 3, 3958–3985. doi: 10.1002/ece3.769
R Core Team (2018). R: A Language and Environment for Statistical Computing. Available online at: https://www.r-project.org/
Rao, A. M. F., Polerecky, L., Ionescu, D., Meysman, F. J. R., and de Beer, D. (2012). The influence of pore-water advection, benthic photosynthesis, and respiration on calcium carbonate dynamics in reef sands. Limnol. Oceanogr. 57, 809–825. doi: 10.4319/lo.2012.57.3.0809
Rao, C. R. (1964). The use and interpretation of principal component analysis in applied research. Sankhya Indian J. Stat. 26, 329–358.
Renaud, P. E., Morata, N., Ambrose, W. G. Jr., Bowie, J. J., and Chiuchiolo, A. (2007). Carbon cycling by seafloor communities on the eastern Beaufort Sea shelf. J. Exp. Mar. Bio. Ecol. 349, 248–260. doi: 10.1016/j.jembe.2007.05.021
Riaux-Gobin, C., and Klein, B. (1993). “Microphytobenthic biomass measurement using HPLC and conventional pigment analysis,” in Aquatic Microbial Ecology, eds P. F. Kemp, B. F. Sherr, E. B. Sherr, and J. J. Cole (Boca Raton, FL: Lewis Publishers), 369–376. doi: 10.1201/9780203752746-43
Roberts, J. M., and Cairns, S. D. (2014). Cold-water corals in a changing ocean. Curr. Opin. Environ. Sustain. 7, 118–126. doi: 10.1016/j.cosust.2014.01.004
Rossi, S. (2013). The destruction of the ‘ animal forests ’ in the oceans: towards an over- simplification of the benthic ecosystems. Ocean Coast. Manag. 84, 77–85. doi: 10.1016/j.ocecoaman.2013.07.004
Rossi, S., Bramanti, L., Broglio, E., and Gili, J. M. (2012). Trophic impact of long-lived species indicated by population dynamics in the short-lived hydrozoan Eudendrium racemosum. Mar. Ecol. Prog. Ser. 467, 97–111. doi: 10.3354/meps09848
Rossi, S., Gili, J. M., and Garrofé, X. (2011). Net negative growth detected in a population of Leptogorgia sarmentosa: quantifying the biomass loss in a benthic soft bottom-gravel gorgonian. Mar. Biol. 158, 1631–1643. doi: 10.1007/s00227-011-1675-x
Rysgaard, S., Glud, R. N., Risgaard-Petersen, N., and Dalsgaard, T. (2004). Denitrification and anammox activity in Arctic marine sediments. Limnol. Oceanogr. 49, 1493–1502. doi: 10.4319/lo.2004.49.5.1493
Secretariat of the Convention on Biological Diversity (2014). Global Biodiversity Outlook 4. Gland: IUCN.
Snelgrove, P. V. R., Thrush, S. F., Wall, D. H., and Norkko, A. (2014). Real world biodiversity – ecosystem functioning: a seafloor perspective. Trends Ecol. Evol. 29, 398–405. doi: 10.1016/j.tree.2014.05.002
Soetaert, K., Vanaverbeke, J., Heip, C., Herman, P. M. J., Middelburg, J. J., Sandee, A., et al. (1997). Nematode distribution in ocean margin sediments of the Goban Spur (northeast Atlantic) in relation to sediment geochemistry. Deep Res. Part I 44, 1671–1683. doi: 10.1016/s0967-0637(97)00043-5
Sundby, B., Gobeil, C., Silverberg, N., and Mucci, A. (1992). The phosphorus cycle in coastal marine sediments. Limnol. Oceanogr. 37, 1129–1145. doi: 10.1016/S0074-6142(08)62697-2
Thrush, S. F., and Dayton, P. K. (2002). Disturbance to marine benthic habitats by trawling and dredging: implications for marine biodiversity. Annu. Rev. Ecol. Syst. 33, 449–473. doi: 10.1146/annurev.ecolsys.33.010802.150515
Tremblay, J. É., Anderson, L. G., Matrai, P., Coupel, P., Bélanger, S., Michel, C., et al. (2015). Global and regional drivers of nutrient supply, primary production and CO2 drawdown in the changing Arctic Ocean. Prog. Oceanogr. 139, 171–196. doi: 10.1016/j.pocean.2015.08.009
Van Oevelen, D., Duineveld, G., Lavaleye, M., Mienis, F., Soetaert, K., and Heip, C. H. R. (2009). The cold-water coral community as hotspot of carbon cycling on continental margins: a food-web analysis from Rockall Bank (northeast Atlantic). Limnol. Oceanogr. 54, 1829–1844. doi: 10.4319/lo.2009.54.6.1829
Villéger, S., Mason, N. W. H., and Mouillot, D. (2008). New multidimensional functional diversity indices for a multifaceted framework in functional ecology. Ecology 89, 2290–2301. doi: 10.1890/07-1206.1
Vogel, S. (1977). Current induced through living sponges in nature. Proc. Natl. Acad. Sci. U.S.A. 74, 2069–2071. doi: 10.1073/pnas.74.5.2069
Vopel, K., Thistle, D., and Rosenberg, R. (2003). Effect of the brittle star Amphiura filiformis (Amphiuridae, Echinodermata) on oxygen flux into the sediment. Limnol. Ocean 48, 2034–2045. doi: 10.4319/lo.2003.48.5.2034
Wassmann, P., and Reigstad, M. (2011). Future Arctic Ocean seasonal ice zones and implications for pelagic-benthic coupling. Oceanography 24, 220–231. doi: 10.5670/oceanog.2011.74
Wentworth, C. K. (1922). A scale of grade and class terms for clastic sediments. J. Geol. 30, 377–392. doi: 10.1086/622910
Keywords: biogenic structures, infaunal community, nutrient fluxes, functional traits, Arctic
Citation: Pierrejean M, Grant C, Neves BM, Chaillou G, Edinger E, Blanchet FG, Maps F, Nozais C and Archambault P (2020) Influence of Deep-Water Corals and Sponge Gardens on Infaunal Community Composition and Ecosystem Functioning in the Eastern Canadian Arctic. Front. Mar. Sci. 7:495. doi: 10.3389/fmars.2020.00495
Received: 29 May 2019; Accepted: 03 June 2020;
Published: 30 June 2020.
Edited by:
Susana Carvalho, King Abdullah University of Science and Technology, Saudi ArabiaReviewed by:
Sandra Maier, Royal Netherlands Institute for Sea Research (NIOZ), NetherlandsLenaick Menot, Institut Français de Recherche pour l’Exploitation de la Mer (IFREMER), France
Marina R. Cunha, University of Aveiro, Portugal
Copyright © 2020 Pierrejean, Grant, Neves, Chaillou, Edinger, Blanchet, Maps, Nozais and Archambault. This is an open-access article distributed under the terms of the Creative Commons Attribution License (CC BY). The use, distribution or reproduction in other forums is permitted, provided the original author(s) and the copyright owner(s) are credited and that the original publication in this journal is cited, in accordance with accepted academic practice. No use, distribution or reproduction is permitted which does not comply with these terms.
*Correspondence: Marie Pierrejean, bWFyaWUucGllcnJlamVhbi4xQHVsYXZhbC5jYQ==