- 1Key Laboratory of Tropical Marine Bio-resources and Ecology, South China Sea Institute of Oceanology, Chinese Academy of Sciences, Guangzhou, China
- 2Guangdong Provincial Key Laboratory of Applied Marine Biology, South China Sea Institute of Oceanology, Chinese Academy of Sciences, Guangzhou, China
- 3Innovation Academy of South China Sea Ecology and Environmental Engineering, South China Sea Institute of Oceanology, Chinese Academy of Sciences, Guangzhou, China
- 4Hainan Tropical Marine Biological Research Station, South China Sea Institute of Oceanology, Chinese Academy of Sciences, Sanya, China
- 5Hainan Key Laboratory of Tropical Marine Biotechnology, Sanya, China
- 6University of Chinese Academy of Sciences, Beijing, China
In the foreseeable future, coastal coral reef ecosystems are likely to face further increases in eutrophication. Lipids and fatty acids (FAs), as important components of corals, are becoming a hot topic to study the effects of eutrophication on corals. This study investigated the effects of nitrate (NO3–) enrichment (0, 5, 10, 20, and 40 μM) on the respiration, photosynthesis, and FA compositions of Pocillopora damicornis larvae. Our results showed that saturated FAs (SFAs) were the most abundant in P. damicornis larvae over all treatment groups, followed by polyunsaturated FAs (PUFAs). The unsaturated-to-SFA ratio (U/S) and unsaturation index (UI) reduced at low nitrate concentrations (<10 μM), since the level of SFAs (mainly 16:0 and 18:0) increased whereas PUFAs (mainly 18:3n3, 20:3n6, and 22:6n3) decreased. Consequently, the biomembranes of the larvae may have become more rigid and viscous, which slowed excessive nitrate entry. Moreover, significantly enhanced photosynthetic functions of zooxanthellae in larvae were found in the N5 group (5 μM). However, the opposite FA patterns were observed in P. damicornis larvae at higher nitrate concentrations (>20 μM). The UI and U/S levels were elevated due to the increased PUFAs levels and decreased SFA levels at higher nitrate concentrations. Compared with the N5 and N10 groups, the fluidity of the biomembrane of the larvae did not continue to decrease but instead increased at higher nitrate concentrations, indicating that the biomembrane restructuring in the larvae may have become ineffective. Moreover, respiration increased and the consumption of numerous lipids led to a significant decrease in TFAs. These could adversely affect the dispersal, settlement, and development of larvae. Overall, P. damicornis larvae can adapt to low levels of nitrate (<10 μM) due to biomembrane restructuring through changes in FA composition. However, negative effects occur in larvae when nitrate exceeds 20 μM.
Introduction
Reef ecosystems are among the world’s most productive and biodiverse marine ecosystems (Hughes et al., 2018; Kubicek et al., 2019). Scleractinian corals serve as the framework for these ecosystems (Zaneveld et al., 2016). Coral reef waters usually contain low levels of inorganic nutrients (Renegar and Riegl, 2005). However, increased human population densities, the use of chemical fertilizers in agriculture, and domestic sewage discharge could significantly increase the input of nutrients into coastal zones (D’Angelo and Wiedenmann, 2014; Serrano et al., 2018). For the foreseeable future, coastal coral reef ecosystems are likely to face further increases in eutrophication (Humanes et al., 2016). The effect of nutrients on corals and their algal symbionts is still subject to debate. Some researchers have suggested that elevated nutrient levels can significantly decrease the growth and calcification of coral (Ferrierpagès et al., 2000; Renegar and Riegl, 2005). However, positive responses in coral to increased nutrient availability have also been found, including reduced thermal stress, zooxanthellae supplementation, and moderate bleaching (Atkinson et al., 1995; Bender-Champ et al., 2017).
Lipids and fatty acids (FAs) are the primary constituents of the cells and subcellular organelle membranes in marine organisms. They are vital structural components of biomembranes that adapt to variations in environmental conditions by changing their FA composition (Sinensky, 1974; Hazel, 1979; Wijekoon, 2011; Bennett et al., 2018). This process, called homeoviscous adaptation, can affect membrane-associated physical attributes and biological functions such as fluidity, phase behavior, thickness, permeability, and related enzymes (Yeagle, 1989; Ernst et al., 2016; Bennett et al., 2018). Lipids represent a major component of the coral composition (10–40% of dry biomass in adult corals and 34–85% of dry biomass in larvae) (Bhojoo et al., 2017; Conlan et al., 2017). Although most endogenous lipids and FAs in coral are provided by CO2 fixation of zooxanthellae, corals also modify these lipids and FAs according to their own needs for development, reproduction, and adaptation to environmental change. Lipids and FAs are becoming a hot topic to study the effects of environmental changes on corals.
Successful dispersal, settlement, and development of larvae are critical for the maintenance of coral populations (Figueiredo et al., 2012; Jiang et al., 2019). Many physiological characteristics of coral larvae (e.g., immature cellular defenses and smaller biomass) may render them more susceptible than adult corals when exposed to the same stressors (Jiang et al., 2017; Serrano et al., 2018). However, there are few studies on the effects of nutrient enrichment on the early life stages of coral. The limited data available suggest that nitrate enrichment significantly affects performance (such as survival, growth, and settlement) (Ward and Harrison, 2000; Harrison and Ward, 2001; Lam et al., 2015; Humanes et al., 2016) and metabolism (Serrano et al., 2018). To our knowledge, no studies have yet investigated the effects of nitrate enrichment on lipids and FA composition of coral larvae.
Sanya (Hainan Province, China) is a typical tourist city, and the coastal region around it suffers from eutrophication, especially in terms of nitrates. Indeed, the nutrient levels have become significantly elevated with the rapid development of tourism and increasing population (Jing et al., 2017). Moreover, Pocillopora damicornis is widely distributed in this region and functions as a major hermatypic coral on reef flats. The present study assessed the respiration, photosynthesis, and FA composition of P. damicornis larvae with different nitrate concentrations and explored the tolerance and acclimation mechanism of P. damicornis larvae to nitrate enrichment from the perspective of biomembrane restructuring. These findings could provide a theoretical basis to evaluate and predict the recruitment and community reassembly of P. damicornis in coastal regions, especially in eutrophic areas.
Materials and Methods
Coral Sampling and Larvae Collection
On 18 August 2018, 10 adult colonies of P. damicornis were collected from Luhuitou Fringing Reef (N18°12.7’, E109°28.5’) at depths of 2–3 m. The colonies were transported to the Tropical Marine Biological Research Station and acclimated for 24 h in fiberglass cylindrical tanks, then placed individually into 18 L flow-through tanks at ambient temperature (28.7 ± 0.5°C) under partially shaded conditions (noon irradiance, ∼300 μmol photonsm–2s–1). The outflow of each tank was passed through a cup fitted with a 180-μm net on the bottom to trap larvae. Larvae released from these colonies were collected at 07:00 on 19 August 2018 and then pooled.
Experimental Design
Laboratory experiments were performed to study the effects of nitrate enrichment on P. damicornis larvae. Five different treatments were established: a control group (N0) and the N5, N10, N20, and N40 groups (nitrate concentrations for each treatment are shown in Table 1). The P. damicornis larvae were stocked at a density of 300 per plastic tank (500 mL; 10.0 cm height × 10.0 cm diameter). The experiment was run for 5 days, and each treatment had three replicates. The larvae in the control group were treated with 0.5-μm-filtered seawater, and the other four nitrate-enrichment treatments involved seawater with different KNO3 levels. The temperature was maintained at 29 ± 0.5°C, and a series of full-spectrum fluorescent bulbs (Giesemann, Nettetal, Germany) was used for irradiance (∼300 μmol photonsm–2s–1, photoperiod 12:12).
Respiration and Photosynthesis
Dark respiration (RD) and net photosynthesis (PNet) of the larvae were measured after the fifth day of the experiment. A miniature stir bar (3 × 5 mm) and 20 larvae randomly sampled from each tank were transferred into a 2-mL glass vial with an oxygen optical sensing patch on the inside wall. The filtered seawater in the vial was from the corresponding experimental tank. After turning on the magnetic stirrer (300 r/min) and dissolved oxygen meter (OXY-4 mini; Presens, Regensburg, Germany), we continuously recorded the oxygen concentration at 10-s intervals over 10 min under the same illumination as the experiment. Then, PNet was calculated using the least squares linear regression of the oxygen concentration plotted against time and expressed as nanomoles of oxygen per min per larvae. RD was measured after 2 h dark adaptation. Except that the measurement of RD was taken with no light, the details and methods were the same as those for the PNet measurements. Two additional vials containing only filtered seawater were run as the blank control for each treatment, and the results showed that the background respiration was negligible. The ratios of PNet to RD (PNet/RD) were calculated to assess autotrophic capacity (Muscatine et al., 1981). A PNet/RD ratio > 1 indicated that the net organic carbon fixed by photosynthesis of symbiotic zooxanthellae was enough to sustain the consumption of respiration. Otherwise, it implied that the endogenous reserves of the larvae were consumed.
Lipid Extraction and FA Analysis
Approximately 500 larvae (0.1 g) were collected from each group and analyzed (n = 3). The samples was homogenized, and each lipid fraction was extracted using chloroform/methanol (2:1, v/v) containing 0.01% butylated hydroxytoluene as an antioxidant, as previously described by Liu et al. (2019). The chloroform layer was separated from the methanol layer and dried to a constant weight under a stream of nitrogen to obtain lipids. FA methyl esters (FAMEs) were obtained by esterification with 2 mL methyl esterification reagent (hydrochloric acid/methanol, 1:5, v/v) at 90°C for 3 h, as described by Liu et al. (2018). The upper phase was dried under nitrogen and resuspended in hexane.
Fatty acid methyl esters were quantified by injecting 1 μL of sample into a gas chromatograph (GC-2010 Plus; Shimadzu, Kyoto, Japan) equipped with a flame-ionization detector (GC-2010; Shimadzu) and an RTX-WAX fused-silica capillary column (length, 30 m; internal diameter, 0.25 mm; thickness, 0.25 μm; Phenomenex, Torrance, CA, United States). The gradient temperature program was set as follows: (i) initial temperature of 60°C for 1.0 min; (ii) increase at a rate of 10°Cmin–1 to 190°C, (iii) increase at 2.0°Cmin–1 to 260°C; (iv) hold at 260°C for 0.6 min. FAME identification and quantification were performed by comparing the retention times (identification) and peak areas (quantification) with 37-FAME Mix calibration solution (Supelco, Bellefonte, PA, United States).
Statistical Analyses
SAS statistical software version 9.4 (SAS Institute, Cary, NC, United States) was used for statistical analyses. The distribution of data was evaluated for normality with the Kolmogorov–Smirnov test (P > 0.05). The Levene’s test (P > 0.05) was used to assess the homogeneity of variance. All data were then evaluated by one-way analysis of variance, followed by the Student–Newman–Keuls multiple-comparisons test to identify significant differences (P < 0.05) between the means of the different treatment groups. Moreover, principal component analysis (PCA) was used to display significant differences in FA profiles among the five treatment groups.
To facilitate comparison of the FA compositions, we calculated the unsaturation index (UI) and the unsaturated-to-saturated FA ratio (U/S) as reported by Wallaert and Babin (1994) and Snyder and Hennessey (2003). The UI and U/S algorithms were as follows:
where monoenes, dienes, trienes… are FAs containing 1, 2, 3… double bonds, respectively; %: weight percentage; UFA: unsaturated FAs; SFA: saturated FAs.
Results
Respiration and Photosynthesis of P. damicornis Larvae With Nitrate Enrichment
The PNet of P. damicornis larvae showed an initial increase and then a decrease with increasing nitrate concentration (Figure 1A). The highest PNet (0.2180 nmol O2 larvae–1 min–1) was observed in the N5 group, which was more than double that of the control group (N0, 0.1012 nmol O2 larvae–1 min–1). Subsequently, PNet decreased with increasing nitrate concentration, and the lowest PNet was observed in N40 (0.0726 nmol O2 larvae–1 min–1). Although the RD showed no change (p > 0.05) at nitrate concentrations ≤ 10 μM (N0, N5, and N10 groups), it significantly increased (p < 0.05) in the N20 and N40 groups (Figure 1B). The ratio of PNet/RD ranged from 0.4151 to 1.4724 (Figure 1C). The PNet/RD showed similar changes to those of PNet and, except for N5, PNet/RD of the treatment groups were less than 1. The lowest PNet/RD was in the N40 group (0.4151).
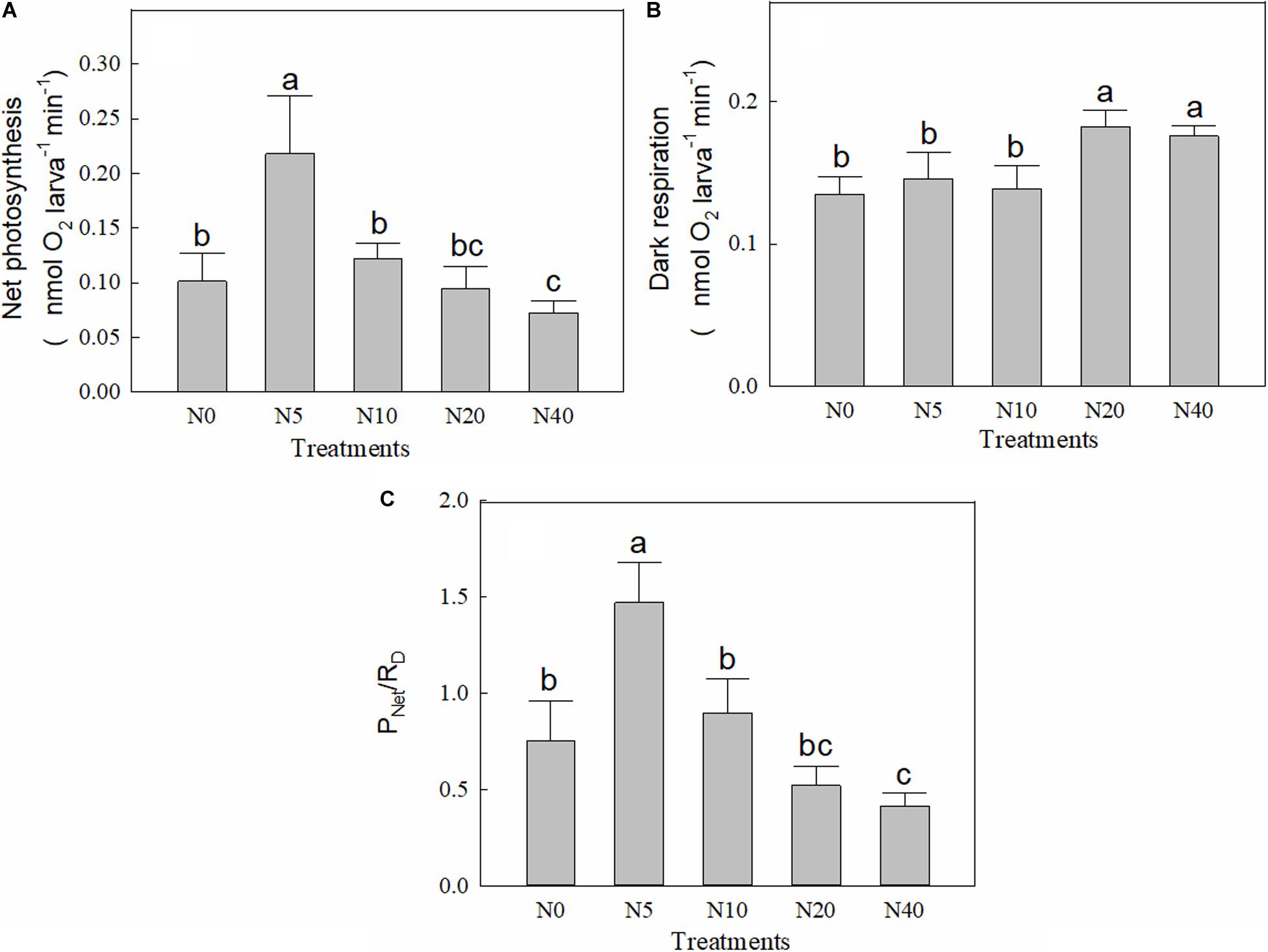
Figure 1. Net photosynthesis (A), dark respiration (B), ratio of photosynthesis to respiration (C) of P. damicornis larvae in each treatment.
Total Fatty Acids (TFAs) of P. damicornis Larvae
The TFA levels present in the P. damicornis larvae after each treatment are shown in Table 2. When the nitrate concentration was less than 10 μM (N0, N5, and N10 groups), the TFA levels in P. damicornis larvae were not significantly different (p > 0.05). When the nitrate concentration was further elevated, the TFAs significantly decreased (p < 0.05). The lowest TFA levels (45.87 μg/g) were observed when the nitrate concentration was 40 μM (N40 group), and were less than half those in the control group.

Table 2. Total fatty acids of Pocillopora damicornis larvae with different nitrate concentrations (μg/g).
FA Compositions of P. damicornis Larvae
Twenty-three FA species were identified among the P. damicornis larvae, including nine SFAs, eight monounsaturated FAs (MUFAs), and nine polyunsaturated FAs (PUFAs). Seven major FAs were recurrently found in P. damicornis larvae, including palmitic acid (16:0), stearic acid (18:0), heneicosanoic acid (21:0), oleic acid (18:1n9), palmitoleic acid (16:1n7), EPA (20:5n3), and DHA (22:6n3).
The P. damicornis larvae had the highest abundance of SFAs (more than 60%), followed by PUFAs (approximately 30%), and the lowest abundance of MUFAs (only 10%). The predominant SFA, MUFA, and PUFA were 16:0, 16:1n7, and 22:6n3, respectively. Moreover, the UI ranged between 1.35 and 1.07, and the U/S ratio ranged between 0.65 and 0.51. The maximum and minimum UI and U/S levels occurred in the N5 and N0 groups, respectively.
FA Composition Change in P. damicornis Larvae With Nitrate Enrichment
The FA profiles of the P. damicornis larvae with different nitrate enrichments are shown in Table 3 and Figure 2. To better visualize the FA changes in the P. damicornis larvae with different nitrate concentrations, six line charts for UI, U/S, SFA, MUFA, and PUFA are presented in Figure 3.
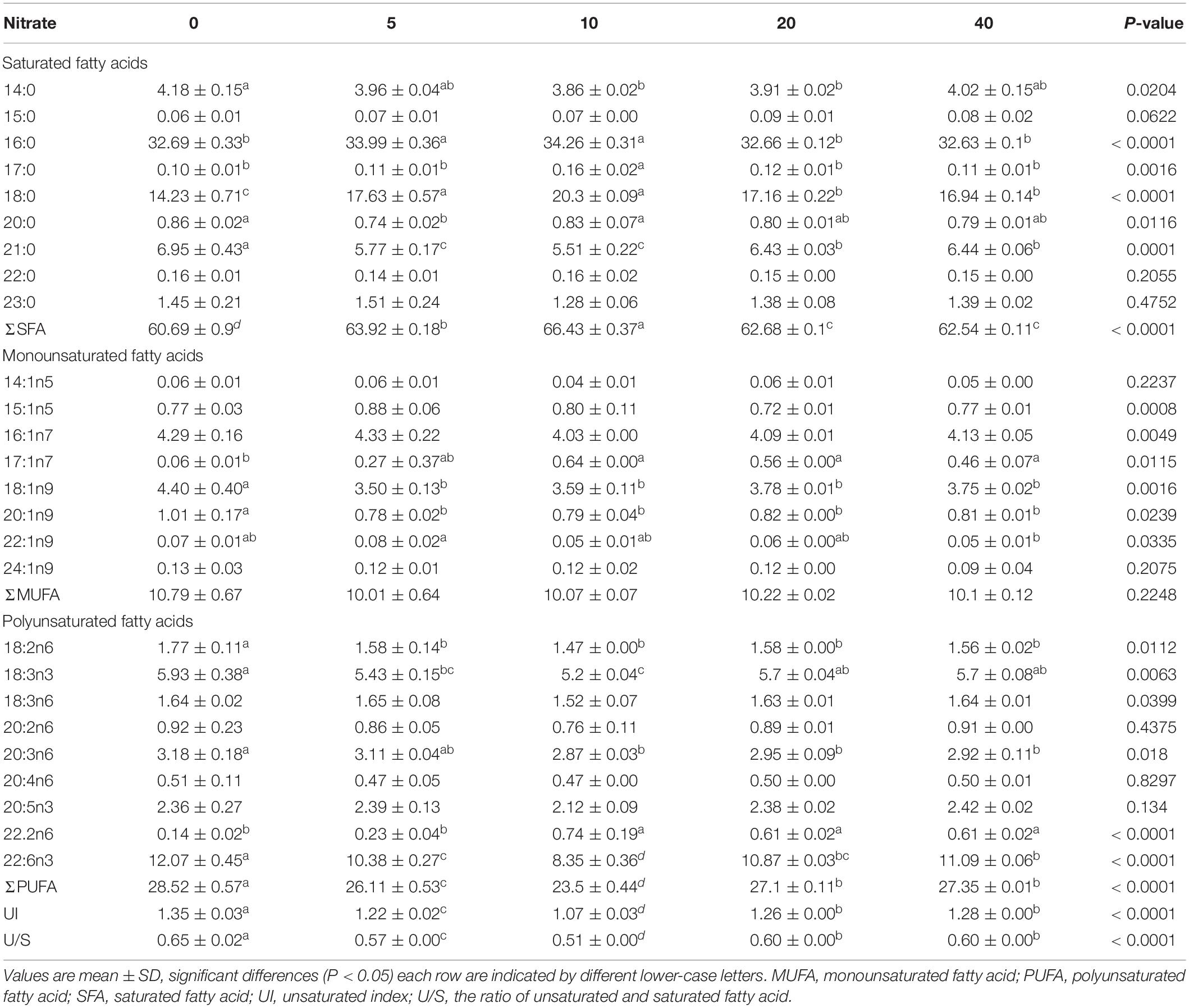
Table 3. Fatty acid compositions (% total fatty acids) of P. damicornis larvae with different nitrate concentrations.
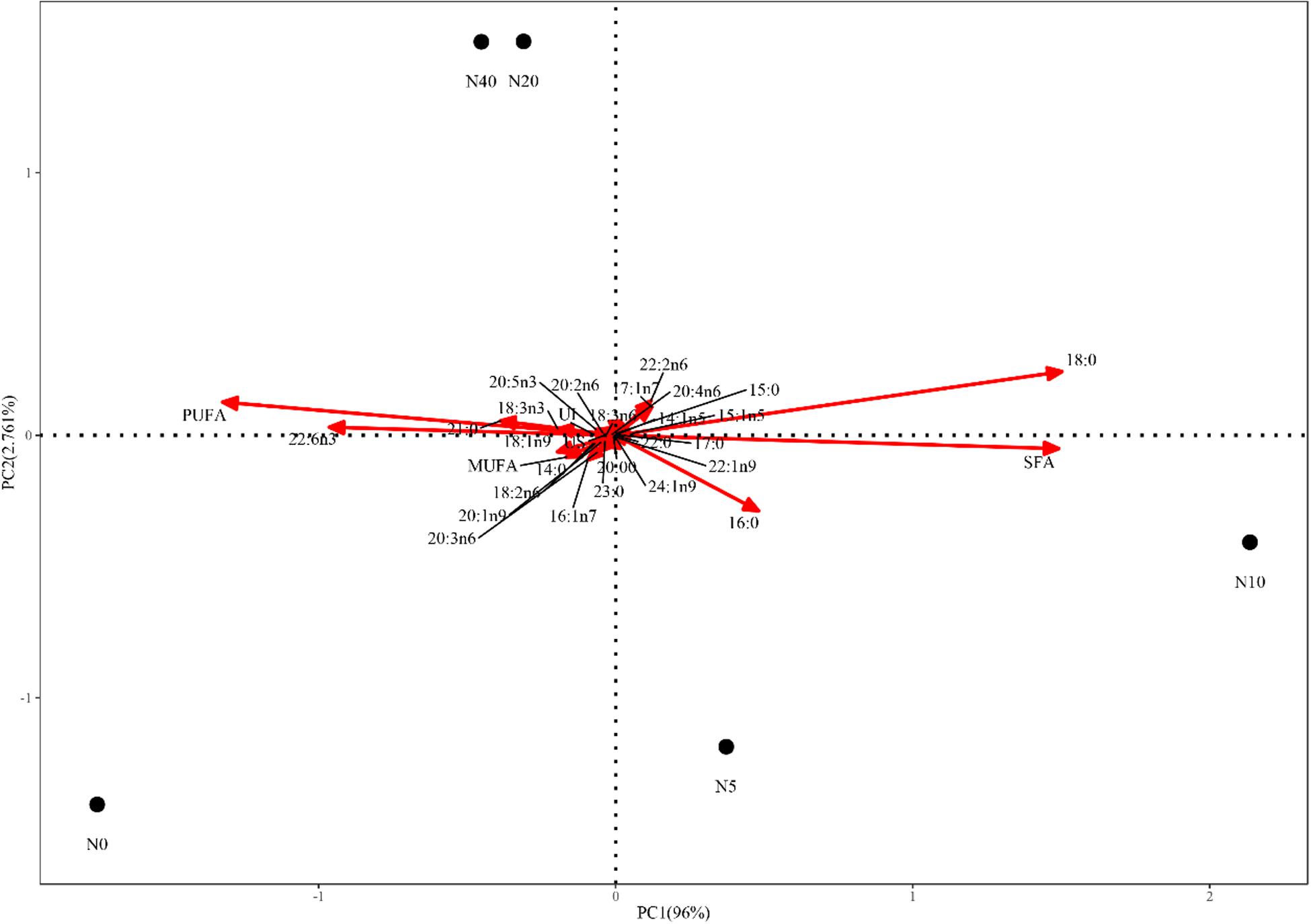
Figure 2. Principal component analysis of P. damicornis larvae with different nitrate concentrations.
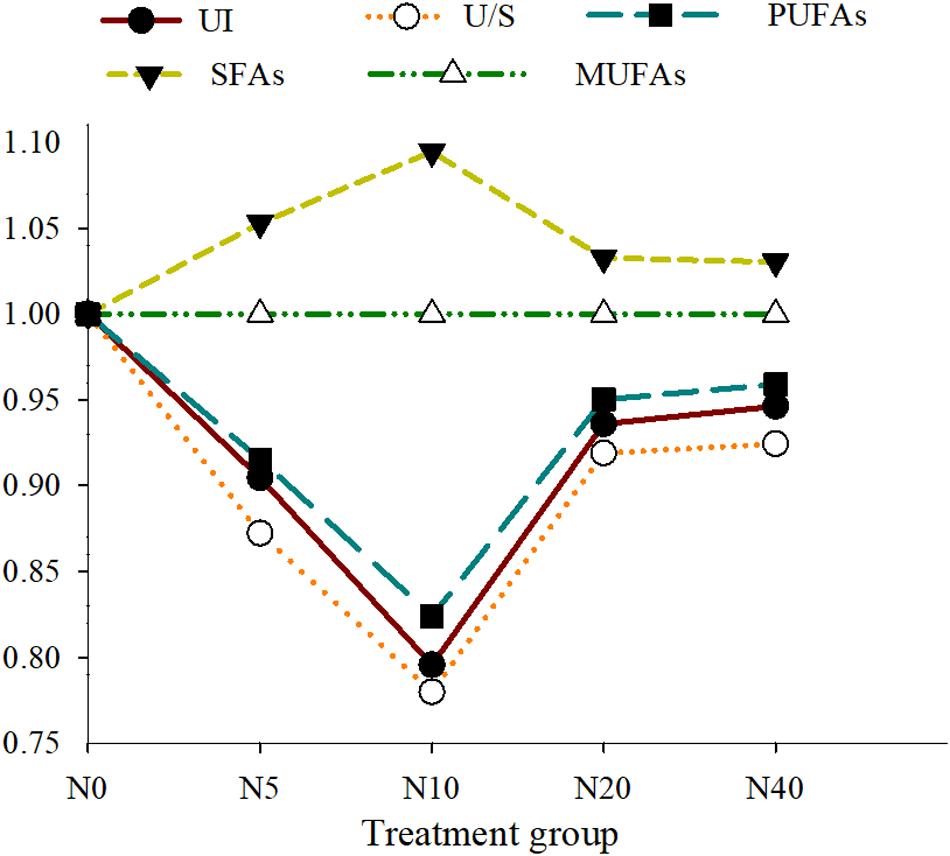
Figure 3. Changes in UI, U/S, SFA, PUFA, and MUFA of P. damicornis larvae with different nitrate concentrations. Note: Each value is the ratio of the different treatment groups to the N0 group. MUFA, monounsaturated fatty acid; PUFA, polyunsaturated fatty acid; SFA, saturated fatty acid; UI, unsaturation index; U/S, unsaturated to saturated fatty acid ratio.
When the nitrate concentration was less than 10 μM, the SFA levels in the P. damicornis larvae were significantly elevated, primarily due to increased 16:0 and 18:0 levels and decreased PUFA concentrations (18:2n6, 18:3n3, 20:3n6, and 22:6n3), with corresponding reductions in UI and U/S.
In contrast, the SFA levels of larvae decreased and the UFAs increased in the N20 group, leading to elevated UI and U/S. Compared with the N20 group, no significant difference (p > 0.05) was observed in the FA profiles of the P. damicornis larvae in the N40 group. Although the levels of some monoenes altered significantly throughout the experiment (major change in 18:1n9), the total MUFA levels of the larvae did not change.
Discussion
Respiration and Photosynthesis of P. damicornis Larvae With Nitrate Enrichment
Among all the groups, N5 had the highest PNet and PNet/RD, which implied that low nitrate enrichment could promote photosynthesis in coral larval zooxanthellae. Marubini and Davies (1996) reported that low nitrate enrichment (>5 μM) enhances the photosynthesis of coral zooxanthellae by increasing zooxanthellae size and chlorophyll concentrations. Atkinson et al. (1995) also demonstrated that coral living in aquaria can thrive in relatively high-nutrient water (nitrate: about 5 μM). Nonetheless, coral species-specific responses to nitrate enrichment have been found in previous studies (Ferrier-Pagès et al., 2001; D’Angelo and Wiedenmann, 2014; Serrano et al., 2018). Contrary to our findings, Ferrier-Pagès et al. (2001) found that nitrate enrichment had no effect on Stylophora pistillata.
In this study, relatively higher nitrate concentrations produced PNet decreases and RD increases, resulting in decreased PNet/RD. This indicated that high levels of nitrate (>20 μM) would have a negative impact on P. damicornis larvae. Serrano et al. (2018) found that nitrate enrichment (12 μM) could significantly increase respiration in Porites astreoides larvae. Since RD represents the metabolic rate of coral larvae, we hypothesized that P. damicornis larvae increase respiration in order to provide enough energy to ameliorate environmental stress. Nordemar et al. (2003) documented that the primary production of Porites cylindrica declined after 2 weeks of nitrate enrichment (15 μM), while zooxanthellae density and chlorophyll concentrations (photosynthesis) remained unaltered. Alternatively, increased respiration rates in nutrient-enriched P. damicornis larvae may affect their stress response to potential photo-physiological damage to their algal symbionts (D’Angelo and Wiedenmann, 2014).
FA Compositions of P. damicornis Larvae
In this study, the SFA level (mainly 16:0 and 18:0) was the highest (over 60%) for FAs in the P. damicornis larvae. The FA patterns of P. damicornis larvae were similar to those of adults of this species (previous unpublished research) and to those of Goniastrea retiformis (Figueiredo et al., 2012), Acropora millepora, Acropora tenuis, and Montipora digitata eggs (Arai et al., 1993). Both Papina et al. (2003) and Chen et al. (2015) found that palmitic acid (16:0) acted as a trophic marker of zooxanthellae and was abundant in coral larvae, especially those that inherited algal symbionts maternally from oocytes. P. damicornis release larvae that carry symbiotic micro-algae, which may explain the higher SFAs observed in our study. Moreover, compared to PUFAs, SFAs have a more stable structure and are commonly used for bio-energy storage. Many investigators have reported that SFAs (such as 16:0 and 18:0) are the preferred sources of metabolic energy in marine organisms (Arai et al., 1993; Figueiredo et al., 2012; Wijekoon, 2012). Consequently, P. damicornis larvae need to store sufficient SFAs to have a capacity to delay metamorphosis until suitable settlement cues appear.
Likewise, a high abundance of several PUFAs (such as 18:3n3, 20:3n6, 20:5n3, and 22:6n3) was found. This may be due to the fact that symbiotic zooxanthellae contain high PUFA levels (Zhukova and Titlyanov, 2003) and symbionts transfer PUFAs to host tissue (Figueiredo et al., 2012), after which they are incorporated into their larvae. Although the specific functions of PUFAs for coral remain poorly studied, PUFAs, especially the n-3 and n-6 PUFAs, have been confirmed as critical to the growth, survival, and reproduction of many organisms, including corals (Tchernov et al., 2004; Imbs, 2013; Chen et al., 2015; Ernst et al., 2016; Conlan et al., 2017).
Effect of Nitrate Enrichment on the FA Composition of P. damicornis Larvae
Significant changes in the FA compositions of P. damicornis larvae were observed under nitrate enrichment. Our results indicate that the lipids of P. damicornis larvae become progressively saturated (lower UI and U/S) when nitrate is less than 10 μM, which can be attributed to decreased PUFAs and a concomitant increase in SFAs. Moreover, no changes in TFA levels were found at the lower nitrate concentrations. Lipids and proteins are the primary constituents of biomembranes. Changes in FA composition could affect membrane-associated physical attributes and biological functions, such as membrane-phase behaviors, membrane thickness, and membrane permeability (Losa and Murata, 2004; Wijekoon, 2012; Ernst et al., 2016). Compared to UFAs, homologous SFAs have a higher melting point and occupy a smaller space within the membrane lipid bilayer, which enhances their rigidity and viscosity (Hazel, 1979; Yeagle, 1989; Liu et al., 2018). The more rigid and viscous the biomembrane, the more slowly harmful ions enter the organism. Thus, the present data indicated that P. damicornis larvae can adapt to low-level nitrate enrichment by biomembrane restructuring. While it is well established that FAs play an important role in stress resistance and that the ability of an organism to maintain appropriate membrane function integrity and cell homeostasis in the face of environmental change is intimately linked to tolerance, this is the first time that these responses have been demonstrated in coral larvae.
Moreover, with increases in environmental stress, organisms may produce more reactive oxygen species (ROS) (Cunning and Baker, 2013; Liu et al., 2018). Irrespective of the MUFA or PUFA contents, a higher proportion of UFAs in lipids was associated with increased ROS susceptibility, whereas SFAs did not undergo lipid peroxidation (Porter, 2013; Cengiz et al., 2017). Organisms with a higher proportion of SFAs could reduce the influence of ROS (Wada et al., 1994; Crockett, 2008; Liu et al., 2019). Thus, such adaptations with respect to lipids can minimize the influence of nitrate enrichment on P. damicornis larvae. Similarly, with nitrate enrichment, thermal stress can significantly affect the coral FA composition. While studying symbiotic algae in thermally bleached corals, Tchernov et al. (2004) found that higher SFAs enhanced the thermal stability of the eukaryotic thylakoid membranes of coral and simultaneously reduced the susceptibility of the membrane lipids to attacks by ROS.
One interesting result was that while UFAs were lowest in the N10 group, the PNet dropped compared to the N5 group. Although higher SFA can increase biomembrane rigidity and anti-oxidation, it reduces membrane-bound enzyme activity and material exchange (Oku et al., 2002; Bachok et al., 2006; Ernst et al., 2016), which may reduce the photosynthesis of zooxanthellae. Bennett et al. (2018) reported that PUFA enrichment in the phototrophic sponge Carteriospongia foliascens can significantly facilitate enzyme activity. Ernst et al. (2016) showed that lipids with saturated acyl chains are packed at higher densities and tend to form non-fluid gel phases, which slow substance exchange.
Different FA patterns were observed in the P. damicornis larvae in the N20 and N40 groups. The UI and U/S levels were elevated due to the increased PUFA levels and decreased SFA levels at higher nitrate concentrations. Although the nitrate concentration increased, compared with the N5 and N10 groups, the fluidity of the biomembrane of the larvae did not continue to decrease but instead increased at higher nitrate concentrations, indicating that the biomembrane restructuring in the larvae may have become ineffective. In parallel, the TFAs decreased significantly. This result showed that numerous lipids were consumed rapidly. When ions exceed the threshold that the larvae can tolerate (hyperosmotic stress), the larvae need to excrete the ions via active transport in order to maintain homeostasis (Losa and Murata, 2004). Thus, a large amount of SFA, as the preferred source of metabolic energy, is oxidized to provide energy for this process. This is consistent with our findings that P. damicornis larval RD increased significantly at high nitrate concentrations (N20 and N40 groups). In addition, to some extent, the increase in UFAs could promote biomembrane fluidity, enzyme activity, and internal and external exchange of substances that could enhance metabolism (Brenner, 1984; Bennett et al., 2018; Liu et al., 2018). However, a higher proportion of UFAs would reduce the antioxidant capacity of larvae. Hence, numerous lipids were consumed, and the increased risk of lipid peroxidation could adversely affect the successful settlement and development of the larvae.
Conclusion
The present research showed that, in all treatment groups, P. damicornis larvae had the highest proportion of SFAs, followed by PUFAs, and the lowest proportion of MUFAs. The FA pattern of larvae could store sufficient energy to delay metamorphosis until suitable settlement cues appeared. Nitrate enrichment significantly changed the FA composition of P. damicornis larvae. The lipids of P. damicornis larvae became progressively saturated (lower UI and U/S) when the nitrate concentration was less than 10 μM, which could be attributed to decreased PUFAs and concomitant increases in SFAs. Such changes enabled the P. damicornis larvae to adapt to low-level nitrate enrichment (<10 μM). Moreover, the N5 group had the highest PNet and PNet/DR, which implied that low nitrate enrichment could promote photosynthesis of coral larvae zooxanthellae. However, the opposite pattern for FA compositions was found with higher nitrate concentrations (>20 μM), in which the UI and U/S levels were elevated due to increased PUFAs and decreased SFAs. With high nitrate levels, biomembrane restructuring in larvae may became ineffective, increasing respiration and rapidly consuming numerous lipids, which could adversely affect the successful settlement and development of larvae.
Data Availability Statement
The raw data supporting the conclusions of this article will be made available by the authors, without undue reservation.
Author Contributions
C-YL, FZ, and HH designed the experiments. C-YL, FZ, Y-FS, and X-LY carried out the experiments. C-YL, FZ, and HH analyzed the experimental results and wrote the manuscript.
Funding
This study was jointly funded by the National Natural Science Foundation of China (NSFC) (Nos. 41906097 and 41976120), Science and Technology Planning Project of Guangdong Province, China (2017B0303014052), Innovation Academy of South China Sea Ecology and Environmental Engineering, Chinese Academy of Sciences (ISEE2018PY01), and the Science and Technology Service Network Initiative of the Chinese Academy of Sciences (KFJ-STS-ZDTP-055).
Conflict of Interest
The authors declare that the research was conducted in the absence of any commercial or financial relationships that could be construed as a potential conflict of interest.
Acknowledgments
The authors would like to express their gratitude for the staff of the Hainan Tropical Marine Biological Research Station for providing technical assistance and facilities for conducting the work, as well as to those who critically reviewed this manuscript.
Supplementary Material
The Supplementary Material for this article can be found online at: https://www.frontiersin.org/articles/10.3389/fmars.2020.00531/full#supplementary-material
References
Arai, I., Kato, M., Heyward, A., Ikeda, Y., and Maruyama, T. (1993). Lipid composition of positively buoyant eggs of reef building corals. Coral Reefs 12, 71–75. doi: 10.1007/bf00302104
Atkinson, M. J., Carlson, B., and Crow, G. L. (1995). Coral growth in high-nutrient, low-pH seawater: a case study of corals cultured at the Waikiki Aquarium, Honolulu, Hawaii. Coral Reefs 14, 215–223. doi: 10.1007/bf00334344
Bachok, Z., Mfilinge, P., and Tsuchiya, M. (2006). Characterization of fatty acid composition in healthy and bleached corals from Okinawa, Japan. Coral Reefs 25, 545–554. doi: 10.1007/s00338-006-0130-9
Bender-Champ, D., Diaz-Pulido, G., and Dove, S. (2017). Effects of elevated nutrients and CO2 emission scenarios on three coral reef macroalgae. Harmful Algae 65:40. doi: 10.1016/j.hal.2017.04.004
Bennett, H., Bell, J. J., Davy, S. K., Webster, N. S., and Francis, D. S. (2018). Elucidating the sponge stress response; lipids and fatty acids can facilitate survival under future climate scenarios. Glob. Change Biol. 24, 3130–3144. doi: 10.1111/gcb.14116
Bhojoo, U., Chen, M., and Zou, S. (2017). Temperature induced lipid membrane restructuring and changes in Nanomechanics. Biochim. Biophys. Acta 1860, 700–709. doi: 10.1016/j.bbamem.2017.12.008
Brenner, R. R. (1984). Effect of unsaturated acids on membrane structure and enzyme kinetics. Prog. Lipid Res. 23, 69–96. doi: 10.1016/0163-7827(84)90008-0
Cengiz, E. I., Bayar, A. S., Kızmaz, V., Başhan, M., and Satar, A. (2017). Acute toxicity of deltamethrin on the fatty acid composition of phospholipid classes in liver and gill tissues of Nile tilapia. Int. J. Environ. Res. 11, 1–9.
Chen, H.-K., Shin-Ni, S., Li-Hsueh, W., Mayfield, A. B., Yi-Jyun, C., Chen, W. N. U., et al. (2015). A compartmental comparison of major lipid species in a coral-Symbiodinium endosymbiosis: evidence that the coral host regulates lipogenesis of its cytosolic lipid bodies. PLoS One 10:e0132519. doi: 10.1371/journal.pone.0132519
Conlan, J. A., Rocker, M. M., and Francis, D. S. (2017). A comparison of two common sample preparation techniques for lipid and fatty acid analysis in three different coral morphotypes reveals quantitative and qualitative differences. PeerJ 5:e3645. doi: 10.7717/peerj.3645
Crockett, E. L. (2008). The cold but not hard fats in ectotherms: consequences of lipid restructuring on susceptibility of biological membranes to peroxidation, a review. J. Comp. Physiol. B 178, 795–809. doi: 10.1007/s00360-008-0275-7
Cunning, R., and Baker, A. C. (2013). Excess algal symbionts increase the susceptibility of reef corals to bleaching. Nat. Clim. Change 3, 259–262. doi: 10.1038/nclimate1711
D’Angelo, C., and Wiedenmann, J. (2014). Impacts of nutrient enrichment on coral reefs: new perspectives and implications for coastal management and reef survival. Curr. Opin. Env. Sust. 7, 82–93. doi: 10.1016/j.cosust.2013.11.029
Ernst, R., Ejsing, C. S., and Antonny, B. (2016). Homeoviscous adaptation and the regulation of membrane lipids. J. Mol. Biol. 428, 4776–4791. doi: 10.1016/j.jmb.2016.08.013
Ferrierpagès, C., Gattuso, J. P., Dallot, S., and Jaubert, J. (2000). Effect of nutrient enrichment on growth and photosynthesis of the zooxanthellate coral Stylophora pistillata. Coral Reefs 19, 103–113. doi: 10.1007/s003380000078
Ferrier-Pagès, C., Schoelzke, V., Jaubert, J., Muscatine, L., and Hoegh-Guldberg, O. (2001). Response of a scleractinian coral, Stylophora pistillata, to iron and nitrate enrichment. J. Exp. Mar. Biol. Ecol. 259, 249–261. doi: 10.1016/s0022-0981(01)00241-6
Figueiredo, J., Baird, A. H., Cohen, M. F., Flot, J.-F., Kamiki, T., Meziane, T., et al. (2012). Ontogenetic change in the lipid and fatty acid composition of scleractinian coral larvae. Coral Reefs 31, 613–619. doi: 10.1007/s00338-012-0874-3
Harrison, P., and Ward, S. (2001). Elevated levels of nitrogen and phosphorus reduce fertilisation success of gametes from scleractinian reef corals. Mar. Biol. 139, 1057–1068. doi: 10.1007/s002270100668
Hazel, J. R. (1979). Influence of thermal acclimation on membrane lipid composition of rainbow trout liver. Proc. Natl. Acad. Sci. U.SA. 236:R91.
Hughes, T. P., Kerry, J. T., Baird, A. H., Connolly, S. R., Dietzel, A., Eakin, C. M., et al. (2018). Global warming transforms coral reef assemblages. Nature 556:492. doi: 10.1038/s41586-018-0041-2
Humanes, A., Noonan, S. H. C., Willis, B. L., Fabricius, K. E., de Beer, D., and Negri, A. P. (2016). Cumulative effects of nutrient enrichment and elevated temperature compromise the early life history stages of the coral Acropora tenuis. PLoS One 8:e0161616. doi: 10.1016/j.marpolbul.2017.08.003
Imbs, A. B. (2013). Fatty acids and other lipids of corals: composition, distribution, and biosynthesis. Russ. J. Mar. Biol. 39, 153–168. doi: 10.1134/s1063074013030061
Jiang, L., Guo, Y.-J., Zhang, F., Zhang, Y.-Y., McCook, L. J., Yuan, X.-C., et al. (2019). Diurnally fluctuating pCO2 modifies the physiological responses of coral recruits under ocean acidification. Front. Physiol. 9:1952. doi: 10.3389/fphys.2018.01952
Jiang, L., Sun, Y. F., Zhang, Y. Y., Zhou, G. W., Li, X. B., McCook, L. J., et al. (2017). Impact of diurnal temperature fluctuations on larval settlement and growth of the reef coral Pocillopora damicornis. Biogeosciences 14, 5741–5752. doi: 10.5194/bg-14-5741-2017
Jing, G., Yu, K., Wang, Y., Xu, D., Huang, X., Zhao, M., et al. (2017). Nutrient distribution in coral reef degraded areas within sanya bay, South China Sea. J. Coast. Res. 33, 1148–1160.
Kubicek, A., Breckling, B., Hoegh-Guldberg, O., and Reuter, H. (2019). Climate change drives trait-shifts in coral reef communities. Sci. Rep. 9:3721.
Lam, E. K. Y., Chui, A. P. Y., Kwok, C. K., Ip, A. H. P., Chan, S. W., Leung, H. N., et al. (2015). High levels of inorganic nutrients affect fertilization kinetics, early development and settlement of the scleractinian coral Platygyra acuta. Coral Reefs 34, 837–848. doi: 10.1007/s00338-015-1317-8
Liu, C., Dong, S., Zhou, Y., Shi, K., Pan, Z., Sun, D., et al. (2019). Temperature-dependent fatty acid composition change of phospholipid in steelhead trout (Oncorhynchus mykiss) tissues. J. Ocean Univ. China 18, 519–527. doi: 10.1007/s11802-019-3775-z
Liu, C., Zhou, Y., Dong, K., Sun, D., Gao, Q., and Dong, S. (2018). Differences in fatty acid composition of gill and liver phospholipids between Steelhead trout (Oncorhynchus mykiss) and Atlantic salmon (Salmo salar) under declining temperatures. Aquaculture 495, 815–822. doi: 10.1016/j.aquaculture.2018.06.045
Losa, D. A., and Murata, N. (2004). Membrane fluidity and the perception of environmental signals. Biochim. Biophys. Acta 1666, 142–157. doi: 10.1016/j.bbamem.2004.08.002
Marubini, F., and Davies, P. S. (1996). Nitrate increases zooxanthellae population density and reduces skeletogenesis in corals. Mar. Biol. 127, 319–328. doi: 10.1007/bf00942117
Muscatine, L., McCloskey, L. R., and Marian, R. E. (1981). Estimating the daily contribution of carbon from zooxanthellae to coral animal respiration. Limnol. Oceanogr. 26, 601–611. doi: 10.4319/lo.1981.26.4.0601
Nordemar, I., Nystrm, M., and Dizon, R. (2003). Effects of elevated seawater temperature and nitrate enrichment on the branching coral Porites cylindricain the absence of particulate food. Mar. Biol. 142, 669–677. doi: 10.1007/s00227-002-0989-0
Oku, H., Yamashiro, H., Onaga, K., Iwasaki, H., and Takara, K. (2002). Lipid distribution in branching coral Montipora digitata. Fish. Sci. 68, 517–522. doi: 10.1046/j.1444-2906.2002.00456.x
Papina, M., Meziane, T., and Woesik, R. V. (2003). Symbiotic zooxanthellae provide the host-coral Montipora digitata with polyunsaturated fatty acids. Comp. Biochem. Physiol. B 135, 533–537. doi: 10.1016/s1096-4959(03)00118-0
Porter, N. A. (2013). A perspective on free radical autoxidation: the physical organic chemistry of polyunsaturated fatty acid and sterol peroxidation. J. Org. Chem. 78, 3511–3524. doi: 10.1021/jo4001433
Renegar, D. A., and Riegl, B. M. (2005). Effect of nutrient enrichment and elevated CO2 partial pressure on growth rate of atlantic scleractinian coral Acropora cervicornis. Mar. Ecol. Prog. Ser. 293, 69–76. doi: 10.3354/meps293069
Serrano, X. M., Miller, M. W., Hendee, J. C., Jensen, B. A., Gapayao, J. Z., Pasparakis, C., et al. (2018). Effects of thermal stress and nitrate enrichment on the larval performance of two Caribbean reef corals. Coral Reefs 37, 173–182. doi: 10.1007/s00338-017-1645-y
Sinensky, M. (1974). Homeoviscous adaptation-a homeostatic process that regulates the viscosity of membrane lipids in Escherichia coli. Proc. Natl. Acad. Sci. U.S.A. 71, 522–525. doi: 10.1073/pnas.71.2.522
Snyder, R. J., and Hennessey, T. M. (2003). Cold tolerance and homeoviscous adaptation in freshwater alewives (Alosa pseudoharengus). Fish Physiol. Biochem. 29, 117–126. doi: 10.1023/b:fish.0000035920.60817.11
Tchernov, D., Gorbunov, M. Y., Vargas, C. D., Yadav, S. N., Milligan, A. J., Haggblom, M., et al. (2004). Membrane lipids of symbiotic algae are diagnostic of sensitivity to thermal bleaching in corals. Proc. Natl. Acad. Sci. U.S.A. 101, 13531–13535. doi: 10.1073/pnas.0402907101
Wada, H., Gombos, Z., and Murata, N. (1994). Contribution of membrane lipids to the ability of the photosynthetic machinery to tolerate temperature stress. Proc. Natl. Acad. Sci. U.S.A. 91, 4273–4277. doi: 10.1073/pnas.91.10.4273
Wallaert, C., and Babin, P. J. (1994). Thermal adaptation affects the fatty acid composition of plasma phospholipids in trout. Lipids 29, 373–376. doi: 10.1007/bf02537193
Ward, S., and Harrison, P. (2000). Changes in gametogenesis and fecundity of acroporid corals that were exposed to elevated nitrogen and phosphorus during the ENCORE experiment. J. Exp. Mar. Biol. Ecol. 246, 179–221. doi: 10.1016/s0022-0981(99)00182-3
Wijekoon, M. P. A. (2011). Effect of Water Temperature and Diet on Cell Membrane Fluidity and Fatty Acid Composition of Muscle, Liver, Gill and Intestine Mucosa of Adult and Juvenile Steelhead Trout, Oncorhynchus Mykiss. Ph.D. Thesis, Memorial University of Newfoundland, Newfoundland.
Wijekoon, M. P. A. (2012). Effect of Water Temperature and Diet on Cell Membrane Fluidity and Fatty Acid Composition of Muscle, Liver, Gill and Intestine Mucosa of Adult and Juvenile Steelhead Trout, Oncorhynchus Mykiss. Newfoundland: Memorial University of Newfoundland.
Yeagle, P. L. (1989). Lipid regulation of cell membrane structure and function. FASEB J. 3, 1833–1842. doi: 10.1096/fasebj.3.7.2469614
Zaneveld, J. R., Burkepile, D. E., Shantz, A. A., Pritchard, C. E., Mcminds, R., Payet, J. P., et al. (2016). Overfishing and nutrient pollution interact with temperature to disrupt coral reefs down to microbial scales. Nat. Commun. 7:11833.
Keywords: Pocillopora damicornis larvae, fatty acid composition, nitrate enrichment, biomembrane restructuring, respiration, photosynthesis
Citation: Liu C-Y, Zhang F, Sun Y-F, Yu X-L and Huang H (2020) Effects of Nitrate Enrichment on Respiration, Photosynthesis, and Fatty Acid Composition of Reef Coral Pocillopora damicornis Larvae. Front. Mar. Sci. 7:531. doi: 10.3389/fmars.2020.00531
Received: 09 April 2020; Accepted: 11 June 2020;
Published: 07 July 2020.
Edited by:
Zhi Zhou, Hainan University, ChinaReviewed by:
Tingting Xiang, University of North Carolina at Charlotte, United StatesZhaoqun Liu, Dalian Ocean University, China
Copyright © 2020 Liu, Zhang, Sun, Yu and Huang. This is an open-access article distributed under the terms of the Creative Commons Attribution License (CC BY). The use, distribution or reproduction in other forums is permitted, provided the original author(s) and the copyright owner(s) are credited and that the original publication in this journal is cited, in accordance with accepted academic practice. No use, distribution or reproduction is permitted which does not comply with these terms.
*Correspondence: Hui Huang, aHVhbmdodWlAc2NzaW8uYWMuY24=