- 1School of Biological Sciences, The University of Western Australia, Perth, WA, Australia
- 2The UWA Oceans Institute, The University of Western Australia, Perth, WA, Australia
- 3British Antarctic Survey, Cambridge, United Kingdom
- 4Centre for Evolutionary Biology, School of Biological Sciences, The University of Western Australia, Perth, WA, Australia
Global oceans are absorbing over 90% of the heat trapped in our atmosphere due to accumulated anthropogenic greenhouse gases, resulting in increasing ocean temperatures. Such changes may influence marine ectotherms, such as sharks, as their body temperature concurrently increases toward their upper thermal limits. Sharks are high trophic level predators that play a key role in the regulation of ecosystem structure and health. Because many sharks are already threatened, it is especially important to understand the impact of climate change on these species. We used shark occurrence records collected by commercial fisheries within the Australian continental Exclusive Economic Zone (EEZ) to predict changes in future (2050–2099) relative to current (1956–2005) habitat suitability for pelagic sharks based on an ensemble of climate models and emission scenarios. Our predictive models indicate that future sea temperatures are likely to shift the location of suitable shark habitat within the Australian EEZ. On average, suitable habitat is predicted to decrease within the EEZ for requiem and increase for mackerel sharks, however, the direction and severity of change was highly influenced by the choice of climate model. Our results indicate the need to consider climate change scenarios as part of future shark management and suggest that more broad-scale studies are needed for these pelagic species.
Introduction
Climate change is predicted to have unprecedented effects on the marine environment, with changes in ocean temperature increasing extinction risk for many species (Dulvy et al., 2003; Barnosky et al., 2011; Bruno et al., 2018; Pinsky et al., 2019) and altering the global distribution of marine life (Tittensor et al., 2010; Garciá Molinos et al., 2016). Changes in species distribution (Perry et al., 2005; Poloczanska et al., 2013) and community structure (Doney et al., 2012) are already being observed in marine ecosystems due to temperature shifts associated with rising emissions and accumulation of atmospheric carbon dioxide (Hoegh-Guldberg and Bruno, 2010; Doney et al., 2012; Gattuso et al., 2015). Recent modeling of biodiversity under different future climate change scenarios, across a wide range of marine and terrestrial ecosystems, predicts abrupt and irreversible ecosystem disruption during the late 21st century (Trisos et al., 2020). With predicted increases of up to ∼5°C in worldwide sea-surface temperature (SST) by the end of the 21st century (IPCC, 2015), there is a critical need to investigate how marine species will be affected, especially ectotherms which are dependent on external sources for body heat. As ectotherms, sharks may be influenced by climate change (Bernal et al., 2012; Rosa et al., 2014, 2017; Syndeman et al., 2015; Pinsky et al., 2019), with higher temperatures increasing their metabolism and oxygen demand (Pistevos et al., 2015; Lawson et al., 2019). The exception to this may be Lamnid mackerel sharks, which have some endothermic capability (Watanabe et al., 2015).
Many shark species are already globally threatened due to fisheries overexploitation (Queiroz et al., 2019) coupled with their low fecundity, late age at maturity, and slow growth (Cortés, 2000; Garcia et al., 2008; Yokoi et al., 2017). In fact, 16.6% of shark species are estimated to be threatened with extinction, and another 37.9% of shark species are categorized as “Data Deficient” by the International Union for Conservation of Nature (IUCN, 2020). Nevertheless, sharks are known to have direct economic value in fisheries (Dulvy et al., 2017) and ecotourism (Cisneros-Montemayor et al., 2013; Huveneers et al., 2017). They also play a key role in ecosystem functioning and stability, connecting distant ecosystems via their long-distance migrations (Rogers et al., 2015), and altering prey behavior, distribution and energy use (Heupel et al., 2015; Roff et al., 2016; Dulvy et al., 2017). Climate change may exacerbate existing threats for sharks, for example, suitable pelagic shark habitat in the north Pacific Ocean is projected to decline by the year 2100 (Hazen et al., 2013).
Future projections based on existing observations and modeling techniques can be used to investigate the effects of climate change on pelagic sharks (Barange et al., 2016). Using Earth System Models from the Coupled Model Intercomparison Project Phase 5 (CMIP5; hereafter called “climate models”), complex relationships between ecosystem health, human activities and global climate can be included to evaluate alternative future scenarios with varying severity of emissions (Moss et al., 2010; Freer et al., 2017). There are four emission scenarios commonly referred to as Representative Concentration Pathways (RCP 2.6, RCP 4.5, RCP 6, and RCP 8.5) (IPCC, 2013). These RCP scenarios are used to predict radiative forcing values, a measure of absorbed and retained energy in the lower atmosphere, for the year 2100 (Moss et al., 2010; Vuuren et al., 2011). RCP 4.5, also referred to as “stabilization scenario,” is an optimistic scenario assuming a decline in overall energy usage from fossil fuel sources that limits emissions and radiative forcing (Thomson et al., 2011). Conversely, RCP 8.5, also referred to as “business-as-usual,” is the most pessimistic scenario assuming minimal stabilization of greenhouse gas emissions alongside a large human population with high energy demands (Riahi et al., 2011).
The Australian Exclusive Economic Zone (EEZ) is already being impacted by climate change with waters off south-east Australia warming at almost four times the global average (Oliver et al., 2017) and range extensions already documented in several fish species (Last et al., 2011). Australia has one of the world’s most diverse communities of sharks, with 182 recognized species (Simpfendorfer et al., 2019), and SST has been shown consistently to be a strong predictor of pelagic shark occurrence in Australian waters (Rogers et al., 2009, 2015; Stevens et al., 2010; Heard et al., 2017; Birkmanis et al., 2020). It is therefore important to investigate the likely impact of temperature changes on pelagic shark distribution and the location of suitable habitat on a continental scale if these species are to be appropriately managed into the future – especially if such changes may require a reassessment of interactions with fisheries in the future. Sharks comprise approximately 27% of the total catch (by number) of Australian pelagic longline fisheries (Gilman et al., 2008), with Australian stocks of the IUCN classified “Critically Endangered” oceanic whitetip (Carcharhinus longimanus), “Endangered” shortfin mako (Isurus oxyrinchus), and “Endangered” longfin mako (Isurus paucus) sharks listed respectively as “overfished,” “depleting,” and “undefined” due to a lack of data (Simpfendorfer et al., 2019; IUCN, 2020).
This study follows on from Birkmanis et al. (2020) in which occurrence records of pelagic sharks belonging to the Carcharhinidae and Lamnidae families (hereafter “requiem” and “mackerel,” respectively) were obtained from commercial fisheries and used to develop generalized linear models with which to predict suitable habitat for these species within the Australian continental EEZ. After accounting for fishing effort bias, these models showed that SST was an important predictor of shark distributions, with the highest ranked model also including turbidity. Here, we extend our modeling to assess the impact of climate change on pelagic shark habitat across the entire continental Australian EEZ.
Materials and Methods
Shark Occurrence
Catch records of 3,973 individual sharks from two families; requiem (silky Carcharhinus falciformis, oceanic whitetip Carcharhinus longimanus, dusky Carcharhinus obscurus and blue Prionace glauca) and mackerel (shortfin mako Isurus oxyrinchus, longfin mako Isurus paucus and porbeagle Lamna nasus) were obtained through the Global Biodiversity Information Facility online database (GBIF.org, 2017), as per details included in Birkmanis et al. (2020). These oceanic sharks were caught predominantly using commercial longlines in Commonwealth managed fisheries (more detailed data unavailable), with catch locations depicted in Supplementary Figure S1.
Predictors for Modeling Baseline and Future Climate Environmental Data
A climatological baseline was used as a reference point for projected future climate changes. According to Birkmanis et al. (2020), SST and turbidity were the most suitable predictors of requiem and mackerel shark occurrence within the Australian EEZ. We therefore focused on these two predictors to develop a climatological baseline to use as a reference for projected future climate changes. To calculate the SST baseline data, we downloaded monthly SST values for the years 1956–2005, covering the time period of our observed shark occurrence data, from the Integrated Marine Observing System (IMOS, 2016). We then averaged the SST values for each 0.1° grid-cell in the study area using ArcGIS 10.5 from Environmental Systems Research Institute (ESRI, 2017). We incorporated the observed turbidity values (measured as mean diffuse attenuation coefficient at wavelength 490 nm, downloaded using the Marine Geospatial Ecology Tool; Roberts et al., 2010) from 2000 to 2002 into our models with the assumption that turbidity will remain unchanged in the future.
Future SST data were taken from 24 CMIP5 climate models, using only one realization per climate model, under two emission scenarios, RCP 4.5 and RCP 8.5, amounting to 48 total simulations (Table 1). We downloaded the SST field and the anomaly statistic for each climate model (Table 1) under both RCP 4.5 and RCP 8.5 from the Climate Change Web Portal (Earth Systems Research Laboratory, 2014), developed by the National Oceanic and Atmospheric Administration’s Earth System Research Laboratory to collate climate data and climate model outputs from the CMIP5 (see Scott et al., 2016 for details). We used the portal to calculate the difference in the mean SST between the future climate (2050–2099) and the model baseline reference period (1956–2005), hereafter called “anomaly” data. We then added these anomaly data to our baseline data across the extent of the Australian EEZ using ArcGIS and included this as the SST predictor for the future values.
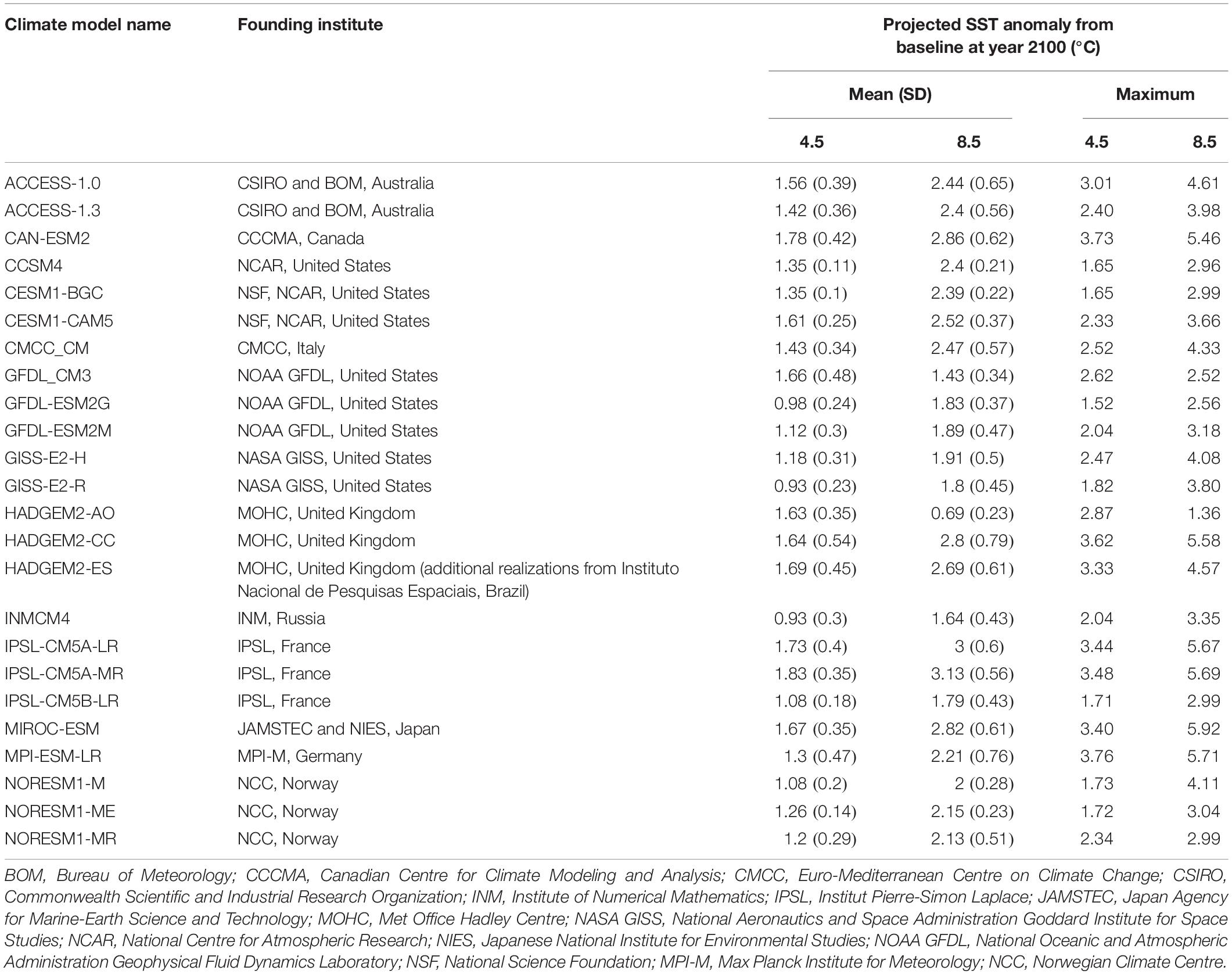
Table 1. Mean and maximum projected sea surface temperature (SST) anomalies for the end of the 21st century (2050–2099) in Australian waters for each climate model under both emission scenarios (RCP 4.5 and RCP 8.5; standard deviation shown in parentheses).
Modeling Habitat Suitability for Baseline and Future Climate Data
We developed binomial generalized linear models with a logit link function for each of the two pelagic shark families, following Birkmanis et al. (2020). In brief, the probability of shark occurrence (calculated as the number of sharks caught divided by the number of fishing boats occurring in the same grid-cell) was used as the response variable, with turbidity and SST values for either the climatological baseline or the future used as predictors. We included effort, defined as the number of boats recorded in each grid-cell from the same time period as the occurrence data (2000–2002), as a model weight to account for differing amounts of catch per unit effort (CPUE) within the entire EEZ. As in, we weighted our models by fishing effort to estimate the probability of finding a shark in each grid-cell within the Australian EEZ which minimized the effect of fisheries effort on the data (Birkmanis et al., 2020). To stabilize parameter estimation, we standardized both predictors to z-scores using the scale function in R statistical software (R Core Team, 2017) before inclusion in our models (James et al., 2015). We also included a quadratic term for SST using the poly function from the stats package (R Core Team, 2017) in R statistical software to account for likely preferential SST ranges. We then quantified the goodness-of-fit for each model using the percentage of deviance explained, and used the predict function from the stats package in R statistical software to predict shark habitat suitability for the baseline data and also for the end of the century using the future climate data. To calculate the amount of change in suitable habitat under each climate model and emission scenario, we subtracted the number of grid-cells with resulting suitable habitat ≥0.5 in the future climate scenarios from those obtained in the baseline scenario. Differences between baseline and future scenarios show the change in suitable habitat area predicted for each family under possible future conditions.
Results
Anomalies in SST in the Australian EEZ varied according to the climate model and emission scenario used (Figure 1 and Table 1). The mean SST anomaly for all climate models was 2.27°C (SD: 0–1.2) for RCP 4.5 and 3.78°C (SD: 0–1.21) for RCP 8.5. Our results show that the predicted mean SST anomaly ranged from minima of 0.93°C (for climate model GISS-E2-R, RCP 4.5) and 0.69°C (for climate model HADGEM2-AO, RCP 8.5), to mean maxima of 1.83°C (for climate model IPSL-CM5A-MR for both emission scenarios) at the end of the century (Figure 1 and Table 1). The climate model MPI-ESM_LR resulted in the maximum SST anomaly projected by all climate models (3.76°C for RCP 4.5 and 5.71°C for RCP 8.5, respectively). Despite model-to-model variation in the magnitude of anomalies, all climate models predicted south-eastern Australia would experience the greatest SST increases by the end of the century (Figure 1).
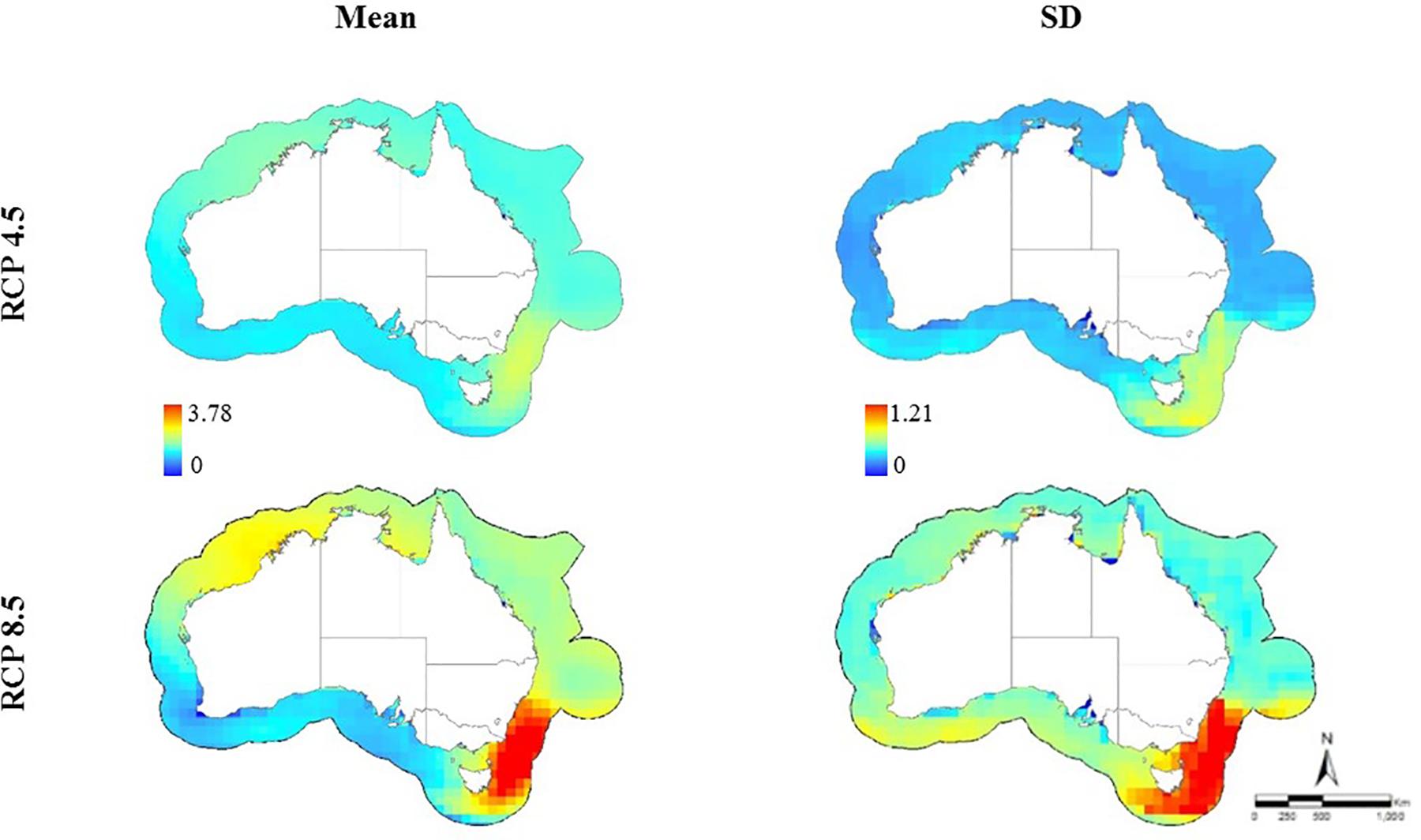
Figure 1. Anomalies in sea surface temperatures throughout the Australian EEZ for the end of the twenty-first century (2050–2099) relative to the baseline period (1956–2005) under two emission scenarios (RCP 4.5 mean anomaly: 0–2.27, SD: 0–1.2; RCP 8.5 mean anomaly: 0–3.78, SD: 0–1.21).
Both the baseline and future habitat suitability models explained slightly higher deviance for requiem than mackerel sharks but all values were around 30% (Supplementary Table S1). The baseline models explained 31.13 and 27.33% for requiem and mackerel sharks, respectively. The future models explained 29.91 and 31.76% for RCP 4.5 and RCP 8.5, respectively for requiem, and 26.47 and 26.22% for RCP 4.5 and RCP 8.5, respectively for mackerel sharks. The resulting predicted habitat suitability maps are presented as the mean across all climate models for requiem (Figure 2) and mackerel sharks (Figure 3), with the predicted change per climate model presented in Figures 4, 5. Predicted habitat suitability was highly dependent on the climate model used, with similar baseline values for requiem and mackerel sharks (0.65 and 0.63, respectively). For both requiem and mackerel sharks, the maximum habitat suitability (∼0.8) was predicted by climate model NORESM1-ME under both emission scenarios (Figure 4). Regions where habitat was predicted to be suitable (i.e., ≥0.5) at the end of the century varied by family, with southern Australia suitable for mackerel sharks, and north-eastern Australia for requiem sharks (Figures 2, 3).
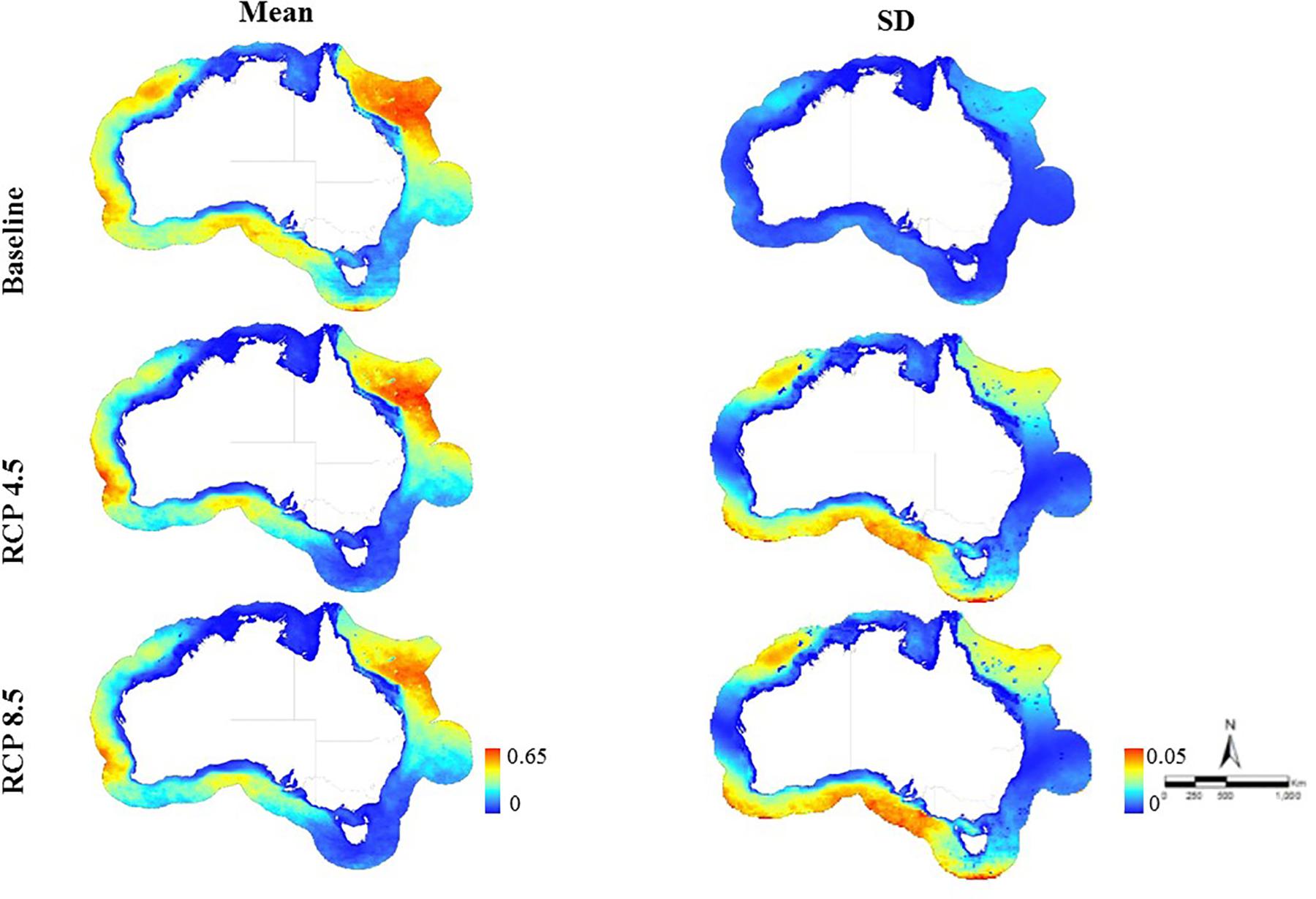
Figure 2. Predicted habitat suitability for requiem sharks across the Australian EEZ for baseline time period (1956–2005; mean habitat suitability: 0–0.65, SD: 0–0.02) and at the end of the twenty-first century (2050–2099) under two emission scenarios (RCP 4.5 mean habitat suitability: 0–0.62, SD: 0–0.04; RCP 8.5 mean habitat suitability: 0–0.6, SD: 0–0.05).
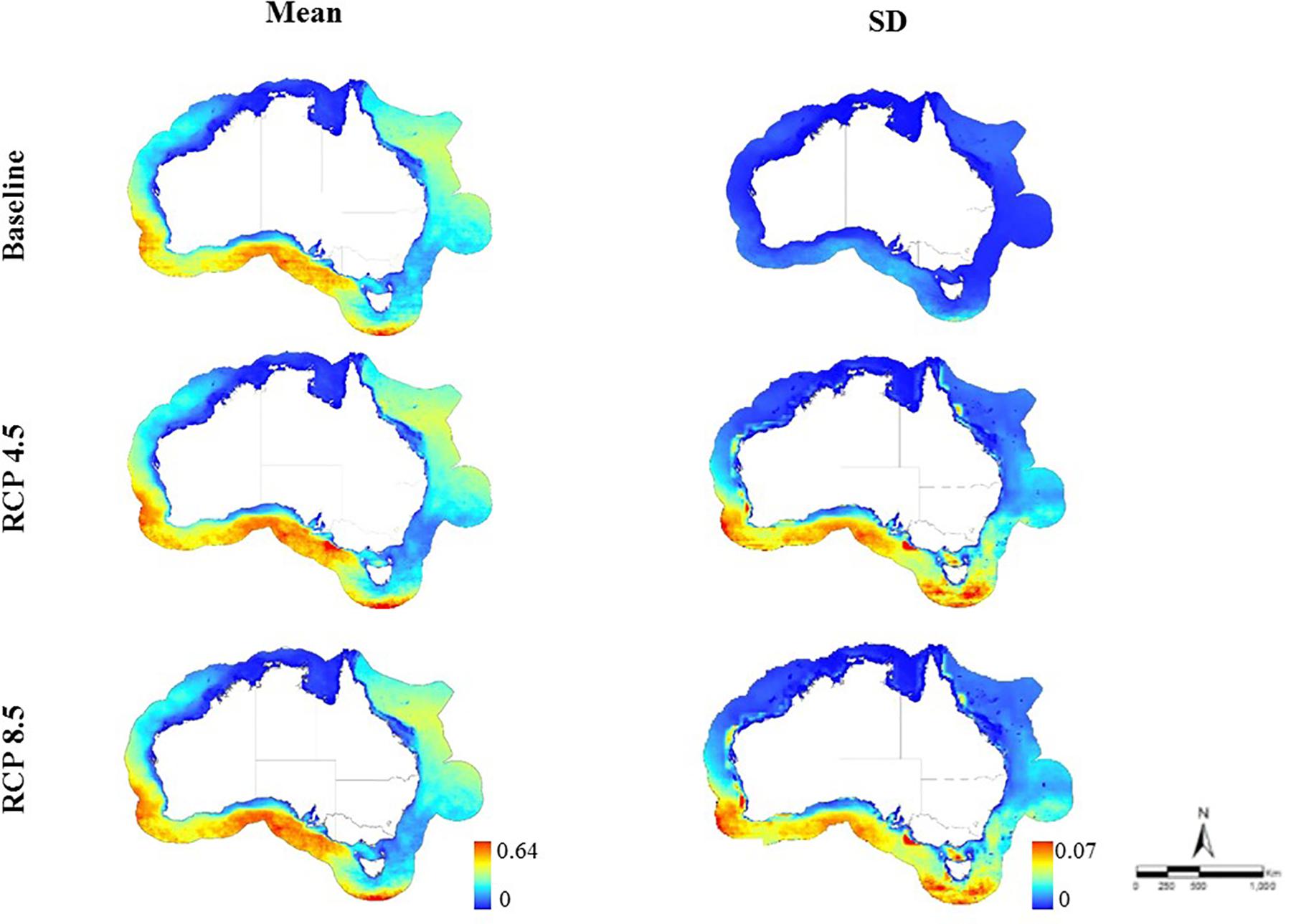
Figure 3. Predicted habitat suitability for mackerel sharks in the Australian EEZ for baseline time period (1956–2005; mean habitat suitability: 0–0.63, SD: 0–0.02) and at the end of the twenty-first century (2050–2099) under two emission scenarios (RCP 4.5 mean habitat suitability: 0–0.64, SD: 0–0.05; RCP 8.5 mean: 0–0.62, SD: 0–0.07).
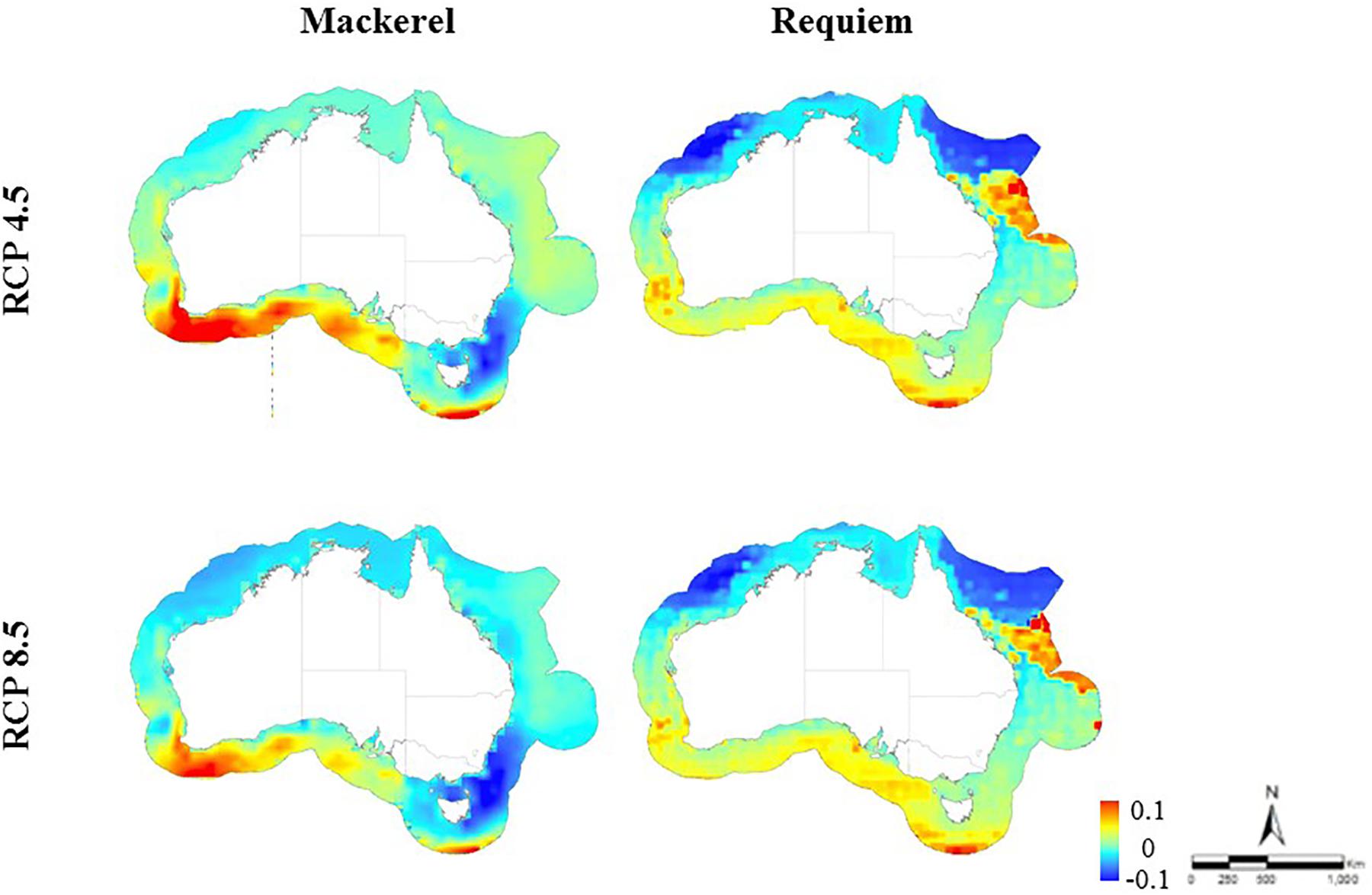
Figure 4. Change in predicted habitat suitability for requiem and mackerel sharks in the Australian EEZ between the baseline time period (1956–2005) and at the end of the twenty-first century (2050–2099) under two emission scenarios (RCP 4.5 and 8.5).
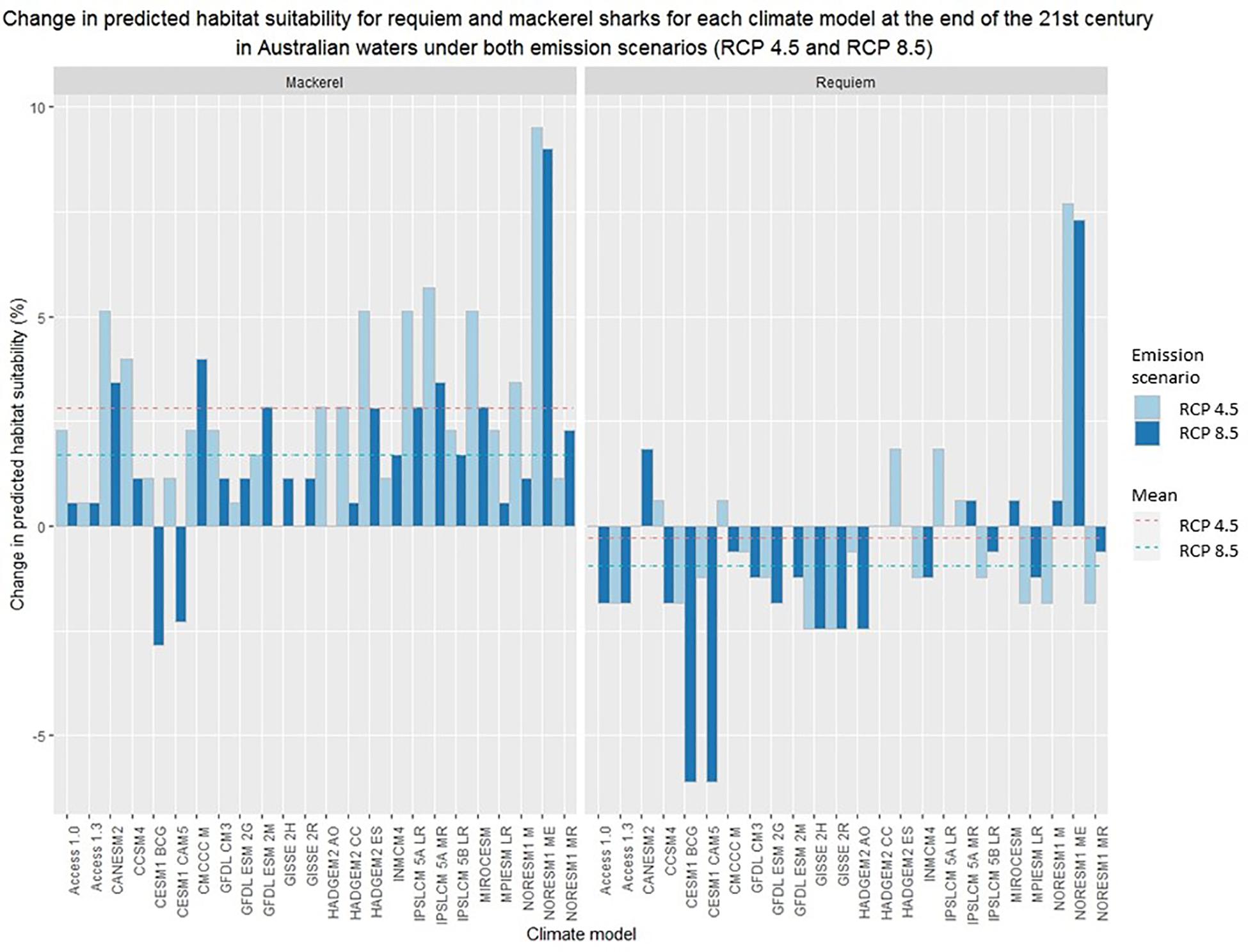
Figure 5. Change in predicted habitat suitability averaged across all cells for requiem and mackerel sharks for each climate model for the end of the 21st century (2050–2099) in continental Australian waters under two emission scenarios (RCP 4.5 and 8.5). Refer to Table 1 for model name abbreviations.
Based on 48 climate simulations, our results suggest a shift in suitable habitat for both requiem and mackerel sharks within the Australian EEZ in the last half of the twenty-first century (2050–2099). The severity and direction of this shift varied, with suitable habitat for requiem sharks predicted to decrease under most climate models, while habitat suitability for mackerel sharks varied to a greater degree depending on the climate model and emission scenario. On average, predicted suitable habitat for requiem sharks under RCP 4.5 extended south on the north-eastern (∼600 km) and south-western coast (∼200 km), but decreased in the north-west (∼400 km). For RCP 8.5, suitable habitat was projected to extend south on the north-eastern coast (∼650 km) and decrease across the north-west (∼500 km) with similar increases on the south-western coast (Figure 2). For mackerel sharks, the average of all climate models predicted an increase in suitable habitat across on the southern coast (∼900 km) and off the southern extent of the EEZ south of Tasmania (∼400 km) for RCP 4.5, with increases also projected to occur under RCP 8.5 (∼700 km across and ∼200 km south along the southern coast and ∼150 km south off the southern extent of the EEZ south of Tasmania) (Figures 3, 4).
Discussion
Significant shifts in the distributions of marine organisms are being observed in the global ocean due to anthropogenic climate change (Poloczanska et al., 2013). Our results highlight that shifts in the location of suitable habitat for requiem and mackerel sharks by the end of the century are to be expected, with a decrease in predicted suitable habitat for requiem sharks off the south-western coast under both emission scenarios. This agrees with predicted habitat shifts for silky, blue (both requiem family; Cheung et al., 2015; Lezama-Ochoa et al., 2016), and mako sharks (mackerel family; Hazen et al., 2013) in other areas. The waters of south-western and south-eastern Australia are warming at an increased rate, almost three and four times higher than the global average, respectively (Hartmann et al., 2013; Robinson et al., 2015a) as indicated in Figure 1. Our models predict that this area will become unsuitable for both requiem and mackerel sharks, likely due to the water temperatures at the end of the century exceeding the thermal tolerance of these pelagic sharks. In our analysis, and those of Robinson et al. (2015b) and Hobday (2010), southward shifts in suitable habitat for blue and mako sharks on the eastern coast of Australia are predicted. This is in line with ocean climate zones (areas with distinct climate, based on annual SST values) shifting southwards by 200 km along the north-eastern coast and approximately 100 km along the north-western coast in tropical Australian waters (Lough, 2008). In the north Pacific Ocean suitable habitat loss was predicted for both blue and mako sharks by the end of the century (Hazen et al., 2013). Such differences in predictions may be due to currents and northern latitude prey species being able to migrate poleward along the coastline (Perry et al., 2005). Due to the east-west orientation of the temperate Australian coastline and limited continental shelf area to the south of the continent (Urban, 2015), there are few opportunities for continental shelf marine organisms, including fish that are shark prey species, to move to higher latitudes and avoid increased water temperatures. Even with suitable habitat available for pelagic sharks within Australian waters these predators will follow prey species, such as tuna (Hobday and Poloczanska, 2010), which are expected to decline in the tropics and shift poleward in response to a warming ocean (Erauskin-Extramiana et al., 2019).
Although relatively little is known about how elevated temperatures will affect sharks (Pistevos et al., 2015), pelagic sharks are vulnerable to climate change impacts (Jones and Cheung, 2018) and life history strategies may play a part in determining ultimate patterns of species distribution. For relatively sedentary, benthic shark species, exposure to projected end-of-century temperatures has been shown to result in both positive and negative impacts. Port Jackson sharks (Heterodontus portusjacksoni) exposed to elevated temperatures exhibited an increase in mortality, altered behavior, increased learning performance and feeding, but reduced growth and embryonic development time (Pistevos et al., 2015; Vila et al., 2018, 2019). Conversely, brownbanded bamboo sharks (Chiloscyllium punctatum) showed decreased survival alongside significantly increased embryonic growth and ventilation rates (Rosa et al., 2014), while juvenile epaulet sharks (Hemiscyllium ocellatum) showed significantly decreased growth rates and 100% mortality (Gervais et al., 2018). It is likely that the physiological impacts of increasing ocean temperature will be greater for more active pelagic sharks than for benthic species (Rosa et al., 2014), given their reliance on ram ventilation and continuous movement (Lawson et al., 2019). Sharks already at their provisioning limit may be faced with starvation if temperature-driven increases in metabolic rates are not met with higher food intake (Pistevos et al., 2015), and this risk will be heightened should environmental perturbations concurrently influence prey availability and abundance. However, the thermal tolerance of requiem and mackerel sharks (Francis and Stevens, 2002; Last and Stevens, 2009; Corrigan et al., 2018; Hueter et al., 2018; Young and Carlson, 2020) may enable them to cope with changing temperatures.
Even though we predicted an overall increase in the amount of suitable habitat for mackerel sharks at the end of the 21st century, temperature acclimatization comes with an energetic cost that impacts other functions such as reproduction, growth, foraging and swimming. Changes in the marine environment may result in novel ecosystems requiring predators to alter foraging behaviors and adapt to new prey species (Nagelkerken and Munday, 2016; Rivest et al., 2019). Under such stresses, individuals become less competitive with decreases in reproduction and population density (Beaugrand and Kirby, 2018) and may exploit habitat heterogeneity by undertaking vertical migrations to suitable temperatures to maximize biological efficiency and minimize physiological adjustment costs (Chin et al., 2010; Beaugrand and Kirby, 2018). The endothermic ability to swim faster and farther (Watanabe et al., 2015) may allow mackerel sharks to migrate longer distances and forage over wider areas with greater access to prey and seasonal resources, although at higher energetic costs than ectothermic species. However, the ability of pelagic sharks to move and follow the shifting suitable habitat outside their current ranges, may potentially alter their interactions with fisheries. It is worth noting that latitudinal species shifts in response to warming can be misleading with some pelagic species migrating vertically not latitudinally (Perry et al., 2005; Beaugrand and Kirby, 2018) and this may be the case with some pelagic shark species. In Australian waters, pelagic sharks have been recorded regulating their depth to occupy regions of favorable temperatures, although this behavior could also be related to prey movements (Rogers et al., 2009; Stevens et al., 2010; Heard et al., 2017) as well as habitat suitability.
Our study predicts changes in habitat suitability for pelagic sharks in the Australian EEZ, but predictions at the end of the century are highly dependent on the climate model and emission scenario chosen to represent future conditions. This has been the case for similar studies on other species, for example, freshwater fish assemblages (Buisson et al., 2010) and mesopelagic lanternfish in the Southern Ocean (Freer et al., 2019) highlighting the benefit of using an ensemble approach to capture high climate uncertainty. Moreover, the SST anomalies across the Australian EEZ also vary according to the climate model and emission scenario used in the analysis. Our analysis was done at the family level due to the sample size available. Analysis at family level, whilst valuable for relatively homogenous species groups, inevitably results in loss of information at lower taxonomic levels. Further research is needed in more localized areas, including telemetry studies on single species, to add greater certainty to species distribution model predictions. There is no consensus about how turbidity may vary under a changing climate, and in our models we assumed that turbidity levels would remain stable at the end of the century. However, a predicted increase in extreme rainfall influenced by changes in atmospheric circulation may increase coastal turbidity due to terrestrial-derived nutrients and pollutant input (Harley et al., 2006). Additionally, turbidity is correlated with chlorophyll-a in pelagic systems, and warmer water temperatures drive phytoplankton blooms, with elevated temperatures increasing both cyanobacterial and algal chlorophyll-a concentrations (Lürling et al., 2018; Trombetta et al., 2019). As aquatic nutrients have a greater impact on chlorophyll-a concentrations than temperature, and salinity and wind are also correlated with plankton blooms (Lürling et al., 2018; Trombetta et al., 2019), the impact this may have on pelagic systems in Australian waters is still unclear. Despite the uncertainties associated with predicting future conditions, studies such as ours using remotely sensed environmental information and occurrence data from fisheries over a large spatial scale, are important to understand how pelagic species with broad geographic ranges might fare in the future. Such studies are a first component of broader research in which the distribution of multiple species are predicted in a likely altered future marine environment.
Data Availability Statement
All datasets generated for this study are included in the article/Supplementary Material.
Author Contributions
CB, AS, and JP conceived the study. CB, JP, AS, and LS designed the methodology with assistance from JF. CB collated and analyzed the data and led the writing of the manuscript with significant contributions from AS. All authors contributed critically to the drafts and gave final approval for publication.
Funding
CB was supported by a Research Training Scheme scholarship and a Robson and Robertson Award from the Jock Clough Marine Foundation. AS was supported by an ARC grant DE170100841 and research funds from Australian Institute of Marine Science (AIMS).
Conflict of Interest
The authors declare that the research was conducted in the absence of any commercial or financial relationships that could be construed as a potential conflict of interest.
Acknowledgments
We thank R. Summerson for assistance with accessing the fisheries data and acknowledge Australian Bureau of Agricultural and Resource Economics and Sciences (ABARES) as the source of the fisheries data, originally supplied by Australian Fisheries Management Authority (AFMA) and state fisheries management agencies.
Supplementary Material
The Supplementary Material for this article can be found online at: https://www.frontiersin.org/articles/10.3389/fmars.2020.00570/full#supplementary-material
References
Barange, M., King, J., Valdes, L., and Turra, A. (2016). The evolving and increasing need for climate change research on the oceans. ICES J. Mar. Sci. 73, 1267–1271. doi: 10.1093/icesjms/fsw052
Barnosky, A. D., Matzke, N., Tomiya, S., Wogan, G. O. U., Swartz, B., Quental, T. B., et al. (2011). Has the Earth’s sixth mass extinction already arrived? Nature 471, 51–57. doi: 10.1038/nature09678
Beaugrand, G., and Kirby, R. R. (2018). How do marine pelagic species respond to climate change? Theories and observations. Ann. Rev. Mar. Sci. 10, 169–197. doi: 10.1146/annurev-marine-121916-063304
Bernal, D., Carlson, J. K., Goldman, K. J., and Lowe, C. G. (2012). “Energetics, metabolism, and endothermy in sharks and rays,” in Biology of Sharks and Their Relatives, eds J. C. Carrier, J. A. Musick, and M. R. Heithaus (Boca Raton, FL: CRC Press), 211–237.
Birkmanis, C. A., Partridge, J. C., Simmons, L. W., Heupel, M. R., and Sequeira, A. M. M. (2020). Shark conservation hindered by lack of habitat protection. Glob. Ecol. Conserv. 21:e00862. doi: 10.1016/j.gecco.2019.e00862
Bruno, J. F., Bates, A. E., Cacciapaglia, C., Pike, E. P., Amstrup, S. C., Van Hooidonk, R., et al. (2018). Climate change threatens the world’s marine protected areas. Nat. Clim. Chang. 8, 499–503. doi: 10.1038/s41558-018-0149-2
Buisson, L., Grenouillet, G., Casajus, N., and Lek, S. (2010). Predicting the potential impacts of climate change on stream fish assemblages. Am. Fish. Soc. Symp. 73, 327–346.
Cheung, W. W. L., Brodeur, R. D., Okey, T. A., and Pauly, D. (2015). Projecting future changes in distributions of pelagic fish species of Northeast Pacific shelf seas. Prog. Oceanogr. 130, 19–31. doi: 10.1016/j.pocean.2014.09.003
Chin, A., Kyne, P. M., Walker, T. I., and McAuley, R. B. (2010). An integrated risk assessment for climate change: analysing the vulnerability of sharks and rays on Australia’s Great Barrier Reef. Glob. Chang. Biol. 16, 1936–1953. doi: 10.1111/j.1365-2486.2009.02128.x
Cisneros-Montemayor, A. M., Barnes-Mauthe, M., Al-Abdulrazzak, D., Navarro-Holm, E., and Sumaila, U. R. (2013). Global economic value of shark ecotourism: Implications for conservation. Oryx 47, 381–388. doi: 10.1017/S0030605312001718
Corrigan, S., Lowther, A. D., Beheregaray, L. B., Bruce, B. D., Cliff, G., Duffy, C. A., et al. (2018). Population connectivity of the highly migratory shortfin mako (Isurus oxyrinchus Rafinesque 1810) and implications for management in the Southern Hemisphere. Front. Ecol. Evol. 6:187. doi: 10.3389/fevo.2018.00187
Cortés, E. (2000). Life history patterns and correlations in sharks. Rev. Fish. Sci. 8, 299–344. doi: 10.1080/10641260008951115
Doney, S. C., Ruckelshaus, M., Duffy, J. E., Barry, J. P., Chan, F., English, C. A., et al. (2012). Climate change impacts on marine ecosystems. Ann. Rev. Mar. Sci. 4, 11–37. doi: 10.1146/annurev-marine-041911-111611
Dulvy, N. K. N., Sadovy, Y., and Reynolds, J. D. (2003). Extinction vulnerability in marine populations. Fish Fish. 4, 25–64. doi: 10.1046/j.1467-2979.2003.00105.x
Dulvy, N. K. N., Simpfendorfer, C. A., Davidson, L. N. K., Fordham, S. V., Bräutigam, A., Sant, G., et al. (2017). Challenges and priorities in shark and ray conservation. Curr. Biol. 27, 565–572. doi: 10.1016/j.cub.2017.04.038
Earth Systems Research Laboratory (2014). Climate Change Web Portal. NOAA’s Ocean Clim. Chang. Web Portal. Available online at: www.esrl.noaa.gov/psd/ipcc/ (accessed January 10, 2018).
Erauskin-Extramiana, M., Arrizabalaga, H., Hobday, A. J., Cabré, A., Ibaibarriaga, L., Arregui, I., et al. (2019). Large-scale distribution of tuna species in a warming ocean. Glob. Chang. Biol. 19:gcb.14630. doi: 10.1111/gcb.14630
Francis, M. P., and Stevens, J. D. (2002). Reproduction, embryonic development, and growth of the porbeagle shark, Lamna nasus, in the southwest Pacific Ocean. Fish. Bull. 98, 1–120. doi: 10.1007/b136546
Freer, J. J., Partridge, J. C., Tarling, G. A., Collins, M. A., and Genner, M. J. (2017). Predicting ecological responses in a changing ocean: the effects of future climate uncertainty. Mar. Biol. 165:7. doi: 10.1007/s00227-017-3239-1
Freer, J. J., Tarling, G. A., Collins, M. A., Partridge, J. C., and Genner, M. J. (2019). Predicting future distributions of lanternfish, a significant ecological resource within the Southern Ocean. Divers. Distrib. 25, 1259–1272. doi: 10.1111/ddi.12934
Garcia, V. B., Lucifora, L. O., and Myers, R. A. (2008). The importance of habitat and life history to extinction risk in sharks, skates, rays and chimaeras. Proc. R. Soc. B Biol. Sci. 275, 83–89. doi: 10.1098/rspb.2007.1295
Garciá Molinos, J., Halpern, B. S., Schoeman, D. S., Brown, C. J., Kiessling, W., Moore, P. J., et al. (2016). Climate velocity and the future global redistribution of marine biodiversity. Nat. Clim. Chang. 6, 83–88. doi: 10.1038/nclimate2769
Gattuso, J. P., Magnan, A., Billé, R., Cheung, W. W. L., Howes, E. L., Joos, F., et al. (2015). Contrasting futures for ocean and society from different anthropogenic CO2 emissions scenarios. Science 349:4722. doi: 10.1126/science.aac4722
GBIF.org (2017). GBIF Occurrence Download. GBIF Occur. Download. Available online at: https://doi.org/10.15468/dl.1ij1w6 (accessed November 15, 2017).
Gervais, C. R., Nay, T. J., Renshaw, G., Johansen, J. L., Steffensen, J. F., and Rummer, J. L. (2018). Too hot to handle? Using movement to alleviate effects of elevated temperatures in a benthic elasmobranch, Hemiscyllium ocellatum. Mar. Biol. 165, 1–12. doi: 10.1007/s00227-018-3427-7
Gilman, E., Clarke, S. C., Brothers, N., Alfaro-Shigueto, J., Mandelman, J., Mangel, J., et al. (2008). Shark interactions in pelagic longline fisheries. Mar. Policy 32, 1–18. doi: 10.1016/j.marpol.2007.05.001
Harley, C. D. G., Hughes, A. R., Hultgren, K. M., Miner, B. G., Sorte, C. J. B., Thornber, C. S., et al. (2006). The impacts of climate change in coastal marine systems. Ecol. Lett. 9, 228–241. doi: 10.1111/j.1461-0248.2005.00871.x
Hartmann, D., Klein, T. A., Rusticucci, M., Alexander, L., Brönnimann, S., Charabi, Y., et al. (2013). in Climate Change 2013 the Physical Science Basis: Working Group I Contribution to the Fifth Assessment Report of the Intergovernmental Panel on Climate Change, eds T. Stocker, D. Qin, G.-K. Plattner, M. Tignor, S. Allen, J. Boschung, et al. (Cambridge: Cambridge University Press), doi: 10.1017/CBO9781107415324.008
Hazen, E. L., Jorgensen, S., Rykaczewski, R. R., Bograd, S. J., Foley, D. G., Jonsen, I. D., et al. (2013). Predicted habitat shifts of Pacific top predators in a changing climate. Nat. Clim. Chang. 3, 234–238. doi: 10.1038/nclimate1686
Heard, M., Rogers, P. J., Bruce, B. D., Humphries, N. E., and Huveneers, C. (2017). Plasticity in the diel vertical movement of two pelagic predators (Prionace glauca and Alopias vulpinus) in the southeastern Indian Ocean. Fish. Oceanogr. 27, 199–211. doi: 10.1111/fog.12245
Heupel, M. R., Simpfendorfer, C. A., Espinoza, M., Smoothey, A. F., Tobin, A., and Peddemors, V. (2015). Conservation challenges of sharks with continental scale migrations. Front. Mar. Sci. 2:12. doi: 10.3389/fmars.2015.00012
Hobday, A. J. (2010). Ensemble analysis of the future distribution of large pelagic fishes off Australia. Prog. Oceanogr. 86, 291–301. doi: 10.1016/j.pocean.2010.04.023
Hobday, A. J., and Poloczanska, E. S. (2010). Adapting Agriculture to Climate Change: Preparing Australian Agriculture, Forestry and Fisheries for the Future, eds C. Stokes and M. Howden (Collingwood, VIC: CSIRO Publishing).
Hoegh-Guldberg, O., and Bruno, J. F. (2010). The impact of climate change on the world’s marine ecosystems. Science 328, 1523–1528. doi: 10.1126/science.1189930
Hueter, R. E., Tyminski, J. P., Pina-Amargós, F., Morris, J. J., Abierno, A. R., Valdés, J. A. A., et al. (2018). Movements of three female silky sharks (Carcharhinus falciformis) as tracked by satellite-linked tags off the Caribbean coast of Cuba. Bull. Mar. Sci. 94, 345–358. doi: 10.5343/bms.2017.1162
Huveneers, C., Meekan, M. G., Apps, K., Ferreira, L. C., Pannell, D., and Vianna, G. M. S. (2017). The economic value of shark-diving tourism in Australia. Rev. Fish Biol. Fish. 27, 665–680. doi: 10.1007/s11160-017-9486-x
IMOS (2016). Ocean Acidification Historical Reconstruction–Temperature. Available online at: https://portal.aodn.org.au/ (accessed October 15, 2018).
IPCC (2013). “2013: Technical Summary,” in Climate Change 2013: The Physical Science Basis. Contribution of Working Group I to the Fifth Assessment Report of the Intergovernmental Panel on Climate Change, eds T. F. Stocker, Q. Dahe, G.-K. Plattner, L. V. Alexander, S. K. Allen, N. L. Bindoff, et al. (Cambridge: Cambridge University Press), 33–115. doi: 10.1017/CBO9781107415324.005
IUCN (2020). The IUCN Red List of Threatened Species. Available online at: www.iucnredlist.org (accessed January 6, 2020).
James, G., Witten, D., Hastie, T., and Tibshirani, R. (2015). An Introduction to Statistical Learning With Applications in R. Berlin: Springer, 618. doi: 10.1016/j.peva.2007.06.006
Jones, M. C., and Cheung, W. W. L. (2018). Using fuzzy logic to determine the vulnerability of marine species to climate change. Glob. Chang. Biol. 24, e719–e731. doi: 10.1111/gcb.13869
Last, P. R., and Stevens, J. D. (2009). Sharks and Rays of Australia, 2nd Edn. Collingwood, VIC: CSIRO Publishing.
Last, P. R., White, W. T., Gledhill, D. C., Hobday, A. J., Brown, R., Edgar, G. J., et al. (2011). Long-term shifts in abundance and distribution of a temperate fish fauna: a response to climate change and fishing practices. Glob. Ecol. Biogeogr. 20, 58–72. doi: 10.1111/j.1466-8238.2010.00575.x
Lawson, C. L., Halsey, L. G., Hays, G. C., Dudgeon, C. L., Payne, N. L., Bennett, M. B., et al. (2019). Powering ocean giants: The energetics of shark and ray megafauna. Trends Ecol. Evol. 19, 1–13. doi: 10.1016/j.tree.2019.07.001
Lezama-Ochoa, N., Murua, H., Chust, G., Van Loon, E., Ruiz, J., Hall, M., et al. (2016). Present and future potential habitat distribution of Carcharhinus falciformis and Canthidermis maculata by-catch species in the tropical tuna purse-seine fishery under climate change. Front. Mar. Sci. 3:34. doi: 10.3389/fmars.2016.00034
Lough, J. M. (2008). Shifting climate zones for Australia’s tropical marine ecosystems. Geophys. Res. Lett. 35, 1–5. doi: 10.1029/2008GL034634
Lürling, M., Mello, M. M., van Oosterhout, F., Domis, L., de, S., and Marinho, M. M. (2018). Response of natural cyanobacteria and algae assemblages to a nutrient pulse and elevated temperature. Front. Microbiol. 9:1851. doi: 10.3389/fmicb.2018.01851
Moss, R. H., Edmonds, J. A., Hibbard, K. A., Manning, M. R., Rose, S. K., Van Vuuren, D. P., et al. (2010). The next generation of scenarios for climate change research and assessment. Nature 463, 747–756. doi: 10.1038/nature08823
Nagelkerken, I., and Munday, P. L. (2016). Animal behaviour shapes the ecological effects of ocean acidification and warming: Moving from individual to community-level responses. Glob. Chang. Biol. 22, 974–989. doi: 10.1111/gcb.13167
Oliver, E. C. J., Benthuysen, J. A., Bindoff, N. L., Hobday, A. J., Holbrook, N. J., Mundy, C. N., et al. (2017). The unprecedented 2015/16 Tasman Sea marine heatwave. Nat. Commun. 8, 1–12. doi: 10.1038/ncomms16101
Perry, A. L., Low, P. J., Ellis, J. R., and Reynolds, J. D. (2005). Climate change and distribution shifts in marine fishes. Science 308, 1912–1915. doi: 10.1126/science.1111322
Pinsky, M. L., Eikeset, A. M., McCauley, D. J., Payne, J. L., and Sunday, J. M. (2019). Greater vulnerability to warming of marine versus terrestrial ectotherms. Nature 569, 108–111. doi: 10.1038/s41586-019-1132-4
Pistevos, J. C. A., Nagelkerken, I., Rossi, T., Olmos, M., and Connell, S. D. (2015). Ocean acidification and global warming impair shark hunting behaviour and growth. Sci. Rep. 5:16293. doi: 10.1038/srep16293
Poloczanska, E. S., Brown, C. J., Sydeman, W. J., Kiessling, W., Schoeman, D. S., Moore, P. J., et al. (2013). Global imprint of climate change on marine life. Nat. Clim. Chang. 3, 919–925. doi: 10.1038/nclimate1958
Queiroz, N., Humphries, N. E., Couto, A., Vedor, M., da Costa, I., Sequeira, A. M. M., et al. (2019). Global spatial risk assessment of sharks under the footprint of fisheries. Nature 461–466. doi: 10.1038/s41586-019-1444-4
R Core Team (2017). R: A Language and Environment for Statistical Computing. Available at: https://www.r-project.org/ (accessed January 15, 2017).
Riahi, K., Rao, S., Krey, V., Cho, C., Chirkov, V., Fischer, G., et al. (2011). RCP 8.5–A scenario of comparatively high greenhouse gas emissions. Clim. Change 109, 33–57. doi: 10.1007/s10584-011-0149-y
Rivest, E. B., Jellison, B., Ng, G., Satterthwaite, E. V., Bradley, H. L., Williams, S. L., et al. (2019). Mechanisms involving sensory pathway steps inform impacts of global climate change on ecological processes. Front. Mar. Sci. 6:346. doi: 10.3389/fmars.2019.00346
Roberts, J. J., Best, B. D., Dunn, D. C., Treml, E. A., and Halpin, P. N. (2010). Marine geospatial ecology tools: an integrated framework for ecological geoprocessing with ArcGIS. Python, R, MATLAB, and C++. Environ. Model. Softw. 25, 1197–1207. doi: 10.1016/j.envsoft.2010.03.029
Robinson, L. M., Gledhill, D. C., Moltschaniwskyj, N. A., Hobday, A. J., Frusher, S., Barrett, N., et al. (2015a). Rapid assessment of an ocean warming hotspot reveals “high” confidence in potential species’ range extensions. Glob. Environ. Chang. 31, 28–37. doi: 10.1016/j.gloenvcha.2014.12.003
Robinson, L. M., Hobday, A. J., Possingham, H. P., and Richardson, A. J. (2015b). Trailing edges projected to move faster than leading edges for large pelagic fish habitats under climate change. Deep. Res. Part II Top. Stud. Oceanogr. 113, 225–234. doi: 10.1016/j.dsr2.2014.04.007
Roff, G., Doropoulos, C., Rogers, A., Bozec, Y.-M., Krueck, N. C., Aurellado, E., et al. (2016). The ecological role of sharks on coral reefs. Trends Ecol. Evol. 31, 395–407. doi: 10.1016/j.tree.2016.02.014
Rogers, P. J., Huveneers, C., Page, B., and Goldsworthy, S. (2009). Movement Patterns of Pelagic Sharks in the Southern and Indian Oceans: Determining Critical Habitats and Migration Paths. : SARDI Publication Number F2009/000167-1. Adelaide, SA: South Australian Research and Development Institute.
Rogers, P. J., Huveneers, C., Page, B., Goldsworthy, S. D., Coyne, M., Lowther, A. D., et al. (2015). Living on the continental shelf edge: habitat use of juvenile shortfin makos Isurus oxyrinchus in the Great Australian Bight, southern Australia. Fish. Oceanogr. 24, 205–218. doi: 10.1111/fog.12103
Rosa, R., Baptista, M., Lopes, V., Pegado, M., Ricardo, P. J., Trubenbach, K., et al. (2014). Early-life exposure to climate change impairs tropical shark survival. Proc. R. Soc. B 281:20141738. doi: 10.1098/rspb.2014.1738
Rosa, R., Rummer, J. L., and Munday, P. L. (2017). Biological responses of sharks to ocean acidification. Biol. Lett. 13:20160796. doi: 10.1098/rsbl.2016.0796
Scott, J. D., Alexander, M. A., Murray, D. R., Swales, D., and Eischeid, J. (2016). The climate change web portal: A system to access and display climate and earth system model output from the CMIP5 archive. Bull. Am. Meteorol. Soc. 97, 523–530. doi: 10.1175/BAMS-D-15-00035.1
Simpfendorfer, C., Chin, A., Kyne, P., Rigby, C., Sherman, S., and White, W. (2019). A Report Card for Australia’s Sharks. Townsville, QL: Centre for Sustainable Tropical Fisheries and Aquaculture, James Cook University.
Stevens, J. D., Bradford, R. W., and West, G. J. (2010). Satellite tagging of blue sharks (Prionace glauca) and other pelagic sharks off eastern Australia: depth behaviour, temperature experience and movements. Mar. Biol. 157, 575–591. doi: 10.1007/s00227-009-1343-6
Syndeman, W. J., Poloczanska, E., Reed, T. E., and Thompson, S. A. (2015). Climate change and marine vertebrates. Science 350, 772–777. doi: 10.1126/science.aac9874
Thomson, A. M., Calvin, K. V., Smith, S. J., Kyle, G. P., Volke, A., Patel, P., et al. (2011). RCP4.5: A pathway for stabilization of radiative forcing by 2100. Clim. Change 109, 77–94. doi: 10.1007/s10584-011-0151-4
Tittensor, D. P., Mora, C., Jetz, W., Lotze, H. K., Ricard, D., Berghe, E., et al. (2010). Global patterns and predictors of marine biodiversity across taxa. Nature 466, 1098–1101. doi: 10.1038/nature09329
Trisos, C. H., Merow, C., and Pigot, A. L. (2020). The timing and abruptness of potential ecological disruption from climate change. Nature 20, 1–39. doi: 10.1038/s41586-020-2189-9
Trombetta, T., Vidussi, F., Mas, S., Parin, D., Simier, M., and Mostajir, B. (2019). Water temperature drives phytoplankton blooms in coastal waters. PLoS One 14:214933. doi: 10.1371/journal.pone.0214933
Urban, M. C. (2015). Accelerating extinction risk from climate change. Science 348, 571–573. doi: 10.1126/science.aaa4984
Vila, P. C., Gervais, C., Reed, J., and Brown, C. (2018). Incubation under climate warming affects behavioral lateralisation in Port Jackson sharks. Symmetry (Basel). 10, 1–9. doi: 10.3390/sym10060184
Vila, P. C., Gervais, C., Reed, J., Michard, J., and Brown, C. (2019). Quantity discrimination in Port Jackson sharks incubated under elevated temperatures. Behav. Ecol. Sociobiol. 73:93. doi: 10.1007/s00265-019-2706-8
Vuuren, D. P., van, Edmonds, J., Kainuma, M., Riahi, K., Thomson, A., et al. (2011). The representative concentration pathways: an overview. Clim. Chang. 109, 5–31. doi: 10.1007/s10584-011-0148-z
Watanabe, Y. Y., Goldman, K. J., Caselle, J. E., Chapman, D. D., and Papastamatiou, Y. P. (2015). Comparative analyses of animal-tracking data reveal ecological significance of endothermy in fishes. Proc. Natl. Acad. Sci. U.S.A. 112, 6104–6109. doi: 10.1073/pnas.1500316112
Yokoi, H., Ijima, H., Ohshimo, S., and Yokawa, K. (2017). Impact of biology knowledge on the conservation and management of large pelagic sharks. Sci. Rep. 7:10619. doi: 10.1038/s41598-017-09427-3
Keywords: sea surface temperature, climate change, marine ecosystems, species distribution models, global warming, Lamnidae, Carcharhinidae
Citation: Birkmanis CA, Freer JJ, Simmons LW, Partridge JC and Sequeira AMM (2020) Future Distribution of Suitable Habitat for Pelagic Sharks in Australia Under Climate Change Models. Front. Mar. Sci. 7:570. doi: 10.3389/fmars.2020.00570
Received: 07 February 2020; Accepted: 22 June 2020;
Published: 14 July 2020.
Edited by:
Darren Pilcher, University of Washington and NOAA/PMEL, United StatesReviewed by:
Stephanie Brodie, University of California, Santa Cruz, United StatesBrad Wetherbee, The University of Rhode Island, United States
Copyright © 2020 Birkmanis, Freer, Simmons, Partridge and Sequeira. This is an open-access article distributed under the terms of the Creative Commons Attribution License (CC BY). The use, distribution or reproduction in other forums is permitted, provided the original author(s) and the copyright owner(s) are credited and that the original publication in this journal is cited, in accordance with accepted academic practice. No use, distribution or reproduction is permitted which does not comply with these terms.
*Correspondence: Charlotte A. Birkmanis, Y2hhcmxvdHRlLmJpcmttYW5pc0ByZXNlYXJjaC51d2EuZWR1LmF1