- 1Department of Biology, Norwegian University of Science and Technology (NTNU), Trondheim, Norway
- 2SINTEF Ocean, Trondheim, Norway
With the increasing interest and activities regarding seaweed cultivation in Europe, it is becoming crucial to utilize the opportunities that lies within environmental resources, such as light and nutrients, to produce biomass of a high yield and quality. The chemical composition of seaweed varies between seasons and depths as an effect of resource supply and environmental conditions. These factors are particularly important to assess for economically feasible species like the kelp Saccharina latissima. This paper examines how differences in light conditions and nutrient availability affect the growth and intracellular nitrogen in S. latissima. This was done through cultivating sporophytes in land-based tanks with four different combinations of high/low light and high/low nutrient supply over an experimental period of 20 days, with measurements of growth rate and subsequent analysis of tissue nitrogen metabolites. The results revealed that the mean growth rate and the intracellular nitrogen components of the sporophytes were positively related to the external nitrate concentration during the experimental period, indicating that S. latissima requires high nutrient concentration to maintain a rapid growth.
Introduction
Seaweed cultivation is increasing rapidly along the North Atlantic coasts (Stévant et al., 2017; Grebe et al., 2019; Forbord et al., 2020), while farming of seaweed has been an established industry for decades in many Asian countries, producing over 30 million metric tons per year (FAO, 2020). Seaweed biomass is mainly used for human consumption and in the phycocolloid industry, but it also has a wide range of applications as feed ingredients, fertilizers, biofuels, and biochemicals (Skjermo et al., 2014; Barbier et al., 2019; Buschmann and Camus, 2019). Deposition of seaweed biomass has even been considered for future carbon dioxide removal (CDR, Krause-Jensen et al., 2018).
One of the most common and promising species for large scale cultivation in Norway is the kelp Saccharina latissima (Linnaeus) with a production of 174 metric tons in 2018 and 66 metric tons in 2019 (Directorate of Fisheries 2020). It has a high potential of growth (Handå et al., 2013; Peteiro and Freire, 2013; Bak et al., 2018; Sharma et al., 2018), high content of valuable components (Holdt and Kraan, 2011; Schiener et al., 2015; Bak et al., 2019) and a well described life cycle (Flavin et al., 2013; Redmond et al., 2014; Forbord et al., 2018). The growth rate of S. latissima varies over the seasons and the important factors for controlling growth are believed to be light intensity, environmental nutrient concentration, temperature, salinity, and seawater currents (Bartsch et al., 2008; Broch and Slagstad, 2012; Xiao et al., 2019). In the same way that macroalgae have adapted to various light and temperature regimes, they have also adapted to different ambient nutrient levels. Because S. latissima can store nutrients as internal nitrate in the vacuoles (Hurd et al., 2014) and grow at relatively low temperatures, they benefit from growing in temperate areas where the nutrient availability varies over the seasons (Pedersen and Borum, 1996; Gordillo, 2012).
Nitrogen (N) can exist in different forms in seawater; as an inorganic form like nitrate (NO3–), nitrite (NO2–), or ammonium (NH4+); or as part of organic substances like dissolved (DON) or particulate (PON) nitrogen (Capone et al., 2008; Hurd et al., 2014). In temperate regions, the ambient concentration of NO3– will vary with depth and season, and will normally show an inverse trend to temperature, with a reduction in spring and summer due to uptake by phytoplankton (Rey et al., 2007; Young et al., 2007; Ibrahim et al., 2014; Broch et al., 2019). The opposite pattern is common for DON and PON (Sipler and Bronk, 2015). For most temperate areas, NO3– concentration will vary from undetectable to around 420 μg L–1 (Hurd et al., 2014). In the Trondheimsfjord (Central Norway) the concentration of NO3– is typically around 140 μg L–1 at 70 m depth across all seasons (Forbord et al., 2012), and remain at this level for deeper depths. This is a typical feature of Norwegian North East Atlantic deep-water.
Light intensity affects the N uptake indirectly because the demanded energy for the active uptake and fixating of N to molecules and proteins comes from photosynthesis (Lapointe and Tenore, 1981; Hurd et al., 2014). It has been shown that S. latissima grown between 10 and 15°C at a high light intensity of 250 μmol m–2 s–1 showed a 50% lower growth rate compared with kelps grown at 110 μmol m–2 s–1, which was found to be the optimum for photon flux in this temperature interval (Fortes and Lüning, 1980). The degree of photo inhibition under conditions of high light exposure was shown to be proportional to light intensity and exposure time (Bruhn and Gerard, 1996). Chapman et al. (1978) found that photosynthesis in S. latissima increased with increasing internal NO3– pools. Moreover, Chapman and Craigie (1977) found that the growth of Laminaria longicruris in temperate seawater increased during spring as a result of the high environmental NO3– concentrations during winter, assuming storage of NO3– in the tissues followed by depletion of NO3– reserves and decreased growth during the summer months.
The aim of this experiment was to examine how various light conditions and nutrient availability affected the growth and intracellular nitrogen components in cultivated S. latissima. This was carried out by collecting cultivated sporophytes which had been growing for 7 months at sea and later exposing them to different growth environments in land-based tanks. The environments were set up with four different combinations of high/low light intensities and high/low nutrient supply with subsequent analysis of tissue nitrogen metabolites over an experimental period of 20 days. The chemical composition of macroalgae varies between seasons, environmental conditions, and depths, and exploring the relationship between these are particularly important to assess for economically feasible species like S. latissima in different environments.
Materials and Methods
The experiment with S. latissima was carried out in the outdoor research facility of Seaweed Energy Solution AS (63°44′N, 10°34′E) in Trondheim, between May and June 2014.
Experimental Design and Sampling
Collection of Sporophytes and Experimental Set Up
Sporophytes of wild S. latissima population with mature sori were collected near the sea farm (63°42′N, 8°52′E) and shipped to the seaweed laboratory for production of seed lines. Spores were released from the sori and seeded on horizontal ropes. The seeded ropes were cultivated in nutrient-rich seawater in a flow through, light- and temperature-controlled system in the seaweed hatchery, according to Forbord et al. (2018). The incubation time in the laboratory was 8 weeks and the seedling were subsequently deployed at sea in the outer coastal area of Central Norway in the period October–November 2013. In May 2014, after 7 months of sea cultivation, visible epiphyte-free sporophytes were harvested with an average frond length of 45.8 ± 2.4 cm (n = 40), and transported, cold and moist, to the experimental site. Immediately after arrival, the sporophytes were transferred to seawater filled outdoor tanks (r = 73 m, h = 0.75 m, surface area = 1.67 m2, filled water volume = 1111 L) and were later fixed to ropes on PVC frames (100 × 50 cm) with cable ties and placed in the bottom of the tanks. The sporophytes that had visible mechanical damage from the sampling were discarded. The experiment included four treatments, each with two replicate tanks. Two different sources of water were used: surface water to simulate growth in a nutrient depleted environment and deep water to simulate growth in a nutrient saturated environment. Plant cover filters (Axley) were used on top of the experimental tanks to simulate growth at high or low light levels and to ensure that the sporophytes did not suffer from photo inhibition (see Supplementary Figure 1). One layer of plant cover diminished light to 30% compared to no cover and represented high light intensity, while four layers of plant cover represented low light intensity. The treatments combined either surface water or deep water (low and high nutrient concentrations, respectively) and either one or four layers of plant cover filter (high and low light intensity, respectively). This gave four treatments with the following combinations of light intensity and water quality:
S1: Surface water and one layer of plant cover filter (“high light, low nutrient”)
S4: Surface water and four layers of plant cover filter (“low light, low nutrient”)
D1: Deep water and one layer of plant cover filter (“high light, high nutrient”)
D4: Deep water and four layers of plant cover filter (“low light, high nutrient”)
The environmental conditions of S1 represented the low nutrient situation in surface waters during late spring and summer, whereas D4 represented the high nutrient situation in deeper waters. The environmental conditions of S4 and D1 are more uncommon in nature, but were biologically interesting to include.
The surface water was pumped from 1 m and the deep water from 100 m depth from the fjord outside the experimental location. Both water sources were unfiltered. Each tank had an aeration system at the bottom to ensure water circulation and to keep the sporophytes afloat. The inflow rate in the tanks was set to 3 L min–1 and gave a mean hydraulic retention time of roughly 6 h. To even out the variation in temperature that occurred due to the different depth of the water sources, the surface water was pumped through a cooling system placed in the deep-water basin before transported to the experimental tanks. The experimental tanks were placed in an order of 3 × 3 outdoor on a pier with direct temporal exposure sunlight from south and east (Figure 1). One of the replicate tanks for each treatment was placed on the edge of the pier facing east or south and the rest in the middle to spread the light exposure among the replicas.
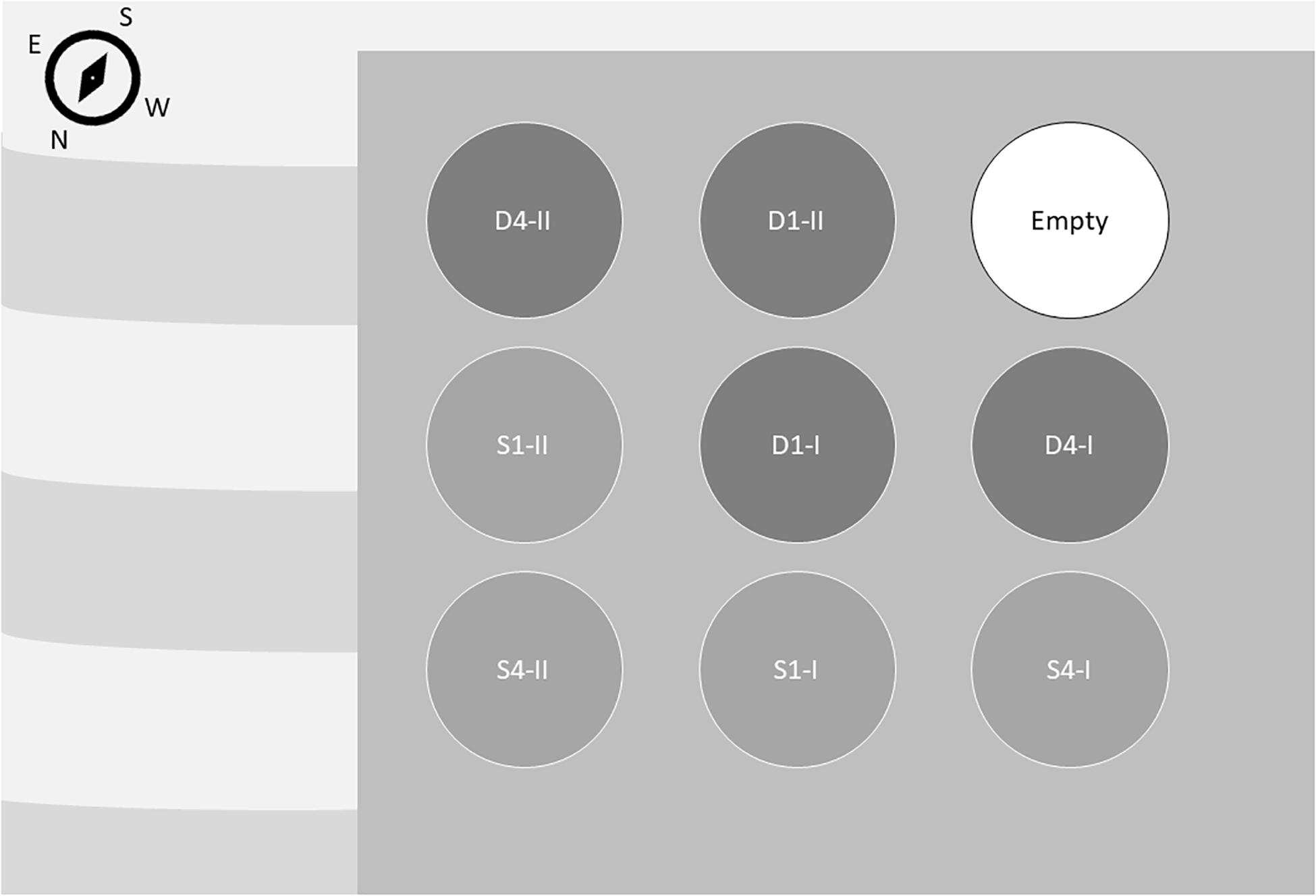
Figure 1. Experimental tank placement. High nutrient treatments (deep water; D) are shown in dark gray circles and low nutrient treatments (surface water; S) are shown in light gray circles. High light treatments are marked with 1, representing one layer of plant cover. Low light treatments are marked with 4. The two replicate tanks are marked with I and II. Stripes illustrate the sea.
Sampling and Measurements
The sporophytes were acclimatized to the new culture conditions for 4 weeks after harvesting from the seafarm and transfer to the experimental tanks. After the start of the experiment, five individuals from each tank were harvested for chemical analysis every fifth day from day 5, in total four times over the experimental period of 20 days. The sporophytes were placed in pre-labeled zip-lock bags and frozen at −18°C until analyzed. Frond elongation was measured using the punch hole method at each sampling day (Parke, 1948). The growth rate in terms of length (mm day–1) in periods between sampling was calculated based on length measurements.
Environmental Conditions
Onset Application HOBO pendant loggers (Bourne, United States; temperature accuracy ± 0.53°C, resolution 0.14°C) placed in the bottom of the tanks were used to log temperature (°C) and light intensity (LUX) every 15 min throughout the experimental period. Light was converted from LUX to PAR with the conversion factor for blue sky only (Lux × 52–1) (Thimijan and Heins, 1983). Light was then averaged for light received between 08:00 and 20:00 to exclude night-time values. One of the loggers malfunctioned during the experiment and light levels were not captured in that tank (S4-I). In addition, the light intensity was measured before start in one of the tanks with one layer of plant cover filter with a spectrophotometer (JAZ, Ocean Optics). The temperature at the surface of the tanks was measured manually once a week as a control. Water samples were taken for nutrient analysis twice a week from the tanks and filtered through a 0.2 μm polysulfone filter before being frozen at −18°C until analyzed.
Chemical Analysis
Five semi-frozen individual sporophytes from each sampling were milled with a kitchen grinder (Kenwood PRO, 1500) or a hand-blender and put back in the freezer.
Dry Weight
The dry weight (DW) was determined by weighing samples of 1.19 ± 0.01 g (mean ± SE) algal material before and after drying for 24 h at 70°C in a Fermaks oven.
Carbon and Nitrogen Analysis
Carbon and nitrogen (CN) content were analyzed for the whole thalli. The samples were dried for 24 h at 70°C in a Fermaks oven and milled (MFC mill, Janke & Kunkel) into a fine powder that was used for further analysis. Samples of 0.4–1.0 mg dried kelp powder were transferred to tin capsules and CN was analyzed in quadruplicate with a Carlo Erba Element Analyzer (model 1106).
Protein Content
The nitrogen component was used to calculate the protein content of the samples by using a nitrogen-to-protein conversion factor (Kp) of 3.8. This factor was determined based on measurements of total amino acids for locally cultivated S. latissima sporophytes (Forbord et al., 2020). Protein results that are further discussed in this article are based on this method. Protein content was also analyzed with the colorimetric Bio-Rad DC Protein Assay according to the manufacturer’s specification (BIO-RAD, 2013). This Bio-Rad method is an improved version of the Lowry assay (Lowry et al., 1951), and the results were compared with the protein estimates based on nitrogen and the Kp factor. In the Bio-Rad protein extraction procedure, semi-thawed algae material (1.16 ± 0.01 g, mean ± SE) were measured and transferred to 15 mL centrifuge tubes (Cellstar) with triplicates from each sample. Sodium hydroxide (NaOH, 0.5 M, 10 mL) was added to the tubes, which were then vortexed before they were frozen in liquid nitrogen. The tubes were thawed at room temperature, boiled for 30 min, cooled to room temperature, and stored in a refrigerator for 24 h. One hour before centrifuging the samples, they were shaken and put in the refrigerator again. The samples were then centrifuged for 15 min (4000 RCF) in a table centrifuge (Kendro megafuge). The supernatants were extracted and kept at −18°C. NaOH (0.5 M, 10 mL) was added to the precipitates, which were then shaken, boiled for 30 min and cooled for 24 h. This was repeated until the supernatants from three rounds were collected in the same tube for each parallel. From the thawed supernatant, 100 μL was pipetted into glass test tubes. There were two parallels for each collection of supernatants. BIO-RAD DC reagent A (500 μL) was added to the test tubes and they were vortexed. Then, 4.0 mL BIO-RAD DC reagent B was added and the tubes were vortexed again. After 15 min the absorbance was read at 750 nm in a spectrophotometer (JENWAY 6715 UV/Vis). Absorbance values were used to calculate the concentration of proteins in the algal material, through comparison with a standard curve of absorbance measurements from known concentrations of bovine serum albumin.
Intracellular Dissolved Nitrate (I-DIN) and Small Molecules of Intracellular Organic Nitrogen (I-DON)
Semi-frozen S. latissima material (0.12 ± 0.00 g; mean ± SE) from each sample was transferred to a test tube with a cork and filled with 12 mL of distilled water. The samples were boiled at 100 °C for 30 min, cooled, and filtered through a 0.45 μm polysulfone syringe filter to remove algal debris. The concentration of intracellular dissolved nitrate (NO3–, NO2–) in 0.6 mL of extract was determined using an auto analyzer (Flow Solution IV System, OI Analytical), according to Norwegian Standard 4745 (NSF, 1975). The remaining 0.6 mL of extract was oxidized by adding sodium hydroxide (3 mL) and potassium peroxide sulfate (12 g L–1), autoclaving for 20 min, and analyzing for nitrate using the auto analyzer. The small molecules of intracellular organic nitrate (I-DON) were calculated as the difference between the total dissolved nitrate and inorganic nitrate in the same sample.
External Inorganic Nitrate (E-DIN)
The water samples from the experimental tanks were analyzed for dissolved nitrate in the water (E-DIN) using the auto analyzer, as described above. In order to better capture the environment that the sporophytes were grown in, the E-DIN measures were calculated as the moving average of the last five measurements.
Statistics
Variables were checked for normality (using a Shapiro–Wilk test), outliers, and homogen variance between groups (using a Levene’s test). Based on these tests, the variables I-DIN, E-DIN and QN (mg N g–1 DW) were subjected to transformation by the natural logarithm to conform to normality inside groups, no outliers, and homogeneity of the variances. Generalized linear mixed models (GLMMs) were used to check if there were tank specific effects, by including “Tank” as a random effect variable. Bayesian information criterion (BIC) were used to asses model fit. Where models were nested a likelihood ratio test were used to check if the additional variable significantly improved the model fit (α = 0.05). A forward stepwise regression was used to build up the model based on the predictors assumed to affect the variable. “Time” was included as a random effect or a fixed effect. In all cases, model assumptions were checked by diagnostic plots. When there were found no significant random effect from “Tank” or “Time,” linear regression was used. Where there was evidence of heteroscedasticity, a model was fitted using generalized least squares, where it is possible to allow for different variances in the grouping factors. A comparison of protein yield between two different methods, and between QC between water sources was tested by an independent-samples t-test.
All statistical analysis was done in R (3.6.1; R Core Team, 2019) except the independent-samples t-tests, which were run in IBM SPSS Statistical software (Version 25). The significant threshold was α = 0.05. Values are given as an average of the duplicate tanks ± standard error (SE), if not otherwise specified. For values given as median, the variation is given as ± median absolute deviation (MAD). Effect sizes are given as Cohen’s d (Sullivan and Feinn, 2012). In addition to R, plots were made using Systat SigmaPlot software (version 14). The independent variables Light, Temperature and E-DIN all had a significant relationship with Time, and a generalized linear mixed model (GLMM) was fitted for Light and E-DIN, and a generalized least squares (GLS) was fitted for Temperature (see Supplementary Material 2 for model selection).
Results
Light Conditions
When accounting for tank effects, the GLMM for average light showed no significant difference between the high light treatments (D1 and S1) and the low light treatments (D4 and S4, Figure 2). However, there was a large variance among the high light replicates regarding how much light they had received. The final model for light were therefore fitted with a new light variable separating the tanks in the high light treatment standing on the edge of the pier (High light2: S1-II and D1-II) and the other tanks (Low light2: D1-I, D4-I, D4-II, S1-I, and S4-II). The final model for average light for the last 5 days of the experiment was fitted as a function of high light (D1-II and S1-II) vs. low light (D1-I, D4-I, D4-II, S1-I, and S4-II) and time. The model had a random intercept for each tank, and variance were allowed to vary between tanks (see Table 1 for regression coefficients, and Supplementary Material 2 for model selection). The tanks on the edge of the pier in the high light treatments (D1-II and S1-II) received significantly higher PAR intensity than the other tanks [b = 48.1, t(5) = 5.1, p = 0.004], and the PAR intensity decreased significantly with time [b = −0.2, t(223) = −5.0, p < 0.001]. After removing the two high light2 tanks, there was no significant difference in average light received between the two tanks originally in the high light treatment and the other tanks in the low light2 treatment (b = −5.42, 95% CI: −14.41, 3.58, p = 0.15).
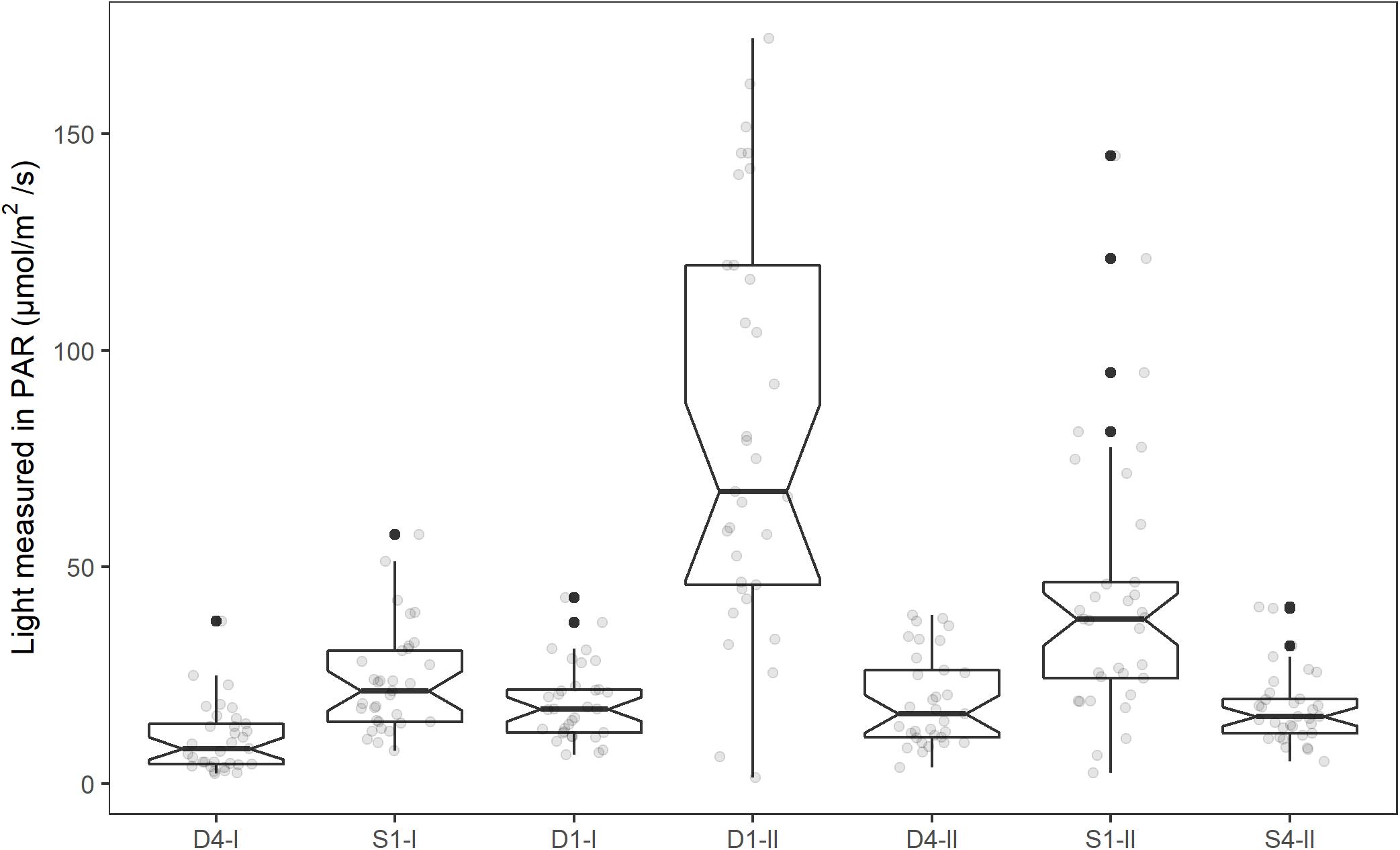
Figure 2. Mean light intensity (PAR, μmol m–2 s–1) for the tanks in the experimental period (1st–19th of June, 2014). The individual mean light intensities each day (08:00–20:00) is shown as light gray dots in the plot. Notches extend 1.58*IQR/sqrt(n), giving roughly a 95% CI. Whiskers extend from the hinge to the largest or smallest value within 1.5*IQR from the hinge. Values outside this are shown as a black dot.
Temperature
The mean water temperature was 10.2 ± 0.1°C in tanks receiving deep water (D1 and D4) and 13.1 ± 0.2°C in tanks receiving temperature acclimated surface water (S1 and S4, Figure 3). This difference between water sources was significant (b = 2.9, 95% CI: 2.7, 3.1, p < 0.001). Temperature was also affected by time in a cubic relationship (Table 1, see Supplementary Material 2 for model selection), there was, however, no significant variation in the intercepts between tanks [χ2(1) = 1.6, p = 0.2]. The temperatures received in this experiment were within the optimal range of 10–17°C for S. latissima (Druehl, 1967; Fortes and Lüning, 1980).
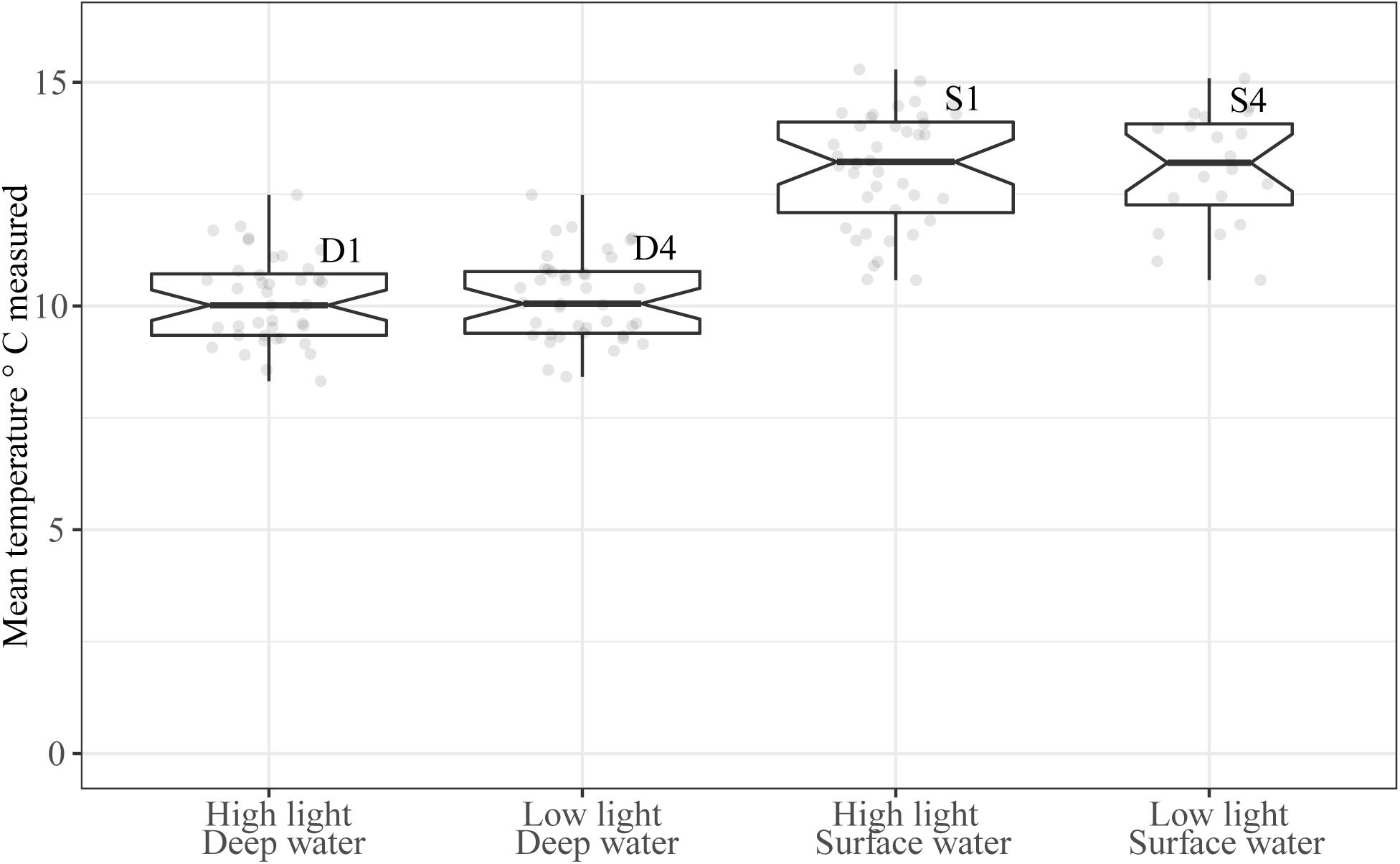
Figure 3. Mean water temperature (°C) in the experimental period (1st–19th of June 2014). Boxplot width is scaled for number of observations, and the mean individual temperature measurements are shown as light gray dots in the plot. Notches extend 1.58 * IQR/sqrt(n) giving roughly a 95% CI. Whiskers extend from the hinge to the largest or smallest value within 1.5*IQR form the hinge.
External Nitrate Concentration (E-DIN)
The relationship between the external nitrate concentration (NO3 + NO2; E-DIN; μg NO3-N L–1) and time, showed significant variation in intercepts across tanks [SD = 0.17 (95% CI: 0.07, 0.42), χ2(1) = 33, p < 0.001]. In addition, the slopes varied across tanks [SD = 0.008 (95% CI: 0.004, 0.015), χ2(2) = 8.7, p = 0.013], and the slopes and intercepts were negatively and significantly correlated [cor = −0.75 (95% CI: −0.96, 0.009)]. The E-DIN concentration increased with time [b = 0.04 (95% CI: 0.03, 0.04)], and there was a significant difference in E-DIN concentration between the tanks receiving deep water and those receiving surface water [b = 0.70 (95% CI: 0.57, 0.82), p < 0.001]. There was also a significant difference between the tanks receiving deep water (D1 and D4, b = 0.29, 95% CI: 0.11, 0.47, p = 0.015), but not for the tanks receiving surface water (S1 and S4, b = 0.07, 95% CI: −0.11, 0.04, p = 37). Figure 4 summarizes the range of values, medians, and quartiles found for the different treatments in the experimental period.
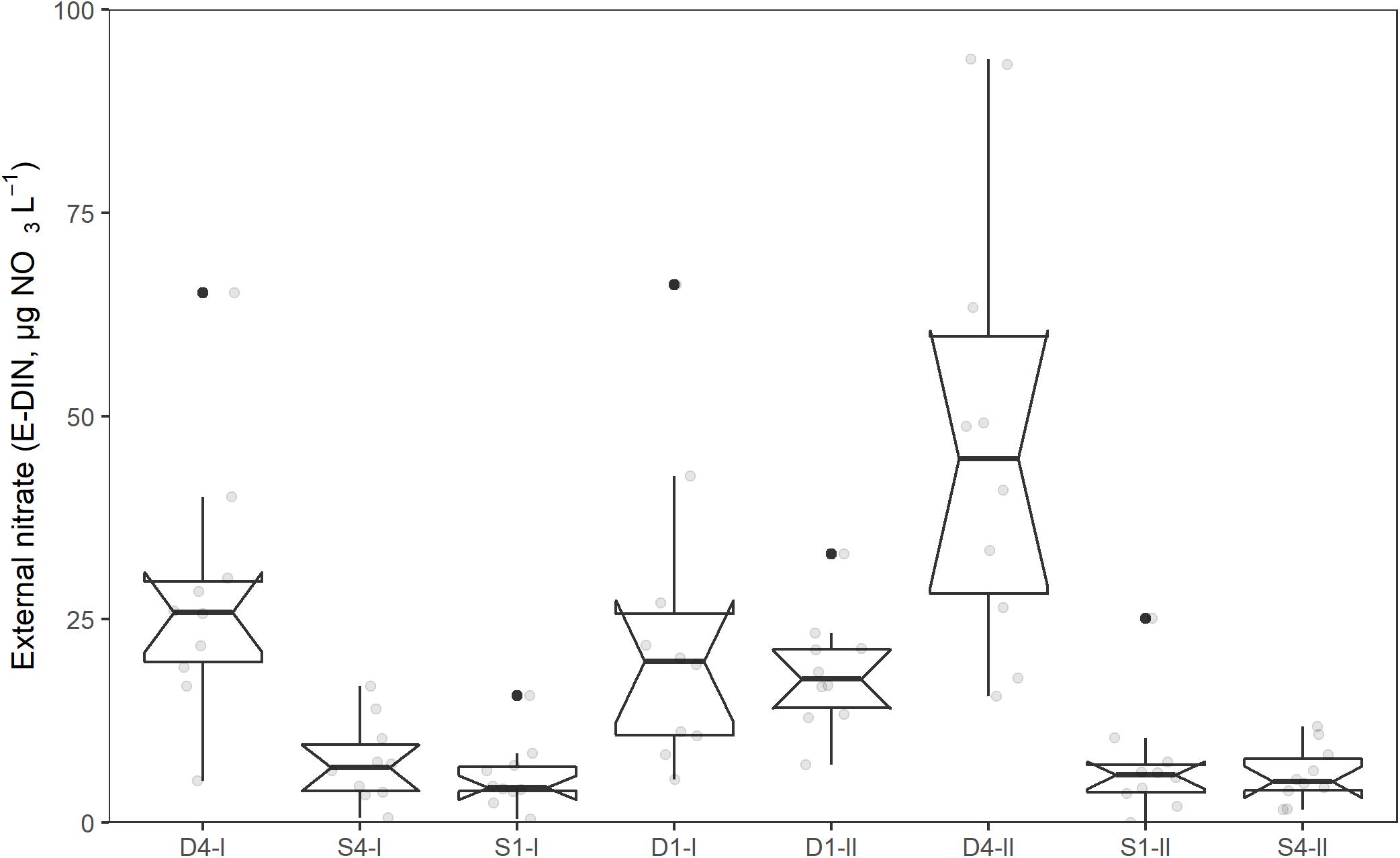
Figure 4. Concentration of external nitrate (E-DIN, μg NO3-N L–1) in different tanks during the acclimation and experimental period (11th of May–19th of June, 2014). Boxplot show lower, middle, and upper quantiles. Notches extend to median ±1.58*IQR/sqrt(n) and gives a rough 95% confidence interval for the median. Whiskers extend from the hinge to the largest or smallest value within 1.5*IQR form the hinge.
Variation in Treatments
Including the two tanks D1-I and S1-I into the low light treatment did not violate the assumption of normality or homogeneity of variance (Shapiro–Wilk’s test: 0.08, Levene’s test: 0.53). For the rest of this paper the two high light2 tanks are omitted, and the remaining tanks are divided for water source with three tanks in each treatment; Deep water (n = 3) and Surface water (n = 3).
Intracellular N-Components and External Nitrate (E-DIN)
The measured intracellular nitrogen components showed a positive but variable relationship with E-DIN in the period before harvesting (I-DIN, Figure 5A). Sporophytes maintained in tanks supplied by surface water showed the lowest I-DIN levels (0.05 ± 0.01 mg N g–1 DW), whereas those supplied by deep water showed highly variable contents (0.50 ± 0.12 mg N g–1 DW). Regression analysis on the logarithmically transformed variables showed a significant relationship between ln(I-DIN) and ln(E-DIN +1) and time (Table 1). The relationship had a significant variation in the intercepts across tanks SD = 0.51 (95% CI: 0.23, 1.11), χ2(1) = 4.5, p = 0.03.
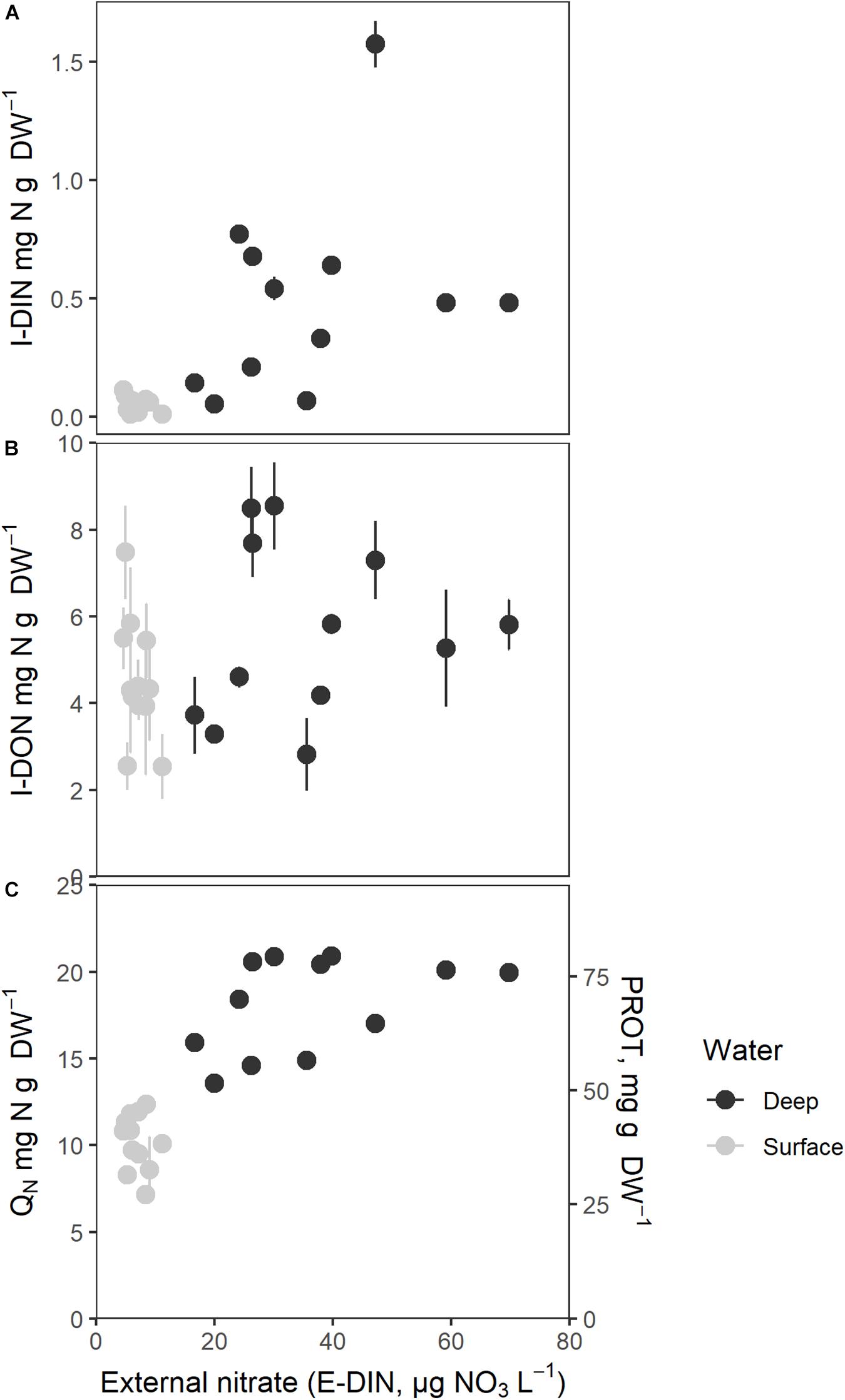
Figure 5. Intracellular nitrogen components as a function of mean external nitrate concentration (E-DIN, μg N L–1). (A) Intracellular nitrate (I-DIN, mg N g–1 DW), (B) Intracellular organic small molecular nitrogen (I-DON, mg N g–1 DW), (C) Total intracellular nitrogen (QN, mg N g–1 DW) and protein (PROT, mg protein g–1 DW). Mean E-DIN was estimated as the mean of the last five measurements. Bars express 1 ± SE of the mean if exceeding symbols.
I-DON did not exhibit a clear relationship to E-DIN (Figure 5B). Values were scattered and sometimes very high for low E-DIN, suggesting that the N-deprived sporophytes may have undergone intracellular hydrolysis before or during analysis; it cannot be excluded that this also affected the I-DON values for less nutrient deprives sporophytes at high E-DIN. The pattern found for sporophytes maintained in deep water suggested saturation in I-DON above an E-DIN concentration of 30 μg N L–1. Regression analysis on I-DON and the logarithmically transformed variable E-DIN did not show a significant positive relationship between the two [I-DON and ln(E-DIN +1), p > 0.05].
Figure 5C shows QN (mg N g–1 DW) and protein contents (PROT, mg g–1 DW, proportional to QN) as a function of E-DIN, showing an increasing relationship between the log transformed variables (b = 0.37, 95% CI: 0.29, 0.46, p < 0.001, Table 1). Time affected the relationship in a decreasing manner (b = −0.02, 95% CI: −0.03, −0.00, p = 0.011). The overall pattern suggested a saturation level for QN of ∼20 mg N g–1 DW and ∼80 mg protein g–1 DW for E-DIN concentrations above 30 μg L–1. Sporophytes supplied with surface water had a median QN of 10.5 ± 1.72 mg N g–1 DW whereas those supplied by deep water had median QN of 18.1 ± 2.53 mg N g–1 DW. Because the protein content is based on QN, protein showed the same relationship with E-DIN as QN.
The total carbon contents (QC, mg C g–1 DW) was 289 ± 16.3 g–1 DW in surface water tanks and 272 ± 23.7 mg C g–1 DW in deep water tanks, and accordingly slightly lower in the most protein rich sporophytes. The difference between surface water and deep water was, however, only borderline significant [t(19.53) = −2.10, p = 0.05].
Intracellular N-Components and Intracellular Nitrate (I-DIN)
The measured intracellular nitrogen components in the sporophytes showed an overall positive relationship to I-DIN (Figure 6). I-DON values for low I-DIN sporophytes were highly variable (Figure 6A) for reasons suggested above in section “Intracellular N-Components and External Nitrate (E-DIN).”
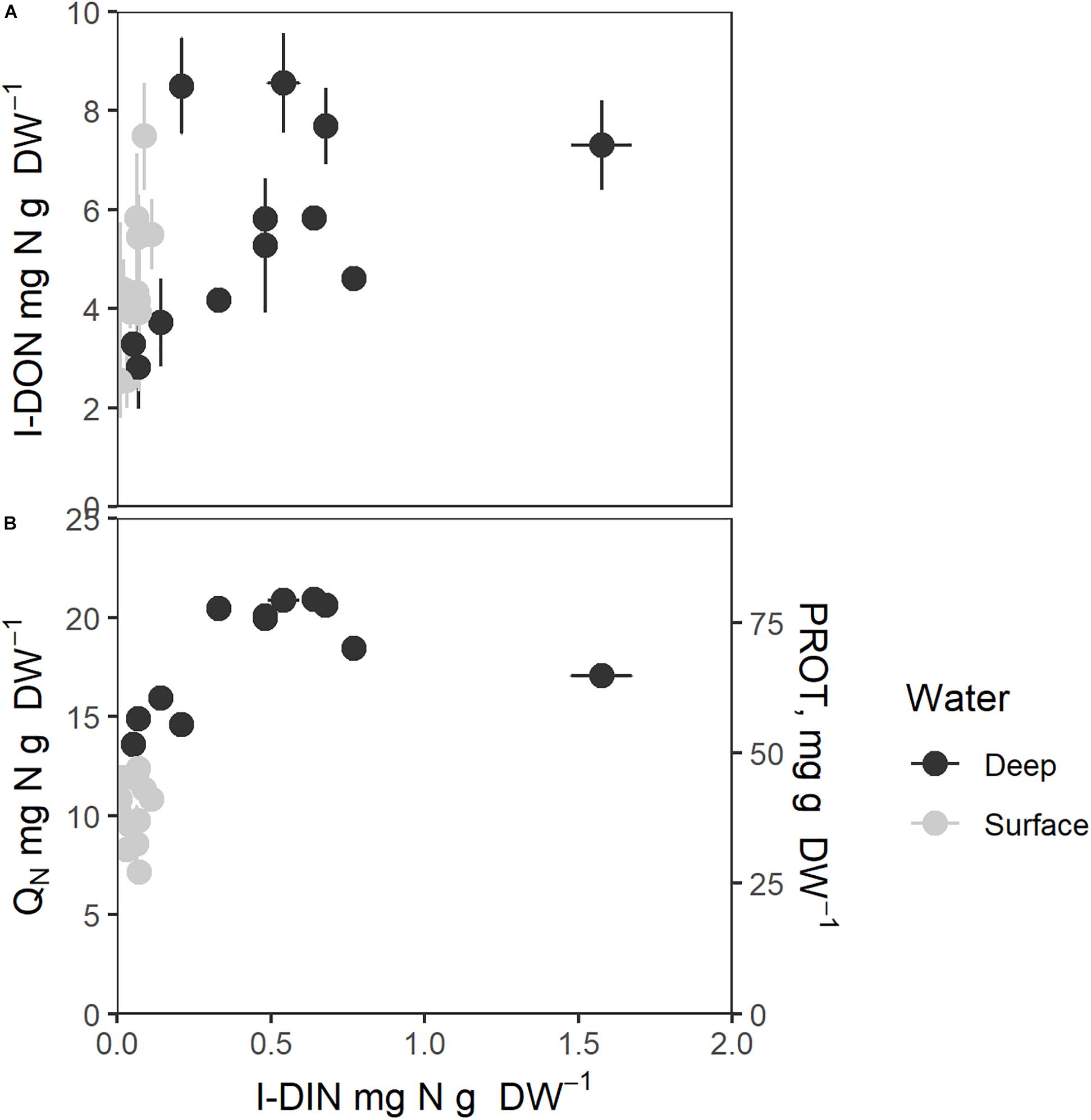
Figure 6. Intracellular nitrogen components as function of intracellular nitrate (I-DIN, mg N g–1 DW). (A) Intracellular organic small molecular nitrogen (I-DON, mg N g–1 DW), (B) Total intracellular nitrogen (QN, mg N g–1 DW, left axis) and protein contents (mg g–1 DW, right axis). Bars express 1 ± SE of the mean if exceeding symbols. Correlation coefficients are given in Table 1.
QN and protein (Figure 6B) showed saturation type relationships with an increasing cellular I-DIN. The patterns of variation of both were slightly different from that seen for E-DIN (Figure 5), as D1 values grouped with S values to greater degree, whereas D4 values were higher throughout. All cellular N components in Figure 6 showed saturation levels for I-DIN content beyond 0.3–0.4 mg N g–1 DW.
Relationship Between Algal Growth Rate and Nitrogen Components
The mean growth rate in surface water was 0.23 ± 0.03 mm day–1 while the mean growth rate in deep water was 0.66 ± 0.06 mm day–1. The mean growth rate (mm day–1) of the sporophytes is plotted as functions of extracellular (E-DIN) and intracellular variables in Figure 7. The growth rate of frond length showed clear saturation relationships to both E-DIN (Figure 7A) and I-DIN (Figure 7B), whereas the relationship between growth rate, QN, and protein appeared linear and more scattered. The growth rates showed a linear relationship with the average concentration of E-DIN the previous sample times which could explain 44% of the variation (Table 1).
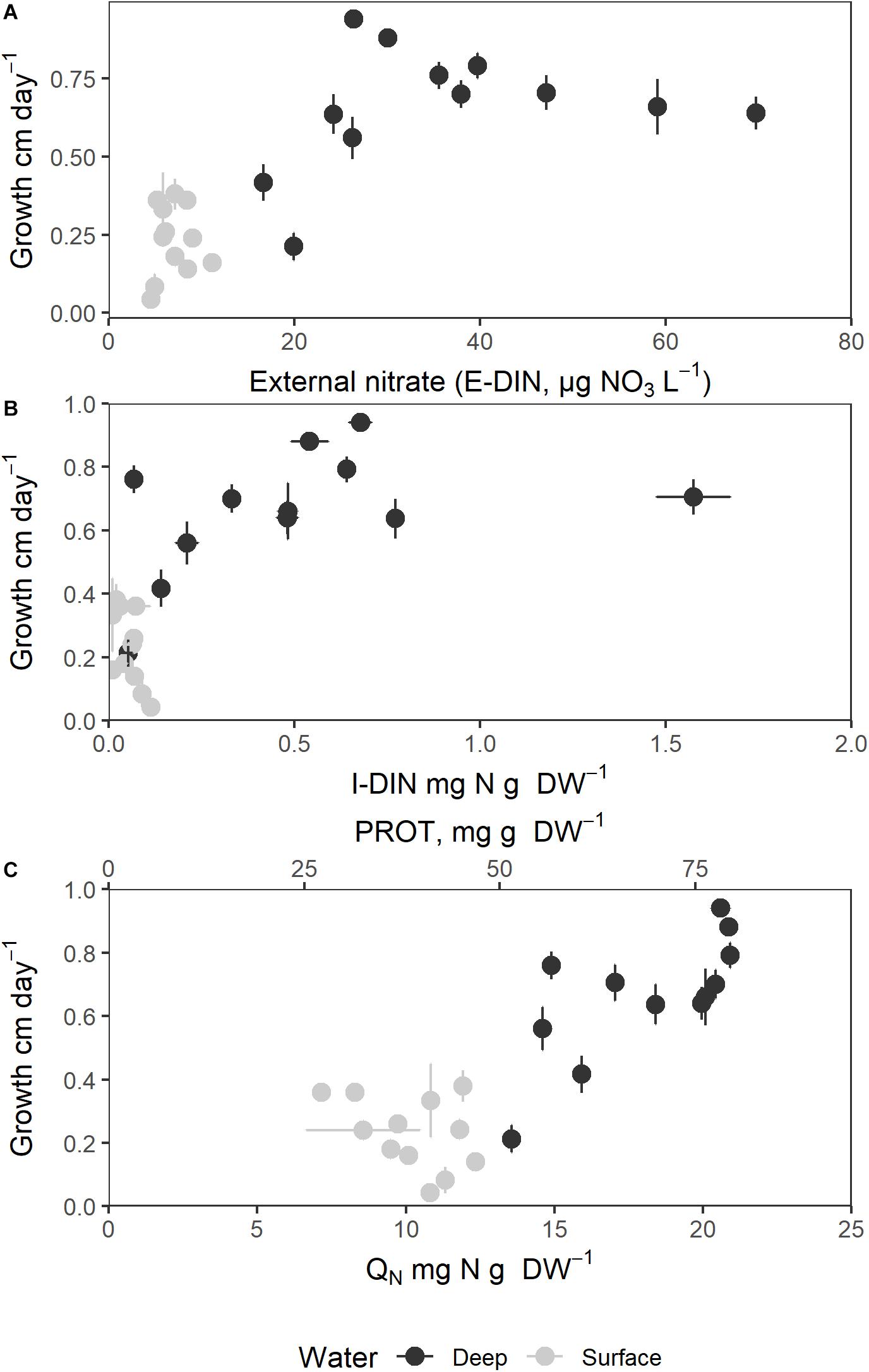
Figure 7. Sporophyte growth rate in length (mm day–1) as a function of external and internal nitrogen components. (A) Growth rate (mm day–1) as function of external nitrate concentration (E-DIN); (B) Growth rate (mm day–1) as a function of intracellular nitrate contents (I-DIN); (C) Growth rate (mm day–1) as a function of total intracellular nitrogen (QN, mg N g–1 DW) and protein (mg protein g–1 DW. Bars express 1 ± SE of the mean if exceeding symbols.
The regression of growth rate versus I-DIN x water source was significant (F3,20 = 22.4, p < 0.001). The interaction term ln(I-DIN) x water source was found to be significant (F1,20 = 7.5, p = 0.013), and including it made the model significantly better [χ2(2) = 17.6, p < 0.001, Figure 7B].
Growth rate showed a similar relationship with QN x water source (F3,20 = 26.9, p < 0.001). The interaction term QN x water source was found to be significant (F1,20 = 7.8, p < 0.01), and including it made the model significantly better [χ2(2) = 10.1, p = 0.006, Figure 7C].
The mean fraction of I-DIN associated with QN varied from 0.43 to 0.79% of QN for the S4 and S1 treatments, respectively, and from 0.79 to 3.6% of QN for the D1 and D4 treatments, respectively. The sporophytes cultivated in deep water showed accordingly higher (although variable) contents of I-DIN than those cultivated in surface water, and I-DIN constituted only a minor part of the total intracellular nitrogen.
Comparison of Protein Estimation Methods
The nitrogen-to-protein conversion factor (Kp) of 3.8 gave slightly higher and less scattered protein contents in S. latissima than the Bio-Rad method for all treatments, except for the S1 treatment (Figure 8). The differences were, however, not significant for any of the treatments (p > 0.05).
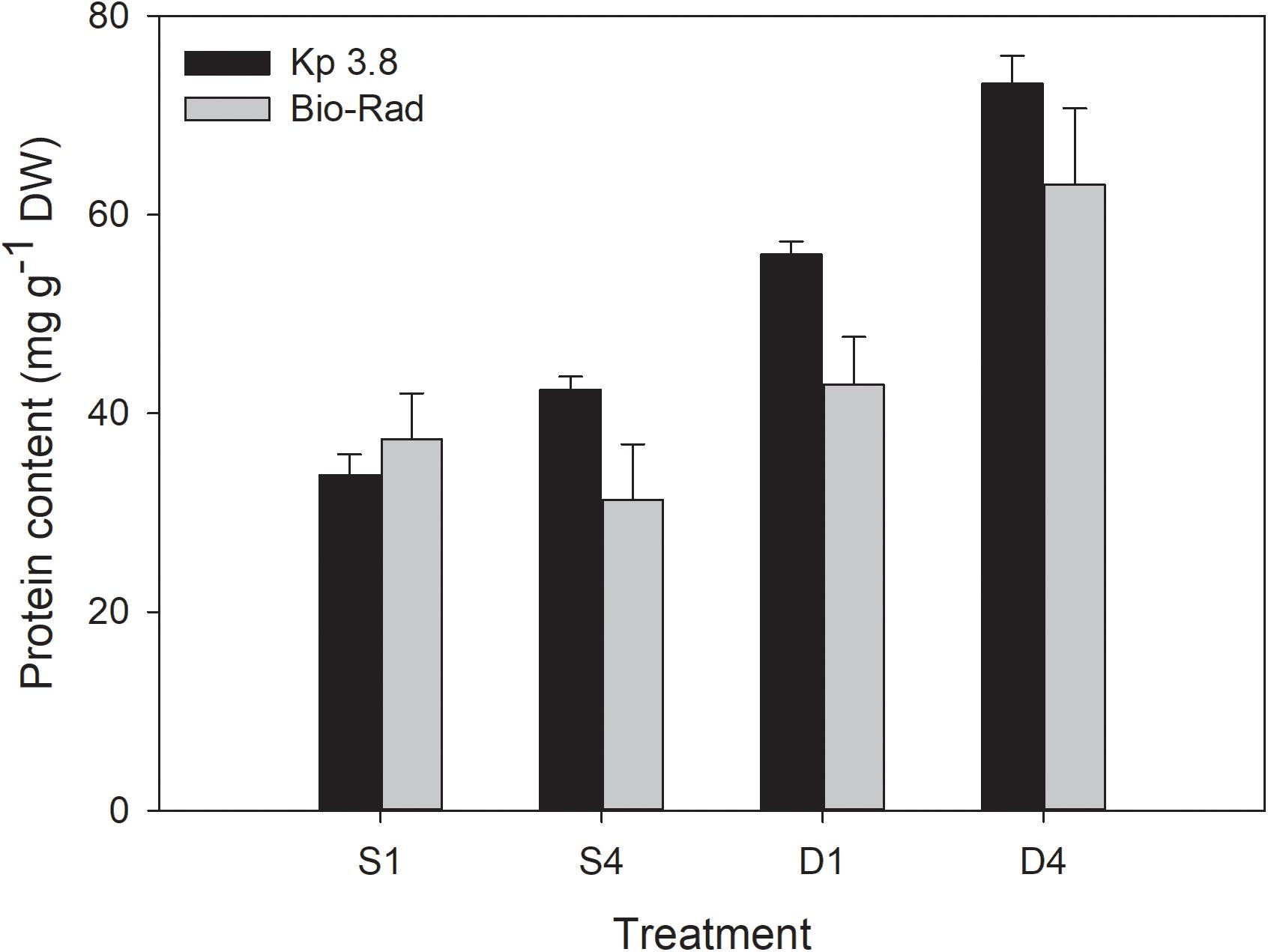
Figure 8. Comparison of protein content (mg g–1 DW) based on the nitrogen-to-protein conversion factor (Kp) of 3.8 mg protein mg–1 N and Bio-Rad analysis for the different treatments. Mean ± SE, n = 8.
Discussion
The mean growth rate of the sporophytes for all treatments combined was positively related to the mean E-DIN concentration during the experimental period. The significant positive relationship between growth rate and E-DIN concentration found in this study has also been found in earlier experiments (Handå et al., 2013; Kerrison et al., 2015; Boderskov et al., 2016).
The E-DIN concentration measured in the D1-I tank was significantly lower than that measured in the D4-II tank even though both tanks received deep water. A possible explanation for the difference in E-DIN concentration in tanks receiving similar deep water might be that there was a difference in the community of opportunistic diatoms, which was observed growing on some of the tank walls, but not quantified. It may also be an effect of accidental differences in the water supply rate of deep water to the tanks or of minor variation in the biomass distribution. The measured increase in E-DIN concentration for all tanks during the experimental period is probably explained by the decrease in biomass as sporophytes were removed systematically for chemical analysis.
An earlier study showed that the specific growth rate of S. latissima increased with the E-DIN concentration up to 70–140 μg NO3-N L–1 (Wheeler and Weidner, 1983). It is therefore likely that the algae of our experiment did not experience growth saturating concentrations of external NO3–. This supports our conclusion that NO3– is limiting for all tanks in this experiment, even those exposed to the highest concentrations. This conclusion is also in agreement with previous results for the growth capabilities of S. latissima. The highest growth rates recorded in earlier studies are 8–17 mm day–1, obtained in June (Parke, 1948), and 10–20 mm day–1, obtained in March to May (Nielsen et al., 2014). The highest growth rate found in deep water in our study was 6.6 mm day–1, likely lower than the maximum growth rate that might be obtained in saturating conditions of NO3– and light.
Our combined results revealed a complete pattern of variation in intracellular nitrogen components of nutrient deprived and more nutrient sufficient S. latissima. Sporophytes grown in surface waters (S treatments) experienced low ambient nitrate concentrations and were characterized by low growth rates and low contents of the measured nitrogen components. Sporophytes grown in temperature acclimated deep water (D treatments) were exposed to higher, although variable, ambient nitrate concentrations and were characterized by higher growth rates and higher contents of intracellular nitrogen components. The tanks formed together a continuum characterizing the intracellular contents of nitrogen components for variable ambient nitrate concentrations and growth rates. It is important to note that the overall pattern of variation in intracellular components found is similar to that obtained in earlier studies of both freshwater and marine microalgae (Healey and Hendzel, 1979; Sterner and Elser, 2002).
The growth rates were found to increase with increasing nitrogen levels for all variables measured in the current experiment (E-DIN, I-DIN, QN, and protein). Both E-DIN and I-DIN could explain much of the variation in growth rate. These data must be carefully interpreted because the nitrogen metabolites also correlated with each other, making it somewhat difficult to extract the exact relationship between growth rate and each variable. The most obvious finding to emerge from the relationships between I-DIN and E-DIN is the increasing positive relationship between these variables, where sporophytes grown in the highest concentration of E-DIN consistently showed higher concentrations of I-DIN, QN, and protein. The concentration of E-DIN measured in the tanks could account for 55% of the variation in the measured I-DIN concentrations. A higher concentration of I-DIN with increasing E-DIN concentrations is in accordance with S. latissima’s ability to store nitrate when the nutrient is available in excess (Wheeler and Weidner, 1983; Young et al., 2007; Bartsch et al., 2008; Hurd et al., 2014). This storage capability is, however, found to be relatively low, constituting only a small fraction (3.6%) of the maximum QN content for the D4 treatments. This level of I-DIN agrees with earlier field studies and an experiment made to characterize nitrate uptake kinetics of S. latissima (Forbord et al., 2020, 2021). Even though the value of I-DIN is low, the variable correlates well with other nitrogen metabolites and growth rate.
The relationship between nutrients used for growth and uptake is not directly coupled because the algae assimilate and store nitrogen. Moreover, in times of low nitrate concentrations and uptake, growth may take place on the expense of intracellular nitrogen components. It therefore makes more sense to evaluate their nutritional status by assessing the tissue N contents like QN and I-DIN (Dalsgaard and Krause-Jensen, 2006; Manns et al., 2017). Seaweeds are in general more sensitive to environmental changes when their QN reserves are exhausted (Gerard, 1997; Gao et al., 2013). The QN content only exceeded the critical minimum concentration of 17–20 mg N g–1 DW suggested for sustaining growth at maximum rates (Chapman et al., 1978; Hanisak, 1983; Pedersen and Borum, 1997) in the D4 tanks (19.8 mg N g–1 DW), whereas the other treatments had mean QN values of 9.0–14.7 mg N g–1 DW, suggesting that the sporophytes were N-deprived. The previously published critical QN concentrations do not necessarily regard the physiological state of the seaweed, so the QN concentration for sustaining growth will most likely vary between species, developmental stage, and nutritional status.
The quantity of small molecules of I-DON, calculated as the difference between the total dissolved nitrate and inorganic nitrate in the same sample, was believed to be overestimated due to its high fraction compared to, e.g., protein. A part of this fraction could be hydrolyzed proteins from the process of boiling samples to release small molecules from algal tissue. Severely nutrient starved S. latissima from low E-DIN treatments could have contained a high share of dead cells, characterized by an increased rate of intracellular protein hydrolysis, which may be further enhanced during boiling. It cannot be excluded that this also may take place in nitrogen sufficient algae during boiling. The results obtained for I-DON are, in our view, uncertain, and the method is questionable. I-DON results are therefore omitted from further discussion.
The highest measured protein content of 77.0 ± 2.7 mg g–1 DW (median ± MAD), found for the D4 treatment, was higher or similar to values registered in May–June for S. latissima in several other experiments using the same estimation method based on amino acids (Marinho et al., 2015; Bak et al., 2019; Forbord et al., 2019, 2020). The D4 sporophytes had almost twice the protein content compared to the surface water treatments. This coincided with previous experiments, where higher protein content was found in macroalgae in deeper waters, interpreted to be a result of a higher E-DIN concentration (Harnedy and FitzGerald, 2011).
Calculating the protein content from the nitrogen-to-protein conversion factor (Kp) gave a higher, while not significantly, protein content for all treatments compared to the Bio-Rad method, except for the S1 treatment. The Bio-Rad method estimates the soluble protein fraction of the sample, while the Kp method based on QN, estimates total protein (Safi et al., 2018). Some uncertainty is connected with the use of Kp factors, because they may change with season, depth, location and species. Kp factors have been shown to vary in the range of 2.0–6.25 (Lourenço et al., 2002; Schiener et al., 2015; Angell et al., 2016; Biancarosa et al., 2017; Manns et al., 2017; Sharma et al., 2018; Bak et al., 2019; Forbord et al., 2020). The 6.25 value is the standard factor used for pure protein and is obviously not relevant for biomass samples that contain other N-rich components like nucleic acids and pigments. One should be careful to compare the outcome of the different protein assays with each other as the methods are based on different principles and can result in different values (Barbarino and Lourenço, 2005; Mæhre et al., 2018). The protein estimates from the current experiment are supported by two independent methods.
The high variability in the light intensity measured in the different tanks within the same light treatments was assumed to be because of edge effects, i.e., the sides of the tanks were not lightproof, leading to higher light intensities for tanks not shaded by others. Two of the high light treatment tanks placed on the edge were exposed to light levels that temporarily reached 250 μmol m–2 s–1 during the day (D1-II 24 days, S1-II 8 days, data not shown), which could have been high enough to cause photo inhibition (Fortes and Lüning, 1980). The differences experienced by these tanks, which were both on the edge, were likely due to the position of the sun. During midday, the tanks furthest south would cast a shadow on the tanks further north. On the other hand, periodic shading of the light sensors situated at the bottom of the tanks by the growing sporophytes may have resulted in lower measured irradiance than what the sporophytes experienced.
Conclusion
The present study was designed to determine the effects of varying environmental conditions on the growth and nitrogen metabolites of cultivated S. latissima. The experiment confirmed a positive relationship between total intracellular nitrogen components (QN, protein and I-DIN) and external inorganic nitrate, and between growth rate and external nitrate. Our combined results revealed a complete pattern of variation of intracellular nitrogen components of nutrient deprived and nutrient saturated S. latissima. This is also supported by the pattern found in contents of intracellular nitrogen metabolites related to external nitrate concentrations. These results indicates that S. latissima requires high ambient nitrate concentrations to maintain rapid growth, concentrations usually not found along the Norwegian coast in the late spring and summer, where nutrient starvation is experienced and an increase in biomass in these periods is mainly based on consumption of intracellular nitrogen components.
Data Availability Statement
The original contributions presented in the study are included in the article/Supplementary Material, further inquiries can be directed to the corresponding author/s.
Author Contributions
LJ participated in planning the experiment, carried out the practical work including field measurements and chemical analysis, most of the data treatment, and contributed to writing the manuscript. SF wrote the main part of the manuscript, and contributed to analysis of samples and data treatment. YO conceived the idea of the study, acquired funding and participated in the planning and writing process, and contributed to data treatment. All authors contributed to the article and approved the submitted version.
Funding
This work formed part of the Research Council of Norway project no. 254883 (MacroSea), together with internal funding from the Norwegian University of Science and Technology (NTNU).
Conflict of Interest
The authors declare that the research was conducted in the absence of any commercial or financial relationships that could be construed as a potential conflict of interest.
Acknowledgments
We are grateful to the staff at Seaweed Energy Solutions (SES) for kindly providing research facilities, experimental biomass, and support. Thanks to Kjersti Andresen and Siv Anina Etter (NTNU) for analyzing the water samples and Marte Schei (SINTEF Ocean) for conducting the CN analysis.
Supplementary Material
The Supplementary Material for this article can be found online at: https://www.frontiersin.org/articles/10.3389/fmars.2020.557460/full#supplementary-material
Abbreviations
E-DIN, external nitrate concentration; I-DIN, intracellular nitrate content; I-DON, small molecules of intracellular organic nitrate; Kp, specific nitrogen-to-protein conversion factor; QN, total tissue nitrogen content.
References
Angell, A. R., Mata, L., de Nys, R., and Paul, N. A. (2016). The protein content of seaweeds: a universal nitrogen-to-protein conversion factor of five. J. Appl. Phycol. 28, 511–524.
Bak, U. G., Mols-Mortensen, A., and Gregersen, O. (2018). Production method and cost of commercial-scale offshore cultivation of kelp in the Faroe Islands using multiple partial harvesting. Algal Res. 33, 36–47.
Bak, U. G., Nielsen, C. W., Marinho, G. S., Gregersen, Ó, Jónsdóttir, R., and Holdt, S. L. (2019). The seasonal variation in nitrogen, amino acid, protein and nitrogen-to-protein conversion factors of commercially cultivated Faroese Saccharina latissima. Algal Res. 42:101576.
Barbarino, E., and Lourenço, S. O. (2005). An evaluation of methods for extraction and quantification of protein from marine macro-and microalgae. J. Appl. Phycol. 17, 447–460.
Barbier, M., Charrier, B., Araujo, R., Holdt, S. L., Jacquemin, B., and Rebours, C. (2019). PEGASUS - PHYCOMORPH European Guidelines for a Sustainable Aquaculture of Seaweeds. COST Action FA1406. Available online at:http://sciencethics.org/phycomorph-european-guidelines-for-a-sustainable-aquaculture-of-seaweeds (accessed September 1, 2019).
Bartsch, I., Wiencke, C., Bischof, K., Buchholz, C. M., Buck, B. H., Eggert, A., et al. (2008). The genus Laminaria sensu lato: recent insights and developments. Eur. J. Phycol. 43, 1–86.
Biancarosa, I., Espe, M., Bruckner, C., Heesch, S., Liland, N., Waagbø, R., et al. (2017). Amino acid composition, protein content, and nitrogen-to-protein conversion factors of 21 seaweed species from Norwegian waters. J. Appl. Phycol. 29, 1001–1009.
BIO-RAD (2013). DC Protein Assay US. Available online at: http://www.bio-rad.com/webroot/web/pdf/lsr/literature/LIT33.pdf (accessed April 30, 2020).
Boderskov, T., Schmedes, P., Bruhn, A., Rasmussen, M., Nielsen, M., and Pedersen, M. (2016). The effect of light and nutrient availability on growth, nitrogen, and pigment contents of Saccharina latissima (Phaeophyceae). grown in outdoor tanks, under natural variation of sunlight and temperature, during autumn and early winter in Denmark. J. Appl. Phycol. 28, 1153–1165.
Broch, O. J., Alver, M. O., Bekkby, T., Gundersen, H., Forbord, S., Handå, A., et al. (2019). The kelp cultivation potential in coastal and offshore regions of Norway. Front. Mar. Sci. 5:529. doi: 10.3389/fmars.2018.00529
Broch, O. J., and Slagstad, D. (2012). Modelling seasonal growth and composition of the kelp Saccharina latissima. J. Appl. Phycol. 24, 759–776.
Bruhn, J., and Gerard, V. (1996). Photoinhibition and recovery of the kelp Laminaria saccharina at optimal and superoptimal temperatures. Mar. Biol. 125, 639–648.
Buschmann, A. H., and Camus, C. (2019). An introduction to farming and biomass utilisation of marine macroalgae. Phycologia 58, 443–445.
Capone, D. G., Bronk, D. A., Mulholland, M. R., and Carpenter, E. J. (2008). Nitrogen in the Marine Environment, 2 Edn. New York, NY: Academic Press.
Chapman, A., and Craigie, J. (1977). Seasonal growth in Laminaria longicruris: relations with dissolved inorganic nutrients and internal reserves of nitrogen. Mar. Biol. 40, 197–205.
Chapman, A., Markham, J., and Lüning, K. (1978). Effects of nitrate concentration on the growth and physiology of Laminaria saccharina (Phaeophyta). in culture. J. Phycol. 14, 195–198.
Dalsgaard, T., and Krause-Jensen, D. (2006). Monitoring nutrient release from fish farms with macroalgal and phytoplankton bioassays. Aquaculture 256, 302–310.
Directorate of Fisheries (2020). Akvakulturstatistikk. (tidsserier)-Alger. Available online at: http://www.fiskeridir.no/Akvakultur/Statistikk-akvakultur/Akvakulturstatistikk-tidsserier/Alger (accessed September 24, 2020).
Druehl, L. D. (1967). Distribution of two species of Laminaria as related to some environmental factors. J. Phycol. 3, 103–108.
Flavin, K., Flavin, N., and Flahive, B. (2013). Kelp Farming Manual: A Guide to the Processes, Techniques, and Equipment for Farming Kelp in New England Waters. Saco, ME: Ocean Approved LLC.
Forbord, S., Etter, S. A., Broch, O. J., Dahlen, V. R., and Olsen, Y. (2021). Initial short-term nitrate uptake in juvenile, cultivated Saccharina latissima (Phaeophyceae). of variable nutritional state. Aquat. Bot. 168:103306. doi: 10.1016/j.aquabot.2020.103306
Forbord, S., Matsson, S., Brodahl, G. E., Bluhm, B. A., Broch, O. J., Handå, A., et al. (2020). Latitudinal, seasonal and depth-dependent variation in growth, chemical composition and biofouling of cultivated Saccharina latissima (Phaeophyceae) along the Norwegian coast. J. Appl. Phycol. 32, 2215–2232. doi: 10.1007/s10811-020-02038-y
Forbord, S., Skjermo, J., Arff, J., Handå, A., Reitan, K., Bjerregaard, R., et al. (2012). Development of Saccharina latissima (Phaeophyceae) kelp hatcheries with year-round production of zoospores and juvenile sporophytes on culture ropes for kelp aquaculture. J. Appl. Phycol. 24, 393–399.
Forbord, S., Steinhovden, K. B., Rød, K. K., Handå, A., and Skjermo, J. (2018). “Cultivation protocol for Saccharina latissima,” in Protocols for Macroalgae Research, 1st Edn, eds B. Charrier, T. Reddy, and C. Wichard (Boca Raton, FL: CRC Press), 37–59.
Forbord, S., Steinhovden, K. B., Solvang, T., Handå, A., and Skjermo, J. (2019). Effect of seeding methods and hatchery periods on sea cultivation of Saccharina latissima (Phaeophyceae): a Norwegian case-study. J. Appl. Phycol. 32, 2201–2212. doi: 10.1007/s10811-020-02042-2
Fortes, M. D., and Lüning, K. (1980). Growth rates of North Sea macroalgae in relation to temperature, irradiance and photoperiod. Helgol. Mar. Res. 34, 15–29.
Gao, X., Endo, H., Taniguchi, K., and Agatsuma, Y. (2013). Combined effects of seawater temperature and nutrient condition on growth and survival of juvenile sporophytes of the kelp Undaria pinnatifida (Laminariales; Phaeophyta). cultivated in northern Honshu, Japan. J. Appl. Phycol. 25, 269–275.
Gerard, V. A. (1997). The role of nitrogen nutrition in high-temperature tolerance of the kelp, Laminaria saccharina (Chromophyta). J. Phycol. 33, 800–810.
Gordillo, F. J. (2012). “Environment and algal nutrition,” in Seaweed biology. Ecological Studies. (Analysis and Synthesis), Vol. 219, eds C. Wiencke and K. Bischof (Berlin: Springer), 67–86.
Grebe, G. S., Byron, C. J., Gelais, A. S., Kotowicz, D. M., and Olson, T. K. (2019). An ecosystem approach to kelp aquaculture in the Americas and Europe. Aquac. Rep. 15:100215.
Handå, A., Forbord, S., Wang, X., Broch, O. J., Dahle, S. W., Størseth, T. R., et al. (2013). Seasonal- and depth-dependent growth of cultivated kelp (Saccharina latissima) in close proximity to salmon (Salmo salar) aquaculture in Norway. Aquaculture 414-415, 191–201.
Hanisak, M. (1983). “The nitrogen relationships of marine macroalgae,” in Nitrogen in the Marine Environment, eds E. J. Carpenter and D. G. Capone (New York, NY: Academic Press), 699–730.
Harnedy, P. A., and FitzGerald, R. J. (2011). Bioactive proteins, peptides and amino acids from macroalgae. J. Phycol. 47, 218–232.
Healey, F., and Hendzel, L. (1979). Indicators of phosphorus and nitrogen deficiency in five algae in culture. J. Fish. Res. Board Can. 36, 1364–1369.
Holdt, S. L., and Kraan, S. (2011). Bioactive compounds in seaweed: functional food applications and legislation. J. Appl. Phycol. 23, 543–597.
Hurd, C. L., Harrison, P. J., Bischof, K., and Lobban, C. S. (2014). Seaweed Ecology and Physiology, 2 Edn. Cambridge: Cambridge University Press.
Ibrahim, A., Olsen, A., Lauvset, S., and Rey, F. (2014). Seasonal variations of the surface nutrients and hydrography in the Norwegian Sea. Int. J. Environ. Sci. Dev. 5, 496–505.
Kerrison, P. D., Stanley, M. S., Edwards, M. D., Black, K. D., and Hughes, A. D. (2015). The cultivation of European kelp for bioenergy: site and species selection. Biomass Bioenergy 80, 229–242.
Krause-Jensen, D., Lavery, P., Serrano, O., Marbà, N., Masque, P., and Duarte, C. M. (2018). Sequestration of macroalgal carbon: the elephant in the Blue Carbon room. Biol. Lett. 14:20180236.
Lapointe, B. E., and Tenore, K. R. (1981). Experimental outdoor studies with Ulva fasciata Delile. I. Interaction of light and nitrogen on nutrient uptake, growth, and biochemical composition. J. Exp. Mar. Biol. Ecol. 53, 135–152.
Lourenço, S. O., Barbarino, E., De-Paula, J. C., Pereira, L. O. D. S., and Marquez, U. M. L. (2002). Amino acid composition, protein content and calculation of nitrogen-to-protein conversion factors for 19 tropical seaweeds. Phycol. Res. 50, 233–241.
Lowry, O. H., Rosebrough, N. J., Farr, A. L., and Randall, R. J. (1951). Protein measurement with the Folin phenol reagent. J. Biol. Chem. 193, 265–275.
Mæhre, H. K., Dalheim, L., Edvinsen, G. K., Elvevoll, E. O., and Jensen, I.-J. (2018). Protein determination—method matters. Foods 7:5.
Manns, D., Nielsen, M. M., Bruhn, A., Saake, B., and Meyer, A. S. (2017). Compositional variations of brown seaweeds Laminaria digitata and Saccharina latissima in Danish waters. J. Appl. Phycol. 29, 1493–1506.
Marinho, G. S., Holdt, S. L., and Angelidaki, I. (2015). Seasonal variations in the amino acid profile and protein nutritional value of Saccharina latissima cultivated in a commercial IMTA system. J. Appl. Phycol. 27, 1991–2000.
Nielsen, M., Krause-Jensen, D., Olesen, B., Thinggaard, R., Christensen, P., and Bruhn, A. (2014). Growth dynamics of Saccharina latissima. (Laminariales, Phaeophyceae). in Aarhus Bay, Denmark, and along the species’ distribution range. Mar. Biol. 161, 2011–2022.
NSF (1975). NS 4746 Water analysis. Determination of the sum of Nitrite-Nitrogen and Nitrate-Nitrogen. (in Norwegian). Oslo: Norges Standardiseringsforbund.
Parke, M. (1948). Studies on British Laminariaceae. I. Growth in Laminaria saccharina (L.). Lamour. J Mar. Biol. Assoc. U.K. 27, 651–709.
Pedersen, M. F., and Borum, J. (1996). Nutrient control of algal growth in estuarine waters. Nutrient limitation and the importance of nitrogen requirements and nitrogen storage among phytoplankton and species of macroalgae. Mar. Ecol. Prog. Ser. 142, 261–272.
Pedersen, M. F., and Borum, J. (1997). Nutrient control of estuarine macroalgae: growth strategy and the balance between nitrogen requirements and uptake. Mar. Ecol. Prog. Ser. 161, 155–163.
Peteiro, C., and Freire, Ó. (2013). Biomass yield and morphological features of the seaweed Saccharina latissima cultivated at two different sites in a coastal bay in the Atlantic coast of Spain. J. Appl. Phycol. 25, 205–213.
R Core Team (2019). R: A Language and Environment for Statistical Computing. Vienna: R Foundation for Statistical Computing.
Redmond, S., Green, L., Yarish, C., Kim, J., and Neefus, C. (2014). New England Seaweed Culture Handbook-Nursery Systems. Available online at: http://seagrant.uconn.edu/publications/aquaculture/handbook.pdf (accessed September 1, 2019).
Rey, F., Aure, J., and Danielssen, D. (2007). “Temporal and spatial distribution of nutrients,” in The Norwegian Coastal Current- Oceanography and Climate. Tapir, ed. R. Sætre (Trondheim: Academic Press), 73–88.
Safi, C., van Leeuwen, J., Telleman, Y., Engelen-Smit, N., van den Broek, L., and Harmsen, P. (2018). “Quantification of proteins in seaweeds,” in Protocols for Macroalgae Research, eds B. Charrier, T. Wichard, and C. Reddy (Boca Raton, FL: CRC Press), 211–224.
Schiener, P., Black, K. D., Stanley, M. S., and Green, D. H. (2015). The seasonal variation in the chemical composition of the kelp species Laminaria digitata, Laminaria hyperborea, Saccharina latissima and Alaria esculenta. J. Appl. Phycol. 27, 363–373.
Sharma, S., Neves, L., Funderud, J., Mydland, L. T., Øverland, M., and Horn, S. J. (2018). Seasonal and depth variations in the chemical composition of cultivated Saccharina latissima. Algal Res. 32, 107–112.
Sipler, R. E., and Bronk, D. A. A. Hd and A. Cg (eds) (2015). “Dynamics of dissolved organic nitrogen,” in Biogeochemistry of Marine Dissolved Organic Matter, 2nd Edn, (Amsterdam: Elsevier), 127–232.
Skjermo, J., Aasen, I. M., Arff, J., Broch, O. J., Carvajal, A., Christie, H., et al. (2014). A New Norwegian Bioeconomy Based on Cultivation and Processing of Seaweeds: Opportunities and R&D Needs. Available online at: https://docplayer.net/20719609-Report-a-new-norwegian-bioeconomy-based-on-cultivation-and-processing-of-seaweeds-opportunities-and-r-d-needs.html (accessed September 1, 2019).
Sterner, R. W., and Elser, J. J. (2002). Ecological Stoichiometry: the Biology of Elements from Molecules to the Biosphere. Princeton, NJ: Princeton university press.
Stévant, P., Rebours, C., and Chapman, A. (2017). Seaweed aquaculture in Norway: recent industrial developments and future perspectives. Aquac. Int. 25, 1373–1390.
Sullivan, G. M., and Feinn, R. (2012). Using effect size—or why the P value is not enough. J. Grad. Med. Educ. 4, 279–282.
Thimijan, R. W., and Heins, R. D. (1983). Photometric, radiometric, and quantum light units of measure: a review of procedures for interconversion. Hortscience 18, 818–822.
Wheeler, W., and Weidner, M. (1983). Effects of external inorganic nitrogen concentration on metabolism, growth and activities of key carbon and nitrogen assimilatory enzymes of Laminaria saccharina (Phaeophyceae) in culture. J. Phycol. 19, 92–96.
Xiao, X., Agusti, S., Lin, F., Yu, Y., Pan, Y., Li, K., et al. (2019). Resource (light and nitrogen) and density-dependence of seaweed growth. Front. Mar. Sci. 6:618. doi: 10.3389/fmars.2019.00618
Keywords: Saccharina latissima, growth rates, nutrient availability, seaweed aquaculture, intracellular nitrogen components, protein content
Citation: Jevne LS, Forbord S and Olsen Y (2020) The Effect of Nutrient Availability and Light Conditions on the Growth and Intracellular Nitrogen Components of Land-Based Cultivated Saccharina latissima (Phaeophyta). Front. Mar. Sci. 7:557460. doi: 10.3389/fmars.2020.557460
Received: 30 April 2020; Accepted: 08 October 2020;
Published: 23 October 2020.
Edited by:
Jose Luis Iriarte, Austral University of Chile, ChileReviewed by:
Pedro Murua, Scottish Association for Marine Science, United KingdomBela H. Buck, Alfred Wegener Institute (AWI), Germany
Copyright © 2020 Jevne, Forbord and Olsen. This is an open-access article distributed under the terms of the Creative Commons Attribution License (CC BY). The use, distribution or reproduction in other forums is permitted, provided the original author(s) and the copyright owner(s) are credited and that the original publication in this journal is cited, in accordance with accepted academic practice. No use, distribution or reproduction is permitted which does not comply with these terms.
*Correspondence: Lone Sunniva Jevne, bG9uZS5zLmpldm5lQGdtYWlsLmNvbQ==