- 1Departamento de Biología, Observatorio de Ecología Microbiana, Facultad de Ciencias Naturales y Exactas, Universidad de Playa Ancha, Valparaíso, Chile
- 2HUB Ambiental UPLA, Universidad de Playa Ancha, Valparaíso, Chile
- 3Facultad de Ciencias de la Vida, Universidad Andres Bello, Santiago, Chile
- 4Departamento de Oceanografía, Universidad de Concepción, Concepción, Chile
- 5Instituto Milenio de Oceanografía, Universidad de Concepción, Concepción, Chile
- 6Facultad de Ciencias, Universidad de Chile, Santiago, Chile
- 7Centro de Investigacion en Estudios Avanzados del Maule - CIEAM, Vicerrectoría de Investigación y Postgrado, Universidad Católica del Maule, Talca, Chile
- 8Departamento de Obras Civiles, Facultad de Ciencias de la Ingeniería, Universidad Católica del Maule, Talca, Chile
- 9Centro Regional de Estudios Ambientales, Universidad Católica de la Santísima Concepción, Talcahuano, Chile
- 10Instituto Geofísico del Perú, Lima, Peru
The strong seasonal variability in physical-chemical conditions of the Eastern South Pacific Ocean creates an ideal setting to study spatiotemporal distribution of key marine microbial communities. We herein report a nearly 4-year-long time series of the variability in amoA gene counts of ammonia oxidizing archaea (AOA) and bacteria (betaproteobacteria, bAOB) by quantitative PCR, GI.1a Thaumarchaeota and MG-II Euryarchaeota by CARD-FISH, and the picoplanktonic community by flow cytometry for this area. During spring-summer, non-photosynthetic picoplankton such as MG-II Euryarchaeota and GI.1a Thaumarchaeota peaked at the surface and deeper waters, respectively. General AOA and bAOB achieved higher abundances at the oxycline mainly in summer (up to 105–104 amoA copies mL–1). Generalized additive models for location, scale, and shape (GAMLSS) indicated that season and depth account for 19–46% of variations in the abundance of the groups studied, particularly GI.1a Thaumarchaeota and AOA. The oxygen and nitrite concentration were statistically meaningful predictors for the studied groups. GAMLSS models indicate that ammonia oxidizing assemblage’s variability is coupled with ammonia, nitrite, and nitrate variations. Our results indicate that microbial abundances fluctuation is associated with upwelling variability and oxygen-deficient water conditions that shape the substrates availability and metabolic response of marine microbes, including keystone ammonia oxidizing assemblages and their ecological interactions. Overall, our results support planktonic nitrification activity and its contribution to nitrous oxide excess production in the time series off Concepción and the ecological dynamics regarding AOA and bAOB in coastal waters.
Introduction
Coastal marine areas hold a diverse microbial community but with the predominance of abundant taxa, such as Gamma- and Alpha-proteobacteria, Bacteroidetes among other bacterial phyla, and archaea from Thaumarchaea and MGII Euryarchaea which has been reported to have a spatialtemporal dynamics, e.g., surface versus subsurface, and in response to climatic and oceanographic conditions, e.g., San Pedro Ocean Time series station (Cram et al., 2015; Parada and Fuhrman, 2017); Monterey Bay (Reji et al., 2019; Tolar et al., 2020), based on their ecological and metabolic traits (e.g., Northwest Mediterranean coast, Galand et al., 2018; Monterey Bay, Reji et al., 2019). Marine microbes from coastal zones are also subjected to anthropological pressure, such as, nutrient fertilization and other pollutants, influencing biogeochemical conditions and oxygen-deficiency (Cavicchioli et al., 2019).
Besides photosynthetic communities, chemoautotrophic assemblages associated with nitrification have been reported to play a central role in the functioning of coastal microbial assemblages (e.g., network analyses, Parada and Fuhrman, 2017; Reji et al., 2020). Nitrification is a chemoautotrophic process associated with a two-steps aerobic reaction that oxidizes ammonia into nitrite followed by the conversion of nitrite into nitrate. Two functional groups represented by ammonia- and nitrite-oxidizing microorganisms catalyze complete marine nitrification, with the rate-limited first step of this reaction performed by ammonia-oxidizing bacteria (AOB) and archaea (AOA) (Ward, 2008). Nitrospira bacteria able to catalyze one-step ammonia oxidation to nitrate (Comammox) represents the only known exception to this rule (Daims et al., 2015; van Kessel et al., 2015). However, the biogeochemical impact of Nitrospira-like Comammox bacteria on the marine nitrogen cycle is still unclear, since these microorganisms appear to be absent in marine ecosystems (Daims et al., 2016).
Phylogenetically complex natural assemblages of ammonia-oxidizing microorganisms include Thaumarchaea, groups 1.1a, 1.1b, and Hot Water Crenarchaeota Group (HWCGIII) and Nitrosocaldus group (Pester et al., 2011), with the group 1.1a representing one of the most ubiquitous and abundant microbial lineages on earth (Karner et al., 2001). In contrast, AOB are less abundant and often undetectable in marine ecosystems (Mincer et al., 2007; Beman et al., 2010; Tolar et al., 2013). Nonetheless, according to the quantification of the functional marker amoA genes (encoding the alpha subunit of the ammonia monooxygenase enzyme), AOB could be more abundant than AOA. For example, higher ratios of benthic AOB-to-AOA amoA gene copies were detected in freshwater and eutrophic waters (Bouskill et al., 2012) or in association with increased amounts of substrate and salinity in estuarine sediments (Bernhard and Bollmann, 2010; Li et al., 2015). In addition, the abundance and potential activity of AOB (as amoA gene counts and transcript counts, respectively) may be important in other marine environments characterized by strong physical-chemical gradients such as nitrification zones in oxygen-deficient areas (Lam et al., 2007) and near-surface depths (Tolar et al., 2013), including diel variability in sunlit coastal waters (Levipan et al., 2016).
Ecophysiological studies indicate a niche differentiation among AOA and AOB strains associated to factors such as temperature (Bayer et al., 2016), pH (Pommerening-Röser and Koops, 2005; Qin et al., 2014; Bayer et al., 2016), light (Guerrero and Jones, 1996; Qin et al., 2014), substrate concentrations (Martens-Habbena et al., 2009), and substrates used for nitrification (Qin et al., 2014; Bayer et al., 2016). For instance, AOA isolates are more photosensitive and grow at lower ammonia concentrations than AOB isolates (Martens-Habbena et al., 2009; Merbt et al., 2012). This is consistent with field studies indicating that natural AOB and AOA populations are commonly associated with high and intermediate to low ammonia concentrations, respectively (Bouskill et al., 2012; Sintes et al., 2013). Moreover, natural AOB and AOA populations may use different substrates such as urea and cyanate to fuel nitrification (Alonso-Sáez et al., 2012; Bowen et al., 2013; Connelly et al., 2014; Palatinszky et al., 2015).
Marine microbial time series (oceanic and coastal) could help to identify long-term trends in the biogeochemical cycles and predict future environmental sceneries, based on their inherent physical-chemical variability. However, there are still relatively few marine time series studies related to the variability of major marine archaeal lineages, besides ammonia oxidizing assemblages, especially in highly productive, oxygen-deficient and dynamic coastal upwelling areas. For example, exhaustive studies have been carried out along the California coast at San Pedro Ocean time series (SPOT) and Monterey Bay Aquarium Research Institute (MBARI) time series (e.g., Murray et al., 1999; Mincer et al., 2007; Beman et al., 2010, 2011; Steele et al., 2011; Robidart et al., 2012; Parada and Fuhrman, 2017; Tolar et al., 2020), at the station ALOHA in Hawai (Karner et al., 2001), at Devil’s Hole in Bermuda (Parsons et al., 2015), and in marine ecosystems such as Chesapeake Bay (Bouskill et al., 2011), the Mediterranean Sea (Galand et al., 2010, 2018; Lambert et al., 2019), and the North Sea (Wuchter et al., 2006). These studies concluded that AOA are the main responsible for ammonia oxidation in oceanic and coastal areas in general.
The coastal area off central-southern Chile (∼36°S) in the eastern South Pacific Ocean is an ideal location to determine the effects of oceanographic dynamics on microbial communities and their biogeochemical activities, since this is a highly productive seasonal upwelling system. Upwelling period occurs during the austral spring-summer modulated by southwesterly winds (Sobarzo et al., 2007). The upwelled water is influenced by the Equatorial Subsurface Waters (ESSW), characterized by cold (∼9–12°C), high-salinity (>34.5 psu), nutrient-rich (reaching > 30 μM of nitrate), and oxygen-depleted conditions (< 1 mL O2 L–1) (Ahumada and Chuecas, 1979). In contrast, strong water mixing events caused by intense northerly winds occur during the austral fall-winter, with water column being characterized by warmer temperatures (10–13°C) and low-nutrient concentrations (e.g., <20 μM of nitrate). Moreover, winter mixing events ventilate the surface and middle depths (>1–6 mL O2 L–1), although low oxygen concentrations (<0.5 mL O2 L–1) persist at bottom depths almost the entire year (Sobarzo et al., 2007). During fall-winter, high precipitation and river-runoff reduce salinity (between ∼32.5 to <34.1 psu) at the surface generating strong stratification in the first 10–20 m depth (Sobarzo et al., 2007; Saldías et al., 2012).
The oceanographic dynamic in central-southern Chile along with the high solar radiation in spring-summer promotes a high primary productivity (Montero et al., 2007; Hernández et al., 2012) and faster remineralization rates (Daneri et al., 2000, 2012), that stimulate nitrification activity. Nitrification is a relevant process in the study area, reaching high net rates in the oxycline during the spring-summer (200–316 nmol L–1 d–1) compared with winter, c.a. 21 nmol L–1 d–1 (Fernandez and Farías, 2012). Recently, ammonia and nitrite oxidation has been detected in this area at nanomolar concentrations of oxygen (5–33 nM, Bristow et al., 2016). Furthermore, molecular studies indicate the presence of an abundant and rich AOA community (Molina et al., 2010; Bertagnolli and Ulloa, 2017), as well as of Nitrospina spp., as the main drivers of nitrification under oxygen-deficient conditions at bottom waters during the spring-summer time (Levipan et al., 2014). In general, the water column is characterized by higher bacterioplankton abundances in summer (Galán et al., 2012), and Nitrosopumilus maritimus predominance (an important AOA ecotype at the Sta.18) in winter months based on metagenomic data (Murillo et al., 2014). Additionally, annual variability of light intensity can favor transient co-variability between bAOB and certain AOA ecotypes to catalyze the first step of nitrification (Levipan et al., 2016).
Here, we investigate physical-chemical variability contribution in shaping the spatiotemporal distribution of marine microbial assemblages including ammonia oxidizers at a coastal upwelling station (Sta.18) using data from a nearly 4-year-long time series. We hypothesize that vertical and seasonal environmental fluctuations will significantly modulate the abundance variability of the microbial community in the study area, whereas oxygen and ammonium will be explanatory variables shaping the distribution and abundances of keystone ammonia oxidizing assemblages.
Materials and Methods
Study Area and Environmental Data Collection
The Center for Oceanographic Research in the Eastern South Pacific (COPAS1; now transformed to other initiatives http://www.sur-austral.cl/). Time-series station 18 is located over the continental shelf off central-southern Chile (36°30′ S; 73°08′ W) and has a maximum depth of approximately 90 m. Station 18 (hereafter, Sta.18) extends approximately 18 nautical miles offshore, and was visited monthly since 2005–2009 on board the R/V Kay Kay-I and -II (University of Concepcion). The wind speed and direction were measured every 10 min at a meteorological station (HOBO) located at Coliumo Bay (36° 31′ 41.2′′ S; 72° 57′ 57.3′′ W) between August 2004 and August 2009. Now different open observatory programs are available further south off Concepción2.
Temperature, salinity, and dissolved oxygen concentrations were obtained using a CTD-O probe (Seabird 23B Electronics, Bellevue, United States). Water samples for chemical (NH4+, NO3–, NO2– and Chlorophyll-a) and biological analyses (picoplankton, DNA extractions, and CARD-FISH) were collected using a rosette sampler equipped with 10 L Niskin bottles. Triplicated NH4+ samples (40 mL) were fixed on board and fluorometrically analyzed (Turner Designs 10-AU fluorometer) using the method proposed by Holmes et al. (1999). Seawater samples for NO2– and NO3– analyses (20 mL) were filtered through glass filter (0.7 μm of pore-size, Millipore) and stored frozen (−20°C) until further processing (Parsons et al., 1984). Chlorophyll-a was determined in discrete seawater samples according to the method described by Holm-Hansen et al. (1965). In addition, discrete seawater samples were fixed in duplicated with glutaraldehyde (final concentration, 0.1% v/v) and stored at −80°C for flow cytometry analysis. Non-fluorescent picoplankton was stained with SYBR-Green I (Molecular Probes) and processed following Marie et al. (1997) on a FACSCaliburTM flow cytometer (Becton Dickinson Biosciences, CA, United States) equipped with a 15-mW argon-ion laser tuned at 488 nm.
DNA Extraction
Seawater samples (3 L) were collected monthly for DNA extraction at different depths (0, 5, 10, 15, 20, 30, 40, 50, and 80 m) from January 2005 to January 2009. The samples were pre-filtered serially through a 20-μm-mesh (Nitex® nylon) and 3-μm-filters (MCE, mixed cellulose ester, Millipore membrane filter), and then filtered onto cellulose ester membrane filters (47 mm diameter, 0.22 μm pore-size GPWP04700) through peristaltic pumping. Each membrane filter was soaked with 1 ml of DNA buffer (50 mM Tris-HCl [pH 9.0], 0.75 M sucrose, 400 mM NaCl), flash-frozen in liquid nitrogen, and stored at −80°C. The DNA was isolated from thawed filters using the phenol-chloroform extraction method as previously described (Molina et al., 2007). In order to estimate the contribution of different ecotypes, seawater samples (3 L) collected at 0, 5, 10, 20, 30, 50, and 80 m depth from two oceanographically contrasting months (August versus December 2011) were extracted by using the PowerSoilTM DNA Isolation Kit (MoBio Laboratories, Solana Beach, CA, United States) in accordance to the manufacturer’s specifications, with minor modifications described by Levipan et al. (2014). All DNA extracts were resuspended in 100 μl of nuclease-free water and their concentration and quality (A260/A280 ratio) were determined by optical absorption spectroscopy on a Synergy Mx Microplate Reader (BioTek Instruments, Winooski, VT, United States). The DNA extracts were stored at −80°C until further analysis.
Quantification of Ammonia-Oxidizing Assemblages
The quantification of AOA and bAOB amoA genes was carried out with the primers Arch-amoAF/Arch-amoAR (Francis et al., 2005) and amoA-1F/amoA-2R (Rotthauwe et al., 1997), respectively. amoA gene copies were quantified for these two groups using standard curves of 10-fold serial dilutions made from amoA gene clones (dilution range, 2 × 107 to 20 copies) as previously described (Molina et al., 2010). The copy number of the amoA clones was calculated by dividing its DNA concentration (in ng μL–1) by its mass (in ng) calculated using the following formula: mass = [(n) × (M/NA)] × 109 where n is the clone size (vector plus insert, in bp); M, is the average molecular weight of a base pair (660 g mol–1); NA, is the Avogadro’s number (6,0221 × 1023 bp mol–1); and 109 is the factor to convert grams to nanograms. Efficiencies and correlation coefficients of standard curves were computed for AOA (E = 91 ± 6%, r2 = 0.99 ± 0.012) and bAOB (E = 84 ± 6%, r2 = 0.994 ± 0.004).
Profiling of amoA gene counts for AOA belonging to the shallow and deep water column clades named as WCA and WCB, and N. maritimus (Group 1.1a) were determined in two oceanographically contrasting months (August vs. December 2011). qPCR assays for these ecotypes were performed with the primer Arch-amoAR along with primers Arch-amoAFA and Arch-amoAFB for WCA and WCB amoA, respectively (Beman et al., 2008). The ecotype N. maritimus-like was quantified with the primer Nmar423F (Levipan et al., 2016) used in combination with the primer Arch-amoAR. These primers combination could also amplify other species such as Nitrosopumilus oxyclinae and Ca. Nitrosarchaeum but not the WCA cultivated representative Nitrosopelagicus brevis (Santoro et al., 2015, see alignment in Supplementary Table 2). qPCR standard curves were generated from 10-fold dilutions of amoA gene clones (2 × 107 to 20 copies) available in our laboratory for N. maritimus (GenBank accession number KJ555107) and WCA and WCB (Molina et al., 2010). The copy number of these amoA clones was calculated using the mentioned formula. PCR efficiencies and correlation coefficients of standard curves for WCA and WCB (E = 91 ± 6%, r2 = 0.989 ± 0.012) and N. maritimus (E = 95.492%, r2 = 0.986) were determined.
All qPCR reactions were performed in triplicate using a StepOneTM Real-Time PCR System (Applied Biosystems, Lincoln Centre Drive, Foster City, CA, United States); data were analyzed with the StepOneTM software package (v.2.2.2; Foster city, CA, United States). Each reaction was conducted on a volume of 20 μL containing 5–10 ng of template DNA, forward and reverse primers (0.4 μM, final concentration), and 2X Fast SYBR® Green Master Mix (1X; Applied Biosystems, 850 Lincoln Centre Drive, Foster City, CA, United States). The qPCR program consisted of an initial denaturation for 20 s at 95°C followed by 40 amplification cycles consisting of 95°C for 3 s, 20 s annealing at a temperature that varied depending on the primer pair used (Supplementary Table 1), and an extension of 20 s at 72°C. A melting curve was performed at the end of each qPCR experiment. The detection limit for standards was always observed at CT values lower than 30. The specificity of qPCR assays was verified by checking dissociation curves and standard electrophoresis of resulting amplicons.
CARD-FISH
Seawater samples were collected monthly since September 2006 to September 2008 (2-year-long time series), fixed after collection with paraformaldehyde (final concentration, 1% v/v), and stored at 4°C until processing. Subsamples (50 mL) obtained from different depths and dates were filtered by vacuum (<10 cm Hg) through 0.2-μm polycarbonate filters (Millipore; 45-mm diameter filters placed over 0.45-μm cellulose nitrate support filters) and then stored frozen (at −20°C) until further CARD-FISH assays. Specific oligonucleotide probes targeting 16S rRNA genes of Thaumarchaea GI.1a (CREN55: HRP-TTAGGCCCAATAATCMTCCT-3′; Massana et al., 1997) and Euryarchaea MG-II (EURY806: HRP-CACAGCGTTTACACCTAG-3′; Teira et al., 2004) were used following procedures described by Sekar et al. (2004) and Woebken et al. (2007). A mix of three eubacterial probes (EUB338, HRP-GCTGCCTCCCGTAGGAGT-3′; EUB338-II, HRP-GCAGCCACCCGTAGGTGT-3′; and EUB338-III, HRP-GCTGCCACCCGTAGGTGT-3′) was used to detect total bacterial cells (Amann et al., 1990; Daims et al., 1999). The NON338 probe (HRP-ACTCCTACGGGAGGCAGC-3′; Wallner et al., 1993) was used to detect false positive in hybridization experiments. Briefly, probes were hybridized during 4 h (EUB338 mix and NON338) and 8 h (CREN55 and EURY806) at 35°C in a standard hybridization buffer (360 mM NaCl, 8 mM Tris-HCl pH 8, 0.008% w/v SDS, 0.4% w/v blocking reagent, 1% w/v dextran sulfate, and the respective probe). The wash temperature for removing non-specifically hybridized probes was set at 37°C for 15 min. Depending upon the probe, the stringency of the hybridization reaction was regulated with different formamide percentages (% v/v) in the hybridization buffer and different NaCl concentrations in a standard washing buffer (2.5 mM EDTA, 20 mM Tris-HCl). The amount of formamide/NaCl (%/mM) used for archaeal (CREN554 and EURY806) and non-archaeal probes (EUB338 mix and NON338) was 20%/3 mM and 55%/135 mM, respectively. Prokaryotic cell abundance was estimated using 4, 6-diamidino-2-phenylindole (DAPI) staining (Porter and Feig, 1980) and an epifluorescence microscope (Zeiss Axioplan 2) equipped with a 100 W Hg lamp (HBO 103W/2, Osram, Germany) and an appropriate filter set for 5-(6-) FAM and DAPI dyes. Ten epifluorescence photomicrographs (1000 X) were randomly taken from each sample, counting between 500-1000 DAPI-stained cells with the AxioVision software (Release 4.6.3.).
Statistical Analyses
The abundances of total non-photosynthetic picoplankton, Thaumarchaeota (formerly known as marine GI Crenarchaeota; Massana et al., 1997), AOA, and bAOB, were modeled in response to the spatiotemporal variability (season and depth) and chemical variability (ammonium, nitrite, nitrate, and oxygen) using Generalized Additive Models for Location, Scale, and Shape (GAMLSS) from R ‘gamlss’ package (Rigby and Stasinopoulos, 2005). MG-II Euryarchaea was not included in this analysis because there were temporal gaps in its abundance, but a Spearman correlation analysis indicates that it is significantly correlated with non-photosynthetic picoplankton in surface waters (R = 0.4; P = 0.0013). Predictive variables showed a high collinearity (up to Spearman’s R = −0.79, P < 0.001) due to the strong seasonality in wind-driven upwelling conditions. To solve this problem, for each microbial group, we fitted a spatiotemporal model with season and depth as predictive variables, and independent models for every chemical parameter used as a predictive variable (i.e., nitrite, nitrate, ammonium, and oxygen). Before running the analysis, predictive variables were scaled (Z-transformed) to allow a direct comparison between parameters derived from resultant models (Schielzeth, 2010). In order to count both skewness and leptokurtosis of the response variables, microbial groups were modeled by using the follow distributions (Rigby and Stasinopoulos, 2005): (i) negative binomial type I, (ii) negative binomial type II, and (iii) Box-Cox t (Table 1). Model diagnostic procedures were conducted (to choose the most appropriate distribution) using (i) the plot.gamlss function which provided a series of plots for checking normalized quantile residuals of the fitted models, and (ii) the Generalized Akaike Information Criterion (GAIC). Microbial groups were modeled as a linear function of variables seasonality, ammonium, nitrite, nitrate, and oxygen. The depth was adjusted using a non-parametric cubic smoothing spline function available in R ‘gamlss’ package.
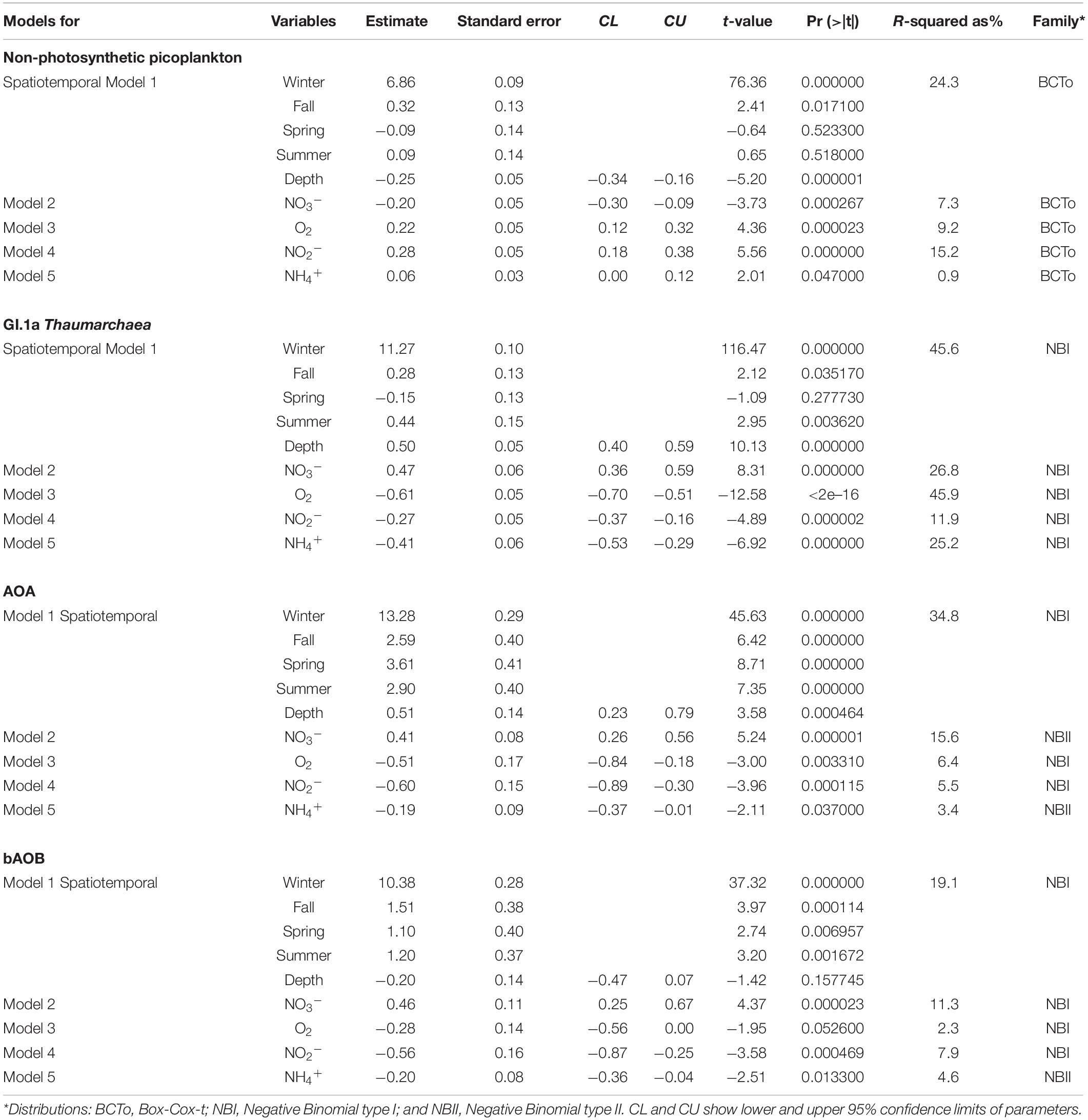
Table 1. Parameters obtain from the microbial modeling (GAMLSS) as function of different predictor variables.
The data that support the findings of this study are available from the corresponding author upon reasonable request.
Results
Seasonal Regime of Upwelling-Favorable Winds and Physical-Chemical Variables in the Study Area
Upwelling-favorable winds (i.e., southwesterly) usually extended from September (early austral spring) to April (early austral fall) in the study area, however, inter-annual differences were detected during our temporal framework (Supplementary Figure 1). The 2004–2005 annual wind cycle showed the most intense southerly winds and the highest accumulation of wind-stress, whereas the lowest accumulated wind-stress was observed during the 2005–2006 annual wind cycle. Upwelling-favorable winds in the 2007–2008 annual wind cycle were similar to those from 2005 to 2006 cycle in terms of their respective starting points-spring transition (early October), but the former was more intense and prolonged. Moreover, upwelling-favorable winds from the 2006 to 2007 and 2008 to 2009 annual wind cycles showed the earliest start (mid-July to early August) and strongest intensities (Supplementary Figure 1).
During the austral spring-summer, upwelled waters were characterized by low temperatures (∼9–12°C), and high salinities (>34.5) and densities (≥26 kg m–3), influencing surface layer; whereas during non-upwelling periods the densest water was just restricted to bottom depths (Supplementary Figure 2). The upwelled water was also characterized by suboxic conditions (≤22 μM), and its influence was perceptible up to almost 15 m depth, mostly from November to April (Figure 1). Oxic conditions (>200 μM) were restricted in the first 5–10 m depth over the entire study period and at intermediate depths during the austral fall-winter (i.e., upwelling-unfavorable period) (Figure 1). In general, during the upwelling season, high concentrations of ammonium (>1 μM) were detected at the base of the mixed layer (∼10 m depth), except during the 2005–2006 upwelling period, when elevated ammonium concentrations (up to ∼3 μM) were found at almost the entire water column (Figure 1). During this period, also high nitrite concentrations (∼7 μM) were normally found in the water layer below 40 m depth (Figure 1), except during the 2005–2006 upwelling period, when higher nitrite concentrations were registered both in bottom waters and oxycline. Surface waters showed lower concentrations of nitrate (≤10 μM) during the entire study period, while the higher concentrations of this nutrient (up to ∼35 μM) were detected at mid-depth waters (between ∼20 and 50 m) but not just associated with the seasonal upwelling signature (Figure 1).
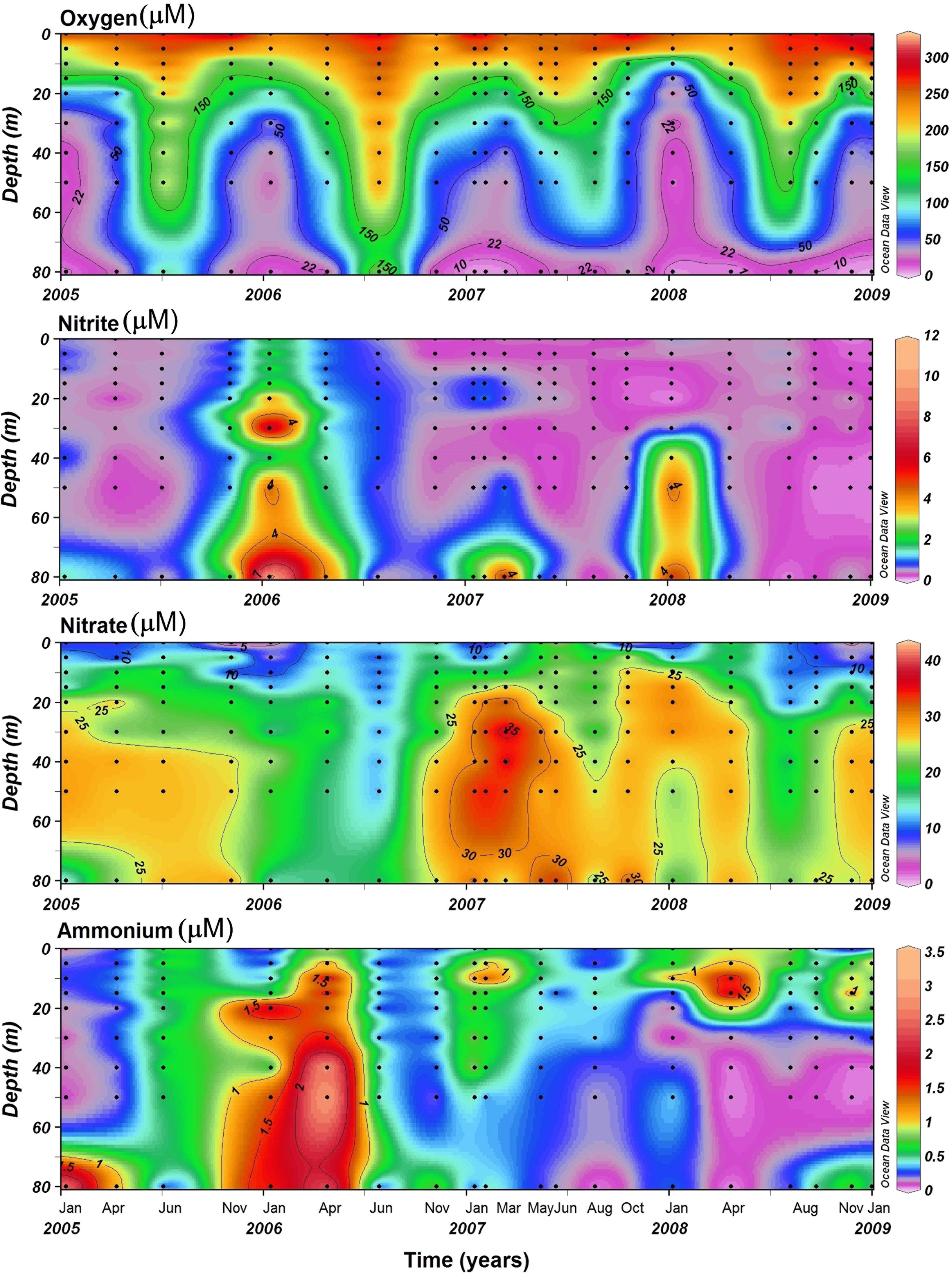
Figure 1. Spatiotemporal variability of dissolved oxygen, ammonium, nitrite, and nitrate at the time series station (St.18). Dots indicate specific date and depth samplings.
Spatiotemporal Variability of Chlorophyll-a and Microbial Assemblages
During fall-winter months (upwelling-unfavorable seasons), chlorophyll-a concentrations were always ≤ 5 mg m–3 throughout the water column (Figure 2). In contrast, during spring-summer months, the highest chlorophyll-a concentration (i.e., >10 mg m–3) was tightly associated with surface waters (between 0 and ∼20 m depth), except by the 2006–2007 spring-summer period, when the lower chlorophyll-a concentrations were detected and the span of maximal chlorophyll-a concentration finished earlier than the other years (Figure 2). Cell abundances of the non-photosynthetic picoplankton ranged between 0.23–6.50 × 106 cells mL–1 in the water column, reaching their maxima at surface and bottom waters during late summer and fall, and minima in winter months (Figure 2).
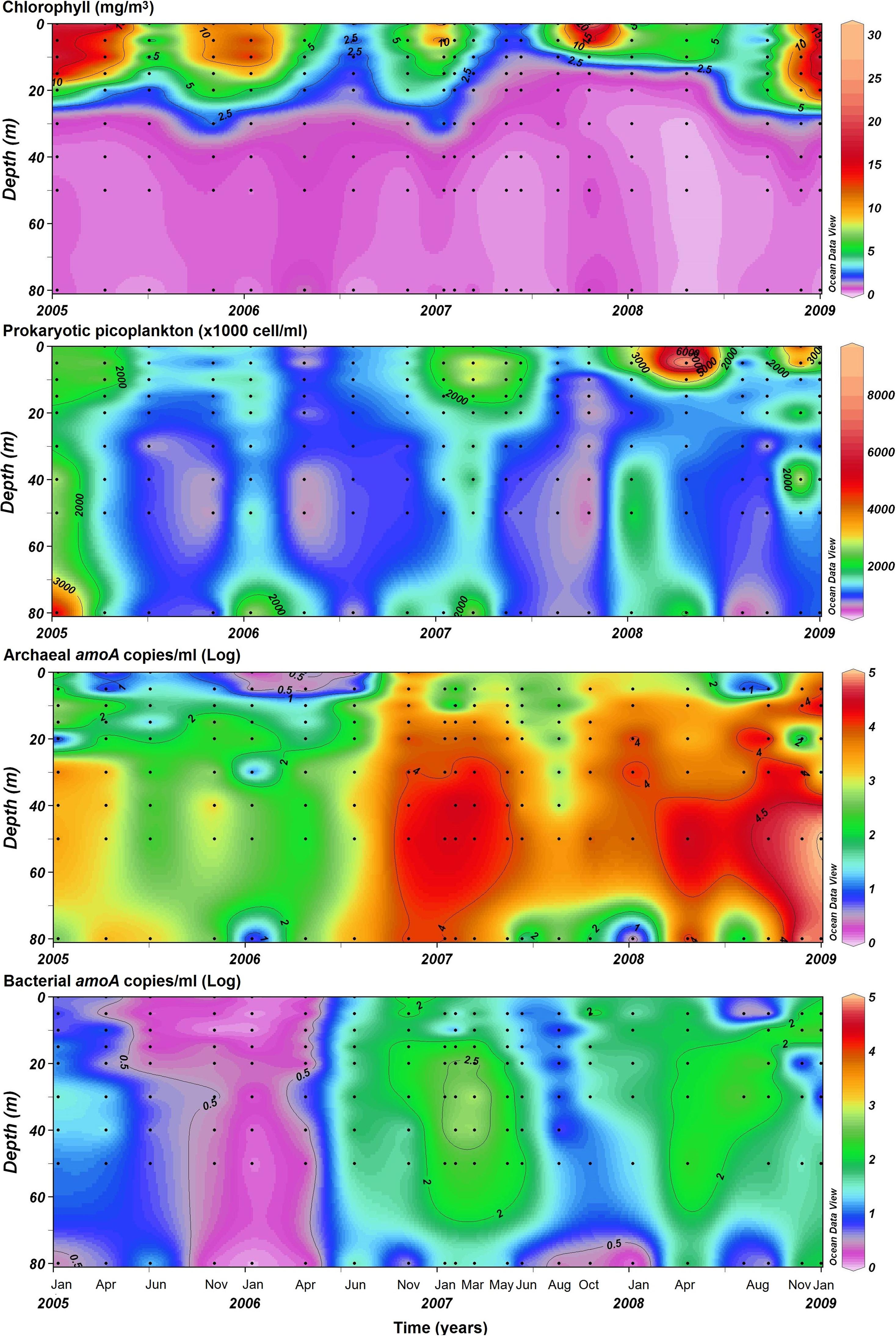
Figure 2. Spatiotemporal variability of chlorophyll concentration, non-photosynthetic picoplankton abundance, and amoA gene counts for general AOA and bAOB at the time series station (St.18). Dots indicate specific date and depth samplings.
Cell abundances of GI.1a Thaumarchaea and MG-II Euryarchaea (referred to as Thaumarchaea and Euryarchaea in the following sections) are shown in Supplementary Figure 3. The abundance of Euryarchaeota range between zero and 78 × 104 cells mL–1, accounting for 9.6 ± 9.0% of total picoplankton community (determined by counting DAPI-stained cells) and showing four maxima in the first approximately 30 m depth (Supplementary Figure 3). Moreover, its abundance decreased with depth, with only one Euryarchaeota maxima detected at depth during late summer 2008 (see march-associated peak). In contrast, cell abundances of Thaumarchaea were usually between ∼0.2 and 85 × 104 cells mL–1 and more abundant during late summer and winter. This pattern was observed in deeper waters, where this group was accounted for 25.1 ± 13.8% of DAPI counts detected below the oxycline (Supplementary Figure 3). In addition, the contribution of Thaumarchaea to the whole picoplankton community (as % of the total number of DAPI-stained cells) was as high as 50% below 50 m depth (Supplementary Figure 3).
In total, ammonia-oxidizing assemblages amoA gene copies quantification indicated that AOA amoA outnumbered bAOB amoA by up to an order of magnitude (Figure 2). Moreover, if it is considered that one amoA gene copy corresponds to one cell, AOA and bAOB were accounted for 1.2 ± 2.9% and 0.008 ± 0.015% of total abundance of the non-photosynthetic picoplankton (determined from flow cytometry) in the entire water column over time, respectively. In addition, AOA amoA was detected in the entire water column, but especially at the oxycline (up to 105 gene copies mL–1 between ∼20 and 40 m), during the summer 2006–2007. In 2005, 2006, and 2008, high AOA amoA abundance was determined below the oxycline (40 m depth) during fall and winter (between April and August, Figure 2). The AOA amoA -to- Thaumarchaeota cell ratios ranged between 2.75 × 10–7 and 0.72, showing higher numbers in spring followed by fall, summer and winter (Supplementary Figure 4). The number of bAOB amoA ranged from undetectable (i.e., <2 copies mL–1) during the 2005–2006 upwelling-favorable period up to 104 copies mL–1 in the oxycline from mid-2006 onward, following the variability of AOA amoA, but usually centered at the oxycline and intermediate depths, when detected (Figure 2).
The comparison of bAOB and AOA amoA gene copies and with the AOA amoA/Thaumarchaeota cell ratios showed that the relative contribution of bAOB amoA was separated in spatial and seasonal scale; bAOB were favored at surface and intermediate waters but mainly during summer (Supplementary Figure 5A). Summertime was characterized by low ratios of archaeal nitrifying assemblages based on AOA amoA/Thaumarchaeota cells ratios (Supplementary Figure 5B). In fact, a plateau of ∼181 bAOB amoA (i.e., ∼2.26 as log-transformed value) was determined when AOA amoA/Thaumarchaeota cells ratios were >0.2, usually associated with surface and intermediate depths during summer (Supplementary Figure 5B).
The spatiotemporal differences in gene number or cell abundance between the studied microbial groups (except for Euryarchaeota) are shown in Figure 3. Data derived from Generalized Additive Models for Location, Scale, and Shape (GAMLSS) statistical analyses are shown in Table 1. In general, a significant decrease in microbial abundance was observed during winter, more evident for ammonia oxidizing assemblages and spring for Thaumarchaeota. GAMLSS analyses indicated that spatiotemporal variations (i.e., season and depth) account for >19.1% of the fluctations, i.e., in non-photosynthetic picoplankton (24.3%), Thaumarchaeota, (45.6%), AOA amoA (34.8%) and bAOB amoA (19.1%) in the water column (see model 1 in Table 1).
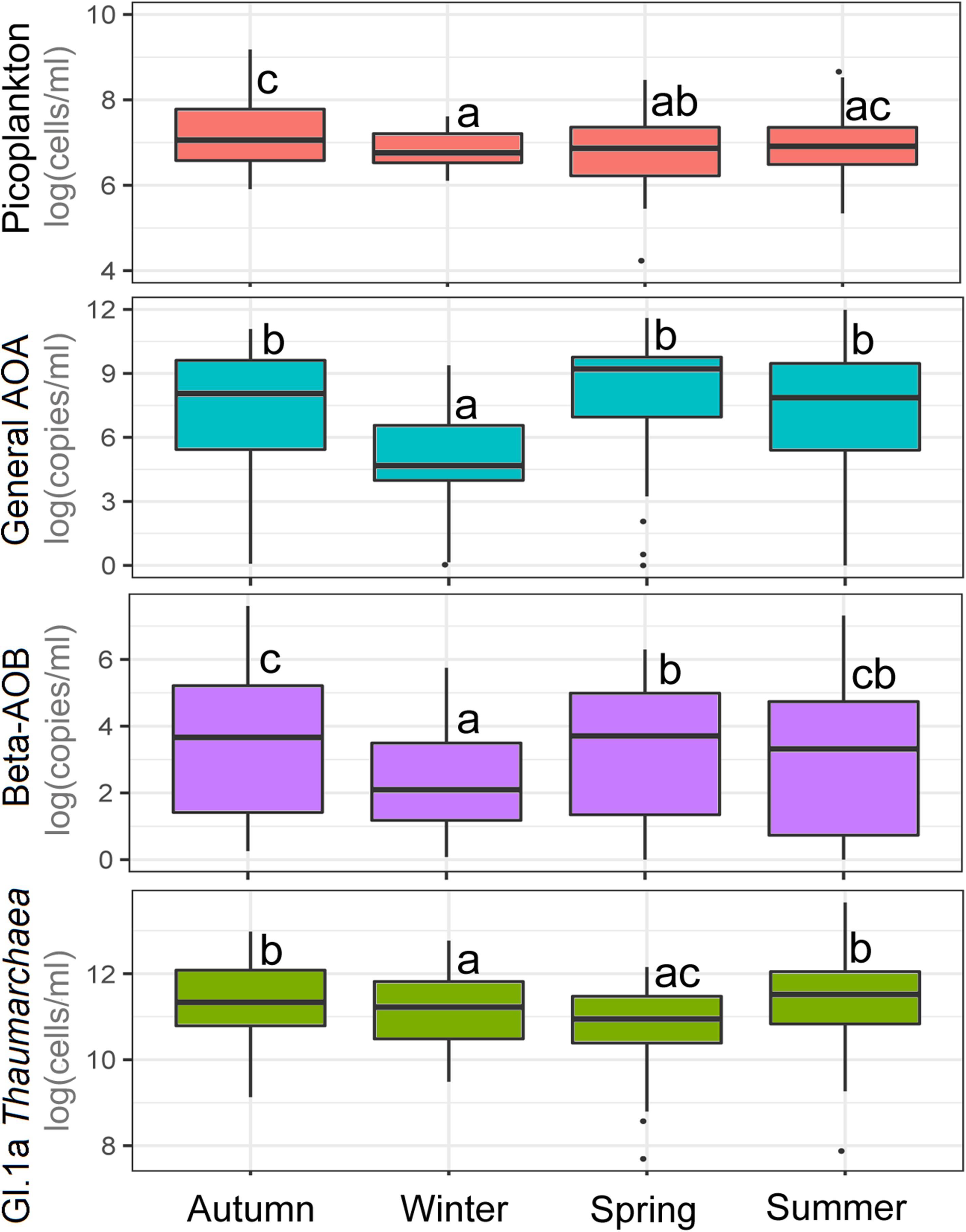
Figure 3. Seasonal variation in abundance of major structural components of prokaryote community in the study area. Box plots show the medians, upper/lower quartiles (boxes) and value range (vertical lines) of data sets. Different letters denote significant differences at a P-value of <0.05.
The distribution of different archaeal ecotypes (N. maritimus, WCA and WCB) was studied only for selected months during winter and summer of 2011 in order to decipher the relevance of specific groups within the AOA amoA (Figure 4). The ammonia-oxidizing ecotypes presented similar trends with depth than the average values obtain at the time series distribution, showing a weak peak at the subsurface (∼10 m depth) and higher values below 30 m depth for AOA amoA during both summer and winter months. N. maritimus amoA presented higher magnitudes followed by WCA, WCB, and bAOB amoA. The most relevant difference observed was that during winter, N. maritimus, WCA, and bAOB amoA peaked at intermediate depths (50 m depth), whereas during summer, WCB amoA maximum was determined at intermediate to bottom depths (50–90 m) (Figure 4).
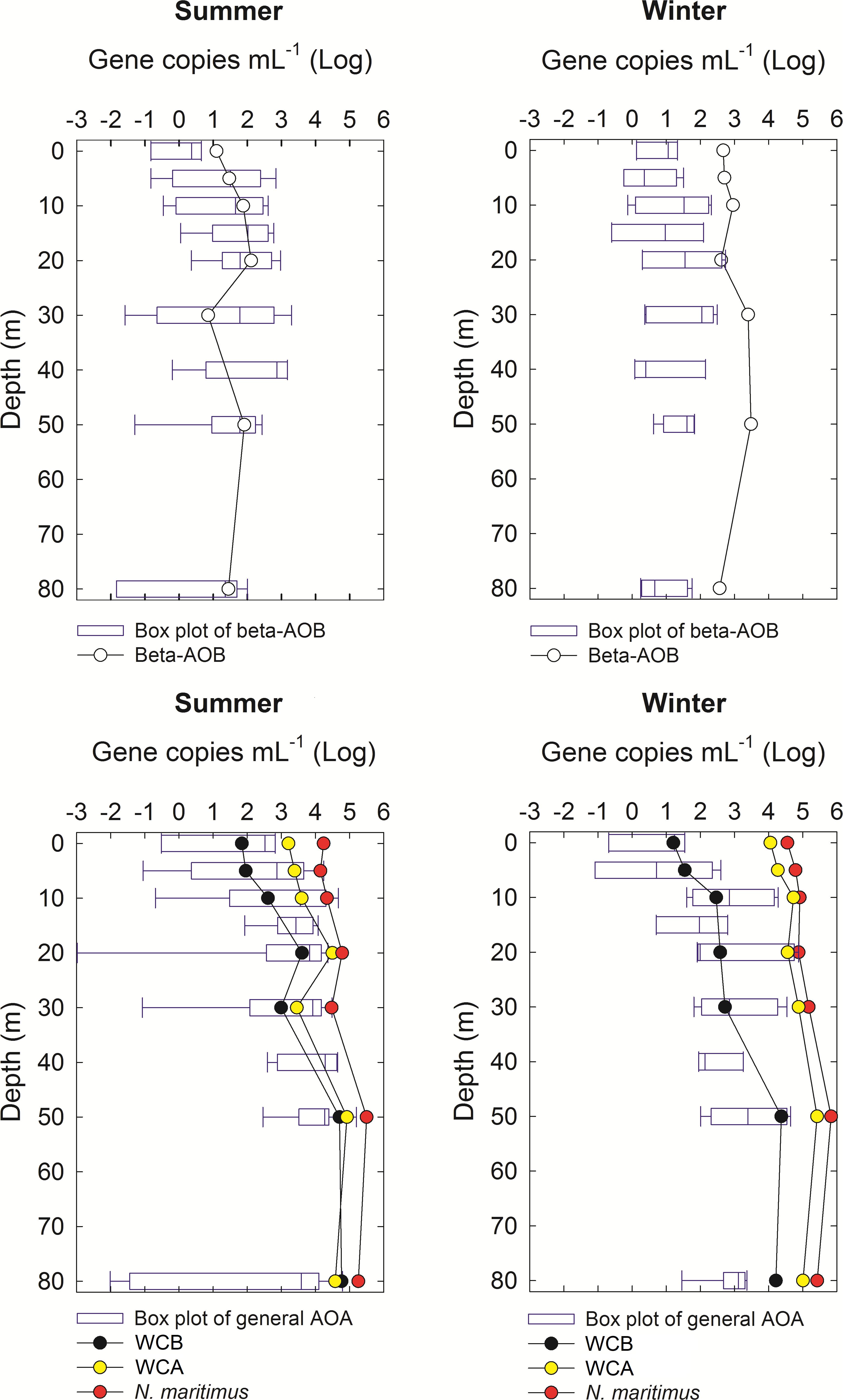
Figure 4. Vertical profiles of the main ammonia-oxidizer ecotypes (amoA gene counts in a log-transformed scale) at the St.18 during two contrasting oceanographic months, that is, a representative month for the unfavorable (August 2011, austral winter) and favorable conditions (December 2011, austral summer) for upwelling. For the sake of comparison, the seasonal average for bAOB and general AOA are shown in blue. Error bars are standard deviations.
Environmental Forcing of Microbial Communities, Including Ammonia-Oxidizers
The distribution of total non-photosynthetic picoplankton (flow cytometry) was significantly and positively associated with oxygen and nitrite, and negatively associated with nitrate and depth (Figure 5). Nitrite account for 15.1% of non-photosynthetic picoplankton spatiotemporal variability (Table 1). In contrast, Thaumarchaeota cell abundances were negatively correlated with oxygen, ammonium, and nitrite, and positively associated with nitrate and depth (Figure 5). Oxygen (45.9%, ammonium (25.2%) and nitrate (26.8%) were the explanatory variables for the Thaumarchaeota fluctuations (Table 1). A similar tendency was found for AOA amoA (Figure 5), but the most explicative variable was nitrate (15.6%) (Table 1). Except for depth, bAOB amoA were significantly correlated with ammonium, nitrite, and nitrate (Figure 5). Nitrate (11.3%) was the variable with the higher explanatory percentage to the bAOB amoA distribution in the time series (Table 1).
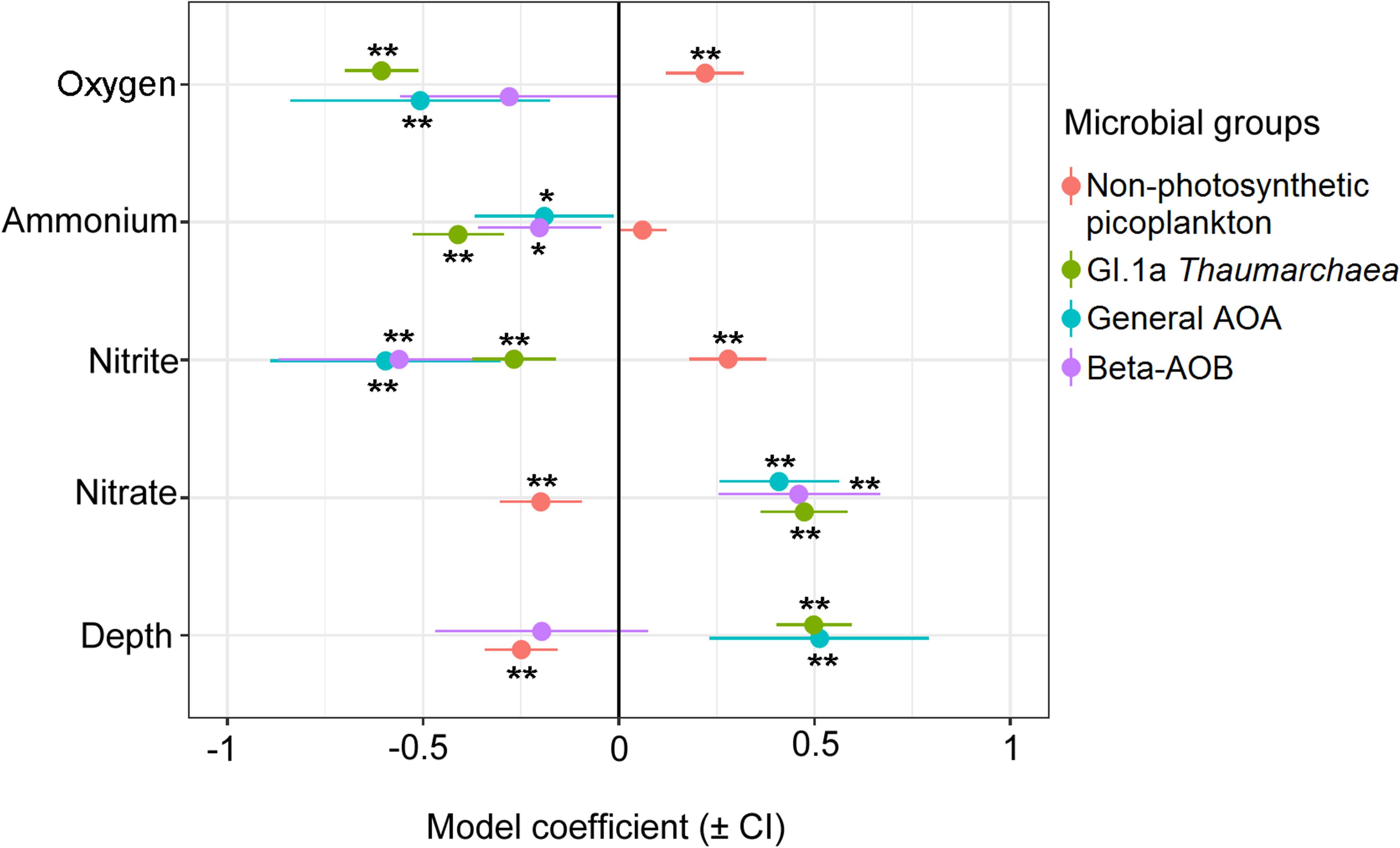
Figure 5. Coefficient plot of prokaryote community in response to physical-chemical variables. Error bars represent confidence intervals. Significance are indicated by asterisks (i.e., *P < 0.05; **P < 0.001).
Discussion
The biological activity in the study area is mainly influenced by seasonal upwelling events and its remote-forcing variability; see examples in the special volume for the COPAS time series (Escribano and Morales, 2012; Farías et al., 2015). In the present study, upwelling-favorable conditions were associated with higher phytoplanktonic biomass (chlorophyll) and abundances of total non-photosynthetic picoplanktonic communities, except during the 2005–2006 upwelling-favorable period for the non-photosynthetic picoplanktonic component (Figure 2). In fact, upwelling favorable winds cumulative intensity denoted an evident interannual upwelling variability during our study timeframe, characterized by a delayed and less intense events triggered during 2005–2006 (Supplementary Figure 1). The influence of upwelling variability on non-photosynthetic picoplankton has been reported in other seasonal upwelling ecosystems, such as, northwestern Indian Ocean (Wiebinga et al., 1997) and Benguela (Bergen et al., 2015) and agrees with previous results from the study area (Cuevas et al., 2004; Daneri et al., 2012).
In the study area, upwelling not only fertilizes the surface with high-nutrient content waters fueling primary and secondary productivity (e.g., Montero et al., 2007), but also develops a seasonal oxygen deficiency in the subsurface associated with Equatorial Subsurface Waters (ESSW) which was evident during our study (Ahumada and Chuecas, 1979; Sobarzo et al., 2007). Oxygen has been reported as a controlling factor of microbial community abundance (Eissler et al., 2010), structure (Aldunate et al., 2018), and biogeochemical active processes (Galán et al., 2014, 2017; Srain et al., 2020) in the study area. During our study, GAMLSS analyses indicated that the spatiotemporal variability in non-photosynthetic picoplanktonic cell abundance (studied by flow cytometry) was significant in the study area, and among the variables studied, oxygen and nitrite largely account for the changes observed (Table 1). Both, oxygen and nitrite are associated variables in subsurface waters, since nitrite accumulation depends on the redox conditions in the water column triggered by oxygen deficiency (e.g., Farías et al., 2015). Nitrite is a key intermediary of many biogeochemical processes, i.e., as a product of aerobic ammonia oxidation and nitrate reduction, and as a substrate for aerobic nitrite oxidation, anammox and nitrite reduction related with heterotrophic and chemoautotrophic denitrification (Ward, 2008). In fact, coupling of keystone OMZ microbial communities such as SUP05 clade with anammox and denitrifying sulfur-oxidizers were determined to be associated to nitrite based in a biogeochemical model in the Saanich Inlet seasonally anoxic fjord (Louca et al., 2016) and in bottom waters during upwelling periods in the study area (Galán et al., 2014).
Variability of Euryarchaea Cells Using CARD-FISH
Euryarchaea and Thaumarchaea presented a differential temporal and spatial distributions in the water column. Euryarchaea was characterized by a higher contribution at surface seawater at fall or spring. This result agrees with other findings on a temporal rather than a spatial scale since Euryarchaeota showed a narrower distribution than previously reported (Levipan et al., 2007a; Quiñones et al., 2009). However, sampling methodological constraints could influence these spatial differences, that is, our study was focused on free-living fraction cell counts, whereas the mentioned reports involved bulk DNA analyses (7 L samples) that favor the presence of different particle sizes. Particle aggregates seem to enhance the contribution of Euryarchaea off northern Chile (Ganesh et al., 2014) and in the study area (Levipan et al., 2007a), where methylotrophic methanogens were abundant and viable at depth in a large-particle fraction (between 0.22 and 25 μm). Moreover, differences in vertical distribution patterns of Euryarchaea caused by the filter size fraction have also been reported in contrasting areas near ALOHA time series station (Lincoln et al., 2014) and Oregon coast (Smith et al., 2013). In addition, our results could indicate that using CARD-FISH distinct phylotypes were detected, associated with euphotic MGII during 2006 – 2007, and also at subsurface during 2008, supported by previous findings of a wider distribution and diversity within Euryarchaeota as in other coastal areas, e.g., SPOT (Parada and Fuhrman, 2017).
The Spatiotemporal Distribution of Thaumarchaeota Cell Abundances
Unlike Euryarchaeaota, thaumarchaeal cells were found to be favored in subsurface waters, characterized by peaks at the oxycline during fall and spring (2007) and maxima at the bottom during wintertime and late summer 2008. Thaumarchaeal cells made up the bulk fraction of the prokaryote community at deep waters and represented a large fraction of the archaea considering the sum of Euryarchaeota and Thaumarchaeota (Supplementary Figure 3). These results are supported by previous findings in the Eastern South Pacific (ESP) off Chile, based on rRNA dot blot hybridization (Levipan et al., 2007b; Quiñones et al., 2009) and lipid-based approach (Rossi et al., 2012; Srain et al., 2015). GALMSS analysis indicates that Thaumarchaeota was the microbial group with the greater percentage of predictability associated with its spatial and temporal variability (Table 1), and with all the other variables (oxygen, nitrate, ammonium and nitrate), associated with substrates and products of nitrification. In general, these results support a recent report considering the water column position (euphotic, 50 m, and deeper waters 60 – 500 m) and other factors such as hydrographic explanatory variables, including oxygen, nitrite and ammonium Thaumarchaeota contribution to total microbial community (iTag-16S rRNA) variability in the Monterey Bay coastal time series (Tolar et al., 2020). However, in the case of the time series off Concepción the Thaumarchaeota depth distribution is resolved within a shallow water column depth (92 m depth).
Contribution of Ammonia Oxidizing Archaea in the Study Area
The AOA amoA abundance results indicate that AOA in the study area reached comparable abundances with those determined in other marine ecosystems, e.g., the northern Gulf of Mexico (up to 105 copies mL–1 at ≤100 m depth; Tolar et al., 2013), Sargasso Sea or ETNA (up to 105 copies mL–1, Löscher et al., 2012; Newell et al., 2013), North Sea in winter months (from 104 up to 105 copies mL–1, Wuchter et al., 2006; Herfort et al., 2007; Pitcher et al., 2011b), and within the oxycline and just over the upper limit of the OMZ in the Arabian Sea (up to 105 copies mL–1, Newell et al., 2011; Pitcher et al., 2011a; Bouskill et al., 2012). However, variable ratios between amoA and 16S rRNA genes or cell counts have been observed in marine ecosystems (e.g., Wuchter et al., 2006; Mincer et al., 2007; Agogué et al., 2008). In our study, a variable contribution of AOA amoA versus Thaumarchaeota cell counts determined through CARD-FISH was observed, reaching higher ratios during spring and fall (0.2 – 0.7) compared with <0.2 ratios observed during summer and winter (Supplementary Figure 4). Similar values were obtained in surface waters from Monterey Bay (Mincer et al., 2007) and in subsurface (100 – 150 m depth) from subtropical and equatorial regions of the North Atlantic (Agogué et al., 2008). However, lower ratios of AOA amoA to Thaumarchaeota 16S rRNA gene ratios (<0.01) were associated with the presence of non-nitrifying Thaumarchaea from bathypelagic waters (Agogué et al., 2008) or with methodological biases (Mincer et al., 2007). Alternatively, different ecotypes could account for a wide range of AOA amoA versus Thaumarchaeota 16S rRNA gene ratios (e.g., Sintes et al., 2013). GAMLSS modeling indicates that similar predictive variables account for both Thaumarchaeota cell and AOA amoA fluctuation (Table 1).
Contribution of Ammonia Oxidizing Archaea Ecotypes in a Representative Upwelling Versus Non-upwelling Season Profile
AOA in the study area were found to be associated to the candidate order Nitrosopumilales (Stieglmeier et al., 2014), and to other ecotypes, i.e., Water Column Surface clade (WCA) with one culture Candidate Nitrosopelagicus brevis and Water Column Deep clades (WCB), as in other coastal marine time series (e.g., Beman et al., 2008; Santoro et al., 2010, 2015; Reji et al., 2019; Tolar et al., 2020). The ammonia-oxidizing assemblages were predominantly characterized by N. maritimus-like followed by WCA amoA reaching higher abundances during winter, whereas the deep WCB amoA ecotype showing maxima during summer at the bottom. This result was supported by previous reports in the study area based on AOA amoA survey using clone libraries (Molina et al., 2010), and iTag sequencing (Bertagnolli and Ulloa, 2017) using primers designed by Pester et al. (2012). These previous studies revealed specific predominant contribution of the WCA amoA in both upwelling and non-upwelling season and of the deep WCB amoA during the upwelling period. The WCB was strongly coupled to oxygen deficient conditions, and was suggested to be advected by upwelling waters toward the continental shelf (Bertagnolli and Ulloa, 2017). Indeed, WCA and N. maritimus amoA ecotypes are the main AOA in the study area in terms of the abundances of amoA genes and transcripts, and related to ammonium oxidation (Molina et al., 2010; Bristow et al., 2016; Levipan et al., 2016). In addition, both archaeal ecotypes, but mainly WCA, appear to have an important role in the ammonium oxidation in other marine ecosystems; e.g., the Gulf of California (Beman et al., 2008), Monterey Bay (Smith et al., 2014; Tolar et al., 2020), from the equatorial Pacific to the Arctic (Shiozaki et al., 2016), and the suboxic zone of the central Baltic Sea (Labrenz et al., 2010). Nonetheless, N. maritimus-like ecotypes may be less abundant at lower latitudes of the ETSP (∼12 and 18°S; Peng et al., 2013).
Ammonia Oxidizing Bacteria and Its Interaction With Their Archaea Counterparts in the Study Area
The abundance of bAOB amoA ranged from the limit of detection (2 copies) to 104 copies mL–1 (Figures 2, 4), reaching higher numbers mainly during spring-summer at intermediate depths. This range was lower than that previously described in the ETSP (103 to 105 copies mL–1, Bouskill et al., 2012; Löscher et al., 2012), similar to the range reported by Wuchter et al. (2006) in the North Sea, and wider than that found in locations such as Monterey Bay (undetectable to 103 copies mL–1, Mincer et al., 2007), shallow waters in the California Current (undetectable to 102 copies mL–1, Santoro et al., 2010, 2013), Gulf of California or ETNP (undetectable to 102 copies mL–1, Beman et al., 2008, 2012), and within the oxycline in the Arabian Sea (from ∼102 to 103 copies mL–1, Newell et al., 2011; Bouskill et al., 2012).
Spatiotemporal followed by nitrate and ammonium were significant explanatory variables for bAOB amoA, distribution. In addition, the variability in bAOB amoA appears to be constrained by AOA distribution. Shifts in bAOB amoA were more obvious when plotted against the AOA amoA versus Thaumarchaeota cell ratios estimated from CARD-FISH counts (Supplementary Figure 5B). These results show that the number of bAOB amoA was maximum in surface waters during summer-fall when low AOA amoA versus Thaumarchaeota cell ratios were found, and reached a plateau at spring-time. This finding suggests that competitive interactions between AOA and bAOB amoA are more intense in surface waters during springtime and were potentially associated with lower ammonium availability and/or oxygen deficiency. The numerical dominance of AOA amoA in the study area could be attributed to the well-known kinetic differences in ammonium uptake between AOA and bAOB (Martens-Habbena et al., 2009). It is known that ammonium concentration promotes niche separation not only between phylogenetically distant species of AOA and AOB (Schleper, 2010), but also between different clusters of AOA (Sintes et al., 2016). In the present study, ammonia-oxidizing assemblages significantly varied with ammonium (model 5 in Table 1), suggesting niche overlapping between AOA and AOB and hence substrate competition for ammonium. Nevertheless, in contrast to Fernandez and Farías (2012), who reported that bacterial Ammonium oxidation might benefit from low oxygen concentrations, we found that the oxygen concentration was a statistically meaningful predictor for AOA amoA but not for bAOB amoA distribution (models 3 in Table 1), suggesting that oxygen concentration can be involved in niche differentiation between AOA and bAOB amoA (models 1 and 3 in Table 1). Moreover, our results support the key role that oxygen concentration plays in AOA-A and B ecotypes partitioning in this and other areas (Beman et al., 2008; Bertagnolli and Ulloa, 2017), as well as in controlling (at low concentrations) the distribution and activity of marine AOA populations (Qin et al., 2017).
Ammonia oxidizing assemblages are significant contributors to biogeochemical cycling in the study area associated with high rates of nitrification that are correlated with the availability of ammonium mainly in the oxyclines in the study area (Fernandez and Farías, 2012). Moreover, in a 10-year (2002–2012) biogeochemical report associated with nitrous oxide dynamics the COPAS time series, nitrous oxide positive excess, mainly in the oxyclines and subsurface waters, was associated with nitrification (Farías et al., 2015; Galán et al., 2017), as in other oxygen deficient areas of the Pacific Ocean, e.g., Löscher et al. (2012) and Frey et al. (2020). In order to compare potential role of microbial communities studied here, the average monthly inventories of nitrous oxide reported by Farías et al. (2015) for the 2005 – 2009 upwelling periods were compared with nitrate, ammonium, AOA, bAOB and non-fluorescent picoplanktonic community estimated inventories in our study (Supplementary Figure 6 and Table 2). This comparison helps us identify the connection between ammonia oxidizing assemblages with Nitrous oxide and nitrate mainly during 2007 – 2009, but not for the previous upwelling periods. During 2005–2007, nitrous oxide and nitrate inventories could be associated with high ammonium accumulation and an enrichment of picoplanktonic communities with unknown identity in the bottom (Supplementary Figure 6). Therefore, changes in microbial assemblages are associated with upwelling variability, generating significant impact in the water column biogeochemistry in this natural oxygen – deficient upwelling ecosystem.
Conclusion
In total, our results indicate the physical-chemical oceanographic conditions associated with the seasonal upwelling systems modulate the distribution and dynamics of abundant marine microbial groups including key ammonia oxidizing assemblages. The statistical GAMLSS analyses indicate that spatiotemporal changes associated with biogeochemical conditions trigger by oxygen-deficiency, including substrate and products of nitrification, were significant predictors shaping the marine microbial community distribution. Biological interactions including AOA and bAOB, and possibly with other microbial groups in surface waters influences the temporal dynamics of ammonia oxidizing assemblages. Considering the key role that coastal marine microbial community plays in the cycle and the balance of nutrients and carbon at a global scale, an appropriate understanding of their natural variability will allow to better predict the metabolic and biogeochemical consequences associated with the expected expansion and intensification of oxygen deficient zones due to anthropogenic forcing.
Data Availability Statement
The raw data supporting the conclusions of this article are given in Supplementary Table 2 and complementary data will be made available by the authors, without undue reservation.
Author Contributions
VM, LB, and HL wrote the manuscript with the support from SR-F, CA, AG, IM, and OU. VM and LB conceived the original idea. VM and OU supervised the project. All the authors contributed to the article and approved the submitted version.
Funding
This study was funded by the Comisión Nacional de Investigaciones Científicas y Tecnológicas (CONICYT) through the FONDECYT Grant Nos. 1110824, 1171324 (CONICYT, Chile), FONDAP COPAS Center and by the ANID – Millennium Science Initiative Program, Grant ICN12_019-IMO.
Conflict of Interest
The authors declare that the research was conducted in the absence of any commercial or financial relationships that could be construed as a potential conflict of interest.
Acknowledgments
We thank the time series station program of the COPAS Center of the University of Concepción for providing environmental data. We acknowledge the help provided by Kay Kay crew and technical support of S. Collado, M. Montoya, F. Santibañez, R. De la Iglesia, and G. Alarcon at the field and laboratory.
Supplementary Material
The Supplementary Material for this article can be found online at: https://www.frontiersin.org/articles/10.3389/fmars.2020.561597/full#supplementary-material
Supplementary Figure 1 | Annual cumulative alongshore (south–north) wind-stress starting from August. Different line colors indicate different annual wind cycles.
Supplementary Figure 2 | Spatiotemporal variability of temperature, salinity, and density at the time series station (Sta.18).
Supplementary Figure 3 | Spatiotemporal variability of cell abundances (1 × 104 cells mL–1) for MG-II Euryarchaea (A, upper panel) and GI.1a Thaumarchaea (B, bottom panel) at the time series station (Sta.18). Black dots indicate the sampling dates.
Supplementary Figure 4 | Ratio of AOA amoA copy-to-GI.1a Thaumarchaea cell ratios variability associated with surface, intermediate and bottom water layers at the different periods studied.
Supplementary Figure 5 | Counts of bAOB amoA compared with AOA amoA gene copies (A) and with AOA amoA copy-to-GI.1a Thaumarchaea cell ratios. Data from surface (S), intermediate (I), and bottom water layers (B) are tagged.
Supplementary Figure 6 | Comparison of average monthly inventories estimated for upwelling periods studied at different depth layers ML (mixed layer), OX (oxycline), BOT (Bottom). (A) Nitrous oxide (Farías et al., 2015; ML, mixed layer; SSL, subsurface layer) versus AOA, (B) Nitrate and bAOB, and (C) ammonium and non-photosynthetic picoplankton abundance.
Footnotes
References
Agogué, H., Brink, M., Dinasquet, J., and Herndl, G. J. (2008). Major gradients in putatively nitrifying and non-nitrifying Archaea in the deep North Atlantic. Nature 456, 788–791. doi: 10.1038/nature07535
Ahumada, R., and Chuecas, L. (1979). Algunas características hidrográficas de la Bahía Concepción (36°40′S-73°02′W) y áreas adyacentes. Chile. Gayana Misc. 8, 1–56.
Aldunate, M., De la Iglesia, R., Bertagnolli, A. D., and Ulloa, O. (2018). Oxygen modulates bacterial community composition in the coastal upwelling waters off central Chile. Deep Sea Res. II 156, 68–79. doi: 10.1016/j.dsr2.2018.02.001
Alonso-Sáez, L., Waller, A. S., Mende, D. R., Bakker, K., Farnelid, H., Yager, P. L., et al. (2012). Role for urea in nitrification by polar marine Archaea. Proc. Natl. Acad. Sci. U.S.A. 109, 17989–17994. doi: 10.1073/pnas.1201914109
Amann, R. I., Binder, B. J., Olson, R. J., Chisholm, S. W., Devereux, R., and Stahl, D. A. (1990). Combination of 16S rRNA-targeted oligonucleotide probes with flow cytometry for analyzing mixed microbial populations. Appl. Environ. Microbiol. 56, 1919–1925. doi: 10.1128/aem.56.6.1919-1925.1990
Bayer, B., Vojvoda, J., Offre, P., Alves, R. J., Elisabeth, N. H., Garcia, J. A., et al. (2016). Physiological and genomic characterization of two novel marine thaumarchaeal strains indicates niche differentiation. ISME J. 10, 1051–1063. doi: 10.1038/ismej.2015.200
Beman, J. M., Popp, B. N., and Alford, S. E. (2012). Quantification of ammonia oxidation rates and ammonia-oxidizing archaea and bacteria at high resolution in the Gulf of California and eastern tropical North Pacific Ocean. Limnol. Oceanogr. 57, 711–726. doi: 10.4319/lo.2012.57.3.0711
Beman, J. M., Popp, B. N., and Francis, C. A. (2008). Molecular and biogeochemical evidence for ammonia oxidation by marine Crenarchaeota in the Gulf of California. ISME J. 2, 429–441. doi: 10.1038/ismej.2007.118
Beman, J. M., Sachdeva, R., and Fuhrman, J. A. (2010). Population ecology of nitrifying Archaea and Bacteria in the Southern California Bight. Environ. Microbiol. 12, 1282–1292. doi: 10.1111/j.1462-2920.2010.02172.x
Beman, J. M., Steele, J. A., and Fuhrman, J. A. (2011). Co-occurrence patterns for abundant marine archaeal and bacterial lineages in the deep chlorophyll maximum of coastal California. ISME J. 5, 1077–1085. doi: 10.1038/ismej.2010.204
Bergen, B., Herlemann, D. P. R., and Jürgens, K. (2015). Zonation of bacterioplankton communities along aging upwelled water in the northern Benguela upwelling. Front. Microbiol. 6:621. doi: 10.3389/fmicb.2015.00621
Bernhard, A. E., and Bollmann, A. (2010). Estuarine nitrifiers: new players, patterns and processes. Estuar Coast Shelf Sci. 88, 1–11. doi: 10.1016/j.ecss.2010.01.023
Bertagnolli, A. D., and Ulloa, O. (2017). Hydrography shapes community composition and diversity of amoA-containing Thaumarchaeota in the coastal waters off central Chile. Environ. Microbiol. Rep. 9, 717–728. doi: 10.1111/1758-2229.12579
Bouskill, N. J., Eveillard, D., Chien, D., Jayakumar, A., and Ward, B. B. (2012). Environmental factors determining ammonia-oxidizing organism distribution and diversity in marine environments. Environ. Microbiol. 14, 714–729. doi: 10.1111/j.1462-2920.2011.02623.x
Bouskill, N. J., Eveillard, D., O’Mullan, G. D., Jackson, G. A., and Ward, B. B. (2011). Seasonal and annual reoccurrence in betaproteobacterial ammonia-oxidizing bacterial population structure. Environ. Microbiol. 13, 872–886. doi: 10.1111/j.1462-2920.2010.02362.x
Bowen, J. L., Kearns, P. J., Holcomb, M., and Ward, B. B. (2013). Acidification alters the composition of ammonia-oxidizing microbial assemblages in marine mesocosms. Mar. Ecol. Prog. Ser. 492, 1–8. doi: 10.3354/meps10526
Bristow, L. A., Dalsgaard, T., Tiano, L., Mills, D. B., Bertagnolli, A. D., Wright, J. J., et al. (2016). Ammonium and nitrite oxidation at nanomolar oxygen concentrations in oxygen minimum zone waters. Proc. Natl. Acad. Sci. U.S.A. 113, 10601–10606. doi: 10.1073/pnas.1600359113
Cavicchioli, R., Ripple, W. J., Timmis, K. N., Azam, F., Bakken, L. R., Baylis, M., et al. (2019). Scientists’ warning to humanity: microorganisms and climate change. Nat. Rev. Microbiol. 17, 569–586. doi: 10.1038/s41579-019-0222-5
Connelly, T. L., Baer, S. E., Cooper, J. T., Bronk, D. A., and Wawrik, B. (2014). Urea uptake and carbon fixation by marine pelagic bacteria and archaea during the Arctic summer and winter seasons. Appl. Environ. Microbiol. 80, 6013–6022. doi: 10.1128/AEM.01431-14
Cram, J. A., Chow, C.-E. T., Sachdeva, R., Needham, D. M., Parada, A. E., Steele, J. A., et al. (2015). Seasonal and interannual variability of the marine bacterioplankton community throughout the water column over ten years. ISME J. 9, 563–580. doi: 10.1038/ismej.2014.153
Cuevas, L. A., Daneri, G., Jacob, B., and Montero, P. (2004). Microbial abundance and activity in the seasonal upwelling area off Concepción (36°S), central Chile: a comparison of upwelling and non-upwelling conditions. Deep Sea Res. II 51, 2427–2440. doi: 10.1016/j.dsr2.2004.07.026
Daims, H., Brühl, A., Amann, R., Schleifer, K.-H., and Wagner, M. (1999). The domain-specific probe EUB338 is insufficient for the detection of all Bacteria: development and evaluation of a more comprehensive probe set. Syst. Appl. Microbiol. 22, 434–444. doi: 10.1016/S0723-2020(99)80053-8
Daims, H., Lebedeva, E. V., Pjevac, P., Han, P., Herbold, C., Albertsen, M., et al. (2015). Complete nitrification by Nitrospira bacteria. Nature 528, 504–509. doi: 10.1038/nature16461
Daims, H., Lücker, S., and Wagner, M. (2016). A new perspective on microbes formerly known as nitrite-oxidizing bacteria. Trends Microbiol. 24, 699–712. doi: 10.1016/j.tim.2016.05.004
Daneri, G., Dellarossa, V., Quiñones, R., Jacob, B., Montero, P., and Ulloa, O. (2000). Primary production and community respiration in the humboldt current system off chile and associated oceanic areas. Mar. Ecol. Prog. Ser. 197, 41–49. doi: 10.3354/meps197041
Daneri, G., Lizárraga, L., Montero, P., González, H. E., and Tapia, F. J. (2012). Wind forcing and short-term variability of phytoplankton and heterotrophic bacterioplankton in the coastal zone of the Concepción upwelling system (Central Chile). Prog. Oceanogr. 92-95, 92–96. doi: 10.1016/j.pocean.2011.07.013
Eissler, Y., Letelier, J., Cuevas, L. A., Morales, C. E., and Escribano, R. (2010). The microbial community in the coastal upwelling system off Concepción, Chile, 36°S, 2002–2003 period. Rev. Biol. Mar. Oceanog. 45, 1–18. doi: 10.17485/ijst/2018/v11i22/102251
Escribano, R., and Morales, C. E. (2012). Spatial and temporal scales of variability in the coastal upwelling and coastal transition zones off central-southern Chile (35-40°S). Prog. Oceanogr. 92-95, 1–7. doi: 10.1016/j.pocean.2011.07.019
Farías, L., Besoain, V., and García-Loyola, S. (2015). Presence of nitrous oxide hotspots in the coastal upwelling area off central Chile: an analysis of temporal variability based on ten years of a biogeochemical time series. Environ. Res. Lett. 10:044017. doi: 10.1088/1748-9326/10/4/044017
Fernandez, C., and Farías, L. (2012). Assimilation and regeneration of inorganic nitrogen in a coastal upwelling system: ammonium and nitrate utilization. Mar. Ecol. Prog. Ser. 451, 1–14. doi: 10.3354/meps09683
Francis, C. A., Roberts, K. J., Beman, J. M., Santoro, A. E., and Oakley, B. B. (2005). Ubiquity and diversity of ammonia-oxidizing archaea in water columns and sediments of the ocean. Proc. Natl. Acad. Sci. U.S.A. 102, 14683–14688. doi: 10.1073/pnas.0506625102
Frey, C., Bange, H. W., Achterberg, E. P., Jayakumar, A., Löscher, C. R., Arévalo-Martínez, D. L., et al. (2020). Regulation of nitrous oxide production in low-oxygen waters off the coast of Peru. Biogeosciences 17, 2263–2287. doi: 10.5194/bg-17-2263-20202020
Galán, A., Faúndez, J., Thamdrup, B., Santibáñez, J. F., and Farías, L. (2014). Temporal dynamics of nitrogen loss in the coastal upwelling ecosystem off central Chile: evidence of autotrophic denitrification through sulfide oxidation. Limnol. Oceanogr. 59, 1865–1878. doi: 10.4319/lo.2014.59.6.1865
Galán, A., Molina, V., Belmar, L., and Ulloa, O. (2012). Temporal variability and phylogenetic characterization of planktonic anammox bacteria in the coastal upwelling ecosystem off central Chile. Prog. Oceanogr. 92, 110–120. doi: 10.1016/j.pocean.2011.07.007
Galán, A., Thamdrup, B., Saldías, G. S., and Farías, L. (2017). Vertical segregation among pathways mediating nitrogen loss (N2 and N2O production) across the oxygen gradient in a coastal upwelling ecosystem. Biogeosciences 14, 4795–4813. doi: 10.5194/bg-14-4795-2017
Galand, P. E., Gutiérrez-Provecho, C., Massana, R., Gasol, J. M., and Casamayor, E. O. (2010). Inter-annual recurrence of archaeal assemblages in the coastal NW Mediterranean Sea (Blanes Bay Microbial Observatory). Limnol. Oceanogr. 55, 2117–2125. doi: 10.4319/lo.2010.55.5.2117
Galand, P. E., Pereira, O., Hochart, C., Auguet, J. C., and Debroas, D. (2018) A strong link between marine microbial community composition and function challenges the idea of functional redundancy. ISME J. 12, 2470–2478. doi: 10.1038/s41396-018-0158-1
Ganesh, S., Parris, D. J., DeLong, E. F., and Stewart, F. J. (2014). Metagenomic analysis of size-fractionated picoplankton in a marine oxygen minimum zone. ISME J. 8, 187–211. doi: 10.1038/ismej.2013.144
Guerrero, M. A., and Jones, R. D. (1996). Photoinhibition of marine nitrifying bacteria. I. Wavelength-dependent response. Mar. Ecol. Prog. Ser. 141, 183–192. doi: 10.3354/meps141183
Herfort, L., Schouten, S., Abbas, B., Veldhuis, M. J. W., Coolen, M. J. L., Wuchter, C., et al. (2007). Variations in spatial and temporal distribution of archaea in the North Sea in relation to environmental variables. FEMS Microbiol. Ecol. 62, 242–257. doi: 10.1111/j.1574-6941.2007.00397.x
Hernández, K., Yannicelli, B., Montecinos, A., Ramos, M., González, H. E., and Daneri, G. (2012). Temporal variability of incidental solar radiation and modulating factors in a coastal upwelling area (36°S). Prog. Oceanogr. 92-95, 18–32. doi: 10.1016/j.pocean.2011.07.011
Holmes, R. M., Aminot, A., Kérouel, R., Hooker, B. A., and Peterson, B. J. (1999). A simple and precise method for measuring ammonium in marine and freshwater ecosystems. Can. J. Fish Aquat. Sci. 56, 1801–1808. doi: 10.1139/cjfas-56-10-1801
Holm-Hansen, O., Lorenzen, C. J., Holmes, R. W., and Strickland, J. D. H. (1965). Fluorometric determination of chlorophyll. J. Cons. Int. Explor. Mer. 30, 3–15. doi: 10.1093/icesjms/30.1.3
Karner, M. B., DeLong, E. F., and Karl, D. M. (2001). Archaeal dominance in the mesopelagic zone of the Pacific Ocean. Nature 409, 507–510. doi: 10.1038/35054051
Labrenz, M., Sintes, E., Toetzke, F., Zumsteg, A., Herndl, G. J., Seidler, M., et al. (2010). Relevance of a crenarchaeotal subcluster related to Candidatus Nitrosopumilus maritimus to ammonia oxidation in the suboxic zone of the central Baltic Sea. ISME J. 4, 1496–1508. doi: 10.1038/ismej.2010.78
Lam, P., Jensen, M. M., Lavik, G., McGinnis, D. F., Müller, B., Schubert, C. J., et al. (2007). Linking crenarchaeal and bacterial nitrification to anammox in the Black Sea. Proc. Natl. Acad. Sci. U.S.A. 104, 7104–7109. doi: 10.1073/pnas.0611081104
Lambert, S., Tragin, M., Lozano, J. C., Ghiglione, J. F., Vaulot, D., Bouget, F. Y., et al. (2019). Rhythmicity of coastal marine picoeukaryotes, bacteria and archaea despite irregular environmental perturbations. ISME J. 13, 388–401. doi: 10.1038/s41396-018-0281-z
Levipan, H. A., Molina, V., Anguita, C., Rain-Franco, A., Belmar, L., and Fernandez, C. (2016). Variability of nitrifying communities in surface coastal waters of the Eastern South Pacific (∼36° S). Environ. Microbiol. Rep. 8, 851–864. doi: 10.1111/1758-2229.12448
Levipan, H. A., Molina, V., and Fernandez, C. (2014). Nitrospina-like bacteria are the main drivers of nitrite oxidation in the seasonal upwelling area of the Eastern South Pacific (central Chile ∼36° S). Environ. Microbiol. Rep. 6, 565–573. doi: 10.1111/1758-2229.12158
Levipan, H. A., Quiñones, R. A., Johansson, H. E., and Urrutia, H. (2007a). Methylotrophic methanogens in the water column of an upwelling zone with a strong oxygen gradient off central Chile. Microbes Environ. 22, 268–278. doi: 10.1264/jsme2.22.268
Levipan, H. A., Quiñones, R. A., and Urrutia, H. (2007b). A time series of prokaryote secondary production in the oxygen minimum zone of the Humboldt Current System, off central Chile. Prog. Oceanogr. 75, 531–549. doi: 10.1016/j.pocean.2007.08.029
Li, J., Nedwell, D. B., Beddow, J., Dumbrell, A. J., McKew, B. A., Thorpe, E. L., et al. (2015). amoA gene abundances and nitrification potential rates suggest that benthic ammonia-oxidizing bacteria and not archaea dominate N cycling in the Colne Estuary, United Kingdom. Appl. Environ. Microbiol. 81, 159–165. doi: 10.1128/AEM.02654-14
Lincoln, S. A., Wai, B., Eppley, J. M., Church, M. J., Summons, R. E., and DeLong, E. F. (2014). Planktonic Euryarchaeota are a significant source of archaeal tetraether lipids in the ocean. Proc. Natl. Acad. Sci. U.S.A. 111, 9858–9863. doi: 10.1073/pnas.1409439111
Löscher, C. R., Kock, A., Könneke, M., LaRoche, J., Bange, H. W., and Schmitz, R. A. (2012). Production of oceanic nitrous oxide by ammonia-oxidizing archaea. Biogeosciences 9, 2419–2429. doi: 10.5194/bgd-9-2095-2012
Louca, S., Hawley, A. K., Katsev, S., Torres-Beltran, M., Bhatia, M. P., Kheirandish, S., et al. (2016). Integrating biogeochemistry with multiomic sequence information in a model oxygen minimum zone. Proc. Natl. Acad. Sci. U.S.A. 113, 5925–5933. doi: 10.1073/pnas.1602897113
Marie, D., Partensky, F., Jacquet, S., and Vaulot, D. (1997). Enumeration and cell cycle analysis of natural populations of marine picoplankton by flow cytometry using the nucleic acid stain SYBR Green I. Appl. Environ. Microbiol. 63, 186–193. doi: 10.1128/aem.63.1.186-193.1997
Martens-Habbena, W., Berube, P. M., Urakawa, H., De La Torre, J. R., and Stahl, D. A. (2009). Ammonia oxidation kinetics determine niche separation of nitrifying Archaea and Bacteria. Nature 461, 976–979. doi: 10.1038/nature08465
Massana, R., Murray, A., Preston, C., and DeLong, E. (1997). Vertical distribution and phylogenetic characterization of marine planktonic archaea in the Santa Barbara Channel. Appl. Environ. Microbiol. 63, 50–56. doi: 10.1128/aem.63.1.50-56.1997
Merbt, S. N., Stahl, D. A., Casamayor, E. O., Martí, E., Nicol, G. W., and Prosser, J. I. (2012). Differential photoinhibition of bacterial and archaeal ammonia oxidation. FEMS Microbiol. Lett. 327, 41–46. doi: 10.1111/j.1574-6968.2011.02457.x
Mincer, T. J., Church, M. J., Taylor, L. T., Preston, C. M., Karl, D. M., and DeLong, E. F. (2007). Quantitative distribution of presumptive archaeal and bacterial nitrifiers in Monterey Bay and the North Pacific Subtropical Gyre. Environ. Microbiol. 9, 1162–1175. doi: 10.1111/j.1462-2920.2007.01239.x
Molina, V., Belmar, L., and Ulloa, O. (2010). High diversity of ammonia-oxidizing archaea in permanent and seasonal oxygen-deficient waters of the eastern South Pacific. Environ. Microbiol. 12, 2450–2465. doi: 10.1111/j.1462-2920.2010
Molina, V., Ulloa, O., Farías, L., Urrutia, H., Ramírez, S., Junier, P., et al. (2007). Ammonia-oxidizing β-Proteobacteria from the oxygen minimum zone off northern Chile. Appl. Environ. Microbiol. 73, 3547–3555. doi: 10.1128/AEM.02275-06
Montero, P., Daneri, G., Cuevas, L. A., González, H. E., Jacob, B., Lizárraga, L., et al. (2007). Productivity cycles in the coastal upwelling area off Concepción: The importance of diatoms and bacterioplankton in the organic carbon flux. Prog. Oceanogr. 75, 518–530. doi: 10.3856/vol45-issue5-fulltext-16
Murillo, A. A., Ramírez-Flandes, S., DeLong, E. F., and Ulloa, O. (2014). Enhanced metabolic versatility of planktonic sulfur-oxidizing γ-proteobacteria in an oxygen-deficient coastal ecosystem. Front. Mar. Sci. 1:18. doi: 10.3389/fmars.2014.00018
Murray, A. E., Wu, K. Y., Moyer, C. L., Karl, D. M., and DeLong, E. F. (1999). Evidence for circumpolar distribution of planktonic archaea in the Southern Ocean. Aquat. Microb. Ecol. 18, 263–273. doi: 10.3354/ame018263
Newell, S. E., Babbin, A. R., Jayakumar, A., and Ward, B. B. (2011). Ammonia oxidation rates and nitrification in the Arabian Sea. Glob. Biogeochem. Cy. 25:GB4016. doi: 10.1029/2010GB003940
Newell, S. E., Facwett, S. E., and Ward, B. B. (2013). Depth distribution of ammonia oxidation rates and ammonia-oxidizer community composition in the Sargasso Sea. Limnol. Oceanogr. 58, 1491–1500. doi: 10.4319/lo.2013.58.4.1491
Palatinszky, M., Herbold, C., Jehmlich, N., Pogoda, M., Han, P., von Bergen, M., et al. (2015). Cyanate as an energy source for nitrifiers. Nature 524, 105–108. doi: 10.1038/nature14856
Parada, A. E., and Fuhrman, J. A. (2017). Marine archaeal dynamics and interactions with the microbial community over 5 years from surface to seafloor. ISME J. 11, 2510–2525. doi: 10.1038/ismej.2017.104
Parsons, R. J., Nelson, C. E., Carlson, C. A., Denman, C. C., Andersson, A. J., Kledzik, A. L., et al. (2015). Marine bacterioplankton community turnover within seasonally hypoxic waters of a subtropical sound: Devil’s Hole, Bermuda. Environ. Microbiol. 7, 3481–3499. doi: 10.1111/1462-2920.12445
Parsons, T. R., Maita, Y., and Lalli, C. M. (1984). A Manual of Chemical and Biological Methods for Seawater Analysis, 1 Edn. Oxford: Pergamon Press.
Peng, X., Jayakumar, A., and Ward, B. B. (2013). Community composition of ammonia-oxidizing archaea from surface and anoxic depths of oceanic oxygen minimum zones. Front. Microbiol. 4:177. doi: 10.3389/fmicb.2013.00177
Pester, M., Rattei, T., Flechl, S., Gröngröft, A., Richter, A., Overmann, J., et al. (2012). amoA-based consensus phylogeny of ammonia-oxidizing archaea and deep sequencing of amoA genes from soils of four different geographic regions. Environ. Microbiol. 14, 525–539. doi: 10.1111/j.1462-2920.2011.02666.x
Pester, M., Schleper, C., and Wagner, M. (2011). The Thaumarchaeota: an emerging view of their phylogeny and ecophysiology. Curr. Opin. Microbiol. 14, 300–306. doi: 10.1016/j.mib.2011.04.007
Pitcher, A., Villanueva, L., Hopmans, E. C., Schouten, S., Reichart, G.-J., and Sinninghe Damsté, J. S. (2011a). Niche segregation of ammonia-oxidizing archaea and anammox bacteria in the Arabian Sea oxygen minimum zone. ISME J. 5, 1896–1904. doi: 10.1038/ismej.2011.60
Pitcher, A., Wuchter, C., Siedenberg, K., Schouten, S., and Sinninghe Damsté, J. S. (2011b). Crenarchaeol tracks winter blooms of ammonia-oxidizing Thaumarchaeota in the coastal North Sea. Limnol. Oceanogr. 56, 2308–2318. doi: 10.4319/lo.2011.56.6.2308
Pommerening-Röser, A., and Koops, H. P. (2005). Environmental pH as an important factor for the distribution of urease positive ammonia-oxidizing bacteria. Microbiol. Res. 160, 27–35. doi: 10.1016/j.micres.2004.09.006
Porter, K. G., and Feig, Y. S. (1980). The use of DAPI for identifying and counting aquatic microflora. Limnol. Oceanogr. 25, 943–948. doi: 10.4319/lo.1980.25.5.0943
Qin, W., Amin, S. A., Martens-Habbena, W., Walker, C. B., Urakawa, H., Devol, A. H., et al. (2014). Marine ammonia-oxidizing archaeal isolates display obligate mixotrophy and wide ecotypic variation. Proc. Natl. Acad. Sci. U.S.A. 111, 12504–12509. doi: 10.1073/pnas.1324115111
Qin, W., Meinhardt, K. A., Moffett, J. W., Devol, A. H., Armbrust, E. V., Ingalls, A. E., et al. (2017). Influence of oxygen availability on the activities of ammonia-oxidizing archaea. Environ. Microbiol. Rep. 9, 250–256. doi: 10.1111/1758-2229.12525
Quiñones, R. A., Levipan, H. A., and Urrutia, H. (2009). Spatial and temporal variability of planktonic archaeal abundance in the Humboldt Current System off Chile. Deep Sea Res. II 56, 1073–1082. doi: 10.1016/j.dsr2.2008.09.012
Reji, L., Tolar, B. B., Chavez, F. P., and Francis, C. A. (2020) Depth-differentiation and seasonality of planktonic microbial assemblages in the Monterey Bay upwelling system. Front. Microbiol. 11:1075. doi: 10.3389/fmicb.2020.01075
Reji, L., Tolar, B. B., Smith, J. M., Chavez, F. P., and Francis, C. A. (2019). Differential co-occurrence relationships shaping ecotype diversification within Thaumarchaeota populations in the coastal ocean water column. ISME J. 13, 1144–1158. doi: 10.1038/s41396-018-0311-x
Rigby, R. A., and Stasinopoulos, D. M. (2005). Generalized additive models for location, scale and shape (with discussion). Appl. Statist. 54, 507–554.
Robidart, J. C., Preston, C. M., Paerl, R. W., Turk, K. A., Mosier, A. C., Francis, C. A., et al. (2012). Seasonal Synechococcus and Thaumarchaeal population dynamics examined with high resolution with remote in situ instrumentation. ISME J. 6, 513–523. doi: 10.1038/ismej.2011.127
Rossi, S., Isla, E., Fietz, S., Martínez-Garcia, A., Sañé, E., and Teixidò, N. (2012). Temporal variation of seston biomarkers within the Humboldt Current System off northern Chile (21°S): first simultaneous records on fatty acids, n-alkanes and glyceroldialkyl-glycerol-tetraethers (GDGT). Adv. Oceanogr. Limnol. 3, 17–40. doi: 10.1080/19475721.2012.676068
Rotthauwe, J.-H., Witzel, K.-P., and Liesack, W. (1997). The ammonia monooxygenase structural gene amoA as a functional marker: molecular fine-scale analysis of natural ammonia-oxidizing populations. Appl. Environ. Microbiol. 63, 4704–4712. doi: 10.1128/aem.63.12.4704-4712.1997
Saldías, G. S., Sobarzo, M., Largier, J., Moffat, C., and Letelier, R. (2012). Seasonal variability of turbid river plumes off central Chile based on high-resolution MODIS imagery. Remote Sens. Environ. 123, 220–233. doi: 10.1016/j.rse.2012.03.0102012
Santoro, A. E., Casciotti, K. L., and Francis, C. A. (2010). Activity, abundance and diversity of nitrifying archaea and bacteria in the central California Current. Environ. Microbiol. 12, 1989–2006. doi: 10.1111/j.1462-2920.2010.02205.x
Santoro, A. E., Dupont, C. L., Richter, R. A., Craig, M. T., Carini, P., McIlvin, M. R., et al. (2015). Genomic and proteomic characterization of “Candidatus Nitrosopelagicus brevis”: an ammonia-oxidizing archaeon from the open ocean. Proc. Natl. Acad. Sci. U.S.A. 112, 1173–1178. doi: 10.1073/pnas.1416223112
Santoro, A. E., Sakamoto, C. M., Smith, J. M., Plant, J. N., Gehman, A. L., Worden, A. Z., et al. (2013). Measurements of nitrite production in and around the primary nitrite maximum in the central California Current. Biogeosciences 10, 7395–7410. doi: 10.5194/bg-10-7395-2013
Schielzeth, H. (2010). Simple means to improve the interpretability of regression coefficients. Meth. Ecol. Evol. 1, 103–113. doi: 10.1111/j.2041-210X.2010.00012.x
Schleper, C. (2010). Ammonia oxidation: different niches for bacteria and archaea? ISME J. 4, 1092–1094. doi: 10.1038/ismej.2010.111
Sekar, R., Fuchs, B. M., Amann, R., and Pernthaler, J. (2004). Flow sorting of marine bacterioplankton after fluorescence in situ hybridization. Appl. Environ. Microbiol. 70, 6210–6219. doi: 10.1128/AEM.70.10.6210-6219.2004
Shiozaki, T., Ijichi, M., Isobe, K., Hashihama, F., Nakamura, K. I., Ehama, M., et al. (2016). Nitrification and its influence on biogeochemical cycles from the equatorial Pacific to the Arctic Ocean. ISME J. 10, 2184–2197. doi: 10.1038/ismej.2016.18
Sintes, E., Bergauer, K., De Corte, D., Yokokawa, T., and Herndl, G. J. (2013). Archaeal amoA gene diversity points to distinct biogeography of ammonia-oxidizing Crenarchaeota in the ocean. Environ. Microbiol. 15, 1647–1658. doi: 10.1111/j.1462-2920.2012.02801.x
Sintes, E., DeCorte, D., Haberleitner, E., and Herndl, G. J. (2016). Geographic distribution of archaeal ammonia oxidizing ecotypes in the Atlantic Ocean. Front. Microbiol. 7:77. doi: 10.3389/fmicb.2016.00077
Smith, J. M., Casciotti, K. L., Chavez, F. P., and Francis, C. A. (2014). Differential contributions of archaeal ammonia oxidizer ecotypes to nitrification in coastal surface waters. ISME J. 8, 1704–1714. doi: 10.1038/ismej.2014.11
Smith, M. W., Zeigler Allen, L., Allen, A. E., Herfort, L., and Simon, H. M. (2013). Contrasting genomic properties of free-living and particle-attached microbial assemblages within a coastal ecosystem. Front. Microbiol. 4:120. doi: 10.3389/fmicb.2013.00120
Sobarzo, M., Bravo, L., Donoso, D., Garcés-Vargas, J., and Schneider, W. (2007). Coastal upwelling and seasonal cycles that influence the water column over the continental shelf off central Chile. Prog. Oceanogr. 75, 363–382. doi: 10.1016/j.pocean.2007.08.022
Srain, B. M., Sepúlveda, J., Pantoja, S., Summons, R. E., Quiñones, R. A., and Levipan, H. A. (2015). Archaeal and bacterial assemblages in the oxygen minimum zone of the upwelling ecosystem off central Chile as determined by organic biomarkers. Gayana 79, 26–44. doi: 10.4067/S0717-65382015000100005
Srain, B. M., Sobarzo, M., Daneri, G., González, H. E., Testa, G., Farías, L., et al. (2020). Fermentation and anaerobic oxidation of organic carbon in the oxygen minimum zone off the upwelling ecosystem off Concepción, in Central Chile. Front. Mar. Sci. 7:533. doi: 10.3389/fmars.2020.00533
Steele, J. A., Countway, P. D., Xia, L., Vigil, P. D., Beman, J. M., Kim, D. Y., et al. (2011). Marine bacterial, archaeal and protistan association networks reveal ecological linkages. ISME J. 5, 1414–1125. doi: 10.1038/ismej.2011.24
Stieglmeier, M., Mooshammer, M., Kitzler, B., Wanek, W., Zechmeister-Boltenstern, S., Richter, A., et al. (2014). Aerobic nitrous oxide production through N-nitrosating hybrid formation in ammonia-oxidizing archaea. ISME J. 8, 1135–1146. doi: 10.1038/ismej.2013.220
Teira, E., Reinthaler, T., Pernthaler, A., Pernthaler, J., and Herndl, G. J. (2004). Combining catalyzed reporter deposition-fluorescence in situ hybridization and microautoradiography to detect substrate utilization by Bacteria and Archaea in the deep ocean. Appl. Environ. Microbiol. 70, 4411–4414. doi: 10.1128/AEM.70.7.4411-4414.2004
Tolar, B. B., King, G. M., and Hollibaugh, J. T. (2013). An analysis of Thaumarchaeota populations from the Northern Gulf of Mexico. Front. Microbiol. 4:72. doi: 10.3389/fmicb.2013.00072
Tolar, B. B., Reji, L., Smith, J. M., Blum, M., Pennington, J. T., Chavez, F. P., et al. (2020). Time series assessment on Thaumarchaeota ecotypes in Monterey Bay reveals the importance of water column position in predicting distribution-environment relationships. Limnol. Oceanogr. 20, 1–15. doi: 10.1002/lno.11436
van Kessel, M. A. H. J., Speth, D. R., Albertsen, M., Nielsen, P. H., Op den Camp, H. J. M., Kartal, B., et al. (2015). Complete nitrification by a single microorganism. Nature 528, 555–559. doi: 10.1038/nature16459
Wallner, G., Amann, R., and Beisker, W. (1993). Optimizing fluorescent in situ hybridization with rRNA-targeted oligonucleotide probes for flow cytometric identification of microorganisms. Cytometry 14, 136–143. doi: 10.1002/cyto.990140205
Ward, B. B. (2008). “Nitrification in marine systems,” in Nitrogen in the Marine Environment, eds D. G. Capone, D. A. Bronk, M. R. Mulholland, and E. J. Carpenter (Amsterdam: Elsevier), 199–248. doi: 10.1016/b978-0-12-372522-6.00005-0
Wiebinga, C. J., Veldhuis, M. J. W., and De Baar, H. J. W. (1997). Abundance and productivity of bacterioplankton in relation to seasonal upwelling in the northwest Indian Ocean. Deep Sea Res. I 44, 451–476. doi: 10.1016/S0967-0637(96)00115-X
Woebken, D., Fuchs, B. M., Kuypers, M. M. M., and Amann, R. (2007). Potential interactions of particle-associated anammox bacteria with bacterial and archaeal partners in the namibian upwelling system. Appl. Environm. Microbiol. 73, 4648–4657. doi: 10.1128/AEM.02774-06
Keywords: Thaumarchaea, chemolithotrophic nitrification, environmental forcing, coastal upwelling time-series station, oxygen minimum zone, ecological niche, quantitative PCR, CARD-FISH
Citation: Molina V, Belmar L, Levipan HA, Ramírez-Flandes S, Anguita C, Galán A, Montes I and Ulloa O (2020) Spatiotemporal Distribution of Key Pelagic Microbes in a Seasonal Oxygen-Deficient Coastal Upwelling System of the Eastern South Pacific Ocean. Front. Mar. Sci. 7:561597. doi: 10.3389/fmars.2020.561597
Received: 13 May 2020; Accepted: 31 August 2020;
Published: 25 September 2020.
Edited by:
Anne Bernhard, Connecticut College, United StatesReviewed by:
J. Michael Beman, University of California, Merced, United StatesBradley B. Tolar, Stanford University, United States
Copyright © 2020 Molina, Belmar, Levipan, Ramírez-Flandes, Anguita, Galán, Montes and Ulloa. This is an open-access article distributed under the terms of the Creative Commons Attribution License (CC BY). The use, distribution or reproduction in other forums is permitted, provided the original author(s) and the copyright owner(s) are credited and that the original publication in this journal is cited, in accordance with accepted academic practice. No use, distribution or reproduction is permitted which does not comply with these terms.
*Correspondence: Verónica Molina, dmVyb25pY2EubW9saW5hQHVwbGEuY2w=