- Department of Molecular Sciences, Macquarie University, Sydney, NSW, Australia
Marine plastic pollution is a well-recognized, global problem. Research addressing plastic pollution has largely focused on investigating impacts on macroorganisms, with few studies investigating effects on marine microbes. We previously showed that marine Prochlorococcus, which are important contributors to oceanic primary production, suffer declines in growth and photosynthetic activity following exposure to leachates from new plastic bags (HDPE) and plastic matting (PVC). However, as such plastics reside in the environment they will be subject to weathering processes, so it is also important to consider how these may alter the composition and amounts of substances available to leach. Here we report on how plastic leachate toxicity is affected by environmental weathering (17- and 112-days in estuarine water) of these common plastic materials. We found that while toxicity was reduced by weathering, materials weathered for up to 112-days continued to leach substances that negatively affected Prochlorococcus growth, photophysiology and membrane integrity. Weathered plastics were found to continue to leach zinc, even after up to 112-days in the environment. The two Prochlorococcus strains tested, NATL2A and MIT9312, showed differences in the sensitivity and timing of their responses, indicating that exposure to leachate from weathered plastics may affect even closely related strains to different degrees. As many marine regions inhabited by Prochlorococcus are likely to be subject to continued accumulation of plastic pollution, our findings highlight the potential for ongoing impacts on these important primary producers.
Introduction
Plastics now play an important role in human society (Andrady and Neal, 2009) due to their relatively low cost and strong, durable, lightweight nature (Thompson et al., 2009b). Consequently, annual plastic consumption is increasing rapidly, with ∼350 million tones plastic produced globally in 2017, with 35% more plastic produced from 2007 to 2017 than ever before (Plastics Europe, 2018). Physical hazards to biota associated with marine plastic ingestion and entanglement have been reported across marine and estuarine environments worldwide (Derraik, 2002; Gregory, 2009; Wright et al., 2013; Gall and Thompson, 2015). Marine plastic debris has also been shown to leach a “cocktail of chemicals” (Rochman, 2015). However, the impact of leached chemicals and trace elements from plastics on biota has been less rigorously studied compared to physical hazards (Hermabessiere et al., 2017). Plastic leachates can include substances added during manufacturing (Browne et al., 2007; Thompson et al., 2009a) and other compounds accumulated from the surrounding marine environments (Mato et al., 2001; Teuten et al., 2009). Additives used in plastic manufacture include polymerization solvents, plasticizers, metals, dyes, flame-retardants, UV stabilizers and antioxidants (Hahladakis et al., 2018). Some of these additives are thought to have the potential to enter the marine food web and adversely affect marine ecosystems (Avio et al., 2017).
Potential impacts of plastic leachate and additive compounds, singularly and as mixtures, have now been investigated for a variety of multicellular eukaryotic organisms. The chemical mixture leached from a range of different plastic items has been shown to have adverse effects on copepods Daphnia magna (Lithner et al., 2012b) and Nitocra spinipes (Bejgarn et al., 2015), barnacle Amphibalanus amphitrite larvae (Li et al., 2016), mussel Perna perna embryos (Gandara et al., 2016), sea urchin Paracentrotus lividus embryos (Oliviero et al., 2019), and microalgae Chlorella vulgaris (Luo et al., 2019). While less is known regarding leachate toxicity in prokaryotes, recent work has shown that a key photosynthetic marine bacteria, Prochlorococcus, is also adversely affected by exposure to leachates from new PVC and HDPE plastic items (Tetu et al., 2019). These studies together show many types of plastics have the potential to act as chemical toxicants but this area of research has mainly relied on leachate from new plastics and further work is required to ascertain how environmental weathering affects toxicity.
Plastics may take hundreds to thousands of years to degrade in the environment (Barnes et al., 2009), and degradation is thought to encourage leaching of toxic substances (Lithner et al., 2009) and transport of organic contaminants (León et al., 2018). Plastic debris released into the environment also has the potential to accumulate additional toxicants, with metals such as cadmium being found to adsorb to plastics in marine waters (Boucher et al., 2016). Organic pollutants, such as DDT, PCBs, and PAHs, have been observed on plastics collected from marine environments as well, implying adsorption of these compounds onto plastics (Rios et al., 2007). However, the conditions under which plastic additives or adsorbed toxicants may leach from plastics, and how this varies for different polymer types and weathering environments has only recently begun to be investigated. Laboratory experiments using artificial sunlight (UV irradiation) to look at how simulated weathering affects leachate toxicity found that effects varied for different plastic types, with some plastic products becoming more toxic, others less toxic, and some not changing significantly in their toxicity following UV irradiation (Bejgarn et al., 2015). A recent report from another laboratory experiment also showed temperature and mechanical stress can affect the toxicological impact of tire leachates to minnow embryos, with affects varying between different tire brands (Kolomijeca et al., 2020). Plastic aging experiments performed in marine waters showed that weathering processes involves both adsorption and desorption of different organic and inorganic substances and also found that different changes occur with different plastic items and polymer types (Kedzierski et al., 2018). These findings indicate that multiple issues would benefit from further exploration, including how specific physical conditions during weathering affect leachate toxicity through to how different plastic types are affected by weathering in terms of leachate toxicity, the latter being a focus of this work.
The marine photosynthetic microorganism, Prochlorococcus, is highly abundant in the world’s oceans (Partensky et al., 1999a) and significantly contributes to global primary production, estimated to be 4 Gt C y–1 in some oligotrophic ocean regions (Flombaum et al., 2013). Prochlorococcus populations, composed of multiple, coexisting phylotypes, many of which are known to have distinct ecophysiological underpinnings, can be characterized as falling into two broad groupings distinguished by their photophysiology: high-light (HL) and low-light (LL) adapted ecotypes (Biller et al., 2015). Their ecological importance and population diversity make them an important organism to investigate regarding the impact of environmental stressors. Past work has shown Prochlorococcus to be negatively impacted by different organic pollutants (Fernández-Pinos et al., 2017), UV radiation (Llabrés and Agustí, 2006), exposure to high concentrations of copper (Mann et al., 2002), and exposure to leachate from unweathered HDPE and PVC plastic items (Tetu et al., 2019). Here we have investigated the response of two representative isolates of Prochlorococcus, LLI strain NATL2A and HLII strain MIT9312, to leachates from HDPE and PVC plastic that was weathered for 17- and 112-days in estuarine waters, as a first step in determining how environmental weathering affects leachate toxicity.
Materials and Methods
Cell Culturing and Growth Monitoring
The two Prochlorococcus strains used in this experiment represent different ecotypes; NATL2A is a LL clade I (LLI) strain generally found in deeper euphotic zone layers of the ocean water column, while MIT9312 is a ubiquitous strain tolerant to higher light levels (Biller et al., 2015) falling within the HL clade I. Prochlorococcus NATL2A and MIT9312 cell cultures were grown in AMP1 media (Moore et al., 2007) with LED cool white irradiance of 20 and 40 μmol photons m–2 s–1, respectively. All cultures were maintained at 22°C and 100 rpm shaking and experimental glassware was acid-washed as previously described (Tetu et al., 2019). Prior to each experiment all cultures were acclimated under experimental light and temperature conditions for at least three transfers to ensure balanced growth.
Plastic Weathering and Leachate Preparation
The plastic materials used in this study were gray plastic grocery bags (HDPE) and textured black matting (PVC), as used previously in Tetu et al. (2019). Both plastics were weathered during the Austral autumn-winter in tidal estuarine water of the Lane Cove river (GPS coordinates: 33° 49′37.2″S, 151° 09′14.4″E). In preparation for weathering, plastics were cut into ∼30 × 40 cm pieces (∼40–50 individual pieces for HDPE bags and ∼8–10 pieces for the PVC matting), then all pieces of each type of plastic were tied together at one corner using a nylon line. Material was placed into the environment on 8 April 2018 during low tide, with both plastic types secured to a single weight with sufficient line for both buoyant plastics to float ∼20 cm above their anchor point but at a depth sufficient for all plastic material to remain submerged at low tide. On 25 April roughly half of each type of plastic was cut from the anchor line and taken back to the laboratory (referred to as 17-days weathered plastic), while the remaining plastic was left, until 29 July, 2018 when all remaining material was collected (referred to as 112-days weathered plastic).
During the 17-days weathering period temperature and salinity (measured as conductivity) of the estuarine water were 23.4–25.1°C and 50,000–50,400 μS cm–1, respectively (Beachwatch report for Tambourine Bay, Office of Environment and Heritage, NSW)1, and the UV index ranged from 4.1 to 6.72. The 112-days weathered plastics experienced these same conditions for first 17 days, then experienced an additional weathering period that had a lower and wider range of temperature and UV index (13.6–21°C and 0.9–5.1, respectively) and a more variable range of salinity, 49,600–53,300 μS cm–1. No major storm or wind events were reported by the Australian Bureau of Meteorology during either period (BOM.gov.au).
At each collection time, material was transported immediately to the laboratory where each piece was washed thoroughly with sterile MQ water to remove any mud or debris until there was no visible fouling. The cleaned, weathered material was then used to generate leachates following the methodology applied in Tetu et al. (2019). Briefly, multiple large pieces of weathered, washed plastic were cut with ethanol-sterilized scissors into ∼1 cm2 pieces, weighed, placed into sterile artificial seawater base for AMP1 (5 g per100 mL), then left for 5 days at 22°C and 100 rpm to allow leaching to occur. Following this leaching period, the contents of each flask were filtered through a 0.2 micron filter in order to obtain a sterile, particle-free leachate stock. Leachates were also prepared from unweathered HDPE and PVC materials using the same methodology. To each volume of filtered leachate stock, the standard AMP1 macronutrients, trace metals and HEPES buffer were aseptically added prior to use in culturing. Leachate stocks were diluted with vol/vol dilutions in AMP1 to make the experimental media. For weathered HDPE, leachate dilutions of 6.25 to 50% were tested, representing 3.125–25 mg mL–1 (or equivalent to ∼0.18–1.45 pieces per mL of media). For weathered PVC leachate dilutions of 1 to 50% in AMP1 were used, representing 0.5–25 mg mL–1 (or ∼0.002–0.1 pieces per mL of media).
Experimental Setup
Four independent cultures of each strain were set up from acclimated culture stocks and allowed to grow to mid-exponential phase before use as inocula for the replicates in each plastic experiment. Each experiment comprised of replicate control cultures in AMP1 (no leachate) and experimental treatments containing leachate from weathered and unweathered plastics at a range of dilutions. For weathered HDPE dilutions of 6.25, 12.5, 25, and 50% were tested, while unweathered HDPE dilutions of 12.5 and 25% were tested. For weathered PVC, dilutions of 1, 2, 10, and 50% were tested as well as 1 and 2% unweathered PVC. At initiation of the experiment (0 hr) the four independent parent cultures were measured to determine the number of chlorophyll fluorescent cells per mL, cell integrity, and photophysiology parameters (see below) then used to inoculate each of the control and leachate dilution treatments. All control and treatment cultures were then subsampled at 3, 24, and 48 h for all parameters as described below.
Flow Cytometry
The concentration and relative chlorophyll fluorescence of Prochlorococcus experimental cultures was quantified using a CytoFLEX S flow cytometer and data analyzed with CytExpert software (Beckman Coulter) as previously described (Tetu et al., 2019). Prochlorococcus cells were identified by chlorophyll fluorescence using blue laser (488 nm) excitation and violet side angle light scattering properties using violet laser (405 nm) excitation. Samples were gated so that only cells with chlorophyll fluorescence intensities indicative of healthy cells were counted. At each time point, subsamples were collected from each flask and analyzed immediately with the Cytoflex flow cytometer for all AMP1 control and weathered leachate treatments, while samples from unweathered leachate treatments were collected, fixed with paraformaldehyde (final concentration of 1%), stored at −80°C and run as a batch after conclusion of the experiment.
SYTOX Assay of Membrane Integrity
The membrane integrity of Prochlorococcus cells was measured on fresh samples within 1 h of collection using “live/dead” SYTOX Green stain (Invitrogen # S7020) and flow cytometry, based on the method of Mikula et al. (2012) with slight modifications optimized for our cells. Briefly, SYTOX stain was applied at a final concentration of 0.2 μM and cells were incubated for 15 min in the dark before measurement on a CytoFLEX S flow cytometer. Healthy Prochlorococcus cultures grown in AMP1 media were treated with 1% paraformaldehyde and stained with SYTOX as above as a dead control, used to determine the green fluorescence level (emission at 525/40 nm) of SYTOX positive stained cells. SYTOX-positive cells, i.e., those with damaged or compromised membranes, were identified by high green fluorescence and the proportion of SYTOX stained cells within the total chlorophyll fluorescence population was calculated for each treatment.
Functional Chlorophyll a Fluorescence Measurement
The effective quantum yield of photosystem II was measured using a Phyto Pulse Amplitude Modified (PAM) Fluorometer (Walz Heinz GmbH, Effeltrich, Germany) and the saturation pulse technique as described previously (Tetu et al., 2019). Briefly, light adapted fluorescence yield (F) was determined under growth light conditions for each strain and a saturation pulse was applied to determine maximum light adapted fluorescence yield (Fm′) to calculate the effective quantum yield of photosystem II, ΦPSII (ΦPSII = [Fm′−F]/Fm′). Three technical replicates were run for each individual biological replicate, and an average of these for each biological replicate was used to calculate the average and standard deviation presented for each time point.
Elemental Analysis of Leachates
Experimental leachate and AMP1 media were subsampled into acid washed glass vials at the start of each experiment, acidified with 3.5% nitric acid and sent for Inductively Coupled Plasma-Mass Spectrometry (ICP-MS) and Inductively Coupled Plasma-Optical Emission Spectrometry ICP-OES analysis at the Mark Wainwright Analytical Centre, University of New South Wales, Sydney, NSW, Australia. ICP-MS and OES analyses were conducted on NexION 300D and Optima 7300DV instruments (Perkin Elmer, United States) with three technical replicates run for each sample. For ICP-MS internal standards, Rh, Ir, and Te (Choice Analytical, Australia) were used and analyses performed with a plasma gas flow rate of 15–17 L min–1, auxiliary gas flow rate of 1–2 L min–1, nebulizer gas flow rate of 0.85–1.2 L min–1, and radio frequency power of 1500 W. The data were analyzed using Syngistix for ICP-MS software (Perkin Elmer, United States). For ICP-OES analyses, the internal standard Y, 371.029, was used and analyses performed with the plasma gas flow of 10–15 L min–1, auxiliary gas flow rate of 0.30 L min–1, nebulizer gas flow of 0.70 L min–1, forward power of 1200–1400 W and reflected power of 20 W. The data were analyzed using Winlab32 software.
Statistical Analysis
The growth, photophysiology and SYTOX data were analyzed using one-way ANOVA followed by Tukey’s multiple comparison test with 95% confidence interval. Inter-strain comparisons of SYTOX staining were performed using two-way ANOVA with a Sidak’s multiple comparisons test for the length of weathering and for the % leachate addition for each plastic. For all the data treatments were compared to the control at the matching timepoint and considered significant where p < 0.01. The change in population over time (day–1) was calculated as growth rates are calculated, by fitting linear regression models to the flow cytometric population density measurements from 3 to 48 h. All statistical tests were performed using GraphPad Prism 8 for Windows (GraphPad Software, La Jolla, CA, United States).
Results
Changes in Population Growth
To investigate how environmental weathering of plastics affected leachate toxicity, PVC and HDPE plastics were weathered in estuarine waters for 17- and 112-days, then placed in artificial seawater to generate sterile-filtered, particle-free leachate stocks which were added to Prochlorococcus MIT9312 and NATL2A cultures at a range of dilutions. Unweathered HDPE and PVC materials were tested in parallel, allowing direct comparison of toxic effects from leachates derived from weathered and unweathered products. Weathered HDPE and PVC leachates reduced growth of Prochlorococcus at some concentrations but resulted in less severe declines in growth rates than for the equivalent unweathered leachate dilutions tested here (Figures 1A–D). For both plastic types, exposure to the 112-days weathered leachates resulted in more modest reductions in growth rate than for the 17-days weathered leachates when comparing equivalent dilutions, indicating that duration of weathering affected leachate toxicity (Figure 1).
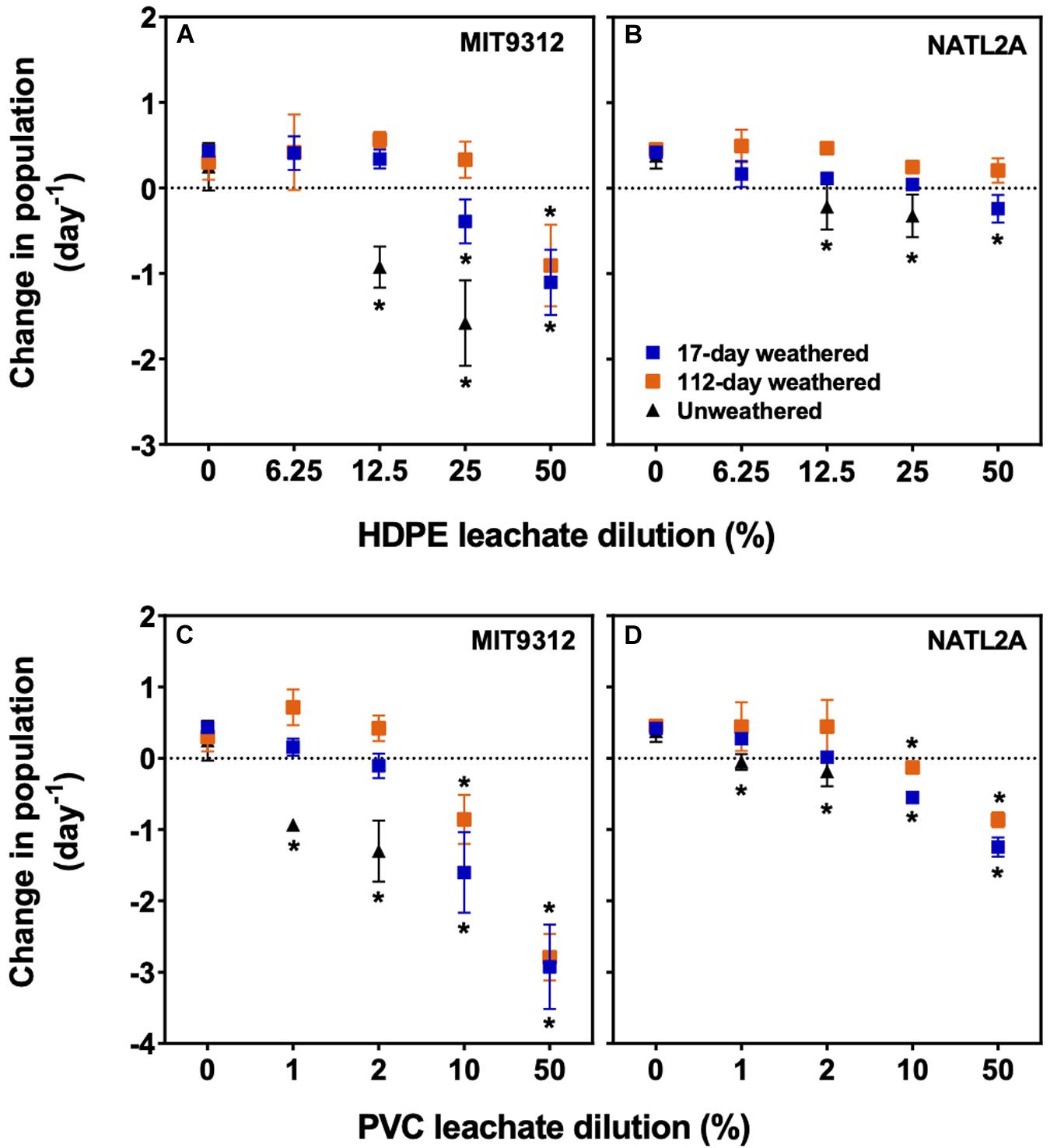
Figure 1. Rate of population change (A–D) of Prochlorococcus MIT9312 and NATL2A in AMP1 media in the presence of different dilutions of leachates from HDPE and PVC materials subjected to two weathering treatments (17- and 112-days in estuarine sea water). Experiments were conducted over 48 h using biological replicates (n = 4; except unweathered treatment, n = 3) for each condition shown as mean values of all replicate cultures with error bars representing the standard deviation. Asterisks (*) indicate that the rate was significantly (p < 0.01) different for a specific leachate concentration relative to the no leachate control (0% leachate dilution). Symbols shown in panel (B) indicate the weathering treatment for all panels.
The two Prochlorococcus strains showed differences in their growth rate responses to each of the weathered leachates, as seen for unweathered leachates. In MIT9312 exposure to weathered HDPE resulted in a significantly reduced (p < 0.01) growth rate compared to zero leachate controls for the two highest concentrations of 17-days weathered HDPE and the highest concentration of 112-days weathered HDPE. For NATL2A, growth rates were reduced to a lesser degree with only 50% weathered HDPE leachate from the 17-days treatment resulting in negative growth rates and showing a significant (p < 0.01) reduction relative to the control (Figures 1A,B).
For weathered PVC, MIT9312 also showed a strong response, with 10 and 50% treatments of 17-days weathered PVC resulting in statistically significant (p < 0.01) growth rate reductions relative to the control (Figure 1C). For this strain 112-days weathered PVC exposure also strongly reduced growth rates at the two highest concentrations (10 and 50%) (Figure 1C). NATL2A showed reductions in growth rate following exposure to the higher weathered PVC concentrations from both 17- and 112-days weathering (Figure 1D), but these were less severe than seen for MIT9312, reflecting what was observed for the unweathered PVC leachate.
Photophysiology
To determine if weathering altered the capacity for plastic leachates to affect photochemical efficiency of photosystem II (PSII) in Prochlorococcus, PSII quantum yield (ΦPSII) was also measured following MIT9312 and NATL2A exposure to the weathered and unweathered PVC and HDPE leachates. Weathered leachate exposure affected ΦPSII in a dose dependent manner but was less pronounced than for equivalent treatments with unweathered plastic leachates (HDPE shown in Figure 2, PVC in Figure 3).
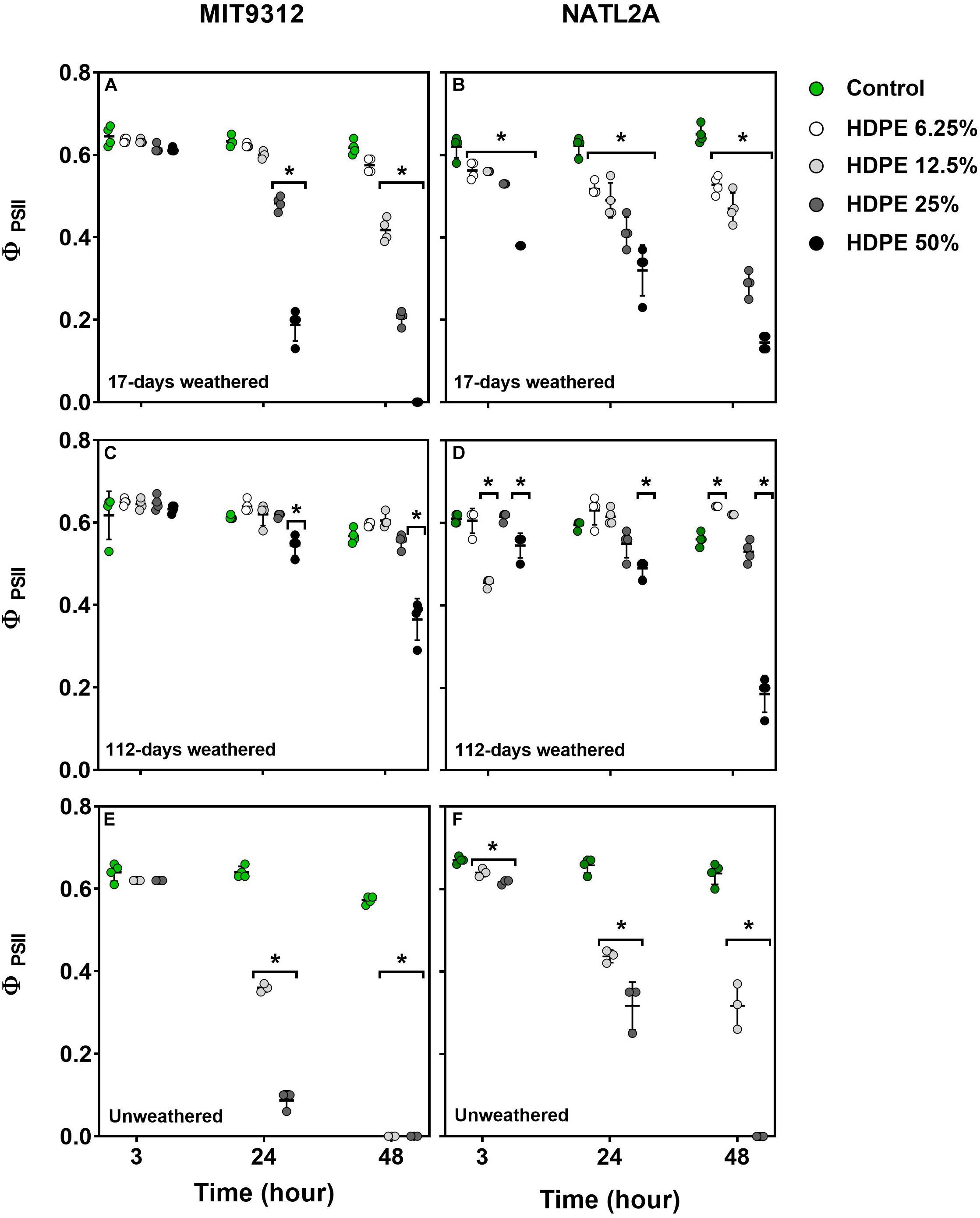
Figure 2. Effective quantum yield of photosystem II (ΦPSII) for Prochlorococcus MIT9312 and NATL2A exposed to different dilutions of HDPE leachates (A,B, weathered for 17-days; C,D, weathered for 112-days; E,F, unweathered) following different exposure times in hours. Experiments were conducted over 48 h using biological replicates (n = 4 for all weathered control and leachate treatments; n = 3 for all unweathered control and leachate treatments). Symbols represent the value for each replicate culture, the bar represents the mean ± standard deviations for the treatment at each time point. Asterisks (*) signify treatments for which measurements were significantly (p < 0.01) different compared to the control at the same time-point.
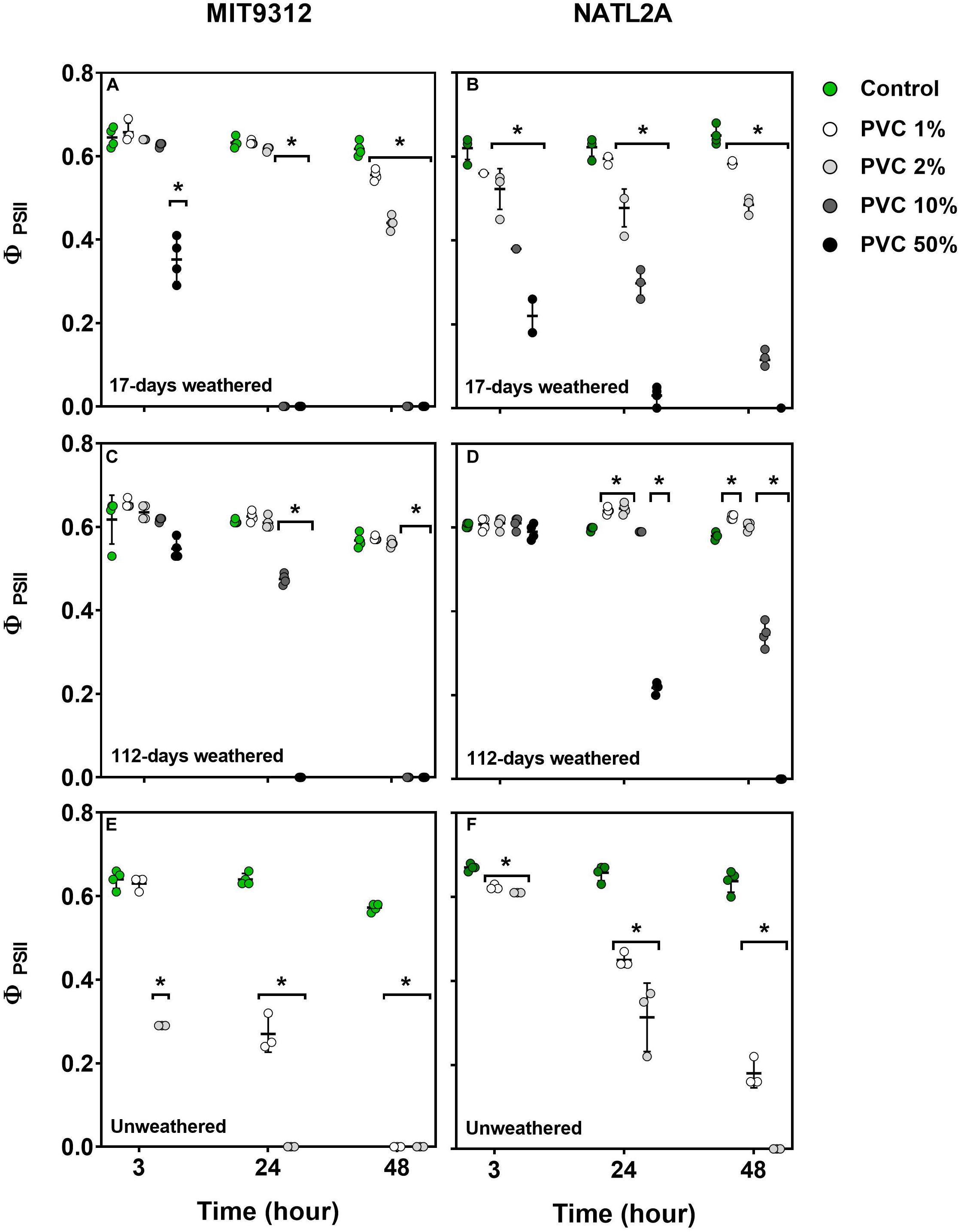
Figure 3. Effective quantum yield of photosystem II (ΦPSII) for Prochlorococcus MIT9312 and NATL2A exposed to different dilutions of PVC leachates (A,B, weathered for 17-days; C,D, weathered for 112-days; E,F, unweathered) following different exposure times. Experiments were conducted over 48 h using biological replicates (n = 4 for all weathered control and leachate treatments; n = 3 for all unweathered control and leachate treatments). Symbols represent the value for each replication, the bar represents the mean ± standard deviations for the treatment at each time point. Asterisks (*) signify treatments for which measurements were significantly (p < 0.01) different comparing to the control at the examined time-point.
The two strains showed differences in their photophysiological responses with respect to leachate dose sensitivity and timing. In strain MIT9312 photophysiology was not noticeably impacted at the 3 h time point by 17-days weathered HDPE leachate at any of the tested concentrations (Figure 2A). Significant (p < 0.01) declines in MIT9312 ΦPSII were not observed until 24 h of exposure for 25 and 50% dilutions, and 48 h for 12.5%. In contrast NATL2A showed significant (p < 0.01) reductions in ΦPSII starting at 3 h of exposure to all tested dilutions of 17-days weathered HDPE leachates. However, while the 17-days weathered HDPE elicited an earlier photophysiological response in NATL2A, this strain still retained measurable ΦPSII after 48 h exposure to the 50% dilution, whereas MIT9312 was completely inhibited by this (Figures 2A,B).
The longer weathering period of 112-days resulted in HDPE leachates which affected Prochlorococcus photophysiology to a lesser degree than leachates from 17-days of weathering, consistent with what was observed for growth rates. For both strains exposure to 112-days weathered HDPE only exhibited strongly impaired ΦPSII when the 50% dilution was applied. In NATL2A short term ΦPSII impairment was observed following exposure to 112-days weathered HDPE of 12.5% at 3 h and 25% at 24 h, but by 72 h these treatments had recovered and were similar to the control (Figure 2D). Measurement of ΦPSII in MIT9312 remained unchanged for 112-days weathered HDPE concentrations below 50% over the 48 h monitoring period (Figure 2C).
Weathered PVC leachate exposure also resulted in differences in the timing and degree of response between the two strains, particularly for exposure to leachates from the 17-days weathered material (Figure 3). Similar to what was observed for weathered HDPE, 3 h of exposure to 17-days weathered PVC did not affect ΦPSII in MIT9312 for most concentrations, with only the 50% leachate sample significantly affected at this early time point (Figure 3A). In NATL2A 3 h exposure to 17-days weathered PVC leachate resulted in significant reductions in ΦPSII across all tested concentrations (Figure 3B). Again, as for HDPE, while NATL2A ΦPSII responded more quickly to leachate exposure at all dilution levels, MIT9312 ΦPSII exhibited stronger declines by 24 h to the higher levels of weathered PVC leachate (10 and 50%) compared to NATL2A, where ΦPSII was still measurable for these treatments (Figures 3A,B).
After the longer weathering of 112-days, PVC leachates were still inhibitory to photosystem II in both strains when higher leachate percentages were applied (Figures 3C,D). Exposure to this longer weathered PVC leachate resulted in significant (p < 0.01) reductions in ΦPSII in MIT9312 when applied at 10% and higher from 24 h onward (Figure 3C). In NATL2A the 112-days weathered PVC leachate at 50% concentration also strongly reduced ΦPSII, at 24 h and after, while the 10% concentration resulted in strong reductions only at 48 h (Figure 3D). Exposure to lower concentrations of 112-days weathered PVC did not strongly affect ΦPSII, with values remaining similar or slightly higher than controls (Figures 3C,D).
Cell Membrane Integrity
For both Prochlorococcus strains, cell populations were examined using the “live/dead” SYTOX Green stain at 3, 24, and 48 h to determine whether leachate exposure resulted in membrane damage. The strains of Prochlorococcus showed differences in the proportion of the population showing membrane damage and in the timing at which this damage occurred following weathered leachate exposure. In strain MIT9312 noticeably higher proportions of the population showed cell membrane damage than for NATL2A when comparing most weathered and unweathered HDPE and PVC leachate treatments by 48 h (Figure 4 and Supplementary Table S1).
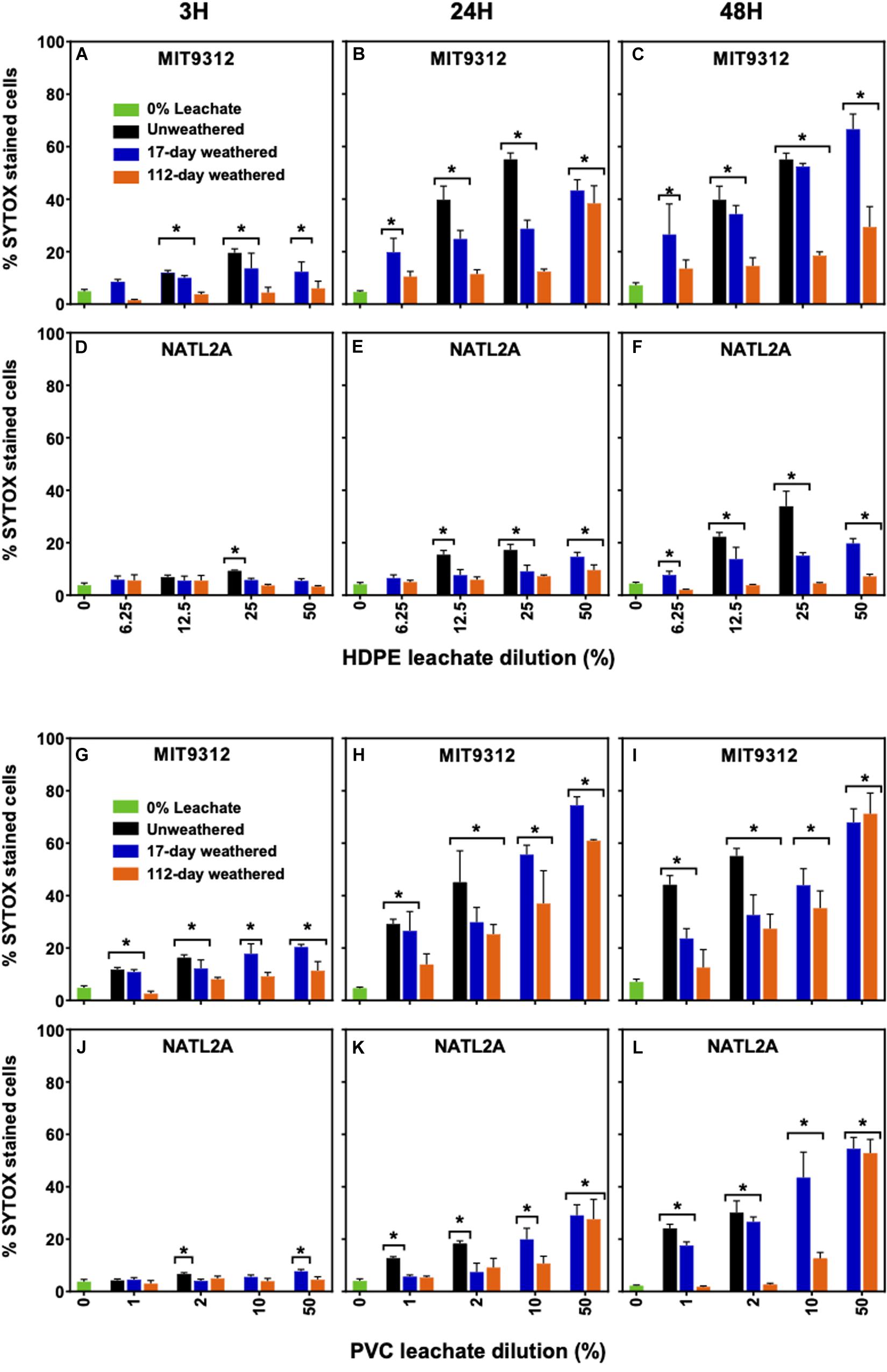
Figure 4. SYTOX Green stained (i.e., membrane compromised) populations after exposure to different dilutions of weathered HDPE leachates in Prochlorococcus (A–C) MIT9312, (D–F) NATL2A and PVC leachates in Prochlorococcus (G–I) MIT9312, (J–L) NATL2A. Leachate from HDPE and PVC weathered for 17-days in blue bars, weathered for 112-days in orange bars, unweathered in black bars. Zero leachate control are represented by green bars. Column height corresponds to mean ± standard deviation values of the biological replicates (n = 4 for all except unweathered where n = 3). Asterisks (*) are used to signify data points where measurements were significantly (p < 0.01) different compared to the 0% leachate control at each time point to eliminate the small changes in % SYTOX stained cells for the control.
Exposure to leachate from weathered HDPE plastic items resulted in a significant (p < 0.01) increase in the proportion of MIT9312 cells showing membrane damage compared to the no leachate control, with 17-days weathering effects observed as early as 3 h for 12.5% HDPE and higher. Additionally, the proportion of cells showing damage continued to increase over time (Figures 4A–C). By 48 h up to 70% of the MIT9312 population was membrane compromised for the 17-days weathered 50% HDPE treatment. The longer weathered (112-days) HDPE leachate also resulted in a significant increase in membrane compromised cells in MIT9312 for the 50% treatment at 24 h as well as both 25 and 50% at 48 h. Unweathered HDPE leachate had a similar or higher impact on membrane damage to the 17-days weathered leachates in MIT9312 (Figures 4A–C).
In NATL2A no significant changes in membrane integrity were observed at the early time point (3H) for any of the weathered HDPE leachate treatments (Figure 4D). For this strain small but significant (p < 0.01) changes were reported for 17-days weathered leachate at 24 h for 25% HDPE and higher, and for all % HDPE treatments at the 48 h point, while 112-days weathered HDPE only impacted membrane integrity at 50% after 24 h exposure or longer (Figures 4E,F). Unweathered leachate treatments affected NATL2A membrane integrity sooner and to a higher degree than weathered leachates, but the proportion of population affected remained below 40% (Figures 4D–F).
Plastic PVC matting leachates from both weathering periods resulted in significantly (p < 0.01) increased proportions of membrane compromised cells after 48 h exposure for concentrations of 10% or higher for both tested strains (Figure 4). For MIT9312 17-days weathered PVC leachate significantly (p < 0.01) increased the population of SYTOX stained cells after as little as 3 h for dilutions as low as 1% PVC (Figures 4G–I). Longer weathering (112-days) resulted in leachates that damaged cell membranes more slowly. However, by 24 h MIT9312 exposure to 2% PVC and above had significantly increased the % SYTOX stained populations, indicating that plastics were still leaching substances damaging to cells after close to 4 months of weathering. In NATL2A after 3 h, only exposure to 50% of 17-days weathered PVC leachate exhibited significantly (p < 0.01) compromised membranes, and not until 48 h of exposure did all tested 17-days weathered leachate treatments show significant membrane damage (Figures 4J–L). For both strains unweathered PVC showed a similar or higher impact on membrane integrity than equivalent dilutions of leachate from weathered material (Figure 4).
Elemental Analysis
Leachate elemental composition analyses conducted using Inductively Coupled Plasma-Optical Emission Spectrometry and Mass Spectrometry (ICP-OES and ICP-MS) identified elements enriched in leachates relative to AMP1 control medium with no plastic leachate added. The concentration of zinc (Zn) was elevated in all analyzed leachates, with the highest degree of enrichment in leachates from unweathered material, followed by 17-days weathered, then 112-days weathered material (Tables 1A,B). PVC consistently leached significantly (p < 0.01) higher amounts of Zn than HDPE with leachates from unweathered HDPE and PVC plastics containing ∼30X and ∼458X higher Zn than the AMP1 control, respectively, similar to what was observed previously (Tetu et al., 2019). After 17-days of weathering, average Zn levels dropped in both HDPE and PVC leachates such that they were ∼13X and ∼70X higher, respectively, compared to what was measured for the AMP1 control, however, these differences were not statistically significant. After the longer 112-days period of environmental weathering, HDPE leachate contained slightly elevated Zn (∼3X) while levels were still ∼30X enriched in the PVC leachates relative to AMP1 control media (both differences not statistically significant). Both HDPE and PVC weathered leachates also showed somewhat higher levels of strontium (Sr) than the AMP1 control media, however, this was only significant (p < 0.01) for 112-days weathered HDPE (Tables 1A, B). The concentrations of chromium (Cr), copper (Cu), manganese (Mn), and nickel (Ni) were below the method detection limit (MDL) in the AMP1 control media but within the measurable range in some leachates suggesting these may have also been slightly enriched in some leachates. Of the other elements measured, most were below the MDL in both media and leachate (Si, As, Cd, Mo, Pb, Sn, V) or were observed at similar levels in leachates and the AMP1 base media (Ca, Na, S, Al, B, Ba) (Tables 1A, B).
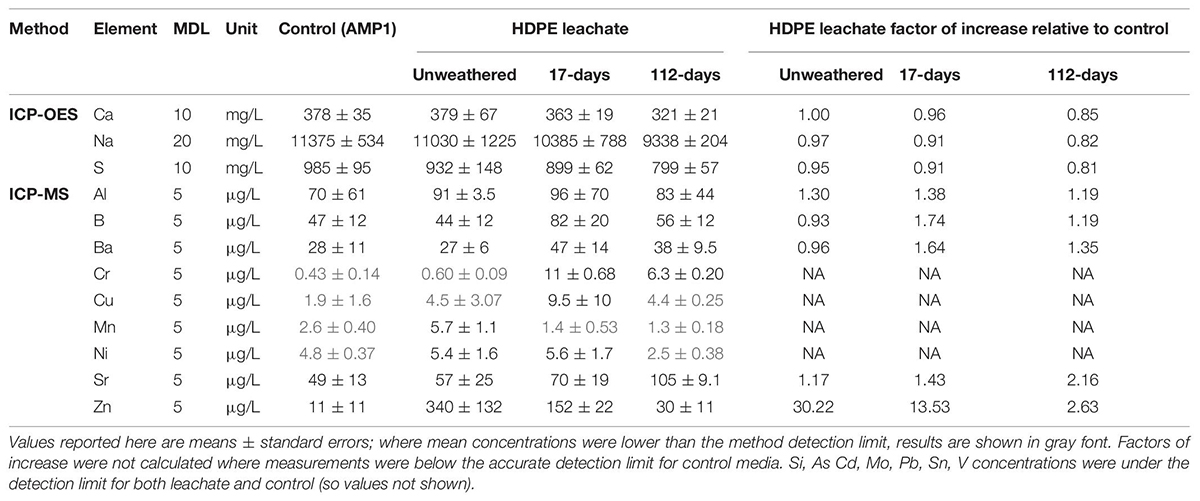
Table 1A. Concentrations of selected elements in unweathered and weathered (17- and 112-days) HDPE leachates AMP1 and control media detected by ICP-OES and ICP-MS, and their factor of increase in leachates relative to control.
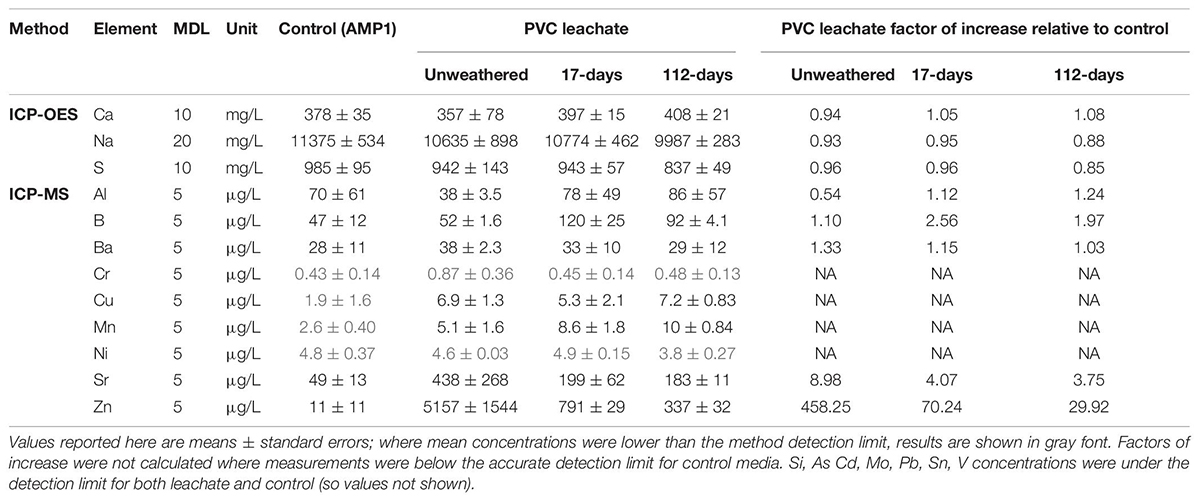
Table 1B. Concentrations of selected elements in unweathered and weathered (17 and 112-days) PVC leachates and AMP1 control media detected by ICP-OES and ICP-MS, and their factor of increase in leachates relative to control.
Discussion
While there is now some recognition that plastic pollution may impact marine organisms via leaching of harmful chemicals (Hermabessiere et al., 2017), the effect of weathering on leachate toxicity is still poorly understood. To our knowledge only a few studies have investigated how weathering of plastics affects the toxicity of substances which leach from this material and these have focused on toxicity to eukaryotes rather than to bacteria (Bejgarn et al., 2015; Kedzierski et al., 2018; Gardon et al., 2020; Kolomijeca et al., 2020). In this study, we report that common HDPE and PVC plastic items continue to leach substances following environmental weathering for up to 112-days which can adversely impact Prochlorococcus growth, photosynthesis and membrane integrity. However, the weathering process did substantially reduce toxicity.
The environmental weathering applied here to HDPE bags and PVC matting resulted in leachates that were considerably less toxic than leachates from unweathered material, and there was a continued reduction in toxicity between the 17- and 112-days leaching period, based on growth rate, photophysiology and membrane integrity measurements. This indicates that over time, weathering in estuarine waters can change the composition or concentration of leachable compounds that are capable of adversely affecting marine Prochlorococcus growth. This is similar to what was recently reported in work on pearl oysters comparing leachate toxicity from new and aged plastic pearl-farming gear (a mix of polyethylene and polypropylene), which reported reduced toxicity for leachates from aged material (Gardon et al., 2020).
Leachates derived from weathered PVC showed higher toxicity than was observed with the equivalent HDPE weathered leachate, as seen with unweathered leachates from these materials. Toxicity of PVC was also maintained over a longer period of weathering with 112-days weathered PVC leachate still able to significantly impair growth and photophysiology at concentrations as low as 10%. The elemental analysis performed in this study showed PVC continued to leach zinc at amounts higher than the basal media at the longer 112-days weathering period. The HDPE product, which leached lower levels of zinc to begin with, showed zinc levels only slightly higher than the base media in 112-days weathered material. PVC products have been shown to leach higher levels of zinc than other polymer types, in our previous study (Tetu et al., 2019) and by others (Oliviero et al., 2019). Our results here indicate that PVC litter may also be particularly problematic as it has the potential to continue to leach zinc over a considerable period of environmental weathering. Additionally, it is likely that leachate components not measured in this study, including organic substances, were also different across the two tested weathered materials and the composition and concentrations of these other substances could also have contributed to the observed differences in toxicity. Both organic and inorganic additives are likely to be substantially higher in weathered PVC leachate than from other plastics types, as production of PVC uses much higher amounts of additives than any other polymer type, with an estimated 73% of world production of additives directed to PVC plastics (Rochman, 2015). Copper, a known toxicant of Prochlorococcus at concentrations as low as 2.3 pM (Mann et al., 2002), and manganese, known to reduce chlorophyll a biosynthesis and photosynthesis in Synechocystis sp. PCC 6803 at 150 μM (Brandenburg et al., 2017; Eisenhut, 2020), also continued to leach from weathered PVC at amounts above those measured in the AMP1 control media and may have also contributed to the toxicity of weathered PVC.
Environmental weathering processes have the potential to alter plastic leachate composition in a variety of ways. A wide range of plastic additives, including metals and organic compounds used during manufacturing, can leach out from plastic debris immersed in water (Hermabessiere et al., 2017; Hahladakis et al., 2018). Work by Kedzierski et al. (2018) examining PVC plastic weathered in seawater showed that additives released from this material had increasing estrogenic activity on Saccharomyces cerevisiae from 14 to 63 days, following which it declined for the remainder of the 502-days study period. They suggest this may be due to immersion resulting in an initial loosening of the PVC polymer matrix, facilitating the desorption of entrapped compounds into the seawater (Kedzierski et al., 2018). However, desorption of substances from weathered plastics likely varies for different starting materials, as seen in this study and in previous work with marine copepods which showed simulated weathering (UV) of a variety of plastics resulted in leachates with increased toxicity for some items but unaltered or decreased toxicity for other materials (Bejgarn et al., 2015). Recent lab-based experiments which have begun to explore how certain characteristics of the weathering environment, such as temperature and mechanical stress, can affect leachate toxicity have also indicated that the nature of such changes is complex and product dependent (Kolomijeca et al., 2020).
The other main potential source of toxicants from weathered plastic are substances from the environment that adsorb to plastic debris whilst immersed in marine waters (Rochman, 2015), which may, under some set of conditions, later desorb. While the dynamics of adsorption and desorption relating to plastics are variable and generally not well understood (Ashton et al., 2010; Holmes et al., 2012, 2014), it is thought that this process may be a potential source of metals in some marine ecosystems (Munier and Bendell, 2018; Prunier et al., 2019). Our elemental analysis showed that higher levels of Cr and Sr were leached from weathered HDPE than from unweathered material, while leached Mn was elevated in weathered PVC, with levels increasing as the weathering period extended, indicating these metals may have been adsorbed from the surrounding estuarine waters or sediments. However, it is also possible they represent additives which have only begun to be released after some period of weathering. In either case it is clear there is potential for complex alterations in the makeup of leached substances and for continued toxicity as plastic weathering proceeds.
While this work sheds light on the potential for environmental weathering to affect the composition and toxicity of plastic leachates, it is important to point out that these experiments were conducted using methodology based on previous leachate ecotoxicology testing (Lithner et al., 2012a) rather than seeking to mimic environmental exposure scenarios. Indeed, it is difficult to determine what marine bacteria in the oceans may currently experience in terms of leachate exposure, as there are very few measurements of the substances that leach from plastic debris, or their concentrations, in the marine environment. The patterns and timeframes associated with movement of plastics from coastal source locations to open ocean regions are also complex and variable, influenced by temporal and spatial factors (e.g., season and currents), water properties (e.g., sea surface height and current velocities), and plastic particle properties (e.g., size, type and extent of biofouling) (Kako et al., 2010; Maximenko et al., 2012; Liubartseva et al., 2018; Jalón-Rojas et al., 2019). In one study based on models of drifters, Maximenko et al. (2012) estimated that the minimum time for microplastic debris to reach the Great Pacific Garbage Patch from the West Coast of the United States was less than 100 days. While there are still many unknowns, studies to date do suggest the HDPE bags and PVC matting used in this work represent the types of plastic that are likely to maintain buoyancy, even when degraded into smaller particles, remaining for some time in the photic zone down to at least 50 m (Erni-Cassola et al., 2019; Lebreton and Andrady, 2019) where the Prochlorococcus strains used in this study reside (Partensky et al., 1999b; Malmstrom et al., 2010). Although the marine cyanobacterium Prochlorococcus used in this study is the dominant open ocean photosynthetic genus, it is also found in mesotrophic and coastal seas, including the Adriatic Sea (Santic et al., 2011) and East China Seas (Jiao et al., 2005) which receive significant amounts of plastic waste that are estimated to have a floating half-life before beaching of 7–80 days (Kako et al., 2014; Liubartseva et al., 2018).
The two Prochlorococcus strains used in this study showed noticeable differences in the timing and extent of certain aspects of their response to each of the tested weathered leachates, similar to what was observed in our previous study (Tetu et al., 2019). Growth rate measurements indicated that MIT9312, a high light ecotype, was more susceptible to strong growth rate reduction than NATL2A, a low light ecotype, when exposed to HDPE and PVC weathered leachates. Photophysiological measurements showed MIT9312 exhibited stronger reductions in ΦPSII at a wider range of weathered leachate concentrations at 24 and 48 h, but responses tended to be observed at earlier timepoints in NATL2A than for MIT9312. Transcriptomics results from our earlier study showed that each of these strains co-opts a different regulatory network in response to unweathered leachate exposure, which likely accounts for the different growth and photophysiological responses and sensitivities also observed here for weathered leachate exposure. Interestingly, previous work on copper toxicity in Prochlorococcus, using different strains to those tested here, showed high-light adapted Prochlorococcus to be more copper resistant than low-light adapted ecotypes (Mann et al., 2002), contrasting with what we observed here for plastic leachate toxicity. Although there are no known studies on metal toxicity for the strains of Prochlorococcus used in our study, it is possible that the differences in plastic leachate toxicity between these two strains may be related to ecotypic differences in responses to the high amounts of zinc released by these weathered plastic items. Investigating plastic leachate sensitivities and transcriptional responses across a wider range of Prochlorococcus strains and ecotypes would assist with determining if the leachate sensitivity differences seen here are specific to particular strains, or generally consistent among different representatives of high light and low light ecotypes.
We also observed clear differences between our tested strains in regard to membrane damage following leachate exposure, with membrane integrity compromised in MIT9312 sooner (as early as the 3 h timepoint) and across a wider range of weathered leachate concentrations than seen in NATL2A. We measured changes in membrane integrity in this work as transcriptomic results from our previous study (Tetu et al., 2019) implied that MIT9312 might be more sensitive to membrane damage following plastic leachate exposure. In the previous work MIT9312 showed significantly reduced transcription of a number of cell wall and membrane biogenesis-associated genes in response to unweathered leachate exposure, while few genes in these categories showed significantly different transcription in NATL2A, which is consistent with the greater membrane sensitivity observed here for MIT9312. While it is not possible to identify whether specific leachate components are responsible for membrane damage, one possibility is that zinc in the leachates may have contributed, as previous investigations into the response of freshwater cyanobacteria to high levels of zinc showed cell membrane impairment (Newby et al., 2017). Future studies focused on toxicity of specific plastic leachate components, including organic substances, would help determine which component, or mix of components, contributes to membrane damage and would allow the investigation of mechanisms by which specific toxicants affect cell membranes and other processes in these organisms.
Conclusion
Both HDPE and PVC plastic products investigated here showed reduced toxicity after environmental weathering, whilst retaining some capacity to negatively affect Prochlorococcus cell membrane integrity and photophysiology. Weathering was observed to alter the elemental composition of plastic leachates, with the substances leached into artificial seawater following weathering, potentially representing both additives and substances adsorbed from the estuarine environment. Zinc, a widely used plastic additive and a known toxicant, was particularly noticeable in PVC products and leached at relatively high levels, suggesting PVC materials may retain the capacity to adversely impact some photosynthetic marine bacteria for at least 112 days after introduction to the environment, as observed in this work. Further studies into adsorption/desorption dynamics of different plastics under environmental weathering conditions looking at both organic and inorganic leached substances are needed to better understand how plastic litter may affect key marine organisms, particularly microorganisms. In this work we have also shown that two strains of Prochlorococcus differed in the timing and severity of their response to the weathered leachate, in ways reminiscent of their responses to unweathered plastic leachate. Extending such investigations across a wider variety of Prochlorococcus strains, other marine photosynthetic microorganisms and natural communities will help determine the range of organisms which respond negatively to plastic leachates and potentially identify different stress response mechanisms employed to respond to toxicants. Such information could help with predicting whether release of toxicants from marine plastic debris during weathering has the potential to alter the composition or functionality of marine microbial communities.
Data Availability Statement
The raw data supporting the conclusions of this article will be made available by the authors, without undue reservation.
Author Contributions
ST conceptualized the research with input from IS. IS performed the experiments and carried out the data analysis with help from LM and ST. All authors contributed to writing the manuscript.
Funding
This work was primarily supported by the Australian Research Council fellowships to ST (#DE150100009). IS was supported by a Macquarie University iMQRES scholarship.
Conflict of Interest
The authors declare that the research was conducted in the absence of any commercial or financial relationships that could be construed as a potential conflict of interest.
Supplementary Material
The Supplementary Material for this article can be found online at: https://www.frontiersin.org/articles/10.3389/fmars.2020.571929/full#supplementary-material
Footnotes
References
Andrady, A. L., and Neal, M. A. (2009). Applications and societal benefits of plastics. Philos. Trans. R. Soc. B Biol. Sci. 364, 1977–1984. doi: 10.1098/rstb.2008.0304
Ashton, K., Holmes, L., and Turner, A. (2010). Association of metals with plastic production pellets in the marine environment. Mar. Pollut. Bull. 60, 2050–2055. doi: 10.1016/j.marpolbul.2010.07.014
Avio, C. G., Gorbi, S., and Regoli, F. (2017). Plastics and microplastics in the oceans: from emerging pollutants to emerged threat. Mar. Environ. Res. 128, 2–11. doi: 10.1016/j.marenvres.2016.05.012
Barnes, D. K., Galgani, F., Thompson, R. C., and Barlaz, M. (2009). Accumulation and fragmentation of plastic debris in global environments. Philos. Trans. R. Soc. B Biol. Sci. 364, 1985–1998. doi: 10.1098/rstb.2008.0205
Bejgarn, S., MacLeod, M., Bogdal, C., and Breitholtz, M. (2015). Toxicity of leachate from weathering plastics: an exploratory screening study with Nitocra spinipes. Chemosphere 132, 114–119. doi: 10.1016/j.chemosphere.2015.03.010
Biller, S. J., Berube, P. M., Lindell, D., and Chisholm, S. W. (2015). Prochlorococcus: the structure and function of collective diversity. Nat. Rev. Microbiol. 13, 13–27. doi: 10.1038/nrmicro3378
Boucher, C., Morin, M., and Bendell, L. I. (2016). The influence of cosmetic microbeads on the sorptive behavior of cadmium and lead within intertidal sediments: a laboratory study. Region. Stud. Mar. Sci. 3, 1–7. doi: 10.1016/j.rsma.2015.11.009
Brandenburg, F., Schoffman, H., Kurz, S., Krämer, U., Keren, N., Weber, A. P. M., et al. (2017). The Synechocystis manganese exporter Mnx is essential for manganese homeostasis in cyanobacteria. Plant Physiol. 173:1798. doi: 10.1104/pp.16.01895
Browne, M. A., Galloway, T., and Thompson, R. (2007). Microplastic-an emerging contaminant of potential concern? Integr. Environ. Assess. Manag. 3, 559–561. doi: 10.1002/ieam.5630030412
Derraik, J. G. (2002). The pollution of the marine environment by plastic debris: a review. Mar. Pollut. Bull. 44, 842–852. doi: 10.1016/s0025-326x(02)00220-5
Eisenhut, M. (2020). Manganese homeostasis in cyanobacteria. Plants 9:18. doi: 10.3390/plants9010018
Erni-Cassola, G., Zadjelovic, V., Gibson, M. I., and Christie-Oleza, J. A. (2019). Distribution of plastic polymer types in the marine environment; A meta-analysis. J. Hazard. Mater. 369, 691–698. doi: 10.1016/j.jhazmat.2019.02.067
Fernández-Pinos, M.-C., Vila-Costa, M., Arrieta, J. M., Morales, L., González-Gaya, B., Piña, B., et al. (2017). Dysregulation of photosynthetic genes in oceanic Prochlorococcus populations exposed to organic pollutants. Sci. Rep. 7:8029. doi: 10.1038/s41598-017-08425-9
Flombaum, P., Gallegos, J. L., Gordillo, R. A., Rincon, J., Zabala, L. L., Jiao, N., et al. (2013). Present and future global distributions of the marine Cyanobacteria Prochlorococcus and Synechococcus. Proc. Natl. Acad. Sci. U.S.A. 110, 9824–9829. doi: 10.1073/pnas.1307701110
Gall, S. C., and Thompson, R. C. (2015). The impact of debris on marine life. Mar. Pollut. Bull. 92, 170–179. doi: 10.1016/j.marpolbul.2014.12.041
Gandara, E., Silva, P. P., Nobre, C. R., Resaffe, P., Pereira, C. D. S., and Gusmão, F. (2016). Leachate from microplastics impairs larval development in brown mussels. Water Res. 106, 364–370. doi: 10.1016/j.watres.2016.10.016
Gardon, T., Huvet, A., Paul-Pont, I., Cassone, A.-L., Koua, M. S., Soyez, C., et al. (2020). Toxic effects of leachates from plastic pearl-farming gear on embryo-larval development in the pearl oyster Pinctada margaritifera. Water Res. 179:115890. doi: 10.1016/j.watres.2020.115890
Gregory, M. R. (2009). Environmental implications of plastic debris in marine settings-entanglement, ingestion, smothering, hangers-on, hitch-hiking and alien invasions. Philos. Trans. R. Soc. B Biol. Sci. 364, 2013–2025. doi: 10.1098/rstb.2008.0265
Hahladakis, J. N., Velis, C. A., Weber, R., Iacovidou, E., and Purnell, P. (2018). An overview of chemical additives present in plastics: migration, release, fate and environmental impact during their use, disposal and recycling. J. Hazard Mater. 344, 179–199. doi: 10.1016/j.jhazmat.2017.10.014
Hermabessiere, L., Dehaut, A., Paul-Pont, I., Lacroix, C., Jezequel, R., Soudant, P., et al. (2017). Occurrence and effects of plastic additives on marine environments and organisms: a review. Chemosphere 182, 781–793. doi: 10.1016/j.chemosphere.2017.05.096
Holmes, L. A., Turner, A., and Thompson, R. C. (2012). Adsorption of trace metals to plastic resin pellets in the marine environment. Environ. Pollut. 160, 42–48. doi: 10.1016/j.envpol.2011.08.052
Holmes, L. A., Turner, A., and Thompson, R. C. (2014). Interactions between trace metals and plastic production pellets under estuarine conditions. Mar. Chem. 167, 25–32. doi: 10.1016/j.marchem.2014.06.001
Jalón-Rojas, I., Wang, X. H., and Fredj, E. (2019). A 3D numerical model to track marine plastic debris (TrackMPD): sensitivity of microplastic trajectories and fates to particle dynamical properties and physical processes. Mar. Pollut. Bull. 141, 256–272. doi: 10.1016/j.marpolbul.2019.02.052
Jiao, N., Yang, Y., Hong, N., Ma, Y., Harada, S., Koshikawa, H., et al. (2005). Dynamics of autotrophic picoplankton and heterotrophic bacteria in the East China Sea. Continent. Shelf Res. 25, 1265–1279. doi: 10.1016/j.csr.2005.01.002
Kako, S. I., Isobe, A., Kataoka, T., and Hinata, H. (2014). A decadal prediction of the quantity of plastic marine debris littered on beaches of the East Asian marginal seas. Mar. Pollut. Bull. 81, 174–184. doi: 10.1016/j.marpolbul.2014.01.057
Kako, S. I., Isobe, A., Seino, S., and Kojima, A. (2010). Inverse estimation of drifting-object outflows using actual observation data. J. Oceanogr. 66, 291–297. doi: 10.1007/s10872-010-0025-9
Kedzierski, M., D’Almeida, M., Magueresse, A., Le Grand, A., Duval, H., César, G., et al. (2018). Threat of plastic ageing in marine environment. Adsorption/desorption of micropollutants. Mar. Pollut. Bull. 127, 684–694. doi: 10.1016/j.marpolbul.2017.12.059
Kolomijeca, A., Parrott, J., Khan, H., Shires, K., Clarence, S., Sullivan, C., et al. (2020). Increased temperature and turbulence alter the effects of leachates from tire particles on fathead minnow (Pimephales promelas). Environ. Sci. Technol. 54, 1750–1759. doi: 10.1021/acs.est.9b05994
Lebreton, L., and Andrady, A. (2019). Future scenarios of global plastic waste generation and disposal. Palgrave Commun. 5:6. doi: 10.1057/s41599-018-0212-7
León, V. M., García, I., González, E., Samper, R., Fernández-González, V., and Muniategui-Lorenzo, S. (2018). Potential transfer of organic pollutants from littoral plastics debris to the marine environment. Environ. Pollut. 236, 442–453. doi: 10.1016/j.envpol.2018.01.114
Li, H. X., Getzinger, G. J., Ferguson, P. L., Orihuela, B., Zhu, M., and Rittschof, D. (2016). Effects of toxic leachate from commercial plastics on larval survival and settlement of the barnacle Amphibalanus amphitrite. Environ. Sci. Technol. 50, 924–931. doi: 10.1021/acs.est.5b02781
Lithner, D., Damberg, J., Dave, G., and Larsson, K. (2009). Leachates from plastic consumer products–screening for toxicity with Daphnia magna. Chemosphere 74, 1195–1200. doi: 10.1016/j.chemosphere.2008.11.022
Lithner, D., Halling, M., and Dave, G. (2012a). Toxicity of electronic waste leachates to Daphnia magna: screening and toxicity identification evaluation of different products, components, and materials. Arch. Environ. Contam. Toxicol. 62, 579–588. doi: 10.1007/s00244-011-9729-0
Lithner, D., Nordensvan, I., and Dave, G. (2012b). Comparative acute toxicity of leachates from plastic products made of polypropylene, polyethylene, PVC, acrylonitrile-butadiene-styrene, and epoxy to Daphnia magna. Environ. Sci. Pollut. Res. 19, 1763–1772. doi: 10.1007/s11356-011-0663-5
Liubartseva, S., Coppini, G., Lecci, R., and Clementi, E. (2018). Tracking plastics in the Mediterranean: 2D lagrangian model. Mar. Pollut. Bull. 129, 151–162. doi: 10.1016/j.marpolbul.2018.02.019
Llabrés, M., and Agustí, S. (2006). Picophytoplankton cell death induced by UV radiation: evidence for oceanic Atlantic communities. Limnol. Oceanogr. 51, 21–29. doi: 10.4319/lo.2006.51.1.0021
Luo, H., Xiang, Y., He, D., Li, Y., Zhao, Y., Wang, S., et al. (2019). Leaching behavior of fluorescent additives from microplastics and the toxicity of leachate to Chlorella vulgaris. Sci. Total Environ. 678, 1–9. doi: 10.1016/j.scitotenv.2019.04.401
Malmstrom, R. R., Coe, A., Kettler, G. C., Martiny, A. C., Frias-Lopez, J., Zinser, E. R., et al. (2010). Temporal dynamics of Prochlorococcus ecotypes in the Atlantic and Pacific oceans. ISME J. 4, 1252–1264. doi: 10.1038/ismej.2010.60
Mann, E. L., Ahlgren, N., Moffett, J. W., and Chisholm, S. W. (2002). Copper toxicity and cyanobacteria ecology in the Sargasso Sea. Limnol. Oceanogr. 47, 976–988. doi: 10.4319/lo.2002.47.4.0976
Mato, Y., Isobe, T., Takada, H., Kanehiro, H., Ohtake, C., and Kaminuma, T. (2001). Plastic resin pellets as a transport medium for toxic chemicals in the marine environment. Environ. Sci. Technol. 35, 318–324. doi: 10.1021/es0010498
Maximenko, N., Hafner, J., and Niiler, P. (2012). Pathways of marine debris derived from trajectories of Lagrangian drifters. Mar. Pollut. Bull. 65, 51–62. doi: 10.1016/j.marpolbul.2011.04.016
Mikula, P., Zezulka, S., Jancula, D., and Marsalek, B. (2012). Metabolic activity and membrane integrity changes in Microcystis aeruginosa – new findings on hydrogen peroxide toxicity in cyanobacteria. Eur. J. Phycol. 47, 195–206. doi: 10.1080/09670262.2012.687144
Moore, L. R., Coe, A., Zinser, E. R., Saito, M. A., Sullivan, M. B., Lindell, D., et al. (2007). Culturing the marine cyanobacterium Prochlorococcus. Limnol. Oceanogr. Methods 5, 353–362. doi: 10.4319/lom.2007.5.353
Munier, B., and Bendell, L. I. (2018). Macro and micro plastics sorb and desorb metals and act as a point source of trace metals to coastal ecosystems. PLoS One 13:e0191759. doi: 10.1371/journal.pone.0191759
Newby, R., Lee, L. H., Perez, J. L., Tao, X., and Chu, T. (2017). Characterization of zinc stress response in cyanobacterium Synechococcus sp. IU 625. Aquat. Toxicol. 186, 159–170. doi: 10.1016/j.aquatox.2017.03.005
Oliviero, M., Tato, T., Schiavo, S., Fernandez, V., Manzo, S., and Beiras, R. (2019). Leachates of micronized plastic toys provoke embryotoxic effects upon sea urchin Paracentrotus lividus. Environ. Pollut. 247, 706–715. doi: 10.1016/j.envpol.2019.01.098
Partensky, F., Blanchot, J., and Vaulot, D. (1999a). Differential distribution and ecology of Prochlorococcus and Synechococcus in oceanic waters: a review. Bull. l’Institut Océanogr. Monaco n° Spéc. 19, 457–475.
Partensky, F., Hess, W. R., and Vaulot, D. (1999b). Prochlorococcus, a marine photosynthetic prokaryote of global significance. Microbiol. Mol. Biol. Rev. 63, 106–127. doi: 10.1128/mmbr.63.1.106-127.1999
Plastics Europe (2018). Plastics - the Facts 2018: An Analysis of European Plastics Production, Demand and Waste Data. Available: https://www.plasticseurope.org/en/resources/publications/619-plastics-facts-2018 (accessed November 15, 2018).
Prunier, J., Maurice, L., Perez, E., Gigault, J., Wickmann, A.-C. P., Davranche, M., et al. (2019). Trace metals in polyethylene debris from the North Atlantic subtropical gyre. Environ. Pollut. 245, 371–379. doi: 10.1016/j.envpol.2018.10.043
Rios, L. M., Moore, C., and Jones, P. R. (2007). Persistent organic pollutants carried by synthetic polymers in the ocean environment. Mar. Pollut. Bull. 54, 1230–1237. doi: 10.1016/j.marpolbul.2007.03.022
Rochman, C. M. (2015). “The complex mixture, fate and toxicity of chemicals associated with plastic debris in the marine environment,” in Marine Anthropogenic Litter, eds M. Bergmann, L. Gutow, and M. Klages (Cham: Springer), 117–140. doi: 10.1007/978-3-319-16510-3_5
Santic, D., Krstulovic, N., Solic, M., and Kuspilic, G. (2011). Distribution of Synechococcus and Prochlorococcus in the central Adriatic Sea. Acta Adriatica 52, 101–113.
Tetu, S. G., Sarker, I., Schrameyer, V., Pickford, R., Elbourne, L. D. H., Moore, L. R., et al. (2019). Plastic leachates impair growth and oxygen production in Prochlorococcus, the ocean’s most abundant photosynthetic bacteria. Commun. Biol. 2:184. doi: 10.1038/s42003-019-0410-x
Teuten, E. L., Saquing, J. M., Knappe, D. R., Barlaz, M. A., Jonsson, S., Bjorn, A., et al. (2009). Transport and release of chemicals from plastics to the environment and to wildlife. Philos. Trans. R. Soc. B Biol. Sci. 364, 2027–2045. doi: 10.1098/rstb.2008.0284
Thompson, R. C., Moore, C. J., vom Saal, F. S., and Swan, S. H. (2009a). Plastics, the environment and human health: current consensus and future trends. Philos. Trans. R. Soc. B Biol. Sci. 364, 2153–2166. doi: 10.1098/rstb.2009.0053
Thompson, R. C., Swan, S. H., Moore, C. J., and Vom Saal, F. S. (2009b). Our plastic age. Philos. Trans. R. Soc. B Biol. Sci. 364, 1973–1976. doi: 10.1098/rstb.2009.0054
Keywords: weathering, plastic leachate, marine cyanobacteria, stress response, effective quantum yield of PSII, zinc
Citation: Sarker I, Moore LR, Paulsen IT and Tetu SG (2020) Assessing the Toxicity of Leachates From Weathered Plastics on Photosynthetic Marine Bacteria Prochlorococcus. Front. Mar. Sci. 7:571929. doi: 10.3389/fmars.2020.571929
Received: 12 June 2020; Accepted: 26 August 2020;
Published: 17 September 2020.
Edited by:
Stephanie Brodie, University of California, Santa Cruz, United StatesReviewed by:
Anna Ruth Robuck, University of Rhode Island, United StatesRhona K. Stuart, Lawrence Livermore National Laboratory (DOE), United States
Copyright © 2020 Sarker, Moore, Paulsen and Tetu. This is an open-access article distributed under the terms of the Creative Commons Attribution License (CC BY). The use, distribution or reproduction in other forums is permitted, provided the original author(s) and the copyright owner(s) are credited and that the original publication in this journal is cited, in accordance with accepted academic practice. No use, distribution or reproduction is permitted which does not comply with these terms.
*Correspondence: Sasha G. Tetu, c2FzaGEudGV0dUBtcS5lZHUuYXU=