- 1Division of Biological and Environmental Science and Engineering, Red Sea Research Center, King Abdullah University of Science and Technology, Thuwal, Saudi Arabia
- 2Department of Biology, University of Konstanz, Konstanz, Germany
- 3Centre for Organismal Studies, University of Heidelberg, Heidelberg, Germany
- 4Department of Animal Ecology and Systematics, Justus Liebig University Giessen, Giessen, Germany
Coral bleaching, i.e., the loss of photosynthetic algal endosymbionts, caused by ocean warming is now among the main factors driving global reef decline, making the elucidation of factors that contribute to thermotolerance important. Recent studies implicate high salinity as a contributing factor in cnidarians, potentially explaining the high thermotolerance of corals from the Arabian Seas. Here we characterized bacterial community composition under heat stress at different salinities using the coral model Aiptasia. Exposure of two Aiptasia host-algal symbiont pairings (H2-SSB01 and CC7-SSA01) to ambient (25°C) and heat stress (34°C) temperatures at low (36 PSU), intermediate (39 PSU), and high (42 PSU) salinities showed that bacterial community composition at high salinity was significantly different, concomitant with reduced bleaching susceptibility in H2-SSB01, not observed in CC7-SSA01. Elucidation of bacteria that showed increased relative abundance at high salinity, irrespective of heat stress, revealed candidate taxa that could potentially contribute to the observed increased thermotolerance. We identified 4 (H2-SSB01) and 3 (CC7-SSA01) bacterial taxa belonging to the orders Alteromonadales (1 OTU), Oligoflexales (1 OTU), Rhizobiales (2 OTUs), and Rhodobacterales (2 OTUs), suggesting that only few bacterial taxa are potential contributors to an increase in thermal tolerance at high salinities. These taxa have previously been implicated in nitrogen and DMSP cycling, processes that are considered to affect thermotolerance. Our study demonstrates microbiome restructuring in symbiotic cnidarians under heat stress at different salinities. As such, it underlines how host-associated bacterial communities adapt to prevailing environmental conditions with putative consequences for the environmental stress tolerance of the emergent metaorganism.
Introduction
Corals are keystone species that, through the secretion of calcium carbonate, create the three-dimensional structures that constitute one of the most biodiverse ecosystems on Earth: coral reefs (Knowlton et al., 2010). Coral reefs are ecologically important because of their high biodiversity, and economically important by providing ecosystem services (Costanza et al., 2014). But reef ecosystems are under threat due to global coral bleaching caused by climate change (Bellwood et al., 2004; Hughes et al., 2017).
Coral bleaching refers to the breakdown of the obligate coral-algal symbiosis, which leads to the expulsion of Symbiodiniaceae from the host and may ultimately entail the death of the coral (Dove and Hoegh-Guldberg, 2006). Although bleaching thresholds are approximately 1°C above average summer maxima, absolute temperatures are different across reef regions. For example, corals in the Red Sea and Persian/Arabian Gulf have much higher bleaching thresholds than corals elsewhere (Coles, 2003; Coles and Riegl, 2013; Fine et al., 2013; Osman et al., 2018). Previous research has identified the symbiosis with a specialized alga, Cladocopium thermophilum (formerly: Symbiodinium thermophilum, Clade C3) to be a contributing factor for this remarkable thermotolerance (D’Angelo et al., 2015; Howells et al., 2016; Hume et al., 2016; Howells et al., 2020). Given that reef corals from the Red Sea and Persian/Arabian Gulf are also exposed to the highest levels of salinity in any of the world’s oceans (41 and 50 PSU, respectively; Ngugi et al., 2012; Riegl and Purkis, 2012), recent research has investigated whether high salinity could affect thermotolerance (D’Angelo et al., 2015; Gegner et al., 2017; Ochsenkühn et al., 2017). In particular, studies using the coral model Aiptasia showed that higher salinities can lead to reduced bleaching in the cnidarian-dinoflagellate symbiosis (Gegner et al., 2017, 2019). This salinity-conveyed thermotolerance could be further linked to an increase of the osmolyte floridoside that is produced by the associated algal endosymbiont, and putatively reduces the effects of bleaching-associated reactive oxygen species (ROS) due to its ROS-scavenging capabilities (Ochsenkühn et al., 2017; Gegner et al., 2019).
Despite the importance of algal endosymbionts, it is important to consider the coral as a holobiont, i.e., as a consortium of associated species (coral animal host, endosymbiont algae, bacteria, viruses, etc.; Rohwer et al., 2002; Bang et al., 2018). In particular, bacteria have been shown and suggested to play important roles in coral health, pathogen defense, metabolism, and nutrient cycling (Rosenberg et al., 2007; Krediet et al., 2013; Ainsworth et al., 2015; Rädecker et al., 2015; Bourne et al., 2016; Neave et al., 2017; Jaspers et al., 2019). Although the precise functional roles of specific bacteria are still largely unknown, association with beneficial bacteria is argued to be an avenue for corals to adapt to rapid environmental change (Mouchka et al., 2010; Bourne and Webster, 2013; Ainsworth and Gates, 2016; Peixoto et al., 2017; Torda et al., 2017). As such, microbiome restructuring has been suggested to play a role in the rapid acclimatization of Fungia granulosa after long-term exposure to high-salinity levels (Röthig et al., 2016b) and the adaptation of Acropora hyacinthus to increased thermal stress (Ziegler et al., 2017b). At large, microbiome flexibility, i.e., the potential for dynamic restructuring of the bacterial microbiome to support prevailing environmental conditions, is now put forward as a fast-track mechanism to support ecological adaption of the coral holobiont (Ziegler et al., 2019; Voolstra and Ziegler, 2020).
The promise of the microbiome to contribute to environmental adaptation prompted our interest to assess whether coral-associated bacteria may play a part in salinity-conveyed thermotolerance (Fine et al., 2013; Gegner et al., 2017; Ochsenkühn et al., 2017; Osman et al., 2018). To begin to address a possible contribution of bacteria to salinity-conveyed thermotolerance, we used the coral model Aiptasia (sensu Exaiptasia pallida; Weis et al., 2008; Baumgarten et al., 2015) and conducted a series of manipulative experiments. Using 16S rRNA gene amplicon sequencing, we assessed bacterial communities under control and heat stress conditions at three different salinity treatments in two Aiptasia strains (H2, CC7) associated with their two distinct native endosymbionts (SSB01, SSA01), drawing directly from a recently conducted experiment that showed salinity-conveyed thermotolerance at high salinities (Gegner et al., 2019). Aiptasia strains H2 and CC7 were shown recently to harbor distinct bacterial microbiomes, with CC7 exhibiting a more diverse microbiome and H2 a more variable one, besides many of the most prevalent taxa being distinct (Röthig et al., 2016a; Herrera et al., 2017). First, we determined overall changes in the bacterial community composition under each treatment, and subsequently, we identified individual bacterial taxa that contributed to the differences observed and their putative contribution.
Materials and Methods
Animal Rearing, Experimental Setup, and Sample Processing
Samples used in this study were taken from the experiment detailed in Gegner et al. (2019). Briefly, symbiotic anemones of the clonal Aiptasia strains H2 (Xiang et al., 2013) and CC7 (Sunagawa et al., 2009) were maintained in salinity-adjusted Red Sea seawater (see below) exposed to a 12 h: 12 h light/dark cycle at 30–40 μmol photons m–2 s–1. H2 anemones were associated with their native endosymbionts of strain SSB01 (type B1, species Breviolum minutum; Xiang et al., 2013; Baumgarten et al., 2015; LaJeunesse et al., 2018), referred to as H2-SSB01; CC7 anemones were associated with their native endosymbionts strain SSA01 (type A4, species Symbiodinium linucheae; Bieri et al., 2016; LaJeunesse et al., 2018), referred to as CC7-SSA01. Anemones were kept at 25°C in three experimental salinities: low (36 PSU), intermediate (39 PSU), and high (42 PSU) for 10 days to acclimate; the experimental salinity levels are well within the tolerance range of Aiptasia (Coles and Jokiel, 1992; Gegner et al., 2017, 2019). Feeding was ceased with the beginning of the acclimation. The experimental salinities were achieved by using autoclaved Red Sea seawater diluted with ddH20 to the lowest salinity (36 PSU) and subsequent adjustment with NaCl until the desired salinities were reached. Seawater samples were collected by filtering 1 L of seawater of each salinity through a 0.22 μm Durapore PVDF filter (Millipore, Billerica, United States). Filters were subsequently stored at −80°C.
After the acclimation phase, anemones were transferred into individual wells of 6-well plates (water volume of 7 ml at corresponding salinities) and wrapped in see-through plastic bags containing wet wipes to minimize evaporation (bags were not sealed to allow for aeration and oxygen levels were normal as determined from a pilot experiment). Salinities were monitored throughout the entire experiment using a refractometer (Aqua Medic GmbH, Germany). Half of the anemones were subjected to heat stress by ramping the temperature from 25°C to 34°C over the course of 9 h (1°C h–1 increments) and subsequently held at 34°C. A total of 52 anemones were used in this study as follows: H2-SSB01: 4–5 animals × 3 salinities × 2 temperatures = 28 anemones (for salinity 39 PSU, temperature 25°C and for salinity 42 PSU, temperature 34°C only 4 replicate anemones were available); CC7-SSA01: 4 animals × 3 salinities × 2 temperatures = 24 anemones. Wells of the 6-well plates were cleaned every second day with a cotton-swab and the water was exchanged. The experiment was completed for all host-symbiont pairings after 6 days. At this time point, the anemones of H2-SSB01 in the lowest salinity (36 PSU) appeared completely bleached, i.e., visually translucent. Upon collection, Aiptasia were rinsed twice with ddH20, briefly drained of excess water, transferred into 1.5 mL microtubes containing 150 μL PBS buffer, homogenized for 10 s using a MicroDisTec homogenizer 125 (Thermo Fisher Scientific, Waltham, MA, United States), and stored in −80°C until further processing. The experimental setup is depicted in Supplementary Figure 1.
DNA Extraction and 16S rRNA Gene Sequencing
For DNA extraction, each sample was thawed on ice, 400 μL AP1 buffer was added and extractions were performed using the DNeasy Plant Mini Kit (Qiagen, Hilden, Germany) according to the manufacturer’s instructions. For DNA isolation from seawater, one PVDF filter for each of the three salinities was divided into three parts using sterile razor blades and transferred into a 2 mL microtube. Next, 400 μL of AP1 buffer were added and incubated on a rotating wheel for 20 min. DNA was extracted following the manufacturer’s instructions (DNeasy Plant Mini Kit, Qiagen). For all samples, DNA concentrations were quantified on a NanoDrop 2000C spectrophotometer (Thermo Fisher Scientific, Waltham, MA, United States). To generate 16S rRNA gene amplicons for sequencing on the Illumina HiSeq2500 Platform, primers 784F [5′- TCGTCGGCAGCGTCAGATGTGTATAAGAGACAGAGGATT AGATACCCTGGTA -3′] and 1061R [5′- GTCTCGTGGGCT CGGAGATGTGTATAAGAGACAGCRRCACGAGCTGACGAC -3′] were used to amplify the variable region 5 and 6 of the 16S rRNA gene (Andersson et al., 2008; Bayer et al., 2013). Primers contained Illumina (San Diego, United States) adaptor overhangs (underlined above). For each sample, triplicate PCRs were performed using the Qiagen Multiplex PCR kit. The final reaction volume was 10 μL, comprised of 1 μL of template DNA (1–10 ng) and a final primer concentration of 0.5 μM. In addition, we performed three no template DNA negative controls in order to test for putative contaminants; notably, a potential source of contamination remains from the extraction procedure, which was not assessed here. PCR conditions were as follows: 95°C for 15 min, 30 cycles each at 95°C for 30 s, 55°C for 90 s, and 72°C for 30 s, followed by a final elongation step at 72°C for 10 min. Successful PCR amplification was checked via visualization of amplicon products in a 1% agarose gel (5 μL). Triplicate PCRs for each sample were pooled and subsequently cleaned with the ExoProStar 1-Step PCR, followed by the Sequence Reaction Clean-up Kit (GE Healthcare, Buckinghamshire, United Kingdom). Subsequently, the indexing PCR was performed over 8 cycles to add Nextera XT sequencing adapters (Illumina), according to the manufacturer’s instructions. Indexed PCR products were purified and normalized using the Invitrogen SequalPrep Normalization Plate (96) Kit (Thermo Fisher Scientific, Carlsbad, CA, United States), involving elution in 20 μl of buffer at normalized concentrations (∼4 nM), and subsequent pooling of samples in equimolar ratios. Sequencing libraries were quality checked on the BioAnalyzer (Agilent Technologies, Santa Clara, CA, United States) and sequenced on an Illumina HiSeq 2500 with 2 × 150 bp in rapid mode, loaded with 10% PhiX.
Data Analysis
The sequence dataset comprised ∼12 million read pairs that were demultiplexed according to barcodes using the bcl2fastq software (version 2.17.1.14, Illumina) resulting in ∼ 200,000 sequences per sample. For sequence data analysis the software mothur (version 1.39.5) was used (Schloss et al., 2009). Briefly, bases with quality scores < 20, ambiguities, or primer sequences were trimmed before merging paired reads. Next, sequences that occurred only once over all samples were discarded and remaining sequences were aligned against the SILVA database (release 128; Pruesse et al., 2007). A single-linkage pre-clustering step, allowing for a 2-bp difference between sequences (Huse et al., 2010) was performed to reduce potential sequencing errors. After that, we screened for chimeras using VSEARCH (Rognes et al., 2016) as implemented in mothur. Sequences were classified using the Greengenes (release gg_13_5_99) and SILVA 138 databases with a 60% bootstrap cutoff; sequences assigned to chloroplast, mitochondria, archaea, eukaryotes, or unknown were excluded (Pruesse et al., 2007; McDonald et al., 2012). Sequences were clustered into operational taxonomic units (OTUs) based on a 0.03 dissimilarity cutoff, after splitting the distance matrix by taxonomical annotation (order level) using mothur’s implementation of the OptiClust algorithm (Westcott and Schloss, 2017). Consensus sequences were calculated for the resulting 2,955 OTUs and a new taxonomical annotation was done as described above. The final dataset comprised ∼6 million sequences, equating to about 100,000 sequences per sample. A taxonomy bar chart at the family level was created in R (R Core Team, 2016) to provide visual assessment of bacterial community composition of anemones and seawater.
For each OTU, we calculated the ratio between the sum of the abundance in the three negative controls to the sum of the abundance in all other samples. OTUs with a ratio > 0.1 (corresponding to a 10% abundance of that sequence in negative control samples in comparison to biological samples) were considered putative contaminants and therefore removed (143 OTUs removed). The remaining 2,812 OTUs were used for the remainder of analyses (Supplementary Data 1). Alpha diversity measures, i.e., Chao1 (Chao, 1984), Inverse Simpson, and Simpson evenness (Simpson, 1949), were determined using the R package Vegan (Dixon, 2003). A non-metric multidimensional scaling (nMDS) plot based on Bray–Curtis dissimilarities of relative OTU abundances were generated using PRIMER v6 (Clarke and Gorley, 2006) to visualize differences between bacterial communities associated with seawater and Aiptasia host-symbiont pairings. Significant differences between bacterial communities associated with seawater and host-algal symbiont pairings were determined using PERMANOVA (Permutational Multivariate Analysis Of Variance; 2 levels: seawater, host-symbiont pairing). Differences in bacterial community composition across temperatures and salinities of host-symbiont pairings (H2-SSB01 and CC7-SSA01) were visualized in a Principle Coordinate Analysis (PCoA) based on a Bray–Curtis dissimilarity matrix (see above). To assess statistically significant differences in bacterial community composition, we conducted a two-factorial PERMANOVA for samples of each host-algal symbiont pairing using the factors temperature (2 levels: 25°C, 34°C), salinity (3 levels: 36 PSU, 39 PSU, 42 PSU), and possible interactions thereof (temperature × salinity), followed by pairwise testing of different salinities using PRIMER v6 (Clarke and Gorley, 2006).
To determine bacterial taxa that contribute to differences in community composition between salinities at ambient and heat stress temperatures for each host-algal symbiont pairing, we performed a similarity percentages (SIMPER) analysis based on a Bray–Curtis dissimilarity matrix of OTU abundances using the R package Vegan (Dixon, 2003). OTUs that contributed up to 50% of the cumulative differences across pairwise comparisons were visualized on heat maps for both temperatures and host-algal symbiont pairings (Supplementary Data 2). Empirical evaluation found that a 50% cutoff provided a good balance between the number of OTUs considered and their relative contribution (i.e., past the 50% threshold many OTUs are found with marginal contribution). For heat map display, OTU abundances were log(x + 1)-transformed and z-score normalization was applied for each OTU across salinities (i.e., rows). OTUs and salinity treatments were clustered by similarity for using Pearson correlation coefficient. This allowed us to identify main contributing OTUs that changed as a consequence of increasing salinity, irrespective of temperature, and thus may represent putative candidates to contribute to salinity-conveyed thermotolerance. The representative sequence of each of these OTUs (Supplementary Data 1) was used as a query in a BLASTn search against the NCBI nt database to elucidate whether identical or similar bacteria were identified in other studies and to get a glimpse at a potential associated function.
Results
Distinct Bacterial Community Compositions of Seawater and Aiptasia Anemones
H2-SSB01 and CC7-SSA01 exhibited differential bleaching susceptibility under heat stress at different salinities, as shown by Gegner et al. (2019) when considering retained algal endosymbionts and visual paling (Supplementary Figure 2). Whereas H2-SSB01 were notably bleached at the low salinity under heat stress (34°C), they retained more Symbiodiniaceae at intermediate and high salinities (Supplementary Figure 2). By comparison, CC7-SSA01 showed no changes in bleaching susceptibility across all three salinities under heat stress (34°C) and generally maintained high algal symbiont densities (Gegner et al., 2019).
The 16S rRNA gene sequencing of the bacterial communities associated with H2-SSB01 and CC7-SSA01 across temperatures and salinities revealed that bacterial community compositions of both host-algal symbiont pairings were significantly different from each other (PPERMANOVA = 0.001), and further, that both were significantly different from the surrounding seawater (PPERMANOVA = 0.001; Figure 1 and Table 1). The latter was further illustrated by a nMDS plot (Supplementary Figure 3), which showed that anemone and water bacterial communities were clearly distinct. At large, bacterial communities of seawater were much more even and we found an overall lower number of bacterial taxa in seawater (on average about 500 OTUs in Aiptasia and about 200 OTUs in seawater samples, Supplementary Table 1). Of note, bacterial communities of seawater were different across salinities, showing that the prevailing environments that the anemones are exposed to under changing salinities are distinct (Figure 1 and Supplementary Table 1). The differences in bacterial communities of seawater across salinities may directly be reflective of the differential preference for bacteria to grow under different salinities as shown for soil and coral communities recently (Röthig et al., 2016b; Zhang et al., 2019). In order to retain focus on our interest to explore differences across salinities and temperatures for a given host-algal symbiont pairing, we excluded seawater samples from subsequent analyses and considered the two hosts separately. H2-SSB01 and CC7-SSA01 were both associated to a similar extent with Rhodobacteraceae (on average 18.35% vs. 22.40%, respectively). In contrast, Flavobacteriaceae were more abundant in H2-SSB01 in comparison to CC7-SSA01 (on average 16.13% vs. 6.25%, respectively). Conversely, bacteria of the order Alteromonadales were comparatively rare in H2-SSB01 and more prevalent in CC7-SSA01 (on average, 4.07% vs. 19.78%).
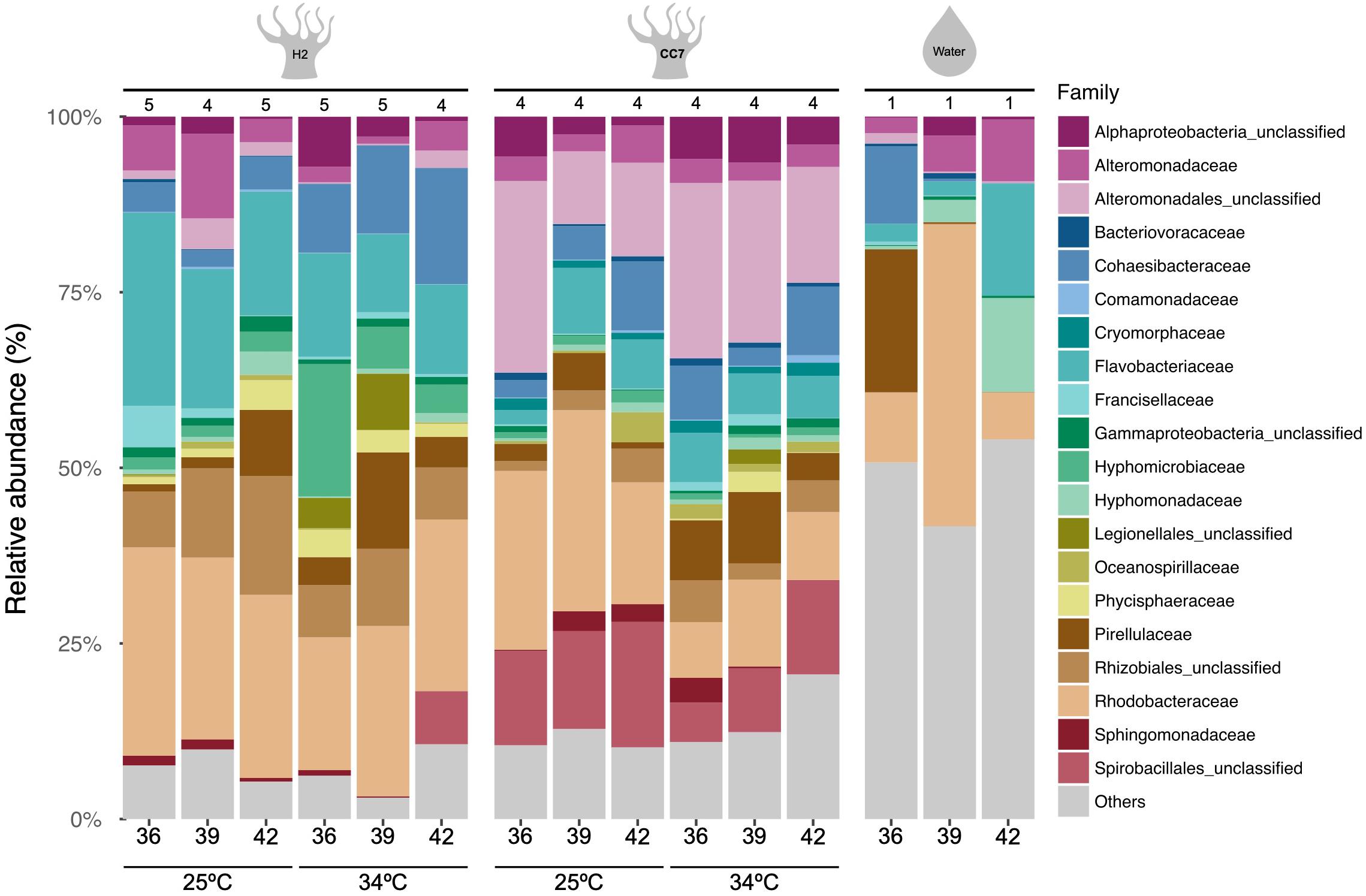
Figure 1. Taxonomy bar chart of bacterial families (Greengenes database, bootstrap ≥ 60) of Aiptasia host-algal symbiont pairings (H2-SSB01, CC7-SSA01) and seawater samples across temperatures and salinities. Each bar denotes the mean percentage of 16S rRNA gene sequences across replicates for each host and treatment (number of replicates are denoted above bars). Each color represents one of the twenty most abundant families across samples. The “Others” category groups the less abundant families.
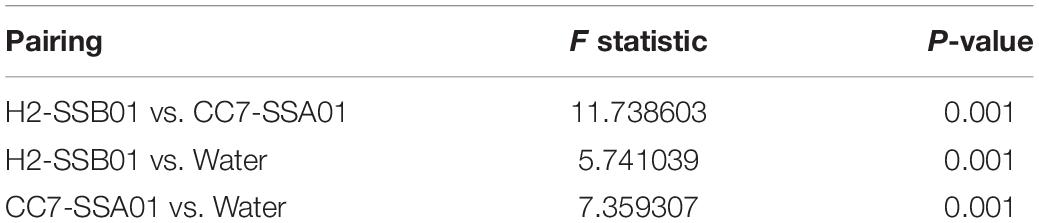
Table 1. Summary statistics of PERMANOVA based on Bray–Curtis dissimilarity of bacterial taxa abundances (OTUs) for seawater and host-algal symbiont pairings.
Distinct Bacterial Community Composition of H2-SSB01 and CC7-SSA01 Anemones at Ambient and Heat Stress Temperatures Across Different Salinities
Bacterial community differences of H2-SSB01 and CC7-SSA01 between the ambient temperature and heat stress across different salinities were assessed at the level of OTUs. Although we found no significant differences with regard to species richness and diversity (Chao1, Inverse Simpson, and Simpson’s evenness; Supplementary Table 1) for both host-algal symbiont pairings (PKruskal–Wallis > 0.05), we found highly significant differences in bacterial community composition for both host-algal symbiont pairings between temperatures and salinities (both factors PPERMANOVA = 0.001 for H2-SSB01 and PPERMANOVA = 0.002 for CC7-SSA01, respectively; Table 2 and Figure 2). Notably, we did not find a significant interaction effect of temperature and salinity for both host-algal symbiont pairings, suggesting that the effects of temperature and salinity are distinct (PPERMANOVA = 0.139 for H2-SSB01 and PPERMANOVA = 0.192 for CC7-SSA01; Table 2, Figure 2, and Supplementary Figure 4). To further explore differences between salinities, subsequent pair-wise testing revealed that bacterial communities at low and intermediate salinities were similar to each other, but significantly different from high salinity bacterial communities for both host-algal symbiont pairings (PPERMANOVA = 0.001 for H2-SSB01 and PPERMANOVA = 0.002 for CC7-SSA01, respectively; Supplementary Table 2).

Table 2. Summary statistics of two-factorial PERMANOVA based on Bray–Curtis dissimilarity of bacterial taxa abundances (OTUs) for both host-algal symbiont pairings.
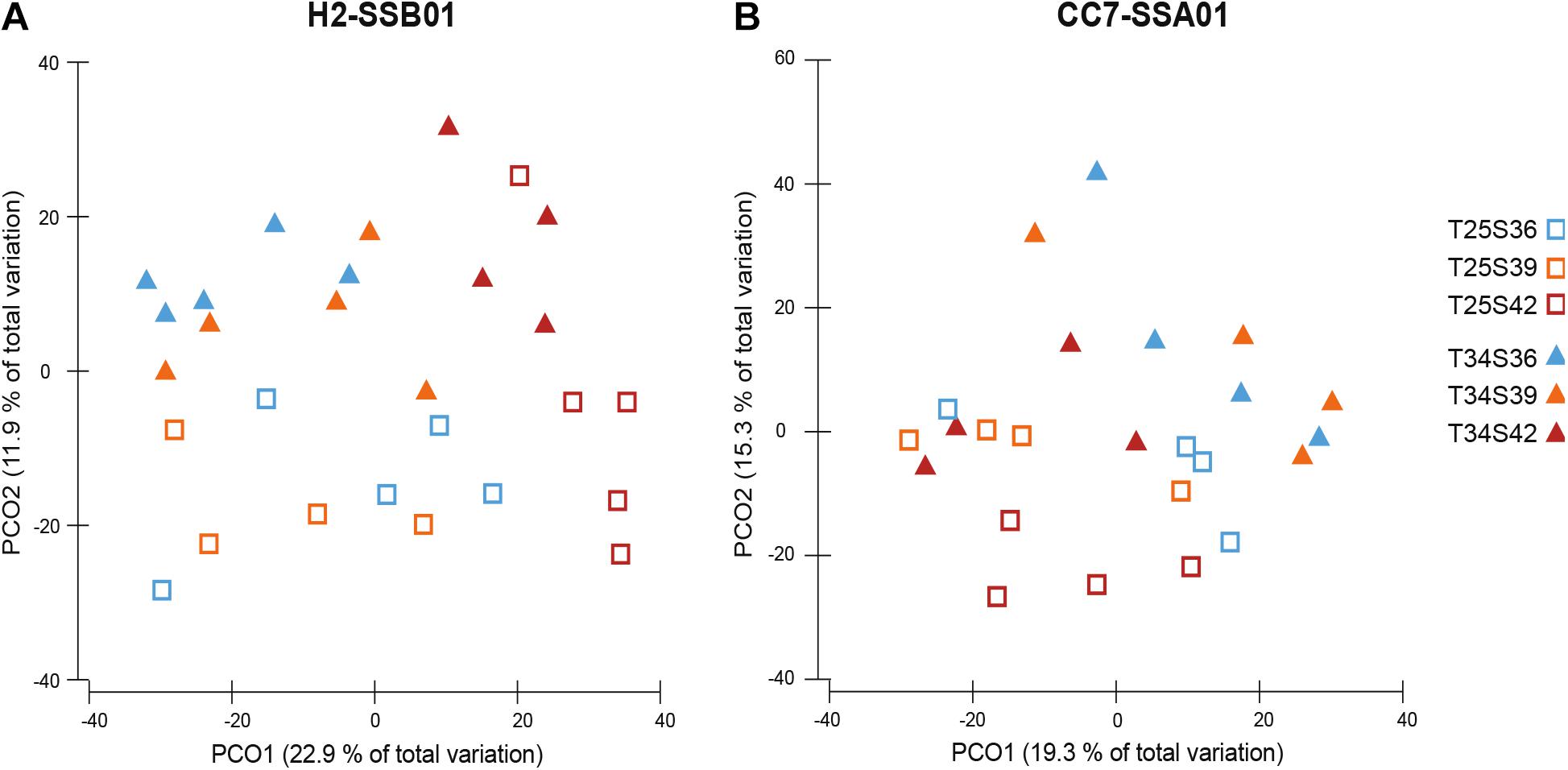
Figure 2. Principal coordinate analysis (PCoA) of Aiptasia host-algal symbiont pairings (A) H2-SSB01 and (B) CC7-SSA01 across temperatures and salinities based on Bray–Curtis dissimilarity of bacterial OTU abundances. Blue = low salinity (36 PSU), orange = intermediate salinity (39 PSU), red = high salinity (42 PSU), open squares = ambient temperature (25°C), and closed triangles = heat stress (34°C). Variation explained by the two coordinates are denoted by percentages on the axes.
Bacterial Taxa That Contribute to Differences Between Salinities as Candidates for Salinity-Conveyed Thermotolerance
To determine the bacterial taxa that contributed to observed differences in microbial community composition, we determined all OTUs that contributed up to 50% of the cumulative differences between salinities for a given temperature and host-symbiont combination using SIMPER (Figure 3 and Supplementary Data 2). For H2-SSB01, 50% of the cumulative differences across salinities were explained by 13 bacterial taxa at ambient temperature and under heat stress, respectively. These taxa belonged to the bacterial families Rhodobacteraceae (6 OTUs), Rhizobiaceae (2 OTUs), Pirellulaceae (2 OTUs), Flavobacteriaceae (2 OTUs), Stappiaceae (2 OTUs), Pseudoalteromonadaceae (1 OTU), Devosiaceae (1 OTU), Marinobacteraceae (1 OTU), and unclassified Gammaproteobacteria (1 OTU). Similarly, for CC7-SSA01 14 and 17 OTUs explained ≥50% of the cumulative differences across salinities at ambient temperature and under heat stress, respectively. These taxa belonged to the bacterial families Rhodobacteraceae (7 OTUs), Flavobacteriaceae (2 OTUs), Rhizobiaceae (2 OTUs), unclassified Oligoflexales (2 OTUs), Alteromonadaceae (1 OTU), Cryomorphaceae (1 OTU), Devosiaceae (1 OTU), Marinobacteraceae (1 OTU), Nannocystaceae (1 OTU), Nitrincolaceae (1 OTU), Pirellulaceae (1 OTU), Pseudoalteromonadaceae (1 OTU), unclassified Rhizobiales (1 OTU), Terasakiellaceae (1 OTU), and unclassified Gammaproteobacteria (1 OTU).
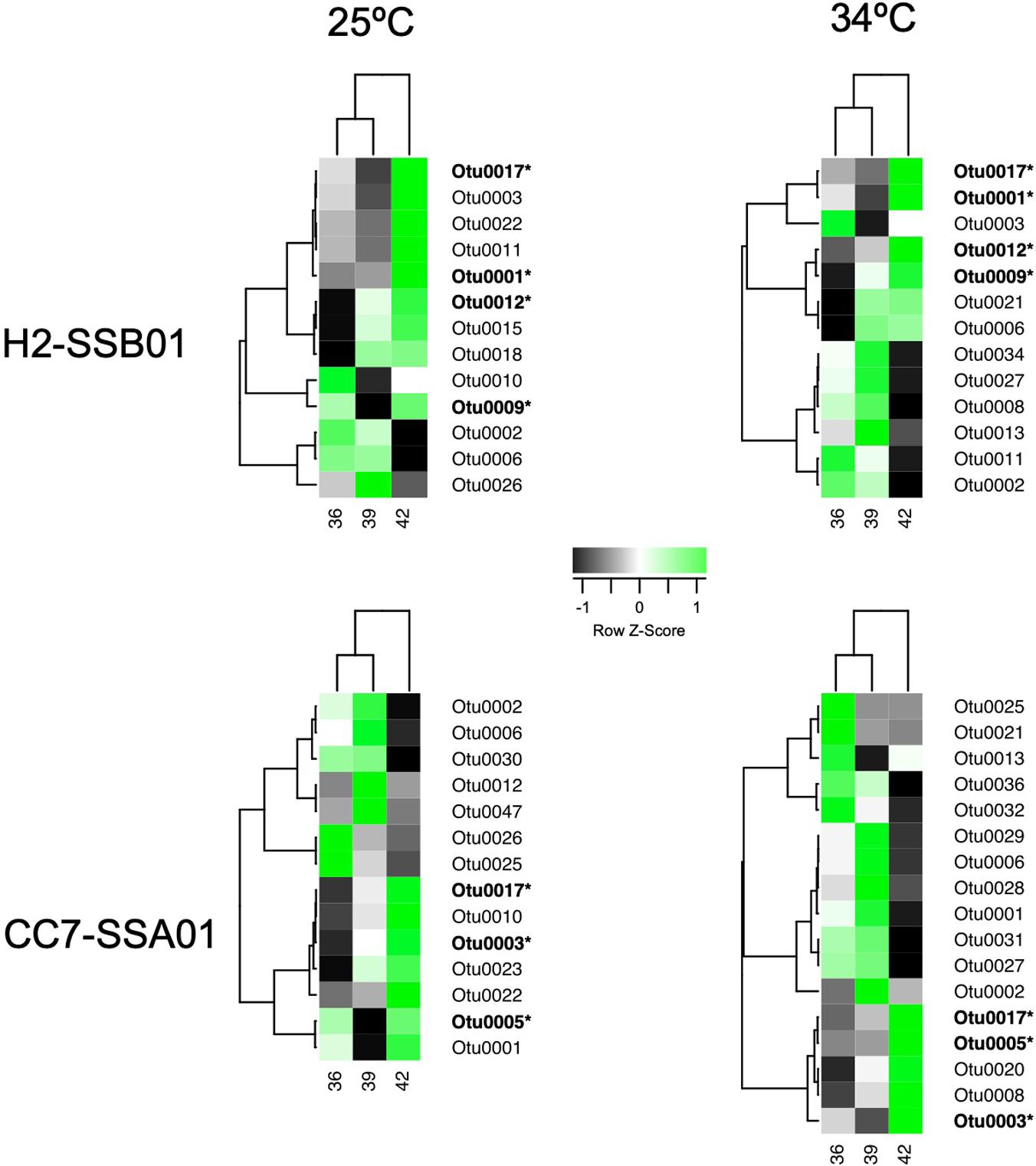
Figure 3. Heat map displaying differentially abundant bacterial taxa across salinities (36 PSU, 39 PSU, and 42 PSU) under ambient temperature (25°C) and heat stress (34°C) for Aiptasia host-algal symbiont pairings H2-SSB01 and CC7-SSA01 based on SIMPER analyses. Shown are bacterial taxa that contribute up to 50% of the cumulative differences between salinities. Color indicates Z-score normalized average abundance scaled by taxa. Taxa were clustered by similarity using Pearson correlation coefficient. OTUs in bold and with asterisk denote bacterial taxa that exhibited consistent relative high abundance at high salinity.
Only few taxa were particularly present at a specific salinity and temperature (e.g., OTU0026 was largely dominant at a salinity of 39 PSU at 25°C in H2-SSB01), and we found that the majority of OTUs were either particularly present at salinities of 36 and 39 PSU or at a salinity of 42 PSU (Figure 3). This allowed us to single out bacterial taxa that were dominant at high salinity, irrespective of temperature, for both host-symbiont pairings. Of note, due to the nature of the 16S amplicon sequencing employed, we can only assess relative abundance differences, although it would be highly interesting to assess differences in absolute bacterial abundance across different salinities. We reasoned that particular dominant taxa are potential candidates for a contribution to the observed salinity-conveyed thermotolerance, as their increased relative abundance was due to the high salinity, and not a consequence of heat stress. While all bacteria present under heat stress may convey a potential beneficial function to the holobiont (or not), they would not necessarily explain the salinity dependence. Thus, we focused our attention on bacteria that were prevalent under high salinity.
For H2-SSB01, four OTUs were prevalent at high salinity irrespective of temperature, i.e., OTU0001, OTU0009, OTU0012, and OTU0017 (Figure 3 and Table 3). OTU001 and OTU0009 belonged to the genus Algicola and Labrenzia, respectively. OTU0012 and OTU0017 were determined to belong to the genera Shimia and Aliiroseovarius, respectively. OTU0001, OTU0009, and OTU0012 were previously identified to be associated with cnidarians, in particular the coral model Aiptasia (OTU0001; Röthig et al., 2016a) and the corals Acropora hyacinthus (OTU009; Ziegler et al., 2017b) and Isopora palifera (OTU0012; Chen et al., 2011). Notably, bacteria in the genus Labrenzia (OTU0009) are associated with nitrate reduction and nitrate fixation (Table 3). For CC7-SSA01, we identified three OTUs that were prevalent at high salinity, i.e., OTU0003, OTU0005, and OTU0017 (Table 3). OTU0003 and OTU0005 belonged to the genus Cohaesibacter and the order Oligoflexales, respectively, and were previously found associated with Aiptasia (OTU0003; Röthig et al., 2016a) and the coral Montastrea faveolata (OTU0005). Notably, OTU0017 was found prevalent at high salinity in both host-symbiont pairings and has been recorded to be particularly tolerant toward high salinity (up to 8% NaCl, Table 3). In addition, OTU0017 is associated with degradation of DMSP and aromatic hydrocarbons, a function found highly enriched in bacteria associated with corals under long-term hypersalinity (Röthig et al., 2016b).
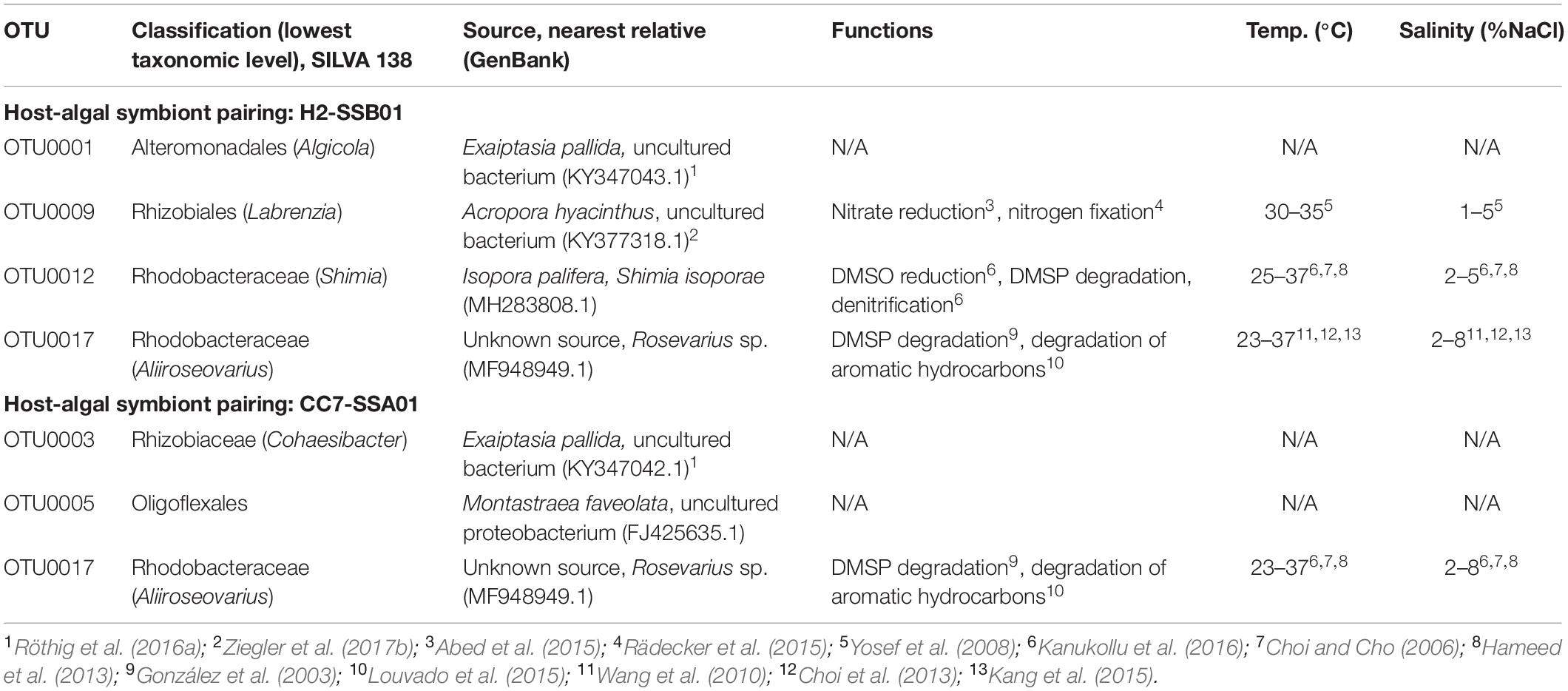
Table 3. Overview of prevalent bacterial taxa associated with Aiptasia anemones H2-SSB01 and CC7-SSA01 under high salinity.
Discussion
Bacteria associated with multicellular animals at large are becoming increasingly acknowledged for providing functions to their respective hosts, together comprising so-called metaorganisms or holobionts (Bosch and McFall-Ngai, 2011; McFall-Ngai et al., 2013; Jaspers et al., 2019; Voolstra and Ziegler, 2020). Microbes may be particularly important for organisms living in environmental extremes (Bang et al., 2018), and for relaying rapid adaptive responses to environmental change (Torda et al., 2017; Ziegler et al., 2017a,b; 2019; Voolstra and Ziegler, 2020). In this regard, the recent observation of salinity-conveyed thermotolerance in Aiptasia (Gegner et al., 2017) and the notion that osmoadaptation of the algal symbiont may be (one of) the driver(s) of this phenomenon (Ochsenkühn et al., 2017; Gegner et al., 2019), and more largely contribute to the extra-ordinary thermotolerance of corals from the Arabian Seas (Fine et al., 2013; D’Angelo et al., 2015; Hume et al., 2015; Osman et al., 2018, 2020), is yet another example of the putative importance of host-associated microbes. Here we wanted to assess whether changes in the microbiome associated with two host-symbiont pairings of the coral model Aiptasia under different salinities at ambient and heat stress temperatures support a putative contribution of bacteria to the observed salinity-conveyed thermotolerance. To our knowledge, this is the first study to assess changes in bacterial community composition of symbiotic cnidarians under heat stress at different salinities. Importantly, at current it is unknown whether bacterial community patterns and the response of the cnidarian host to different salinities are causally linked, or whether we are looking at a parallel response of the host and its associated bacterial assemblage.
Bacterial community composition of H2-SSB01 and CC7-SSA01 were distinct and markedly different from seawater (Figure 1, Table 1, and Supplementary Figures 3, 4), suggesting that both Aiptasia strains harbor a specific microbiome, as shown previously (Röthig et al., 2016a; Herrera et al., 2017). In addition to these overall differences, we found bacterial community composition to significantly differ between salinities and temperatures for both host-algal symbiont pairings (Table 2 and Figure 2). Notably, we did not identify a significant interaction effect of temperature and salinity for both host-algal symbiont pairings (Table 2), suggesting that bacterial taxa that contribute to differences between temperatures are distinct from those that contribute to differences between salinities. Further investigation revealed significant differences in the bacterial microbiome of H2-SSB01 and CC7-SSA01 under high salinity in comparison to low and intermediate salinities, which were not significantly different from each other (Supplementary Table 2). As such, it is tempting to speculate that functionally consequential bacterial community differences may manifest only at salinities past a certain threshold, e.g., >40 PSU, in line with the higher average salinities of the Red Sea and the Persian/Arabian Gulf (Osman et al., 2020).
To identify the bacterial taxa that underlied the observed differences in microbial community assemblage, we determined and considered those bacteria that contributed up to 50% of cumulative differences between salinities and temperatures (Figure 3 and Supplementary Data 2). Notably, the here-employed experimental setup allowed us to disentangle bacterial taxa that increased in relative abundance as a consequence of temperature and those that exhibited relative abundance differences due to salinity differences. An obvious short-coming of the collected data is that we cannot make any definitive statements about the increase or decrease of certain bacterial taxa, given that we assessed relative abundance (differences) of bacterial taxa across temperature/salinity conditions. Focusing on the bacterial taxa that increased in relative abundance at high salinity, and as such, might potentially contribute to the observed salinity-conveyed thermotolerance effect, we identified four and three OTUs for H2-SSB01 and CC7-SSA01, respectively (Table 3). First off, given the hundreds of observed bacterial taxa at each temperature and salinity (Supplementary Table 1), it is interesting to note that we only identified a handful of bacteria that increased (in relative terms) as a consequence of high salinity, and further, that one of these taxa was identical between H2-SSB01 and CC7-SSA01 (OTU0017). As such, if there is a contribution of the bacterial community to the observed salinity-conveyed thermotolerance, then it is likely restricted to few taxa, which makes experimental testing amenable. At the same time, we cannot exclude that rare bacterial taxa exist that were missed in our analysis but potentially contribute to the host phenotype. Nevertheless, functional testing, in particular in a system where the methods to strip off the native microbiome and subsequent introduction of distinct bacterial taxa is actively being developed is feasible and should be pursued (Costa et al., 2019). Second, the finding that OTU0017 is a candidate taxon for both host-symbiont pairings may argue that bacterial community dynamics denote a parallel response to changing salinities, rather than being host-specific.
It should be noted that differences in the microbiome that provide putative functional consequences may only become apparent in the case of H2-SSB01, which exhibits a salinity-conveyed thermotolerance at the experimental temperatures tested, but may be masked in CC7-SSA01 that displays an inherent higher thermotolerance across the evaluated temperature range (Gegner et al., 2017, 2019). Despite of this, two of the six bacterial taxa prevalent at high salinity belonged to the family Rhodobacteraceae (H2-SSB01: OTU0012 and OTU0017, CC7-SSA01: OTU0017). Bacteria of this family are commonly identified in corals, and either denote opportunistic pathogens due to their abundant association in diseased corals (Sunagawa et al., 2009; Godwin et al., 2012; Séré et al., 2013; Roder et al., 2014), or beneficial associates, as they are frequently identified in healthy corals (Morrow et al., 2012; Bayer et al., 2013; Li et al., 2014; Röthig et al., 2016b). Considering this, the presence of Rhodobacteraceae in both Aiptasia host-algal symbiont pairings may suggest functional importance to the holobiont under high salinities and/or during heat stress.
Comparison of the bacterial taxa that exhibited increased relative abundance at high salinity with previously recorded occurrences of the same or similar taxa revealed associated roles related to nitrogen and sulfur cycling. In H2-SSB01, one of the bacterial taxa identified (OTU0009) belonged to the order Rhizobiales. Rhizobiales play a putative role in the nitrogen cycle, as indicated by nitrite reduction in hypersaline microbial mats (Abed et al., 2015) and nitrogen fixation in corals (Rädecker et al., 2015). Of note, bacterial strains identified from this order are both mesophilic (optimum growth at 30–35°C) and halophilic (with optimal salinity ranges of 1–5% NaCl; Yosef et al., 2008). The two further bacterial taxa (OTU0012 and OTU0017) belonged to the family Rhodobacteraceae. OTU0012 belonged to the genus Shimia that has previously been shown to have functional roles in the sulfur cycle through producing DMS by DMSO reduction and DMSP degradation, and in the nitrogen cycle via the denitrification pathway (Kanukollu et al., 2016). OTU0017 from the genus Aliiroseovarius has been found to also play a role in DMSP degradation (González et al., 2003), in addition to the degradation of aromatic hydrocarbons (Louvado et al., 2015). More generally, Shimia and Aliiroseovarius taxa show tolerance and adaptation to high temperature (optimal growth at 25–37°C and 23–37°C, respectively) and rather extreme saline conditions (optimum 2–5% NaCl and 2–8% NaCl, respectively; Choi and Cho, 2006; Wang et al., 2010; Choi et al., 2013; Hameed et al., 2013; Kang et al., 2015; Kanukollu et al., 2016). In CC7-SSA01, functional annotation of similar taxa was available for only one of the three bacterial taxa (OTU0017). As detailed above, OTU0017 in the genus Aliiroseovarius was present in H2-SSB01 and CC7-SSA01.
Taken together, we find distinct bacterial taxa that increase in relative abundance under conditions of high salinity for both host-algal symbiont pairings (H2-SSB01 and CC7-SSA01). Closely related affiliates of these taxa, were previously shown to harbor functions related to the retention of nitrogen and provision of anti-oxidant capacity. In particular, the putative presence of DMSP pathways for two of the six bacterial taxa is in line with its role as an important osmolyte (Ngugi et al., 2020). As such, if these taxa were to have the ascribed functions, one could hypothesize that they are putatively beneficial and may contribute to an increase in thermotolerance. The observed salinity-conveyed thermotolerance is only apparent in H2-SSB01, and any beneficial effect for CC7-SSA01 may be masked by the innate higher thermotolerance of this host-symbiont pairing. At large, the notion that bacteria flexibly associate with host organisms and that the microbiome carries the potential for dynamic restructuring to assist metaorganism function was recently put forward in the microbiome flexibility hypothesis (Ziegler et al., 2019; Voolstra and Ziegler, 2020). Importantly, it does not require or imply sensu stricto selection by the host. Rather, the increased relative presence of certain bacteria as a response to changes in the prevailing environment may initially be detached from the host response, but those bacteria may nevertheless harbor functions beneficial to their host in the new environment. Importantly, hypotheses on specific bacterial associates as put forward here require rigorous testing and examination through means of microbiome manipulation (removal or provision of microbial associates) and subsequent assessment of metaorganism phenotypes, which are currently actively being developed for the coral model Aiptasia.
Data Availability Statement
Data determined in this study are available under NCBI BioProject PRJNA524291 (https://www.ncbi.nlm.nih.gov/bioproject/524291). OTU reference sequences for prevalent bacterial taxa associated with Aiptasia are available under GenBank Accession numbers MK571213-MK571247 for H2-SSB01 (www.ncbi.nlm.nih.gov/nuccore/?term=MK571213: MK571247[accn]) and MK571566-MK571601 for CC7-SSA01 (www.ncbi.nlm.nih.gov/nuccore/?term=MK571566: MK571601[accn]). Scripts used for data analysis, curation, and plotting are available at: github.com/reefgenomics/Aiptasia_ salinity_thermotolerance_16S.
Author Contributions
CV, HG, and MZ: conceived and designed the experiment. CV: contributed reagents, materials and tools. JR: generated data. AC, JR, and CV: analyzed data. CV, JR, AC, and MZ: wrote the manuscript. All authors contributed to the article and approved the submitted version.
Funding
This study was conducted as the Master Thesis of JR. This project was supported by research funding from King Abdullah University of Science and Technology to CV.
Conflict of Interest
The authors declare that the research was conducted in the absence of any commercial or financial relationships that could be construed as a potential conflict of interest.
Acknowledgments
JR would like to thank Dr. Caroline Dubé for help with 16S rRNA gene analysis. The authors would like to thank the Bioscience Core Lab (BCL) at King Abdullah University of Science and Technology for assistance with sequencing.
Supplementary Material
The Supplementary Material for this article can be found online at: https://www.frontiersin.org/articles/10.3389/fmars.2020.573635/full#supplementary-material
References
Abed, R. M. M., de Beer, D., and Stief, P. (2015). Functional-structural analysis of nitrogen-cycle bacteria in a hypersaline mat from the omani desert. Geomicrobiol. J. 32, 119–129. doi: 10.1080/01490451.2014.932033
Ainsworth, T. D., and Gates, R. D. (2016). Corals’ microbial sentinels. Science 352, 1518–1519. doi: 10.1126/science.aad9957
Ainsworth, T. D., Krause, L., Bridge, T., Torda, G., Raina, J. B., Zakrzewski, M., et al. (2015). The coral core microbiome identifies rare bacterial taxa as ubiquitous endosymbionts. ISME J. 9, 2261–2274. doi: 10.1038/ismej.2015.39
Andersson, A. F., Lindberg, M., Jakobsson, H., Backhed, F., Nyren, P., and Engstrand, L. (2008). Comparative analysis of human gut microbiota by barcoded pyrosequencing. PLoS One 3:2836. doi: 10.1371/journal.pone.0002836
Bang, C., Dagan, T., Deines, P., Dubilier, N., Duschl, W. J., Fraune, S., et al. (2018). Metaorganisms in extreme environments: do microbes play a role in organismal adaptation? Zoology. 127, 1–19. doi: 10.1016/j.zool.2018.02.004
Baumgarten, S., Simakov, O., Esherick, L. Y., Liew, Y. J., Lehnert, E. M., Michell, C. T., et al. (2015). The genome of Aiptasia, a sea anemone model for coral symbiosis. Proc. Natl. Acad. Sci. U.S.A. 112, 11893–11898. doi: 10.1073/pnas.1513318112
Bayer, T., Neave, M. J., Alsheikh-Hussain, A., Aranda, M., Yum, L. K., Mincer, T., et al. (2013). The microbiome of the red sea coral stylophora pistillata is dominated by tissue-associated endozoicomonas bacteria. Appl. Environ. Microbiol. 79, 4759–4762. doi: 10.1128/AEM.00695-13
Bellwood, D. R., Hughes, T. P., Folke, C., and Nyström, M. (2004). Confronting the coral reef crisis. Nature 429, 827–833. doi: 10.1038/nature02691
Bieri, T., Onishi, M., Xiang, T., Grossman, A. R., and Pringle, J. R. (2016). Relative contributions of various cellular mechanisms to loss of algae during cnidarian bleaching. PLoS One 11:e0152693. doi: 10.1371/journal.pone.0152693
Bosch, T. C. G., and McFall-Ngai, M. J. (2011). Metaorganisms as the new frontier. Zoology 114, 185–190. doi: 10.1016/j.zool.2011.04.001
Bourne, D. G., Morrow, K. M., and Webster, N. S. (2016). Insights into the Coral microbiome: underpinning the health and resilience of reef ecosystems. Annu. Rev. Microbiol. 70, 317–340. doi: 10.1146/annurev-micro-102215-095440
Bourne, D. G., and Webster, N. S. (2013). “Coral reef bacterial communities,” in The Prokaryotes, eds E. Rosenberg, E. F. DeLong, S. Lory, E. Stackebrandt, and F. Thompson (Berlin: Springer), 163–187. doi: 10.1007/978-3-642-30123-0
Chao, A. (1984). Nonparametric estimation of the number of classes in a population. Scand. J. Stat. 11, 265–270.
Chen, M.-H., Sheu, S.-Y., Chen, C. A., Wang, J.-T., and Chen, W.-M. (2011). Shimia isoporae sp. nov., isolated from the reef- building coral Isopora palifera. Int. J. Syst. Evol. Microbiol. 61, 823–827. doi: 10.1099/ijs.0.022848-0
Choi, D. H., and Cho, B. C. (2006). Shimia marina gen. nov., sp. nov., a novel bacterium of the Roseobacter clade isolated from biofilm in a coastal fish farm. Int. J. Syst. Evol. Microbiol. 56, 1869–1873. doi: 10.1099/ijs.0.64235-0
Choi, E. J., Lee, H. J., Kim, J. M., and Jeon, C. O. (2013). Roseovarius lutimaris sp. nov., isolated from a marine tidal flat. Int. J. Syst. Evol. Microbiol. 63, 3835–3840. doi: 10.1099/ijs.0.050807-0
Coles, S. L. (2003). Coral species diversity and environmental factors in the arabian gulf and the gulf of oman: a comparison to the Indo-Pacific region. Atoll Res. Bull. 507, 1–19. doi: 10.5479/si.00775630.507.1
Coles, S. L., and Jokiel, P. L. (1992). “Effects of salinity on coral reefs,” in Pollution in Tropical Aquatic Systems, eds D. W. Connell and D. W. Hawker (Florida: CRC Press), 147–166. doi: 10.1201/9781351075879-6
Coles, S. L., and Riegl, B. M. (2013). Thermal tolerances of reef corals in the Gulf: a review of the potential for increasing coral survival and adaptation to climate change through assisted translocation. Mar. Pollut. Bull. 72, 323–332. doi: 10.1016/j.marpolbul.2012.09.006
Costa, R. M., Cardneas, A., and Voolstra, C. R. (2019). Protocol for Bacterial Depletion of Aiptasia anemones - Towards the Generation of Gnotobiotic/Germ-Free Cnidarian Host Animals. Berkeley, CA: Protocols.io, doi: 10.17504/protocols.io.7mrhk56
Costanza, R., de Groot, R., Sutton, P., van der Ploeg, S., Anderson, S. J., Kubiszewski, I., et al. (2014). Changes in the global value of ecosystem services. Glob. Environ. Chang. 26, 152–158. doi: 10.1016/j.gloenvcha.2014.04.002
D’Angelo, C., Hume, B. C. C., Burt, J., Smith, E. G., Achterberg, E. P., and Wiedenmann, J. (2015). Local adaptation constrains the distribution potential of heat-tolerant Symbiodinium from the Persian/Arabian Gulf. ISME J. 9, 2551–2560. doi: 10.1038/ismej.2015.80
Dixon, P. (2003). VEGAN, a package of R functions for community ecology. J. Veg. Sci. 14, 927–930. doi: 10.1111/j.1654-1103.2003.tb02228.x
Dove, G., and Hoegh-Guldberg, O. (2006). “Coral bleaching can be caused by stress. the cell physiology of coral bleaching,” in Coral Reefs and Climate change: Science and Management, eds J. T. Phinney, O. Hoegh-Guldberg, J. Kleypas, W. Skirving, and A. Strong (Washington, DC: American Geophysical Union), 1–18. doi: 10.1029/61CE05
Fine, M., Gildor, H., and Genin, A. (2013). A coral reef refuge in the Red Sea. Glob. Chang. Biol. 19, 3640–3647. doi: 10.1111/gcb.12356
Gegner, H. M., Rädecker, N., Ochsenkühn, M., Barreto, M. M., Ziegler, M., Reichert, J., et al. (2019). High levels of floridoside at high salinity link osmoadaptation with bleaching susceptibility in the cnidarian-algal endosymbiosis. Biol. Open 8, 1–7. doi: 10.1242/bio.045591
Gegner, H. M., Ziegler, M., Rädecker, N., Buitrago-López, C., Aranda, M., and Voolstra, C. R. (2017). High salinity conveys thermotolerance in the coral model Aiptasia. Biol. Open 6, 1943–1948. doi: 10.1242/bio.028878
Godwin, S., Bent, E., Borneman, J., and Pereg, L. (2012). The role of coral-associated bacterial communities in australian subtropical white syndrome of Turbinaria mesenterina. PLoS One 7:e0044243. doi: 10.1371/journal.pone.0044243
González, J. M., Covert, J. S., Whitman, W. B., Henriksen, J. R., Mayer, F., Scharf, B., et al. (2003). Silicibacter pomeroyi sp. nov. and Roseovarius nubinhibens sp. nov., dimethylsulfoniopropionate-demethylating bacteria from marine environments. Int. J. Syst. Evol. Microbiol. 53, 1261–1269. doi: 10.1099/ijs.0.02491-0
Hameed, A., Shahina, M., Lin, S. Y., Lai, W. A., Hsu, Y. H., Liu, Y. C., et al. (2013). Shimia biformata sp. nov., isolated from surface seawater, and emended description of the genus Shimia Choi and Cho, 2006. Int. J. Syst. Evol. Microbiol. 63, 4533–4539. doi: 10.1099/ijs.0.053553-0
Herrera, M., Ziegler, M., Voolstra, C. R., and Aranda, M. (2017). Laboratory-cultured strains of the sea anemone exaiptasia reveal distinct bacterial communities. Front. Mar. Sci. 4:115. doi: 10.3389/fmars.2017.00115
Howells, E. J., Abrego, D., Meyer, E., Kirk, N. L., and Burt, J. A. (2016). Host adaptation and unexpected symbiont partners enable reef-building corals to tolerate extreme temperatures. Glob. Chang. Biol. 22, 2702–2714. doi: 10.1111/gcb.13250
Howells, E. J., Bauman, A. G., Vaughan, G. O., Hume, B. C. C., Voolstra, C. R., and Burt, J. A. (2020). Corals in the hottest reefs in the world exhibit symbiont fidelity not flexibility. Mol. Ecol. 29, 899–911. doi: 10.1111/mec.15372
Hughes, T. P., Kerry, J. T., Álvarez-Noriega, M., Álvarez-Romero, J. G., Anderson, K. D., Baird, A. H., et al. (2017). Global warming and recurrent mass bleaching of corals. Nature 543, 373–377. doi: 10.1038/nature21707
Hume, B. C. C., D’Angelo, C., Smith, E. G., Stevens, J. R., Burt, J., and Wiedenmann, J. (2015). Symbiodinium thermophilum sp. nov., a thermotolerant symbiotic alga prevalent in corals of the world’s hottest sea, the Persian/Arabian Gulf. Sci. Rep. 5, 1–8. doi: 10.1038/srep08562
Hume, B. C. C., Voolstra, C. R., Arif, C., D’Angelo, C., Burt, J. A., Eyal, G., et al. (2016). Ancestral genetic diversity associated with the rapid spread of stress-tolerant coral symbionts in response to Holocene climate change. Proc. Natl. Acad. Sci. U.S.A. 113, 4416–4421. doi: 10.1073/pnas.1601910113
Huse, S. M., Welch, D. M., Morrison, H. G., and Sogin, M. L. (2010). Ironing out the wrinkles in the rare biosphere through improved OTU clustering. Environ. Microbiol. 12, 1889–1898. doi: 10.1111/j.1462-2920.2010.02193.x
Jaspers, A. C., Fraune, S., Elizabeth, A., Miller, D. J., Bosch, T., and Voolstra, C. R. (2019). Resolving structure and function of metaorganisms through a holistic framework combining reductionist and integrative approaches. Zoology 133, 81–87. doi: 10.1016/j.zool.2019.02.007
Kang, H., Kim, J. H., Jeon, C. O., Yoon, J. H., and Kim, W. (2015). Roseovarius aquimarinus sp. nov a slightly halophilic bacterium isolated from seawater. Int. J. Syst. Evol. Microbiol. 65, 4514–4520. doi: 10.1099/ijsem.0.000604
Kanukollu, S., Voget, S., Pohlner, M., Vandieken, V., Petersen, J., Kyrpides, N. C., et al. (2016). Genome sequence of Shimia str. SK013, a representative of the Roseobacter group isolated from marine sediment. Stand. Genomic Sci. 11:25. doi: 10.1186/s40793-016-0143-0
Knowlton, N., Brainard, R. E., Fisher, R., Moews, M., Plaisance, L., and Caley, M. J. (2010). “Coral reef biodiversity,” in Life World’s Ocean. Divers. Distrib. Abundance, ed. A. McIntyre (Hoboken, NJ: John Wiley & Sons), 65–78. doi: 10.1002/9781444325508.ch4
Krediet, C. J., Ritchie, K. B., Paul, V. J., and Teplitski, M. (2013). Coral-associated micro-organisms and their roles in promoting coral health and thwarting diseases. Proc. R. Soc. B Biol. Sci. 280:20122328. doi: 10.1098/rspb.2012.2328
LaJeunesse, T. C., Parkinson, J. E., Gabrielson, P. W., Jeong, H. J., Reimer, J. D., Voolstra, C. R., et al. (2018). Systematic revision of symbiodiniaceae highlights the antiquity and diversity of coral endosymbionts. Curr. Biol. 28, 2570.e6–2580.e6. doi: 10.1016/j.cub.2018.07.008
Li, J., Chen, Q., Long, L. J., Dong, J., Yang, J. D., and Zhang, S. (2014). Bacterial dynamics within the mucus, tissue and skeleton of the coral Porites lutea during different seasons. Sci. Rep. 4, 1–8. doi: 10.1038/srep07320
Louvado, A., Gomes, N. C. M., Simões, M. M. Q., Almeida, A., Cleary, D. F. R., and Cunha, A. (2015). Polycyclic aromatic hydrocarbons in deep sea sediments: microbe-pollutant interactions in a remote environment. Sci. Total Environ. 526, 312–328. doi: 10.1016/j.scitotenv.2015.04.048
McDonald, D., Price, M. N., Goodrich, J., Nawrocki, E. P., Desantis, T. Z., Probst, A., et al. (2012). An improved Greengenes taxonomy with explicit ranks for ecological and evolutionary analyses of bacteria and archaea. ISME J. 6, 610–618. doi: 10.1038/ismej.2011.139
McFall-Ngai, M., Hadfield, M. G., Bosch, T. C. G., Carey, H. V., Domazet-Lošo, T., Douglas, A. E., et al. (2013). Animals in a bacterial world, a new imperative for the life sciences. Proc. Natl. Acad. Sci. U.S.A. 110, 3229–3236. doi: 10.1073/pnas.1218525110
Morrow, K. M., Moss, A. G., Chadwick, N. E., and Liles, M. R. (2012). Bacterial associates of two caribbean coral species reveal species-specific distribution and geographic variability. Appl. Environ. Microbiol. 78, 6438–6449. doi: 10.1128/AEM.01162-12
Mouchka, M. E., Hewson, I., and Harvell, C. D. (2010). Coral-associated bacterial assemblages: current knowledge and the potential for climate-driven impacts. Integr. Comp. Biol. 50, 662–674. doi: 10.1093/icb/icq061
Neave, M. J., Michell, C. T., Apprill, A., and Voolstra, C. R. (2017). Endozoicomonas genomes reveal functional adaptation and plasticity in bacterial strains symbiotically associated with diverse marine hosts. Sci. Rep. 7:40579. doi: 10.1038/srep40579
Ngugi, D. K., Antunes, A., Brune, A., and Stingl, U. (2012). Biogeography of pelagic bacterioplankton across an antagonistic temperature-salinity gradient in the Red Sea. Mol. Ecol. 21, 388–405. doi: 10.1111/j.1365-294X.2011.05378.x
Ngugi, D. K., Ziegler, M., Duarte, C. M., and Voolstra, C. R. (2020). Genomic blueprint of glycine betaine metabolism in coral metaorganisms and their contribution to reef nitrogen budgets. iScience 23:101120. doi: 10.1016/j.isci.2020.101120
Ochsenkühn, M. A., Röthig, T., Angelo, C. D., Wiedenmann, J., and Voolstra, C. R. (2017). The role of floridoside in osmoadaptation of coral-associated algal endosymbionts to high-salinity conditions. Sci. Adv. 3:e1602047. doi: 10.1126/sciadv.1602047
Osman, E. O., Smith, D. J., Ziegler, M., Kürten, B., Conrad, C., El-Haddad, K. M., et al. (2018). Thermal refugia against coral bleaching throughout the northern Red Sea. Glob. Chang. Biol. 24, e474–e484. doi: 10.1111/gcb.13895
Osman, E. O., Suggett, D. J., Voolstra, C. R., Pettay, D. T., Clark, D. R., Pogoreutz, C., et al. (2020). Coral microbiome composition along the northern Red Sea suggests high plasticity of bacterial and specificity of endosymbiotic dinoflagellate communities. Microbiome 8, 1–16. doi: 10.1186/s40168-019-0776-5
Peixoto, R. S., Rosado, P. M., de Leite, D. C. A., Rosado, A. S., and Bourne, D. G. (2017). Beneficial microorganisms for corals (BMC): proposed mechanisms for coral health and resilience. Front. Microbiol. 8:341. doi: 10.3389/fmicb.2017.00341
Pruesse, E., Quast, C., Knittel, K., Fuchs, B. M., Ludwig, W., Peplies, J., et al. (2007). SILVA: a comprehensive online resource for quality checked and aligned ribosomal RNA sequence data compatible with ARB. Nucleic Acids Res. 35, 7188–7196. doi: 10.1093/nar/gkm864
Rädecker, N., Pogoreutz, C., Voolstra, C. R., Wiedenmann, J., and Wild, C. (2015). Nitrogen cycling in corals: the key to understanding holobiont functioning? Trends Microbiol. 23, 490–497. doi: 10.1016/j.tim.2015.03.008
Roder, C., Arif, C., Bayer, T., Aranda, M., Daniels, C., Shibl, A., et al. (2014). Bacterial profiling of white plague disease in a comparative coral species framework. ISME J. 8, 31–39. doi: 10.1038/ismej.2013.127
Rognes, T., Flouri, T., Nichols, B., Quince, C., and Mahé, F. (2016). VSEARCH: a versatile open source tool for metagenomics. PeerJ 4:e2584. doi: 10.7717/peerj.2584
Rohwer, F., Seguritan, V., Azam, F., and Knowlton, N. (2002). Diversity and distribution of coral-associated bacteria. Mar. Ecol. Prog. Ser. 243, 1–10. doi: 10.3354/meps243001
Rosenberg, E., Koren, O., Reshef, L., Efrony, R., and Zilber-Rosenberg, I. (2007). The role of microorganisms in coral health, disease and evolution. Nat. Rev. Microbiol. 5, 355–362. doi: 10.1038/nrmicro1635
Röthig, T., Costa, R. M., Simona, F., Baumgarten, S., Torres, A. F., Radhakrishnan, A., et al. (2016a). Distinct bacterial communities associated with the coral model Aiptasia in aposymbiotic and symbiotic States with Symbiodinium. Front. Mar. Sci. 3:234. doi: 10.3389/fmars.2016.00234
Röthig, T., Ochsenkühn, M. A., Roik, A., Van Der Merwe, R., and Voolstra, C. R. (2016b). Long-term salinity tolerance is accompanied by major restructuring of the coral bacterial microbiome. Mol. Ecol. 25, 1308–1323. doi: 10.1111/mec.13567
Schloss, P. D., Westcott, S. L., Ryabin, T., Hall, J. R., Hartmann, M., Hollister, E. B., et al. (2009). Introducing mothur: open-source, platform-independent, community-supported software for describing and comparing microbial communities. Appl. Environ. Microbiol. 75, 7537–7541. doi: 10.1128/AEM.01541-09
Séré, M. G., Tortosa, P., Chabanet, P., Turquet, J., Quod, J. P., and Schleyer, M. H. (2013). Bacterial communities associated with Porites white patch syndrome (PWPS) on three western Indian Ocean (WIO) coral reefs. PLoS One 8:e0083746. doi: 10.1371/journal.pone.0083746
Sunagawa, S., Desantis, T. Z., Piceno, Y. M., Brodie, E. L., Desalvo, M. K., Voolstra, C. R., et al. (2009). Bacterial diversity and white Plague disease-associated community changes in the caribbean coral montastraea faveolata. ISME J. 3, 512–521. doi: 10.1038/ismej.2008.131
Torda, G., Donelson, J. M., Aranda, M., Barshis, D. J., Bay, L., Berumen, M. L., et al. (2017). Rapid adaptive responses to climate change in corals. Nat. Clim. Chang. 7, 627–636. doi: 10.1038/nclimate3374
Voolstra, C. R., and Ziegler, M. (2020). Adapting with microbial help: microbiome flexibility facilitates rapid responses to environmental change. BioEssays 42:2000004. doi: 10.1002/bies.202000004
Wang, B., Sun, F., Lai, Q., Du, Y., Liu, X., Li, G., et al. (2010). Roseovarius nanhaiticus sp. nov., a member of the Roseobacter clade isolated from marine sediment. Int. J. Syst. Evol. Microbiol. 60, 1289–1295. doi: 10.1099/ijs.0.012930-0
Weis, V. M., Davy, S. K., Hoegh-Guldberg, O., Rodriguez-Lanetty, M., and Pringle, J. R. (2008). Cell biology in model systems as the key to understanding corals. Trends Ecol. Evol. 23, 369–376. doi: 10.1016/j.tree.2008.03.004
Westcott, S. L., and Schloss, P. D. (2017). OptiClust, an improved method for assigning amplicon-based sequence data to operational taxonomic units. mSphere 2:e00073-17. doi: 10.1128/mSphereDirect.00073-17
Xiang, T., Hambleton, E. A., Denofrio, J. C., Pringle, J. R., and Grossman, A. R. (2013). Isolation of clonal axenic strains of the symbiotic dinoflagellate Symbiodinium and their growth and host specificity1. J. Phycol. 49, 447–458. doi: 10.1111/jpy.12055
Yosef, D. Z., Ben-Dov, E., and Kushmaro, A. (2008). Amorphus coralli gen. nov., sp. nov., a marine bacterium isolated from coral mucus, belonging to the order Rhizobiales. Int. J. Syst. Evol. Microbiol. 58, 2704–2709. doi: 10.1099/ijs.0.65462-0
Zhang, K., Shi, Y., Cui, X., Yue, P., Li, K., Liu, X., et al. (2019). Salinity is a key determinant for soil microbial communities in a desert ecosystem. mSystems 4:e00225-18. doi: 10.1128/mSystems.00225-18
Ziegler, M., Arif, C., Burt, J. A., Dobretsov, S., Roder, C., LaJeunesse, T. C., et al. (2017a). Biogeography and molecular diversity of coral symbionts in the genus Symbiodinium around the Arabian Peninsula. J. Biogeogr. 44, 674–686. doi: 10.1111/jbi.12913
Ziegler, M., Seneca, F. O., Yum, L. K., Palumbi, S. R., and Voolstra, C. R. (2017b). Bacterial community dynamics are linked to patterns of coral heat tolerance. Nat. Commun. 8:14213. doi: 10.1038/ncomms14213
Keywords: bacterial community, heat stress, salinity, holobiont, metaorganism, thermotolerance, coral reef, coral bleaching
Citation: Randle JL, Cárdenas A, Gegner HM, Ziegler M and Voolstra CR (2020) Salinity-Conveyed Thermotolerance in the Coral Model Aiptasia Is Accompanied by Distinct Changes of the Bacterial Microbiome. Front. Mar. Sci. 7:573635. doi: 10.3389/fmars.2020.573635
Received: 17 June 2020; Accepted: 21 October 2020;
Published: 25 November 2020.
Edited by:
Sebastian Fraune, Heinrich Heine University of Düsseldorf, GermanyReviewed by:
Adam Michael Reitzel, University of North Carolina at Charlotte, United StatesPeter Deines, University of Kiel, Germany
Copyright © 2020 Randle, Cárdenas, Gegner, Ziegler and Voolstra. This is an open-access article distributed under the terms of the Creative Commons Attribution License (CC BY). The use, distribution or reproduction in other forums is permitted, provided the original author(s) and the copyright owner(s) are credited and that the original publication in this journal is cited, in accordance with accepted academic practice. No use, distribution or reproduction is permitted which does not comply with these terms.
*Correspondence: Christian R. Voolstra, Y2hyaXN0aWFuLnZvb2xzdHJhQHVuaS1rb25zdGFuei5kZQ==; Y2hyaXMudm9vbHN0cmFAZ21haWwuY29t
†These authors have contributed equally to this work