- 1Department of Life Sciences, The Natural History Museum, London, United Kingdom
- 2Pharmacognosy, Department Pharmaceutical Biosciences, Uppsala University, Uppsala, Sweden
- 3Analytical Methods-Bioimaging Facility, Royal Botanic Gardens, Kew, Richmond, United Kingdom
- 4Department of Biological Sciences, University of Bergen, Bergen, Norway
- 5Departamento de Biodiversidad y Biología Evolutiva, Museo Nacional de Ciencias Naturales, Consejo Superior de Investigaciones Científicas, Museo Nacional de Ciencias Naturales Calle de José Gutiérrez Abascal, Madrid, Spain
Boreo-arctic sponge grounds are essential deep-sea structural habitats that provide important services for the ecosystem. These large sponge aggregations are dominated by demosponges of the genus Geodia (order Tetractinellida, family Geodiidae). However, little is known about the basic biological features of these species, such as their life cycle and dispersal capabilities. Here, we surveyed five deep-sea species of Geodia from the North-Atlantic Ocean and studied their reproductive cycle and strategy using light and electron microscopy. The five species were oviparous and gonochoristic. Synchronous development was observed at individual and population level in most of the species. Oocytes had diameters ranging from 8 μm in previtellogenic stage to 103 μm in vitellogenic stage. At vitellogenic stages, oocytes had high content of lipid yolk entirely acquired by autosynthesis, with no participation of nurse cells. Intense vertical transmission of bacterial symbionts to the oocytes by phagocytosis through pseudopodia was observed, especially in late stages of oogenesis. The density of oocytes within the sponge tissue was on average 10 oocytes/mm2 across all species, higher than that of most temperate and tropical oviparous species studied elsewhere. Spermatic cysts were widespread over the tissue during early stages, or fused in larger cysts, around the canals in later stages, and occupying between 1.5 and 12% of the tissue in males. The reproductive season spanned similar periods for all Geodia spp.: from late spring to early autumn. During the reproductive peak of each species, between 60 and 90% of the population was engaged in reproduction for most species. Given the present hazards that threaten the boreo-arctic tetractinellid sponge grounds, it becomes crucial to understand the processes behind the maintenance and regeneration of populations of keystone deep-sea species in order to predict the magnitude of human impacts and estimate their ability to recover. The information provided in this study will be useful for developing adequate conservation strategies for these vulnerable deep-sea habitats.
Introduction
Deep-sea sponge grounds are marine habitats first described by Klitgaard et al. (1997) in the Faroe Island shelf and slope areas, and later shown to be widespread across the North-Atlantic Ocean (Klitgaard and Tendal, 2004; Murillo et al., 2012; Beazley et al., 2015). These habitats are formed by three-dimensional structures created by the sponges, which provide refuge for other animals, sometimes of high economic value, and enhance biodiversity (e.g., Klitgaard, 1995; Beazley et al., 2013, 2015; Kenchington et al., 2013). Furthermore, because of their high pumping activity (Kutti et al., 2015) and consumption of organic matter (Leys et al., 2018), sponges play a major role in nutrient cycling (Maldonado et al., 2012), specifically in carbon turnover (Pile and Young, 2006; Yahel et al., 2007; Cathalot et al., 2015; Kahn et al., 2015), nitrogen flow (Hoffmann et al., 2009; Rooks et al., 2020) and the silicon cycle (Maldonado et al., 2020). Indeed, recently, studies point to serious impacts on ecosystem function when sponges are removed from the deep sea due to fishing bycatch (Pham et al., 2019).
Despite their importance at functional and ecological level (Kutti et al., 2013; Maldonado et al., 2016), deep-sea sponge grounds have been highly exposed to commercial bottom trawling (e.g., Freese, 2001; Roberts, 2002; Pham et al., 2019). In addition, sediment disturbance caused by equipment deployment at the seafloor from various anthropogenic activities, can lead to the clogging of their aquiferous system and eventually to their death (Kutti et al., 2015). For these reasons, deep-sea sponge grounds in the North Atlantic are now considered as vulnerable marine ecosystems by the OSPAR convention for the protection of the marine environment of the North-East Atlantic (OSPAR, 2008), needing protection according to United Nations General Assembly Resolution 61/105 and Food and Agriculture Organization (UNGA, 2006; FAO, 2009). Up to now, most areas with dense biomass have been protected from bottom trawling in North-West Atlantic by the North-West Atlantic Fisheries Organization (NAFO, 2012, 2014), but there are still areas of deep-sea sponge grounds with high levels of exposure (Thompson and Fuller, 2020). Nowadays, a huge effort is conducted to better understand sponge ground ecosystems’ services, to estimate their overall importance, and evaluate the consequences of adverse impacts on these habitats (ICES, 2018; NAFO, 2018).
However, few ecophysiological studies have been conducted on deep-sea sponge species (e.g., Strand et al., 2017) and only two studies regarding their reproductive timing have been published so far. One study focused on the species Thenea abyssorum Koltun, 1959 (Koltun, 1959) (order Tetractinellida and suborder Astrophorina), Tentorium semisuberites (Schmidt, 1870), and Radiella sol Schmidt, 1870 (Schmidt, 1870), previously called Trichostemma sol (order Polymastiida) from the Barents Sea (Witte, 1996), which had been described among the most abundant demosponges in abyssal Greenland, Iceland, and Norwegian seas by Barthel and Tendal (1993). The other one focused on the species Geodia barretti Bowerbank, 1858 (Bowerbank, 1858; Spetland et al., 2007), which is very abundant in temperate and boreo-arctic grounds of the North-Atlantic Ocean (Klitgaard and Tendal, 2004; Cárdenas et al., 2013). In both publications, researchers found that the studied species were gonochoristic and oviparous with one or two reproductive cycles per year which were dependant on primary production blooms.
Porifera is one of the earliest diverging clades in the phylogeny of the Metazoa (Dohrmann and Wörheide, 2013; Feuda et al., 2017; Zumberge et al., 2018; Laumer et al., 2019). But despite their early origin, sexual reproduction in sponges is not only morphologically similar to that in other Metazoa, but also the molecular toolkit that regulates the gametogenesis is conserved from Porifera to Chordata (Koutsouveli et al., 2020b). Despite the conserved process of reproduction through evolution, even within the phylum Porifera, the reproductive strategy greatly varies among sponge groups in the following traits: e.g., oviparity/viviparity, gonochorism/hermaphrotidism, larval types, number of reproductive cycles per year, and seasonality. These features are mainly constrained by phylogeny (Riesgo et al., 2014), but there is also a high degree of plasticity correlated to the abiotic conditions of their environment. In this sense, sponges lack sensory organs, however, they are able to respond to a variety of abiotic stimuli. One of the main driving factors in latitudes with high seasonal variations is temperature. Many studies reported a correlation of the temperature increase with sex determination, gametogenesis, or induction of spawning, while others have found a triggering of reproductive period after a thermal drop (Chen, 1976; Fromont and Bergquist, 1994; Corriero et al., 1998; Ereskovsky, 2000; Riesgo and Maldonado, 2008). In habitats where temperature is constant all year round, different stimuli have been proposed to play a role, such as photoperiod, wave fluctuation, rainfall, and salinity variations (e.g., Elvin, 1976; Corriero et al., 1998; Calazans and Lanna, 2019). In the deep sea, the reproductive season seems to be dependent on other environmental stimuli such as the primary productivity blooms that occur once or twice over the year (Young and Eckelbarger, 1994; Witte, 1996).
A crucial biological feature for the ecological success of sponges is the larval type and its dispersal potential, which is directly related to the connectivity patterns of these populations and therefore their resilience. All sponge larvae are lecithotrophic since they do not have any digestive tract to process food. So, the major source of energy during larval dispersal is the yolk previously accumulated during gametogenesis and embryogenesis (Maldonado and Bergquist, 2002), with only few exceptions in which larvae were also observed to phagocytize some nutrients from their environment (Ivanova and Semyonov, 1997). Consequently, the dispersal capabilities of sponge propagules are tightly linked to the quality and quantity of the yolk they acquire even before reaching the larva stage. The origin and type of yolk in the female gametes depends on the reproductive strategy of the species (Riesgo et al., 2015): the yolk can (i) be produced by the oocyte itself, through the Golgi apparatus and the rough endoplasmic reticulum (Fell, 1974; Simpson, 1984), (ii) be acquired by direct phagocytosis from the mesohyl (Fell, 1974; Simpson, 1984), (iii) be provided by nurse cells in the surroundings of the oocytes (Fell, 1974; Simpson, 1984), and (iv) be the product of bacterial phagocytosis by the oocyte (Riesgo and Maldonado, 2009). The yolk in sponges is mainly composed of lipid, protein, and glycogen (Fell, 1969, 1974; Simpson, 1984). The amount and the type of yolk in a given oocyte/embryo can give clues on the duration of the gametogenesis/embryogenesis, and the dispersal time of the released propagules. This knowledge has profound ecological implications for the survival and possible recovery of the populations in case of disturbance, and to a greater extent, of the species in the studied habitat.
Demosponges of the genus Geodia (order Tetractinellida, suborder Astrophorina, family Geodiidae) often dominate in terms of abundance and biomass the boreo-arctic sponge grounds (Klitgaard and Tendal, 2004; Cárdenas et al., 2013). A total of six species of Geodia form two types of astrophorin sponge grounds, (1) the boreal sponge grounds (Faroe Islands, Norway, Sweden, western Barents Sea and south of Iceland), which are mainly composed of Geodia phlegraei (Sollas, 1880), Geodia macandrewii Bowerbank, 1858 (Bowerbank, 1858), Geodia barretti Bowerbank, 1858 (Bowerbank, 1858) and Geodia atlantica (Stephens, 1915) and (2) the arctic sponge grounds (north of Iceland, in most of the Denmark Strait, off East Greenland and north of Spitzbergen) which mainly consist of Geodia hentscheli Cárdenas et al., 2010 and Geodia parva Hansen, 1885 (Hansen, 1885; Klitgaard and Tendal, 2004; Cárdenas et al., 2010, 2013; Murillo et al., 2012; Knudby et al., 2013). The reproduction of only three species of Geodiidae has been studied to date – Geodia cydonium, G. barretti, and Erylus discophorus – which are all gonochoristic and oviparous (Liaci and Sciscioli, 1969, 1970; Mercurio et al., 2007; Spetland et al., 2007). While G. cydonium, and E. discophorus are temperate species, G. barretti is the only deep-sea sponge from northern latitudes. Asexual reproduction (budding) has also been reported in the two arctic species, G. parva and G. hentscheli (Cárdenas et al., 2013). The study of Spetland et al. (2007) mainly focused on describing the reproductive cycle of G. barretti, however without providing detailed observations about its reproductive features at the histological/cytological level. All in all, there is very little information about the reproductive biology of Geodia species.
In this study, fresh material of five out of the six boreo-arctic North-Atlantic Geodia species was collected between 2011 and 2019 in the North-East Atlantic (boreal: G. phlegraei, G. macandrewii, G. barretti, G. atlantica; arctic: G. hentscheli). The main goal was to study the seasonality and the strategy of gametogenesis of these five species through histological and cytological observations. Knowledge on seasonality and reproductive strategies is crucial for developing appropriate conservation strategies for boreo-arctic sponge grounds; a deeper and wider understanding of the reproduction of key sponge ground species could inform us on the population structure and their dispersion pattern, and the capacity of the population to survive and regenerate in case of disturbance.
Materials and Methods
Sample Collection
One hundred and twenty eight specimens were collected from five Geodia species: G. hentscheli, G. phlegraei, G. barretti, G. macandrewii, and G. atlantica during expeditions in the boreo-arctic and North-East Atlantic from the beginning of April to the end of September mainly during the years 2016 and 2017 (Figure 1, Table 1, and Supplementary Table S1). Samples from Hordaland coast, were collected by Agassiz trawl on board the “K. Bonnevie” research vessel (RV), those from the Kosterfjord were collected using an ROV during the expedition of RV “Nereus,” and samples from Korsfjord were collected by triangular dredge on board the RV “Hans Brattstrøm.” In addition, samples from the Sula reef, Schulz Bank, and Tromsøflaket (western Barents Sea), were collected either by Agassiz trawl, or ROV (AEGIR 6000) on board of the RV “G.O. Sars” (Table 1 and Supplementary Table S1). Finally, samples from Rosemary Bank Seamount were collected by Agassiz trawl on board of MRV “Scotia.” All collection details and metadata associated were archived in the PANGAEA data repository1 (Koutsouveli et al., 2020a). In all expeditions, three cubes of sponge tissue from each specimen were collected (∼5 mm3 each) and directly fixed in 2.5% glutaraldehyde solution in 0.4M PBS and 0.34M NaCl and stored at 4°C, until their further processing in the laboratory. The identification of all species was performed based on spicule analyses. Regarding the specimens collected by trawl/dredge, we subsampled tissue only from entire or almost entire collected specimens and not from small pieces of sponges in order to avoid to sample tissue from the same specimen several times.
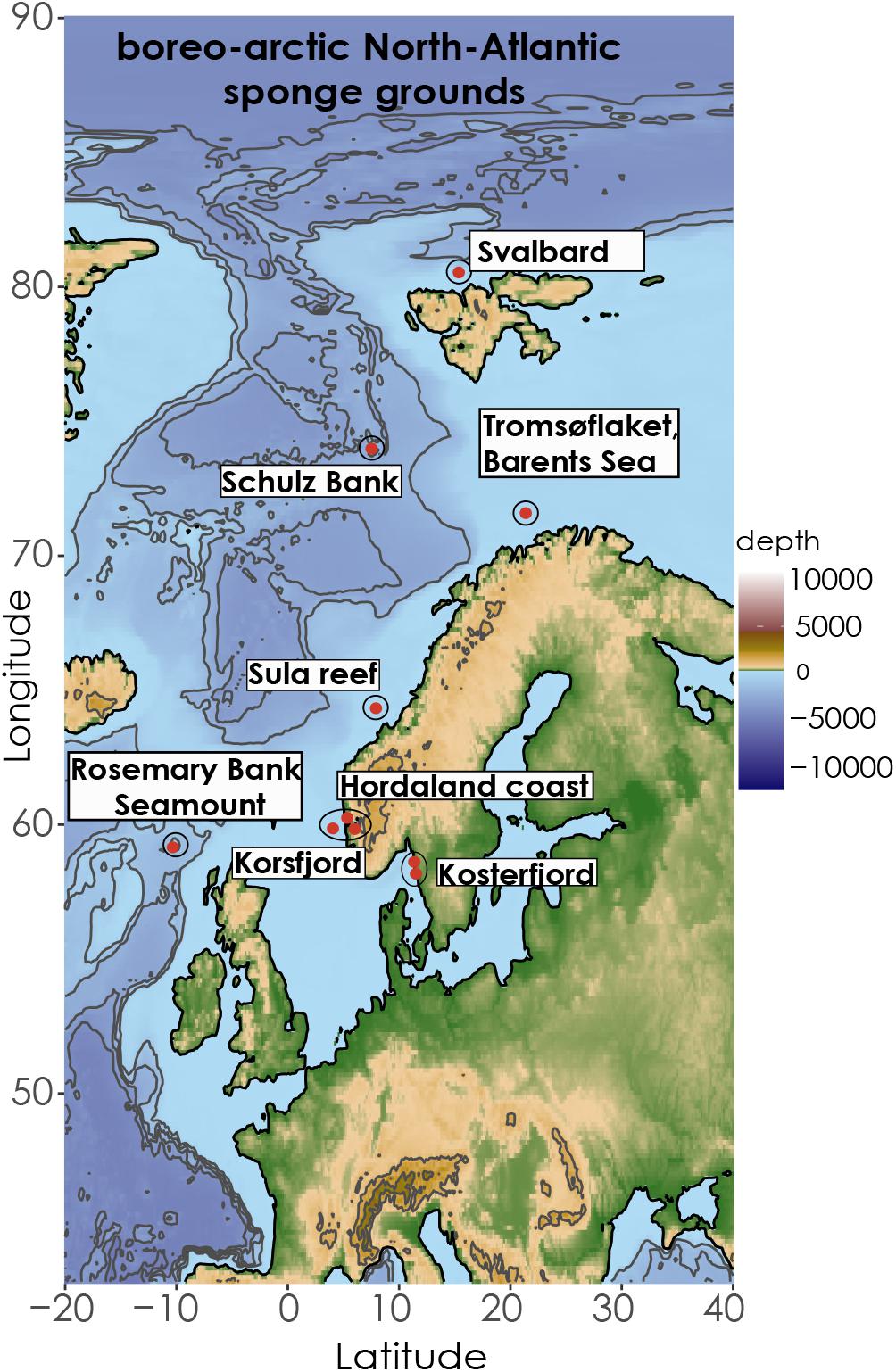
Figure 1. Map of North Atlantic indicating the coordinates of the different sampling areas of the study. The Map was retrieved from the ETOPO1 database from NOAA server (Amante and Eakins, 2009) and illustrated in R.
Sample Processing and Histological Sectioning
For further histological processing, samples were then desilicified by immersing the sponge tissue in 5% hydrofluoric acid overnight and then rinsed profusely with distilled water. After that, the tissue was either processed for light microscopy or Transmission Electron Microscopy (TEM). For light microscopy, tissues were dehydrated through an increasing ethanol series and then embedded in paraffin (60°C, melting point) overnight after a brief bath in xylene. For TEM, sponge tissues were postfixed in 2% osmium tetroxide in 0.4M PBS for 1 h at 4°C and then rinsed in distilled water. Similarly, an increasing ethanol series was used to dehydrate the tissue, and then it was embedded in LRW resin (preparation according to guidelines of the manufacturer).
The protocol for the preparation of the samples was the same as mentioned in Koutsouveli et al. (2020b). Briefly, for light microscopy preparations, thick sections (5 μm) were obtained with an HM 325 rotary microtome (ThermoFisher Scientific) and stained with a standard Harris’ Hematoxylin and Eosin (HandE) and Methylene Blue. For TEM, ultrathin sections (approximately 70 nm) were obtained with an Ultracut ultramicrotome (Reichert-Jung) and then stained with an uranyl acetate/lead citrate staining protocol (Reynolds, 1963). Light microscopy preparations were observed with an Olympus microscope (BX43) with a UC50 camera at the Natural History Museum’s imaging facilities, while ultrathin sections on gold coated grids were observed with a Hitachi Transmission Electron Microscope (H-7650) at 80 kV at the imaging facilities in Kew Botanical Gardens.
Measurements on Histological Sections
Several parameters were recorded to understand the life cycle dynamics of all five species of Geodia, (i) size, density, and fecundity estimations of reproductive features (oocytes and spermatic cysts), (ii) bacteria and yolk abundance within the oocytes, (iii) length of the reproductive period (considering the timing of appearance/disappearance of gametes), and (iv) the percentage of the population engaged in reproduction. In the following sections, specimens with gametes in their tissues were referred as reproductive while specimens without any gamete observed in their tissue sections were called non-reproductive.
Size and Density of Reproductive Features
The measurements of size and density of the reproductive features within the sponge tissue and for the fecundity estimations were obtained from light microscopy images with the Olympus microimaging software CellSens standard, connected to the microscope. Measurements of yolk and bacterial content in the ooplasm were acquired from TEM images with ImageJ (Schneider et al., 2012) on the TEM images.
The size of oocytes and spermatic cysts and density of oocytes were calculated in three different areas (0.58 mm2 each area) of tissue per specimen at 10x magnification. Size and density of spermatic cells could not be obtained, since that would require their individual measurement and counting, but they were so densely packed within the spermatic cysts that they could not be visually separated and counted. As thus, only the size of spermatic cysts was reported here.
Fecundity Estimations
While female fecundity was estimated based on the total number of oocytes produced per sponge, male fecundity estimations were based on the percentage of the sponge area occupied by spermatic cysts. For the female fecundity estimations, firstly the number of oocytes/mm3 was extrapolated with the modified Elvin formula (Elvin, 1976), E = N(t/d + t)/v, as used by Di Camillo et al. (2012), where N is the number of the oocytes in a microscopic section; t, the thickness of the section; d, the diameter of the oocytes and v, is the volume of the histological section. Secondly, the area and the volume of each species were calculated. It was considered that almost all the studied sponge species (except for G. atlantica) had a spherical shape. The average diameter per species (Supplementary Table S2) was based on dimensions previously published (Cárdenas et al., 2013) or observed (personal observations, HTR) for these species, and then the area of the sponge was calculated according to the formula 4×π×r2 and the volume of the sponge according to the formula, ×π×r3. Regarding G. atlantica, its shape was considered more similar to a hollow cone, then using the dimensions (70 cm diameter × 40 cm height) mentioned in Cárdenas et al. (2013) for the maximum volume and using 3 cm as average thickness for the tissue of the sponge, the area of a hollow cone: π×r×s was calculated, where s, is the slant height, and the volume for a hollow cone (outside volume - inside volume; where the volume for the cone is π×r2×h/3) (Supplementary Table S2). We should report here that although the diameters of Geodia spp. might be different in other publications (i.e., Klitgaard and Tendal, 2004; Cárdenas et al., 2013; Kutti et al., 2013) than the mean diameter used here, we mainly used specimens coming from the Arctic region for this analysis and so we extracted the information mainly based on photos of the specimens and personal observations (HTR). Only the dimensions of G. atlantica were retrieved by already published data (Cárdenas et al., 2013) as we never collected a whole specimen for this species to estimate dimensions. Consequently, the fecundity rates for the species of this study are a rough estimation and they should be used with caution.
For the fecundity estimations and the oocyte content (bacterial symbionts and yolk content), individuals with the most mature oocytes (maximum diameter of Vi oocytes) were used for G. phlegraei (Sula reef, July), G. atlantica (Korsfjord, September), and G. hentscheli (Schulz Bank, August) while individuals with mature oocytes, but not those with the most mature oocytes, were used for G. barretti (Barents sea, August) and G. macandrewii (Sula reef, July) because only those specimens had been processed for TEM, having a more complete information. Regarding the male individuals, only some of the G. phlegraei and G. atlantica individuals were in the latest developmental stages, while specimens of G. macandrewii, G. barretti, and G. hentscheli were in less advanced but similar developmental stages. For each species, the individuals with the most mature SP_II spermatic cysts (i.e., largest average size) were chosen to estimate male fecundity.
Results
Histological Features of Geodia Species
General Description of the Mesohyl of Geodia spp.
Several common sponge cell types, described previously in sponges, were observed in the mesohyl of all species, including pinacocytes lining the periphery of the canals and collencytes producing collagen in the mesohyl space (Supplementary Figures S1A-B). In addition, archeocytes (average size of 5–7 μm) (Supplementary Figures S1B-D) and choanocytes (Supplementary Figures S1B-D), forming small choanocyte chambers (average 6–10 μm), were observed in the mesohyl (Supplementary Figures S1C,D). G. barretti and G. atlantica had the highest abundance of spherulous cells in their mesohyl, 15–20 μm in the largest diameter (Supplementary Figures S1E-G); G. phlegraei had spherulous cells of similar size (∼20 μm) but in lower numbers, while G. macandrewii and G. hentscheli completely lacked spherulous cells. The spherules in the spherulous cells were all electron-dense, but slightly different in size and shape (Supplementary Figures S1E-G). All the Geodia spp. were High Microbial Abundance (HMA) sponges (Supplementary Figures S1E-H). Phagocytosis of bacteria from different types of sponge cells, including archeocytes (Supplementary Figure S1A) and choanocytes (Supplementary Figure S1C), was observed within the mesohyl of G. barretti.
Reproductive Features of Geodia spp.
All species of Geodia were gonochoristic; not a single specimen of Geodia spp. had both female and male reproductive features at the same time. In all species, individuals at the beginning of oogenesis with small previtellogenic (PV) oocytes, and individuals with more developed, vitellogenic (Vi) oocytes were found (Supplementary Figure S2A), although sometimes these two stages were found in the same individual (e.g., G. atlantica, Korsfjord June). Similarly, both male individuals with spermatic stage I (SP_I) cysts and more mature, spermatic stage II (SP_II) cysts were identified (Supplementary Figure S2B). In all Geodia spp., the diameter of PV oocytes measured on average 19 ± 6 μm and that of SP_I spermatic cysts was 30 ± 6 μm (Supplementary Figure S2). At a later stage, Vi oocytes had an average diameter of 54 ± 14 μm and SP_II spermatic cysts around 60 ± 25 μm (Supplementary Figure S2). G. hentscheli had the smallest size of both female and male reproductive features among the Geodia species (Supplementary Figure S2), when comparing individuals with either early or relatively late developmental stages, while G. atlantica had the largest oocytes and mature spermatic cysts found among the species studied here (Supplementary Figure S2).
Oogenesis
Oocyte development in most species was synchronous at both individual and population levels. All species showed very similar cytological features which are described together in the following sections. At the beginning of oogenesis (Figure 2), ameboid PV oocytes (8-25 μm in max. diameter) (Supplementary Figure S2A) with a well distinct nucleus and nucleolus were widespread throughout the mesohyl of the sponges (Figures 2A,B). A thin layer of collagen started to form around the PV oocytes (Figures 2C-E). PV oocytes were full of electron light vesicles, with few lipid droplets observed in the ooplasm of the oocytes (Figures 2D-G). While many bacterial symbionts were present in the mesohyl outside the oocyte (Figure 2D), only very few were observed within the oocyte at this stage (Figures 2E-H). Phagocytosis of bacteria was achieved with pseudopodia (Figures 2D,H). Both the Golgi apparatus and the nuage (among mitochondrial clouds) could be observed at this stage in most species (Figures 2E,F).
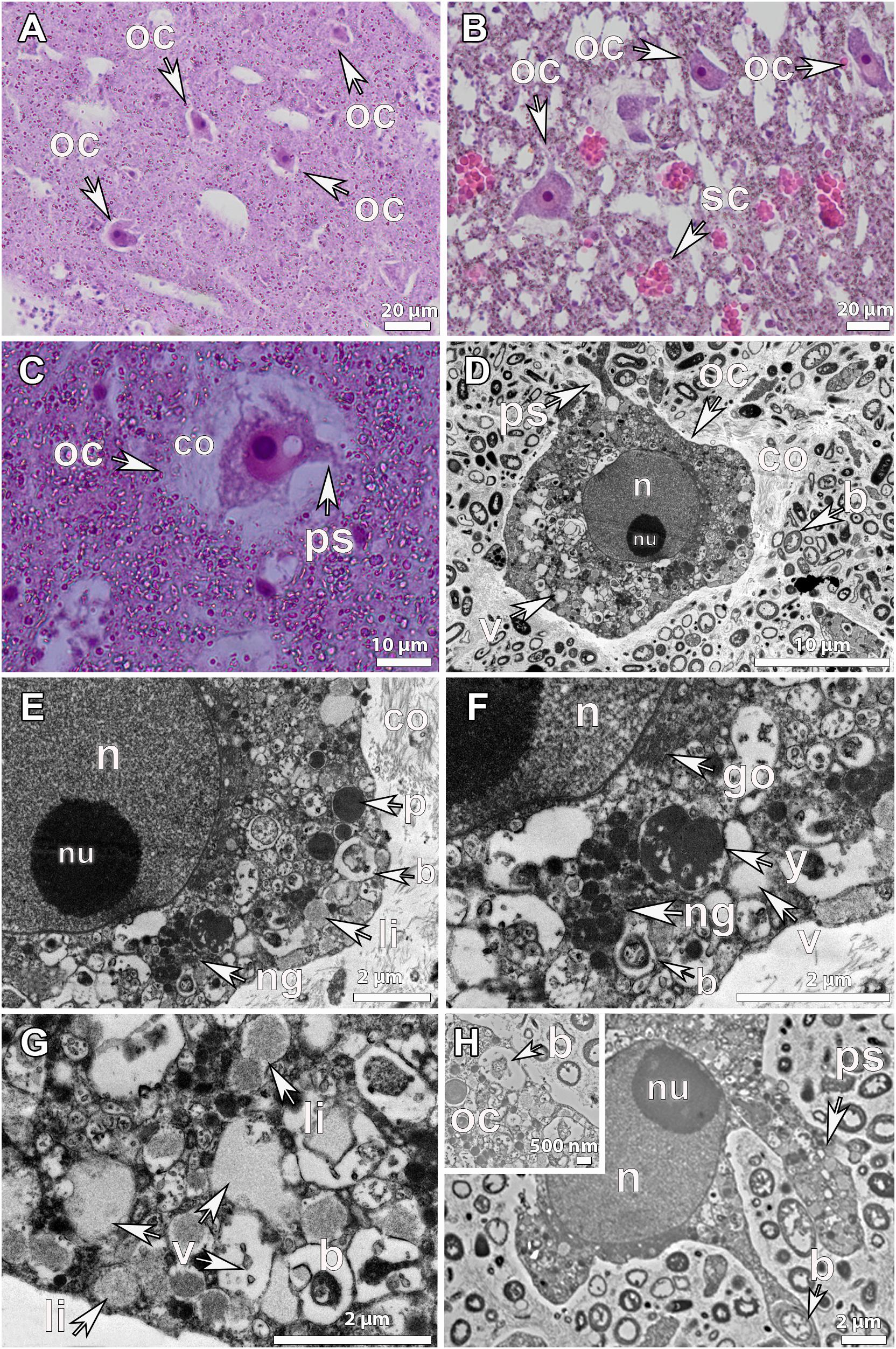
Figure 2. Histological observations and ultrastructure of previtellogenic oocytes from Geodia species. (A) Previtellogenic oocytes (oc) scattered in the mesohyl of G. hentscheli (∼12 μm) from the Schulz Bank in June. (B) Previtellogenic oocytes (oc) scattered in the mesohyl of G. barretti (∼23 μm), from Sula reef, end of July. Spherulous cells (sc) (∼20 μm) in the mesohyl. (C) Previtellogenic oocyte of G. hentscheli (oc) (∼20 μm) in the mesohyl with pseudopodia (ps) projections and a thin layer of collagen (co) formed around the oocyte. (D) Ultrastructure of previtellogenic oocyte of G. hentscheli, from the Schulz Bank in June with a nucleus (n) and a well-formed nucleolus (nu) and pseudopodia (ps) in the periphery. Ooplasm full of vesicles (v) and a thin collagen layer (co) around the oocyte. Bacterial symbionts (b) outside the oocyte in the mesohyl. (E) Close-up of image 2D, showing the nucleus (n) and the well-formed nucleolus (nu), the nuage granules (ng), small droplets of yolk of protein (p) and lipid origin (li) and one bacterium (b) in the ooplasm of the oocyte. A thin layer of collagen (co) surrounded the oocyte. (F) Close-up of image 4E, where the Golgi apparatus (go) around the nucleus, the nuage granules (ng), the lipid yolk droplets (y), the vesicles (v) and the bacteria (b) within the ooplasm were observed. (G) Previtellogenic oocyte of G. hentscheli from the Schulz Bank in June, with ooplasm full of vesicles (v), some lipid droplets (li), and a bacterium (b) within a vesicle in the ooplasm. (H) Ultrastructure of another previtellogenic oocyte of G. hentscheli, from the same individual as above with projections of pseudopodia (ps) in the periphery of the oocyte, phagocytizing bacteria (b) from the surrounding mesohyl. Insert: endocytic process with engulfment of symbiotic bacteria (b) from the mesohyl by the oocyte (oc).
In a later developmental stage, Vi oocytes were larger in general (>50-60 μm) (Figure 3 and Supplementary Figure S2B) and migrated to the vicinity of the canals (Figures 3A,C). Mature oocytes of G. barretti (50-60 μm), G. macandrewii (40-60 μm) and G. atlantica (103 μm in the largest diameter) were ready to get released (Figures 3B,C and Supplementary Figure S2A). When in the canals, mature oocytes were found in clusters, enveloped by a very thin collagen membrane (Figure 3B). A thick collagen layer was formed around the Vi oocytes but no cellular follicle (supportive membrane surrounding the oocyte) was observed in any of the studied species (Figures 3D-F). Vi oocytes contained a high amount of yolk, mainly of lipid origin (Figures 3D-H, 4, 5), and only few protein platelets were observed in the ooplasm (Figures 3D,E, 4, 5). Nurse cells were not observed in any of the Geodia spp. and the yolk was produced by autosynthesis via the rough endoplasmic reticulum and the Golgi apparatus (Figures 4B,D). In fact, lipid content was higher than protein content in both PV and Vi stages, increasing from an average of 7.5–17% compared to protein yolk that increased from 2 to 3% (Figure 5). Both G. barretti and G. hentscheli had the highest percentage of lipid content, 20 and 19% respectively, in their small Vi oocytes (35 ± 5 μm and 30 ± 17 μm in diameter respectively) (Figure 5B). G. macandrewii and G. atlantica had 13 and 15% of lipid yolk occupying their mature oocytes (43 ± 3 μm and 95 ± 7 μm in diameter respectively) (Figure 5B). Although at the beginning of vitellogenesis, vesicles with heterogenous yolk in oocytes of G. phlegraei and G. barretti were observed (Figure 5A), at later stages none of the studied species had heterogenous yolk in the ooplasm (Figures 3D-H, 4, 5B). Unfortunately, no high resolution TEM images of Vi oocytes of G. phlegraei could be obtained to investigate the presence of homogeneous and heterogeneous yolk in the later maturation stages.
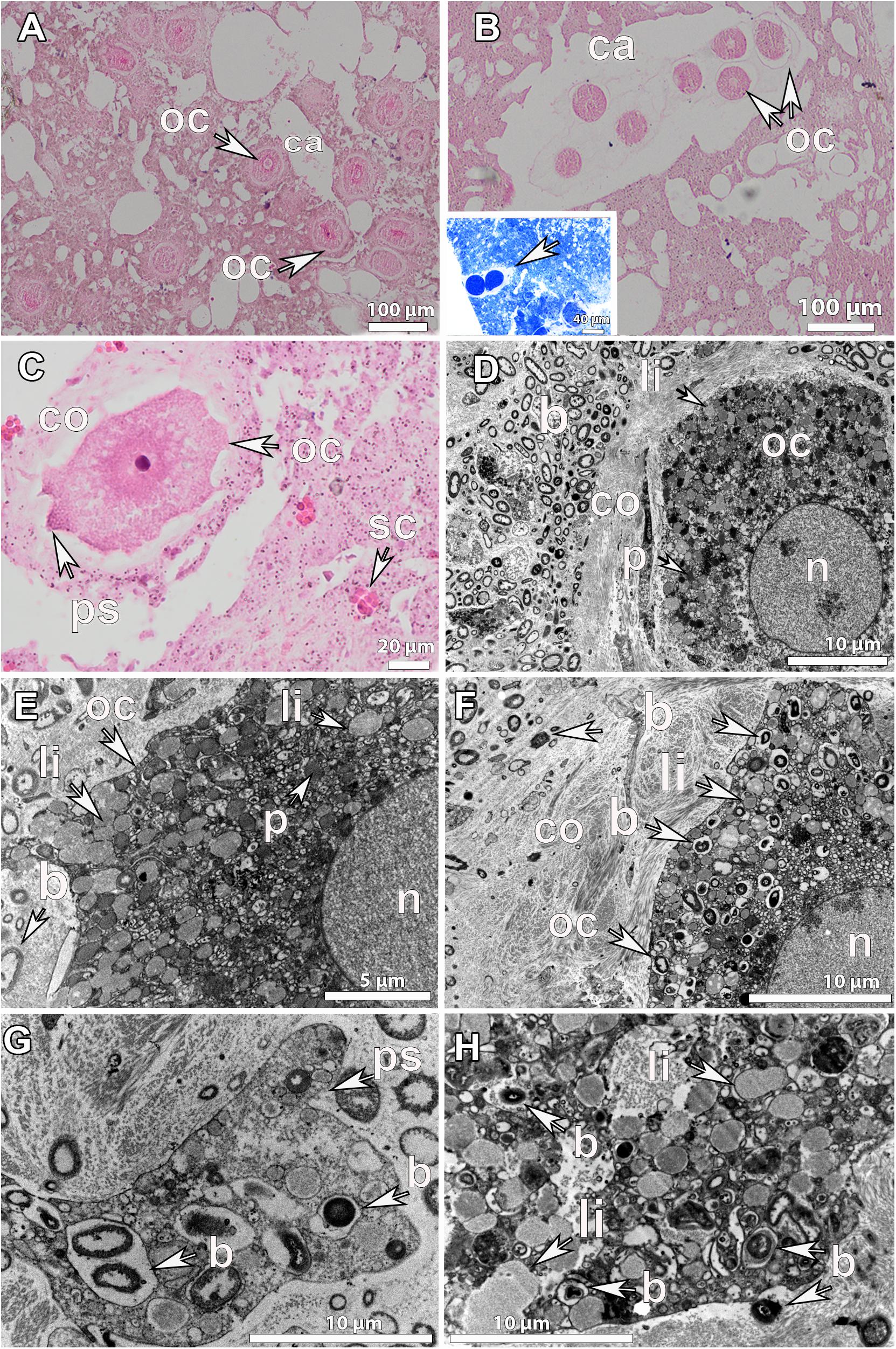
Figure 3. Histological observations and ultrastructure of vitellogenic oocytes from Geodia species. (A) Vitellogenic oocytes (oc) of G. atlantica (∼70 μm), from Rosemary Bank Seamount in September, in clusters around the canals (ca). (B) Vitellogenic oocytes (oc) of G. barretti (50–60 μm), from Rosemary Bank Seamount in September in clusters already released in a canal (ca). Insert: vitellogenic oocytes (oc) of G. macandrewii (∼40 μm), from the Korsfjord in September, grouped in clusters ready to be released into the canal. (C) Vitellogenic oocyte of G. atlantica (∼80 μm), from the Korsfjord in June, with formed pseudopodia (ps) and a thick collagen layer around it. Spherulous cells (sc) (∼20 μm) in the mesohyl. (D) Ultrastructure of vitellogenic oocyte of G. barretti from Tromsøflaket, western Barents Sea in August, with well-formed lipid yolk droplets (li) and some protein platelets (p). A thick collagen layer (co) surrounding the oocyte separates it from the mesohyl which is full of bacterial symbionts (b), though no bacteria were present within the oocyte. The nucleus (n) has chromatin condensations in two areas but no clear nucleolus. (E) Ultrastructure of another vitellogenic oocyte (oc) of G. barretti derived from the same individual with well-formed lipid (li) droplets and some protein (p) platelets in the ooplasm. No bacteria were found within the oocyte. The nucleus (n) was without chromatin condensations. (F) Vitellogenic oocyte (oc) of G. macandrewii, from Sula reef at the end of July, with well-developed lipid droplets (li) and bacterial symbionts (b) within the ooplasm. A thick layer of collagen (co) surrounded the oocyte. (G) Vitellogenic oocyte from the same individual of G. macandrewii as in 3F, with pseudopodia (ps) phagocytizing the encapsulated bacteria (b) from the mesohyl. (H) Close-up of vitellogenic I oocyte of G. hentscheli, from Schulz Bank at the beginning of August, with well-developed lipid droplets (li) and bacteria (b) within the ooplasm.
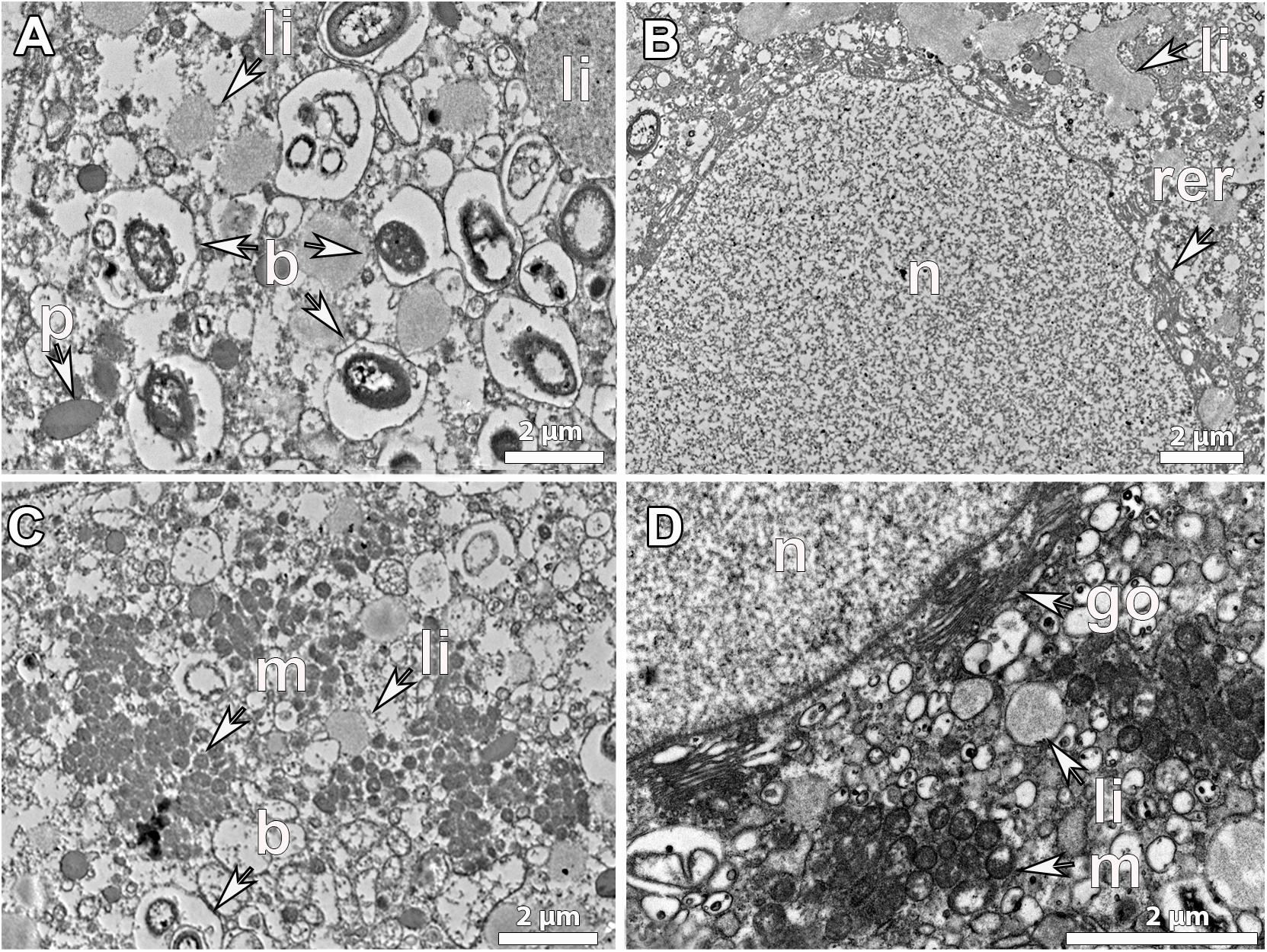
Figure 4. Ultrastructure of mature vitellogenic oocytes. Mature oocyte of G. atlantica, from Korsfjord, in September, showing (A), a large amount of lipid yolk droplets (li) and bacterial symbionts (b) within its ooplasm; (B) a well-developed rough endoplasmic reticulum (rer) close to the nucleus and large lipid droplets probably the result of fusion of several droplets, (C) an ooplasm full of mitochondrial clouds (m). (D) The structure of nucleus (n), the mitochondria (m) and the Golgi apparatus (go) as well as the formed lipid yolk (li).
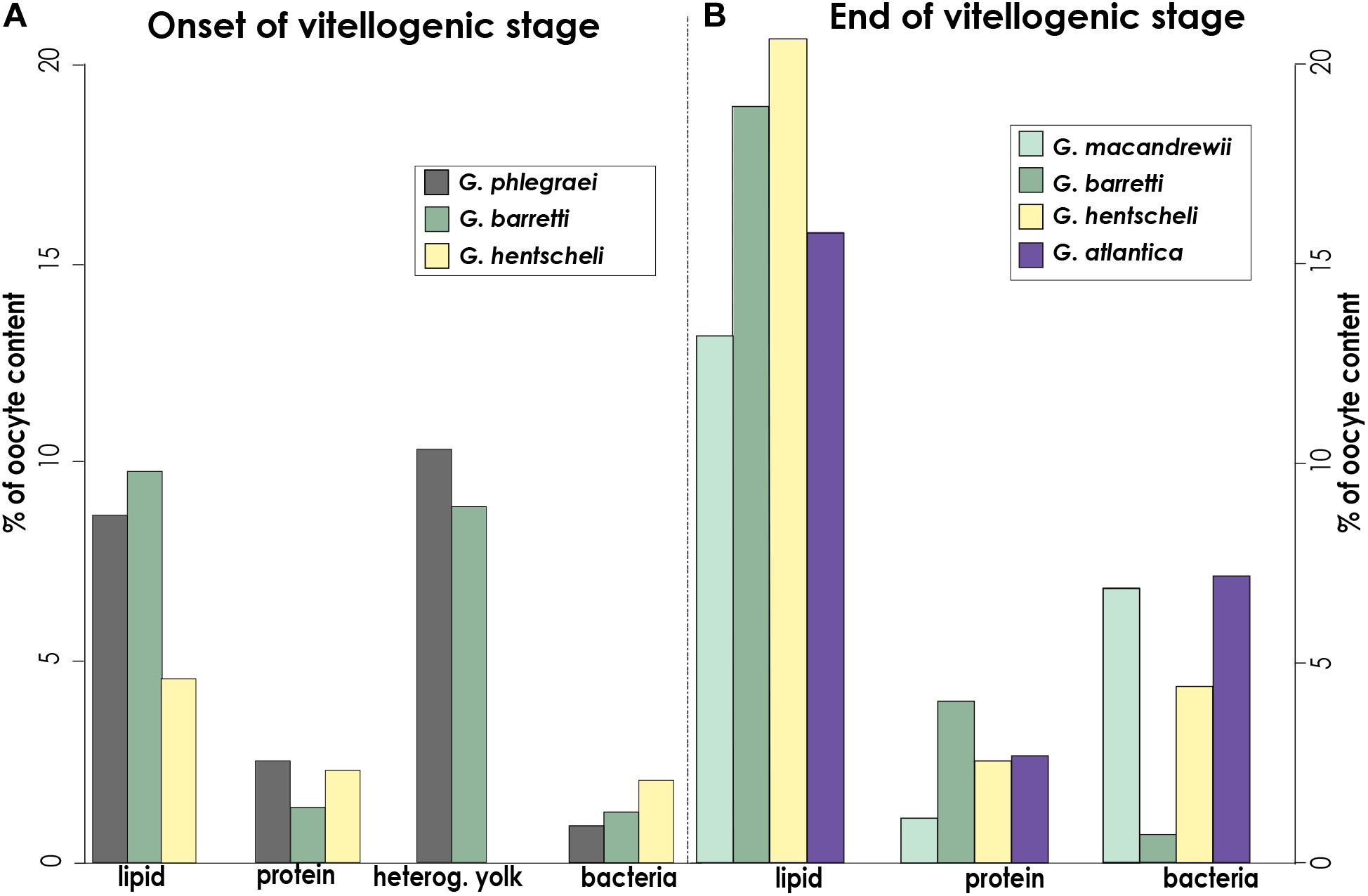
Figure 5. Oocyte content: types of yolk and number of bacteria. Percentage of area occupied by lipid, protein, heterogeneous yolk and bacteria at (A), the onset of the vitellogenenis in oocytes of G. phlegraei, G. barretti, and G. hentscheli, and (B), later vitellogenic stage in oocytes of G. macandrewii, G. barretti, G. hentscheli and G. atlantica. No ultrastructural observations were possible for early stages of vitellogenic oocytes of G. macandrewii and G. atlantica and the mature oocyte of G. phlegraei. These measurements were obtained from several oocytes of a selected specimen with oocytes, at the onset of vitellogenesis and another specimen with mature vitellogenic oocytes (G. barretti and G. macandrewii) or even with the most mature vitellogenic oocytes (G. atlantica, G. hentscheli) for each species.
Extensive vertical transmission of bacterial symbionts from the mother to the descendant at Vi stage in most species was also observed (Figures 3F-H, 4A, 5). Bacteria were collected by oocyte pseudopods and each bacterium was stored in vesicle in which bacterial division was observed in some cases (Figure 3G, insert). No signs of digestion were observed in the stored bacteria in the oocytes (Figure 3G). The average bacterial content increased from 1% in PV oocytes to 4% in Vi oocytes across species (Figure 5). The Vi oocytes of G. macandrewii (diameter ∼43 μm) and G. atlantica (with diameter ∼95 μm) had the largest area occupied by bacteria (∼7%) (Figures 3F, 4, 5B), followed by G. hentscheli with 4.4% in Vi oocytes (∼30 μm in diameter) (Figures 3E, 5B). However, Vi oocytes of G. barretti of similar size to those of G. hentscheli (diameter ∼35 μm), had a very low bacterial content (0.6-0.7%) without a significant increase in bacteria between the PV and Vi stages (Figures 3D,E, 5).
Spermatogenesis
SP_I spermatic cysts were scattered throughout the mesohyl, measuring on average 20-40 μm in all species (Figure 6A and Supplementary Figure S2B). Very early spermatic cysts contained only a few cells, mainly spermatogonia (Figures 6A-C). Bacteria were very abundant in the surroundings of the spermatic cysts, but they were seldomly observed within the lumen of the spermatic cyst. In the SP_I spermatic cysts of G. hentscheli, a few bacteria were phagocytosed by the spermatogonia, which were also full of vesicles and stored reserves (Figures 6C,D). The spermatogonia did not show a fully functional flagellum, but the axonemal structure could be seen in the cytoplasm of the spermatogonium, probably being resorbed (Figure 6D). This indicates that sperm cells, clearly derived from choanocytes, lose the choanocyte flagellum during early spermatogenesis.
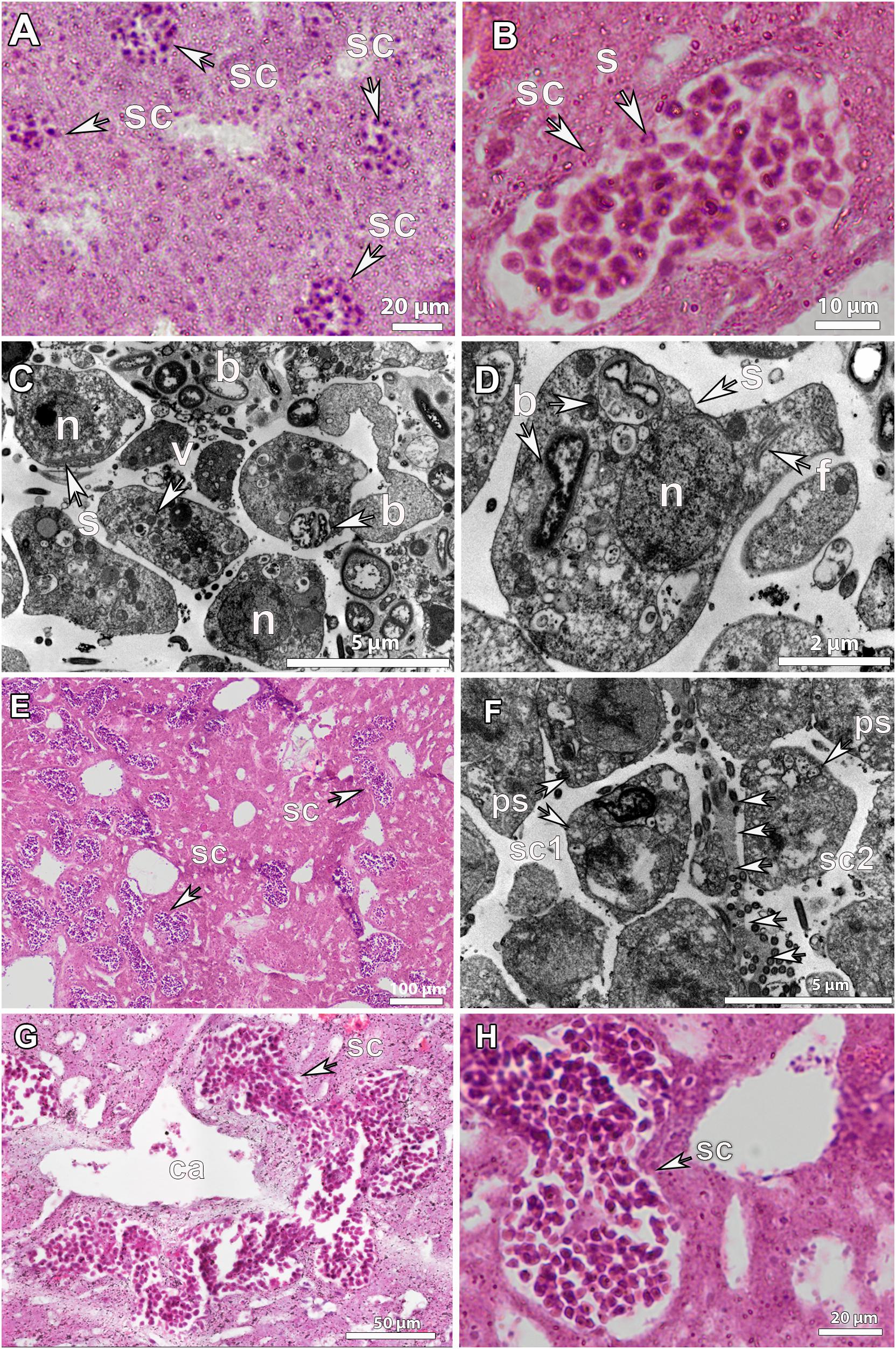
Figure 6. Histological observations and ultrastructure of spermatocytes in Geodia spp. (A) Spermatic cysts (sc) of early developmental stage (SP_I) scattered in the mesohyl of G. atlantica, from Hordaland coast in May. (B) SP_I spermatic cyst (sc) in G. phlegraei with spermatogonial cells (s) collected in Tromsøflaket, western Barents Sea, in August (C,D), ultrastructure of a SP_I spermatic cyst in G. hentscheli, from the Schulz Bank in August. Spermatogonia (s) with a distinct nucleated nucleus (n), vesicles (v), and few bacteria (b). The axonemal structure of the flagellum (f) was in the interior of the spermatogonium (s). (E) Spermatic cysts in developmental stage II (SP_II) of G. phlegraei, from Tromsøflaket, western Barents Sea in August, accumulated close to the canals. (F) Ultrastructure of SP_II spermatic cysts of G. phlegraei with primary spermatocytes (ps) in the periphery and thin mesohyl layer (arrows) between the two spermatic cysts (sc1 and sc2). (G) Spermatic cysts fused into one large spermatic cyst after mesohyl layer disintegration in G. phlegraei and were gathered around the canals (ca). (H) Close-up of a SP_II spermatic cyst of G. phlegraei with several spermatic cells, smaller than spermatogonial cells in size, compared to those in Figure 6B.
At a more mature stage, SP_II spermatic cysts measured on average 40-80 μm (Figure 6E and Supplementary Figure S2B) and formed clusters around the canals (Figure 6E). In some cases, a thin layer of mesohyl between two spermatic cysts seems to disintegrate, inducing the fusion of different spermatic cysts into one (Figure 6F). Fused clusters of spermatic cysts reached a maximum length of ∼200 μm (Figure 6G). No cellular or collagenous follicle layer surrounding the spermatic cyst was observed at any stage in any of the studied Geodia spp. (Figures 6A,B,H, 7A).
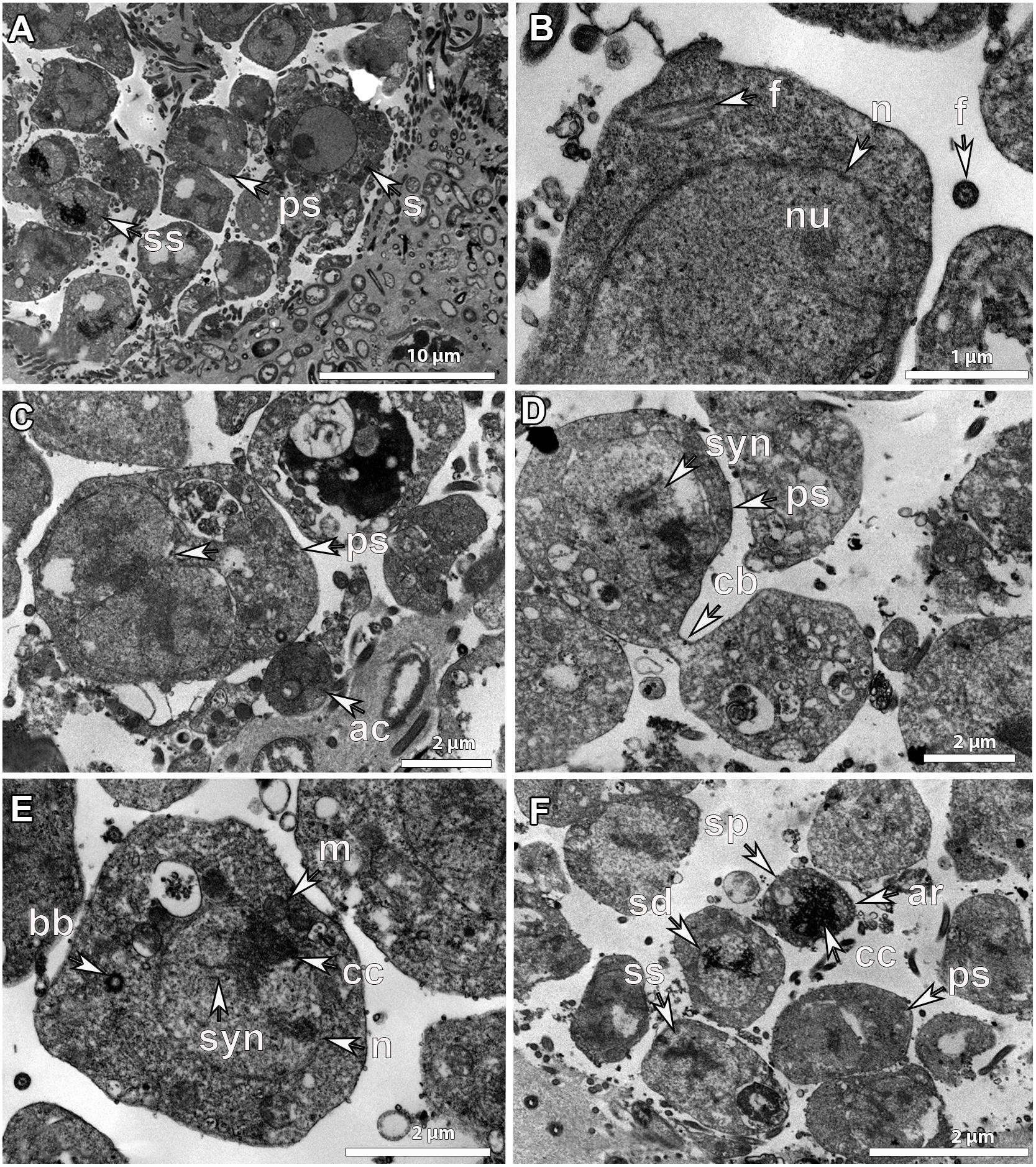
Figure 7. Ultrastructure of developmental stage_II (SP_II) spermatic cysts. SP_II spermatic cysts of G. phlegraei from Tromsøflaket, western Barents Sea in August showing (A), asynchronous development of sperm cells within the spermatic cyst with spermatogonia (s) in the outer part of the spermatic cyst, primary spermatocytes (ps) and secondary spermatocytes (ss) toward the inner part with increasing chromatin compactions. (B) Details of the structure of a spermatogonium with a nucleated (nu) nucleus (n) and the internalized axonemal structure of the flagellum (f). (C) Dividing primary spermatocyte with chromatin compaction after nuclear division. In the periphery of the spermatic cyst, ameboid-like cells (ac) are present. (D) Dividing primary spermatocytes with a cytoplasmic bridge (cb) before cytokinesis is completed and the synaptonemal complexes (syn) in the nucleus, (E) a secondary spermatocyte within a spermatic cyst, with chromatin compaction (cc) within the nucleus (n), synaptonemal complexes (syn), mitochondria (m), and the basal body (bb) before the new flagellum formation. (F) SP_II spermatic cyst with primary spermatocytes (ps), secondary spermatocytes (ss), spermatids (sd) and a potential spermatozoon (sp). Chromatin compaction (cc) and the acrosome (ar) of the cone-shaped late spermatid/early spermatozoon.
Inside SP_II spermatic cysts, there were more than 300 cells in the process of division to be developed into sperm cells (Figures 6G,H). Their development within the SP_II spermatic cyst was clearly asynchronous as cells in different developmental stages were observed, the ones dividing at early stages in the outer parts and the ones in late developmental stages, including spermatids and spermatozoa, toward the lumen of the cyst (Figure 7A). Spermatogonia were around 5-10 μm in maximum diameter; they had an amoeboid shape, with a well-developed nucleus and nucleolus (Figure 7A), and an internalized flagellum in the cytoplasm (Figure 7B). Primary spermatocytes were frequently observed dividing, they had a slightly smaller size (∼4-6 μm), no nucleolus, and showed chromatin condensations in the nucleus (Figures 7C,D), as well as synaptonemal complexes (Figures 7D,E). Secondary spermatocytes had more compacted chromatin and a smaller size (∼4 μm) than the previous developmental stage (Figures 7E,F). The internalized flagellum already disappeared at the stage of primary spermatocyte, and in the secondary spermatocyte, the basal body was observed indicating that a new flagellum is being formed during late spermatogenesis in Geodia spp. (Figure 7E). Then, spermatids had an even higher degree of chromatin compaction and a smaller size (∼2 μm) (Figure 7F). Finally, although we did not manage to obtain a clear picture of a spermatozoon with a flagellum, a potential spermatozoon (or a mature spermatid) was observed with several acrosomal vesicles in the apical part of the conical cell body (Figure 7F). The nucleus at its majority was composed by compacted chromatin and the mitochondria in the cytoplasm could not be observed, probably because of the angle of the image. Putative spermatozoa had the smallest size (∼1.5 μm) within the spermatic cyst, suggesting that this might be indeed the mature sperm cell (Figure 7F). Finally, we did not observe bacteria within sperm cells at later developmental stages.
Density and Fecundity Estimations
Density of Oocytes and Female Fecundity (Number of Oocytes Produced per Individual)
The number of oocytes per square millimeter of sponge tissue varied among individuals of the same species and among species (Figure 8). In average, Geodia spp. produced 7.2 ± 3.6 oocytes/mm2 of sponge tissue. However, some extreme cases were also identified. For instance, in two specimens of G. hentscheli with oocytes in PV stage, one had on average 37 oocytes/mm2 and the other 1.7 oocytes/mm2 (Figure 8). Additionally, a specimen of G. macandrewii with Vi oocytes, had the second highest value, 27 oocytes/mm2 on average (Figure 8).
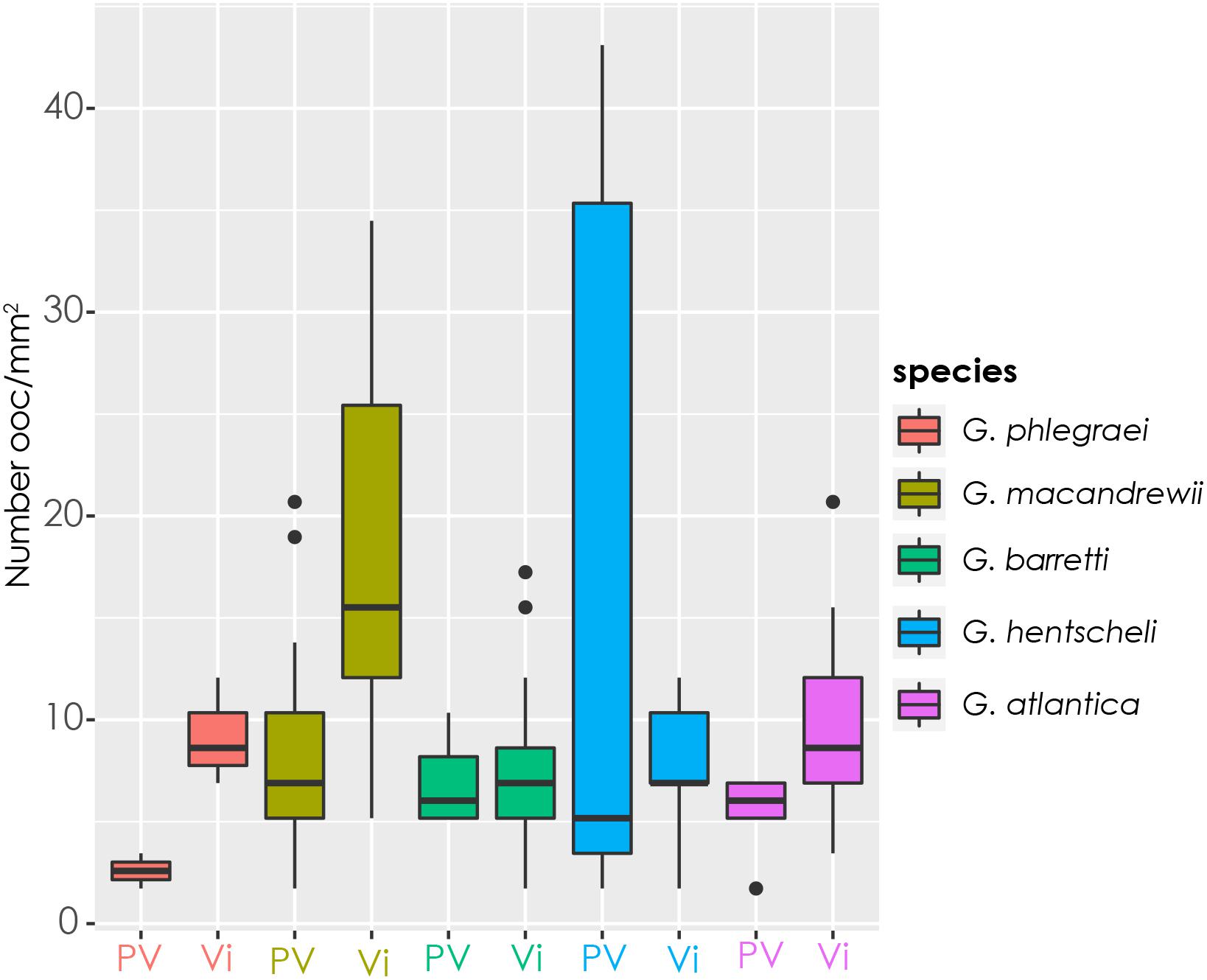
Figure 8. Female gamete density. Number of oocytes per square millimeter of sponge tissue in specimens with previtellogenic (PV) and vitellogenic (Vi) oocytes for each species. Specimens from all the sampling points for each species were included.
According to the calculations based on the modified Elvin’s formula and the volume of each species, G. macandrewii (which had the second largest body volume among the studied Geodia species) contained the maximum number of oocytes within its body (∼116 million oocytes), followed by G. atlantica which was estimated to contain ∼78 million oocytes per individual. G. barretti and G. phlegraei were estimated to potentially release ∼30 and ∼17 million oocytes respectively, and finally, G. hentscheli was expected to produce only ∼4 million oocytes per individual (Table 2).
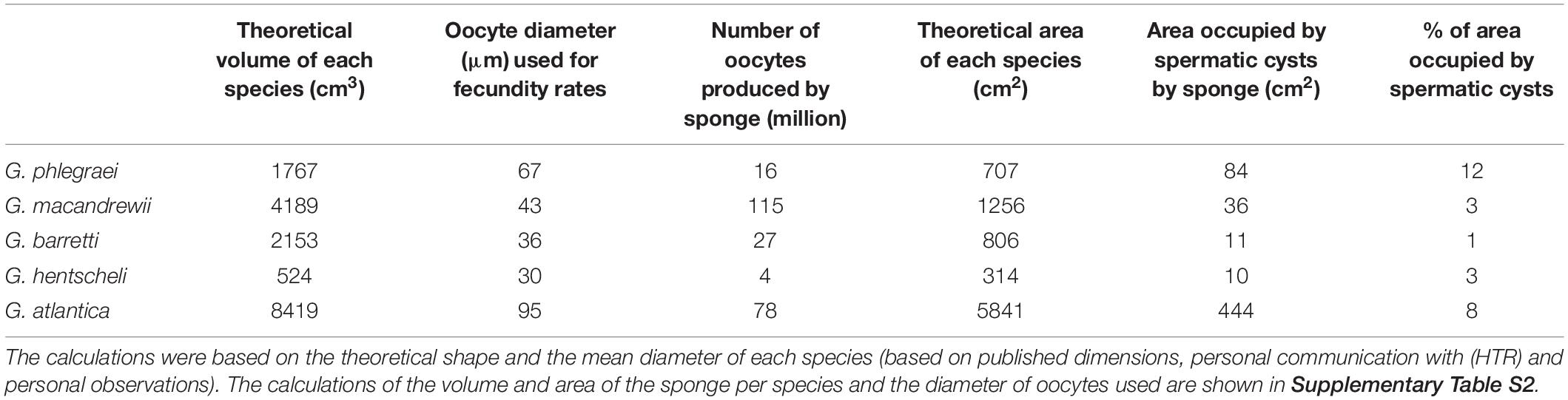
Table 2. Estimation of number of oocytes and the area occupied by spermatic cysts in the whole individual, extrapolated by calculations of a theoretical volume and area for each species.
Male Fecundity (Area of Tissue Occupied by Spermatic Cysts per Individual)
In G. phlegraei, the SP_II spermatic cysts occupied at least 12% of the total area of the sponge, and for G. atlantica only 7% of the total area (Table 2). For the other three species, G. macandrewii and G. hentscheli had a similar investment of spermatic cyst production (∼3%) and G. barretti had the lowest investment of all species (1.5%) (Table 2).
Reproductive Season of Geodia Species
From May to September, several individuals from the five Geodia spp. were producing gametes (Figure 9A, Table 1, and Supplementary Table S1). An exception was G. macandrewii: even though it was reproductive in September (in Svalbard and western Norway), none of the eight specimens collected during August (Tromsøflaket, western Barents Sea) was reproductive (Figure 9A, Table 1, and Supplementary Table S1). For G. phlegraei, only one specimen was collected in September (in Svalbard) and it was not engaged in reproduction (Table 1). For G. atlantica, no samples were collected in August, but otherwise, this species was reproductive throughout the rest of the sampling months and sampling points (Figure 9A, Table 1, and Supplementary Table S1). Finally, some G. hentscheli specimens collected in late June and August in Schulz Bank were reproductive (Figure 9A, Table 1, and Supplementary Table S1). Based on the maturation level of the oocytes observed in specimens the specific months and studied areas, the approximate period of spawning for all the Geodia spp. was estimated from May to November, depending on the area considered (Figure 9B).
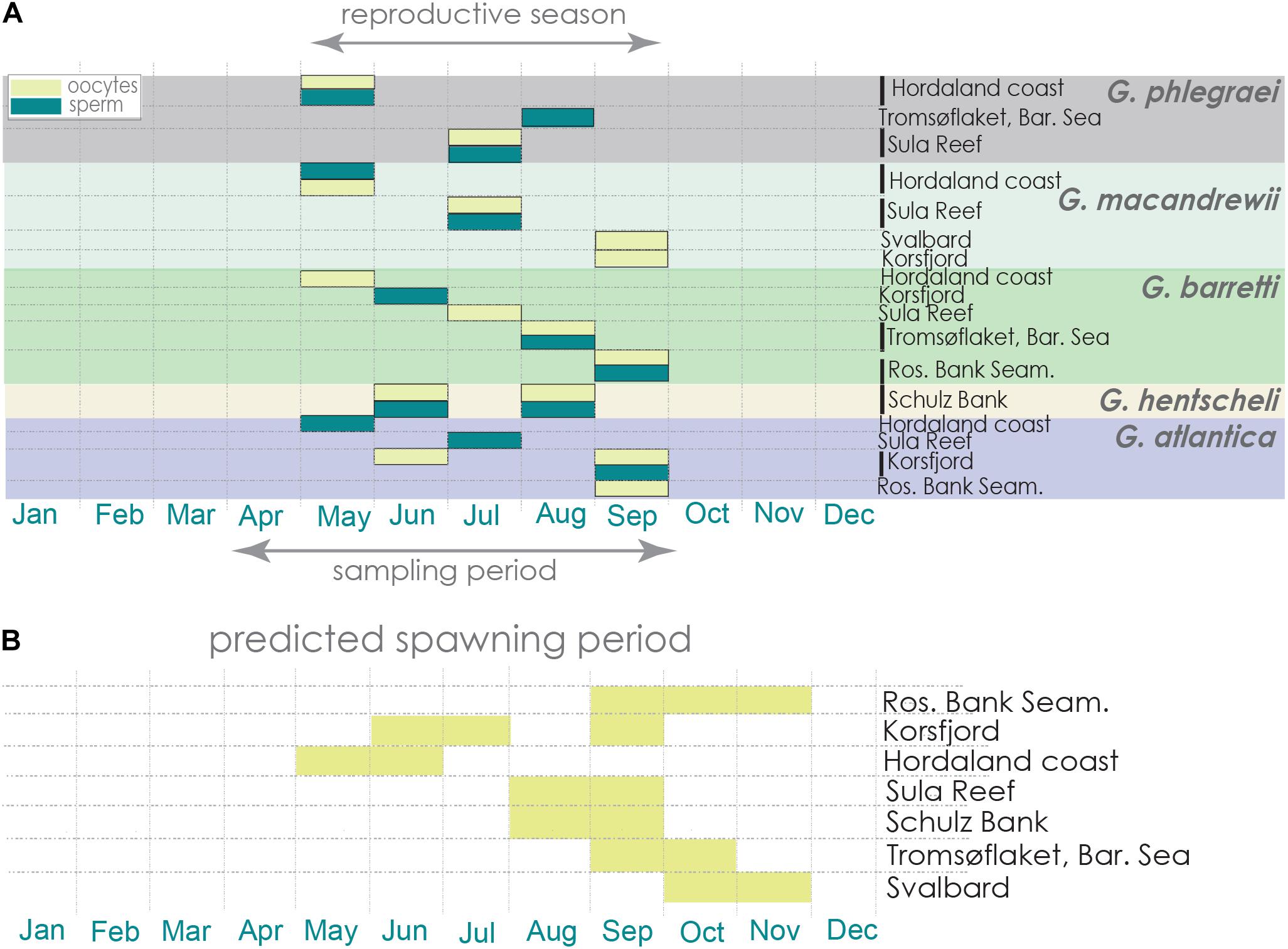
Figure 9. Reproductive period and estimated spawning period. (A) Reproductive period of each Geodia species across the different locations and dates of sampling. (B) Estimation of spawning events for Geodia spp. in each sampling area are represented in yellow, based on the maturation level of their oocytes at the time the specimens were collected.
In detail, in May and June, almost all the studied species contained reproductive features in early stages of gametogenesis and the size and maturation of gametes increased over the months (Figure 10 and Supplementary Figures S3, S4). During July and August, oocytes of most species were Vi (Figure 10 and Supplementary Figure S3). An exception was G. barretti, in which one reproductive individual had already Vi oocytes (56.1 ± 9 μm) in May (in Hordaland coast) when most other individuals had PV oocytes, and another individual had PV oocytes (23.3 ± 6.4 μm) in July (in Sula reef) when all others had Vi oocytes. Other exceptions were an individual of G. phlegraei (from Tromsøflaket, western Barents Sea) and another one of G. hentscheli (from Schulz Bank) which were also in early developmental stages in August (Figure 10 and Supplementary Figure S3), and surprisingly, one individual of G. phlegraei with Vi oocytes obtained in July in Sula reef (Figure 10 and Supplementary Figure S3). For G. macandrewii, G. barretti and G. atlantica, their largest Vi oocytes (in the final Vi stage) were observed in September (Figure 10 and Supplementary Figure S3). However, in September in addition to females with mature (Vi) oocytes, there were also females of G. macandrewii and G. atlantica with PV oocytes in Svalbard (not shown in the graph) and Rosemary Bank Seamount respectively (Figure 10 and Supplementary Figure S3). Regarding the spermatocytes, there was a general tendency of enlargement over the period from May to September (Supplementary Figures S3, S4) in all species.
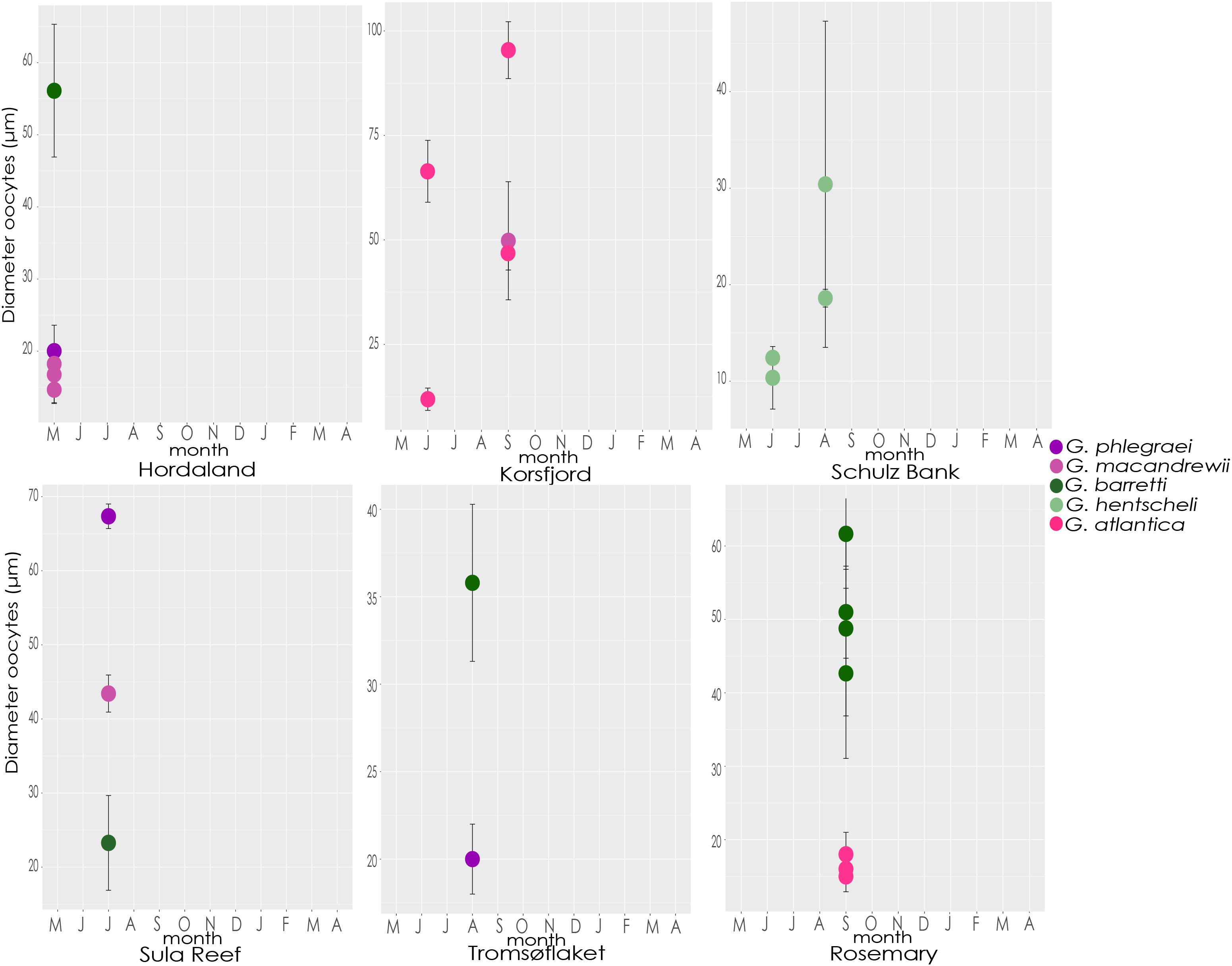
Figure 10. Size of oocytes. Average diameter and standard deviation of oocytes from each specimen of each species collected throughout the different months and locations.
Ratio of Reproductive Individuals (Males/Females) at Population Level
During the reproductive months, a high percentage (65-90%) of G. phlegraei individuals participated in reproduction and most of them were male with a sex ratio of 3:1 (Figure 11A and Table 1). A high percentage (65-75%) of the population of G. macandrewii was also engaged in reproduction but with either females predominating (1:2) or with equal proportions of males and females (1:1) (Figure 11B and Table 1). Regarding G. barretti (Figure 11C and Table 1), although a low percentage of individuals were producing gametes (11.6-20%) in May, June, and August, 80% of the individuals were reproductive in September in the Rosemary Bank, Seamount, while interestingly in the Korsfjord, western Norway, none of the seven individuals were reproducing. For G. barretti, the number of male and female individuals was equal (1:1). On the same note, for G. atlantica, 30% of the individuals collected were reproducing in May – June. In September, in contrast to G. barretti, the latter species had 40% of individuals engaged in reproduction in the Rosemary Bank, Seamount (Rockall Trough, 1034 m) and almost 80% of the individuals reproducing in the Korsfjord (western Norway, continental shelf). The sex ratio for G. atlantica was 1:1 (Figure 11D and Table 1). Finally, G. hentscheli recruited between 60 and 80% of its individuals for reproduction in June and August respectively, with more females than males, approximately in a ratio of 1:3 (Figure 11E and Table 1).
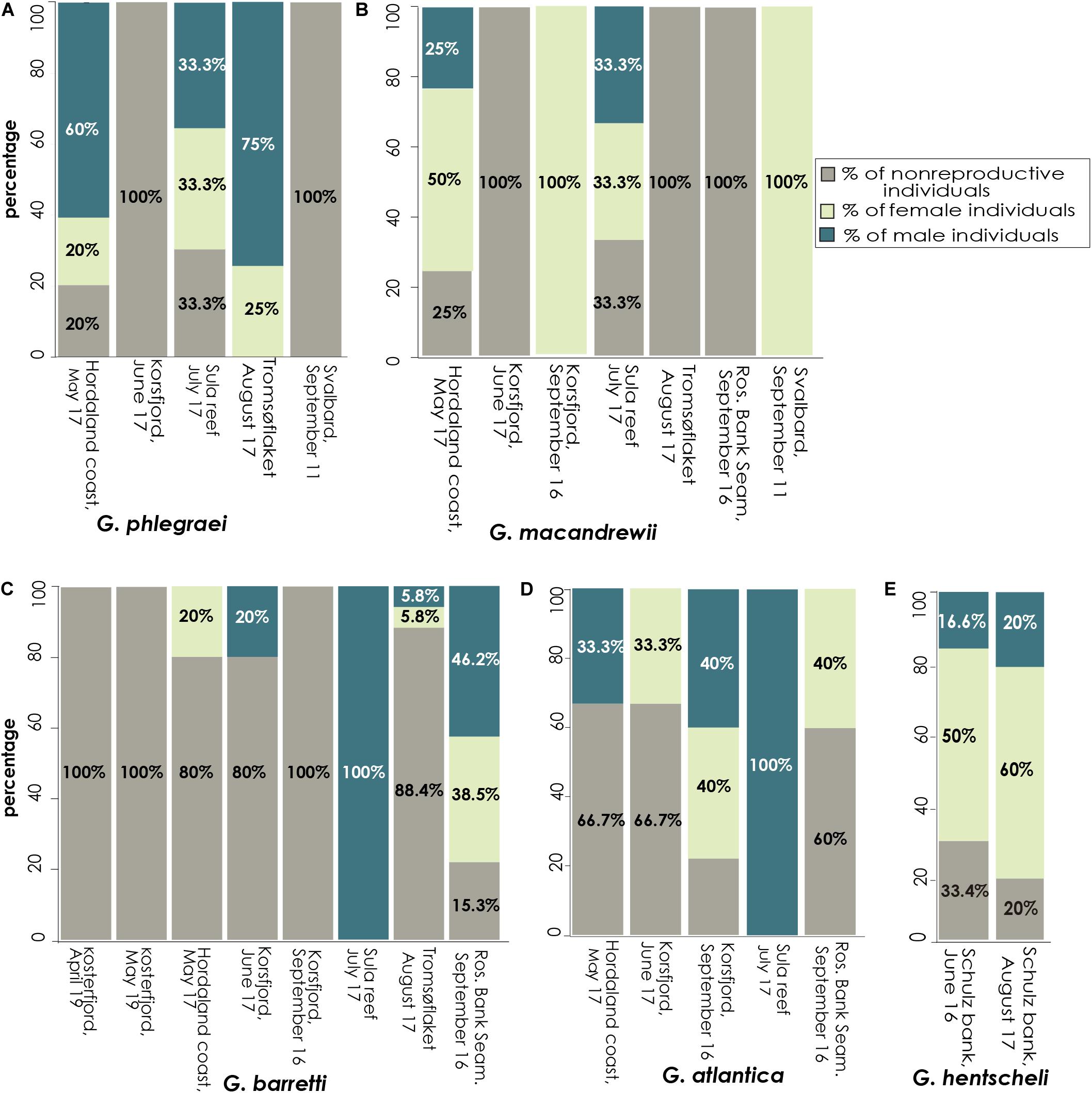
Figure 11. Investment in reproduction at the population level. Percentage of specimens with female and male gametes found per population (separated by location and time of collection) for each species. Data are provided for (A) G. phlegraei, (B) G. macandrewii, (C) G. barretti, (D) G. atlantica, and (E), G. hentscheli.
Discussion
Morphological Characteristics of Gametes
The Geodia species studied here were all gonochoristic and oviparous, as observed in previous studies on the Geodiidae family (Liaci and Sciscioli, 1969, 1970; Mercurio et al., 2007; Spetland et al., 2007), which belongs to the Astrophorina suborder, one of the three suborders currently recognized in the Tetractinellida order (Astrophorina, Spirophorina and Thoosina). Thenea abyssorum, another deep-sea astrophorin (family Theneidae) has also been shown to be gonochoristic and oviparous (Witte, 1996), suggesting that this could be the rule for the whole suborder. In the suborder Spirophorina, about half of the Tetillidae studied are also oviparous and gonochoristic (Liaci et al., 1976; Watanabe, 1978; Lepore et al., 2000) while another half, e.g., Tetilla polyura (Topsent, 1904) and Tetilla longipilis (Topsent, 1904), are viviparous (Topsent, 1904; P. Cárdenas, unpublished data). The suborder Thoosina is entirely hermaphroditic and viviparous (Bautista-Guerrero et al., 2010; Carballo et al., 2018). With the current knowledge of the Tetractinellida phylogenetic tree, and based on previous analyses of ancestral character reconstruction for demosponges (Riesgo et al., 2014), we hypothesize an ancestral gonochoristic and oviparous condition for Tetractinellida which later shifted to hermaphroditism/viviparism in Thoosina, although data are missing on the reproduction of one entire clade of Tetractinellida including the Stupendidae and the Scleritodermidae/Siphonidiidae/Azoricidae (Carballo et al., 2018). Besides the phylogenetic constraints, gonochorism and oviparity seem to be predominant in deep-sea invertebrates as more than 80% of deep-sea corals are also broadcast spawners (oviparous) and gonochoristic, while the most common pattern in shallow-water corals is hermaphroditism and oviparity (Brooke and Young, 2003; Burgess and Babcock, 2005; Waller, 2005; Harrison, 2011; Pires et al., 2014). Whether there is an ecological advantage for gamete release in deep-sea areas, in terms of lower predation over eggs is still unknown, but it would be necessary to investigate it to understand the ecology of deep-sea organisms.
Oogenesis in the studied Geodia spp. had very similar characteristics to that described previously for several astrophorins (Geodia conchilega, G. cydonium, G. barretti, Stelletta grubii, Erylus discophorus, T. abyssorum), including the formation of a collagen layer outside the oocyte, vertical transmission of bacterial symbionts acquired by phagocytosis, absence of nurse cells and autosynthesis of nutrients by the oocyte itself via the Golgi and endoplasmic reticulum apparatus (Liaci and Sciscioli, 1969; Sciscioli et al., 1989, 1991, 1994; Witte, 1996; Mercurio et al., 2007; Spetland et al., 2007). Oocytes in all Geodia spp. seemed to derive from archaeocytes, which at the beginning of the gametogenic cycle have a very similar shape and size (Liaci and Sciscioli, 1969; Sciscioli et al., 1994). On the other hand, spermatic cysts in all the studied species were clearly derived from choanocyte chambers, as previously suggested for other Geodiidae (Liaci and Sciscioli, 1969; Mercurio et al., 2007) but questioned for G. barretti (Spetland et al., 2007). These results clearly show a choanocyte origin of spermatic cysts, even for G. barretti. Spetland et al. (2007) suggested an alternative origin of the spermatic cysts based on the larger size of cysts than chambers, probably because they did not consider the fusion of several cysts. In this study, there is evidence that the early cysts did show a similar size and morphology to chambers (i.e., no collagen or cellular envelope), and fusion events were observed in later stages across all species.
Reproductive Strategy and Sex Ratio: Commonalities and Differences
Oocytes of deep-sea Geodia spp. from this study and of G. barretti from Spetland et al. (2007), have overall larger sizes (individuals with oocytes measuring more than 45 μm in diameter were detected in all species), with some species reaching 100 μm when fully grown, than mature oocytes of the shallower species, G. cydonium, that measure 30–40 μm (Sciscioli et al., 1994; Mercurio et al., 2007). Similarly, specimens of the family Tetillidae from deeper waters had 30% larger mature female gametes (∼74 μm) than shallow-water specimens (∼57 μm) (Meroz-Fine et al., 2005). The increasing size of eggs with depth had also been observed in deep-sea crustaceans and echinoderms that have larger lecithotrophic eggs than shallow water species (Mauchline, 1973; Corey, 1981; Cameron et al., 1988), indicating a possible general strategy for several marine invertebrates (Thorson, 1950). The size of mature oocytes in marine organisms with lecithotrophic embryonic and larval stages, is indicative of the pelagic larval duration (PLD) as larger oocytes are expected to accumulate a larger content of nutrients. Larger egg size can have profound ecological implications in marine invertebrates, such as longer planktonic phases and PLD and advantages in external fertilization given the larger targets for sperm (Marshall and Keough, 2007).
The planktonic phase duration in lecithotrophic propagules is dictated not only by the amount but also the type of yolk. In their oocytes, all the Geodia spp. had far more lipid than protein yolk. Even though lipid yolk in cold-water sponges is not always the rule (Koutsouveli et al., 2018; Koutsouveli et al., unpublished data), it is usually predominant over protein yolk in dispersal propagules of some sponge species from cold environments, while being 30% or less of the nutrient reserves in temperate and tropical species (Riesgo et al., 2015). For example, Stelletta grubii, an astrophorin species from temperate shallow waters, produces more protein than lipid yolk (Sciscioli et al., 1991). Furthermore, the temperate species G. cydonium also seems to have higher amounts of protein yolk accumulated in their oocytes (Sciscioli et al., 1994) than the Geodia spp. of this study. The predominance of fat provides more energy than protein and is metabolized faster, which is important for quick developmental changes in environments that are poor in nutrients, like the deep sea (Giese, 1966; Clarke, 1980). In addition, lipids also provide high buoyancy to the eggs which allows them to float in the water column (Clarke, 1980). The high content of lipids in the released oocytes of the deep-sea Geodia spp. could suggest an adaptation to a longer stay in the water column than the propagules of their temperate counterparts, until fertilization and ultimately embryogenesis take place, allowing longer dispersion distances and ensuring population connectivity over larger areas.
The vertical transmission of the bacterial symbionts in Geodia spp. was observed in oocytes of late developmental stages. This phenomenon has also been described in G. cydonium, as also in other astrophorid species (i.e., E. discophorus and S. grubii) (Sciscioli et al., 1989, 1991), in which bacteria were acquired by phagocytosis from the mesohyl, and were not digested but rather protected in vesicles within the oocytes, where they divided. As thus, these bacteria are true symbionts and are not consumed for nutritional needs at least during gametogenesis. Vacelet (1975) has suggested that these bacteria can serve as nutrients at a later embryonic stage. A recent study, not yet peer-reviewed, has showed that bacterial symbionts of Geodia spp. (including G. barretti, G. hentscheli, and G. atlantica), provide the host with precursor lipid building blocks, contributing to the synthesis of sponge unsaturated long-chain fatty acids (De Kluijver et al., 2020). So, the high content in lipid yolk might be related to the presence of high microbial abundance in these species, and it might be the case that by-products of bacterial symbionts within the oocytes contribute to the “autosynthetic” activity of lipid formation. On the other hand, in spermatogenesis, some bacteria were engulfed by early spermatogonia but disappeared in later spermatogenic stages. So, these sparse phagocytized bacteria are more likely to be used directly as a source of energy. This is a relatively novel observation in sponges, previously found only in the sperm of Spongia officinalis (Gaino et al., 1984) and Petrosia ficiformis (Maldonado and Riesgo, 2009), and it clearly points to choanocytes as the cells producing sperm.
Although the major reproductive patterns (i.e., oviparity and gonochorism) of the deep-sea Geodia spp. are clearly constrained by phylogeny (Riesgo et al., 2014), being the strategy at the genus, and possibly at suborder levels (e.g., Liaci and Sciscioli, 1969, 1970; Witte, 1996; Mercurio et al., 2007; Spetland et al., 2007), there is plasticity in some of the reproductive traits adopted by each species in order to cope with the energetic demands required for gametogenesis in these relatively oligotrophic habitats (Witte, 1996). First of all, slight variations were observed in seasonality among the species. For instance, individuals of G. barretti with mature female gametes and those of G. atlantica with premature female gametes were found at the same location and year, e.g., in Rosemary Bank Seamount in September 2016. This phenomenon has also been observed in Mediterranean species sharing the same habitat, which have to cope with the same nutrient regimes, staggering in time their resource allocation (Riesgo and Maldonado, 2008; Pérez-Porro et al., 2012; De Caralt et al., 2018). The different timing in gametogenesis and spawning among species could also be a strategy for avoiding cross-fertilization between different species and therefore hybridization. Furthermore, differences in terms of reproductive investment at individual and population levels were observed. Specifically, G. barretti seems to be the species with least investment in reproduction as only a small fraction of the population produces gametes and in relatively low numbers compared to the other studied species. This potentially could lead to a fragile situation of G. barretti if the environmental conditions or the populations themselves are perturbed. Interestingly, the gametes of G. barretti and G. hentscheli have smaller sizes compared to G. phlegraei or G. atlantica (Figure 10 and Supplementary Figures S2, S3) but they contain a higher amount of yolk (even though not the most mature vitellogenic oocytes were measured for G. barretti) (Figure 5), which can give them all the necessary reserves and buoyancy for longer drifting and thus ensure a higher fertilization success, expecting even higher yolk content in more mature oocytes of G. barretti. On the other hand, G. atlantica, with relatively lower amounts of yolk (Figure 5) will have shorter survival rate and lower buoyancy, so shorter drifting of its oocytes. However, G. atlantica produced more oocytes than the rest of species (three times more oocytes per individual, compared to G. barretti) (Figure 8 and Table 2), and a high percentage of the population is reproducing each year (Figure 11D), which increases the chances for successful reproduction. These species-specific reproductive traits can be correlated with observations of a most continuous presence of G. barretti compared to the patchier distribution of G. atlantica for instance, in Northern Norwegian continental shelf, while both showed a high abundance (Kutti et al., 2013). Although phylogenetically distantly related to G. atlantica (Cárdenas et al., 2010), G. macandrewii seems to follow a fairly similar strategy. Finally, in G. phlegraei, a comparatively higher percentage of the population participate in reproduction (Figure 11A), producing fewer oocytes (Figure 8 and Table 2) but highly enriched with nutrients (Figure 5).
Overall, oocyte densities in Geodia spp. (N of oocytes/mm2) were comparable or even higher to those reported on other oviparous sponges from temperate and tropical habitats. Riesgo and Maldonado (2008) found that the three oviparous and gonochoristic Mediterranean sponge species, Cymbaxinella damicornis (called Axinella damicornis in the publication), Raspaciona aculeata, and Chondrosia reniformis reached a maximum of 3–7 oocytes/mm2, while the coral reef sponge species Cliona vermifera had a maximum density of 3.5 oocytes/mm2 and 60–85% of its population participated in reproduction (Bautista-Guerrero et al., 2014). Two other Mediterranean oviparous, hermaphrodite Cliona spp., C. viridis and C. celata, showed a density of ∼10 oocytes/mm2 and a 70–90% of the population to participate in reproduction (Piscitelli et al., 2011). An exception was specimens of the family Tetillidae (most possibly the species Levantiniella levantinensis) which produced up to 155 oocytes/mm2 in some Mediterranean deeper habitats (Meroz-Fine et al., 2005). In the Geodia spp. of this study, a mean of 10 oocytes/mm2 was observed across species (Figure 8), with some specimens having a much higher number than the average (e.g., 38 ± 4 oocytes/mm2 in PV individual of G. hentscheli and 27 ± 6 oocytes/mm2 in Vi individual of G. macandrewii). In addition, a high percentage of their population participated in reproduction, as also mentioned for G. cydonium (Mercurio et al., 2007). So, despite the scarcity of nutrients in these habitats throughout the year, these species seemed to have a high reproductive effort, that together with their interplay with microbial symbionts (Radax et al., 2012; De Kluijver et al., 2020) could explain the dense aggregations of these species in the sponge grounds. This pattern contrasts to the theory that deep-sea benthos has usually a low reproductive effort due to unfavorable abiotic conditions, such as lack of nutrients (e.g., Thorson, 1950).
Even though, a relatively low number of specimens for each species was available, a dominance of females over male individuals (or equal ratios) was still observed in most species of the study, thus confirming previous studies on Geodia spp. (Liaci and Sciscioli, 1969; Mercurio et al., 2007; Spetland et al., 2007), as well as in most demosponges (e.g., Wapstra and van Soest, 1987; Tanaka-Ichihara and Watanabe, 1990; Witte et al., 1994, 1996; Corriero et al., 1996, 1998; Lepore et al., 2000; Meroz-Fine et al., 2005; Maldonado and Riesgo, 2008). G. phlegraei was the only species where males were three times the females. Also, there was a lower number of oocytes produced by female individuals compared to sperm generation by male individuals (e.g., in G. phlegraei ∼16 million oocytes/sponge and ∼30 billion spermatozoa/sponge respectively), which is possibly related to the higher energetic demands of oogenesis. Therefore, more female individuals should be engaged in each reproductive cycle in order to increase the percentage of oocytes released in the water column and a successful fertilization. Male dominance is a rare characteristic for sponges (Simpson, 1984; Sarà, 1993) but the energy requirements and allocation might be behind such a pattern: as the production of yolky female gametes requires higher energetic demands, this implies that the more individuals are involved in female gamete production, the more individuals of a population will be vulnerable at the end of the reproductive cycle due to lack of reserves, and so the whole habitat can be more vulnerable in case of disturbance. An indication that males require less energy to produce their gametes was shown in Oscarella lobularis, in which the male to female ratio increased massively (10 male:1 female) during food limitation (Ereskovsky et al., 2013). However, the feature of G. phlegraei cannot be directly correlated with abiotic conditions, and further sampling is needed to derive any conclusion.
Seasonality and Adaptations of the Reproductive Strategy to the Deep Sea
Organisms in most deep-sea environments normally face relatively constant abiotic conditions with some very predictable changes along the year, such as nutrient blooms after a seasonal primary production (Southwood, 1977; Clarke, 1980). In these habitats, the phenomenon of seasonality in reproduction is strong, mostly happening during the summer months, after the spring bloom, when the energy reserves have increased due to increased nutrient uptake (George and Menzies, 1967; Clarke, 1980). In general, astrophorin demosponges from the northern hemisphere, both deep-sea (Witte, 1996; Spetland et al., 2007) and shallow ones (Mercurio et al., 2007), reproduce annually, during summer months. In this study, the five boreo-arctic Geodia spp. were also reproducing during the summer months (May-September). However, this pattern presented some deviations in the different locations of the study. For example, reproductive specimens of G. barretti at Korsfjord (western Norway, 100-400 m) were barely found in June and September, an observation that comes in accordance with Spetland et al. (2007), who detected an annual cycle of G. barretti shifted to earlier months of the year (February – June) at this same location. However, reproductive features of G. barretti were found at Rosemary Bank Seamount (Rockall Through, 1304 m) in September. On the other hand, Spetland et al. (2007) also found that populations of G. barretti in Kosterfjord (Sweden, 80 – 200 m) had two annual cycles, one in February – June (estimated spawning time, May/June) and the other in July - October (estimated spawning time, October). Interestingly, no reproductive activity in specimens collected either in April or May in the same location was observed.
Differences in the reproductive cycle of a species between two locations or even between years in the same location can be explained by variations in environmental factors (Witte et al., 1994; Meroz-Fine et al., 2005). Similar observations have been reported for the deep-sea corals Primnoa resedaeformis (Gunnerus, 1763) and Paramuricea placomus (Linnaeus, 1758) in the Gulf of Maine (Fountain et al., 2019), in which populations from different areas showed variations in timing and even in the reproductive potential (e.g., max. size of the gametes, reproductive individuals/population, fecundity ranges). Likewise, populations of the cold-water coral Lophelia pertusa (Linnaeus, 1758) in the NE Atlantic and Trondheimfjord populations had variations in timing, maximum size of oocytes, and duration of their reproductive cycle (Waller and Tyler, 2005; Brooke and Järnegren, 2013). Brooke and Järnegren (2013) also attributed those differences likely to regional variations in the food supply. Mercier et al. (2011) found an indirect connection between the increased activity in the spawning of the gametes in six deep-sea species of echinoderms and the new/full moons, suggesting that lunar cycles affect the cyclic currents and the deposition of particular matter to the seafloor, which is then the main factor influencing the reproduction in the deep sea. Nutrient supply determines many aspects of reproduction in deep-sea invertebrates: from initiation of gametogenesis to vitellogenesis and time of spawning, depending on the reproductive strategy of the species (Tyler et al., 1992, 1993; Gooday, 2002; Young, 2003). For instance, deep-sea species tend to generate large eggs and invest a lot of energy on yolk formation of their eggs (even more if they have lecithotrophic larvae), and the stage of maturation/vitellogenesis is synchronized with periods of high food delivery (Eckelbarger and Watling, 1995).
The reproductive cycle of deep-sea sponges, including astrophorins and more specifically Geodia spp., was also suggested to be triggered by primary production (Witte, 1996; Spetland et al., 2007). In this analysis, specimens from locations at different depths and geophysical conditions were studied. In the Schulz Bank, only one plankton bloom is observed per year with the peak of carbon flux (input of organic matter) during June (U. Hanz, personal communication). Similarly in the Barents Sea, the peak of particulate organic carbon flux in the seafloor occurs during June – July (von Bodungen et al., 1995), which coincides with the maturation phase of gametes both in species of this study and in another deep-sea astrophorins (Witte, 1996). In Norwegian fjords though, there is an important spring bloom (March – April) and a smaller autumn bloom (August – September) (Wassmann, 1991; Wassmann et al., 1996), and the latter also seems to be synchronized with oocyte maturation for at least some of the studied species here. Deep-sea corals also synchronize their gametogenesis with the influx of nutrients to the seafloor as they take advantage of the spring/summer blooms to initiate their gametogenesis and then they spawn at the beginning of the following year (Maier et al., 2020). In the northern part of the Kosterfjord, very close to the sampling area of this study, spawning of the deep-sea coral L. pertusa begins in late January to February (Larsson et al., 2014), though other populations in Trondheimfjord (closer to the Sula reef) spawn from mid-February to late March (Brooke and Järnegren, 2013; Larsson et al., 2014) coinciding with a later summer bloom in this area. As the reproductive activity of many deep-sea invertebrates, which are seasonal reproducers, seems to be dependent on nutrient fluxes coming from the surface, it means that fluctuations in annual primary blooms could slightly displace the reproductive cycle of these species.
Marine Conservation in Boreo-Arctic North-Atlantic Deep-Sea Sponge Grounds
In marine conservation, reproductive timing, larval dispersal, and connectivity are key factors to take into account for developing adequate strategies to protect a variety of marine organisms (e.g., corals, mussels, fish) and habitats (Carson et al., 2010; Treml et al., 2012; Schmiing et al., 2016). In deep-sea sponge grounds, due to lack of essential biological information and to discontinuous monitoring, only prediction models based on generalized approaches are used to assess the potential dispersal of sponge populations and their capacity for regeneration in case of disturbance (e.g., Kenchington et al., 2019). The reproductive timing is a crucial parameter to consider when designing a conservation strategy, not only because it is the period of recruitment, but also because reproductive individuals are more vulnerable to disturbances due to their high energetic investment in gamete production and spawning. In addition, in the case of mechanical disturbance during the reproductive season, organisms may release premature propagules that cannot survive (Henry et al., 2003). For instance, samples of the deep-sea coral L. pertusa, from intensively trawled areas on Darwin Mounds, did not produce any gametes, also showing high levels of clonality that indicate a pause of sexual reproduction (Le Goff-Vitry et al., 2004; Waller and Tyler, 2005). In turn, samples of the same species in areas with less exploitation produced larger amounts of gametes during the exact same time (Waller and Tyler, 2005). Shallow-water corals (e.g., Pocillopora damicornis, Linnaeus, 1758) and sponges (e.g., Luffariella variabilis, Poléjaeff, 1884) reduce their reproductive activity during stress and invest more of their energy in survival (e.g., metabolic maintenance, tissue repair) (Brown and Howard, 1985; Ward, 1995; Ettinger-Epstein et al., 2007). Similar behaviors are then expected for their deep-sea counterparts.
In Geodia grounds, the fragmentary information related to lifespan, reproductive activity, reproductive strategy, and population connectivity patterns of the dominant species, has made the development of long-term conservation strategies on these habitats very difficult and in some cases unsupported (Thompson and Fuller, 2020). The species of the genus Geodia have one or two annual reproductive cycles. The spawning period for Geodia spp. in the studied areas has a broad range (May to November) depending on the area considered. Indeed, the reproductive period of Geodia spp. in sponge grounds should be taken into account to implement special protective measures related to fisheries during such times. Estimated spawning periods for Geodia spp. are completely different than that observed for deep-sea corals, which in the boreo-arctic areas of the North Atlantic spans from January to March (Brooke and Järnegren, 2013; Larsson et al., 2014). This therefore calls for specific measures of protection for the different habitats in the deep-sea. Such information combined with population genetic studies on these species and oceanographic circulation patterns would give more accurate predictions of dispersal and connectivity patterns of these populations in order to develop adequate conservation strategies specific to each species and habitat.
Data Availability Statement
The original contributions presented in the study are included in the article/Supplementary Material and archived in the PANGAEA data repository (https://doi.pangaea.de/10.1594/PANGAEA.921626). Further inquiries can be directed to the corresponding author/s.
Author Contributions
VK, AR, PC, and HR collected the samples. AR and VK contributed to conception and design of the study. VK, AR, and MC processed the samples. VK analyzed the data and drafted the manuscript. AR and PC did essential corrections to the manuscript. All the authors contributed to manuscript revision, read, and approved the submitted version.
Funding
This work was supported by the H2020 EU Framework Program for Research and Innovation Project SponGES (deep-sea Sponge Grounds Ecosystems of the North Atlantic: an integrated approach toward their preservation and sustainable exploitation) (Grant Agreement no. 679849). This document reflects only the authors’ view and the Executive Agency for Small and Medium-sized Enterprises (EASME) is not responsible for any use that may be made of the information it contains. Funding was also provided by a grant of the Spanish Ministry of Science and Innovation (PID2019-105769GB-I00) to AR.
Conflict of Interest
The authors declare that the research was conducted in the absence of any commercial or financial relationships that could be construed as a potential conflict of interest.
Acknowledgments
We are grateful to the scientist Jim Drewery (Marine Scotland Science, Aberdeen, United Kingdom) for the collection of samples in Rosemary Bank Seamount and Dr. Joana Xavier (CIIMAR – University of Porto, Portugal), Ph.D. student Karin Steffen (Department of Pharmaceutical Biosciences, Uppsala University, Sweden) and Dr. Francisca Carvalho (Department of Natural History, University of Bergen, Norway) for their help during fieldwork and sample collection. We are also indebted to Dr. Nadia Santodomingo, Dr. Bruna Plese, Dr. Nathan Kenny and Dr. Sergi Taboada for their help during laboratory processes. Finally, we thank the crew from the research vessels: G.O. Sars, Hans Brattstrøm (UiB), Nereus, Scotia and the research team from Tjärnö Marine Laboratory. This work is dedicated to the memory of HR, who was an esteemed colleague and long champion of sponge grounds of the North Atlantic.
Supplementary Material
The Supplementary Material for this article can be found online at: https://www.frontiersin.org/articles/10.3389/fmars.2020.595267/full#supplementary-material
Footnotes
References
Amante, C., and Eakins, B. W. (2009). ETOPO1 1 Arc-Minute Global Relief Model: Procedures, Data Sources and Analysis. NOAA Technical Memorandum NESDIS NGDC-24. Washington, DC: NOAA, 1–19.
Barthel, D., and Tendal, O. S. (1993). The sponge association of the abyssal Norwegian Greenland Sea: species composition, substrate relationships and distribution. Sarsia 78, 83–96. doi: 10.1080/00364827.1993.10413524
Bautista-Guerrero, E., Carballo, J. L., and Maldonado, M. (2010). Reproductive cycle of the coral-excavating sponge Thoosa mismalolli (Clionaidae) from Mexican Pacific coral reefs. Invertebr. Biol. 129, 285–296. doi: 10.1111/j.1744-7410.2010.00209.x
Bautista-Guerrero, E., Carballo, J. L., and Maldonado, M. (2014). Abundance and reproductive patterns of the excavating sponge Cliona vermifera: a threat to Pacific coral reefs? Coral Reefs 33, 259–266. doi: 10.1007/s00338-013-1094-1
Beazley, L., Kenchington, E., Yashayaev, I., and Murillo, F. (2015). Drivers of epibenthic megafaunal composition in the sponge grounds of the Sackville Spur, northwest Atlantic. Deep Sea Res. Part I Oceanogr. Res. Pap. 98, 102–114. doi: 10.1016/j.dsr.2014.11.016
Beazley, L. I., Kenchington, E. L., Murillo, F. J., del Mar, and Sacau, M. (2013). Deep-sea sponge grounds enhance diversity and abundance of epibenthic megafauna in the Northwest Atlantic. ICES J. Mar. Sci. 70, 1471–1490. doi: 10.1093/icesjms/fst124
Bowerbank, J. S. (1858). On the anatomy and physiology of the spongiadae. Part I. on the spicula. Philos. Trans. R. Soc. 148, 279–332. doi: 10.1098/rstl.1858.0016
Brooke, S., and Järnegren, J. (2013). Reproductive periodicity of the scleractinian coral Lophelia pertusa from the Trondheim Fjord, Norway. Mar. Biol. 160, 139–153. doi: 10.1007/s00227-012-2071-x
Brooke, S., and Young, C. M. (2003). Reproductive ecology of a deep-water scleractinian coral, Oculina varicosa. Cont. Shelf Res. 23, 847–858. doi: 10.1016/S0278-4343(03)00080-3
Brown, B. E., and Howard, L. S. (1985). Assessing the effects of ‘stress’ on reef corals. Adv. Mar. Biol. 22, 1–63. doi: 10.1016/S0065-2881(08)60049-8
Burgess, S., and Babcock, R. C. (2005). “Reproductive ecology of three reef-forming, deep-sea corals in the New Zealand region,” in Cold-water corals and ecosystems, eds A. Freiwald and J. M. Roberts (New York: Springer), 701–7013. doi: 10.1007/3-540-27673-4_36
Calazans, V. P. S. B., and Lanna, E. (2019). Influence of endogenous and exogenous factors on the reproductive output of a cryptogenic calcareous sponge. Mar. Biodivers. 49, 2837–2850. doi: 10.1007/s12526-019-01013-2
Cameron, J. L., McEuen, F. S., and Young, C. M. (1988). “Floating lecithotrophic eggs from the bathyal echinothuriid sea urchin Araeosoma fenestratum,” in Echinoderm Biology, eds R. Burke, P. V. Mladenov, P. Lambert, and R. L. Parsley (Rotterdam: A. A. BALKEMA), 23–28.
Carballo, J. L., Bautista-Guerrero, E., Cárdenas, P., Cruz-Barraza, J. A., and Aguilar-Camacho, J. M. (2018). Molecular and morphological data from Thoosidae in favor of the creation of a new suborder of Tetractinellida. Syst. Biodivers. 16, 512–521. doi: 10.1080/14772000.2018.1457100
Cárdenas, P., Rapp, H. T., Klitgaard, A. B., Best, M., Thollesson, M., and Tendal, O. S. (2013). Taxonomy, biogeography and DNA barcodes of Geodia species (Porifera, Demospongiae, Tetractinellida) in the Atlantic boreo-arctic region. Zool. J. Linn. Soc. 169, 251–311. doi: 10.1111/zoj.12056
Cárdenas, P., Rapp, H. T., Schander, C., and Tendal, O. S. (2010). Molecular taxonomy and phylogeny of the Geodiidae (Porifera, Demospongiae, Astrophorida) - combining phylogenetic and Linnaean classification. Zool. Scr. 39, 89–106. doi: 10.1111/j.1463-6409.2009.00402.x
Carson, H. S., López-Duarte, P. C., Rasmussen, L., Wang, D., and Levin, L. A. (2010). Reproductive timing alters population connectivity in marine metapopulations. Curr. Biol. 20, 1926–1931. doi: 10.1016/j.cub.2010.09.057
Cathalot, C., Van Oevelen, D., Cox, T. J. S., Kutti, T., Lavaleye, M., Duineveld, G., et al. (2015). Cold-water coral reefs and adjacent sponge grounds: hotspots of benthic respiration and organic carbon cycling in the deep sea. Front. Mar. Sci. 2:37. doi: 10.3389/fmars.2015.00037
Chen, W. (1976). “Reproduction and speciation in Halisarca,” in Aspects of Sponge Biology, ed. C. R. Harrison (New York: Academic Press), 113–139. doi: 10.1016/b978-0-12-327950-7.50014-2
Clarke, A. (1980). A reappraisal of the concept of metabolic cold adaptation in polar marine invertebrates. Biol. J. Linn. Soc. 14, 77–92. doi: 10.1111/j.1095-8312.1980.tb00099.x
Corey, S. (1981). Comparative fecundity and reproductive strategies in seventeen species of the Cumacea (Crustacea: Peracarida). Mar. Biol. 62, 65–72. doi: 10.1007/BF00396952
Corriero, G., Sarà, M., and Vaccaro, P. (1996). Sexual and asexual reproduction in two species of Tethya (Porifera: Demospongiae) from a Mediterranean coastal lagoon. Mar. Biol. 126, 175–181. doi: 10.1007/BF00347442
Corriero, G., Scalera Liaci, L., Nonnis Marzano, C., and Gaino, E. (1998). Reproductive strategies of Mycale contarenii (Porifera: Demospongiae). Mar. Biol. 131, 319–327. doi: 10.1007/s002270050325
De Caralt, S., González, J., Turon, X., and Uriz, M. J. (2018). Reproductive strategies of two common sympatric Mediterranean sponges: Dysidea avara (Dictyoceratida) and Phorbas tenacior (Poecilosclerida). PeerJ 6:e5458. doi: 10.7717/peerj.5458
De Kluijver, A., Nierop, K. G. J., Morganti, T. M., Bart, M. C., Beate, M., Hanz, U., et al. (2020). Bacterial precursors and unsaturated long-chain fatty acids are biomarkers of North-Atlantic demosponges. Biorivx [Preprint]. doi: 10.1101/2020.10.09.332833
Di Camillo, C. G., Coppari, M., Bartolucci, I., Bo, M., Betti, F., Bertolino, M., et al. (2012). Temporal variations in growth and reproduction of Tedania anhelans and Chondrosia reniformis in the North Adriatic Sea. Hydrobiologia 687, 299–313. doi: 10.1007/s10750-011-0877-z
Dohrmann, M., and Wörheide, G. (2013). Novel scenarios of early animal evolution—is it time to rewrite textbooks? Integr. Comp. Biol. 53, 503–511. doi: 10.1093/icb/ict008
Eckelbarger, K. J., and Watling, L. (1995). Role of phylogenetic constraints in determining reproductive patterns in deep-sea invertebrates. Invertebr. Biol. 114, 256–269. doi: 10.2307/3226880
Elvin, D. W. (1976). Seasonal Growth and Reproduction of an Intertidal Sponge, Haliclona permollis (Bowerbank). Univ. Chicago Press 151, 108–125. doi: 10.2307/1540709
Ereskovsky, A. V. (2000). Reproduction cycles and strategies of the cold-water sponges Halisarca dujardini (Demospongiae, Halisarcida), Myxilla incrustans and Iophon piceus (Demospongiae, Poecilosclerida) from the White Sea. Biol. Bull. 198, 77–87. doi: 10.2307/1542805
Ereskovsky, A. V., Dubois, M., Ivanišević, J., Gazave, E., Lapebie, P., Tokina, D., et al. (2013). Pluri-annual study of the reproduction of two Mediterranean Oscarella species (Porifera, Homoscleromorpha): cycle, sex-ratio, reproductive effort and phenology. Mar. Biol. 160, 423–438. doi: 10.1007/s00227-012-2100-9
Ettinger-Epstein, P., Whalan, S. W., Battershill, C. N., and de Nys, R. (2007). Temperature cues gametogenesis and larval release in a tropical sponge. Mar. Biol. 153, 171–178. doi: 10.1007/s00227-007-0793-y
FAO (2009). International Guidelines for the Management of Deep-sea Fisheries in the High Seas. Rome: FAO.
Fell, P. (1974). “Porifera,” in Reproduction of Marine Invertebrates, eds A. Giese and P. JS (New York: Academic Press), 51–132.
Fell, P. E. (1969). The involvement of nurse cells in oogenesis and embryonic development in the marine sponge, Haliclona ecbasis. J. Morphol. 127, 133–149. doi: 10.1002/jmor.1051270202
Feuda, R., Dohrmann, M., Pett, W., Philippe, H., Rota-Stabelli, O., Lartillot, N., et al. (2017). Improved modeling of compositional heterogeneity supports sponges as sister to all other animals. Curr. Biol. 27, 3864.e4–3870.e4. doi: 10.1016/j.cub.2017.11.008
Fountain, C. T., Waller, R. G., and Auster, P. J. (2019). Individual and population level variation in the reproductive potential of deep-sea corals from different regions within the Gulf of Maine. Front. Mar. Sci. 6:172. doi: 10.3389/fmars.2019.00172
Freese, J. L. (2001). Trawl-induced damage to sponges observed from a research submersible. Mar. Fish. Rev. 63, 7–13.
Fromont, J., and Bergquist, P. R. (1994). Reproductive biology of three sponge species of the genus Xestospongia (Porifera: Demospongiae: Petrosida) from the great barrier Reef. Coral Reefs 13, 119–126. doi: 10.1007/BF00300772
Gaino, E., Burlando, B., Zunino, L., Pansini, M., and Buffa, P. (1984). Origin of male gametes from choanocytes in Spongia officinalis (Porifera, Demospongiae). Int. J. Invertebr. Reprod. Dev. 7, 83–93. doi: 10.1080/01688170.1984.10510077
George, R. Y., and Menzies, R. J. (1967). Indication of cyclic reproductive activity in abyssal organisms. Nature 215:878. doi: 10.1038/215878a0
Giese, A. C. (1966). Lipids in the economy of marine invertebrates. Physiol. Rev. 46, 244–298. doi: 10.1152/physrev.1966.46.2.244
Gooday, A. J. (2002). Biological responses to seasonally varying fluxes of organic matter to the ocean floor: a review. J. Oceanogr. 58, 305–332. doi: 10.1023/A:1015865826379
Gunnerus, J. E. (1763). Om en søevext, allevegne ligesom besat med frøehuuse, Gorgonia resedæformis. Det Trondhiemske Selskabs Skrifter 2, 321–329.
Hansen, G. A. (1885). Spongiadae. The norwegian north-atlantic expedition 1876-1878. Zoology 13, 1–26. doi: 10.1080/00364827.1973.10411241
Harrison, P. L. (2011). “Sexual reproduction of scleractinian corals,” in Coral Reefs: An Ecosystem in Transition, eds Z. Dubinsky and N. Stambler (Dordrecht: Springer), 59–85. doi: 10.1007/978-94-007-0114-4_6
Henry, L. A., Kenchington, E. L. R., and Silvaggio, A. (2003). Effects of mechanical experimental disturbance on aspects of colony responses, reproduction, and regeneration in the cold-water octocoral Gersemia rubiformis. Can. J. Zool. 81, 1691–1701. doi: 10.1139/z03-161
Hoffmann, F., Radax, R., Woebken, D., Holtappels, M., Lavik, G., Rapp, H. T., et al. (2009). Complex nitrogen cycling in the sponge Geodia barretti. Environ. Microbiol. 11, 2228–2243. doi: 10.1111/j.1462-2920.2009.01944.x
ICES (2018). Report of the ICES/NAFO Joint Working Group on Deep-water Ecology (WGDEC). Dartmouth: ICES.
Ivanova, L., and Semyonov, V. (1997). Feeding habits of the larvae of sponges, Berliner geowiss. Abh 20, 93–101.
Kahn, A., Yahel, G., Chu, J., Tunnicliffe, V., and Leys, S. (2015). Benthic grazing and carbon sequestration by deep-water glass sponge reefs. Limnol. Oceanogr. 60, 78–88. doi: 10.1002/lno.10002
Kenchington, E., Power, D., and Koen-Alonso, M. (2013). Associations of demersal fish with sponge grounds on the continental slopes of the northwest Atlantic. Mar. Ecol. Prog. Ser. 477, 217–230. doi: 10.3354/meps10127
Kenchington, E., Wang, Z., Lirette, C., Murillo, F. J., Guijarro, J., Yashayaev, I., et al. (2019). Connectivity modelling of areas closed to protect vulnerable marine ecosystems in the northwest Atlantic. Deep Sea Res. Part I Oceanogr. Res. Pap. 143, 85–103. doi: 10.1016/j.dsr.2018.11.007
Klitgaard, A. (1995). The fauna associated with outer shelf and upper slope sponges (Porifera, Demospongiae) at the Faroe Islands, northeastern Atlantic. Sarsia 80, 1–22.
Klitgaard, A. B., and Tendal, O. (2004). Distribution and species composition of mass occurrences of large-sized sponges in the northeast Atlantic. Prog. Oceanogr. 61, 57–98. doi: 10.1016/j.pocean.2004.06.002
Klitgaard, A. B., Tendal, O., and Westerberg, H. (1997). “Mass occurrence of large sponges (Porifera) in Faroe Island (NE Atlantic) shelf and slope areas: characteristics, distribution and possible causes,” in The Responses of Marine Organisms to their Environments. Proceedings of the 30th European Marine Biology Symposium, eds L. E. Hawkins, S. Hutchinson, A. C. Jensen, M. Sheader, and J. A. Williams (Southampton: University of Southampton), 129–142.
Knudby, A., Kenchington, E., and Murillo, F. J. (2013). Modeling the distribution of Geodia sponges and sponge grounds in the Northwest Atlantic. PLoS One 8:e0082306. doi: 10.1371/journal.pone.0082306
Koltun, V. M. (1959). Donnaia fauna abissalnuich glubin Zhentralnovo Poljarnovo basseina [Fauna from the abyssal of central polar basin]. Dokl. Akad. Nauk SSSR. Seriya Biol. 129, 662–665.
Koutsouveli, V., Cárdenas, P., Conejero, M., Rapp, H. T., and Riesgo, A. (2020a). Collection Information of The Genus Geodia from Boreo-Arctic North-Atlantic Deep-Sea Sponge Grounds. London: PANGAEA.
Koutsouveli, V., Cárdenas, P., Santodomingo, N., Marina, A., Morato, E., Rapp, H. T., et al. (2020b). The molecular machinery of gametogenesis in Geodia demosponges (Porifera): evolutionary origins of a conserved toolkit across animals. Mol. Biol. Evol. doi: 10.1093/molbev/msaa183 [Epub ahead of print].
Koutsouveli, V., Taboada, S., Moles, J., Cristobo, J., Ríos, P., Bertran, A., et al. (2018). Insights into the reproduction of some Antarctic dendroceratid, poecilosclerid, and haplosclerid demosponges. PLoS One 13:e0192267. doi: 10.1371/journal.pone.0192267
Kutti, T., Bannister, R., Helle, K., Krogness, C., Tjensvoll, I., and Søvik, G. (2015). Metabolic responses of the deep-water sponge Geodia barretti to suspended bottom sediment, simulated mine tailings and drill cuttings. J. Exp. Mar. Bio. Ecol. 473, 64–72. doi: 10.1016/j.jembe.2015.07.017
Kutti, T., Bannister, R. J., and Fosså, J. H. (2013). Community structure and ecological function of deep-water sponge grounds in the Traenadypet MPA — Northern Norwegian continental shelf. Cont. Shelf Res. 69, 21–30. doi: 10.1016/j.csr.2013.09.011
Larsson, A. I., Järnegren, J., Strömberg, S. M., Dahl, M. P., Lundälv, T., and Brooke, S. (2014). Embryogenesis and larval biology of the cold-water coral Lophelia pertusa. PLoS One 9:e102222. doi: 10.1371/journal.pone.0102222
Laumer, C. E., Fernandez, R., Lemer, S., Combosch, D., Kocot, K. M., Riesgo, A., et al. (2019). Revisiting metazoan phylogeny with genomic sampling of all phyla. Proc. Biol. Sci. 286:20190831. doi: 10.1098/rspb.2019.0831
Le Goff-Vitry, M. C., Pybus, O. G., and Rogers, A. D. (2004). Genetic structure of the deep-sea coral Lophelia pertusa in the northeast Atlantic revealed by microsatellites and internal transcribed spacer sequences. Mol. Ecol. 13, 537–549. doi: 10.1046/j.1365-294X.2004.2079.x
Lepore, E., Sciscioli, M., Liaci, L. S., Santarelli, G., and Gaino, E. (2000). Sexual reproduction of Cinachyra tarentina (porifera, demospongiae). Ital. J. Zool. 67, 153–158. doi: 10.1080/11250000009356308
Leys, S. P., Kahn, A. S., Fang, J. K. H., Kutti, T., and Bannister, R. J. (2018). Phagocytosis of microbial symbionts balances the carbon and nitrogen budget for the deep-water boreal sponge Geodia barretti. Limnol. Oceanogr. 63, 187–202. doi: 10.1002/lno.10623
Liaci, L. S., and Sciscioli, M. (1969). La riproduzione sessuale di alcuni tetractinellidi (porifera). Boll. Zool. 36, 61–67. doi: 10.1080/11250006909440854
Liaci, L. S., and Sciscioli, M. (1970). The sexual cycle of Erylus discophorus (Schmidt) (Porifera, Tetractinellida)]. Riv. Biol. 63, 255–270.
Liaci, L. S., Sciscioli, M., and Piscitelli, G. (1976). The sexual reproduction of Tetilla sp. (Tetractinellida-Porifera). Rivista Biol. 69, 331–342.
Linnaeus, C. (1758). Systema Naturae per regna tria naturae, secundum classes, ordines, genera, species, cum characteribus, differentiis, synonymis, locis. doi: 10.5962/bhl.title.542
Maier, S. R., Bannister, R. J., van Oevelen, D., and Kutti, T. (2020). Seasonal controls on the diet, metabolic activity, tissue reserves and growth of the cold-water coral Lophelia pertusa. Coral Reefs 39, 173–187. doi: 10.1007/s00338-019-01886-6
Maldonado, M., Aguilar, R., Bannister, R., Bell, J., Conwa, K. W., Dayton, P., et al. (2016). “Sponge grounds as key marine habitats: a synthetic review of types, structure, functional roles, and conservation concerns,” in Marine Animal Forests: Ecology of Benthic Biodiversity, ed. S. Rossi (Switzerland: Springer International Publishing), 145–183. doi: 10.1007/978-3-319-21012-4_24
Maldonado, M., and Bergquist, P. R. (2002). “Phylum porifera,” in Atlas of Marine Invertebrate Larvae, eds M. Craig, Young, E. Mary, Rice, A. Mary, and Sewell (London: Academic Press), 21–50.
Maldonado, M., Beazley, L., López-Acosta, M., Kenchington, E., Casault, B., Hanz, U., et al. (2020). Massive silicon utilization facilitated by a benthic-pelagic coupled feedback sustains deep-sea sponge aggregations. Limnol. Oceanogr. doi: 10.1002/lno.11610 [Epub ahead of print].
Maldonado, M., Ribes, M., and van Duyl, F. C. (2012). “Chapter three – nutrient fluxes through sponges: biology, budgets, and ecological implications,” in Advances in Sponge Science: Physiology, Chemical and Microbial Diversity, Biotechnology, eds. M. A. Becerro, M. J. Uriz, M. Maldonado, and M. B. Turon (Academic Press), 113–182. doi: 10.1016/B978-0-12-394283-8.00003-5
Maldonado, M., and Riesgo, A. (2008). Reproduction in the phylum Porifera: a synoptic overview. Treballs SCB 59, 29–49. doi: 10.2436/20.1501.02.56
Maldonado, M., and Riesgo, A. (2009). Gametogenesis, embryogenesis, and larval features of the oviparous sponge Petrosia ficiformis (Haplosclerida, Demospongiae). Mar. Biol. 156, 2181–2197. doi: 10.1007/s00227-009-1248-4
Marshall, D. J., and Keough, M. J. (2007). The evolutionary ecology of offspring size in marine invertebrates. Adv. Mar. Biol. 53, 1–60. doi: 10.1016/S0065-2881(07)53001-4
Mauchline, J. (1973). The broods of British mysidacea (Crustacea). J. Mar. Biol. Assoc. U.K. 53, 801–817. doi: 10.1017/S0025315400022487
Mercier, A., Sun, Z., Baillon, S., and Hamel, J.-F. (2011). Lunar rhythms in the deep sea: evidence from the reproductive periodicity of several marine invertebrates. J. Biol. Rhythms 26, 82–86. doi: 10.1177/0748730410391948
Mercurio, M., Corriero, G., and Gaino, E. (2007). A 3-year investigation of sexual reproduction in Geodia cydonium (Jameson 1811) (Porifera, Demospongiae) from a semi-enclosed Mediterranean bay. Mar. Biol. 151, 1491–1500. doi: 10.1007/s00227-006-0584-x
Meroz-Fine, E., Shefer, S., and Ilan, M. (2005). Changes in morphology and physiology of an East Mediterranean sponge in different habitats. Mar. Biol. 147, 243–250. doi: 10.1007/s00227-004-1532-2
Murillo, F., Muñoz, P., Cristobo, J., Ríos, P., González, C., Kenchington, E., et al. (2012). Deep-sea sponge grounds of the Flemish Cap, Flemish Pass and the Grand Banks of Newfoundland (Northwest Atlantic Ocean): distribution and species composition. Mar. Biol. Res. 8, 842–854. doi: 10.1080/17451000.2012.682583
NAFO (2012). Northwest Atlantic Fisheries Organization Conservation and Enforcement Measures. Available online at: https://www.nafo.int/Portals/0/PDFs/fc/2012/fcdoc12-01.pdf
Pérez-Porro, A., Gonzalez Ramos, J., and Uriz, M. (2012). Reproductive traits explain contrasting ecological features in sponges: the sympatric poecilosclerids Hemimycale columella and Crella elegans as examples. Hydrobiologia 687, 315–330. doi: 10.1007/s10750-011-0919-6
Pham, C. K., Murillo, F. J., Lirette, C., Maldonado, M., Colaço, A., Ottaviani, D., et al. (2019). Removal of deep-sea sponges by bottom trawling in the Flemish Cap area: conservation, ecology and economic assessment. Sci. Rep. 9, 1–13. doi: 10.1038/s41598-019-52250-1
Pile, A., and Young, C. (2006). The natural diet of a hexactinellid sponge: benthic-pelagic coupling in a deep-sea microbial food web. Deep Sea Res. Part I Oceanogr. Res. Pap. 53, 1148–1156. doi: 10.1016/j.dsr.2006.03.008
Pires, D. O., Silva, J. C., and Bastos, N. D. (2014). Reproduction of deep-sea reef-building corals from the southwestern Atlantic. Deep Sea Res. Part II Top. Stud. Oceanogr. 99, 51–63. doi: 10.1016/j.dsr2.2013.07.008
Piscitelli, M., Corriero, G., Gaino, E., and Uriz, M. J. (2011). Reproductive cycles of the sympatric excavating sponges Cliona celata and Cliona viridis in the Mediterranean Sea. Invertebr. Biol. 130, 1–10. doi: 10.1111/j.1744-7410.2010.00216.x
Poléjaeff, N. (1884). “Report on the Keratosa collected by H.M.S. Challenger during the years 1873-1876,” in Report on the Scientific Results of the Voyage of H.M.S. Challenger During the Years 1873–76, ed. N. N. Polezhaev (Wyoming: Creative Media Partners).
Radax, R., Rattei, T., Lanzen, A., Bayer, C., Rapp, H. T., Urich, T., et al. (2012). Metatranscriptomics of the marine sponge Geodia barretti: tackling phylogeny and function of its microbial community. Environ. Microbiol. 14, 1308–1324. doi: 10.1111/j.1462-2920.2012.02714.x
Reynolds, E. S. (1963). The use of lead citrate at high ph as an electron-opaque stain in electron microscopy. J. Cell Biol. 17, 208–212. doi: 10.1083/jcb.17.1.208
Riesgo, A., and Maldonado, M. (2008). Differences in reproductive timing among sponges sharing habitat and thermal regime. Invertebr. Biol. 127, 357–367. doi: 10.1111/j.1744-7410.2008.00128.x
Riesgo, A., and Maldonado, M. (2009). Ultrastructure of oogenesis of two oviparous demosponges: Axinella damicornis and Raspaciona aculeata (Porifera). Tissue Cell 41, 51–65. doi: 10.1016/j.tice.2008.07.004
Riesgo, A., Novo, M., Sharma, P. P., Peterson, M., Maldonado, M., and Giribet, G. (2014). Inferring the ancestral sexuality and reproductive condition in sponges (Porifera). Zool. Scr. 43, 101–117. doi: 10.1111/zsc.12031
Riesgo, A., Taboada, S., Sánchez-Vila, L., Solà, J., Bertran, A., and Avila, C. (2015). Some like it fat: comparative ultrastructure of the embryo in two demosponges of the genus Mycale (order Poecilosclerida) from Antarctica and the Caribbean. PLoS One 10:e0118805. doi: 10.1371/journal.pone.0118805
Roberts, C. (2002). Deep impact: the rising toll of fishing in the deep sea. Trends Ecol. Evol. 17, 242–245. doi: 10.1016/s0169-5347(02)02492-8
Rooks, C., Fang, J. K.-H., Mørkved, P. T., Zhao, R., Rapp, H. T., Xavier, J. R., et al. (2020). Deep-sea sponge grounds as nutrient sinks: denitrification is common in boreo-Arctic sponges. Biogeosciences 17, 1231–1245. doi: 10.5194/bg-17-1231-2020
Sarà, M. (1993). “Porifera,” in Reproductive Biology of Invertebrates, Sexual Differentiation and Behaviour, eds. K. Adiyodi, and R. Adiyodi, (Chichester: Wiley), 1–29.
Schmidt, O. (1870). Grundzüge einer Spongien-Fauna des atlantischen Gebietes. Leipzig: Lhelm Engelmann.
Schmiing, M., Fontes, J., and Afonso, P. (2016). Predictive mapping of reproductive fish habitats to aid marine conservation planning. Can. J. Fish. Aquat. Sci. 74, 1016–1027. doi: 10.1139/cjfas-2015-0538
Schneider, C. A., Rasband, W. S., and Eliceiri, K. W. (2012). NIH Image to ImageJ: 25 years of image analysis. Nat. Methods 9, 671–675. doi: 10.1038/nmeth.2089
Sciscioli, M., Lepore, E., Gherardi, M., and Liaci, L. S. (1994). Transfer of symbiotic bacteria in the mature oocyte of Geodia cydonium (Porifera, Demosponsgiae): an ultrastructural study. Cah. Biol. Mar. 35, 471–478.
Sciscioli, M., Lepore, E., and Scalera-Liaci, L. G. M. (1989). Indagine ultrastrutturale sugli ovociti di Erylus discophorus (Schmidt) (Porifera, Tetractinellida). Oebalia 15, 939–941.
Sciscioli, M., Liaci, L. S., Lepore, E., Gherardi, M., and Simpson, T. L. (1991). Ultrastructural study of the mature egg of the marine sponge Stelletta grubii (porifera demospongiae). Mol. Reprod. Dev. 28, 346–350. doi: 10.1002/mrd.1080280406
Sollas, W. J. (1880). The sponge-fauna of Norway; a Report on the Rev. A.M. Norman’s collection of sponges from the Norwegian Coast. Ann. Mag. Nat. Hist. Zool. Bot. Geol. 5, 130–144.
Southwood, T. R. E. (1977). Habitat, the templet for ecological strategies? J. Anim. Ecol. 46, 336–365. doi: 10.2307/3817
Spetland, F., Rapp, H. T., Hoffmann, F., and Tendal, O. S. (2007). Sexual reproduction of Geodia barretti Bowerbank, 1858 (Porifera, Astrophorida) in two Scandinavian fjords. Mus. Nac. Ser. Livros 1858, 613–620.
Stephens, J. (1915). Sponges of the coasts of Ireland. I. The triaxonia and part of the tetraxonida. Fish. Irel. Sci. Investig. 4, 1–43.
Strand, R., Whalan, S., Webster, N. S., Kutti, T., Fang, J. K. H., Luter, H. M., et al. (2017). The response of a boreal deep-sea sponge holobiont to acute thermal stress. Sci. Rep. 7, 1–12. doi: 10.1038/s41598-017-01091-x
Tanaka-Ichihara, K., and Watanabe, Y. (1990). “Gametogenic cycle in Halichondria okadai,” in New Perspectives in Sponge Biology, ed. K. Rützler (Washington DC: Smithsonian Institution Press), 170–174.
Thompson, T., and Fuller, S. D. (2020). Technical Measures and Environmental Risk Assessments for Deep-Sea Sponge Conservation. Rome: FAO.
Thorson, G. (1950). Reproductive and larval ecology of marine bottom invertebrates. Biol. Rev. 25, 1–45. doi: 10.1111/j.1469-185X.1950.tb00585.x
Topsent, E. (1904). Spongiaires des Açores. Résultats des campagnes Sci. Accompl. par le Prince Albert I. Monaco 25, 1–280.
Treml, E. A., Roberts, J. J., Chao, Y., Halpin, P. N., Possingham, H. P., and Riginos, C. (2012). Reproductive output and duration of the pelagic larval stage determine seascape-wide connectivity of marine populations. Integr. Comp. Biol. 52, 525–537. doi: 10.1093/icb/ics101
Tyler, P. A., Gage, J. D., Paterson, G. J. L., and Rice, A. L. (1993). Dietary constraints on reproductive periodicity in two sympatric deep-sea astropectinid seastars. Mar. Biol. 115, 267–277. doi: 10.1007/BF00346344
Tyler, P. A., Harvey, R., Giles, L. A., and Gage, J. D. (1992). Reproductive strategies and diet in deep-sea nuculanid protobranchs (Bivalvia: Nuculoidea) from the rockall trough. Mar. Biol. 114, 571–580. doi: 10.1007/BF00357254
UNGA (2006). The Impacts of Fishing on Vulnerable Marine Ecosystems: Actions taken by States and Regional Fisheries Management Organizations and Arrangements to Give Effect to Paragraphs 66 to 69 of General Assembly Resolution 59/25 on Sustainable Fisheries, Regarding the Impacts of Fishing on Vulnerable Marine Ecosystems. New York, NY: UNGA.
Vacelet, J. (1975). Étude en microscopie électronique de l’association entre bactéries et Spongiaires du genre Verongia (Dictyoceratida). J. Microsc. Biol. Cell 23, 271–288.
von Bodungen, B., Antia, A., Bauerfeind, E., Haupt, O., Koeve, W., Machado, E., et al. (1995). Pelagic processes and vertical flux of particles: an overview of a long-term comparative study in the Norwegian Sea and Greenland Sea. Geol. Rundschau 84, 11–27. doi: 10.1007/BF00192239
Waller, R. G. (2005). ““Deep-water Scleractinia (Cnidaria: Anthozoa): current knowledge of reproductive processes”,” in Cold-Water Corals And Ecosystems, eds A. Freiwald and J. M. Roberts (New York: Springer), 691–700. doi: 10.1007/3-540-27673-4_35
Waller, R. G., and Tyler, P. A. (2005). The reproductive biology of two deep-water, reef-building scleractinians from the NE Atlantic Ocean. Coral Reefs 24, 594–602. doi: 10.1007/s00338-005-0501-7
Wapstra, M., and van Soest, R. (1987). “Sexual reproduction, larval morphology and behaviour in demosponges from the Southwest of the Netherlands,” in Taxonomy of Porifera. NATO ASI Series (Series G: Ecological Sciences), eds J. Vacelet and N. Boury-Esnault (Berlin: Springer), 281–307. doi: 10.1007/978-3-642-70892-3_15
Ward, S. (1995). Two patterns of energy allocation for growth, reproduction and lipid storage in the scleractinian coral Pocillopora damicornis. Coral Reefs 14, 87–90. doi: 10.1007/BF00303428
Wassmann, P. (1991). Dynamics of primary production and sedimentation in shallow fjords and polls of western Norway. Oceanogr. Mar. Biol. an Annu. Rev. 29, 87–154.
Wassmann, P., Svendsen, H., Keck, A., and Reigstad, M. (1996). Selected aspects of the physical oceanographic and particle fluxes in fjords of northern Norway. J. Mar. Syst. 8, 53–71. doi: 10.1016/0924-7963(95)00037-2
Witte, U. (1996). Seasonal reproduction in deep-sea sponges - triggered by vertical particle flux? Mar. Biol. 124, 571–581. doi: 10.1007/BF00351038
Witte, U., Barthel, D., and Tendal, O. (1994). The reproductive cycle of the sponge Halichondria panicea Pallas (1766) and its relationship to temperature and salinity. J. Exp. Mar. Bio. Ecol. 183, 41–52. doi: 10.1016/0022-0981(94)90155-4
Yahel, G., Whitney, F., Reiswig, H. M., Eerkes-Medrano, D. I., and Leys, S. P. (2007). In situ feeding and metabolism of glass sponges (Hexactinellida, Porifera) studied in a deep temperate fjord with a remotely operated submersible. Limnol. Oceanogr. 52, 428–440. doi: 10.4319/lo.2007.52.1.0428
Young, C. M. (2003). “Reproduction, development and life history traits,” in Ecosystems of the Deep Oceans. Ecosystems of the World 28, ed. P. Tyler (Amsterdam: Elsevier), 381–426.
Young, C. M., and Eckelbarger, K. J. (1994). Reproduction, Larval Biology, and Recruitment of the Deep-Sea Benthos. New York, NY: Columbia University Press.
Keywords: deep sea, sponge grounds, Geodia, reproduction, conservation
Citation: Koutsouveli V, Cárdenas P, Conejero M, Rapp HT and Riesgo A (2020) Reproductive Biology of Geodia Species (Porifera, Tetractinellida) From Boreo-Arctic North-Atlantic Deep-Sea Sponge Grounds. Front. Mar. Sci. 7:595267. doi: 10.3389/fmars.2020.595267
Received: 15 August 2020; Accepted: 19 November 2020;
Published: 18 December 2020.
Edited by:
Chiara Romano, Centre for Advanced Studies of Blanes (CEAB), Spanish National Research Council, SpainReviewed by:
Sylvie Marylène Gaudron, Sorbonne Universités, FranceRhian G. Waller, University of Gothenburg, Sweden
Copyright © 2020 Koutsouveli, Cárdenas, Conejero, Rapp and Riesgo. This is an open-access article distributed under the terms of the Creative Commons Attribution License (CC BY). The use, distribution or reproduction in other forums is permitted, provided the original author(s) and the copyright owner(s) are credited and that the original publication in this journal is cited, in accordance with accepted academic practice. No use, distribution or reproduction is permitted which does not comply with these terms.
*Correspondence: Vasiliki Koutsouveli, Vi5Lb3V0c291dmVsaUBuaG0uYWMudWs=; dmFzc2lha291dHM4OEBnbWFpbC5jb20=; Ana Riesgo, QS5SaWVzZ29AbmhtLmFjLnVr; YW5hcmllc2dvZ2lsQGdtYWlsLmNvbQ==