- 1School of Veterinary Medicine, National Taiwan University, Taipei, Taiwan
- 2Department of Engineering Science and Ocean Engineering, National Taiwan University, Taipei, Taiwan
- 3College of Veterinary Medicine, National Chiayi University, Chiayi City, Taiwan
- 4Institute of Ecology and Evolutionary Biology, National Taiwan University, Taipei, Taiwan
- 5Biology Department, Woods Hole Oceanographic Institution, Falmouth, MA, United States
- 6School of Veterinary Medicine, University of California, Davis, Davis, CA, United States
- 7Farglory Ocean Park, Hualien City, Taiwan
Many cetaceans are exposed to increasing pressure caused by anthropogenic activities in their marine environment. Anthropogenic sound has been recognized as a possible stressor for cetaceans that may have impacts on health. However, the relationship between stress, hormones, and cytokines secretion in cetaceans is complex and not fully understood. Moreover, the effects of stress are often inconsistent because the character, intensity, and duration of the stressors are variable. For a better understanding of how anthropogenic sounds affect the psychophysiology of cetaceans, the present study compared the changes of cortisol concentration and cytokine gene transcriptions in blood samples and behaviors of captive bottlenose dolphins (Tursiops truncatus) after sound exposures. The sound stimuli were 800 Hz pure-tone multiple impulsive sound for 30 min at three different sound levels (estimated mean received SPL: 0, 120, and 140 dB re 1 μPa) that likely cause no permanent and temporary hearing threshold shift in dolphins. Six cytokine genes (IL-2Rα, IL-4, IL-10, IL-12, TNF-α, and IFN-γ) were selected for analysis. Cortisol levels and IL-10 gene transcription increased and IFNγ/IL-10 ratio was lower after a 30-min high-level sound exposure, indicating the sound stimuli used in this study could be a stressor for cetaceans, although only minor behavior changes were observed. This study may shed light on the potential impact of pile driving-like sounds on the endocrine and immune systems in cetaceans and provide imperative information regarding sound exposure for free-ranging cetaceans.
Introduction
Marine mammals use their auditory system for foraging (Madsen et al., 2006), socialization (Nowacek et al., 2007), and for communication and orientation (David, 2006). Therefore, noise in their habitat could lead to potentially harmful effects on these important activities. As human presence in the offshore environment has grown, so have the anthropogenic sound levels (Wright et al., 2007). Anthropogenic noise generated by activities, such as shipping, seismic survey, explosives, and marine construction can interrupt important behaviors, interfere with communication between animals, and cause undesirable physiological effects on marine mammals (Erbe, 2013). In more extreme instances, exposures to sounds at high levels or for extended periods of time have the potential to cause hearing damage or physiological impacts (Nowacek et al., 2007; Erbe, 2013).
Another effect caused by underwater sound from human activities is stress (Romano et al., 2004). The physiology of a stress response in cetaceans is similar to that of terrestrial mammals (Schmitt et al., 2010; Atkinson et al., 2015). There are two different but interconnected systems involving the stress response in animals (Hall, 2015). The first is the sympathetic neuroendocrine response which initiates short-term physiological changes by releasing epinephrine and norepinephrine. These acute stress responses, such as increases in heart rate and blood pressure and redistribution of blood to the brain and muscles could prevent potentially life-threatening events. The second is a chain of endocrine reactions, predominantly in the hypothalamic-pituitary-adrenal (HPA) axis, aiming to restore homeostasis. When the stressor is perceived or anticipated, the HPA process can begins within seconds and can remain stimulated for hours after the event has ceased, depending on the duration or intensity of the stressor. Chronic stress responses can result in various harmful effects including lower reproductive success, inferior immune response, and increased susceptibility to infections (Romero and Butler, 2007). If animals are in a constant state of stress for days or longer, stress responses may become maladaptive, that means crucial behaviors may be hampered, such as foraging ability, escape from predators, and socialization (reviewed in Chrousos and Gold, 1992).
Anthropogenic underwater noise may be a stressor in marine mammals (Romano et al., 2004). Although the auditory and behavioral responses of cetaceans to sounds have been investigated (reviewed in Erbe et al., 2018), there are limited studies on measurements of the nervous, endocrine, and immune systems, such as catecholamines, glucocorticoids, and cytokines in cetaceans before and after stressors, especially related to anthropogenic sound (Thomas et al., 1990; Romano et al., 2004; Rolland et al., 2012). Therefore, it has been emphasized that there is much work to be done in the field of stress physiology and behavior in cetaceans (Wright et al., 2007; Atkinson et al., 2015). Thomas et al. (1990) examined behavioral responses and levels of blood catecholamines of four captive beluga whales (Delphinapterus leucas) before and after playbacks of noise from an oil drilling platform as indicators of physiological stress. The results showed the noise caused no temporary behavioral or physiological effects on these belugas. Romano et al. (2004) assayed the physiological responses of a captive beluga whale and a captive bottlenose dolphin (Tursiops truncatus) before and after exposure to several different levels of a seismic water gun as well as a pure tone resembling a sonar ping. The analyses of several physiological parameters, such as adrenaline, noradrenaline and dopamine concentrations in blood samples indicated high-level sound exposure-caused stress responses in both animals. In North Atlantic right whales (Eubalaena glacialis), it was shown that exposure to low-frequency noise from ships may cause not only habitat displacement, behavioral changes and vocalization pattern alterations, but also chronic stress according to the analysis of fecal glucocorticoid levels (Rolland et al., 2012), and has implications for all baleen whales in heavy ship traffic areas.
Recently it was demonstrated that the cytokine gene transcript profile in blood samples could change after low-frequency sound exposure (800-Hz pure-tone sound) in captive bottlenose dolphins identified by quantitative reverse transcription PCR (qRT-PCR) (Chen et al., 2018). The selected target genes were representing the following activities: (I) pro-inflammatory cytokines [Interleukin-12 (IL-12) and tumor necrosis factor α (TNFα)] (II) establishing [Interferon γ (IFNγ)], and suppressing (IL-4) the Th1 response (III) T cell activation (TAC) receptor (IL-2 receptor α subunit, IL-2Rα) and (IV) immunoregulatory/anti-inflammatory features and Th2 response (IL-10). Th1 response promotes cell-mediated immune response while Th2 response is associated with humoral immune response, and animals with Th1/Th2 polarization may become more susceptible to certain kinds of infection (Padgett and Glaser, 2003). The authors compared the gene transcript profiles between two dolphins with sound exposure and reference values from routine examinations of 4 dolphins. The study provides an accurate and reliable analysis technique of mRNA expression using reference genes (Chen et al., 2015), and stress-induced Th2 shift (decreased IFNγ and TNFα gene transcriptions and increased transcript levels of IL-10) was noticed in animals exposed to sounds. However, it would lead to a better understanding of the effect if the measurements before and after the stressor exposure were available. In addition, it was suggested that more samples and additional parameters, such as neural-immune factors, endocrine concentrations, levels of sound, and behavior monitoring accompanying with immunological experiment should be considered.
The current study was designed to measure cortisol concentrations and cytokine gene transcript profiles in blood and behavior response parameters before and after exposure to low-frequency multiple pure-tone sounds. The research hypothesis is that sound exposures may cause changes in endocrine, immune, and behavior parameters. The aim of this study was to provide critical information for recognizing the non-auditory effects of sound on cetacean health and make recommendations for future studies on the impacts of anthropogenic ocean noise on cetaceans.
Materials and Methods
Animals and Sound Source
Three clinically healthy 12-year-old non-pregnant female common bottlenose dolphins (T. truncatus gilli) (A, B, C) in Farglory Ocean Park in Hualien, Taiwan, were included in this study. Dolphin B was dominant to the other two dolphins according to the trainers’ observation. The dolphins were trained to voluntarily present tail flukes for blood collection in accordance with international guidelines (Ramirez, 1999). The protocol has been reviewed and approved by Council of Agriculture of Taiwan (Approval number 1051700175). Hearing measurements were made at the tested stimuli from 10 to 130 kHz using the non-invasive and rapid auditory evoked potential (AEP) technique (Mooney et al., 2012). AEP is a type of electroencephalogram signal emanated from neurons in the auditory system in response to acoustic stimuli. The AEP examination of dolphin A and B were finished before the sound exposure experiment following the method described previously (Mooney et al., 2015), and both animals showed generally good hearing abilities, similar to those of other bottlenose dolphins (Houser et al., 2008). Therefore, only dolphin A and B participated following measurements. Including dolphin C in this study was to investigate how dolphins react to noise when they live in a social group. Three dolphins were arranged in one of the pools (15 × 10 m, 4.5-m deep) in ocean park and exposed to sounds at the same time. The water filtration system was still on before and during sessions. The sound pressure level (SPL) distribution of the sound playback and the ambient sound in the pool were measured before the sound exposure experiment started. The ambient noise level was low (<100 dB re 1 μPa from 100 Hz to 1 kHz). The test stimuli were 30-min pure-tone sounds (800-Hz, 40 strikes/min, duration 150 ms). Three source levels were used in this study: (1) control (no sound but underwater transducer presented), (2) low and (3) high. The received SPL at 1 m from the underwater transducer (LL9162T, Lubell, Whitehall, OH, United States) was 153 dB re 1 μPa and the estimated mean received SPL (measured at 31 locations in the pool) was 140 dB re 1 μPa (SD = 4.79), which were supposed to cause no permanent and temporary shift in the auditory threshold in dolphins (NMFS, 2016). This was selected as the high level to be used in the experiment. The low level of sound was 133 dB re 1 μPa and the estimated mean received SPL was 120 dB re 1 μPa.
Experimental Procedure
Sessions consisted of 5–6 min voluntary blood collection, followed by a 30-min test period, and immediately followed by the second voluntary blood collection. For diminishing habituation and cumulative effects, one exposure session was performed every 3 days. The session interval was comparatively longer than those in previous studies, which were 1–2 sessions per day (Thomas et al., 1990; Romano et al., 2004; Kastelein et al., 2013). Every session began at 10:00 AM. The three sound levels were tested in random order, and each level was tested in 5 sessions, resulting in 15 sessions in all. The tests were not performed when background noise was possibly high (rainfall or wind speeds above Beaufort 4) to prevent masking of the test stimuli. During the sessions, no one was allowed within 10 m of the pool.
Whole blood was collected from dolphins in serum-separating tube and anticoagulated tubes, and 500-μl EDTA-anticoagulated samples were preserved in 1.3 ml of RNAlater® (Ambion, Applied Biosystems, Foster City, CA, United States) immediately for protecting cellular RNA in situ, and were stored at −20°C until analysis. The RNA extraction, complementary DNA (cDNA) synthesis from messenger RNA, target gene choice, primer design and quantitative PCR were conducted as described previously (Chen et al., 2015, 2018). Briefly, total RNA was extracted using RiboPureTM-Blood Kit (Ambion). RNA integrity and concentration were monitored using denaturing gel electrophoresis and fluorescence-based quantitation method. QuantiTect® Reverse Transcription kit (Qiagen) was used for cDNA synthesis. The target genes (IL-2 receptor α subunit, IL-4, IL-10, IL-12, IFNγ, and TNFα) were selected to cover a broad range of immunological events in dolphins (Chen et al., 2018). Species-specific primers and corresponding probes of the six selected genes and two reference genes (PGK1 and HPRT1) were used. Quantitative PCR were conducted using Eco Real-Time PCR System (Illumina, San Diego, CA, United States). The thermocycle conditions were set as follows: polymerase activation at 95°C for 10 min, followed by 45 cycles of denaturation at 95°C for 10 s and combined primer annealing/elongation at 60°C for 30 s. All reactions including no template controls (NTC) and plate controls were conducted in triplicate. This study was conducted according to MIQE (Minimum information for publication of quantitative real-time PCR experiments) guidelines (Bustin et al., 2009). Cortisol concentrations from serum samples was measured on the collection day by an automated competitive chemiluminescence enzyme immunoassay (ADVIA Centaur XPT Immunoassay System, Siemens, Erlangen, Germany, Intra-Assay: CV 3.28%, Inter-Assay: 4.5%). Chemiluminescence immunoassay technique has been successfully used in cortisol research in beluga whales (Chemiluminescent enzyme immunoassay, IMMULITE) (Schmitt et al., 2010), harbor porpoises (Electrochemiluminescent immunoassay, ECLIA) (Müller et al., 2013), and bottlenose dolphins (Electrochemiluminescence immunoassay, ECLIA) (Noda et al., 2006) (Immunochemiluminesence assays, ICLIA) (Reidarson and McBain, 1999).
The animals’ behavior was filmed from above by a waterproof camera (GoPro Hero4) with a wide-angle lens and the entire surface of the pool was captured on the video image. A researcher seated out of the animals’ sight for observing and documenting dolphins’ behavior. Instantaneous focal sampling method (Altmann, 1974) was applied to record the same dolphin’s behavior for the first and last 5-min periods of each sound exposure session. The observation record was compared with the film. The ethogram is shown in Table 1.
Statistics
Data from qRT-PCR were analyzed as transcription ratio (R) of normalized values (NVs) as described in a previous study (Pfaffl et al., 2002) with modifications. R values were calculated using the equation NVB/NVA, and NV = ETCqT/Geomean (ER1CqR1 and ER2CqR2), where ET, ER1, and ER2 are efficiencies of target and reference genes, CqT, CqR1, and CqR2 are Cq values of target and reference genes, and NVB and NVA are NVs of pre-exposure and post-exposure samples, respectively. NVs are inversely correlated with transcription levels. If NVB/NVA is larger than 1, it means the transcription level of post-exposure samples is higher than that of pre-exposure samples, and vice versa. The R values (NVB/NVA) were utilized instead of using median NVs from untreated healthy individuals for comparison as in previous studies (Tsai et al., 2017; Chen et al., 2018). Cortisol concentrations were analyzed as a ratio calculated as CA/CB, where CA and CB are concentrations of post-exposure and pre-exposure samples, respectively. Mann-Whitney U test, Spearman’s rho test, regression analysis, and Kruskal-Wallis test with post-hoc Dunn’s pairwise multiple comparison adjusted by Holm-Bonferroni method for dolphin A, dolphin B, and A + B pooled data (for increasing statistical power) were used to evaluate the effects of sound on cortisol level, gene transcription and behavioral response.
Results
The experimental subjects were clinically healthy before initiation and throughout the duration of the experiment as no obvious abnormalities in routine serum chemistries, complete blood cell counts, physical examination and behaviors were observed. Sixty blood samples (pre- and post-session samples of 15 sessions from 2 dolphins) were collected successfully (Supplementary Table 1). The cortisol concentrations of all pre-exposure samples (n = 15) from dolphin B were significantly higher than those (n = 15) from dolphin A (Mann-Whitney U test; z-score = −4.60407, p < 0.00001). Kruskal-Wallis tests showed significant differences among the control, low, and high sound exposure groups for dolphin A, dolphin B, and A + B pooled data for cortisol concentration ratio of post/pre-exposure samples (Table 2). Dunn’s post-hoc tests revealed that cortisol ratio elevated significantly after high-level test stimuli but not after low-level test stimuli or in control in individual and pooled data. Statistically significant differences among the control, low, and high sound exposure groups were also observed in the transcription ratio (R) of IL-10 in dolphin B, and IL-10, and IFNγ in pooled data (Table 3). Post-hoc tests revealed that the R of IL-10 elevated significantly after a high-level sound exposure only in pooled data. The R of IFNγ/IL-10 was calculated as (NVB–IFNγ/NVB–IL10)/(NVA–IFNγ/NVA–IL10), and it was detected to be significantly lower in high sound exposure group in pooled data, indicating a shift to Th2 immune response. Only the R of IFNγ in high group in dolphin B showed strong direct relationship with time (r = 0.6482) although the last R was still lower than 1 (0.88). The obviously disparate data distribution of IL-12 between two dolphins in high group was observed (Table 3). There is a strong inverse relationship between cortisol concentration ratio (CA/CB) and R of IL-12 in high group (r = −0.6704; rs = −0.73333, p < 0.05). No strong relationship between CA/CB and R of other cytokine genes was noticed.
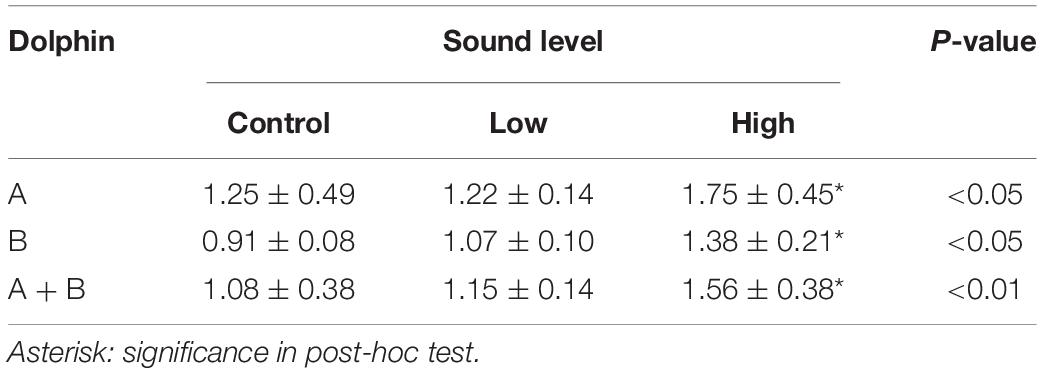
Table 2. Results of Kruskal-Wallis tests with post-hoc tests of cortisol concentration ratio (mean ± SD) of post/pre-exposure samples.
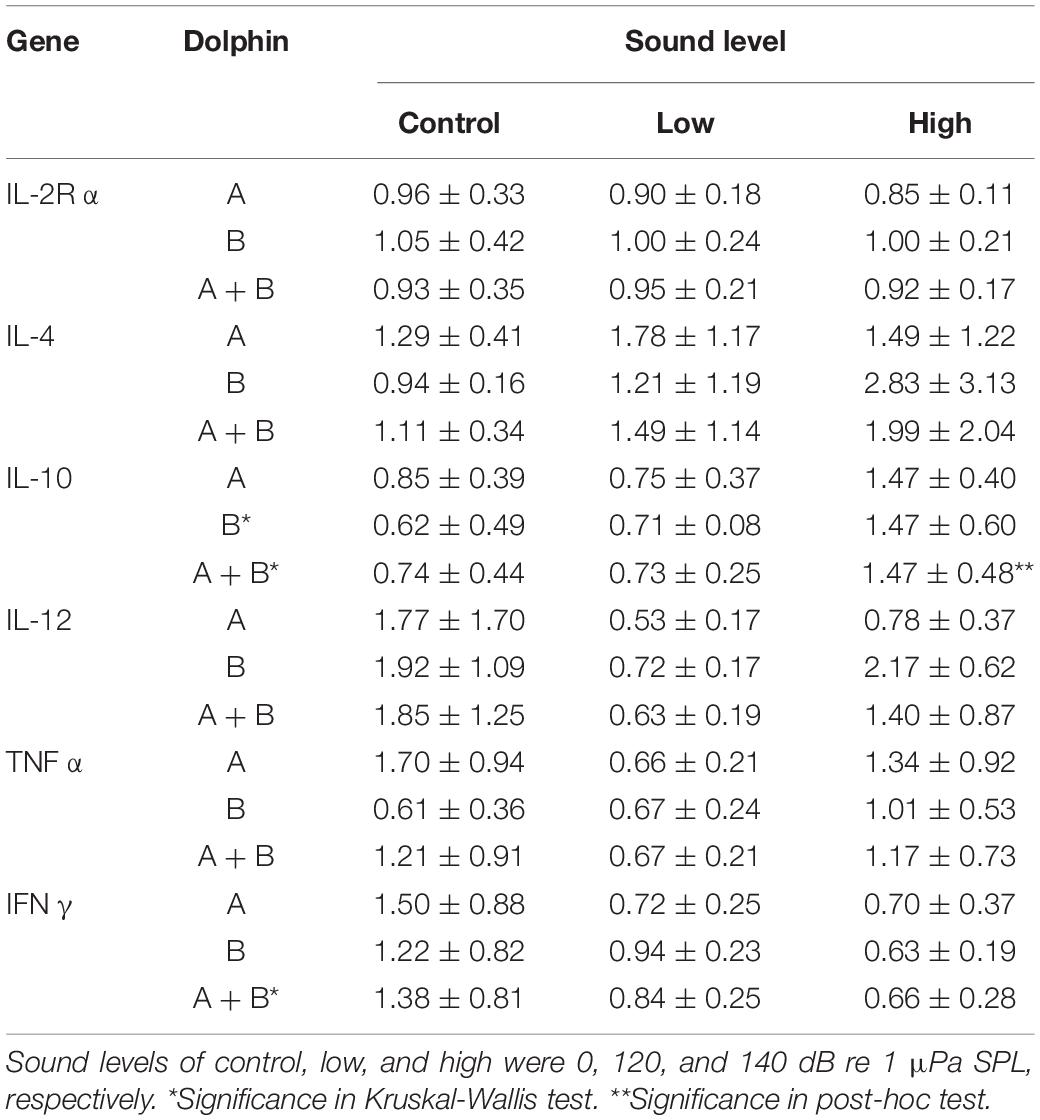
Table 3. Results of Kruskal-Wallis tests with post-hoc Dunn’s tests of the transcription ratio (mean ± SD) of post-exposure and pre-exposure samples.
Kruskal-Wallis tests and post-hoc tests showed the respiration rate was significantly higher in low and high sound exposure groups (7.2 ± 1.7 and 9.7 ± 1.2 times/5 min, respectively) than in control group (5.1 ± 0.7 times/5 min) in the first 5 min section in dolphin B but not in dolphin A (Low: 12.8 ± 0.6 times/5 min; high: 13.2 ± 2.2; control: 13.9 ± 1.9) nor pooled data. Kruskal-Wallis tests and post-hoc tests also showed that both dolphins significantly paid much more time on “examining” the transducer in control group (A: 11.2 ± 3.1 s, B: 10.5 ± 2.6 s) than in sound exposure groups (Low/High: A: 1.4 ± 1.4 s/1.4 ± 1.0 s, B: 2.2 ± 1.5 s/2.2 ± 1.2 s) in the first 5 min section. Compared to the data in the first 5 min, both dolphins spent no time on “examining” in high sound exposure group in the last 5 min. There were no significant differences found for other behavior analyses.
Discussion
This research investigated the effect of low-frequency multiple impulsive sounds on the cortisol and cytokine gene transcript profiles in blood and the behavioral response of captive bottlenose dolphins. It was identified that exposure to high-level sound may lead to changes in the neural-immune, endocrine, and behavioral parameters. Cetaceans exist near mammalian physiological limits and are exposed to challenges, such as those associated with prolonged fasting, deep diving, osmotic pressure and body temperature maintaining (Atkinson et al., 2015). These processes are managed by the endocrine system, and the disintegration of such systems may contribute to considerably lower fitness of an individual (Wright et al., 2007). Cortisol is the key glucocorticoid that is released in response to stressors, such as noise (Romano et al., 2004) to maintain physiological and biochemical homeostasis. Previous studies have showed that increased serum cortisol levels could be observed in captive beluga whales (Delphinapterus leucas) during physical examination (being separated from social group, weighed in an stretcher, and gastroscopy under manual restraint on deck) (Schmitt et al., 2010) and in free-ranging bottlenose dolphins (T. truncatus) following chase capture (being encircled in shallow water with a seine net and restrained) for capture-release health assessment (Hart et al., 2015). In this study, cortisol concentrations of both dolphins elevated significantly after a 30-min high-level sound exposure, which is consistent with findings from Hart et al. (2015) where cortisol concentrations were increased in 30 min following a stressful event. The results support the suggestions in previous studies that anthropogenic sound, the low-frequency multiple impulsive sound where the level is lower than the thresholds for onset of temporary threshold shift in dolphins in this study, could be a stressor for cetaceans (Romano et al., 2004; Rolland et al., 2012). If the stressor lasts only for a brief time, the cortisol upsurge contributes to keep normal physiologic function when the animal is controlling the effects of the stressor (e.g., fleeing unpleasant sounds causing foraging area abandonment). However, if cortisol levels persist elevated for extended period of time (exposure to high or cumulative noise levels for days to months), the high hormone levels can have negative effects on immune response, growth, and reproduction (Fair and Becker, 2000), causing the animal to potentially become more vulnerable when other stressors are present, such as microorganism infection, prey scarcity and competition.
Cortisol has been shown to affect numbers and distribution of leukocytes including particular subpopulations of lymphocytes as well as changes in immune functions in both animals and humans (Calcagni and Elenkov, 2006). IL-10 has regulatory and anti-inflammatory features that downregulates the production of proinflammatory cytokines, such as IL-2, IFNγ, and TNFα (Mosser and Zhang, 2008). Increase in IL-10 production can cause a shift in the CD4+ Th cells, from a Th1 profile, which functions in cell-mediated immune activities, to a Th2 profile, which is involved in antibody production. The positive correlation between IL-10 and cortisol in cetaceans has been reported (Müller et al., 2013). It was explained that free-ranging porpoises may encounter higher stress levels and more exposure to infectious disease than the animals in human care. In a capture-release health assessment study in wild beluga whales, a significant down-regulation for IFNγ in post-exam samples suggested an acute stressor during capture, handling and examinations (Unal et al., 2018). A previous human subject research study showed that the stress associated with taking academic exams causes a shift toward the Th2 response in healthy individuals, as seen by a significant increase in IL-10 production, a slight decrease in IFNγ production, and decrease in IFNγ/IL-10 ratio (Marshall Jr., Agarwal et al., 1998). In the present study, no measurements except the R of IFNγ in high group in dolphin B showed strong relationship with time. It indicated that there may be nearly no acclimation to the sound stimuli, possibly due to comparatively long test interval (3 days) with random order of sound levels. IL-10 gene transcription increased and IFNγ/IL-10 ratio was lower after a 30-min high-level sound exposure. Accompanied by elevated cortisol concentrations, the results indicated a sound-related stress-induced shift to Th2 immunity. The consequences of the shift away from Th1 response may lead to enhanced-susceptibility to infection and inflammatory or autoimmune diseases if the stress continues for a long period of time (Calcagni and Elenkov, 2006). Besides, it would be interesting to investigate further whether a dominant individual (dolphin B) shows elevated R of IL-12 under noise exposure and its implications.
Many odontocete species, such as bottlenose dolphins, present complex social behavior that helps in coping with environmental stressors, maintaining individual survival and reproductive success (Waples and Gales, 2002). Because responses to sound exposure are different between individuals and the response of one animal to the sounds may not be appropriate to represent its species, it has also been suggested that behavioral response studies should be conducted with as many animals as possible (Kastelein et al., 2013). In this study, three dolphins that belong to the same training group were used simultaneously in the sound exposure experiment, aiming to provide more ecologically valid stress model that shows whether immune and behavior changes appear following stressors met in routine social network. Dolphin A and dolphin B showed similar responses to the sound exposure, including paying less time on examining the transducer during sound exposure and spending no time on this behavior in the last 5 min when sound level was high. It should be noticed that no avoidance behavior (actively moving away from the transducer) was observed. A previous study showed that the respiration rate of a harbor porpoise increased in response to the pile driving sounds (Kastelein et al., 2013). In this study, dolphin B’s respiration rate increased even under low-level sound exposure in the first 5 min but not in dolphin A, although both dolphins’ cortisol levels elevated significantly after high-level sound exposure. It was suggested that respiratory rate of dolphins may not be a sensitive indicator of stress during noise presentation (Lyamin et al., 2011). Further research is warranted to understand if dominant individual shows more obvious response on respiration rate. Besides, the dolphins’ behaviors were evaluated as “normal” by trainers after each 30-min session, indicating that being exposed to the low-frequency multiple impulsive sound at the levels used in this study had no obvious continuing effect on the dolphins’ behavior. A previous pile-driving sound study also noticed the quick return to normal behavior in a harbor porpoise (Phocoena phocoena) (Kastelein et al., 2013). Therefore, it could be misinterpreted that the anthropogenic sounds used in this study have no apparent effect on the dolphins if physiological measurements, such as cortisol concentrations or cytokine gene transcription, were not performed.
It was hypothesized before the experiment that the ranges of R would be narrow in the control group since there was no sound effect. However, comparatively wider ranges of R in the control group of IFNγ and IL-12 in both dolphins and TNFα in dolphin A were noticed, while those of IL-4 were narrow (Table 3 and Figure 1). Accompanying the results in cortisol and behavior, this occurrence might reflect normal variations when three dolphins were in complex social network as mentioned above. A similar phenomenon has been reported that the shift in pro- and anti-inflammatory signaling was not regarded as being caused by differences in circulating cortisol concentrations in a genes and social behavior study (Cole et al., 2007). Intriguingly, it showed the opposite in low-level group in the present study. IL-12 induces proliferation and differentiation of T cells, and TNFα mediates cytotoxicity and cytokine secretions. The potential downregulation (R < 1) of these two proinflammatory cytokines would be a negative impact on health. When the high-level sound exposure may cause stress on dolphins, the low-level 800-Hz sound (mean received SPL 120 dB re 1 μPa) was initially hypothesized to be barely heard by dolphins according to previous studies (reviewed in 12), and the results indicated that even low-level anthropogenic sound might affect dolphins. It might be explained by the social cohesiveness of this dolphin group that may play a role in the individual responses. This brings up the questions of why the dolphins reacted in different ways when they encountered different levels of anthropogenic sounds and whether the low-level sound has an impact on dolphins’ health when it lasts for a long time. Because of experimental limitations, only two female bottlenose dolphins with five trials of each sound level were monitored in this study. More samples and more measurements, such as catecholamines, mineralocorticoid and heart rate are needed to allow us to identify modest effects from the experimental stimuli for all behavioral and neuro–endocrine–immune measurements tested.
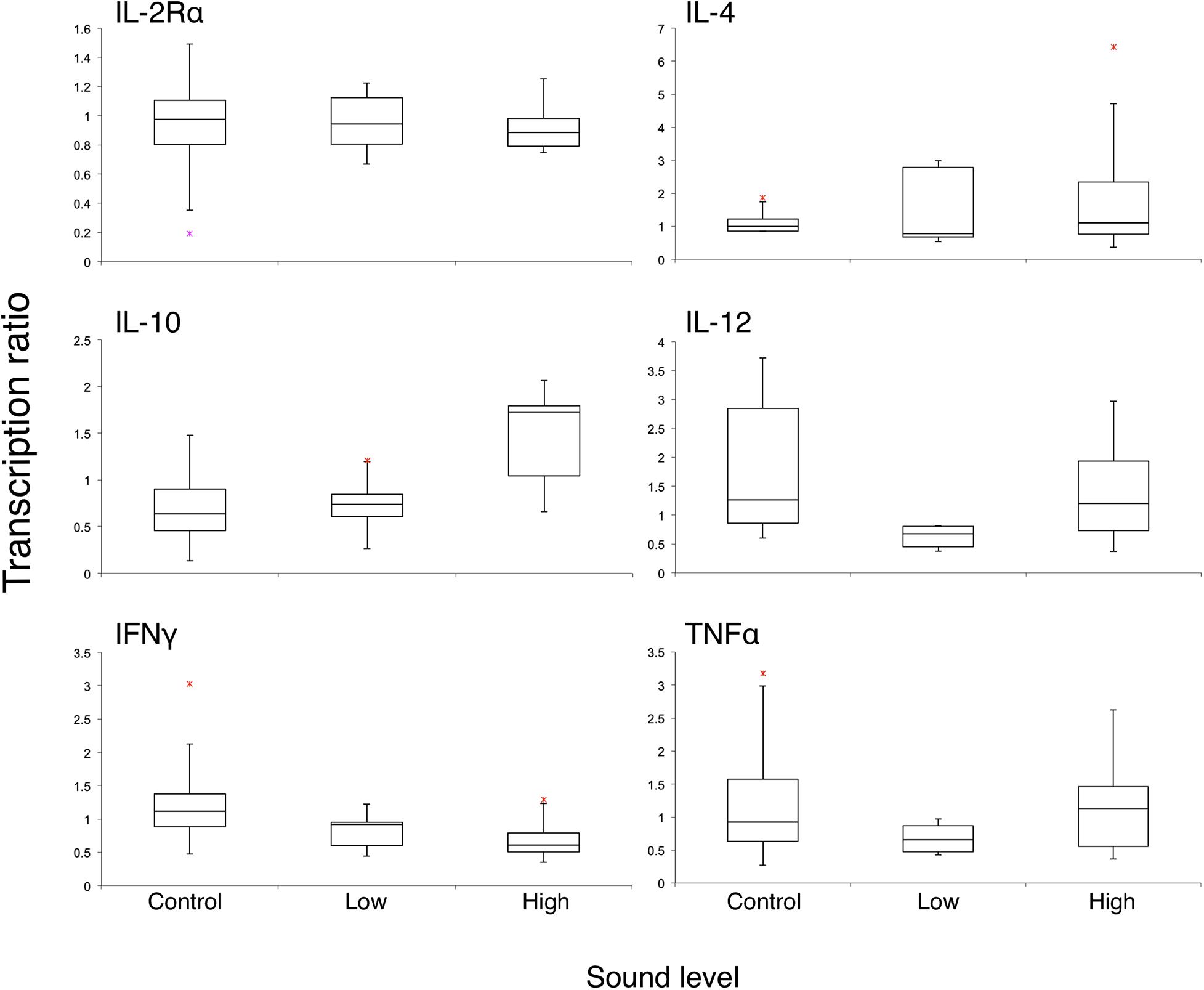
Figure 1. Box plot of transcription ratios of six immunologically relevant genes of two-dolphin pooled data from three different sound levels. Red asterisks are outliers defined as values more than the third quartile + 1.5 × IQR or less than the first quartile – 1.5 × IQR, where IQR is the interquartile range.
The noise exposure used in this study has characteristic features of offshore pile-driving sound including low frequency (main energy was contained below 1 kHz), serious impulses (40–50 strikes/min) and short time duration. Offshore pile driving for wind turbine installation with hydraulic hammers generates noise that has the potential to affect dolphin populations harmfully as it is detectable up to 40 km from the source (Kastelein et al., 2013). This is the first experiment to inspect the effects of pile driving-like sound on the endocrine and immune systems in cetaceans. It offers constructive information regarding sound exposure for free-ranging cetaceans through using captive cetaceans under controlled experimental conditions. Measurements of neuro–endocrine–immune and behavior parameters in the same animals before and after exposures of recording playbacks of offshore pile driving sounds warrant further investigation and future studies.
Data Availability Statement
The raw data supporting the conclusions of this article will be made available by the authors, without undue reservation.
Ethics Statement
The animal study was reviewed and approved by the Council of Agriculture of Taiwan.
Author Contributions
W-CY, C-FC, TM, JS, MB, and L-SC: conceptualization. W-CY, C-FC, Y-CC, C-RZ, I-HC, and I-FJ: wet lab experiments. W-CY, C-FC, Y-CC, C-RZ, and I-FJ: data analysis. W-CY, C-FC, JS, MB, and L-SC: supervision. W-CY, C-FC, Y-CC, C-RZ, and L-SC: writing. W-CY, C-FC, and L-SC: funding. All authors contributed to the article and approved the submitted version.
Funding
This work was supported by the Ministry of Science and Technology in Taiwan (MOST 108-2313-B-002-021 and MOST 109-2628-B-002-028).
Conflict of Interest
I-FJ was employed by Farglory Ocean Park.
The remaining authors declare that the research was conducted in the absence of any commercial or financial relationships that could be construed as a potential conflict of interest.
Acknowledgments
We thank the staffs of Farglory Ocean Park for sample collection.
Supplementary Material
The Supplementary Material for this article can be found online at: https://www.frontiersin.org/articles/10.3389/fmars.2021.606736/full#supplementary-material
References
Altmann, J. (1974). Observational study of behavior: sampling methods. Behaviour 49, 227–267. doi: 10.1163/156853974x00534
Atkinson, S., Crocker, D., Houser, D., and Mashburn, K. (2015). Stress physiology in marine mammals: how well do they fit the terrestrial model? J. Comp. Physiol. B 185, 463–486. doi: 10.1007/s00360-015-0901-0
Bustin, S. A., Benes, V., Garson, J. A., Hellemans, J., Huggett, J., Kubista, M., et al. (2009). The MIQE guidelines: minimum information for publication of quantitative real-time PCR experiments. Clin. Chem. 55, 611–622. doi: 10.1373/clinchem.2008.112797
Calcagni, E., and Elenkov, I. (2006). Stress system activity, innate and T helper cytokines, and susceptibility to immune-related diseases. Ann. N.Y. Acad. Sci. 1069, 62–76. doi: 10.1196/annals.1351.006
Chen, I. H., Chou, L. S., Chou, S. J., Wang, J. H., Stott, J., Blanchard, M., et al. (2015). Selection of suitable reference genes for normalization of quantitative RT-PCR in peripheral blood samples of bottlenose dolphins (Tursiops truncatus). Sci. Rep. 5:15425. doi: 10.1038/srep15425
Chen, I. H., Chou, L. S., Chou, S. J., Wang, J. H., Stott, J., Blanchard, M., et al. (2018). Sound exposure-induced cytokine gene transcript profile changes in captive bottlenose dolphin (Tursiops truncatus) blood identified by a probe-based qRT-PCR. J. Vet. Med. Sci. 80, 601–605. doi: 10.1292/jvms.17-0548
Chrousos, G. P., and Gold, P. W. (1992). The concepts of stress and stress system disorders: overview of physical and behavioral homeostasis. J. Am. Med. Assoc. 267, 1244–1252. doi: 10.1001/jama.1992.03480090092034
Cole, S. W., Hawkley, L. C., Arevalo, J. M., Sung, C. Y., Rose, R. M., and Cacioppo, J. T. (2007). Social regulation of gene expression in human leukocytes. Genome Biol. 8:R189. doi: 10.1186/gb-2007-8-9-r189
David, J. A. (2006). Likely sensitivity of bottlenose dolphins to pile-driving noise. Water Environ. J. 20, 48–54. doi: 10.1111/j.1747-6593.2005.00023
Erbe, C., Dunlop, R., and Dolman, S. (2018). “Effects of noise on marine mammals,” in Effects of Anthropogenic Noise on Animals, eds H. Slabbekoorn, R. J. Dooling, A. N. Popper, and R. R. Fay (New York, NY: Springer New York), 277–309.
Fair, P. A., and Becker, P. R. (2000). Review of stress in marine mammals. J. Aquatic Ecosyst. Stress Recovery 7, 335–354. doi: 10.1023/a:1009968113079
Hall, J. E. (2015). Guyton and Hall Textbook of Medical Physiology, 13th Edn. Philadelphia, PA: Saunders.
Hart, L. B., Wells, R. S., Kellar, N., Balmer, B. C., Hohn, A. A., Lamb, S. V., et al. (2015). Adrenal hormones in common bottlenose dolphins (Tursiops truncatus): influential factors and reference intervals. PLoS One 10:e0127432. doi: 10.1371/journal.pone.0127432
Houser, D. S., Gomez-Rubio, A., and Finneran, J. J. (2008). Evoked potential audiometry of 13 Pacific bottlenose dolphins (Tursiops truncatus gilli). Mar. Mammal Sci. 24, 28–41. doi: 10.1111/j.1748-7692.2007.00148.x
Kastelein, R. A., van Heerden, D., Gransier, R., and Hoek, L. (2013). Behavioral responses of a harbor porpoise (Phocoena phocoena) to playbacks of broadband pile driving sounds. Mar. Environ. Res. 92, 206–214. doi: 10.1016/j.marenvres.2013.09.020
Lyamin, O. I., Korneva, S. M., Rozhnov, V. V., and Mukhametov, L. M. (2011). Cardiorespiratory changes in beluga in response to acoustic noise. Doklady Biol. Sci. 440, 275–278. doi: 10.1134/s0012496611050218
Madsen, P. T., Wahlberg, M., Tougaard, J., Lucke, K., and Tyack, P. (2006). Wind turbine underwater noise and marine mammals: implications of current knowledge and data needs. Mar. Ecol. Prog. Ser. 309, 279–295. doi: 10.3354/meps309279
Marshall, G. D. Jr., Agarwal, S. K., Lloyd, C., Cohen, L., Henninger, E. M., and Morris, G. J. (1998). Cytokine dysregulation associated with exam stress in healthy medical students. Brain Behav. Immunity 12, 297–307. doi: 10.1006/brbi.1998.0537
Mooney, T. A., Yamato, M., and Branstetter, B. K. (2012). Hearing in cetaceans: from natural history to experimental biology. Adv. Mar. Biol. 63, 197–246. doi: 10.1016/B978-0-12-394282-1.00004-1
Mooney, T. A., Yang, W.-C., Yu, H.-Y., Ketten, D. R., and Jen, I. F. (2015). Hearing abilities and sound reception of broadband sounds in an adult Risso’s dolphin (Grampus griseus). J. Comp. Physiol. A 201, 751–761. doi: 10.1007/s00359-015-1011-x
Mosser, D. M., and Zhang, X. (2008). Interleukin-10: new perspectives on an old cytokine. Immunol. Rev. 226, 205–218. doi: 10.1111/j.1600-065X.2008.00706.x
Müller, S., Lehnert, K., Seibel, H., Driver, J., Ronnenberg, K., Teilmann, J., et al. (2013). Evaluation of immune and stress status in harbour porpoises (Phocoena phocoena): can hormones and mRNA expression levels serve as indicators to assess stress? BMC Vet. Res. 9:145. doi: 10.1186/1746-6148-9-145
NMFS (2016). Technical Guidance for Assessing the Effects of Anthropogenic Sound on Marine Mammal Hearing: Underwater Acoustic Thresholds for Onset of Permanent and Temporary Threshold Shifts. U.S. Dept. of Commer., NOAA. NOAA Technical Memorandum NMFS-OPR-55. Silver Spring, MD: National Marine Fisheries Service, 178.
Noda, K., Aoki, M., Akiyoshi, H., Asaki, H., Ogata, T., Yamauchi, K., et al. (2006). Effect of bovine lactoferrin on the immune responses of captive bottlenosed dolphins (Tursiops truncatus) being transported over long distances. Vet. Rec. 159, 885–888.
Nowacek, D. P., Thorne, L. H., Johnston, D. W., and Tyack, P. L. (2007). Responses of cetaceans to anthropogenic noise. Mammal Rev. 37, 81–115. doi: 10.1111/j.1365-2907.2007.00104.x
Padgett, D. A., and Glaser, R. (2003). How stress influences the immune response. Trends Immunol. 24, 444–448. doi: 10.1016/s1471-4906(03)00173-x
Pfaffl, M. W., Horgan, G. W., and Dempfle, L. (2002). Relative expression software tool (REST) for group-wise comparison and statistical analysis of relative expression results in real-time PCR. Nucleic Acids Res. 30:e36.
Ramirez, K. (1999). Animal Training: Successful Animal Management Through Positive Reinforcement. Chicago, IL: Shedd Aquarium Society.
Reidarson, T. H., and McBain, J. F. (1999). Hematologic, biochemical, and endocrine effects of dexamethasone on bottlenose dolphins (Tursiops truncatus). J. Zoo Wildl. Med. 30, 310–312.
Rolland, R. M., Parks, S. E., Hunt, K. E., Castellote, M., Corkeron, P. J., Nowacek, D. P., et al. (2012). Evidence that ship noise increases stress in right whales. Proc. R. Soc. Lond. B Biol. Sci. 279, 2363–2368. doi: 10.1098/rspb.2011.2429
Romano, T. A., Keogh, M. J., Kelly, C., Feng, P., Berk, L., Schlundt, C. E., et al. (2004). Anthropogenic sound and marine mammal health: measures of the nervous and immune systems before and after intense sound exposure. Can. J. Fish Aquat. Sci. 61, 1124–1134. doi: 10.1139/f04-055
Schmitt, T. L., St. Aubin, D. J., Schaefer, A. M., and Dunn, J. L. (2010). Baseline, diurnal variations, and stress-induced changes of stress hormones in three captive beluga whales, Delphinapterus leucas. Mar. Mammal Sci. 26, 635–647. doi: 10.1111/j.1748-7692.2009.00366.x
Thomas, J. A., Kastelein, R. A., and Awbrey, F. T. (1990). Behaviour and blood catecholamines of captive beluga whales during playbacks of noise from an oil drilling platform. Zoo Biol. 9, 393–402. doi: 10.1002/zoo.1430090507
Tsai, M. A., Chen, I. H., Wang, J. H., Chou, S. J., Li, T. H., Leu, M. Y., et al. (2017). A probe-based qRT-PCR method to profile immunological gene expression in blood of captive beluga whales (Delphinapterus leucas). PeerJ 5:e3840. doi: 10.7717/peerj.3840
Unal, E., Goertz, C. E. C., Hobbs, R. C., Suydam, R., and Romano, T. (2018). Investigation of molecular biomarkers as potential indicators of health in wild belugas (Delphinapterus leucas). Mar. Biol. 165:182. doi: 10.1007/s00227-018-3439-3
Waples, K. A., and Gales, N. J. (2002). Evaluating and minimising social stress in the care of captive bottlenose dolphins (Tursiops aduncus). Zoo Biol. 21, 5–26. doi: 10.1002/zoo.10004
Keywords: sound, cortisol, cytokine, behavior, dolphins, stress
Citation: Yang W-C, Chen C-F, Chuah Y-C, Zhuang C-R, Chen I-H, Mooney TA, Stott J, Blanchard M, Jen I-F and Chou L-S (2021) Anthropogenic Sound Exposure-Induced Stress in Captive Dolphins and Implications for Cetacean Health. Front. Mar. Sci. 8:606736. doi: 10.3389/fmars.2021.606736
Received: 15 September 2020; Accepted: 14 April 2021;
Published: 05 May 2021.
Edited by:
Lars Bejder, University of Hawaii at Manoa, United StatesReviewed by:
Nicholas Marc Kellar, National Oceanic and Atmospheric Administration (NOAA), United StatesLeila Soledade Lemos, Florida International University, United States
Copyright © 2021 Yang, Chen, Chuah, Zhuang, Chen, Mooney, Stott, Blanchard, Jen and Chou. This is an open-access article distributed under the terms of the Creative Commons Attribution License (CC BY). The use, distribution or reproduction in other forums is permitted, provided the original author(s) and the copyright owner(s) are credited and that the original publication in this journal is cited, in accordance with accepted academic practice. No use, distribution or reproduction is permitted which does not comply with these terms.
*Correspondence: Wei-Cheng Yang, eWFuZ3dlaWNoZW5nQG50dS5lZHUudHc=; Lien-Siang Chou, Y2hvdWxzQG50dS5lZHUudHc=