- 1School of Marine and Environmental Affairs, University of Washington, Seattle, WA, United States
- 2School of Oceanography, University of Washington, Seattle, WA, United States
- 3Institute for the Oceans and Fisheries, The University of British Columbia, Vancouver, BC, Canada
- 4Hakai Institute, Campbell River, BC, Canada
Zooplankton can serve as indicators of ecosystem health, water quality, food web structure, and environmental change, including those associated with climate change and ocean acidification (OA). Laboratory studies demonstrate that low pH and high pCO2 associated with OA can significantly affect the physiology and survival of zooplankton, with differential responses among taxa. While laboratory studies can be indicative of zooplankton response to OA, in situ responses will ultimately determine the fate of populations and ecosystems. In this perspective, we compare expectations from experimental studies with observations made in Puget Sound (Washington, United States), a highly dynamic estuary with known vulnerabilities to low pH and high pCO2. We found little association between empirical measures of in situ pH and the abundance of sensitive taxa as revealed by meta-analysis, calling into question the coherence between experimental studies and field observations. The apparent mismatch between laboratory and field studies has important ramifications for the design of long-term monitoring programs and interpretation and use of the data produced. Important work remains to be done to connect traits that are sensitive to OA with those that are ecologically relevant and reliably observable in the field.
Introduction
Zooplankton are excellent indicators of ecosystem health, food web function, and water quality (Beaugrand, 2005) because their short life cycles allow them to respond to seasonal variations and abrupt changes in environmental conditions. While the response of zooplankton to ocean acidification (OA) is understudied compared with that of many benthic species, the critical importance of zooplankton to marine food webs underscores the need to better understand their sensitivities to changing seawater carbonate chemistry. Laboratory studies show that low pH and high pCO2 associated with OA can significantly affect the survival, growth, calcification, development, and reproduction of planktonic organisms (Kroeker et al., 2013; Busch et al., 2014; Cripps et al., 2014; Busch and McElhany, 2016; McLaskey et al., 2016; Tills et al., 2016; Bednaršek et al., 2017; Lischka and Riebesell, 2017), and that sensitivity to current and projected levels of pH and pCO2 can vary substantially among zooplankton taxa. Understanding the ecological context in which these responses occur is important, given the co-occurring environmental stressors (e.g., increasing temperature and oxygen stress) to which zooplankton are exposed.
Here, we present the results of an exploratory study to assess the level of correspondence between the results of experimental studies and field observations of zooplankton abundance in a natural setting. Specifically, we asked whether relative sensitivities to pCO2 as reported in the literature and revealed through meta-analysis could explain a significant proportion of the variation in zooplankton abundance at two time points, across six stations, in Puget Sound, Washington. We focused on Puget Sound because pH and pCO2 are known to vary substantially across short spatial and temporal scales, often reaching values that are more extreme than in open-ocean environments (Feely et al., 2010; Bianucci et al., 2018; Pelletier et al., 2018), and because the fate of zooplankton in nearshore environments such as this imposes critical controls on food webs and fisheries in coastal environments. We interpret our results in the context of long-term monitoring studies and their utility in detecting biological response to ocean change.
Evidence From Controlled Experiments
To better understand zooplankton sensitivities to OA conditions in Puget Sound, we performed a meta-analysis of experimental studies reporting responses of seasonally abundant, holoplanktonic taxa known to occur in Washington State’s marine waters. Meroplanktonic larvae of shellfish, including crabs, were also included due to their regional economic and ecological importance. Studies published in peer-reviewed journals over the 10-year period from January 2009 to February 2019 were included. We modified the approach of Wittmann and Pörtner (2013) to determine the effect of increasing pCO2 on major taxonomic groups and to compare sensitivity across groups. The coarse nature of our taxonomic groupings was necessitated by the relative scarcity of published results and was similar to the broad taxonomic levels used by Wittmann and Pörtner (2013). For each species, we interpolated and extrapolated data to help compensate for missing values and cleaned the dataset to eliminate duplicative response categories. For example, because weight and diameter are both measures associated with growth, only one was used in the meta-analysis, thereby reducing the likelihood of over-representation of response categories by including only one experiment per response category per study. Thirty-six studies met our criteria for inclusion in the meta-analysis, representing 17 species and eight taxonomic groups.
Negative responses increased with increasing levels of pCO2 across taxonomic groups, with the exception of shrimp and larvaceans (Figure 1). Pteropods emerged as the taxon most sensitive to OA, showing negative effects at pCO2 levels as low as 530 μatm. Copepods were the second-most sensitive group, with reproduction and survival negatively affected at pCO2 levels as low as 824 μatm. Larval bivalve calcification, growth, development, reproduction, and survival were negatively affected, with calcification affected at pCO2 levels as low as 545 μatm. Shrimp were the only taxon to show positive response to OA conditions, but this was not consistent across studies. Krill development, growth, metabolism, and survival were all negatively affected at pCO2 levels as low as 956 μatm, while crab larvae showed tolerance up to pCO2 levels of 1,361 μatm. Jellyfish growth was affected at pCO2 levels above 1,000 μatm, but other response categories showed no effect. Larvaceans showed the least sensitivity, with no significant response to the levels of pCO2 tested. We note, however, that our meta-analysis included only one study of larvaceans.
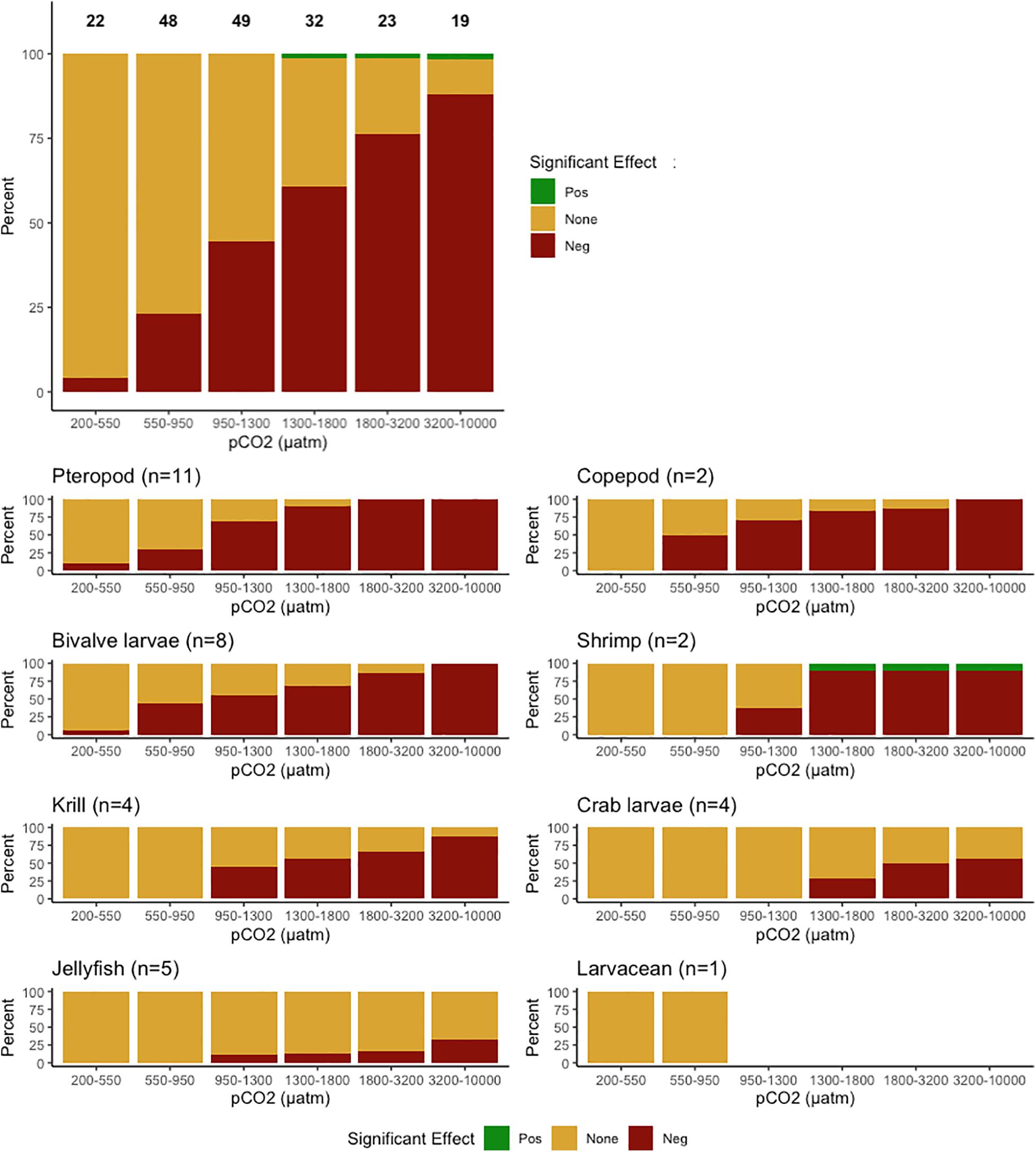
Figure 1. Top panel: Percent (%) of representative experiments across all taxonomic groups (pteropod, copepod, bivalve larvae, shrimp, krill, crab larvae, jellyfish, and larvacean) exhibiting positive (green), negative (red), or no significant response (yellow) to elevated levels of pCO2 (μatm). The number above each bar indicates the number of experiments analyzed for each level of pCO2, not including interpolated or extrapolated data. Bottom panels: Responses to varying levels of pCO2 by taxonomic group, with n representing the number of studies included in the analysis.
Pooling data across all taxa revealed that calcification was the response category most sensitive to increasing pCO2, followed by development, growth, reproduction, survival, and metabolism, in that order.
Matching Experimental Data With Field Observations
To compare results of the meta-analysis with field observations, we collected zooplankton via vertical tows and used standard techniques to characterize water chemistry at six stations in Puget Sound in 2017 from June 23 to 30 and again from August 25 to September 01 (hereafter referred to as June and August). The sampling stations and dates were chosen to cover a range of physical and chemical conditions, targeting stations where high pCO2 or low pH had been previously reported (Feely et al., 2010; McLaskey et al., 2016; Pelletier et al., 2018). We chose abundance as our response variable because abundance or its co-variate, biomass, is the most commonly measured variable in long-term monitoring studies, including those that have been used to detect changes due to ocean condition (e.g., Hays et al., 2005; Mackas and Beaugrand, 2010; McKinstry and Campbell, 2018). Moreover, abundance has been used to detect response to change in short-term studies of marine microbes (Currie et al., 2017; Rahlff et al., 2021).
Twenty-five taxonomic groups of zooplankton were represented in our samples. Copepods were abundant across stations, accounting for 70% of total abundance across all samples, while larvaceans were abundant at two stations in August, accounting for 15% across all samples.
To compare sensitivities between experimental and field settings, we used the taxon-specific sensitivities revealed by the meta-analysis to infer a hierarchical range of susceptibilities to pCO2. We grouped taxa into four categories, with pteropods (and all other gastropods), copepods, and bivalve larvae designated as “most susceptible”; shrimp and krill as “susceptible”; crab larvae, jellyfish, and larvaceans as “least susceptible”; and all other taxa as “insufficient data.” The distribution of differentially susceptible taxa varied across stations and sampling points (Figure 2), but overall we detected no clear association between zooplankton abundance and sensitivity as inferred from meta-analysis.
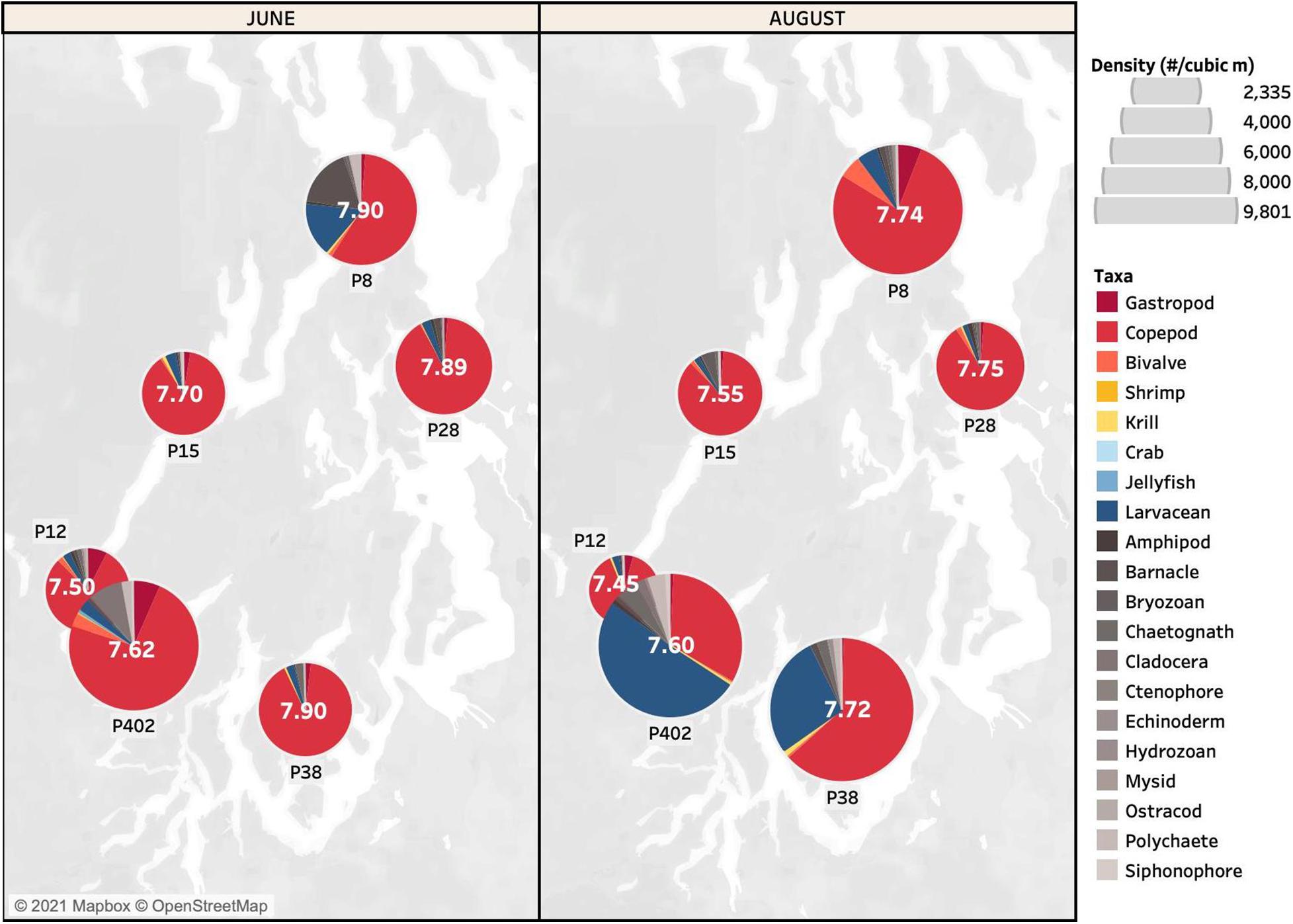
Figure 2. Total abundance of zooplankton (individuals/m3) by broad taxonomic grouping at six stations established by the Puget Sound Regional Synthesis Model program (P8, P12, P15, P28, P38, and P402) sampled in Puget Sound, WA, United States in June and August 2017. Isopods and arachnids were recorded three or fewer times and were omitted. The color of each taxon is based on estimated levels of susceptibility to ocean acidification derived from the meta-analysis: red taxa are most susceptible, yellow taxa are susceptible, blue taxa are least susceptible, and gray taxa have insufficient data. The mean pH for each station is recorded in white in the center of each pie.
The BIOENV function (R package “vegan”: Clarke and Ainsworth, 1993) was used to estimate the combination of environmental variables most highly correlated with the biological matrix. The best fit model did not include pCO2 or its covariate, pH, but instead included a combination of minimum temperature, maximum dissolved oxygen, and minimum fluorescence. Research indicates that zooplankton are negatively affected by pCO2 conditions that currently occur in Puget Sound, including conditions that were observed in this study (Barton et al., 2012; McLaskey et al., 2016; Bednaršek et al., 2017, 2020c). Our results, however, did not suggest that zooplankton abundance was responsive to low pH or waters undersaturated with respect to Ωar; if effects were present, they were not detectable by our methodology. The mean values of pH observed across stations ranged from 7.45 to 7.90; for comparison, the pH predicted for the surface ocean by the end of this century is 7.75 (IPCC, 2014).
Discussion
Zooplankton abundance as measured in the field did not reflect evidence from laboratory studies regarding organismal response to low pH/high pCO2. Taxa reported to be most susceptible to low pH in the laboratory reached high abundances at stations with pH as low as 7.45. Our analysis revealed that temperature, dissolved oxygen, and fluorescence, but not pH, were leading environmental variables influencing zooplankton abundance at the locations and dates sampled.
Zooplankton abundance tends to be positively associated with primary production (or its correlate, chlorophyll fluorescence), and our observations confirm the importance of primary production to zooplankton abundance in Puget Sound. This strong positive relationship is consistent with evidence that food availability can mediate organismal response to OA (Thomsen et al., 2013; Pansch et al., 2014; Ramajo et al., 2016). We note, however, that this is not always the case: for example, Brown et al. (2018, 2020) found that food sufficiency exacerbated the negative effects of low pH on organismal performance. Given our limited dataset, the relationship between food availability and zooplankton abundance in our study should be interpreted with caution with respect to zooplankton response to OA.
Although several of our stations were similar in the physical and chemical properties we measured, their zooplankton communities differed, especially in our samples from August. Notably, the stations differ in depth, but according to our analyses, depth did not appear as a significant explanatory factor. Factors that we did not measure, such as life-cycle timing, competition and predation, and physical factors such as advection, could contribute to differences in community composition. Moreover, while all species considered in this study inhabit Washington waters, most studies included in the meta-analysis used specimens collected from outside of our study area. Consequently, we cannot evaluate the potential influence of local adaptation, including changes in gene expression that can act on short timescales. Accounting for local adaptation will be important in any study that strives to make inferences about the response of local populations to OA conditions, but the difficulties of measuring local adaptation at meaningful scales remain considerable.
The expression of behavioral traits likely plays important but unstudied roles in determining differential sensitivity to OA in natural habitats. Some zooplankton species exhibit behaviors that can influence their ability to tolerate or avoid stressful conditions. Copepods, for example, have been shown to actively adjust their position or motility in response to a variety of stimuli, including turbulent flow (Michalec et al., 2017), light (Martynova and Gordeeva, 2010), pollutants (Michalec et al., 2013), and hypoxia (Keister and Tuttle, 2013), while echinoderm larvae can alter their swimming behavior to avoid low pH (Maboloc et al., 2020). Our data collection methods almost certainly obscured any behavioral responses that might have occurred.
Moreover, we cannot estimate the duration of individual exposure to specific levels of pH or other stressors. While seawater pH varies seasonally in Puget Sound (Feely et al., 2010; Pelletier et al., 2018), variation also occurs over diel cycles and short-term changes in circulation (Murray et al., 2015). Variation in the duration of exposure could partially explain why the meta-analysis revealed copepods to have high sensitivity to OA but our field observations did not. Zooplankton species and life stages with stronger vertical migration capabilities have been associated with higher tolerance to low pH because they experience a wide range of pH daily (Lewis et al., 2013). Our decision to identify samples to higher-order taxonomic levels (i.e., not to genus or species) allowed broad assessment across taxa, but constrained our ability to detect species-specific differences in response to environmental conditions, including those caused by differences in behavior.
Copepods dominated nearly every station we sampled, and much of the mismatch we observed between experimental and field observations could be attributable to our categorization of copepods as “most susceptible.” A more detailed look conceivably would reveal differential responses among species and life stages of copepods, not all of which are likely to be highly susceptible to OA. Our analysis failed to capture variation at this level, and in assigning all copepods to the same category, we could have overestimated the abundance of highly susceptible taxa. This observation suggests that, for monitoring programs, sampling to the lowest possible taxonomic level is advisable. It also cautions against the use of coarse taxonomic data to infer ecological response or for use as input to numerical models.
New research that has been published since the completion of our meta-analysis suggests that our results could over- or underestimate susceptibility among the taxa we included. One such study demonstrates that early life stages of a common copepod in Puget Sound—a taxon in the most susceptible category according to our meta-analysis—are generally tolerant of short-term direct effects of OA (McLaskey et al., 2019). Another study found that larval crabs—a taxon in the least susceptible category—may be more sensitive than indicated by prior laboratory studies (Bednaršek et al., 2020a). These apparent discrepancies likely result from a combination of factors, including a relatively small but growing literature, differential responses among life-history stages, and the short duration of our field sampling.
Over the course of this study, zooplankton at the stations sampled were exposed to a wide range of environmental conditions, including pH as low as 7.30 and Ωar as low as 0.32, conditions that could be expected to elicit responses among susceptible taxa. According to our meta-analysis, survival is among the least sensitive response categories, suggesting that changes in abundance due to differential survival could take time to grow to detectable levels. Sub-lethal effects may emerge more readily, but our data indicate that sublethal effects, if operative in these populations, were not of sufficient magnitude or duration to cause measurable declines in population abundance over the period sampled. Despite the limited temporal duration of our observations, we expected that such effects may have emerged across sites because the large spatial differences in seawater conditions that we sampled across are generally consistent year after year (Feely et al., 2010; McLaskey et al., 2016; Pelletier et al., 2018). This observation is important in the context of long-term monitoring programs that use measures of zooplankton abundance as indicators of environmental conditions. The strength of inferences made possible by monitoring data is likely to be dependent on the strength of the relationship(s) between OA conditions and the abundance of specific taxa. It is important that the scale of the response variable is adequate to reveal effects over the temporal and spatial scales sampled.
Even so, abundance is a logical first factor to examine zooplankton response to OA based on the expectation that significant effects of low pH or high pCO2 on development, reproduction, and other traits that influence individual fitness ultimately will be reflected in measures of population abundance. Moreover, changes in abundance can serve as an indicator of zooplankton response to OA. For example, Smith et al. (2016) used natural gradients in CO2 to show significant reductions in zooplankton biomass and abundance under high CO2 conditions. Other taxa have shown similar declines in abundance in response to increasing pCO2 or acidity (Hall-Spencer et al., 2008; Cigliano et al., 2010; Kroeker et al., 2011). Measures of abundance are commonly used for zooplankton observations in time series, and relative shifts in species abundances have been used extensively to examine zooplankton responses to climate (e.g., Mackas et al., 2007; Mackas and Beaugrand, 2010; Peterson et al., 2017) and as input to models of food web and ecosystem response to OA (Busch et al., 2013; Marshall et al., 2017). Abundance data can also capture shifts in a species’ center of abundance, indicating the directionality and magnitude of responses to climate change (Chivers et al., 2017). Hence, understanding the utility and limitations of abundance measures to broader environmental and ecological inquiries is essential to interpretation of empirical studies and to natural resource management. Importantly, the results of our study suggest that the relationship between measures of zooplankton abundance and seawater pH is not simple, emphasizing the utility of intensive sampling over longer time periods to elucidate underlying relationships. Longer time series that pair chemical and biological observations and distinguish the effects of seawater carbonate chemistry from factors such as temperature and dissolved oxygen will improve attribution of biological effects among co-occurring stressors (Doo et al., 2020). Our results underscore the importance of long-term monitoring of species abundance to determine the direction and magnitude of change associated with climate and OA.
Low statistical power is common among small datasets, and interpretation of our results is further limited by the existing literature. Many zooplankton monitoring efforts lack long-term and consistent data even though it is increasingly recognized that decades-long time series will be needed to confidently identify the effects of OA on zooplankton abundance. Our findings corroborate this by demonstrating that snapshots of zooplankton abundance in relation to seawater pH can fail to detect effects of pH on abundance and community composition, even when spatial gradients in pH are relatively steep and conditions differ sharply among sampling locations.
Although abundance is more easily and frequently measured in field settings, other variables could be more informative. Measuring calcification is a clear choice because of its known sensitivity to low values of Ωar and the relative wealth of relevant laboratory studies concerning the ability of organisms to create calcium carbonate structures under OA conditions. Recent research studying in situ impacts on calcification found reduced calcification in pteropods collected in the California Current Ecosystem (Mekkes et al., 2021) and severe dissolution in pteropods in our study area (Bednaršek et al., 2020b). Importantly, some measures of calcification can be made on preserved samples, potentially alleviating constraints of some monitoring programs. However, calcification is important to only a subset of key zooplankton taxa, and even for calcifiers, is only one of many processes affected by OA. Where possible, adding biological measures in addition to abundance may provide key insights into sensitivity and add substantial value to monitoring programs. Ultimately, including mechanistic studies that can reveal cause-and-effect relationships between OA conditions and biological response will be more informative than correlation-based studies alone.
Overall, we found that observations of zooplankton abundance and community composition in response to pH and pCO2 did not match experimental evidence from the literature. We propose that this mismatch could be explained by a number of factors, including response to co-occurring physical factors such as temperature and dissolved oxygen, data limitations, and biological and ecological factors, including food availability, competition and predation, local adaptation, life history stage, and the taxonomic level at which data are aggregated. Accounting for such factors in monitoring studies should improve our ability to attribute change to specific causes. Finally, and perhaps most importantly, our results underscore the importance of testing evidence from laboratory studies against the response of natural populations in defined settings. Context clearly matters, and assumptions to the contrary are likely to lead to equivocal or even misleading findings.
Data Availability Statement
The raw data supporting the conclusions of this article will be made available by the authors, without undue reservation.
Author Contributions
KK co-designed the research, performed the meta-analysis and field sampling, analyzed results, and led preparation of the manuscript. TK co-designed and supervised the research. JK contributed to the research design and supported laboratory analysis. AM supported the research cruises, collaborated on collection of the field data, and processed environmental samples. All authors contributed to preparation of the manuscript and approved the version submitted.
Funding
TK, AM, and JK were partially supported by funding provided by the Washington Ocean Acidification Center. JK was partially supported by the Washington Sea Grant. AM was partially supported by an NSF GRFP fellowship.
Conflict of Interest
The authors declare that the research was conducted in the absence of any commercial or financial relationships that could be construed as a potential conflict of interest.
The handling editor declared a past co-authorship with one of the authors, AM.
Acknowledgments
This work represents research performed in partial fulfillment of the Master’s degree of KK (Keil, 2021). We thank R. P. Kelly and R. Gallego for analytical assistance and A. Winans and B. Herrmann for laboratory assistance. We gratefully acknowledge the outstanding support of the crew of the R/V Clifford A. Barnes.
References
Barton, A., Hales, B., Waldbusser, G. G., Langdon, C., and Feely, R. A. (2012). The Pacific oyster, Crassostrea gigas, shows negative correlation to naturally elevated carbon dioxide levels: implications for near-term ocean acidification effects. Limnol. Oceanogr. 57, 698–710. doi: 10.4319/lo.2012.57.3.0698
Beaugrand, G. (2005). Monitoring pelagic ecosystems using plankton indicators. ICES J. Mar. Sci. 62, 333–338. doi: 10.1016/j.icesjms.2005.01.002
Bednaršek, N., Feely, R. A., Beck, M. W., Alin, S. R., Siedlecki, S. A., Calosi, P., et al. (2020a). Exoskeleton dissolution with mechanoreceptor damage in larval Dungeness crab related to severity of present-day ocean acidification vertical gradients. Sci. Total Environ. 716:136610. doi: 10.1016/j.scitotenv.2020.136610
Bednaršek, N., Feely, R. A., Tolimieri, N., Hermann, A. J., Siedlecki, S. A., Waldbusser, G. G., et al. (2017). Exposure history determines pteropod vulnerability to ocean acidification along the US West Coast. Sci. Rep. 7:4526. doi: 10.1038/s41598-017-03934-z
Bednaršek, N., Newton, J. A., Beck, M. W., Alin, S. R., Feely, R. A., Christman, N. R., et al. (2020b). Severe biological effects under present-day estuarine acidification in the seasonally variable Salish Sea. Sci. Total Environ. 765:142689. doi: 10.1016/j.scitotenv.2020.142689
Bednaršek, N., Pelletier, G., Ahmed, A., and Feely, R. A. (2020c). Chemical exposure due to anthropogenic ocean acidification increases risks for estuarine calcifiers in the Salish sea: biogeochemical model scenarios. Front. Mar. Sci. 7:580. doi: 10.3389/fmars.2020.00580
Bianucci, L., Long, W., Khangaonkar, T., Pelletier, G., Ahmed, A., Mohamedali, T., et al. (2018). Sensitivity of the regional ocean acidification and carbonate system in Puget Sound to ocean and freshwater inputs. Elem. Sci. Anth. 6:22. doi: 10.1525/elementa.151
Brown, N. E. M., Bernhardt, J. R., Anderson, K. M., and Harley, C. D. G. (2018). Increased food supply mitigates ocean acidification effects on calcification but exacerbates effects on growth. Sci. Rep. 8:9800. doi: 10.1038/s41598-018-28012-w
Brown, N. E. M., Bernhardt, J. R., and Harley, C. D. G. (2020). Energetic context determines species and community responses to ocean acidification. Ecology 101:e03073. doi: 10.1002/ecy.3073
Busch, D. S., Harvey, C. J., and McElhany, P. (2013). Potential impacts of ocean acidification on the puget sound food web. ICES J. Mar. Sci. 70, 823–833. doi: 10.1093/icesjms/fst061
Busch, D. S., Maher, M., Thibodeau, P., and McElhany, P. (2014). Shell condition and survival of puget sound pteropods are impaired by ocean acidification conditions. PLoS One 9:e105884. doi: 10.1371/journal.pone.0105884
Busch, D. S., and McElhany, P. (2016). Estimates of the Direct Effect of Seawater pH on the Survival Rate of Species Groups in the California Current Ecosystem. PLoS One 11:e0160669. doi: 10.1371/journal.pone.0160669
Chivers, W. J., Walne, A. W., and Hays, G. C. (2017). Mismatch between marine plankton range movements and the velocity of climate change. Nat. Commun. 8:14434. doi: 10.1038/ncomms14434
Cigliano, M., Gambi, M. C., Rodolfo-Metalpa, R., Patti, F. P., and Hall-Spencer, J. M. (2010). Effects of ocean acidification on invertebrate settlement at volcanic CO2 vents. Mar. Biol. 157, 2489–2502. doi: 10.1007/s00227-010-1513-6
Clarke, K. R., and Ainsworth, M. (1993). A method of linking multivariate community structure to environmental variables. Mar. Ecol. Progr.Ser. 92, 205–219. doi: 10.3354/meps092205
Cripps, G., Lindeque, P., and Flynn, K. J. (2014). Have we been underestimating the effects of ocean acidification in zooplankton? Glob. Chang. Biol. 20, 3377–3385. doi: 10.1111/gcb.12582
Currie, A. R., Tait, K., Parry, H., de Francisco-Mora, B., Hicks, N., Osborn, A. M., et al. (2017). Marine microbial gene abundance and community composition in response to ocean acidification and elevated temperature in two contrasting coastal marine sediments. Front. Microbiol. 8:1599. doi: 10.3389/fmicb.2017.01599
Doo, S. S., Kealoha, A., Andersson, A., Cohen, A. L., Hicks, T. L., Johnson, Z. I., et al. (2020). The challenges of detecting and attributing ocean acidification impacts on marine ecosystems. ICES J. Mar. Sci. 77, 2411–2422. doi: 10.1093/icesjms/fsaa094
Feely, R. A., Alin, S. R., Newton, J., Sabine, C. L., Warner, M., Devol, A., et al. (2010). The combined effects of ocean acidification, mixing, and respiration on pH and carbonate saturation in an urbanized estuary. Estuarine, Coastal Shelf Sci. 88, 442–449. doi: 10.1016/j.ecss.2010.05.004
Hall-Spencer, J. M., Rodolfo-Metalpa, R., Martin, S., Ransome, E., Fine, M., Turner, S. M., et al. (2008). Volcanic carbon dioxide vents show ecosystem effects of ocean acidification. Nature 454, 96–99. doi: 10.1038/nature07051
Hays, G. C., Richardson, A. J., and Robinson, C. (2005). Climate change and marine plankton. Trends Ecol. Evol. 20, 337–344. doi: 10.1016/j.tree.2005.03.004
IPCC, (2014). “Climate change 2014: synthesis report,” in Contribution of Working Groups I, II and III to the Fifth Assessment Report of the Intergovernmental Panel on Climate Change, eds Core Writing Team Pachauri, R. K., and Meyer, L. A., (Geneva: IPCC), 151.
Keil, K. E. (2021). Estimating Relative Sensitivities of Zooplankton to Ocean Acidification and Comparing to Observations in situ. Master’s Thesis. Seattle (WA): University of Washington.
Keister, J. E., and Tuttle, L. B. (2013). Effects of bottom-layer hypoxia on spatial distributions and community structure of mesozooplankton in a sub-estuary of puget sound, Washington, U.S.A. Limnol. Oceanogr. 58, 667–680. doi: 10.4319/lo.2013.58.2.0667
Kroeker, K. J., Kordas, R. L., Crim, R., Hendriks, I. E., Ramajo, L., Singh, G. S., et al. (2013). Impacts of ocean acidification on marine organisms: quantifying sensitivities and interaction with warming. Glob. Chang. Biol. 19, 1884–1896. doi: 10.1111/gcb.12179
Kroeker, K. J., Micheli, F., Gambi, M. C., and Martz, T. R. (2011). Divergent ecosystem responses within a benthic marine community to ocean acidification. PNAS 108, 14515–14520. doi: 10.1073/pnas.1107789108
Lewis, C. N., Brown, K. A., Edwards, L. A., Cooper, G., and Findlay, H. S. (2013). Sensitivity to ocean acidification parallels natural pCO2 gradients experienced by Arctic copepods under winter sea ice. PNAS 110, E4960–E4967. doi: 10.1073/pnas.1315162110
Lischka, S., and Riebesell, U. (2017). Metabolic response of Arctic pteropods to ocean acidification and warming during the polar night/twilight phase in Kongsfjord (Spitsbergen). Polar Biol. 40, 1211–1227. doi: 10.1007/s00300-016-2044-5
Maboloc, E., Batzel, G., Grünbaum, D., and Chan, K. (2020). Vertical distribution of echinoid larvae in pH stratified water columns. Mar. Biol. 167:13. doi: 10.1007/s00227-019-3629-7
Mackas, D. L., Batten, S., and Trudel, M. (2007). Effects on zooplankton of a warmer ocean: recent evidence from the Northeast Pacific. Progr. Oceanogr. 75, 223–252. doi: 10.1016/j.pocean.2007.08.010
Mackas, D. L., and Beaugrand, G. (2010). Comparisons of zooplankton time series. J. Mar. Syst. 79, 286–304. doi: 10.1016/j.jmarsys.2008.11.030
Marshall, K. N., Kaplan, I. C., Hodgson, E. E., Hermann, A., Busch, D. S., McElhany, P., et al. (2017). Risks of ocean acidification in the California current food web and fisheries: ecosystem model projections. Glob. Chang. Biol. 23, 1525–1539. doi: 10.1111/gcb.13594
Martynova, D. M., and Gordeeva, A. V. (2010). Light-dependent behavior of abundant zooplankton species in the White Sea. J. Plankton Res. 32, 441–456. doi: 10.1093/plankt/fbp144
McKinstry, C. A. E., and Campbell, R. W. (2018). Seasonal variation of zooplankton abundance and community structure in Prince William Sound, Alaska, 2009–2016. Deep Sea Res. Part II Topical Stud. Oceanogr. 147, 69–78. doi: 10.1016/j.dsr2.2017.08.016
McLaskey, A. K., Keister, J. E., McElhany, P., Olson, M. B., Busch, D. S., Maher, M., et al. (2016). Development of Euphausia pacifica (krill) larvae is impaired under pCO2 levels currently observed in the Northeast Pacific. Mar. Ecol. Progr. Ser. 555, 65–78. doi: 10.3354/meps11839
McLaskey, A. K., McElhany, P., Busch, D. S., Maher, M., Winans, A. K., and Keister, J. E. (2019). Early life stages of Calanus pacificus are neither exposed nor sensitive to low pH waters. J. Plankton Res. 41, 893–896. doi: 10.1093/plankt/fbz059
Mekkes, L., Renema, W., Bednaršek, N., Alin, S. R., Feely, R. A., Huisman, J., et al. (2021). Pteropods make thinner shells in the upwelling region of the California current ecosystem. Sci. Rep. 11:1731. doi: 10.1038/s41598-021-81131-9
Michalec, F.-G., Fouxon, I., Souissi, S., and Holzner, M. (2017). Zooplankton can actively adjust their motility to turbulent flow. PNAS 114, E11199–E11207. doi: 10.1073/pnas.1708888114
Michalec, F.-G., Holzner, M., Menu, D., Hwang, J.-S., and Souissi, S. (2013). Behavioral responses of the estuarine calanoid copepod Eurytemora affinis to sub-lethal concentrations of waterborne pollutants. Aquat. Toxicol. 138–139, 129–138. doi: 10.1016/j.aquatox.2013.05.007
Murray, J. W., Roberts, E., Howard, E., O’Donnell, M., Bantam, C., Carrington, E., et al. (2015). An inland sea high nitrate-low chlorophyll (HNLC) region with naturally high pCO2. Limnol. Oceanogr. 60, 957–966. doi: 10.1002/lno.10062
Pansch, C., Schaub, I., Havenhand, J., and Wahl, M. (2014). Habitat traits and food availability determine the response of marine invertebrates to ocean acidification. Glob. Chang. Biol. 20, 765–777. doi: 10.1111/gcb.12478
Pelletier, G., Roberts, M., Keyzers, M., and Alin, S. R. (2018). Seasonal variation in aragonite saturation in surface waters of Puget Sound – a pilot study. Elem. Sci. Anth. 6:5. doi: 10.1525/elementa.270
Peterson, W. T., Fisher, J. L., Strub, P. T., Du, X., Risien, C., Peterson, J., et al. (2017). The pelagic ecosystem in the Northern California Current off Oregon during the 2014–2016 warm anomalies within the context of the past 20 years. J. Geophys. Res. 122, 7267–7290. doi: 10.1002/2017JC012952
Rahlff, J., Khodami, S., Voskuhl, L., Humphreys, M. P., Stolle, C., Arbizu, P. M., et al. (2021). Short-term responses to ocean acidification: effects on relative abundance of eukaryotic plankton from the tropical Timor Sea. Mar. Ecol. Progr. Ser. 658, 59–74. doi: 10.3354/meps13561
Ramajo, L., Pérez-León, E., Hendriks, I. E., Marbà, N., Krause-Jensen, D., Sejr, M. K., et al. (2016). Food supply confers calcifiers resistance to ocean acidification. Sci. Rep. 6:19374. doi: 10.1038/srep19374
Smith, J. N., De’ath, G., Richter, C., Cornils, A., Hall-Spencer, J. M., and Fabricius, K. E. (2016). Ocean acidification reduces demersal zooplankton that reside in tropical coral reefs. Nat. Clim. Chang. 6, 1124–1129. doi: 10.1038/nclimate3122
Thomsen, J., Casties, I., Pansch, C., Körtzinger, A., and Melzner, F. (2013). Food availability outweighs ocean acidification effects in juvenile Mytilus edulis: laboratory and field experiments. Glob. Chang. Biol. 19, 1017–1027. doi: 10.1111/gcb.12109
Tills, O., Sun, X., Rundle, S. D., Heimbach, T., Gibson, T., Cartwright, A., et al. (2016). Reduced pH affects pulsing behaviour and body size in ephyrae of the moon jellyfish, Aurelia aurita. J. Exp. Mar. Biol. Ecol. 480, 54–61. doi: 10.1016/j.jembe.2016.03.014
Keywords: ocean acidification, zooplankton, Puget Sound, meta-analysis, pteropods, calcification, pH
Citation: Keil KE, Klinger T, Keister JE and McLaskey AK (2021) Comparative Sensitivities of Zooplankton to Ocean Acidification Conditions in Experimental and Natural Settings. Front. Mar. Sci. 8:613778. doi: 10.3389/fmars.2021.613778
Received: 03 October 2020; Accepted: 16 April 2021;
Published: 13 May 2021.
Edited by:
Nina Bednarsek, Southern California Coastal Water Research Project, United StatesReviewed by:
Silke Lischka, GEOMAR Helmholtz Center for Ocean Research Kiel, GermanyVladimir G. Dvoretsky, Murmansk Marine Biological Institute, Russia
Grace Saba, Rutgers, The State University of New Jersey, United States
Ella Louise Howes, Centre for Environment, Fisheries and Aquaculture Science (CEFAS), United Kingdom
Copyright © 2021 Keil, Klinger, Keister and McLaskey. This is an open-access article distributed under the terms of the Creative Commons Attribution License (CC BY). The use, distribution or reproduction in other forums is permitted, provided the original author(s) and the copyright owner(s) are credited and that the original publication in this journal is cited, in accordance with accepted academic practice. No use, distribution or reproduction is permitted which does not comply with these terms.
*Correspondence: Terrie Klinger, dGtsaW5nZXJAdXcuZWR1