- 1CCMAR Centro de Ciências do Mar, Universidade do Algarve, Faro, Portugal
- 2Department of Life Sciences, Centre for Functional Ecology, University of Coimbra, Coimbra, Portugal
- 3Institute for Biodiversity and Ecosystem Dynamics, University of Amsterdam, Amsterdam, Netherlands
Genome size variation is of crucial biological importance, however variation in genome sizes within a single individual/organism is rarely reported except for some species groups such as algae where polygenomy, endopolyploidy and mixopolyploidy have previously been reported. The red algal genus Porphyra forms part of very profitable marine food products commonly known as ‘Nori’. Farming of these valuable marine crops was revolutionized by the discovery of their life cycle in the 40’s. One of the most remarkable characteristics of these taxa is the formation of chimeric gametophytic thalli. After meiosis, the four meiotic products are not released as individuals spores, but instead develop together into a single leafy thallus through successive mitotic divisions. In this study, we used flow cytometry to estimate genome sizes in 670 vegetative thallus sections from 195 blades from three Porphyra species, to determine if this chimerism could be related to the presence of multiple genome sizes and mixoploidy within thalli. Our results show a wide variety of genome sizes both within and between thalli. We interpreted these results as the presence of two different genome types of different sizes (a and b) with separate rounds of genome duplications within the vegetative thalli. By analyzing several sections per thallus, we were able to show that the different genome types and ploidy levels are not distributed haphazardly through the thallus, but are distributed along the thallus in a sectorial way in mosaics. In some individuals, the 2C genome size can either be interpreted as diploids or alternatively as haploid cells that are arrested at the G2-stage of the mitotic cycle, acting as diploid with two copies of their genome during most of their life-time. We conclude that Porphyra species belong to an aneuploid/euploid system, where genome duplications, mixoploidy, chromosomal dynamics and the presence of different genome types in the chimeric thalli play a role in shaping the genetic diversity of these taxa. Our results may have important implications to understand red algae biology and evolution and raise further questions on concepts of what constitutes an individual.
Introduction
Genome size can vary considerably between and within species (Biémont, 2008) and it is widely recognized that this variation is of crucial biological importance (Chu et al., 2018; Doležel and Greilhuber, 2010). Genome size variation has been used to study phylogenetic relationships among plants and crop improvement (Le Gall et al., 1993). However, the variation of genome sizes within a single organism/individual is rarely reported, except for a few taxa (e.g., Šmarda and Bureš, 2006). This is because the amount of DNA per nucleus is relatively constant across the somatic cells of a given species (reviewed in Doležel and Greilhuber, 2010), since it is expected that individuals of a species are characterized as physiological units with genetic homogeneity (González and Santelices, 2017).
In marine eukaryotic macroalgae, comparatively little information is available about the size and base composition of their genomes, or on their variation in genome size across and within species. Nuclear DNA content estimates have been published for only a handful of species of the main algal groups, Chlorophyta, Phaeophyta and Rhodophyta (below 1%, according to Kapraun, 2005). The majority of the data comes from work by Kapraun and co-authors obtained mainly by microspectrophotometry, available for comparisons in the Plant DNA C-values database1. With the same technique, earlier studies by Goff and Coleman in the 80’s (e.g., Goff and Coleman, 1984, 1986, 1987, 1990) found in some groups of red algae, major nuclear patterns associated to DNA dynamics such as polygenomy, endopolyploidy and polyploidy. For example, for the red alga Polysiphonia, the apical meristematic cell was found to have significantly more DNA (64–128 times more) in the nucleus than mature somatic or gametic cells (Goff and Coleman, 1986). Such patterns have also been reported for other algal taxa, like Laminariales (e.g., Phillips et al., 2011), Fucales (e.g., Garbary and Clarke, 2002; Coyer et al., 2006), and Caulerpales (e.g., Varela-Álvarez et al., 2012).
Current data on genome sizes in red algae support polyploidy and aneuploidy as widespread features of red algal genome evolution (Kapraun and Freshwater, 2012). Early diverging red algal lineages are characterized by relatively small 2C DNA contents while a wide range of 2C values is found within the more derived class of the Florideophyceae, however an overall correlation between phylogenetic placement and DNA content have not been found for red algae (Kapraun and Freshwater, 2012), neither for brown algae (Phillips et al., 2011). More interesting, recent studies on genomes of some algal groups have related these algal genomes with the evolution of multicellularity (Cock et al., 2010; De Clerck et al., 2018).
The red algal genus Porphyra C. Agardh and its sister genus Pyropia J. Agardh (Sutherland et al., 2011), form part of the very profitable marine food products commonly known as ‘Nori’ (Blouin et al., 2011; Sahoo et al., 2002). Farming of these valuable marine crops was revolutionized by the discovery of their life cycle by Drew (1949). Drew showed that Conchocelis rosea Batters, which was originally described as a separate species, was in fact the germinated zygotospore (sporophyte, 2n phase) from the Porphyra blade (gametophyte, n phase) (Figure 1). Since then, Porphyras/Pyropias life cycles have been described involving two ploidy levels, with the gametophytic blade assumed to be haploid and the sporophytic filamentous phase assumed to be diploid. Several chromosome studies have supported this haploid blade/diploid sporophyte theory (e.g., refs Magne, 1952; Kito, 1966; Kito et al., 1967), by showing that the chromosome number in the sporophyte phase doubles the chromosome number in the gametophytic phase. However, other studies have reported incongruences and found the same chromosome number in both phases (e.g., Conway and Cole, 1973; Mumford and Cole, 1977; Krishnamurthy, 1984; Freshwater and Kapraun, 1986; Kapraun and Freshwater, 1987), different chromosome numbers within a single species (Table 1), or heterozygosity in “haploid” gametophytic blades where only one allele per locus is expected (Fujio et al., 1988; Park et al., 2007; Kong et al., 2009; Niwa et al., 2010; Varela-Álvarez et al., 2017, 2018a). Recently, using flow cytometry and microsatellite analyses, we found multiple genome sizes and multiple alleles at several loci in gametophytic blades of several Porphyra species in the central part of Portugal (Varela-Álvarez et al., 2018b), representing different cell lines with several cytotype combinations among and within individuals, where individuals could present a single ploidy level or combined ploidies (mixoploids).
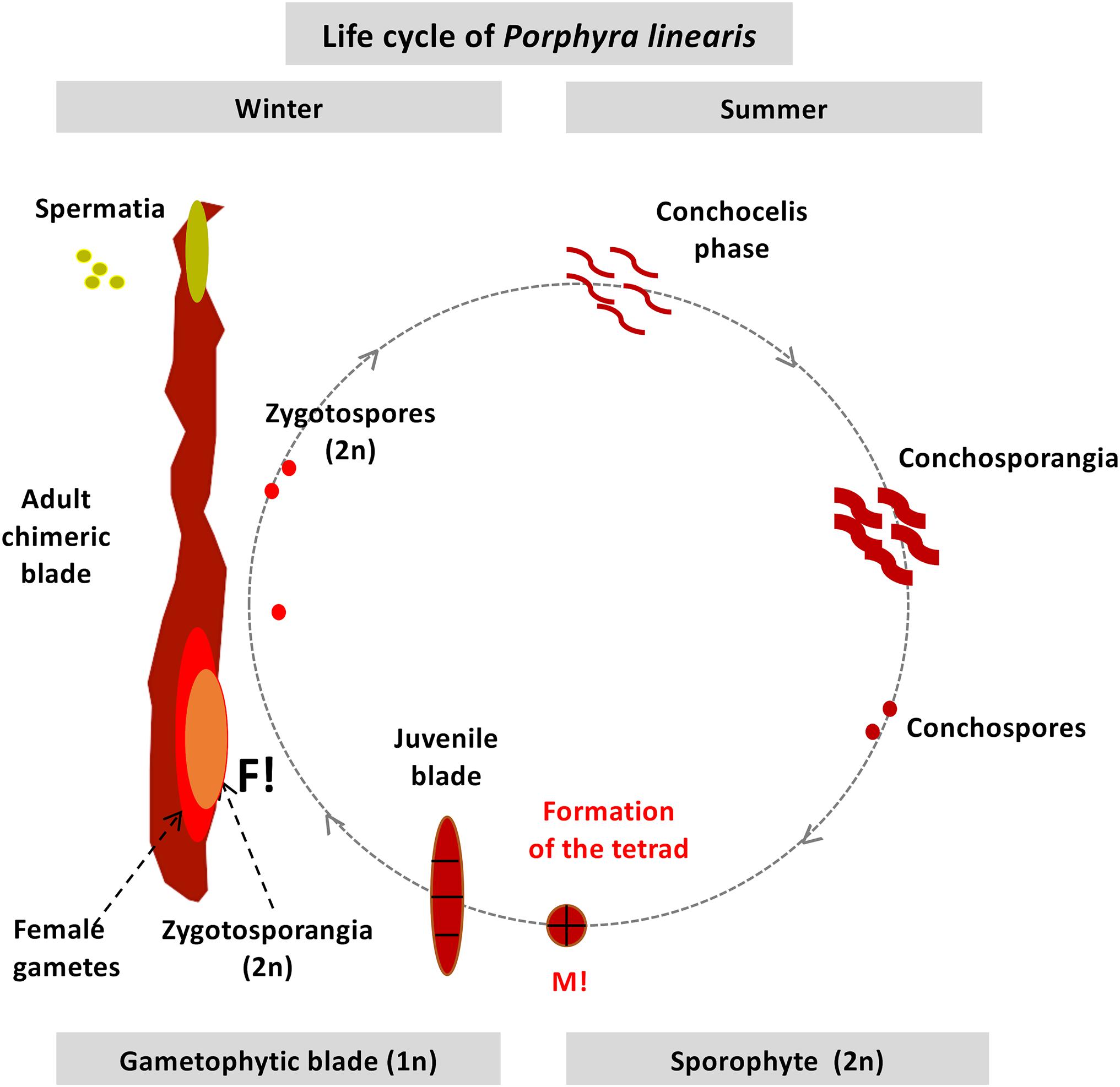
Figure 1. Diagram of the life history in the field of Porphyra linearis. M!: Meiosis. F!: Fertilization (syngamy or fusion of males and females gametes).
Allopolyploids have been found in some Japanese species of Porphyra (Niwa and Sakamoto, 2010; Niwa et al., 2010, 2018), as well as spontaneous diploidization in male gametophytes in Pyropia haitanensis (T.J. Chang and B.F. Zheng) N. Kikuchi and M. Miyata (Zhong and Yan, 2020). The mixed-ploidy in the gametophytic blades observed in Varela-Álvarez et al. (2018b) can possibly be explained by one of the most remarkable characteristics of these red algal taxa: the existence of chimerism or chimeric blades. Ohme and Miura (1988) were one of the first to report this phenomenon, where a single gametophytic blade is composed of several genetically different cell lines. When the sporophyte produces the conchospore, through meiosis a tetrad is formed with the four meiotic products, which are therefore genetically distinct cells, each of which has a different genotype. However, these four cells are not released as four individual spores, but instead develop together into a single leafy thallus through successive mitotic divisions (Figure 1). Furthermore, not all gametophytic blades are chimeric, since blades can also be produced by asexual reproduction by asexual spores, and these blades can be genetically uniform (e.g., Niwa et al., 2009; Niwa and Abe, 2012). We wonder if there is a spatial distribution of the ploidy levels within the mixoploid blades and if so, it is related to the generation of the chimeric blades, and a putative link between mixoploidy and chimerism has yet to be discovered.
This study has two main goals: first to determine if the multiple genome sizes found previously in Porphyra show distinct patterns within the vegetative thalli and if this pattern differs among species with different blade morphologies. Second, and more important, to determine whether the previously observed mixed ploidy individuals are related with the development of the tetrad and the genetic chimeras. If so, since the Bangiales, which includes Porphyra, is a sister clade of the Florideophyceae from which it diverged ca. 1 billion years ago (Yang et al., 2016), our results may have important implications to understand algal evolution and raise further questions on concepts of what constitutes an individual.
Materials and Methods
Plant Material and Experimental Design
Samples of gametophytic blades (n phase, meiotically reduced nuclei) from three different species of Porphyra (Porphyra linearis Greville, Porphyra umbilicalis Kützing and Porphyra dioica J. Brodie and L.M. Irvine) were collected from the center/north area of the Portuguese coast from February 2019 to July 2019. Six localities were visited: Parede (38°41′N 9°21′W, for P. linearis), Paço de Arcos (38°41′15.4″N 9°18′08.1″W, for P. linearis), Belém (38°41′N 9°12′W, for P. linearis and P. dioica), Figueira da Foz (40°10′N 8°53′W for P. dioica and P. umbilicalis), Leirosa Mini Site1 (40°03′20.5″N 8°53′30.5″W for P. dioica and P. umbilicalis) and Leirosa Mini Site2 (40°03′23.0″N 8°53′33.1″W for P. umbilicalis). In the localities where two species were found (Belém, Figueira da Foz or Leirosa Mini Site1), these species occurred in different areas on the shore and were never co-occurring in the same patch. Namely, P. linearis occurred in the high intertidal on rocks or walls, P. dioica in the middle-lower intertidal on rocks and sand, and P. umbilicalis in the high intertidal on rocks. In total 9 populations from 6 sites (3 populations per species) across central Portugal were sampled, and from 52 to 88 individual blades per species were collected in order to perform comparisons of genome sizes between populations and species and to study intraspecific nuclear patterns (see below). Plants were examined under a binocular microscope for identification of vegetative and reproductive thallus areas: spermatia (packets of male gametes), female gametes and zygotospores (packets of spores as a result of mitotic divisions of the zygotes produced by syngamy of males and female gametes).
Thallus Re-sampling to Study Intraspecific Genome Variation
For each blade of P. linearis and P. dioica, at least three 1 cm2 sections of the vegetative thallus were excised in a linear transect from the bottom of each blade (near the holdfast area) to the top of the blade. The sections were labeled R1 (Replicate 1), R2, and R3. R1 was the section at the base of the blade, R2 was in the middle of the blade and R3 was at the top of the blade. In some randomly selected blades, we also excised additional sections which were at randomly selected positions (e.g., R1b or R2b). In some others, we excised a fourth thallus section covering the holdfast area. For P. umbilicalis, where the blade has a rounded morphology, the transect was made from the center of the plant (where the holdfast occurs) to the periphery, at a haphazardly selected angle. These thallus sections were also named R1, R2, and R3, and were placed in the same way as above. For this last species, in a small subset of blades the sections were placed randomly because the size of the blade was too small to place the three 1 cm2 in a linear transect (Online Supplement, Supplementary Table 1). In addition, for the larger blades, we placed extra transects in some randomly selected blades (up to 3 transects per blade for 7 blades) to test the hypothesis that transects placed across the right or left side of the of the blade may represent different genome sizes. In addition, when possible, reproductive thallus sections (with zygotospores) were also collected as a control for the 2n phase (sporophytic phase, meiotically unreduced phase in the life cycle).
Flow Cytometry
The nuclear DNA content of the sampled Porphyra specimens was determined by flow cytometry using fresh material. Nuclei were released into suspension following the procedure of Loureiro et al. (2007) by chopping approximately 1 cm2 of each blade section with a razor blade together. As internal reference standard, we used 50 mg of fresh leaf of Solanum lycopersicum (2C = 1.96 pg, Doležel et al., 1992). Nuclei of both were chopped up together in 1mL of WPB buffer (Loureiro et al., 2007; 0.2 M Tris–HCl, 4 mM MgCl2.6H2O, 1% Triton X-100, 2 mM EDTA Na2.2H2O, 86 mM NaCl, 10 mM metabisulfite, 1% PVP-10, pH adjusted to 7.5 and stored at 4°C). To verify that there were no smaller genome sizes present in Porphyra we also applied to a small set of samples a standard of smaller genome size, Raphanus sativus (2C = 1.11 pg, Doležel et al., 1992). The suspension containing the nuclei was then filtered through a 50 μm nylon filter and 50 μg/mL of PI -Propidium iodide (Fluka, Buchs, Switzerland) was added to stain the nuclei, together with 50 μg/mL of RNase (Fluka, Buchs, Switzerland) to prevent staining of double-stranded RNA. After incubation for 3–5 min, the fluorescence intensity of the suspended nuclei was analyzed using a Partec CyFlow Space flow cytometer (Partec GmbH, Görlitz, Germany), equipped with a green solid-state laser for PI excitation.
Measurements were obtained using the FloMax software v2.4d (Partec GmbH) in the form of six graphs with the next parameters: (a) Fluorescence pulse integral in linear scale (FL); (b) fluorescence pulse integral in linear scale versus time (to monitor fluorescence stability over time); (c) fluorescence pulse integral in linear scale versus side light scatter in logarithmic scale (SSC); (d) side light scatter (SSC) vs. forward light scatter (FSC), both in logarithmic (log) scale; (e) FL vs. fluorescence pulse height; (f) FL vs. SSC. A region of interest comprising mostly the isolated nuclei was defined in the FL vs. SSC cytogram and subsequently used to gate all the other graphs. The presence of aggregates (doublets or triplets) was evaluated in the FL vs. fluorescence pulse height cytogram. The genome size (in picograms, pg) of each sample was determined according to the following formula: (mean fluorescence of the sample/mean fluorescence of the standard) ∗ nuclear DNA content of the standard. The reliability of the genome size measurements was verified by evaluating the quality of the flow cytometry histograms based on the coefficient of variation (CV) of the G1 peaks and the background debris, and the CV of the genome size estimation of each sample (raw data in Online Supplement, Supplementary Table 1 and Supplementary Figure 1) and compared within the range of values reported in similar works for fungi (Bourne et al., 2014; Tavares et al., 2014). Descriptive statistics (mean, standard deviation, maximum and minimum) and analyses of the overall genome size variation across groups and species were carried out in Statgraphics (5.1) and Sigma Plot 14.2.3.
Assignment of Ploidy Levels and Chromosome Numbers Under Different Scenarios
Genome sizes in this study were classified into several distinct size classes (Table 2, Supplementary Table 1). We associated these genome sizes with two genome types (a and b) with different rounds of genome duplications or ploidy levels (type a with average genome sizes 0.17, 0.35, and 0.66 pg and type b with average genome sizes 0.23, 0.45, and 0.84 pg). These are the same as in Varela-Álvarez et al. (2018b), except that we here observed an extra size class corresponding to type a (Size class 1 with average 0.17 pg). We also associated each genome size to a C-value (amount of DNA in picograms contained in a haploid nucleus), 1Ca, 2Ca, 4Ca or 1Cb, 2Cb, 4Cb (top of Table 2) with the minimum value (1C) representing the smaller genome size for each genome type, and the others representing multiples of this level.
For a typical DNA histogram, we interpreted the largest peak to be representing the cells in the G1 phase (pre-DNA replication) of the cell cycle. Occasionally, a small second peak was observed at twice the channel value (less than 10% of the nuclei analyzed), which was assumed to represent cells in G2 phase, so post-DNA replication. This pattern follows the typical eukaryotic cell cycle where most cell time is spent in G1, as also reported in other algal taxa. However, there is an alternative interpretation possible. For some groups of red algae, the major nuclei peak has been interpreted as nuclei arrested at the G2 phase of the cell cycle, and the minor peak has been interpreted to be composed of polyploid nuclei (e.g., Ceramiales, Goff and Coleman, 1984, 1987, 1990). We therefore propose two main scenarios for the assignment of ploidy levels based on the flow cytometry data (Table 2).
In Scenario 1 (SC1), each size class for each genome type (a or b) represents a distinct ploidy level (1x, 2x or 4x, with x representing a chromosomal set). In Scenario 2 (SC2), each size class for each genome type represents either the G1 phase or G2 of the cell cycle within two ploidy levels (1x or 2x). In addition, we also propose a less likely Scenario 3 (SC3) (see section “Discussion”) where all the genome sizes could be considered all belonging to the same genome type, with the genome sizes representing aneuploid (both hyperploid –with extra chromosomes– and hypoploid –with fewer chromosomes) variants of the basic chromosome number. In all the cases, the major nuclei peak in each sample is analyzed but, the minor peak (either considered to be nuclei in G2 or as a polyploid nuclei) is only displayed in the data set in Online supporting information, but not considered in the analyses. In addition, the C-value terminology is used to represent the ploidy levels for the analyses and figures in this study, because the main two scenarios (SC1 and SC2) would be integrated under this nomenclature, since for example 2Ca could represent SC1 (2xa) or SC2 [1xa (G2) + 2xa (G1)], and so on.
In each of our two scenarios (Table 2, bottom part), we equate one of the most abundant size classes found in our samples (size class 3 or size class 4) to the one of the most common basic chromosome numbers cited in the literature, and then infer the other size classes as multiples or halves of these chromosome numbers. For SC3, all the chromosome numbers reported in the literature for each species are used. We use the terminology “mixogenome blades” to describe blades with the two genome types a and b regardless of the ploidy level, and “mixoploid blades” for blades with different ploidy levels regardless of the genome type a or b. In addition, a glossary of ploidy-related terms used in this study is provide in Online Supplement, Glossary list, Supplementary Table 2.
Results
Intraspecific Genome Size Variation
Genome sizes for vegetative thalli in the three Porphyra species in this study varied from 0.15 to 0.73pg (Table 2, Supplementary Figure 2, Supplementary Table 1), which were classified in 6 distinct sizes classes, 5 for vegetative thalli (genome size average for each class 0.17, 0.23, 0.35, 0.45, and 0.66 pg) and 2 for the zygotospores used as controls (genome size averages for each class 0.66 pg and 0.84 pg). The most common size classes found among the three Porphyras were size class 3 (mean: 0.35 pg) and size class 4 (mean: 0.45 pg). For the reproductive thalli when zygotospores were mixed with gametes, we found several genome sizes (Supplementary Table 1), however when zygotospores were isolated alone, only the size class 5 (mean: 0.66 pg) and/or size class 6 (mean 0.84 pg) were found. Size class 6 (mean 0.84 pg) was only observed in zygotospores, whereas size class 5 (mean: 0.66 pg) was also found in vegetative thalli of all three species. Overall genome size variation in each species is displayed in Supplementary Figure 2.
Most single sections of the vegetative thalli showed a single peak, indicating that all nuclei in that section had the same size, however sections taken from the same gametophytic blade but in different areas frequently had differently sized genomes, indicating that these individuals are chimeric. A few sections showed multiple (up to three) peaks, indicating the presence of nuclei with differently sized genomes also in a single section (Figures 2, 3, Table 3).
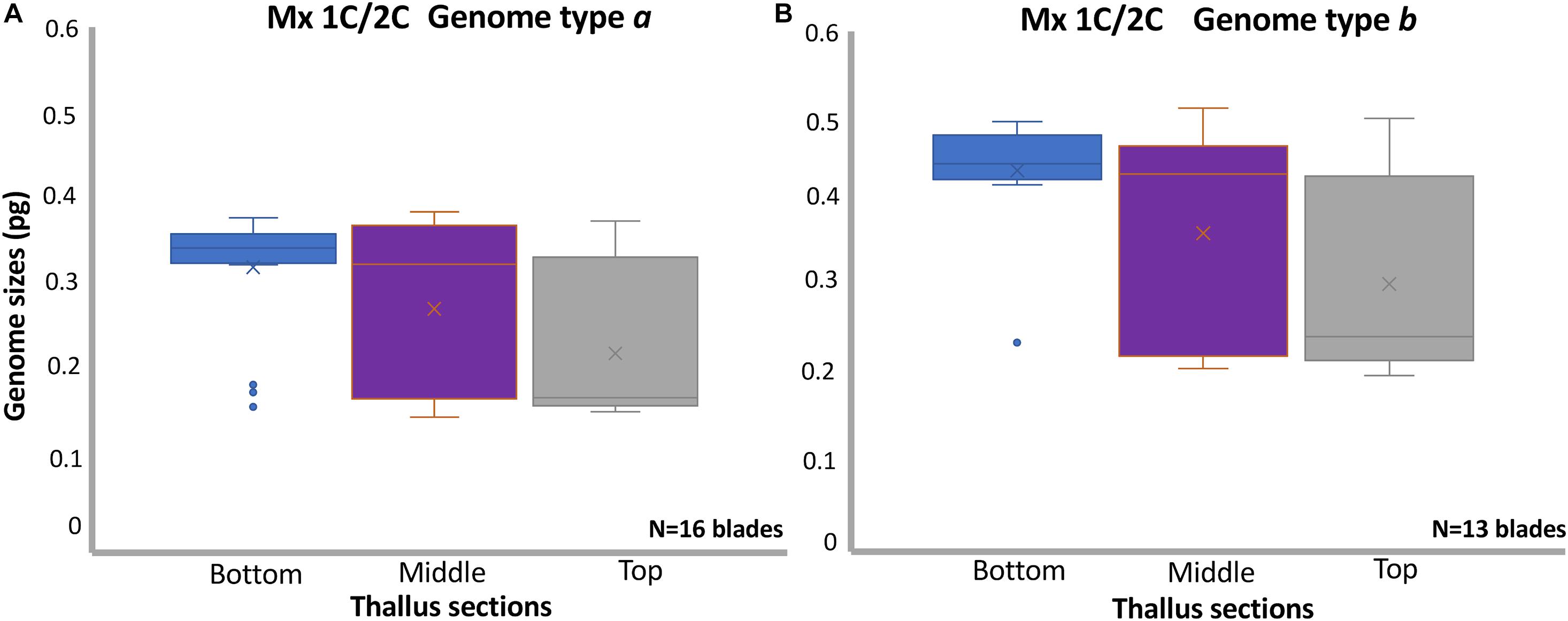
Figure 2. Boxplots displaying the genome size variation in sections of vegetative thalli in Porphyra linearis: (A) Thallus sections (sample size for bottom N = 19, middle N = 26, top N = 23) in 16 mixoploid blades 1C/2C genome type a, (B) Thallus sections (sample size for bottom N = 13, middle N = 17, top N = 18) in 13 mixoploid blades 1C/2C genome type b. The x near the median bar indicates the location of the sample mean. Samples means with standard deviation and medians for each class were for subplot (A), bottom: 0.33 ± 0.06, 0.34, middle: 0.27 ± 0.09, 0.32, top: 0.22 ± 0,08, 0.17; for (B), bottom: 0.43 ± 0.06, 0.44, middle: 0.37 ± 0.12, 0.43, top: 0.30 ± 0.11, 0.24. Differences in mean genome sizes are significantly different when comparing genome sizes in the bottom area of each blade vs the middle area (t-test, P < 0.05) and vs. top area (t-test, P < 0.001) for both mixoploid blades either a or b. Mx, mixoploid.
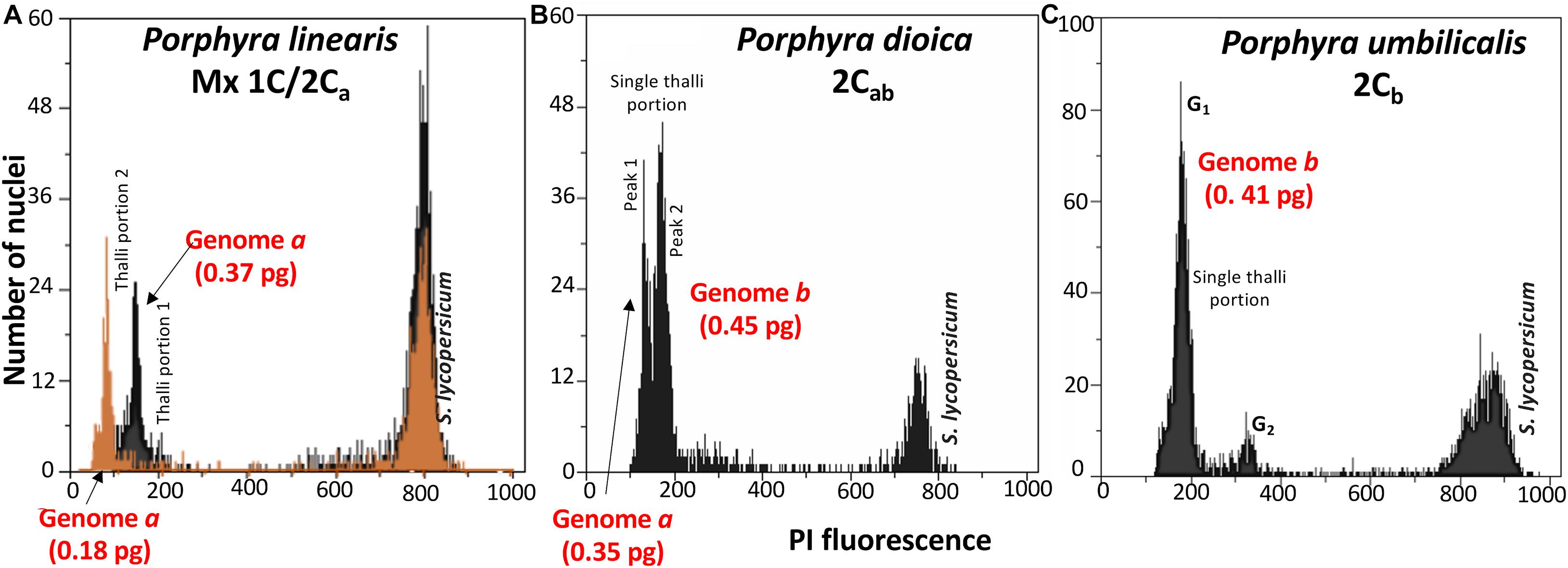
Figure 3. Flow cytometric histograms of relative fluorescence intensities of propidium iodide-stained nuclei in vegetative thalli of Porphyra, internal reference standard Solanum lycopersicum (peak at the right; with 2C = 1.96 pg): (A) Superimposed flow-cytometric histograms for two thallus sections in the same blade in P. linearis with two distinct genome sizes, demonstrating the sectorial distribution of the genome sizes, (B) Two genome sizes in a single thallus portion in P. dioica, (C) Genome size of genome type b in P. umbilicalis.
The spatial distribution of genome sizes along the blade was different for the three species (Figure 4, Supplementary Table 1). In P. linearis, when blades presented intraspecific variation of genome sizes in the same thalli, the bottom of the blade was occupied by genome sizes of classes 3 or 4 (mean of 0.35 pg and 0.45 pg, respectively), and the top of the blade was occupied by size classes 1 or 2 (mean of 0.17 pg and 0.23 pg, respectively), with the exception of 2 single blades out of the 32 with combined nuclei sizes (either mixoploid or mixogenome or both). In P. dioica, out of 49 blades with compound nuclei sizes (either mixoploid or mixogenome or both), 24 displayed the direction of growth (bigger genome sizes on the bottom and smaller genome sizes on the top) and 25 did not, so we assumed that the distribution of genome sizes was random. In P. umbilicalis, out of 13 blades with compound nuclei sizes (either mixoploid or mixogenome or both) where we could measure the three 1cm2 replicates in a linear transect, only 3 displayed the direction of growth, so we made the same assumption and define this species as random sectorial.
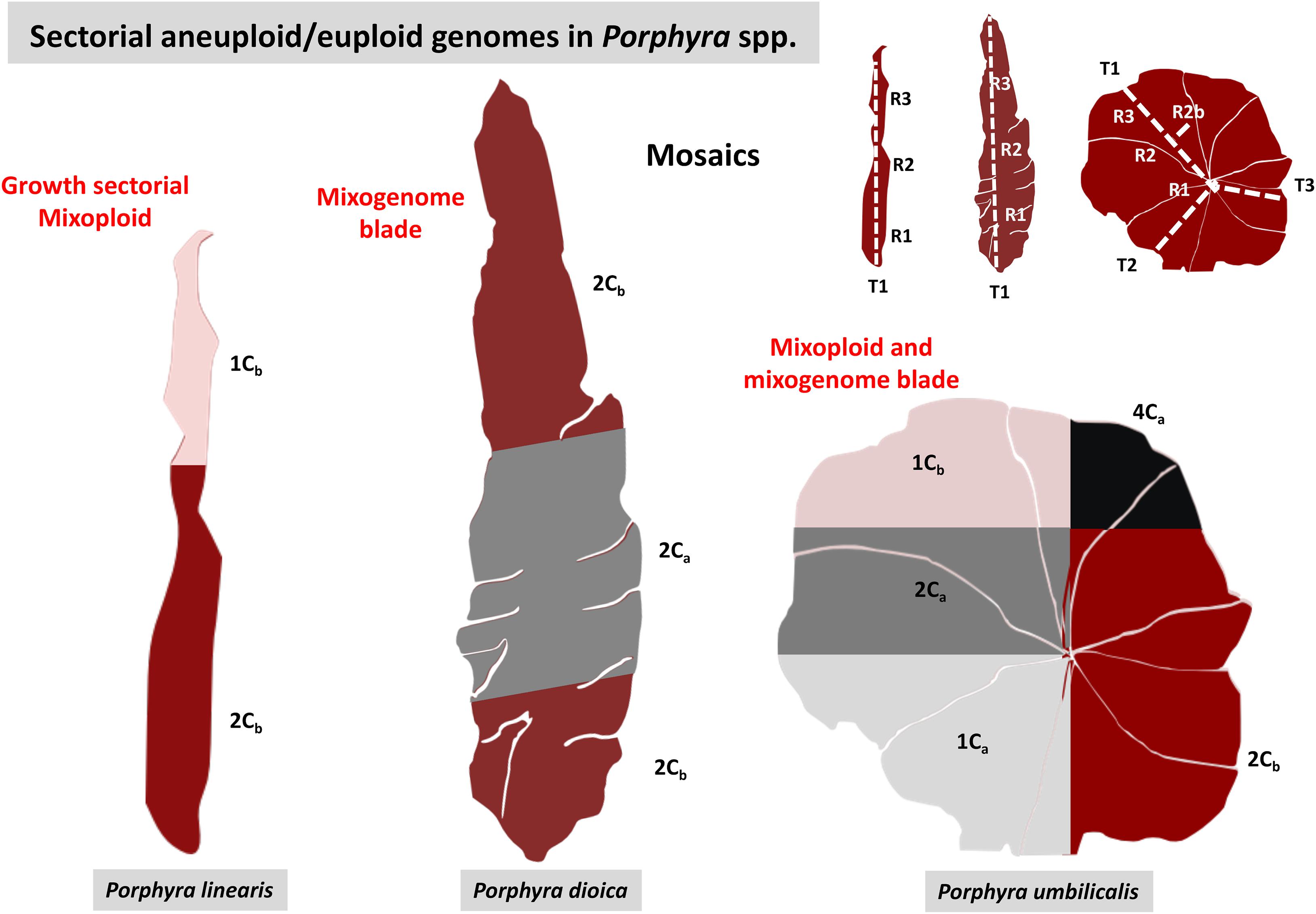
Figure 4. Diagrams showing examples of the distribution of genome sizes along the thalli for each Porphyra species. In P. linearis, the example shows two main sectors, the larger genome sizes on the bottom part of the blade and the smaller genome sizes on the top. In P. umbilicalis and P. dioica genome sizes are distributed randomly but in mosaics. On the right corner, the diagram shows the thallus portions analyzed in the different transects along the blade.
Mixogenome and Mixoploid Blades
When associating each genome size to a C-value, we found two main trends of variability in our data, the distinct genome types (a and b) and the distinct genome size duplications (ploidy) for each genome type, where genome type a would represent an aneuploid genome with two rounds of genome duplications within the blade (1Ca, 2Ca and 4Ca), and genome type b would represent a haploid genome with one round of genome duplication (1Cb and 2Cb), or the other way around, being genome type b the aneuploid genome and genome type a the haploid genome (Table 2).
When looking at the ploidy level of the blades, taking the re-sampling into account, we found that the majority of the blades for both P. umbilicalis and P. dioica have two copies of the genome (2C; 90.91% and 76.14.%, respectively) of either type a or b (Table 3). For those two species we also found a small percentage of mixoploid blades (blades having combined ploidy levels) 1C/2C, 1C/4C, 1C/2C/4C or 2C/4C, in 9.09% for P. umbilicalis and in 21.59% for P. dioica. However, in P. linearis the percentage of diploid blades was smaller (38.47%), and the majority of the blades were mixoploid (59.61%). Truly haploid blades (1C) were also found for both genome types for P. dioica and P. linearis (2.27% and 1.92%, respectively). For all the species, most of the mixoploid blades were detected by comparing the three replicates analyzed in the blade; only in a few samples the mixoploids were detected through sections with combined genome sizes (2.34% for P. umbilicalis, 5.59% for P. dioica, and 20.00% for P. linearis).
When only considering the genome type (a or b), regardless of any genome duplications, we found both uniform blades (with only one genome type) and mixogenome blades (with both genomes types) in all three species (bottom part of Table 3). For P. umbilicalis almost half of the samples analyzed (45.46%) had mixogenome blades ab with combined genome sizes of both genomes and any ploidy level. For P. linearis, the frequency of mixogenome thalli was lower (only 5.77%) and most of the blades were uniform either with genome a or genome b, but with different ploidy levels. For P. dioica most of the blades were uniform with genome b (51.14%), but 38.64% were mixogenome blades ab, and a small percentage (10.23%) had genome a.
This distribution of ploidy levels and genome types across the mixoploid and mixogenome blades clearly showed mosaic patterns for each species (Figure 4). While for P. dioica and P. umbilicalis, mosaics with any ploidy levels and genome types are distributed along the blade randomly, for P. linearis there was a clear pattern from the bottom to the top of the blade, where the bigger genome sizes (and consequently ploidy levels) lay on the bottom of the blade and the smaller genome sizes on the top of the blade, at least for most of the mixoploid thalli analyzed, regardless of the type of genome a or b (Figure 2). Increasing the re-sample size in P. umbilicalis with three transects and up to 12 thallus sections in 7 blades did not increase the number of ploidy levels or genome types in the blade, neither showed a different nuclear distribution pattern (Online Supplement, Supplementary Table 3).
Cytotype Diversity at Different Sites for Each Species
For all the three Porphyra species, the frequencies of the different genome size classes and the incidence of mixoploids differed among the sampling sites (Figure 5). Within P. linearis the frequency of mixoploids at Parede site was the highest, where 83.33% of the blades analyzed were mixoploid, with lower frequencies at the two other analyzed sites (50.00% for Paço de Arcos, 53.33% for Belém). In P. dioica and P. umbilicalis, 2C blades were the dominant blades at the three sites, consisting of either genome type a or b or combined ab. The site Belém had the truly haploid blades 1C for both P. linearis and P. dioica (3.33% and 8.00% respectively), and for P. dioica the number of mixoploids was the highest at this site (48.00%). For P. linearis, mixogenome blades ab and uniform blades a or b were present at all sites with the exception of Paço de Arcos site where only genome type a was present.
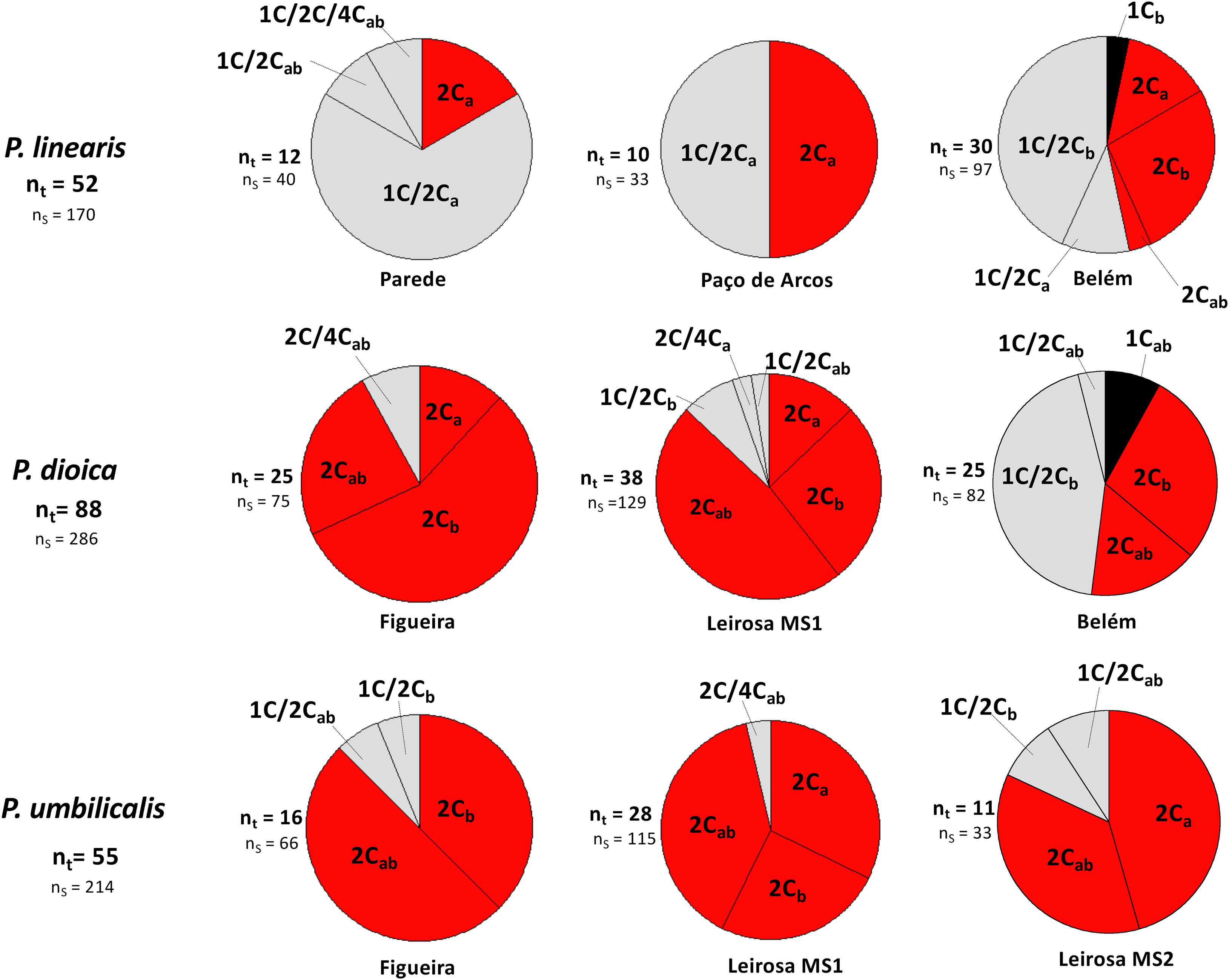
Figure 5. Distributional patterns of cytotypes based in genome sizes of vegetative thalli for each Porphyra species by localities. nt, Total number of blades per species and per locality; ns, Total number of thallus sections analyzed per locality and per species.
Discussion
Sectorial Genome Size Variation and Nuclei Ploidy Patterns in Vegetative Thalli
Our study clearly shows that the Porphyra blades are cytochimeras, or chimeras with different genome sizes distributed along the blade in sectors. This matches previously described results (e.g., Ohme and Miura, 1988) where Porphyra blades were shown to contain sectors of distinct genetic composition. We do not know if the sectors with different genetic composition previously found match the exact sectors with different genome sizes, or if they are distributed in different areas, but the distribution of the genome size and genetic composition could be related to the morphogenesis and development of each species. The sectorial distribution of genome sizes and ploidy levels across the blade follows the growth pattern mainly for P. linearis, and it is randomly distributed for P. umbilicalis and P. dioica. Further research is needed to determine the cause of that distribution.
In mixoploids, the distinct ploidy levels are in different parts of the thallus, with a minor proportion of compound/mixed thallus sections, so it is clear that if only one sample were measured per blade, the frequency of mixoploids and mixogenome blades would have been underestimated. Re-sampling the same individual was crucial to discover the cytochimeras and the different sectors in the blade with different genome sizes.
The obtained genome size data were interpreted under different scenarios (Table 2) to be able to discuss the evidence for all possible hypotheses for the observed results. Considering all available evidence, Scenario 1 is the most plausible to explain our results, where the different genome size classes consist of different combinations of ploidy levels and genome types. This scenario would agree with previous work on Porphyra (Kapraun et al., 1991; Le Gall et al., 1993; Matsuyama-Serisawa et al., 2007), where the cells of the gametophytic blades were found to be at the G1 stage of the cell cycle. According to Scenario 1 (SC1), chromosome numbers for the Porphyra species studied here would be 2, 4 and 8 for type a, and 3 and 6 for type b.
Scenario 2 (SC2) is a more complex system where some parts of the thallus are composed of cells undergoing the normal cell cycle at G1, and other parts are composed of cells arrested in G2 (with chromosomes with two chromatids, and consequently the double amount of DNA). Under this scenario (SC2), size classes 1 and 2 (0.17 and 0.35 pg, respectively) would then represent cells with one set of chromosomes (1x) at G1, with 3 and 4 chromosomes, respectively for P. linearis, 4 and 5 chromosomes for P. umbilicalis, and 3 and 5 chromosomes for P. dioica. However, the next size classes 3 and 4 (0.35 pg and 0.45 pg, for types a and b) would represent cells with the basal ploidy level (1x) with the same number of chromosomes but in G2 (with two chromatids). Size class 5 (0.66 pg) within the vegetative blade would correspond to the next ploidy level (2x) in G2 with 6 chromosomes (P. linearis and P. dioica) and 8 chromosomes in P. umbilicalis. In addition, this size class 5 (0.66 pg) in the vegetative thalli would be a consequence of two rounds of genome duplications probably by irregular mitosis or diploid cells arrested at the G2 when the blade is growing, but in the zygotospores would be originated by fusion of diploid gametes. So the genome size value is the same in the two types of cells but the origin would be different. Size class 3 can then be interpreted as either nuclei in G2 with 3 chromosomes and two chromatids or nuclei in G1 with 6 chromosomes and one chromatid, or a mixture of both (in thallus regions between two sectors). However, we believe this last scenario to be less likely. In SC2 the assumption of a “G2 arrest” would imply in our opinion a dormant stage of the cells, senescent cells, or a stage where the cell cycle is stopped, which is not the case since the three Porphyras studied were at full growth in the field at the time of collection. This interpretation of having cells arrested at the G2 in Porphyras should be considered with caution and specific research on the cell cycle is needed to verify it.
We also can envision a third scenario, scenario 3 (SC3) where our results may be explained by a single genome, but where the different genome sizes are different aneuploids (hyperploids and hypoploids) relative to the basic chromosome number for each species in the literature. For SC3 there are also several inconsistences as the odd chromosome numbers do not fit well with the multiples of the genome sizes. For example, when chromosome number for P. dioica is 5 ± 2 (as reported in the literature), it is difficult to fit the values 3, 5, and 7 into the multiples of the genome sizes, since none of them are exact multiples (Table 2). Moreover if we consider genome type b as an aneuploid (hyperploid) genome, SC1 and SC2 are more likely, since all the genome sizes will represent multiples of a minimum chromosomal set, then no missing chromosomes or genes will exist. In addition, results of heterozygosity and genome duplications in the literature are difficult to fit in this context (see sections below). However, we cannot discard any of these scenarios; with a higher re-sampling and a higher number of samples new patterns may be found and there are other possible explanations for the multiples of the genome sizes and non-proportional changes in DNA. Our results are limited to interpretation of genome size data and further research with different techniques (e.g., FISH and telomere analysis) can add and/or clarify these processes in the future.
The final possibility is that all scenarios are simultaneously true, and the Porphyra blades can be composed of both aneuploid and haploid cell lines, which originate during meiosis when the tetrad is formed, that subsequently undergo different rounds of genome duplications, with some cells at G1 and others arrested at G2. Also, during blade development, there could be some loss and gain of chromosomes by irregular mitosis that can also explain the odd number of chromosomes (see section “The Theory of the Two Genomes”). Regardless of the scenario, our study shows that Porphyra blades are composed of mosaics with genomes of different sizes distributed along the blade in sectors.
The Theory of the Two Genomes
Our results confirm our previous (Varela-Álvarez et al., 2018b) finding of two genome types within Porphyra thalli (type a: 0.17-0.35-0.66; type b: 0.23-0.45-0.84): genome b is the aneuploid genome of genome a (or the other way around) and each genome goes into separate rounds of genome duplications within the vegetative thalli (either for scenario 1 or 2). Very likely the smaller genome type a is the one where the basic chromosome number reside, and the other genome type b is an hyperploid (positive aneuploidy) genome type. The most parsimonious hypothesis supporting our results is the one that states that Porphyra is a genetic chimera combining cells with different genetic compositions all derived from the same spore (conchospore) (e.g., Ohme and Miura, 1988; Niwa et al., 1993; Mitman and van der Meer, 1994). In the first division, the conchospore splits into an upper and lower cell, which both divide vertically; the upper cells dominate the subsequent cell growth (Mitman and van der Meer, 1994; Wang et al., 2010). This would explain why we found two cells lines in most of the blades. It is reasonable to think that the two cells lines are derived from these original cells and the genomes are originated each generation when the conchospore germinates. It is possible that the four genetically distinct daughter cells also have different chromosome numbers due to an irregular segregation of the chromosomes at meiosis, either by non-disjunction of homologous chromosomes in meiosis I (first division) or non-disjunction of sister chromatids in meiosis II (second division), resulting in 4 cells with different chromosome numbers. After that, the two upper cells in the tetrad would dominate the subsequent cell growth (as in Mitman and van der Meer, 1994; Wang et al., 2010) and would colonize the blade by mitosis, producing sectors with different chromosome number. Further supporting evidence for the two-genome theory is provided by the existence of two sizes classes for the 2n zygotospores (for genome type a: 0.66 pg and for genome type b: 0.84 pg). The presence of uniform individuals of both types a and b as well as mixogenome individuals ab with all types of combinations with the ploidy levels, leads us to think that both types of zygotospores are viable. An in-depth study of genome heterogeneity in the sporophytic generation and in other stages of the life cycle are needed to characterize their variability and this will also shed light on the origins of the two genome types; however this will required a cultivation approach, since the conchocelis filaments are very difficult to find in nature (Varela-Álvarez et al., 2018b).
It has been claimed that intraorganismal genetic heterogeneity (IGH) or the existence of different genomes and/or genotypes within a single body (Pineda-Krch and Lehtila, 2004), can have some beneficial consequences, such as increasing resilience and productivity relative to genetically homogenous plants (Santelices et al., 1996; Santelices, 1999, González and Santelices, 2017). In addition, we cannot explain exactly how these genome duplications are happening in the blade for each cell line or genome type. The exact mechanisms initiating the sectorial genome sizes are still to be described, but there is evidence for spontaneous chromosomal duplications in other Porphyra/Pyropia spp. such as P. haitanensis (Zhong and Yan, 2020; Zhong et al., 2019), as well as for other algal taxa e.g., Florideophycidae, with sequential changes in the ploidy level of cells during development (Goff and Coleman, 1986).
Chromosomal Mosaics
According to our study, regardless of any scenario with one or two genomes considered, the Porphyra blades possess sectorial chromosomal mosaics and each genome size represents a distinct chromosomal number in the vegetative thalli, which varies between 2 and 8. Aneuploid numbers of chromosomes and chromosomal dynamics are occasionally mentioned in the literature for these and other Porphyra species. For example, in P. dioica the basic chromosome number 5 varies ± 2 in British populations of these species (M. Holmes, pers. Comm. in Brodie and Irvine, 2003); in P. linearis from the East Coast of the United States the basic chromosome number varies between 2 and 4 (Varela-Álvarez et al., 2005), and in Porphyra columbina Montagne the basic chromosome number varies between 2 and 5 (Badilla et al., 2008). Other evidence of chromosomal variation would be the incongruences between studies on the basic chromosome number for some species, which may be explained by cryptic diversity, but it also could happen if authors were looking at different thallus sectors within the same species (e.g., see listed Porphyra species in Cole, 1990).
It has been speculated that the basic number for this genus would be 3 for North Pacific Porphyra species (Yabu, 1975; Mumford and Cole, 1977) and 4 for North Atlantic species (Kapraun and Freshwater, 1987). The other chromosome numbers would then have evolved from this basic number by loss or gain of chromosomes during irregular separations in mitosis, chromosome doubling, fusion or fission (Krishnamurthy, 1984). All the evidence available therefore suggests that no matter the geographical area, the basic chromosome number should be 2, since it is the minimum chromosome number found in any species of Porphyra and other bladed Bangiales, including when intraspecific variation (different chromosome numbers in the same individual) is found within a species. For example, in an individual blade with cells with 2 chromosomes in one section of the thallus and 4 in another section, assuming 4 as the basic chromosome number would imply that cells with only 2 more than likely would die since they would be missing half of the DNA content of the basic complement and they could not perform their basic functions. However assuming 2 chromosomes as the minimal or basic chromosome number, all the aneuploid cells would be hyperploid (with extra chromosomes), so there would not be missing genes and all essential functions would be covered. Within the context of polyploid or hyperploid number of chromosomes, variations on chromosome number are more easily explained. This hypothesis led us again to explain the data by SC1. An extended karyotype for each species, including the gametophytic and the sporophytic stages, the dimensions and morphology of each chromosome, as well as a linkage map for each chromosome is clearly needed and it would help to resolve this conundrum. Such information can help to determine what is the minimal genome size and basic chromosome number required for a Porphyra species to survive, and the minimal set of genes needed to perform its essential biological functions.
Cytotype Diversity at the Central Area of Portugal
The geographical distribution of the cytotypes differed between the three species, with some sites having a higher number of mixoploids and others with a higher number of diploid blades. P. linearis was the species with the highest number of mixoploids, regardless of sampling site. It is also possible that the distribution of the mixoploids is mostly according to geography across the species barriers, since the southern populations (Parede, Paço de Arcos and Belém) presented more mixoploids, and the northern populations (Figueira, Leirosa MS1, Leirosa MS2) presented mostly diploid blades. However, several sites were very close together, and all are located around the central part of Portugal, therefore more populations and geographical areas would be needed to determine the geographical pattern of cytotypes of these species along the European coasts. In addition, mixogenome blades were present in all sites for the three species with no clear geographical pattern, with the exception of Paço de Arcos for P. linearis where only one genome type was found.
Ploidy Levels in the Context of Multiple Alleles
One of the main remaining questions is how to integrate the results of multiple genome sizes with the results of heterozygosity in Porphyra species. The presence of two or more alleles in the gametophytic blades has been explained by the chimeric nature of the blade (e.g., Lindstrom, 1993; Kong et al., 2009), but also by other mechanisms such as chromosomal reorganization, diploidy and polyploidy. The hypothesis of multiple alleles by chimerism has previously been tested by genotyping different parts of the blades (Fujio et al., 1988), revealing all tested gametophytes to be heterozygotes; this was used as argument against the hypothesis of haploid chimeric blades and in favor of blades that were diploid or had duplicated sets of chromosomes. More recently, Niwa and co-authors in several studies (e.g., Niwa and Sakamoto, 2010; Niwa et al., 2010, 2018) found many sources of evidence for polyploidy on Japanese species of Porphyra and Pyropia. Among their results, they found that gametophytic blades from both natural and cultivated populations were heterozygous at microsatellite loci, corroborating their results from chromosome counts. Archeospores – spores from asexual reproduction – also displayed heterozygous genotypes. In addition, we previously reported Porphyra along the European coasts to have multiple alleles per locus in a single individual (Varela-Álvarez et al., 2017, 2018a), with up to 4 alleles per locus in vegetative tissue (Varela-Álvarez et al., 2018b), which is incompatible with a simple haploid/diploid system.
Our results showing that the blade contains multiple genome sizes that can be associated with different chromosomal mosaics with multiple alleles support the hypothesis that these multiple alleles are probably the combination of both genetic chimeras and genome duplication events. This is because in a context of an exclusively haploid chimeric system, we would have found only a maximum of 2 alleles per locus, but we have found up to 4 alleles in vegetative thalli (Varela-Álvarez et al., 2018b). All three hypotheses of possible scenarios described above are compatible with the presence of multiple alleles when these exist in the genome, either as several copies of the same genome within the blade (SC1 and SC2) or by duplication of some chromosomes (SC3). However, only under SC1 or SC2 we would have heterozygote samples with more than 2 alleles coming from chimeric blades which can be either 2x or 4x, either as a uniform blade or as a mixoploid with combined ploidy levels. Under SC3, heterozygote samples with more than two alleles could be formed by mitotic recombination, which might be a less likely scenario for such a widespread pattern.
The Aneuploid/Euploid Chimeric Porphyra System
Regardless of the scenario, our results show that the Porphyra system is an aneuploid/euploid system composed of blades with different cell lines containing different ploidy levels in a sectorial way in mosaics, patterned or not patterned along the thalli. Aneuploidy is not an exception to the rule and it seems to be quite frequently incorporated in this biological system, having an aneuploid genome type with multiple rounds of genome duplications. These duplication events in the gametophytic thalli can be nuclei arrested in the G2 of the cell cycle at a single level, or a new complete round of genome duplication and consequently a distinct ploidy level, or yet a combination of both. In addition, the presence of heterozygotes and even blades with more than two alleles, suggest the presence of true polyploidy in these three species.
Genetic chimeras or organisms with more than one cell line have been also reported for other algal species, such as some species of Gracilaria (e.g., van der Meer, 1977; van der Meer and Zhang, 1988; Santelices et al., 1996; Meneses et al., 1999) and species from the order Laminariales (González and Santelices, 2017). Those chimeras are mostly formed by simultaneous development of two or more genetically distinct spores, however the precise mechanism that generates these algal chimeric thalli, is still obscure, and more studies are needed. In the chimeric aneuploid/euploid Porphyra system, most of the sites studied revealed a high frequency of chimeric diploid blades, 2Ca or 2Cb or 2Cab, and chimeric mixoploids 1C/2C, 2C/4C, 1C/4C of both genomes a or b or ab. In some individuals, the 2C genome size for either genome type a or genome type b can be interpreted as a diploids 2C = 2x or alternatively as a transitional diploid, 2C = 1x (Arrested at G2), where haploid cells would act as diploid with two copies of their genome during most of their life-time. We wonder if the mechanisms for generating the genetic chimeras or genetic mosaics are also related to the mechanisms generating the different genome sizes or cytochimeras. The alternative, that these two phenomena are independent, seems less likely given the strong coincidence between genome sizes and genotypes. The Porphyra system, at least the gametophytic generation, contains both phenomena, the generation of genetic chimeras probably as source of genetic diversity (González and Santelices, 2017), but also multiple ploidy levels, which can increase heterozygosity and also buffer the effect of lethal mutations in the gametophytic phase (Goff and Coleman, 1990). However, the presence of an aneuploid genome type leaves many questions unanswered such as how a cell line or individuals cells can cope with putatively missing genes (other than all being duplicated), as well as the production of the tetrads with the different cell lines with different genome sizes. Within the context of polyploid or hyperploid genomes types, genome size variations are more easily explained though. Clearly, further researcher is needed in that direction, which may shed light into the evolutionary origin of the gametophytic generation in this enigmatic algal group.
Data Availability Statement
The original contributions presented in the study are included in the article/Supplementary Material, further inquiries can be directed to the corresponding author.
Author Contributions
EV-Á conceived the initial idea following discussion with all the authors, performed the field collections, analyzed the data, and wrote the first draft of the manuscript. EV-Á, JL, and MC performed the flow cytometric measurements. EV-Á, JL, PM, and ES contributed to the interpretation of the data. All the authors reviewed and assisted substantially with the manuscript development and also approved the submitted version.
Funding
This work was supported by FCT (Fundação para a Ciência e a Tecnologia, Portugal) through SFRH/BPD/109452/2015 in transitional norm DL 57/2016/CP1361/CT0037 both attributed to EV-Á, SFRH/BSAB/150485/2019 to ES, and UIDB/04326/2020 to CCMAR.
Conflict of Interest
The authors declare that the research was conducted in the absence of any commercial or financial relationships that could be construed as a potential conflict of interest.
Acknowledgments
We thank Borna Kukurin for his help with the samples at the flow cytometer. We also thank Ana Afonso for useful discussions in plant ploidy.
Supplementary Material
The Supplementary Material for this article can be found online at: https://www.frontiersin.org/articles/10.3389/fmars.2021.628183/full#supplementary-material
Supplementary Figure 1 | Flow cytometric diagram to illustrate how genome sizes are calculated in this study.
Supplementary Figure 2 | Boxplots displaying the overall genome size variation in vegetative thalli and zygotospores in P. linearis, P. umbilicalis and P. dioica. The blue + near the median bar indicates location of the sample means.
Supplementary Table 1 | Flow cytometry data set. Detailed data set containing relative fluorescence intensities and CV (Coefficient of Variation) of the 670 vegetative thallus samples and controls of the nuclei at each sample analyzed in this study with their corresponding genome sizes and inferred ploidy levels.
Supplementary Table 2 | Glossary list of ploidy-related terms used in this study to explain the nuclear patterns of Porphyra samples.
Supplementary Table 3 | Summary of genome sizes in blades with two or three thallus transects in P. umbilicalis.
Footnotes
References
Badilla, R., Von Brand, E., and Collantes, G. (2008). Karyotypes and microfluorometric studies in Porphyra columbina Montagne (Bangiales, Rhodophyta), with reference to morphology and sexuality. Rev. Biol. Mar. Oceanogr. 43, 17–23.
Biémont, C. (2008). Within-species variation in genome size. Heredity 101, 297–298. doi: 10.1038/hdy.2008.80
Blouin, N. A., Brodie, J. A., Grossman, A. C., Xu, P., and Brawley, S. H. (2011). Porphyra: a marine crop shaped by stress. Trends Plant Sci. 16, 29–37. doi: 10.1016/j.tplants.2010.10.004
Bourne, E. C., Mina, D., Gonçalves, S. C., Loureiro, J., Freitas, H., and Muller, L. A. H. (2014). Large and variable genome size unrelated to serpentinea daptation but supportive of cryptic sexuality in Cenococcum geophilum. Mycorrhiza 24, 13–20. doi: 10.1007/s00572-013-0501-3
Brodie, J. A., and Irvine, L. M. (2003). Seaweeds of the British Isles: Volume 1 Rhodophyta. Part 3B Bangiophycidae. London: Natural History Museum.
Chu, Z. -F., Wen, J., Yang, Y.-P., Nie, Z.-L., and Meng, Y. (2018). Genome size variation and evolution in the grape family Vitaceae: genome size variation in Vitaceae. J. Syst. Evol. 56, 273–282. doi: 10.1111/jse.12310
Cock, J., Sterck, L., Rouzé, P., Scornet, D., Allen, A. E., Amoutzias, G., et al. (2010). The Ectocarpus genome and the independent evolution of multicellularity in brown algae. Nature 465, 617–621.
Cole, K. M. (1990). “Chromosomes,” in Biology of the Red Algae, eds K. M. Cole and R. G. Sheath (Cambridge: Cambridge University Press), 221–258.
Conway, E., and Cole, C. (1973). Observations on an unusual form of reproduction in Porphyra (Rhodophyceae, Bangiales). Phycologia 12, 213–225. doi: 10.2216/i0031-8884-12-3-213.1
Coyer, J. A., Hoarau, G., Pearson, G. A., Serrão, E. A., Stam, W. T., and Olsen, J. L. (2006). Convergent adaptation to a marginal habitat by homoploid hybrids and polyploidy ecads in the seaweed genus Fucus. Biol. Lett. 2, 405–408. doi: 10.1098/rsbl.2006.0489
De Clerck, O., Kao, S. M., Bogaert, K. A., Blomme, J., Foflonker, F., Kwantes, M., et al. (2018). Insights into the evolution of multicellularity from the sea lettuce genome. Curr. Biol. 28, 2921–2933.
Doležel, J., and Greilhuber, J. (2010). Nuclear genome size: are we getting closer? Cytometry Part A 77, 635–642. doi: 10.1002/cyto.a.20915
Doležel, J., Sgorbati, S., and Lucretti, S. (1992). Comparison of three DNA fluorochromes for flow cytometric estimation of nuclear DNA content in plants. Physiol. Plant. 85, 625–631.
Drew, K. M. (1949). Conchocelis-phase in the life-history of Porphyra umbilicalis (L.) Kutz. Nature 164, 748–749. doi: 10.1038/164748a0
Freshwater, D. W., and Kapraun, D. F. (1986). Field, culture and cytological studies of Porphyra carolinensis Coll et Cox (Bangiales, Rhodophyta) from North Carolina. Jap. J. Phycol. 34, 251–262.
Fujio, Y., Gil-Kodaka, P., Hara, M., and Akiyama, K. (1988). Electrophoretic variants characteristic of heterozygotes in haploid laver Porphyra sp. Nippon Suisan Gakkaishi 54, 969–974. doi: 10.2331/suisan.54.969
Garbary, D. J., and Clarke, B. (2002). Intraplant variation in nuclear DNA content in Laminaria saccharina and Alaria esculenta (Phaeophyceae). Bot. Mar. 45, 211–216.
Goff, L. J., and Coleman, A. W. (1984). Elucidation of fertilization and development in a red algal by quantitative DNA microspectrofluorometry. Dev. Biol. 102, 173–194. doi: 10.1016/0012-1606(84)90183-0
Goff, L. J., and Coleman, A. W. (1986). A novel pattern of apical cell polyploidy, sequential polyploidy reduction and intercellular nuclear transferrin the red alga Polysiphonia. Am. J. Bot. 73, 1109–1130. doi: 10.2307/2443791
Goff, L. J., and Coleman, A. W. (1987). The solution to the cytological paradox of isomorphy. J. Cell Biol. 104, 739–748. doi: 10.1083/jcb.104.3.739
Goff, L. J., and Coleman, A. W. (1990). “DNA microspectrofluorometric studies,” in Biology of the Red Algae, eds K. M. Cole and R. G. Sheath (New York NY: Cambridge University Press), 43–72.
González, A. V., and Santelices, B. (2017). Frequency of chimerism in populations of the kelp Lessonia spicata in central Chile. PLoS One 12:e0169182. doi: 10.1371/journal.pone.0169182
Holmes, M. J., and Brodie, J. (2004). Morphology, seasonal phenology and observations on some aspects of the life history in culture of Porphyra dioica (Bangiales, Rhodophyta) from Devon, UK. Phycologia 43, 176–188. doi: 10.2216/i0031-8884-43-2-176.1
Kapraun, D. F. (2005). Nuclear DNA content estimates in multicellular green, red and brown algae: phylogenetic considerations. Ann. Bot. 95, 7–44. doi: 10.1093/aob/mci002
Kapraun, D. F., and Freshwater, D. W. (1987). Karyological studies of five species of Porphyra (Bangiales, Rhodophyta) from the North Atlantic and Mediterranean. Phycologia 26, 82–87. doi: 10.2216/i0031-8884-26-1-82.1
Kapraun, D. F., and Freshwater, D. W. (2012). Estimates of nuclear DNA content in red algal lineages. AoB PLANTS 2012:pls005. doi: 10.1093/aobpla/pls005
Kapraun, D. F., Hinson, T. K., and Lemus, A. J. (1991). Karyology and cytophotometric estimation of inter- and intraspecific nuclear DNA variation in four species of Porphyra (Rhodophyta). Phycologia 30, 458–466. doi: 10.2216/i0031-8884-30-5-458.1
Kito, H. (1966). Cytological studies of several species of Porphyra I. Morphological and cytological observations on a species of Porphyra epiphytic on Grateloupia filicina var. porracea (Mert.) Howe. Bull. Fac. Fish. Hokkaido Univ. 16, 206–208.
Kito, H. (1978). Cytological studies on genus Porphyra. Bull. Tohoku Reg. Fish. Res. Lab. 39, 29–84. (in Japanese).,Google Scholar
Kito, H., Ogata, E., and McLachlan, J. (1971). Cytological observations on three species of Porphyra from the Atlantic. Bot. Magazine, Tokyo 84, 141–148. doi: 10.15281/jplantres1887.84.141
Kito, H., Yabu, H., and Tokida, J. (1967). The number of chromosome in some species of Porphyra. Bull. Fac. Fish. Hokkaido Univ. 18, 59–60.
Kong, F. N., Mao, Y. X., Yang, H., Qu, H. J., Yan, X. H., and Wang, L. (2009). Genetic analysis of Porphyra yezoensis using microsatellite markers. Plant Mol. Biol. Rep. 27, 496–502. doi: 10.1007/s11105-009-0101-8
Krishnamurthy, V. (1959). Cytological investigations on Porphyra umbilicalis (L.) Kütz. var. laciniata (Lightf.) J.Ag. Ann. Bot. 23, 147–176. doi: 10.1093/oxfordjournals.aob.a083637
Le Gall, Y., Brown, S., Dominique, M., Meijjad, M., and Kloareg, B. (1993). Quantification of nuclear DNA and G-C content in marine macroalgae by flow cytometry of isolated nuclei. Protoplasma 173, 123–132. doi: 10.1007/bf01379001
Lindstrom, S. C. (1993). Inter and intrapopulation genetic variation in species of Porphyra (Rhodophyta: Bangiales) from British Columbia and adjacent waters. J. Appl. Phycol. 5, 53–62. doi: 10.1007/bf02182422
Loureiro, J., Kopecký, D., Castro, S., Santos, C., and Silveira, P. (2007). Flow cytometric and cytogenetic analyses of Iberian Peninsula Festuca spp. Plant Syst. Evol. 269, 89–105. doi: 10.1007/s00606-007-0564-8
Magne, F. (1952). La structure du noyau et le cycle nucleaire chez Ie Porphyra linearis Greville. C. R. Hebd. Séances Acad. Sci. 234, 986–988.
Matsuyama-Serisawa, K., Yamamoto, M., Fujishita, M., Endo, H., Serisawa, Y., Tabata, S., et al. (2007). DNA content of the cell nucleus in the macroalga Porphyra yezoensis (Rhodophyta). Fish. Sci. 73, 738–740. doi: 10.1111/j.1444-2906.2007.01389.x
Meneses, I., Santelices, B., and Sanchez, P. (1999). Growth-related intraclonal genetic changes in Gracilaria chilensis (Gracilariales: Rhodophyta). Mar. Biol. 135, 391–397. doi: 10.1007/s002270050639
Mitman, G. G., and van der Meer, J. P. (1994). Meiosis, blade development, and sex determination in Porphyra purpurea (Rhodophyta). J. Phycol. 30, 147–159. doi: 10.1111/j.0022-3646.1994.00147.x
Mumford, T. F. Jr., and Cole, K. (1977). Chromosome numbers for fifteen species in the genus Porphyra (Bangiales, Rhodophyta) from the west coast of North America. Phycologia 16, 373–377. doi: 10.2216/i0031-8884-16-4-373.1
Niwa, K., Abe, T., and Kobiyama, A. (2018). Possibility of polyploidy breeding using cryptic species in the marine crop Pyropia yezoensis (Bangiales, Rhodophyta). J. Appl. Phycol. 30, 1197–1205. doi: 10.1007/s10811-017-1317-x
Niwa, K., and Abe, T. (2012). Chimeras with mosaic patterns in archeospore germlings of Pyropia yezoensis Ueda (Bangiales, Rhodophyta). J. Phycol. 48, 706–709. doi: 10.1111/j.1529-8817.2012.01143.x
Niwa, K., and Sakamoto, T. (2010). Allopolyploidy in natural and cultivated populations of Porphyra (Bangiales, Rhodophyta). J. Phycol. 46, 1097–1105. doi: 10.1111/j.1529-8817.2010.00897.x
Niwa, K., Hayashi, Y., Abe, T., and Aruga, Y. (2009). Induction and isolation of pigmentation mutants of Porphyra yezoensis (Bangiales, Rhodophyta) by heavy-ion beam irradiation. Phycol. Res. 57, 194–202. doi: 10.1111/j.1440-1835.2009.00539.x
Niwa, K., Kobiyama, A., and Sakamoto, T. (2010). Interspecific hybridization in the haploid blade- forming marine crop Porphyra (Bangiales, Rhodophyta): occurrence of allopolyploidy in surviving F1 gametophytic blades. J. Phycol. 46, 693–702. doi: 10.1111/j.1529-8817.2010.00853.x
Niwa, K., Miura, A., Shin, J. A., and Aruga, Y. (1993). Characterization and genetic analysis of the violet type pigmentation mutant of Porphyra yezoensis Ueda (Bangiales, Rhodophyta). Korean J. Phycol. 8, 217–230. doi: 10.1111/j.1440-1835.1997.tb00079.x
Ohme, M., and Miura, A. (1988). Tetrad analysis in conchospore germlings of Porphyra yezoensis (Rhodophyta, Bangiales). Plant Sci. 57, 135–140. doi: 10.1016/0168-9452(88)90079-9
Park, E.-J., Fukuda, S., Endo, H., and Saga, N. (2007). Genetic polymorphism within Porphyra yezoensis (Bangiales, Rhodophyta) and related species from Japan and Korea detected by cleaved amplified polymorphic sequence analysis. Eur. J. Phycol. 42, 29–40. doi: 10.1080/09670260601127681
Phillips, N., Kapraun, D. F., Gómez Garreta, A., Ribera Siguan, M. A., Rull, J. L., Salvador Soler, N., et al. (2011). Estimates of nuclear DNA content in 98 species of brown algae (Phaeophyta). AoB PLANTS 2011:plr001.
Pineda-Krch, M., and Lehtila, K. (2004). Costs and benefits of genetic heterogeneity within organisms. J. Evol. Biol. 17, 1167–1177. doi: 10.1111/j.1420-9101.2004.00808.x
Sahoo, D., Tang, X., and Yarish, C. (2002). Porphyra—the economic seaweed as a new experimental system. Curr. Sci. 83, 1313–1316.
Santelices, B., Correa, J. A., Meneses, I., Aedo, D., and Varela, D. (1996). Sporeling coalescence and intraclonal variation in Gracilaria chilensis (Gracilariales, Rhodophyta). J. Phycol. 32, 313–322. doi: 10.1111/j.0022-3646.1996.00313.x
Šmarda, P., and Bureš, P. (2006). Intraspecific DNA content variability in Festuca pallens on different geographical scales and ploidy levels. Ann. Bot. 98, 665–678. doi: 10.1093/aob/mcl150
Sutherland, J. E., Lindstrom, S. C., Nelson, W. A., Brodie, J., Lynch, M. D. J., Hwang, M. S., et al. (2011). A new look at an ancient order: generic revision of the Bangiales (Rhodophyta). J. Phycol. 47, 1131–1151. doi: 10.1111/j.1529-8817.2011.01052.x
Tavares, S., Ramos, A. P., Pires, A. S., Azinheira, H. G., Caldeirinha, P., Link, T., et al. (2014). Genome size analyses of Pucciniales reveal the largest fungal genomes. Front. Plant Sci. 5:422. doi: 10.3389/fpls.2014.00422
van der Meer, J. P. (1977). Genetics of Gracilaria sp. (Rhodophyceae, Gigartinales) II. The life history and genetic implications of cytokinetic failure during tetraspores formation. Phycologia 16, 367–371. doi: 10.2216/i0031-8884-16-4-367.1
van der Meer, J. P., and Zhang, X. (1988). Similar unstable mutations in three species of Gracilaria. J. Phycol. 24, 198–202. doi: 10.1111/j.1529-8817.1988.tb04234.x
Varela-Álvarez, E., Balau, A. C., Paulino, C., Berecibar, E., Pearson, G., and Serrão, E. A. (2018a). Isolation and characterization of microsatellite markers for the red alga Porphyra umbilicalis. Plant Genet. Resour. 16, 390–393. doi: 10.1017/s147926211700034x
Varela-Álvarez, E., Gómez-Garreta, A., Rull-Lluch, J., Salvador Soler, N., Serrão, E. A., and Ribera Siguán, M. A. (2012). Mediterranean species of Caulerpa are polyploid with smaller genomes in the invasive ones. PLoS One 7:e47728. doi: 10.1371/journal.pone.0047728
Varela-Álvarez, E., Loureiro, J., Paulino, C., and Serrão, E. A. (2018b). Polyploid lineages in the Porphyra genus. Sci. Rep. 8:8696.
Varela-Álvarez, E., Paulino, C., and Serrão, E. A. (2017). Development and characterization of twelve microsatellite markers for Porphyra linearis Greville. Genetica 145, 127–130.
Varela-Álvarez, E., Stengel, D., Rindi, F., and Guiry, M. D. (2005). Chromosome studies of two populations of Porphyra linearis Greville from the West Coast of Ireland and from U.S.A. Phycologia 44, 61–65.
Wang, J., Zhu, J., Zhou, W., Jiang, P., Qin, S., and Xu, P. (2010). Early development patterns and morphogenesis of blades in four species of Porphyra (Bangiales, Rhodophyta). J. Appl. Phycol. 22, 297–303.
Yabu, H. (1975). “Cytological studies of the Rhodophyta and Chlorophyta,” in Advance of Phycology in Japan, eds J. Tokida and H. Hirose (The Hague: W. Junk), 125–135.
Yabu, H. (1978). Chromosome numbers in species of Porphyra from Nova Scotia, Canada. Jap. J. Phycol. 26, 97–104.
Yang, E., Boo, S., Bhattacharya, D., Saunders, G. W., Knoll, A. H., Fredericq, S., et al. (2016). Divergence time estimates and the evolution of major lineages in the Florideophyte red algae. Sci. Rep. 6:21361.
Zhong, C., and Yan, X. (2020). Haploid spontaneous diploidization during apogamy of male gametophytes in Pyropia haitanensis (Bangiales, Rhodophyta). J. Appl. Phycol. 32, 1395–1403.
Keywords: cytochimera, genetic mosaics, Nori, mixogenome, mixoploids, polyploid, red algae
Citation: Varela-Álvarez E, Loureiro J, Meirmans PG, Castro M and Serrão EA (2021) Genomes Vary in Size and Spatial Patterns Within Chimeric Blades of Porphyra spp.. Front. Mar. Sci. 8:628183. doi: 10.3389/fmars.2021.628183
Received: 11 November 2020; Accepted: 22 February 2021;
Published: 24 March 2021.
Edited by:
Koji Mikami, Miyagi University, JapanReviewed by:
Thomas Wichard, Friedrich Schiller University Jena, GermanyHwan Su Yoon, Sungkyunkwan University, South Korea
Linbin Huang, Shanghai Ocean University, China
Copyright © 2021 Varela-Álvarez, Loureiro, Meirmans, Castro and Serrão. This is an open-access article distributed under the terms of the Creative Commons Attribution License (CC BY). The use, distribution or reproduction in other forums is permitted, provided the original author(s) and the copyright owner(s) are credited and that the original publication in this journal is cited, in accordance with accepted academic practice. No use, distribution or reproduction is permitted which does not comply with these terms.
*Correspondence: Elena Varela-Álvarez, ZXZhcmVsYUB1YWxnLnB0