- 1Australian Institute of Marine Science, Townsville, QLD, Australia
- 2James Cook University, Townsville, QLD, Australia
- 3National Marine Science Centre, Southern Cross University, Coffs Harbour, NSW, Australia
- 4School of BioSciences, The University of Melbourne, Parkville, VIC, Australia
Novel restoration methods are currently under consideration worldwide to help coral reefs recover or become more resilient to higher temperature stress. Critical field-based information concerning the paradigm of “local is best” is lacking for many methods; information which is essential to determine the risk and feasibility associated with restoration. One method involves breeding corals from different reef regions with expected variation in heat tolerance and moving those offspring to new locations to enhance offspring survival; thereby augmenting local stock to enhance survival for anticipated warming. In this study, surviving colonies from the 2016 to 2017 mass bleaching events on the Great Barrier Reef (GBR) were reproductively crossed and they included colonies sourced from northern (three) and central (two) reefs. The gravid colonies of Acropora tenuis were collected across 6° of latitude, and they were spawned to produce a total of 17 purebred and hybrid crosses. Juvenile corals (3,748 individual colonies settled on 1,474 terracotta tiles) were deployed to Davies reef in the central GBR after 4 months of aquarium rearing. Survival, growth, and coral colour (as a proxy for bleaching) were assessed after 0, 91, and 217 days of field deployment. Overall, a high percentage of juveniles (17% ± 2.5 SE) survived relative to expected survival at the final census. Survival was significantly higher for central purebred crosses, hybrid crosses had intermediate survival while northern purebreds had the lowest survival. Colour and growth rates (0.001−0.006 mm2 day–1) were not significantly different amongst central, northern, or hybrid crosses but were of a reverse pattern compared to survival. On average, northern purebred crosses grew the fastest, followed by hybrid crosses, and then central purebred crosses. Modelled growth trajectories suggest that northern purebreds would take 8 years to grow to reproductive size, hybrids would take nine, and central purebreds would require 12. All deployed juvenile corals paled over time in the field although the colour of A. tenuis juveniles did not differ significantly amongst central, northern, or hybrid crosses. Growth and survival trade-off analysis showed that although most crosses did not outperform the native central juveniles, two of the eight hybrid crosses (SBxLS, DRxCU) demonstrated faster time to reproductive age and increased survival. Overall, reduced time to reach reproductive size and minimal trade-offs in at least two of the eight hybrids suggest that these crosses may accelerate and supplement recovery through natural re-seeding of genes sourced from northern reefs.
Introduction
Local adaptation occurs when selection acts upon the standing genetic variation within populations to increase fitness under local environmental conditions (Aitken and Whitlock, 2013). It is a pervasive evolutionary process that has been documented across many kingdoms of life (Kawecki and Ebert, 2004), driven by the strength and direction of selection (Barrett and Schluter, 2008). The formation of local adaptation is generally negatively correlated with gene flow (Whiteley et al., 2015), although it is possible to maintain structured populations even under scenarios of widescale dispersal and gene flow (e.g., in marine organisms exhibiting planktonic dispersal) (Sanford and Kelly, 2011). As ocean temperatures rise, it is unclear how organisms that exhibit both high dispersal and strong signatures of local adaptation will fare. An understanding of these processes is urgent as ocean temperatures increase due to climate change and as acute thermal anomalies are becoming more frequent in the marine environment. Populations of marine organisms adapted to locally extreme thermal conditions may therefore represent reservoirs of standing genetic variation conducive to facilitating adaptation to future environmental conditions.
Reef-building corals are particularly vulnerable to ocean warming. These species are foundational to the functioning of coral reef ecosystems, but are dying at increasing rates (Hughes et al., 2018). Extensive variation in tolerance to bleaching and growth exists in corals (Jones and Berkelmans, 2011; Cunning et al., 2015a), across many habitat types (van Oppen et al., 2018), latitudes (Howells et al., 2012), and depths (van Oppen et al., 2011; Bongaerts et al., 2015). The presence of substantial standing genetic variation in key fitness traits is promising for the acceleration of thermal adaptation via the provisioning of genetic material by locally adapted populations in response to rapid rates of environmental change (Matz et al., 2017; Quigley et al., 2019). A number of novel methods for genetic management and interventions aim to facilitate the spread of adaptive genetic variation (Aitken and Whitlock, 2013). Interventions may include the movement of heat adapted adult or juvenile corals or the ex situ breeding of locally adapted populations (hybridisation) combined with the subsequent movement of offspring to cooler receiving reefs, generally described as Assisted Gene Flow (Aitken and Whitlock, 2013). The intent of these interventions is to increase standing genetic variation and facilitate the adaptation of populations faster than would occur under current rates of gene flow (Quigley et al., 2019).
The extent to which populations are locally adapted has important implications for the translocation of organisms and for hybridisation of organisms both within and outside their known distributions, where the extent of local adaptation can influence the magnitude of trade-offs between different traits. Trade-offs occur if a locally adapted trait results in a change of relative fitness under different environmental conditions. The correlation between local adaptation and fitness trade-offs does not appear to be strong in a survey of plant and animal taxa (Hereford, 2009) but there are limited studies examining such trade-offs in reef-building corals, especially in the wild. Several studies to date suggest a high cost of translocation. For example, fragments made from Acropora millepora adults reciprocally transplanted between central and southern GBR reefs had higher bleaching, increased mortality, slower growth, and changes to symbiont communities and reproductive timing on translocated reefs compared to native reefs (Howells et al., 2013). Similarly, fragments of Porites astreoides adult corals translocated between inshore and offshore reefs in Florida showed a high degree of local adaptation and growth trade-offs at transplanted sites (Kenkel et al., 2015).
Trade-offs involving algal symbionts and combined host-symbiont (“holobiont”) responses for growth and heat tolerance are better documented. For example, trade-offs in heat tolerance and lipid composition and egg size were found in A. millepora hosting either Cladocopium or Durusdinium symbionts (Jones and Berkelmans, 2011). Adult Pocillopora damicornis corals associating with Cladocopium symbionts at 26°C exhibited higher growth compared to those associating with Durusdinium, with those differences disappearing at 29°C (Cunning et al., 2015b). However, experimental work has shown the incidence of trade-offs in adult corals to be minimal (Wright et al., 2019). Studies using juveniles produced from the hybridisation of gametes sourced from different coral populations have shown either negligible (Quigley et al., 2016) or strong effects of local adaptation (i.e., no survival benefits) (van Oppen et al., 2014), in which the difference in effect size may be driven by the outplant environment (northern × central hybrids to central site or central × southern hybrids to southern site). In the laboratory, several-fold benefits in survival and growth were recorded in hybrid juveniles with at least one warm-adapted dam and in symbiosis with Durusdinium symbionts (Quigley et al., 2020a). Taken together, results in corals from reciprocal translocations and population hybridisation show promise in understanding their use as intervention strategies to enhance the adaptive responses of corals to increasingly warm oceans. These differing responses suggest further work is needed to understand whether the presence and magnitude of trade-offs between traits poses a significant risk to the success of these genetic interventions.
This study looked at trade-offs associated with the “local is best” paradigm to investigate if juvenile corals with assumed higher temperature tolerance perform better or worse when transplanted to new habitats. To evaluate the benefits, risks, and feasibility of hybridisation in the wild, we selectively bred corals from two reef regions (five reefs) across > 6° of latitude and differing thermal environments. We combined this with a common garden experimental approach to examine survival, growth, and symbiosis of purebred and hybrid corals outplanted onto the central Great Barrier Reef. We also evaluated trade-offs in their performance and modelled reproductive potential as a proxy for reef recovery potential. This approach provides both fundamental and applied knowledge regarding the adaptive potential of hybrid reef corals, lessons useful for biodiversity and ecosystem management and conservation.
Materials and Methods
Coral Collection
Reproductively mature Acropora tenuis colonies were collected from three reefs in the far northern region of the Great Barrier Reef (GBR) and two reefs in the central region (Figure 1, ∼15 colonies per reef). These included, in the north, Curd (CU: −12.5850°S, 143.5115°E), Sand Bank 7 (SB: −13.4362°S, 143.9714°E), Long Sandy (LS: −12.5003°S, 143.7848°E), Davies (DR: −18.8217°S, 147.6495°E), and Backnumbers (BK: −18.5075°S, 147.1464°E) reefs in the central region. On average, the annual mean temperatures ranged between ∼28.7 and 29.4°C (eReefs, 01/01/2013–28/02/2018, daily measurements at 1 km resolution), with Davies representing the coolest reef (offshore, central) and Curd the warmest (inshore, north). These gravid colonies were transported to the Australian Institute of Marine Science for spawning (Townsville, Queensland, Australia). Colonies were held in outdoor holding tanks in the National Sea Simulator Facility (Seasim) at constant temperatures representative of each reef.
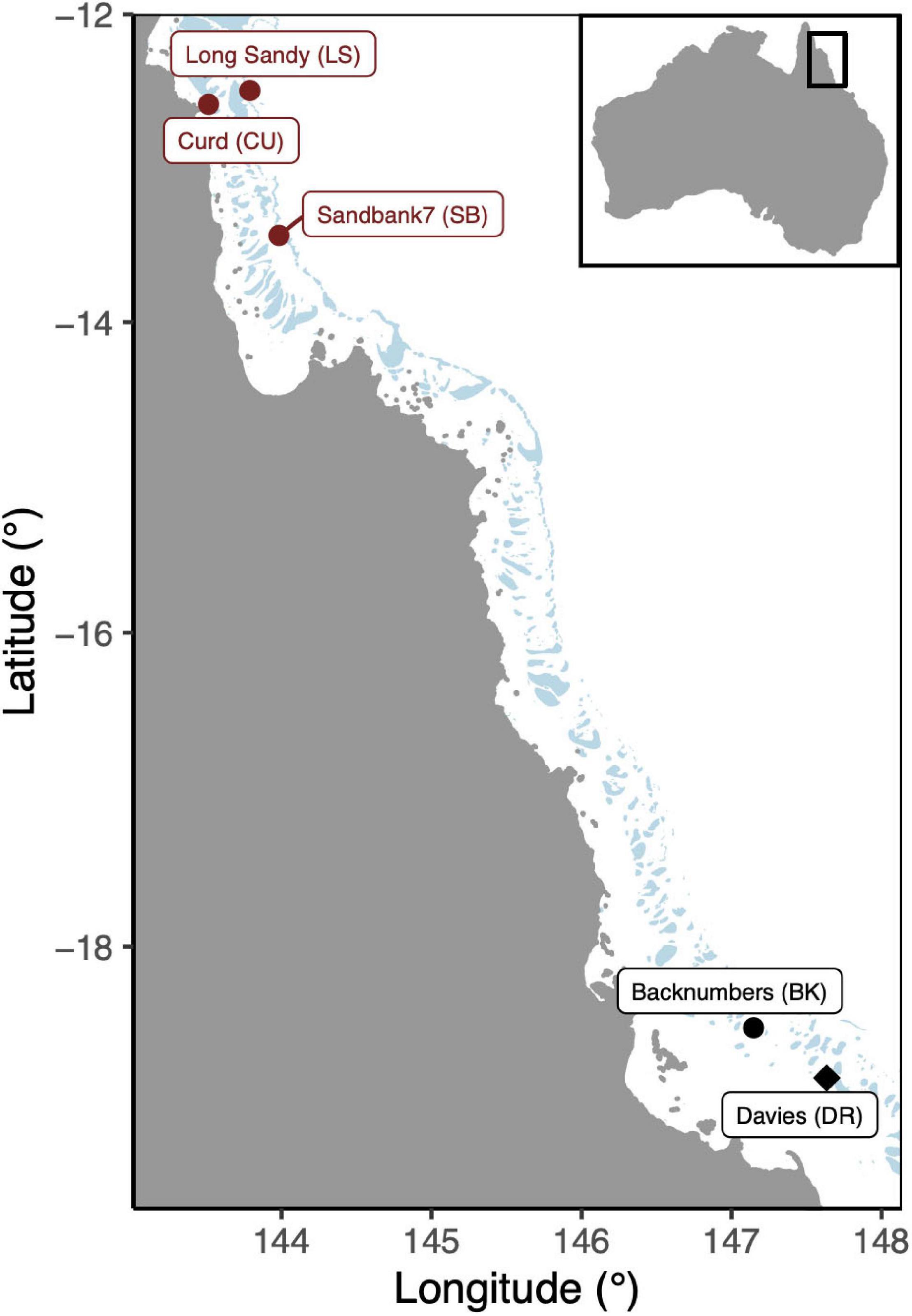
Figure 1. Map of reef locations were gravid Acropora tenuis colonies were sourced. Northern reefs are indicated in red while central reefs are indicated in black. The transplant site (“local” cross), Davies reef, is indicated with a diamond.
Larval Rearing and Deployment
Acropora tenuis colonies were isolated at dusk from the 26th to 29th of November 2018 into individual containers. Gametes were released between 18:00 and 19:30 h and collected from the water surface. Eggs and sperm were separated through a 120 μm sieve and washed three times using 0.2 μm filtered seawater (FSW). Spawning, fertilisation and rearing followed established methods (Quigley et al., 2016). Briefly, eggs were fertilised between 21:00 and 22:00 h by adding equal numbers of eggs from one parental colony to an equal concentration of sperm from a separate parent colony standardised to 1 × 106. Sperm cells were diluted to this concentration per litre following counts using an automated sperm counter (Computer -Assisted Semen Analysis-CASA equipment). Fertilisation success was verified by visually inspecting embryos every hour for 3 h under magnification. Embryos and developing larvae from each cross were added and maintained in separate 15 L flow-through rearing cones (1 larva mL–1). Additional embryos and larvae from purebred crosses were also kept in 500 L flow-through rearing tanks at the same density. In all, 17 crosses were used in this study (Table 1): four central dams × central sires (Central: central purebreds), four central × northern (Central-H: central hybrids), four northern × central (North-H: northern hybrids), and five northern × northern crosses (North: northern purebreds). Purebreds and hybrids distinguish intra- and inter-regional crosses, respectively. All potential cross combinations successfully produced larvae, but only 17 were selected for field deployment due to permit constraints to the number of tiles outplanted at the selected field site.
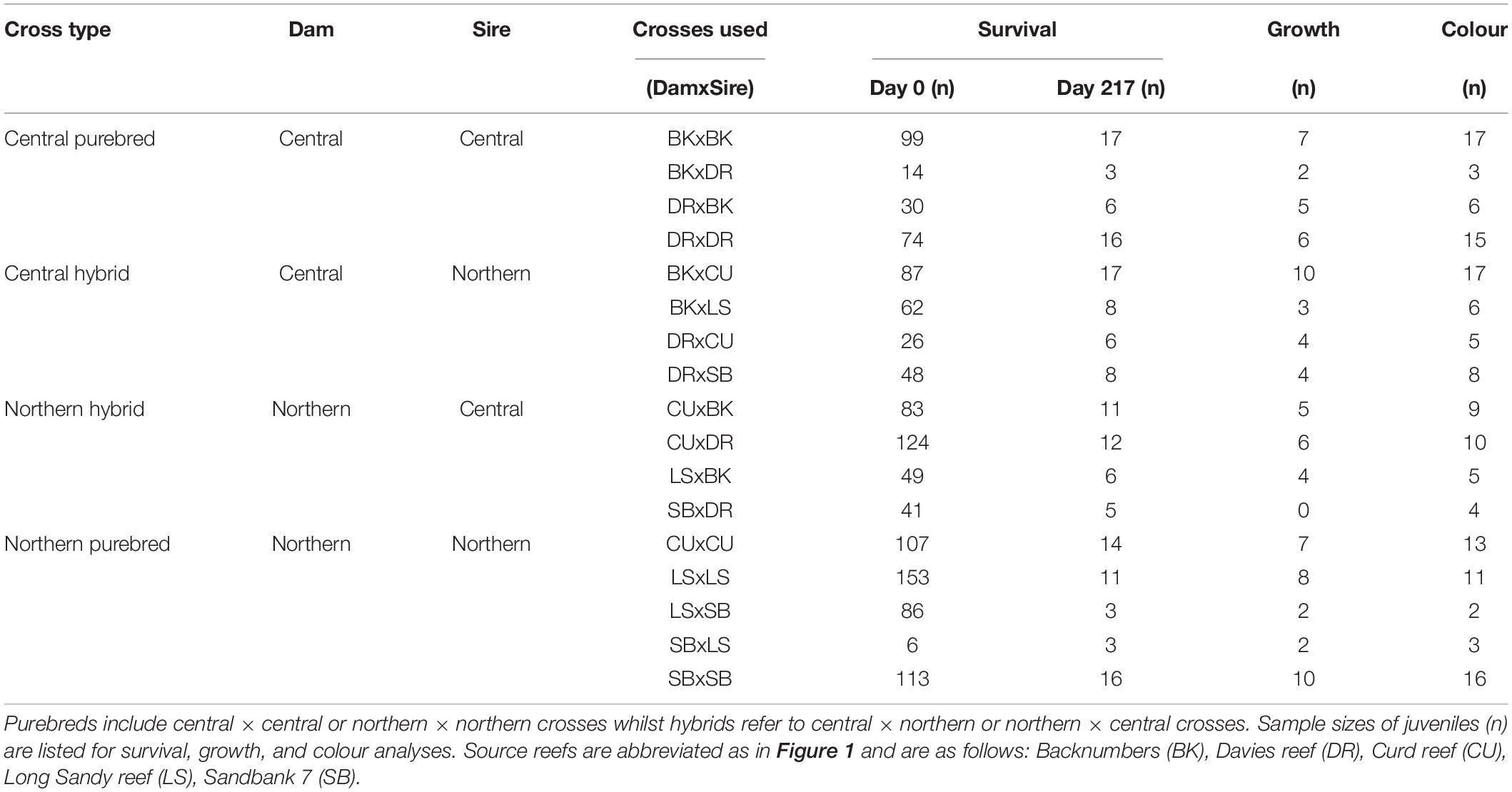
Table 1. Summary of sample sizes of juvenile corals analysed for each of the 17 reproductive crosses.
Larvae were settled in two batches, from the 5th to the 10th of December and again from the 11th to the 16th of December in 2018. Larvae from each cross were added to separate flow-through tanks per cross (1 μm FSW) containing terracotta tiles (n = 1,474 tiles) with added crushed crustose-coralline algae to induce settlement. These 1,474 tiles were laid flat across the bottom of each of the tanks or alternatively, hung vertically in groups of 10 tiles (separated by spacers). After the first batch of tiles was removed, a second set of tiles (plus freshly crushed crustose algae) were added to those tanks. Tiles were then transferred to flow-through outdoor tanks set at 27.5°C (1 μm FSW) that contained adult A. tenuis from Davies reef as a source of Symbiodiniaceae inoculation and held until deployment.
In March 2019, a total of 1,171 tiles with 3,748 juveniles were deployed to Davies reef using SCUBA (Figure 1 and Supplementary Table 1). Tiles were placed onto rods; rods were fixed into cassettes (two rods each); and cassettes were attached onto seven frames that were secured onto the sand at Davies reef. Each frame (1.074 × 2.9 m) contained 16 cassettes that held two rods of 10–16 tiles each. Tiles were orientated vertically and were evenly spaced with 1.5 cm PVC spacers to allow for growth. The position of each tile, rod, cassette, and frame was recorded during tile deployment.
Survival, Growth, and Colour
Survival, growth, and coral colour were measured immediately prior to deployment and after 91 and 217 days in the field. On days 91 and 217, tiles were retrieved using SCUBA, gently cleaned with a silicone brush, and juveniles were assessed by eye and then photographed, then the tiles were re-deployed into the exact same position. A final number of 1,202 juveniles were recorded as alive (including individuals suffering tissue loss) or dead.
Growth and colour were measured from digital images. Three solitary juveniles were selected per photograph for growth and colour measurements. Prior to deployment, juveniles were photographed using a Nikon D810 camera body, Nikon AF-S 60 mm micro lens, with each photo including a 2 cm scale bar and CoralWatch Health Chart (image resolution 7,360∗4,912 pixels; Siebeck et al., 2006). In the field, each juvenile was assessed by eye and photographed in the field with an Olympus Tough TG-5 camera with a 15 cm scale bar and CoralWatch Health chart (image resolution 4,000∗3,000 pixels) and Ikelite DS160 strobes attached to a stationary set-up. The camera was fixed within an Olympus PT-058 waterproof housing, X-Adventurer M1000 video light, and Olympus UFL-3 flash in a fixed frame for constant angle and distance. The housing was mounted onto a Hyperion pro tray and attached to a custom high-density polyethylene frame for consistency in angle and distance per tile photograph. For all photos, the camera lens was positioned 190 mm from the tile, consistent flash settings were used, and the frame contained a built-in coral health chart and scale bar.
Juvenile area was used to calculate growth, it was measured from the images using the polygon selection tool and calibrated to a 10 mm scale in ImageJ2 software (Rueden et al., 2017). The colour of the juveniles was used to indicate changes in Symbiodiniaceae cell density and/or chlorophyll content. Juvenile colour was matched to the closest category in the CoralWatch “D” coral health chart in each image by a single person to minimise observer bias.
Statistical Analysis
Survival was analysed using Kaplan-Meier survival curves in the R packages “survival” and “survminer” (Therneau, 2015; Kassambara et al., 2017). To evaluate differences in survival between the purebred and hybrid crosses, survival curves were estimated for each type with juveniles grouped by each cross. Survival curves also were estimated for each cross individually and compared to the local purebred juvenile corals at the outplant locations (DRxDR).
Pairwise post hoc tests were used to compare data collected from the purebred and hybrid crosses using the Peto and Peto test from the “survminer” package (Kassambara et al., 2017) and p-values were corrected for multiple comparisons using the Benjamini-Hochberg method of controlling the false discovery rate (Benjamini and Hochberg, 1995).
Growth
Linear growth rates were estimated for 85 juveniles that had two or more area measurements. Changes in mm2 area per day were analysed using a linear mixed model with type of cross as a fixed effect and settlement tile as a nested random effect to account for tiles with multiple juveniles (Bates et al., 2014; Kuznetsova et al., 2017). Residuals were inspected for normality and heterogeneity of variance.
To estimate the time until the juveniles from different crosses would reach reproductive size, exponential growth curves [At = A0∗(1 + r)t] were fitted using the R package “nlme” (Pinheiro et al., 2014) to the average areas (A) at day 0, 91, and 217 for each cross. Previous field-based measurements for this species suggest that reproductive maturity (∼4–5 years) is reached at a diameter of 20–25 cm (Iwao et al., 2010). This range of sizes is equivalent to a circular surface area of approximately 314 cm2 when converted using πr2. We solved the exponential growth curve for t when the area at time t (At) was 314 cm2. The median time required to reach this size was compared for each cross type. For comparison, the same calculation was applied to A. tenuis transplanted to Magnetic Island sourced from a similar field-based experiment using purebred juveniles from that reef (Quigley et al., 2020b), which is situated almost directly inshore of Davies reef (Figure 1).
Colour
The proportion of juveniles in each CoralWatch Health Chart category were analysed using a multinomial model with cross type and time as predictors and equidistant categories. A second multinomial model was used to determine whether changes in colour score corresponded to individual crosses rather than types of crosses.
Results
For survival and colour analysis, juveniles growing in contact with other juveniles or those that were dying (i.e., losing tissue) were not considered (i.e., filtered from the data), leaving 1,201 juveniles on 546 tiles for survival analysis and 768 juveniles and 509 tiles for colour analysis. Growth rates were measured per juvenile and included 85 juveniles with repeated area measurements across al timepoints.
Survival
Survivorship decreased from 100% at deployment (n = 1,202 filtered juveniles) to 30.8% ± 4.2 SE after 91 days and then to 17.1% ± 2.5 after 217 days in the field (Figure 2A). Survivorship curves differed significantly amongst the four types of crosses (central purebreds, central hybrids, northern purebreds, northern hybrids; [χ2(16) = 56.5, p < 0.001]. Central crosses had significantly higher survival compared to northern purebred and northern hybrid crosses (both p ≤ 0.001). Similarly, central hybrid crosses had significantly higher survival compared to northern purebreds (p = 0.050) but not northern hybrid crosses (p = 0.112). There was no significant difference in survival between central purebreds and central hybrids (p = 0.112) or between northern purebreds and northern hybrids (p = 0.695).
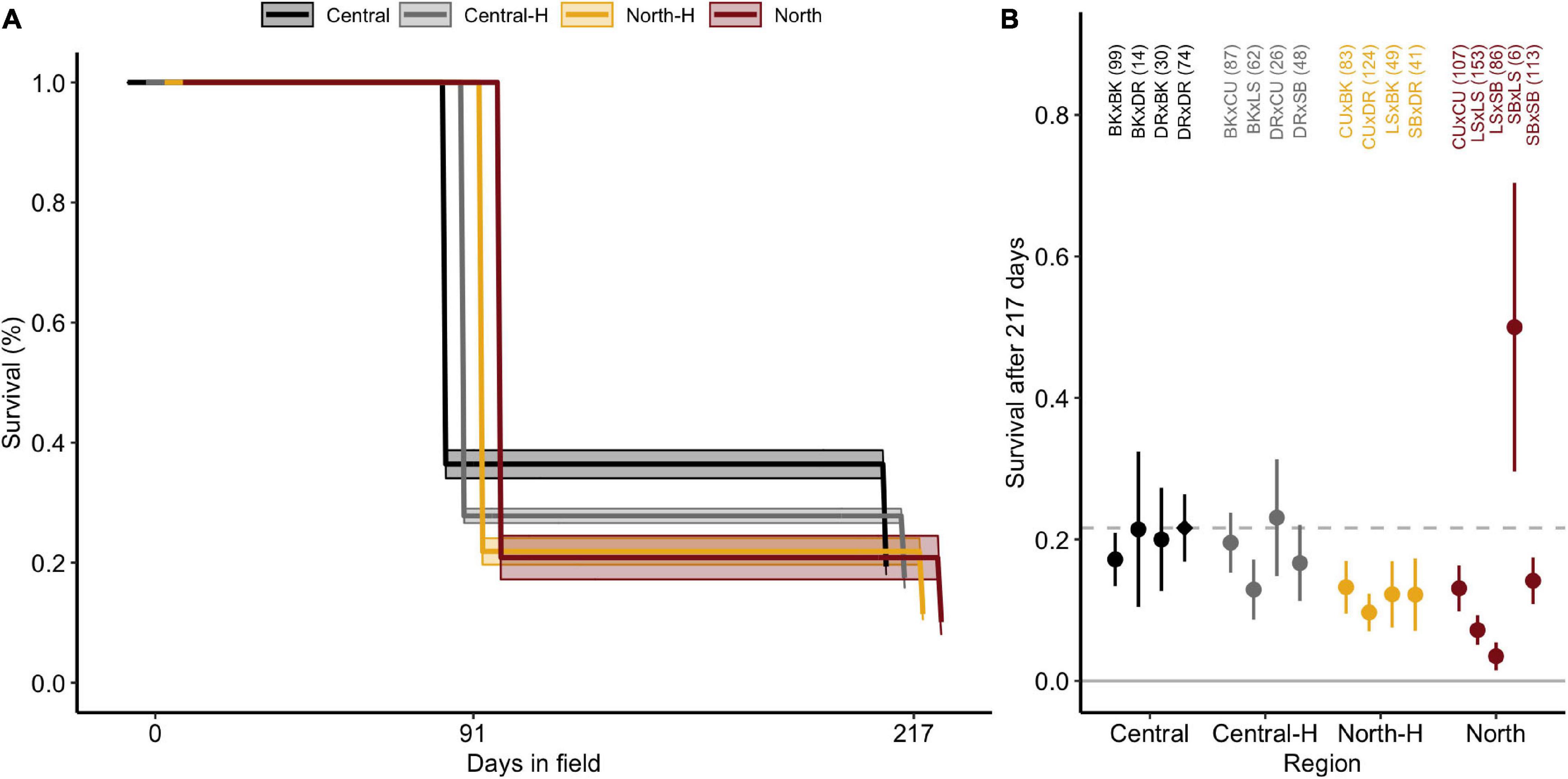
Figure 2. Survival of Acropora tenuis juveniles over 217 days at Davies reef. (A) Kaplan-Meier survival curves for juveniles from each type of cross. Central and North represent the regional purebred crosses while Central-H and North-H represent the regional hybrid crosses (according to dam). Lines and shaded regions indicate estimated survival and ± confidence intervals (CI), respectively, and are staggered to aid interpretation. (B) Mean survival of A. tenuis crosses grouped and coloured by cross. Error bars indicate ± standard errors (SE). The dashed line and diamond symbol delineate the mean survival for the local cross (DRxDR) to aid in comparison. Crosses are abbreviated as in Figure 1, and day 0 sample sizes are listed in parentheses.
In addition to variation amongst the types of crosses, individual crosses exhibited significant differences in survivorship [χ2(16) = 48.1, p < 0.001], predominately driven by the high variation in northern purebreds crosses (Figure 2B and Supplementary Table 2). The northern purebred cross, SBxLS, had the highest survival. However, due to the low initial number of SBxLS juveniles at time of outplant (Table 1), standard error was high, contributing to the lack of significant differences between this cross and the central purebred cross DRxDR (p = 0.158). SBxLS did have significantly higher survival compared to other northern hybrids, northern purebreds, and three of the four central hybrid crosses (all p < 0.046). In contrast, LSxSB had significantly lower survival compared to all other crosses (all p < 0.041). The local cross, DRxDR, had significantly higher survival compared to the two northern purebred and one northern hybrid crosses (CUxDR, LSxLS, LSxSB; all p < 0.008). These three northern crosses had the lowest survival and both LSxLS and CUxDR had significantly lower survival compared to three central purebred crosses, including DRxDR (all p < 0.045). In summary, survival was highly variable by cross and the direction of each cross, but overall, the local cross did perform better than many of the hybrid crosses, with the exception of SBxLS and CUxDR.
Growth and Time to Reproduction
Juvenile A. tenuis doubled in size after 217 days in the field, growing from 1.3 ± 0.1 SE mm2 (n = 65) to 2.6 ± 0.1 mm2 (n = 51, Figure 3A). SBxLS (3.8 ± 0.1 mm2) and DRxSB (3.6 ± 0.4 mm2) were the largest juveniles after 217 days in the field. Growth rates were measured per juvenile (n = 85), which prevented estimation of the growth rate of SBxDR juveniles, where no SBxDR juveniles were measured more than once. The average growth rate of all crosses was 0.007 ± 0.010 mm2 day–1 (0.255 cm2/year). Growth rates were similar amongst the four types of crosses [Figure 3B; F(3, 72.1) = 1.7, p = 0.17] and amongst individual crosses [F(15, 54.5) = 0.9, p = 0.558].
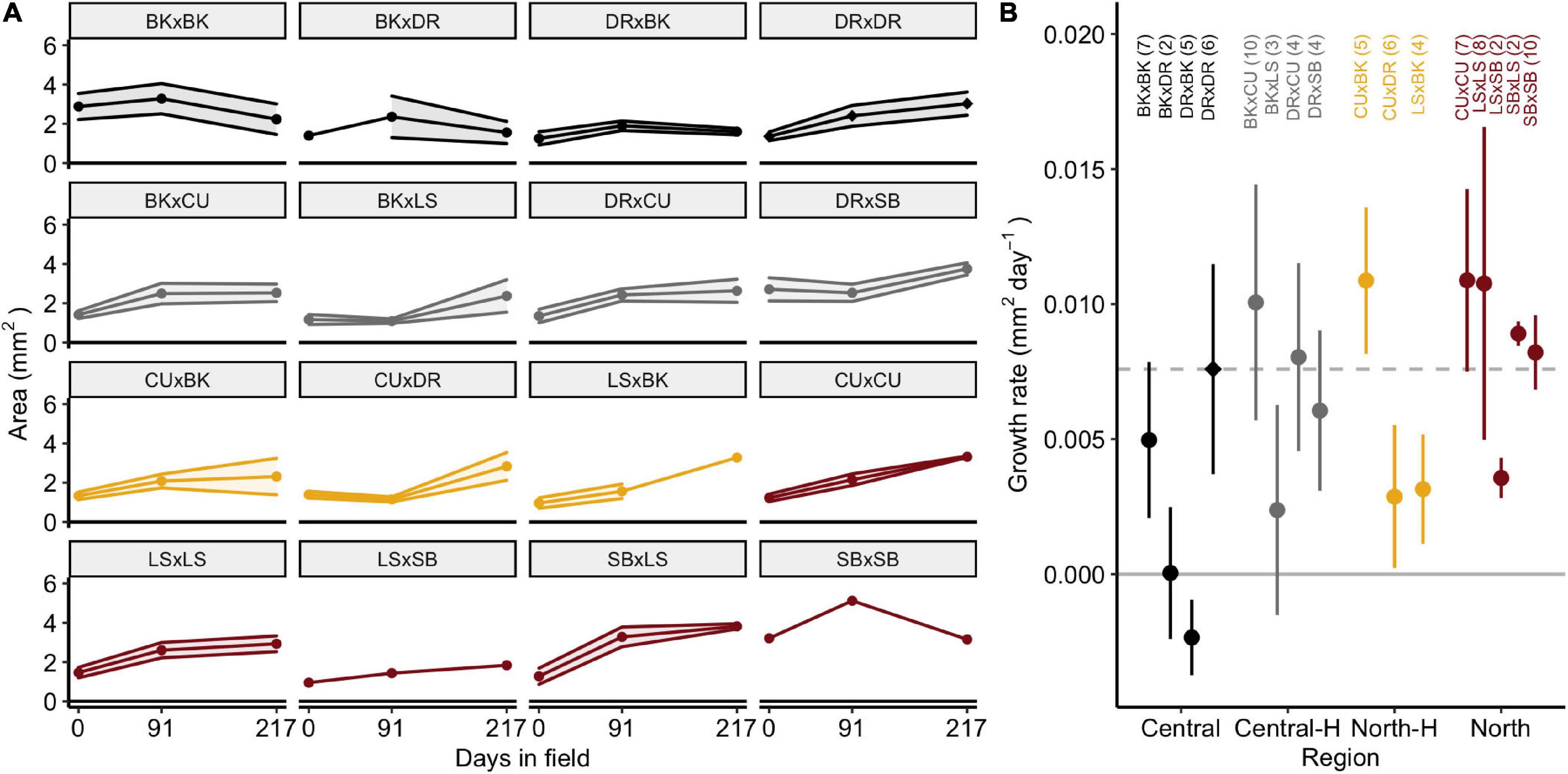
Figure 3. Size and growth rate of Acropora tenuis juveniles. (A) Area (mm2) of juveniles over 217 days in the field. Panels depict crosses in alphabetical order and are coloured according to the type of cross. Points represent means and shaded regions represent ± SE. (B) Growth rates of juveniles (mm2 day–1). Central and North represent the regional purebred crosses while Central-H and North-H represent the regional hybrid crosses (according to dam). Points represent means and error bars represent ± SE. The dashed line and diamond symbol indicate the local cross (DRxDR). Crosses are abbreviated and shaded according to cross as described in Figure 1. Sample sizes are listed in parentheses.
For comparison, exponential growth rates of A. tenuis in this study were compared to previous growth rates measured for A. tenuis juveniles (Figure 4A). Growth rates of A. tenuis at Davies reef in this study (−0.1 to 0.6% area per day) were much slower than previous values for this species (1.8% area per day; Figure 4B). Exponential growth rates in this study ranged from −0.07 (BKxBK) to 0.57% area per day (LSxBK) and followed an approximately normal distribution (Figure 4B). By extrapolating these growth rates, we calculated the time until the juveniles would reach reproductive size. Central purebred crosses would take approximately 12.5 years (median) to reach reproductive size, northern purebreds would take only 7.9 years, and the hybrids (northern and central) would take 9.3 years to reach reproductive size (Figure 4C).
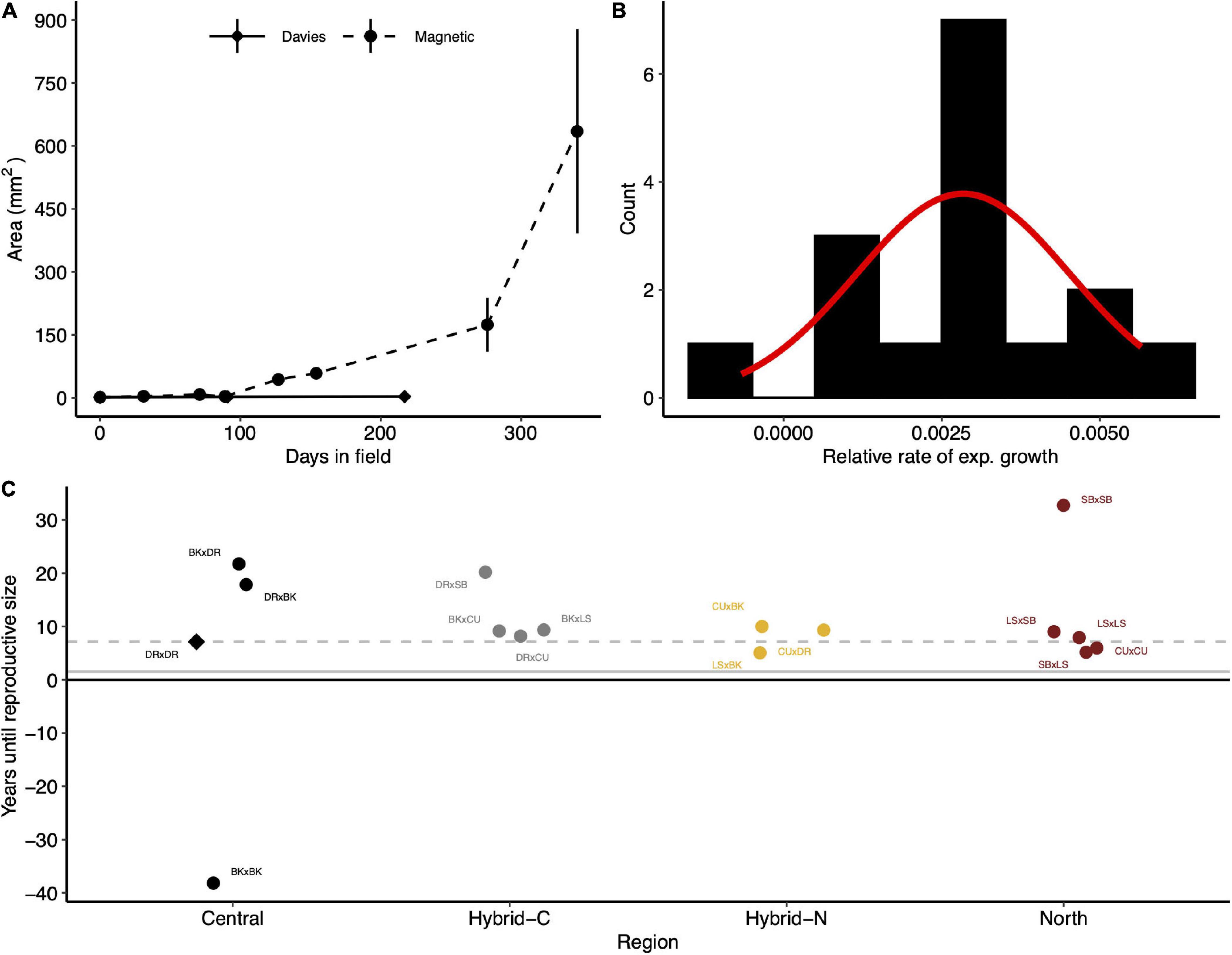
Figure 4. Exponential growth and estimated time until reproductive size for Acropora tenuis juveniles. (A) The area (mm2) of juveniles over time deployed at Davies reef (circles; this study) and Magnetic Island (triangles; Quigley et al., 2020b). Points and error bars represent means and ± SE, respectively. (B) The distribution of the relative growth rate estimates for crosses transplanted to Davies reef. The red line indicates expected counts based on a normal distribution with a mean and SD equal to the relative growth rate estimates. (C) The predicted time (years) until transplanted juveniles reach reproductive size (20 cm diameter). Points represent means of individual crosses grouped and coloured by the type of cross. Central and North represent the regional purebred crosses while Central-H and North-H represent the regional hybrid crosses (according to dam). The grey dashed line indicates the number of years until the local cross (DRxDR, diamond) reaches expected reproductive size. Crosses are abbreviated as in Figure 1.
Colour
The distribution of juvenile colour scores (D1–D6; n = 506) measured with the Coral Colour Reference Card changed significantly over time [Figure 5A; χ2(10) = 61.8, p < 0.001 but was overall similar amongst some types of crosses [χ2(15) = 15.2, p = 0.436] and amongst cross types over time [χ2(30) = 30.8, p = 0.428]. Although the distribution of colour scores changed over time (with some colour scores decreasing in time), the median score was relatively stable between 5–6 (Figure 5A). At deployment, most juveniles were in the highest pigmentation categories of 5 and 6. By days 91 and 217 of field deployment, colour scores had decreased, indicating a paling of juveniles. Specifically, the largest change in colouration occurred between outplant and day 91, with little change in colour occurring between days 91 and 217 (Figure 5A). For individual colour score categories, the proportion of 5 categories decreased significantly by day 91 (33%; p < 0.001) while the proportions of 1 and 2 scores increased significantly by day 91 (1: 7%, p < 0.026; 2: 23%, p < 0.001). Across each of the colour categories, none of the crosses changed significantly between days 91 and 217 (all comparisons < 7.6%; all p < 0.163). On average, colour scores were 5.47 ± 0.04 at deployment, which decreased to 4.45 ± 0.23 by 91 days, and then rose to 4.69 ± 0.17 by 217 days.
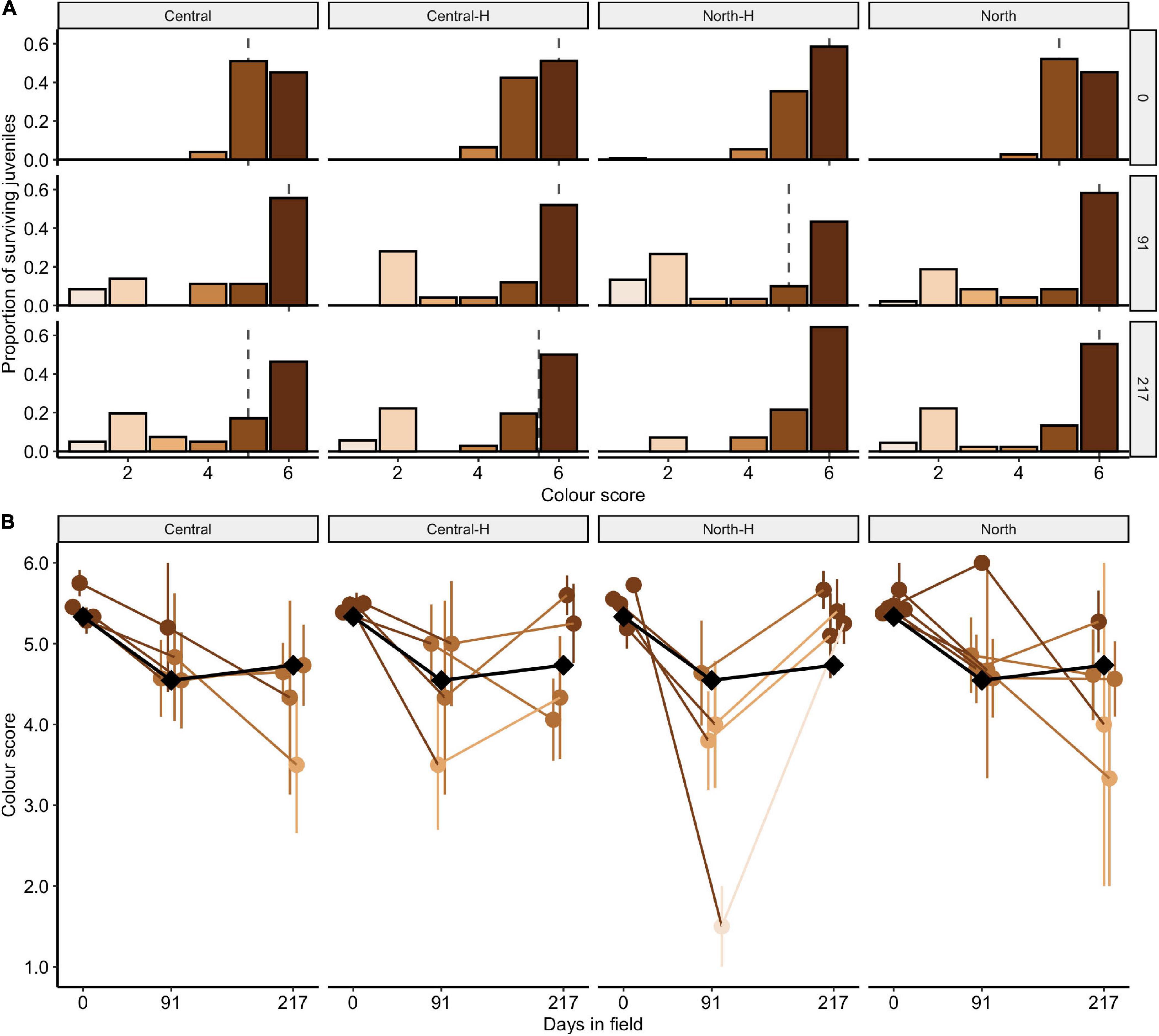
Figure 5. Colour scores for transplanted Acropora tenuis juveniles. (A) The distribution of colour scores (1–6) for each cross after 0, 91, and 217 days in the field. The panels distinguish between cross types and days deployed. Central and North indicate regional purebreds while Central-H and North-H indicate regional hybrids (according to dam). The height of the bars represents the proportion of juveniles in each colour category and the dashed line indicates the median colour score. Bars are coloured to match the CoralWatch “D” colour score. (B) Mean colour scores of crosses over time. Cross are labelled as in (A). Points and error bars represent the mean and ± SE, respectively. The local cross, DRxDR, is indicated by black diamonds and lines. The colour of points and lines for other crosses match the CoralWatch “D” colour score.
Colour scores amongst individual crosses changed over time [χ2(5) = 135.5, p < 0.001], were similar amongst individual crosses [χ2(80) = 81.0, p = 0.447], and amongst individual crosses over time [χ2(80) = 32.0, p = 1.000]. Juveniles from the local cross DRxDR, also decreased in colour over time (black diamonds, Figure 5B). After 217 days in the field, 7 of the 17 crosses maintained colour scores > 5, four of which were northern hybrids (CUxBK, CUxDR, LSxBK, SBxDR), two central hybrids (DRxCU, DRxSB) and one northern purebred cross (LSxLS).
Discussion
The use of intervention methods in coral reef conservation is accelerating (National Academies of Sciences Engineering and Medicine, 2019). To assess the potential risks and benefits associated with these methods, field testing is essential (van Oppen et al., 2014; Quigley et al., 2016). This study provides key baseline field data for assessing the feasibility of hybridisation and answers outstanding questions relating to trade-offs between growth and survival and reproductive potentials of corals produced from AGF methods.
Trade-Offs
Trade-offs are often defined as the increase in the mean value of one trait at the expense of decreases in another, but trade-offs may instead be associated with locally adapted traits and populations (Hereford, 2009), in which moving organisms to new habitats disrupt traits when placed in new environments. Relative to the baseline cross from the deployment reef (DRxDR), there was little evidence of classic patterns in trade-offs in growth and survival from hybrid crosses (Central-H or North-H), with both of these groups (including the individual crosses within them), exhibiting characteristics of both low survival/high growth or low growth/low survival (Figure 6). Specifically, three of the eight hybrid crosses (BKxCU ∼/ = DRxCU < SBxLS) did not exhibit any trade-off between growth and survival, exhibiting both high growth and survival relative to local DRxDR juveniles, thereby suggesting potentially higher overall fitness for these crosses. A minority (one of eight) of the other hybrid crosses exhibited lower survival but higher growth (CUxBK), with the remaining hybrids (five of eight of the crosses) exhibiting lower growth and survival relative to central purebreds (DRxSB, LSxBk, CUxDR, BKxLS, and LSxSB). Interestingly, neither BKxDR or DRxBK juveniles did well at Davies reef, exhibiting both low growth and low survival.
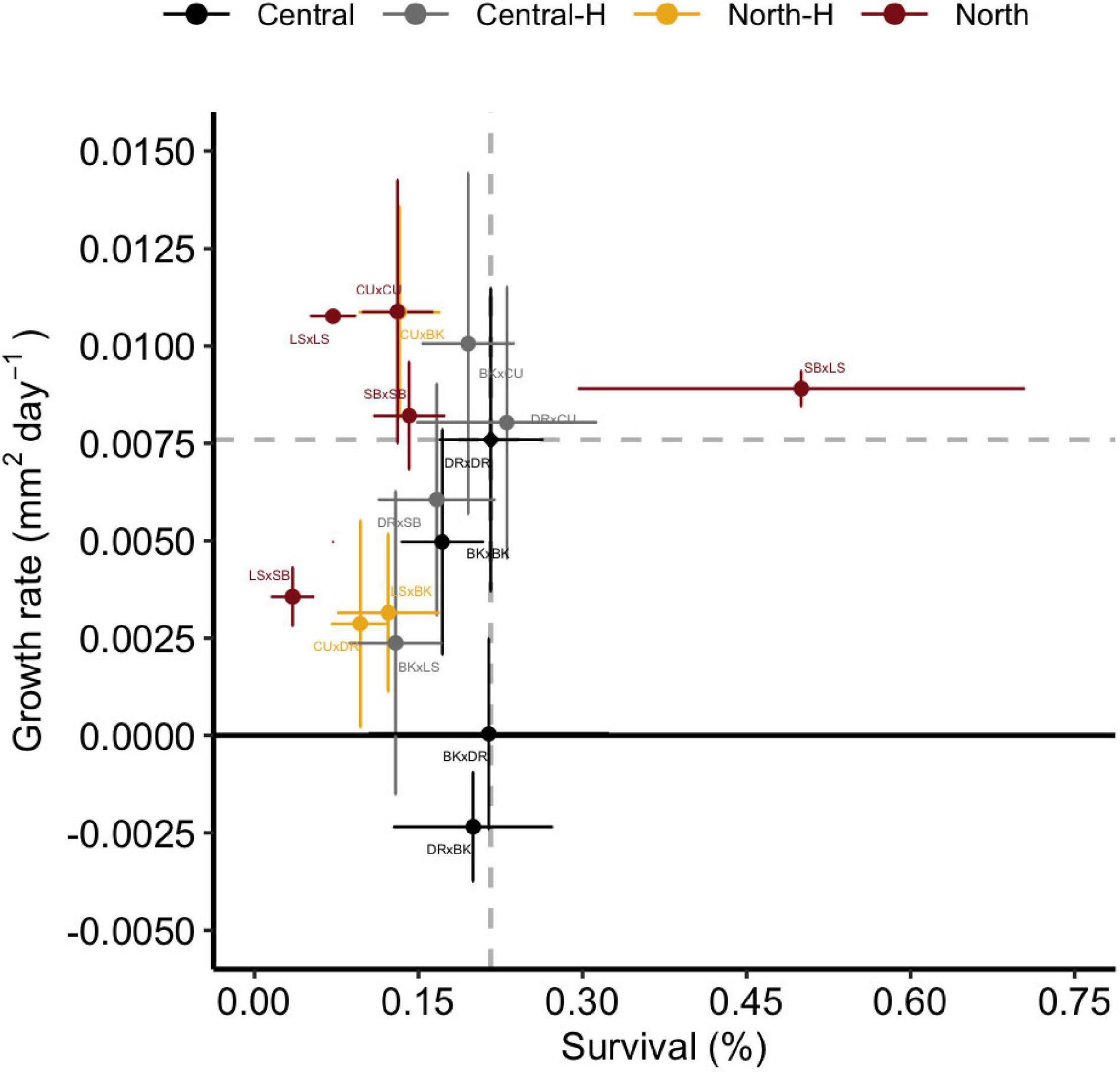
Figure 6. Growth rate vs. survivorship of Acropora tenuis crosses. Points represent the mean growth rate and survival of juveniles in each cross. Points represent means and error bars represent ± SE and are coloured according to the type of cross. Central and North indicate regional purebreds while Central-H and North-H indicate regional hybrids (according to dam). Dashed grey lines and a black diamond indicate the mean growth rate and survival of the local cross, DRxDR. Names of crosses are abbreviated according to Figure 1.
Trade-offs have been examined in corals for traits like survival, growth, and reproduction (Jones and Berkelmans, 2011; Cunning et al., 2015a; Quigley et al., 2020a). Three of the hybrid crosses repeated above did not exhibit any trade-off between these traits. Furthermore, both central hybrids and northern hybrids exhibited characteristics of both low survival/high growth or low growth/low survival, suggesting there was no consistent trade-off between crosses by GBR region but may instead be reef specific. These results mirror multi-factorial tank experiments, where specific genotypes were the main factor influencing phenotypic variation (Wright et al., 2019). Indeed, the assumption formed across terrestrial and aquatic ecosystems is that trade-offs are a persistent feature in ecosystem ecology (Sgrò et al., 2011), although the pervasiveness of trade-offs are likely overstated and, if present, they are likely weak (Hereford, 2009).
The mean survival rate of juveniles after 217 days in the field was high relative to estimates from other reef locations. Post-settlement survival in the field is often low for highly fecund species, including corals (reviewed in Randall et al., 2020) and generally estimated to be between 0.6 and 2% for juveniles within the first 12 months of life (Doropoulos et al., 2019). It is important to note that survival from the literature are generally for purebred corals outplanted to their native reefs. However, survival between population crosses was variable, and when we examined purebred survival rates for juveniles outplanted to their natal reef (DRxDR juveniles), rates also were high (∼20%) and provide an important ground-truthing for baseline survival rates. Decreased survival rates from the literature relative to ours may be attributed to higher densities of juveniles on substrates, in which overcrowding may lead to high mortality (Cameron and Harrison, 2020). A vast majority of juveniles in this study were found on single tiles with adequate space for growth and low competition and all were singles (no clumps). Finally, age-at-outplant is also important for influencing survival, with the lowest survival generally occurring within the first few months of life (reviewed in Doropoulos et al., 2019). Our high survival rates may therefore have been facilitated by the more advanced age of juveniles, and therefore increased size, at the time of outplanting to the field (∼4 months).
One of the key concerns with the adoption of hybridisation is the negative impact on growth rates of individuals produced and transplanted to non-native sites due to the influence of local adaptation. Growth has been identified as one of the potential key performance indicators for restoration success, with normalised summer growth rates the best predictor of rapid growth later (Edmunds and Putnam, 2020). Sizes and growth rates are important as both are linked to reproductive timing. For example, if growth decreases by 2% in non-native crosses, reaching reproductive size may be delayed, leading to a decreased efficacy for the spread of propagules from this intervention. This lag in growth rate is particularly relevant when non-natives are transplanted to colder environments (northern to central GBR), in which rates are expected to decrease (van Oppen et al., 2014) compared to the increased rates when outplanted to warmer climes (Browne et al., 2019). Growth rates in purebred northern crosses were greatest overall, followed by the hybrid crosses and then purebred central crosses, suggesting no decreases in growth rate. These higher growth rates for northern purebred corals in cooler climates instead suggest that risks associated with lineage swamping are more likely (Aitken and Whitlock, 2013). Although we only sourced reefs from two regions here (northern and central GBR), these results suggest that the use of lab-produced hybrids may ameliorate some of this risk given their mixed genomic backgrounds.
Comparisons to known growth rates in the field suggest juvenile crosses at Davies grew slowly. Extrapolating our 0.007 ± 0.010 SE mm2 day–1 estimates results in growth of 0.255 cm2/year, which is well under growth rates for outplanted juveniles that have been estimated at 1 cm/year (Doropoulos et al., 2015) to 4–5.2 cm per year (Iwao et al., 2010; dela Cruz and Harrison, 2017). Slow growth at Davies is further corroborated from A. tenuis juveniles collected from Magnetic Island (Quigley et al., 2020b). When converted to reproductive size (diameter of ∼20 cm), the Davies juveniles would take 9.1 years to reach that diameter. Faster inshore growth has been reported for adult-derived fragments (Rocker et al., 2019), and it is likely tied to increased nutrients loads on inshore reefs. Hence, the slower growth found here is likely influenced by settlement substrate, light environment, and nutrient conditions of the native reef.
At time of outplant in March, most juveniles were pigmented owing to the symbionts provided from Davies reef conspecific adults. Whilst bleaching can be defined as paling of coral tissues either through the decreased density of Symbiodiniaceae or photobleaching of symbiont pigments (Hoegh-Guldberg, 1999), the paling observed here may be attributed to a recalibration of standing-stock densities. The recalibration could be driven by the change in light conditions from within the rearing facility at the Seasim (∼50–100 photosynthetically active radiation–par, μmol photons m–2 s–1) compared to much higher values recorded in the field, which can range from ∼130 to 550 par (Abrego et al., 2009; Jones et al., 2016; Ricardo et al., 2017). Ocean temperatures during this period of assessment were not warmer than average, and the assessments were finalised before the 2020 bleaching event, therefore paling was unlikely due to higher temperatures. Symbiont communities are highly dynamic in coral early life stages (both in regards to infection densities and taxonomic composition, Quigley et al., 2017a), and thus the decrease in densities may not indicate a physiological breakdown between coral and symbiont, but instead a calibration to steady-state dynamics for the reef light environment or seasonal variation in light (Fitt et al., 2000). Interestingly, this same pattern in the reduction of symbiont densities was observed in Magnetic Island juveniles of the species Acropora tenuis after 167 days in the field (Quigley et al., 2020a) and may be a persistent characteristic of coral ontogeny linked with the processes driving the winnowing of symbiont communities.
The risk of incompatibility between host genotype and the symbiont community available for inoculation at Davies reef was also a concern given that the availability of free-living symbiont communities varies across the GBR (Quigley et al., 2017a) and the host genetic identity plays a role in determining the endosymbiont community (Quigley et al., 2017b). However, we did not observe a significant decrease in colour (as a proxy for symbiont densities, see Siebeck et al., 2006) in crosses produced with one or both parents from northern colonies (as reflected in lack of statistical significance between regions over time or between regions overall), which suggests that the paling observed here was not due to an incompatibility between host genetics from northern reefs and symbionts available from central reefs.
Reproduction and Recovery
There is concern that the application of genetic conservation practices may result in offspring that will grow slower (diminishing intervention efficacy) or faster (swamping locals) compared to native offspring (Aitken and Whitlock, 2013). Although a trait like acceleration of growth rates may be desired for restoration goals, information on the limits of growth is essential. To predict how long it would take for offspring produced via AGF to reach reproductive size, estimates of size to reproduction were calculated. Time to reproduction was shortest for northern purebres and longest for central purebreds. Given that the production of coral propagules is costly in both time and resources at land-based facilities, enhancing natural reproductive cycles in the wild is recommended. Hence, the enhancement of the spread of alleles associated with heat tolerance will benefit from AGF stock becoming incorporated into the natural reproductive cycles of reefs, diminishing the reliance on the artificial production of coral offspring and re-seeding (Quigley et al., 2019). Although our results only represent reefs from two regions on the GBR, given this, northern purebred crosses would kickstart self-seeding intervention efforts faster compared to other crosses, reducing the need for future ex situ reproduction and field deployment. The potential for increased recovery due to growth and reproduction of northern purebreds in central reefs may be balanced by limiting the total number outplanted to balance for “migrational meltdown” due to maladaptive alleles (Matz et al., 2018; Baums et al., 2019). Enhancing the spread of alleles may also rely on the fertility of AGF produced corals as it is a key indicator of recovery potential (Álvarez-Noriega et al., 2016), and should be a future area of research.
Recovery of reefs in terms of coral cover would therefore be expected to be faster with the use of northern purebred corals as they would be able to produce sexually-derived propagules the sooner. Recovery potentials via reproductive rates are intrinsically tied to growth rates in corals, with colony size as a well-known predictor of fecundity. Interestingly, initial area did not seem to affect the time until reproduction. As colony size increases in corymbose morphologies like A. tenuis, polyp maturity tends to increase exponentially and oocyte number and reproductive investment tend to increase linearly (Álvarez-Noriega et al., 2016). Furthermore, recovery of coral cover is aided by larger corals that occupy more space (i.e., fewer individual colonies for the same recovered size per unit area of reef), where corals of ∼40 cm in diameter have been found to be of particular value for ecosystem recovery (Ortiz et al., 2009). This size therefore provides a good target for the selective breeding of corals with coral cover recovery as an objective.
Interestingly, SBxLS was the population cross that performed the best in terms of highest survival, fastest growth, and the least amount of paling. Although the number of individuals from this cross was low relative to other crosses in this experiment (Table 1), individuals exhibited both high survival and high growth. Indeed, this signature is suggestive of density-independent mortality, in which a few crosses consisting of a smaller number of individuals exhibited high relative survival whereas other crosses composed of a greater number of juveniles experienced lower relative survival. This suggests that genotype specific differences exist between colonies and those are provisioned to offspring. This response has been observed before in outplants of northern crosses at central reefs (Quigley et al., 2016), although a similar result was not observed when juveniles were transplanted to southern reefs (van Oppen et al., 2014). Finally, although the overall sample size was low for the final number of surviving SBxLS juveniles, the pattern in high survivorship was mirrored in experimental systems in which juveniles of this cross exhibited close to 100% survival at 27°C and 75–100% survival at 32°C across multiple symbiont treatments after 58 days (Quigley et al., in review). Hence, this cross appears to be particularly hardy across a range of temperatures and conditions.
Conclusion
Coral reefs are facing accelerated rates of environmental change, especially continued ocean warming and more severe marine heat waves. Here we show that coral reefs and other environments shaped by disturbances and pressures that have led to potentially strong signatures of local adaption may benefit from interventions focussed on incorporating selective breeding to produce offspring of hybrid stock sourced from warmer locations in the northern GBR, which may match projected future conditions. Although we show that trade-offs in key traits during non-warming years were minimal in a few of the hybrid crosses (25%), the next steps include exposing these crosses to warming to assess their performance under stress. The lack of a consistent pattern in trade-offs between growth and survival of juveniles in these field trials also suggests that perhaps practitioners should look beyond the “local is best” paradigm. This field-based experiment provides an experimental demonstration in the wild for this type of method, thereby contributing essential information that is needed to assess some of the key risks associated with genetic interventions and it informs decision making about the utility of hybridisation for reef restoration. Finally, whilst interventions aimed at accelerating the natural rates of adaptation to heat tolerance to preserve some level of coral reef functionality and diversity are valuable, these interventions must be considered in conjunction with strong action on climate change.
Data Availability Statement
The data and scripts will be made available on Github (https://github.com/LaserKate/AGF2018Field).
Author Contributions
KQ, LB, and MvO designed the research. KQ, MM, DA, LB, and GM performed the fieldwork. BR and KQ analysed the data. KQ, BR, MM, LB, and DA wrote the manuscript. All authors contributed to the critical review of this manuscript.
Funding
We would like to acknowledge support from the Great Barrier Reef Foundation to KQ and LB, and the Australian Institute of Marine Science and the Australian Research Council Laureate Fellowship FL180100036 to MvO.
Conflict of Interest
The authors declare that the research was conducted in the absence of any commercial or financial relationships that could be construed as a potential conflict of interest.
Acknowledgments
We would like to thank the Traditional Owner communities who allowed us to collect northern colonies from their Sea-Country, in particular the Lama Lama Traditional Owner group in the Far Northern Great Barrier Reef. We would also like to thank Carlos Alvarez-Roa, Sam Noonan, and Veronique Mocellin and the GBRLegacy team for their help in collecting the Acropora tenuis colonies from the far north, and the coral collection teams from SeaSim at AIMS for collecting coral colonies from the central reefs. Corals were collected under permits G12/35236.1 and G18/41667.1 and juveniles were deployed under G19/41597.1. We would also like to thank Gina Matthews and Charlie Nicholas for their help with coral spawning, juvenile photographs, and measurements.
Supplementary Material
The Supplementary Material for this article can be found online at: https://www.frontiersin.org/articles/10.3389/fmars.2021.636177/full#supplementary-material
References
Abrego, D., van Oppen, M. J. H., and Willis, B. L. (2009). Onset of algal endosymbiont specificity varies among closely related species of Acropora corals during early ontogeny. Mol. Ecol. 18, 3532–3543.
Aitken, S. N., and Whitlock, M. C. (2013). Assisted gene flow to facilitate local adaptation to climate change. Annu. Rev. Ecol. Evol. Syst. 44, 367–388.
Álvarez-Noriega, M., Baird, A. H., Dornelas, M., Madin, J. S., Cumbo, V. R., and Connolly, S. R. (2016). Fecundity and the demographic strategies of coral morphologies. Ecology 97, 3485–3493.
Barrett, R. D. H., and Schluter, D. (2008). Adaptation from standing genetic variation. Trends Ecol. Evol. 23, 38–44.
Bates, D., Maechler, M., Bolker, B., and Walker, S. (2014). lme4: Linear Mixed-Effects Models Using Eigen and S4. R Packag. version 1.
Baums, I. B., Baker, A. C., Davies, S. W., Grottoli, A. G., Kenkel, C. D., Kitchen, S. A., et al. (2019). Considerations for maximizing the adaptive potential of restored coral populations in the western Atlantic. Ecol. Appl. 29:e01978. doi: 10.1002/eap.1978
Benjamini, Y., and Hochberg, Y. (1995). Controlling the false discovery rate: a practical and powerful approach to multiple testing. J. R. Stat. Soc. Ser. B 57, 289–300.
Bongaerts, P., Frade, P. R., Hay, K. B., Englebert, N., Latijnhouwers, K. R. W., Bak, R. P. M., et al. (2015). Deep down on a Caribbean reef: lower mesophotic depths harbor a specialized coral-endosymbiont community. Sci. Rep. 5: 7652.
Browne, L., Wright, J. W., Fitz-Gibbon, S., Gugger, P. F., and Sork, V. L. (2019). Adaptational lag to temperature in valley oak (Quercus lobata) can be mitigated by genome-informed assisted gene flow. Proc. Natl. Acad. Sci. U.S.A. 116, 25179–25185. doi: 10.1073/pnas.1908771116
Cameron, K. A., and Harrison, P. L. (2020). Density of coral larvae can influence settlement, post-settlement colony abundance and coral cover in larval restoration. Sci. Rep. 10:5488. doi: 10.1038/s41598-020-62366-4
Cunning, R., Gillette, P., Capo, T., Galvez, K., and Baker, A. C. (2015a). Growth tradeoffs associated with thermotolerant symbionts in the coral Pocillopora damicornis are lost in warmer oceans. Coral Reefs 34, 155–160.
Cunning, R., Silverstein, R. N., and Baker, A. C. (2015b). Investigating the causes and consequences of symbiont shuffling in a multi-partner reef coral symbiosis under environmental change. Proc. R. Soc. B 282:20141725.
dela Cruz, D. W., and Harrison, P. L. (2017). Enhanced larval supply and recruitment can replenish reef corals on degraded reefs. Sci. Rep. 7:13985. doi: 10.1038/s41598-017-14546-y
Doropoulos, C., Elzinga, J., ter Hofstede, R., van Koningsveld, M., and Babcock, R. C. (2019). Optimizing industrial-scale coral reef restoration: comparing harvesting wild coral spawn slicks and transplanting gravid adult colonies. Restor. Ecol. 27, 758–767.
Doropoulos, C., Ward, S., Roff, G., González-Rivero, M., and Mumby, P. J. (2015). Linking demographic processes of juvenile corals to benthic recovery trajectories in two common reef habitats. PLoS One 10:e0128535. doi: 10.1371/journal.pone.0128535
Edmunds, P. J., and Putnam, H. M. (2020). Science-based approach to using growth rate to assess coral performance and restoration outcomes. Biol. Lett. 16:20200227.
Fitt, W. K., McFarland, F. K., Warner, M. E., and Chilcoat, G. C. (2000). Seasonal patterns of tissue biomass and densities of symbiotic dinoflagellates in reef corals and relation to coral bleaching. Limnol. Oceanogr. 45, 677–685.
Hereford, J. (2009). A quantitative survey of local adaptation and fitness trade-offs. Am. Nat. 173, 579–588.
Hoegh-Guldberg, O. (1999). Climate change, coral bleaching and the future of the world’s coral reefs. Aust. J. Mar. Freshw. Res. 50, 839–866.
Howells, E. J., Beltran, V. H., Larsen, N. W., Bay, L. K., Willis, B. L., and van Oppen, M. J. H. (2012). Coral thermal tolerance shaped by local adaptation of photosymbionts. Nat. Clim. Chang. 2, 116–120. doi: 10.1038/nclimate1330
Howells, E. J., Berkelmans, R., van Oppen, M. J. H., Willis, B. L., and Bay, L. K. (2013). Historical thermal regimes define limits to coral acclimatization. Ecology 94, 1078–1088.
Hughes, T. P., Anderson, K. D., Connolly, S. R., Heron, S. F., Kerry, J. T., Lough, J. M., et al. (2018). Spatial and temporal patterns of mass bleaching of corals in the Anthropocene. Science 359, 80–83.
Iwao, K., Omori, M., Taniguchi, H., and Tamura, M. (2010). Transplanted Acropora tenuis (Dana) spawned first in their life 4 years after culture from eggs. Galaxea. J. Coral Reef Stud. 12:47. doi: 10.3755/galaxea.12.47
Jones, A. M., and Berkelmans, R. (2011). Tradeoffs to thermal acclimation: energetics and reproduction of a reef coral with heat tolerant Symbiodinium type-D. J. Mar. Biol. 2011:185890.
Jones, R., Bessell-Browne, P., Fisher, R., Klonowski, W., and Slivkoff, M. (2016). Assessing the impacts of sediments from dredging on corals. Mar. Pollut. Bull. 102, 9–29.
Kassambara, A., Kosinski, M., Biecek, P., and Fabian, S. (2017). survminer: Drawing Survival Curves using’ggplot2’. R Packag. version 0.3 1.
Kawecki, T. J., and Ebert, D. (2004). Conceptual issues in local adaptation. Ecol. Lett. 7, 1225–1241.
Kenkel, C., Almanza, A. T., and Matz, M. V. (2015). Fine-scale environmental specialization of reef-building corals might be limiting reef recovery in the Florida Keys. Ecology 96, 3197–3212.
Kuznetsova, A., Brockhoff, P. B., and Christensen, R. H. B. (2017). lmerTest package: tests in linear mixed effects models. J. Stat. Softw. 82, 1–26.
Matz, M. V., Treml, E. A., Aglyamova, G. V., and Bay, L. K. (2018). Potential and limits for rapid genetic adaptation to warming in a Great Barrier Reef coral. PLoS Genet. 14:e1007220.
Matz, M. V., Treml, E. A., Aglyamova, G. V., van Oppen, M. J. H., and Bay, L. K. (2017). Adaptive pathways of coral populations on the Great Barrier Reef. bioRxiv [preprint] doi: 10.1101/114173
National Academies of Sciences Engineering, and Medicine, (2019). A Decision Framework for Interventions to Increase the Persistence and Resilience of Coral Reefs. Washington, DC: National Academies Press.
Ortiz, J. C., Gomez-Cabrera, M., del, C., and Hoegh-Guldberg, O. (2009). Effect of colony size and surrounding substrate on corals experiencing a mild bleaching event on Heron Island reef flat (southern Great Barrier Reef, Australia). Coral Reefs 28:999. doi: 10.1007/s00338-009-0546-0
Pinheiro, J., Bates, D., DebRoy, S., and Sarkar, D. (2014). Nlme: Linear and Nonlinear Mixed Effects Models. R package version 3.1-118.
Quigley, K. M., Alvarez Roa, C., Torda, G., Bourne, D. G., and Willis, B. L. (2020b). Co-dynamics of Symbiodiniaceae and bacterial populations during the first year of symbiosis with Acropora tenuis juveniles. Microbiologyopen 9:e959.
Quigley, K. M., Bay, L. K., and van Oppen, M. J. H. (2019). The active spread of adaptive variation for reef resilience. Ecol. Evol. 9, 11122–11135. doi: 10.1002/ece3.5616
Quigley, K. M., Bay, L. K., and Willis, B. L. (2017a). Temperature and water quality-related patterns in sediment-associated Symbiodinium communities impact symbiont uptake and fitness of juveniles in the genus Acropora. Front. Mar. Sci. 4:401. doi: 10.3389/fmars.2017.00401
Quigley, K. M., Randall, C. J., van Oppen, M. J. H., and Bay, L. K. (2020a). Assessing the role of historical temperature regime and algal symbionts on the heat tolerance of coral juveniles. Biol. Open 9:bio047316.
Quigley, K. M., Willis, B. L., and Bay, L. K. (2016). Maternal effects and Symbiodinium community composition drive differential patterns in juvenile survival in the coral Acropora tenuis. R. Soc. Open Sci. 3, 1–17.
Quigley, K. M., Willis, B. L., and Bay, L. K. (2017b). Heritability of the Symbiodinium community in vertically-and horizontally-transmitting broadcast spawning corals. Sci. Rep. 7:8219. doi: 10.1038/s41598-017-08179-4
Randall, C. J., Negri, A. P., Quigley, K. M., Foster, T., Ricardo, G. F., Webster, N. S., et al. (2020). Sexual production of corals for reef restoration in the Anthropocene. Mar. Ecol. Prog. Ser. 635, 203–232. doi: 10.3354/MEPS13206
Ricardo, G. F., Jones, R. J., Nordborg, M., and Negri, A. P. (2017). Settlement patterns of the coral Acropora millepora on sediment-laden surfaces. Sci. Total Environ. 609, 277–288. doi: 10.1016/j.scitotenv.2017.07.153
Rocker, M. M., Kenkel, C. D., Francis, D. S., Willis, B. L., and Bay, L. K. (2019). Plasticity in gene expression and fatty acid profiles of Acropora tenuis reciprocally transplanted between two water quality regimes in the central Great Barrier Reef, Australia. J. Exp. Mar. Bio. Ecol. 511, 40–53. doi: 10.1016/j.jembe.2018.11.004
Rueden, C. T., Schindelin, J., Hiner, M. C., DeZonia, B. E., Walter, A. E., Arena, E. T., et al. (2017). ImageJ2: ImageJ for the next generation of scientific image data. BMC Bioinformatics 18:529. doi: 10.1186/s12859-017-1934-z
Sanford, E., and Kelly, M. W. (2011). Local adaptation in marine invertebrates. Ann. Rev. Mar. Sci. 3, 509–535.
Sgrò, C. M., Lowe, A. J., and Hoffmann, A. A. (2011). Building evolutionary resilience for conserving biodiversity under climate change. Evol. Appl. 4, 326–337.
Siebeck, U. E., Marshall, N. J., Klüter, A., and Hoegh-Guldberg, O. (2006). Monitoring coral bleaching using a colour reference card. Coral Reefs 25, 453–460.
van Oppen, Bongaerts, P., Underwood, J., Peplow, L., and Cooper, T. (2011). The role of deep reefs in shallow reef recovery: an assessment of vertical connectivity in a brooding coral from west and east Australia. Mol. Ecol. 20, 1647–1660. doi: 10.1111/j.1365-294X.2011.05050.x
van Oppen, M. J. H., Bongaerts, P., Frade, P., Peplow, L. M., Boyd, S. E., Nim, H. T., et al. (2018). Adaptation to reef habitats through selection on the coral animal and its associated microbiome. Mol. Ecol. 27, 2956–2971.
van Oppen, M. J. H., Puill-Stephan, E., Lundgren, P., De’ath, G., and Bay, L. K. (2014). First-generation fitness consequences of interpopulational hybridisation in a Great Barrier Reef coral and its implications for assisted migration management. Coral Reefs 33, 607–611.
Whiteley, A. R., Fitzpatrick, S. W., Funk, W. C., and Tallmon, D. A. (2015). Genetic rescue to the rescue. Trends Ecol. Evol. 30, 42–49.
Keywords: coral, bleaching, restoration, selective breeding, hybridisation, survival, reproduction
Citation: Intrapopulation Hybridisation in the Wild (2021) Variability in Fitness Trade-Offs Amongst Coral Juveniles With Mixed Genetic Backgrounds Held in the Wild. Front. Mar. Sci. 8:636177. doi: 10.3389/fmars.2021.636177
Received: 01 December 2020; Accepted: 08 February 2021;
Published: 26 February 2021.
Edited by:
Baruch Rinkevich, Israel Oceanographic and Limnological Research, IsraelReviewed by:
Charles Alan Jacoby, St. Johns River Water Management District, United StatesEslam O. Osman, Pennsylvania State University (PSU), United States
Copyright © 2021 Quigley, Marzonie, Ramsby, Abrego, Milton, van Oppen and Bay. This is an open-access article distributed under the terms of the Creative Commons Attribution License (CC BY). The use, distribution or reproduction in other forums is permitted, provided the original author(s) and the copyright owner(s) are credited and that the original publication in this journal is cited, in accordance with accepted academic practice. No use, distribution or reproduction is permitted which does not comply with these terms.
*Correspondence: Kate M. Quigley, a2F0ZW1hcmllLnF1aWdsZXlAbXkuamN1LmVkdS5hdQ==