Inclusion of Intra- and Interspecific Facilitation Expands the Theoretical Framework for Seagrass Restoration
- 1Institute of Marine Sciences, University of North Carolina at Chapel Hill, Morehead City, NC, United States
- 2Division of Marine Science and Conservation, Nicholas School of the Environment, Duke University, Beaufort, NC, United States
- 3Department of Biology and Coastal Studies Institute, East Carolina University, Greenville, NC, United States
- 4Department of Biology, East Carolina University, Greenville, NC, United States
- 5Department of Coastal Systems, Royal Netherlands Institute of Sea Research and Utrecht University, Den Burg, Netherlands
- 6Conservation Ecology Group, Groningen Institute for Evolutionary Life Sciences, University of Groningen, Groningen, Netherlands
Restoration is increasingly utilized as a strategy to stymie the loss of coastal habitats. Coastal habitat restoration has predominantly emphasized designs that minimize physical stress and competition. As evidence of the pervasiveness of this approach, we conducted a global survey of seagrass restorationers and found a strong affinity for stress-avoidant designs with adult shoots in dispersed rather than aggregated configurations. To test the alternative hypothesis that including positive interactions can enhance restoration success, we experimentally incorporated: (i) interspecific facilitation (clam additions) into seed sowing, and (ii) both intra- and interspecific facilitation (planting a single-large versus multiple-small patches and adding clams) into shoot planting. Clam additions to seeds significantly enhanced plant biomass and patch size; and nutrient analysis suggested the causative mechanism was clam enhancement of available nitrogen. In contrast, adult outplant growth was enhanced by intra- but not inter-specific facilitation. Dispersed configurations consistently declined, whereas large-intact patches, which had the same initial biomass as dispersed plots, increased in patch area and doubled in shoot density. These results demonstrate that expanding restoration strategies to include positive interactions with respect to seagrass ontogeny has the capability to switch the trajectory of restoration from failure to success.
Introduction
Climate change, pollution, habitat destruction, overharvest of predators, among others, have contributed to the global loss or conversion of roughly 29% of seagrasses (Waycott et al., 2009), 85% of oyster reefs (Beck et al., 2011), and 42% of North American salt marshes (Gedan and Silliman, 2009a). Countries subsequently invest millions of dollars annually toward coastal conservation efforts in order to stem the loss ecosystem services necessary for human well-being (Edwards et al., 2013; BenDor et al., 2015; Saunders et al., 2020). Restoration has recently been promoted as a primary strategy by nations, corporations, and non-profit organizations to bolster shoreline ecosystems and communities, combat habitat losses, compensate for urban development, and create jobs (CWA, 1972; ERA, 2000; RESTORE Act, 2012; Edwards et al., 2013; Sutton-Grier et al., 2015, 2018). To meet this increasing demand, marine restoration approaches must quickly become more affordable and effective, as the failure rate and costs of marine ecosystem restoration are often high (Bayraktarov et al., 2016; Saunders et al., 2020). Implementing ecological theories, such as the increasingly important role of positive species interactions in high stress environments (He et al., 2013; He and Bertness, 2014), in restoration methodologies will be critical for meeting this challenge.
The current theoretical framework in coastal restoration and planting designs is derived from forestry science (Gedan and Silliman, 2009b; Silliman et al., 2015; Shaver and Silliman, 2017), which places an emphasis on maximizing outplant yields by reducing physical stressors and planting propagules in designs aimed at minimizing competitive interactions (Halpern et al., 2007; Gedan and Silliman, 2009b). In contrast, many ecological studies in marine ecosystems have found that coastal plants experience increased growth when grown in clumps adjacent to neighbors of the same species, especially when recovering from disturbance (Van Keulen et al., 2003; Bos and Van Katwijk, 2007; Angelini et al., 2011; Silliman et al., 2015; Gittman et al., 2018). Key to determining if inclusion of positive species interactions can maximize restoration productivity and resilience across diverse ecosystems are tests that examine the utility of incorporating multiple types of facilitation in different marine habitats and at multiple stages of outplant maturity (e.g., seeds vs. adults) during restoration.
In response to accelerated declines in seagrasses across the globe, efforts to restore seagrass beds have increased dramatically over the last two decades (Zhang et al., 2018). While successful restoration efforts have occurred, outcomes are highly variable with an almost two-thirds failure rate (Reise and Kohlus, 2008; Orth et al., 2012; van Katwijk et al., 2016; Lefcheck et al., 2018). Hence, improving the success of small-scale designs is necessary to increase the feasibility of seagrass restoration. One potential way to harness facilitation in seagrass restoration is to plant aggregated or large-intact patches rather than dispersed designs, as large patches self-facilitate to resist and reduce hydrodynamic stress (Van Keulen et al., 2003; Bos and Van Katwijk, 2007). Moreover, clonal organisms such as seagrasses that can internally translocate nutrients may more readily resist abiotic stressors such low oxygen in sediments (de Kroon, 1993). Another promising approach to harness facilitation by design is to incorporate secondary foundation species into seagrass plantings. For example, bivalves in seagrass beds have the capability to enhance benthic-pelagic coupling and benefit seagrass growth (Wall et al., 2008), and some entangled seaweeds can enhance local biodiversity, particularly in low nutrient scenarios (Thomsen et al., 2018).
The high degree of variability in seagrass restoration outcomes necessitates an expansion of the current theoretical framework to include methods, such as harnessing positive interactions, that have been experimentally demonstrated to enhance restoration success. We utilized a multi-disciplinary approach to determine: (i) the extent to which facilitation has been utilized in seagrass restoration schemes and (ii) when and how inter- and intraspecific facilitation affects seagrass growth. Specifically, we conducted a global survey of practitioners to gain a broad understanding of the current and previous methodologies employed for planting and restoring seagrasses. We further conducted two separate field experiments to test our hypothesis that interspecific facilitation by bivalves could enhance the growth of Zostera marina from seed, and that both interspecific facilitation by bivalves and intraspecific facilitation among outplants could increase the growth, expansion, and persistence of mixed eelgrass and shoalgrass (Zostera marina and Halodule wrightii) patches in a restoration setting.
Materials and Methods
To examine the effects of intra- and inter-specific facilitation on seagrass restoration, we took a multi-disciplinary approach. First, we conducted a global survey of restoration practitioners across a wide array of affiliations to determine the extent that positive species interactions were utilized in seagrass restoration projects. Second, we conducted two separate field experiments in the southern Outer Banks of North Carolina, United States to determine the direct effects of (1) infaunal clams on the success of outplanted seeds, and (2) the relative effects of clams and planting configuration on shoot restoration success.
Practitioner Survey
In order to gain a better understanding of previous seagrass restoration efforts and methodologies, we conducted a global survey of practitioners to assess typical project size, location, and the use of various planting techniques. The survey data presented here is a subset of a 20-question survey instrument that was developed and tested by an interdisciplinary team of academic researchers. We used Qualtrics survey software and Google Cloud Computing to solicit and distribute our survey to over 750 restoration individuals and organizations including academics, researchers, consulting companies, government agencies, and non-profit organizations. The list of potential respondents was compiled from known seagrass restoration practitioners and researchers (e.g., members of the Seagrass Restoration Network, scientists who publish on seagrass restoration topics, etc.), state agencies tasked with coastal environmental conservation and management, and organizations or companies frequently contracted for coastal environmental remediation and mitigation. Thus, our solicitation was aimed toward capturing a wide and representative swath of practitioners. Survey participants were recruited via an initial direct solicitation email on 18 February 2020 and a follow-up reminder email was sent 2 weeks after the initial solicitation on 3 March 2020 (Supplementary A). Each respondent was issued an individualized survey link that would allow only one response per link. Survey responses were recorded from 18 February-20 March 2020. Participants were asked various questions regarding location, planting methodologies, monitoring efforts, priorities, and restoration budget (Supplementary A).
Seed Planting Experiment
Reproductive eelgrass shoots were collected by hand in April and May 2017 from a donor seagrass bed near Harker’s Island, NC, United States. Reproductive shoots were stored within an indoor, flow-through seawater system at the Duke Marine Lab in Beaufort, NC, United States with a thin layer of fine sediment. Aquaria were housed in an indoor facility with a 12-h light timer and air bubblers. Tanks were stirred on a daily basis to ensure that shoots did not desiccate and to encourage seeds to drop. After 3 weeks, excess plant material was removed by hand from the tanks, examined for any seeds that had not dropped, and disposed. Seeds were not moved, filtered from sediment, or transferred from initial holding tanks to minimize handling. Seeds were maintained in flow-through tanks until December 2017 (Marion and Orth, 2010). Prior to planting, 50 seeds were tested for viability using tetrazolium staining (Conacher et al., 1994). We found that our seed stock had an average viability of 80%, however, direct tests of germination (e.g., in petri dishes) likely would have revealed lower, more accurate rates of germination.
Seeds were planted in early December 2017 at Oscar Shoal, in Back Sound, NC to coincide with observed natural germination periods in NC when water temperatures consistently reached 15°C or below—the optimal temperature for germination of Zostera marina seeds (Marsh et al., 1986; Moore et al., 1993). We tested four treatments: bare, seed addition only, clam addition only, and clams and seagrass addition (n = 5 per treatment, Figure 1A). We used the quahog, Mercenaria mercenaria, in our experiment, as it is one of the most abundant bivalves in North Carolina and is the subject of extensive aquaculture such that large quantities are readily available at low cost from local hatcheries. Because large adult clams could adversely bioturbate seagrass seeds, juvenile clams (<1 cm width) were purchased from a local aquaculture farm, Morris Family Shellfish Farms, located in Sealevel, NC. Juvenile clams were stored in the same facility as seagrass seeds for 24 h prior to deployment. For clam treatments, 10 seed clams (<1 cm width) were added within the plot. Studies have found that phytoplankton in North Carolina waters is sufficient to maintain adult clams at densities of 60–80 m2 with little effect on growth or survival (Peterson and Beal, 1989; Irlandi and Mehlich, 1996); thus, while our initial densities were high, it is not likely that juvenile clams were competing for resources with each other or with seeds. Previous studies of seed density in our area found an average seed density of 336 seeds per 0.5 × 0.5 m2 plot (∼1,350 seeds m–2) in large, unfragmented seagrass beds (Livernois et al., 2017). Following these estimates, we manually planted 65 seeds within a 20 × 20 cm plot (∼1,300 viable seeds m–2). Both seeds and clams were covered with a thin layer of sediment (<2 cm) after planting. Plots were spaced 1 m apart to ensure that lateral growth could be attributed to growth from the initial quadrat. Prior to planting, cores (n = 5, 12.5 cm diameter) were taken from the planting site to determine natural seed abundances. No seeds were recovered in any of the pre-planting cores.
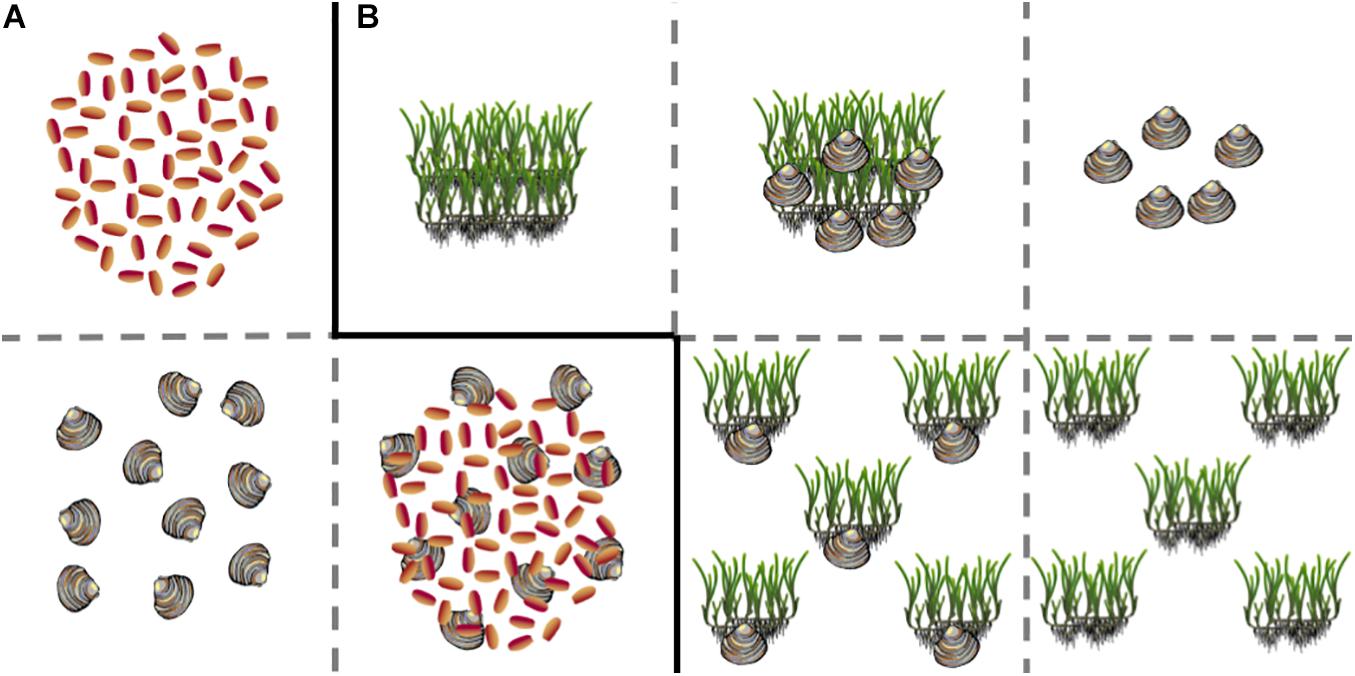
Figure 1. Planting schematic of (A) seed and (B) adult shoot experiments. Solid black lines indicate different experiments. Each experiment also included a control bare plot not depicted here. Graphics courtesy of Abigail Poray, and Catherine Collier, Integration and Application Network, University of Maryland Center for Environmental Science.
Plots were monitored monthly from December through March, and biweekly in April, and May for, patch dimensions, shoot density and grass growth rate. Seagrass growth was measured by marking 10 separate shoots approximately 1 cm below the sheath. After 2 weeks, marked shoots were collected and brought to the lab for processing. At the end of May, plots were entirely excavated and processed in lab to determine above and belowground biomass, shoot density, average shoot length, and reproductive effort. No growth was observed in either control (bare) or clam-only patches; thus, these treatments were dropped from the analysis, and only seed vs. seed-and-clam treatments were compared. Analyses were conducted with Student’s t-tests (α = 0.05) if data met test assumptions.
Because bivalves can increase seagrass growth by increasing nitrogen availability in the sediment through biodeposition, we further measured the carbon content (%C), nitrogen content (%N) and carbon to nitrogen ratio (C:N) in seagrass tissue by clipping, drying, grinding, and acidifying (to remove inorganic nitrogen) samples of above and belowground biomass. CHN analyses were run by the Duke Environmental Stable Isotope Laboratory on a CE FlashEA 1112 (Thermo Fisher Scientific, Waltham, Massachusetts, United States). Total above- and belowground nitrogen was estimated by multiplying the above C:N ratio by plot biomass. Statistical differences in nitrogen content of patches between seed and seed-and-clam treatments were determined using a Student’s t-test (α = 0.05).
Adult Transplant Experiment
To directly test for potential differences between intra- and inter-specific facilitation on the growth of adult shoot transplants of both Zostera marina and Halodule wrightii (eelgrass and shoalgrass, respectively), we conducted a fully factorial experiment crossing planting arrangement with clam additions such that we had six treatments: large-intact patch only, several-dispersed patches only, large-intact patch + clams, several-dispersed patch + clams, clam-only patch, and a bare patch with seven replicates per treatment (Figure 1B). An intact turf of seagrass (25 × 25 cm), including rhizomes and sediment, was manually dug from a nearby seagrass bed and transplanted into an adjacent sandflat (<400 m away) that was determined from historical maps to have supported seagrass beds within the last 15 years at south Core Banks, NC. Transplant patches were sown such that all rhizomes were covered with at least 2–3 cm of sediment. Outplant patches were composed of both eelgrass and shoalgrass at an average of 23 eelgrass shoots and 84 shoalgrass (average total of 107) shoots per patch, with no significant differences between initial shoot densities. Several-dispersed treatments were composed of 5 separated, small patches planted within a 0.5 × 0.5 m monitoring plot for a combined initial seagrass area of 625 cm2 (Figure 1B); whereas, large-intact outplants consisted of a singular, undivided patch measuring 25 × 25 cm (625 cm2). Harvest-sized clams (2.89 cm mean umbo height) were purchased from local fishers and stored in flow-through seawater facilities for 24 h prior to deployment. To each clam addition treatment, five clams were added per plot and allowed to self-bury. Experiments were conducted from June through September 2018 and monitored monthly for seagrass density and patch dimensions as well as clam mortality. We maintained clam density at five per plot when obvious signs of mortality (shell fragments) were observed. To test for a statistical effect of patch configuration (large-intact versus several-dispersed) and clam addition on the above metrics of productivity, we checked to ensure data met test assumptions and used analysis of variance (ANOVA, α = 0.05) and Tukey Honest Significant Differences (Tukey HSD) tests were to determine differences in main effects shown from ANOVA tests. We were unable to obtain samples for Carbon: Nitrogen analyses as experiments were abruptly concluded in September 2018 as a result of Hurricane Florence. Sites were surveyed immediately post-storm and in the 2019 growing season to determine if patches weathered the storm or would return the following year. We did not observe any indications of patch [re]growth post-storm.
Results
Our survey was distributed globally to 750 seagrass restoration practitioners representative of multiple sectors including academic, governmental, non-profit, and private organizations (Supplementary A). From this, 152 respondents completed the survey; 103 indicated that they currently or had previously restored seagrasses, and 80 specifically responded to questions regarding positive interactions in restoration methodologies and approaches (Supplementary B and Supplementary Figure S1). Respondent affiliations were fairly heterogeneous with 24 non-profit, 26 academia, 30 governmental agencies, and 12 private companies (1 respondent indicated multiple) listed. However, respondents were located primarily in the United States, with no respondents from/in either the African continent or the Mediterranean region. Practitioners utilized various methods for restoring grasses including planting shoots, planting seeds, engineering and regrading sites, water quality amendments, among others (68, 32, 27 and, 24 responses, respectively, Figure 2A). More specifically, most respondents planted in dispersed arrangements (86% of respondents, Figure 2B). When planting shoots in particular, seagrasses were more frequently arranged in a dispersed configuration than in an aggregated or single large patch (48% dispersed, 33% aggregated). The reported average distance between shoot outplants was 0.75 ± 0.78 m (N = 56); whereas, the average distance between seed patches was 1.17 ± 1.13 m (N = 19). Respondents who had attempted or conducted co-restoration of seagrasses with other habitats or organisms rarely incorporated within-habitat facilitations such as planting with infaunal bivalves (Supplementary B and Supplementary Table S1), and notably, only one respondent attempted to restore multiple seagrass species in a single project.
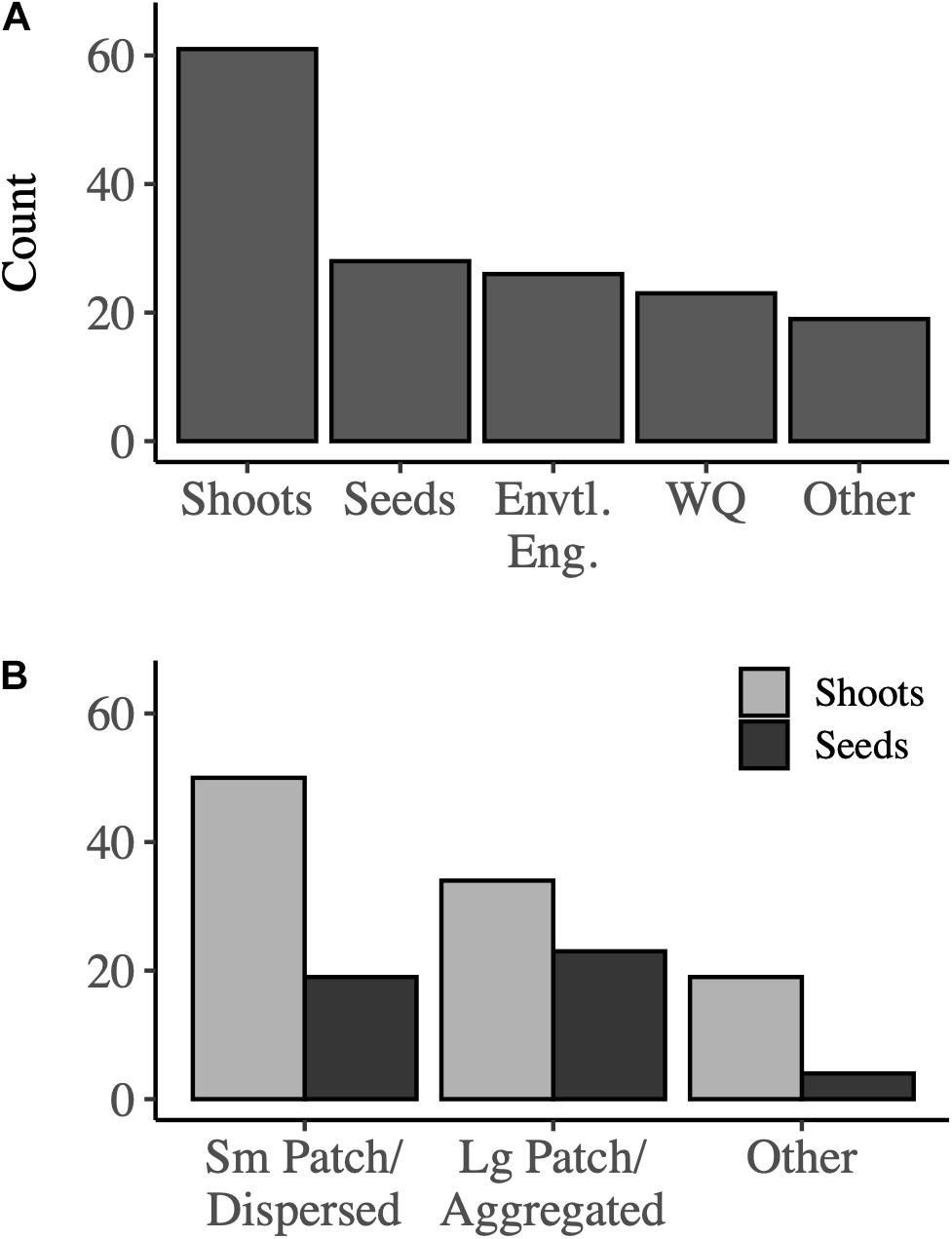
Figure 2. Approaches to (A) seagrass restoration broadly, and (B) specific methodologies regarding planting configuration. Envtl. Eng. = Environmental engineering, and WQ = water quality. Other approaches included sediment filling and remediation, building breakwaters or living shorelines, and alteration of hydrodynamics.
Our from-seed restoration experiment found that clam addition was positively associated with multiple metrics seagrass productivity including significantly greater shoot length (p = 0.013) as well as patch expansion, and belowground biomass (Figures 3A,B and Supplementary Table S2). Seed patches with clams expanded on average 500% from initial area measurements; whereas, patches without clams did not change significantly in size (p = 0.010, −10.63% and 513.62%, Figure 3C). Belowground biomass was also significantly greater in the presence of clams, with almost 10 times more belowground biomass in plots with clams (p = 0.010, 0.37 g and 3.12 g, Figure 3D). However, aboveground biomass at experiment end was not significantly different, despite being, on average, nine times greater (p = 0.083, 0.78 g and 6.80 g). We did not find an effect of clams on the number of seedlings that initially emerged (p = 0.6083), but reproductive effort, measured as both the number of seeds and the total biomass of reproductive grasses was on average 5—6 times greater in patches with clams, but the difference was not significant (p = 0.119, 61.2 and 316.2 seeds/patch, and p = 0.163, 0.35 g and 2.04 g, respectively). Carbon: Nitrogen analysis indicated that seagrass in plots with clams had a significantly lower C:N ratio in both the leaves (p = 0.005) and rhizomes (p = 0.005). Total aboveground nitrogen in seagrasses was over 10 times higher in plots with clams (p < 0.001, 21.06 mg and 131.83 mg, Figure 3E), and total belowground nitrogen was over five times greater in the presence of clams (p < 0.001, 5.12 mg and 27.57 g, Figure 3E). Conversely, we did not find a statistically significant difference in clam survivorship between clam and clams+seagrass treatments (P = 0.095).
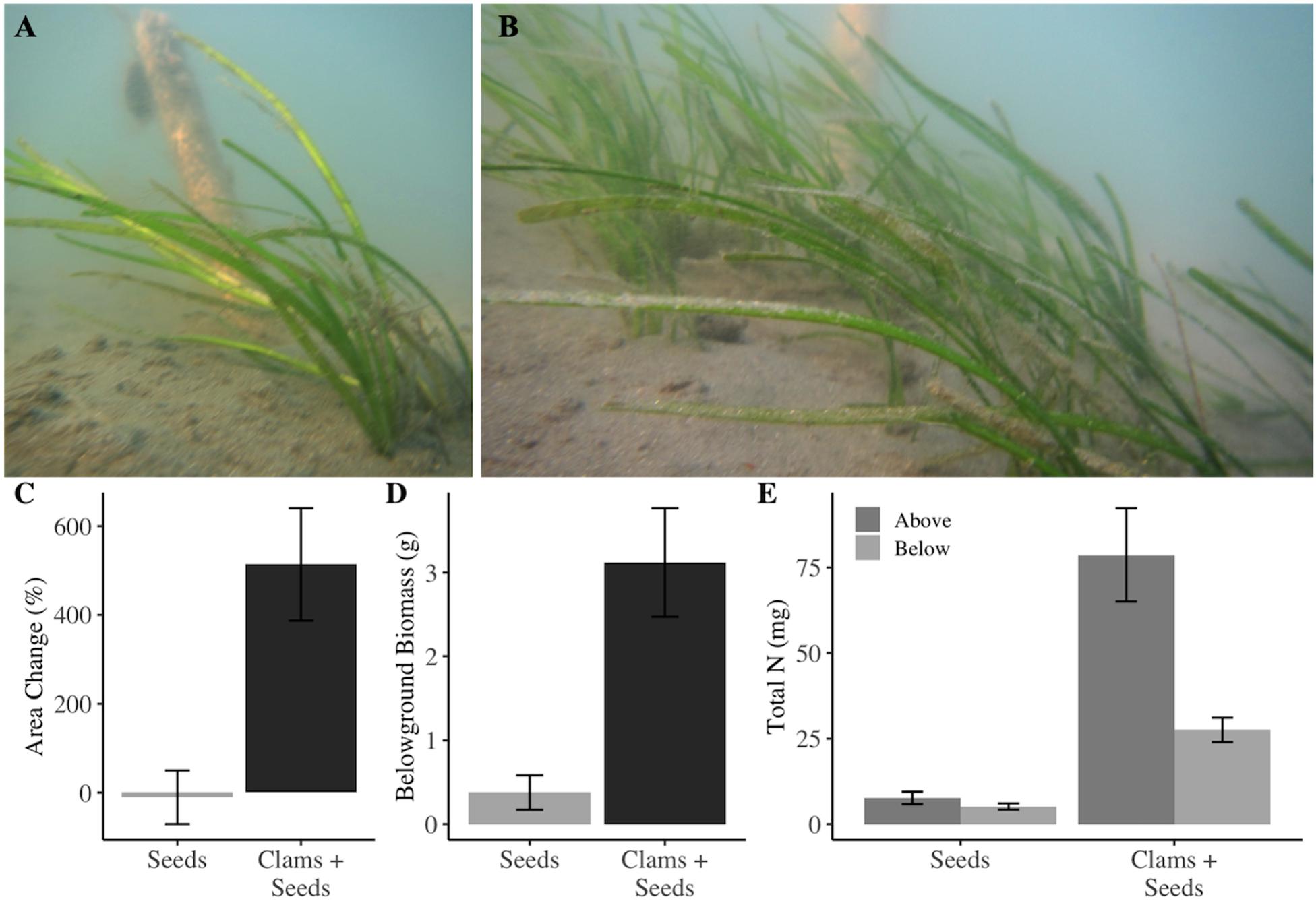
Figure 3. Demonstrative (A) seed only and (B) seeds and clams plots in May 2018 at experiment end. Seagrass seeds sown with infaunal clams exhibited (C) over 500% increase in in patch area relative to initial patch size (p = 0.023), and (D) seven times more belowground biomass, p = 0.04, and significantly greater (E) total nitrogen above- and belowground (p < 0.001, p < 0.001). Error bars represent one standard error.
When outplanting mixed-species seagrass sods, we did not find a significant effect of clam addition or an interactive effect of clams and planting arrangement on patch productivity. However, post hoc tests revealed siginificant variation between large-intact and several-dispersed configurations (Supplementary B and Supplementary Table S3). Patches transplanted as a single large-intact unit gained shoots in both August and September (2 and 3 months post-planting, intact vs. dispersed p < 0.001, Figures 4A–C); whereas, those divided into a several-dispersed arrangement consistently lost 2–21% of shoots throughout the experiment duration. Furthermore, despite all treatments having an initial total grass area of 625 cm2 and no significant difference in initial eelgrass or shoalgrass density, large-intact patches on average increased in area, and several-dispersed patches decreased (p = 0.002, Figure 4D). Similarly, large-intact patches were nearly twice as large in area as several-dispersed patches at experiment end (p < 0.001).
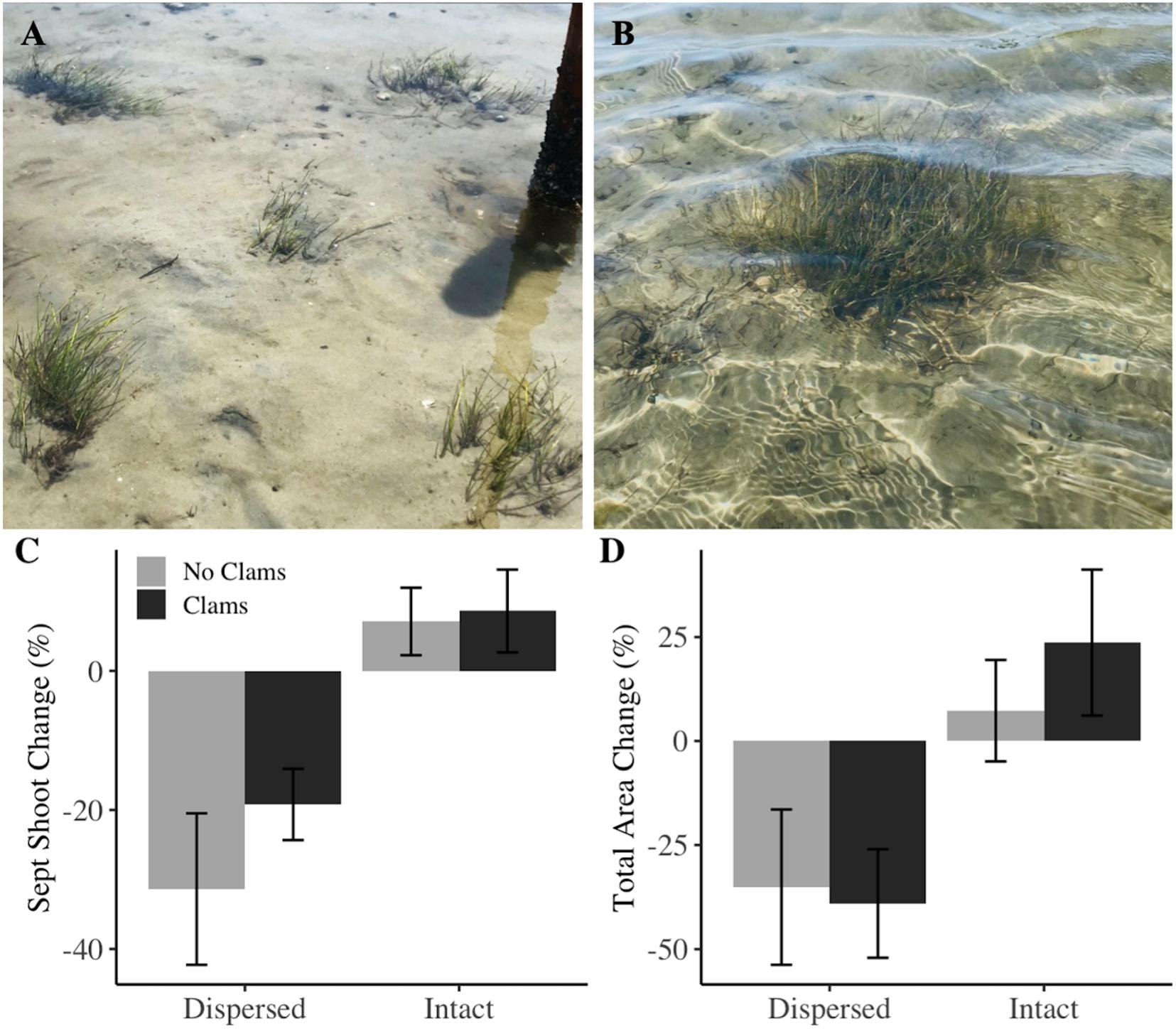
Figure 4. Configurations of (A) dispersed and (B) single-intact plots. Photos taken approximately 1 month after planting in July 2018. Interactive and separate effects of adult planting configuration and clam addition from ANOVA tests indicated that clams did not alter productivity; rather, intact patches had significantly greater (C) proportional change in shoot density from August to September, and (D) total change in patch area over the entire experimental duration from June to September than several-dispersed configurations. Error bars represent one standard error.
Discussion
Facilitations and mutualisms are powerful species interactions that play important roles in the organization, stability, and especially the recovery of coastal ecosystems (He et al., 2013; He and Bertness, 2014; Thomsen et al., 2018). Because restoration frequently takes place in heavily degraded areas that have lost many of the positive feedbacks crucial for maintaining ecosystem structure and stability, it is necessary for methodologies to consider and include approaches that harness beneficial species interactions. Here, we demonstrate that while including positive interactions in seagrass restoration approaches is an uncommon approach, for two different seagrass life stages, it can greatly increase restoration outcomes. For seeds, clam additions reversed restoration outplant trajectory from failure to success, while intraspecific facilitation greatly increased expansion rates of adult outplants. Combined, our survey results and experimental findings suggest a need to expand the theoretical framework of seagrass restoration to consider and incorporate all positive interactions possible, including facilitation cascades, intra- and interspecific facilitations within habitats, mutualisms, biodiversity enhancement, and long-distance facilitations.
Global Survey Reveals Stress-Avoidance Paradigm
In the next decade, efforts to conserve and restore coastal habitats and biodiversity will increase dramatically in frequency. Despite studies demonstrating the many benefits conferred by harnessing positive interactions in restoration (Halpern et al., 2007; Brooker et al., 2008), our practioner survey revealed that previous efforts have steered toward planting methodologies that minimize potential competitive or stressful interactions rather than capitalizing on intra- or interspecific facilitations. Although our survey was completed by respondents located across 23 different countries, it is still limited in geographic representation and is only a subset of all seagrass restorationers. In addition to stress-avoidance, multiple other factors may underlie our observed trends, including a lack of funding to restore multiple species, and/or a perception that multi-species restoration would be too costly and yield limited success compared to a single-species approach likely underlie the distinct lack of respondents who had attempted co-restoration of seagrasses with other habitats or organisms. Determining whether these prevailing approaches in seagrass restoration should be changed to systematically incorporate co-restoration and positive species interactions requires empirical tests that investigate how including facilitation can affect the success of seagrass plantings.
Clams Enhance Seed Establishment and Growth
Our restoration from seed experiment demonstrated that clam addition was associated with greater patch productivity and expansion. From subsequent CN analyses, we hypothesize that the mechanism for enhanced growth in our seed experiment was greater nitrogen uptake and concentration in newly germinated seagrass seeds and/or seedlings. The higher nitrogen content in both the leaves and rhizomes of seagrasses grown with clams suggests that organic matter deposited as pseudofeces from bivalves may elevate early seagrass growth (Peterson and Heck, 2001b). While our treatment of 10 juvenile seed clams (<1 cm umbo height) per 20 x 20 cm quadrat was initially high compared to observed natural adult clam densities in local seagrass beds (up to 6 m–2, pers. obs.), our findings suggest that post-mortality, clam densities per plot (∼2–3 patch–1) leveled out to those of naturally-occurring densities. Decomposition of clam tissues could also have been a significant source of nitrogen in addition to pseudofeces deposits. Regardless, our experimental findings suggest that the addition of nutrients from clams may play a particularly vital role in the early stages of eelgrass development and expansion from seed. In addition, because eelgrasses in North Carolina are annuals, increases in sexual reproductive effort, as observed in our seed-and-clam treatments, may potentially enhance seed bank densities and the likelihood of patches returning in subsequent years.
Previous studies have found that fertilization of outplants in nutrient-poor sediments can increase restoration success (van Katwijk et al., 2016), and bivalves within a habitat may provide a similar function without adding commercial fertilizers (Reusch et al., 1994; Gagnon et al., 2020). Because infaunal clams deposit pseudofeces on sediment rather than into the water column, nutrients provided in pseudofeces are more readily available for seagrasses to utilize (Peterson and Heck, 2001b). To capitalize on this facilitative mechanism, approaches must consider site sediment characteristics, as fertilization in already high-nutrient sediment may inhibit seagrass growth by increasing sediment sulfide concentrations (Vinther et al., 2008). In addition to nutrient dynamics, the abundance and density of bivalves needed will be influenced by predation regimes and predicted rates of natural mortality. Seed predation by fish, marine mammals, and benthic invertebrates can be a major factor in seed loss across multiple localities (Fishman and Orth, 1996; Holbrook et al., 2000; Nakaoka, 2002; Robert et al., 2002), and predators foraging for infauna may bioturbate seagrasses. However, infaunal bivalves could further facilitate seagrasses by aiding in seed burial thus enhancing the likelihood of germination and seedling establishment (Li et al., 2017). While we did not directly measure germination in our experiment and our estimates of viable seeds are not directly interchangeable with germination rates, initial counts of aboveground shoots did not indicate a significant difference between treatments with respect to seedling establishment.
Intra- but Not Interspecific Facilitation Enhances Adult Seagrass Planting Success
In contrast to seeds, we did not find evidence to support an effect of clam addition on the success of transplanted mixed Zostera marina and Halodule wrightii (eelgrass and shoalgrass, respectively) patches. Rather, intra-specific facilitations and planting a single large-intact rather that several-small dispersed patches, was associated with patch growth. Given our context-dependent finding of positive clam effects, more studies are needed to specifically identify when and where clams provide positive or neutral effects on seagrass growth. Considering differences in seagrass ontogeny as well as site characteristics and bivalve type will be key to determining the role of positive interactions and expanding the theoretical framework for restoration. In addition to nitrogen deposition, bivalves may facilitate seagrasses by reducing sulfide concentrations in the sediment (van der Heide et al., 2012; de Fouw et al., 2016; Chin, 2020; Van Der Geest et al., 2020) or by consuming and reducing epiphyte loads that could otherwise hinder seagrass photosynthesis (Peterson and Heck, 2001a, b). Although we did not test for the effect of bivalves on epiphytes in our experiments, we suspect that this mechanism would have had a stronger effect on the adult transplant experiment where clams were significantly larger than seed clams (<1 cm umbo width). Moreover, because adult out plant patches were a mix of eel- and shoalgrass, epiphyte effects may also have been minimized by the more abundant and narrower blades of shoalgrass.
Myriad studies have shown that intraspecific facilitation via positive density dependence is critical for seagrass success under a range of stressful physical and biological conditions (summarized in van Katwijk et al., 2016). Our findings, while challenging restoration paradigms, align thoroughly with what has been found in ecological studies. Seagrasses grown in large-intact patches may facilitate one another by reducing erosion- and flow-related stress, conferring resistance to soil anoxia, and by sharing resources between ramets (Van Keulen et al., 2003; van Katwijk et al., 2016). Because all plots had the same initial area and shoot density, our experiment further demonstrated that intact patches only 25 × 25 cm in dimension were large enough to promote positive intraspecific feedbacks. These advantages are likely to positively scale with patch size but may decrease as patches become extremely large. As patch size increases and positive feedbacks ameliorate stressful environmental conditions, transplants may start to compete (Maestre et al., 2009). Although we were not able to collect measurements that would give inference on mechanisms because of Hurricane Florence in September 2019, we hypothesize that larger clumps increased patch growth via positive effects conferred by intact root systems that facilitate anchoring, erosion reduction, and nutrient acquisition and allocation, given past findings. Future studies should elucidate whether a size-dependent inflection point exists where additional increases in intact patch size does not confer additional benefits, and the extent to which facilitation can increase resilience to large natural disturbances.
When examining changes by seagrass species, we found that shoalgrass drove the overall observed trends in patch shoot density in all treatments (Supplementary Figure S2), as eelgrass continually declined in density and was completely absent from all plots by September. This pattern of growth and dieback matches the natural history of our system, as shoalgrass is a tropical seagrass that is more productive in the summer months and persists through the early fall when the experiment was conducted; whereas, eelgrass is a temperate species growing at the southern limit of its range and is most productive in the spring and dies back mid-summer. Facilitation may not effectively combat heat stress when a species is already at a thermal limit. Instead, our findings suggest that planting multiple species rather than a seagrass monoculture, where appropriate, may infer longer-term resilience of restoration patches similar to the act of successively sowing seeds.
Conclusion
Our results point out that when, where, and what type of positive interaction that is incorporated into restoration designs can depend on the life stage and history strategy of the foundation species being restored. The utility of facilitations can also vary by species, site, and habitat and lead to differential benefits and results (He et al., 2013; Gagnon et al., 2020; Valdez et al., 2020). Our study adds to the growing amount of literature that calls for a new coastal restoration paradigm that systematically includes positive interactions and facilitation theory into designs (van der Heide et al., 2007; Silliman et al., 2015; Maxwell et al., 2017; Shaver and Silliman, 2017; Zhang et al., 2018; Renzi et al., 2019; Valdez et al., 2020). Although our practitioner survey revealed that many restoration methodologies do not incorporate intra or inter-specific facilitations, we empirically demonstrate that small changes in methodology to harness positive species interactions can significantly enhance restoration efficiency at little to no extra cost. Additional advances in restoration efficacy could be achieved by applying temporary (e.g., biodegradable) structures that mimic the facilitation-generating traits of the clumped restoration designs used in this study. Such hybrid ecology- and engineering-based approaches may be particularly useful in situations where the amount of available donor material is limited (Temmink et al., 2020). As restoration moves toward an ecosystem- rather than single-species approach (Palmer et al., 1997), expansion of restoration paradigms and approaches that incorporate systematic harnessing of all types of positive interactions, such as trophic and non-trophic facilitations, microbial mutualisms, intra- and interspecific facilitation within a habitat as well as long-distance facilitations that underscore multi-habitat restoration, is needed to advance and enhance the scale and success of restoration efforts as a whole (Halpern et al., 2007; Shaver and Silliman, 2017; Thomsen et al., 2018; Valdez et al., 2020).
Data Availability Statement
The raw data supporting the conclusions of this article will be made available by the authors, without undue reservation.
Ethics Statement
The studies involving human participants were reviewed and approved by the Duke University Campus IRB. Written informed consent for participation was not required for this study in accordance with the national legislation and the institutional requirements.
Author Contributions
YZ, RG, and BS designed the experiments. YZ, RG, and SD conducted the field experiments. YZ, RG, BS, SD, and ST designed and conducted the practitioner survey. YZ analyzed the data. All authors wrote and revised the manuscript and gave final approval for publication.
Funding
Funds supporting this research were provided by a North Carolina Albemarle-Pamlico National Estuary Preserve and Sea Grant Graduate Student Fellowship, a Duke University Graduate Summer Research Fellowship, a Duke University Marine Lab Writing Fellowship to YZ and a LenFest Ocean Foundation grant to BS.
Conflict of Interest
The authors declare that the research was conducted in the absence of any commercial or financial relationships that could be construed as a potential conflict of interest.
Acknowledgments
We thank A. Boyd, L. Gomez, C. Hoyt, M. Rudd, T. Toone, and J. Morton for field assistance. R. J. Orth and two reviewers provided comments and critiques that substantially improved this manuscript.
Supplementary Material
The Supplementary Material for this article can be found online at: https://www.frontiersin.org/articles/10.3389/fmars.2021.645673/full#supplementary-material
Data Sheet 1/Supplementary A | Practitioner survey solicitations and questionnaire.
Data Sheet 2/Supplementary B | Additional survey responses and experiment statistical analyses.
References
Angelini, C., Altieri, A. H., Silliman, B. R., and Bertness, M. D. (2011). Interactions among foundation species and their consequences for community organization, biodiversity, and conservation. BioScience 61, 782–789. doi: 10.1525/bio.2011.61.10.8
Bayraktarov, E., Saunders, M. I., Abdullah, S., Mills, M., Beher, J., Possingham, H. P., et al. (2016). The cost and feasibility of marine coastal restoration. Ecol. Appl. 26, 1055–1074. doi: 10.1890/15-1077
Beck, M. W., Brumbaugh, R. D., Airoldi, L., Carranza, A., Coen, L. D., Crawford, C., et al. (2011). Oyster reefs at risk and recommendations for conservation. Restor. Manag. BioSci. 61, 107–116. doi: 10.1525/bio.2011.61.2.5
BenDor, T. K., Livengood, A., Lester, T. W., Davis, A., and Yonavjak, L. (2015). Defining and evaluating the ecological restoration economy. Restor. Ecol. 23, 209–219. doi: 10.1111/rec.12206
Bos, A. R., and Van Katwijk, M. M. (2007). Planting density, hydrodynamic exposure and mussel beds affect survival of transplanted intertidal eelgrass. Mar. Ecol. Prog. Ser. 336, 121–129. doi: 10.3354/meps336121
Brooker, R. W., Maestre, F. T., Callaway, R. M., Lortie, C. L., Cavieres, L. A., Kunstler, G., et al. (2008). Facilitation in plant communities: the past, the present, and the future. J. Ecol. 96, 18–34.
Chin, D. W. (2020). Facilitation of a tropical seagrass by a chemosymbiotic bivalve increases with environmental stress. J. Ecol. 109, 204–217. doi: 10.1111/1365-2745.13462
Conacher, C. A., Poiner, I. R., Butler, J., Pun, S., and Tree, D. J. (1994). Germination, storage and viability testing of seeds of Zostera capricorni Aschers. from a tropical bay in Australia. Aquat. Bot. 49, 47–58. doi: 10.1016/0304-3770(94)90005-1
de Fouw, J., Govers, L. L., Van De Koppel, J., Van Belzen, J., Dorigo, W., and Sidi Cheikh, M. A. (2016). Drought, mutualism breakdown, and landscape-scale degradation of seagrass beds. Curr. Biol. 26, 1051–1056. doi: 10.1016/j.cub.2016.02.023
de Kroon, H. (1993). Competition between shoots in stands of clonal plants. Plant Species Biol. 8, 85–94. doi: 10.1111/j.1442-1984.1993.tb00060.x
Edwards, P. E. T., Sutton-Grier, A. E., and Coyle, G. E. (2013). Investing in nature: restoring coastal habitat blue infrastructure and green job creation. Mar. Policy 38, 65–71. doi: 10.1016/j.marpol.2012.05.020
ERA (2000). Estuary Restoration Act of 2000”, in: 33 USC 2901. 106 ed. (United States: Pub. L. 106-457).∗ (accessed August 1, 2019).
Fishman, J. R., and Orth, R. J. (1996). Effects of predation on Zostera marina L. seed abundance. J. Exp. Mar. Biol. Ecol. 198, 11–26. doi: 10.1016/0022-0981(95)00176-x
Gagnon, K., Rinde, E., Bengil, E. G., Carugati, L., Christianen, M. J., Danovaro, R., et al. (2020). Facilitating foundation species: the potential for plant–bivalve interactions to improve habitat restoration success. J. Appl. Ecol. 57, 1161–1179. doi: 10.1111/1365-2664.13605
Gedan, K. B., and Silliman, B. R. (2009a). “Patterns of salt marsh loss within coastal regions of North America: presettlement to present,” Human Impacts on Salt Marshes: A Global Perspective eds B. R. Silliman, M. D. Bertness, and E. D. Grosholz (Oakland, CA: University of California Press), 253–266.
Gedan, K. B., and Silliman, B. R. (2009b). Using facilitation theory to enhance mangrove restoration. Ambio 38:109. doi: 10.1579/0044-7447-38.2.109
Gittman, R. K., Fodrie, F. J., Baillie, C. J., Brodeur, M. C., Currin, C. A., Keller, D. A., et al. (2018). Living on the edge: increasing patch size enhances the resilience and community development of a restored salt marsh. Estuar. Coast. 41, 884–895. doi: 10.1007/s12237-017-0302-6
Halpern, B. S., Silliman, B. R., Olden, J. D., Bruno, J. P., and Bertness, M. D. (2007). Incorporating positive interactions in aquatic restoration and conservation. Front. Ecol. Environ. 5, 153–160. doi: 10.1890/1540-929520075[153:IPIIAR]2.0.CO;2
He, Q., and Bertness, M. D. (2014). Extreme stresses, niches, and positive species interactions along stress gradients. Ecology 95, 1437–1443. doi: 10.1890/13-2226.1
He, Q., Bertness, M. D., and Altieri, A. H. (2013). Global shifts towards positive species interactions with increasing environmental stress. Ecol. Lett. 16, 695–706. doi: 10.1111/ele.12080
Holbrook, S., Reed, D., Hansen, K., and Blanchette, C. (2000). Spatial and temporal patterns of predation on seeds of the surfgrass Phyllospadix torreyi. Mar. Biol. 136, 739–747. doi: 10.1007/s002270050733
Irlandi, E., and Mehlich, M. (1996). The effect of tissue cropping and disturbance by browsing fishes on growth of two species of suspension-feeding bivalves. J. Exp. Mar. Biol. Ecol. 197, 279–293. doi: 10.1016/0022-0981(95)00157-3
Lefcheck, J. S., Orth, R. J., Dennison, W. C., Wilcox, D. J., Murphy, R. R., Keisman, J., et al. (2018). Long-term nutrient reductions lead to the unprecedented recovery of a temperate coastal region. Proc. Natl. Acad. Sci.U.S.A. 115, 3658–3662. doi: 10.1073/pnas.1715798115
Li, C.-J., Li, W.-T., Liu, J., Zhang, X., and Zhang, P. (2017). Zostera marina seed burial can be enhanced by manila clam ruditapes philippinarum: a microcosm study. Ocean Sci. J. 52, 221–229. doi: 10.1007/s12601-017-0016-5
Livernois, M. C., Grabowski, J. H., Poray, A. K., Gouhier, T. C., Hughes, A. R., O’brien, K. F., et al. (2017). Effects of habitat fragmentation on Zostera marina seed distribution. Aquat. Bot. 142, 1–9. doi: 10.1016/j.aquabot.2017.05.006
Maestre, F. T., Callaway, R. M., Valladares, F., and Lortie, C. J. (2009). Refining the stress-gradient hypothesis for competition and facilitation in plant communities. J. Ecol. 97, 199–205. doi: 10.1111/j.1365-2745.2008.01476.x
Marion, S. R., and Orth, R. J. (2010). Innovative techniques for large-scale seagrass restoration using Zostera marina (eelgrass) seeds. Restor. Ecol. 18, 514–526. doi: 10.1111/j.1526-100x.2010.00692.x
Marsh, J. A., Dennison, W. C., and Alberte, R. S. (1986). Effects of temperature on photosynthesis and respiration in eelgrass (Zostera marina L.). J. Exp. Mar. Biol. Ecol. 101, 257–267. doi: 10.1016/0022-0981(86)90267-4
Maxwell, P. S., Eklöf, J. S., Van Katwijk, M. M., O’brien, K. R., De La Torre−Castro, M., and Boström, C. (2017). The fundamental role of ecological feedback mechanisms for the adaptive management of seagrass ecosystems–a review. Biol. Rev. 92, 1521–1538. doi: 10.1111/brv.12294
Moore, K. A., Orth, R. J., and Nowak, J. F. (1993). Environmental regulation of seed germination in Zostera marina L. (eelgrass) in chesapeake bay: effects of light, oxygen and sediment burial. Aquat. Bot. 45, 79–91. doi: 10.1016/0304-3770(93)90054-z
Nakaoka, M. (2002). Predation on seeds of seagrasses Zostera marina and Zostera caulescens by a tanaid crustacean Zeuxo sp. Aquat. Bot. 72, 99–106. doi: 10.1016/s0304-3770(01)00213-3
Orth, R. J., Moore, K. A., Marion, S. R., Wilcox, D. J., and Parrish, D. B. (2012). Seed addition facilitates eelgrass recovery in a coastal bay system. Mar. Ecol. Prog. Ser. 448, 177–195. doi: 10.3354/meps09522
Palmer, M. A., Ambrose, R. F., and Poff, N. L. (1997). Ecological theory and community restoration ecology. Restor. Ecol. 5, 291–300. doi: 10.1046/j.1526-100x.1997.00543.x
Peterson, B. J., and Heck, K. L. Jr. (2001a). An experimental test of the mechanism by which suspension feeding bivalves elevate seagrass productivity. Mar. Ecol. Prog. Ser. 218, 115–125. doi: 10.3354/meps218115
Peterson, B. J., and Heck, K. L. Jr. (2001b). Positive interactions between suspension-feeding bivalves and seagrass-a facultative mutualism. Mar. Ecol. Prog. Ser. 213, 143–155. doi: 10.3354/meps213143
Peterson, C. H., and Beal, B. F. (1989). Bivalve growth and higher order interactions: importance of density, site, and time. Ecology 70, 1390–1404. doi: 10.2307/1938198
Reise, K., and Kohlus, J. (2008). Seagrass recovery in the Northern wadden sea? Helgol. Mar. Res. 62, 77–84. doi: 10.1007/s10152-007-0088-1
Renzi, J. J., He, Q., and Silliman, B. R. (2019). Harnessing positive species interactions to enhance coastal wetland restoration. Front. Ecol. Evol. 7:131. doi: 10.3389/fevo.2019.00131
RESTORE Act (2012). Resources and Ecosystems Sustainability, Tourist Opportunities, and Revived Economies of the Gulf Coast States Act of 2012”, in: 33 USC 1321. 112 ed. (United States).∗ (accessed August 1, 2019).
Reusch, T. B. H., Chapman, A. R. O., and Groger, J. P. (1994). Blue mussels Mytilus edulis do not interfere with eelgrass Zostera marina but fertilize shoot growth through biodeposition. Mar. Ecol. Prog. Ser. 108, 265–282. doi: 10.3354/meps108265
Robert, J. O., Kenneth, L. H. Jr., and David, J. T. (2002). Predation on seeds of the seagrass posidonia australis in Western Australia. Mar. Ecol. Prog. Ser. 244, 81–88. doi: 10.3354/meps244081
Saunders, M. I., Doropoulos, C., Bayraktarov, E., Babcock, R. C., Gorman, D., Eger, A. M., et al. (2020). Bright spots in coastal marine ecosystem restoration. Curr. Biol. 30, R1500–R1510.
Shaver, E. C., and Silliman, B. R. (2017). Time to cash in on positive interactions for coral restoration. PeerJ 5:e3499. doi: 10.7717/peerj.3499
Silliman, B. R., Schrack, E., He, Q., Cope, R., Santoni, A., Van Der Heide, T., et al. (2015). Facilitation shifts paradigms and can amplify coastal restoration efforts. Proc. Natl. Acad. Sci. U.S.A. 112, 14295–14300. doi: 10.1073/pnas.1515297112
Sutton-Grier, A. E., Gittman, R. K., Arkema, K. K., Bennett, R. O., Benoit, J., Blitch, S., et al. (2018). Investing in natural and nature-based infrastructure: building better along our coasts. Sustainability 10:523. doi: 10.3390/su10020523
Sutton-Grier, A. E., Wowk, K., and Bamford, H. (2015). Future of our coasts: the potential for natural and hybrid infrastructure to enhance the resilience of our coastal communities, economies and ecosystems. Environ. Sci. Policy 51, 137–148. doi: 10.1016/j.envsci.2015.04.006
Temmink, R. J. M., Christianen, M. J. A., Fivash, G. S., Angelini, C., Boström, C., Didderen, K., et al. (2020). Mimicry of emergent traits amplifies coastal restoration success. Nat. Commun. 11, 3668–3668.
Thomsen, M. S., Altieri, A. H., Angelini, C., Bishop, M. J., Gribben, P. E., Lear, G., et al. (2018). Secondary foundation species enhance biodiversity. Nat. Ecol. Evol. 2, 634–639. doi: 10.1038/s41559-018-0487-5
Valdez, S. R., Zhang, Y. S., Van Der Heide, T., Vanderklift, M. A., Tarquinio, F., Orth, R. J., et al. (2020). Positive ecological interactions and the success of seagrass restoration. Front. Mar. Sci. 7:91. doi: 10.3389/fmars.2020.00091
Van Der Geest, M., Van Der Heide, T., Holmer, M., and De Wit, R. (2020). First field-based evidence that the seagrass-lucinid mutualism can mitigate sulfide stress in seagrasses. Front. Mar. Sci. 7:11. doi: 10.3389/fmars.2020.00011
van der Heide, T., Govers, L. L., De Fouw, J., Olff, H., Van Der Geest, M., Van Katwijk, M. M., et al. (2012). A three-stage symbiosis forms the foundation of seagrass ecosystems. Science 336, 1432–1434. doi: 10.1126/science.1219973
van der Heide, T., Van Nes, E. H., Geerling, G. W., Smolders, A. J., Bouma, T. J., and Van Katwijk, M. M. (2007). Positive feedbacks in seagrass ecosystems: implications for success in conservation and restoration. Ecosystems 10, 1311–1322. doi: 10.1007/s10021-007-9099-7
van Katwijk, M. M., Thorhaug, A., Marbà, N., Orth, R. J., Duarte, C. M., Kendrick, G. A., et al. (2016). Global analysis of seagrass restoration: the importance of large−scale planting. J. Appl. Ecol. 53, 567–578.
Van Keulen, M., Paling, E. I., and Walker, C. J. (2003). Effect of planting unit size and sediment stabilization on seagrass transplants in Western Australia. Restor. Ecol. 11, 50–55. doi: 10.1046/j.1526-100x.2003.00036.x
Vinther, H. F., Laursen, J. S., and Holmer, M. (2008). Negative effects of blue mussel (Mytilus edulis) presence in eelgrass (Zostera marina) beds in Flensborg fjord, Denmark. Estuar. Coast. Shelf Sci. 77, 91–103. doi: 10.1016/j.ecss.2007.09.007
Wall, C. C., Peterson, B. J., and Gobler, C. J. (2008). Facilitation of seagrass Zostera marina productivity by suspension-feeding bivalves. Mar. Ecol. Prog. Ser. 357, 165–174. doi: 10.3354/meps07289
Waycott, M., Duarte, C. M., Carruthers, T. J. B., Orth, R. J., Dennison, W. C., Olyarnik, S., et al. (2009). Accelerating loss of seagrasses across the globe threatens coastal ecosystems. Proc. Natl. Acad. Sci.U.S.A. 106, 12377–12381. doi: 10.1073/pnas.0905620106
Keywords: conservation, facilitation, positive interactions, restoration, seagrass
Citation: Zhang YS, Gittman RK, Donaher SE, Trackenberg SN, van der Heide T and Silliman BR (2021) Inclusion of Intra- and Interspecific Facilitation Expands the Theoretical Framework for Seagrass Restoration. Front. Mar. Sci. 8:645673. doi: 10.3389/fmars.2021.645673
Received: 23 December 2020; Accepted: 08 March 2021;
Published: 22 April 2021.
Edited by:
Romuald Lipcius, College of William & Mary, United StatesReviewed by:
Inés Mazarrasa, Environmental Hydraulics Institute (IHCantabria), SpainElisabeth Marijke Anne Strain, University of Tasmania, Australia
Copyright © 2021 Zhang, Gittman, Donaher, Trackenberg, van der Heide and Silliman. This is an open-access article distributed under the terms of the Creative Commons Attribution License (CC BY). The use, distribution or reproduction in other forums is permitted, provided the original author(s) and the copyright owner(s) are credited and that the original publication in this journal is cited, in accordance with accepted academic practice. No use, distribution or reproduction is permitted which does not comply with these terms.
*Correspondence: Y. Stacy Zhang, stacy.zhang@unc.edu