Dietary Lipid Effects on Gut Microbiota of First Feeding Atlantic Salmon (Salmo salar L.)
- 1Department of Ichthyology and Aquatic Environment, School of Agricultural Sciences, University of Thessaly, Volos, Greece
- 2Department of Biotechnology and Food Science, Norwegian University of Science and Technology (NTNU), Trondheim, Norway
- 3Department of Biology, Norwegian University of Science and Technology (NTNU), Trondheim, Norway
Decline in fish oil and fish meal availability has forced the aquaculture sector to investigate alternative and sustainable aquafeed ingredients. Despite that several studies have evaluated the effect of fish oil replacement in aquaculture fish species, there is a knowledge gap on the effects of alternative dietary lipid sources on the gut microbiota in early life stages of Salmo salar. The present study evaluated the influence of dietary administration of two different lipid sources (fish oil and vegetable oil) on the intestinal microbiota of first feeding Atlantic salmon (S. salar) up to 93 days post first feeding (dpff). The two diets used in this study, FD (fish oil diet) and VD (blend of rapeseed, linseed and palm oils diet), were formulated to cover the fish nutritional requirements. Apart from the lipid source, the rest of the feed components were identical in the two diets. Hindgut samples were collected at 0, 35, 65, and 93 dpff. Moreover, fertilized eggs, yolk sac larvae, rearing water and feed were also collected in order to assess a possible contribution of their microbiota to the colonization and bacterial succession of the fish intestines. To analyze the bacterial communities, amplicon sequencing was used targeting the V3–V4 region of the 16S rRNA gene. The findings indicate that feeding on either fish oil or vegetable oil-based diet, fish growth variables (mean wet weight and total length) did not differ significantly during the experiment (p > 0.05). No significant differences were also found between the two dietary groups, regarding their gut bacteria composition, after the analysis of the 16S rRNA sequencing data. Instead, gut microbiota changed with age, and each stage was characterized by different dominant bacteria. These operational taxonomic units (OTUs) were related to species that provide different functions and have been isolated from a variety of environments. The results also show little OTUs overlap between the host and rearing environment microbiota. Overall, this study revealed the occurrence of a core microbiota in early life of Atlantic salmon independent of the feed-contained oil origin.
Introduction
Fishmeal and fish oil have been the main ingredients in diets for farmed carnivorous fish species, providing the fed fish with the necessary proteins and lipids for high growth performance and resulting in a nutritionally rich final product (International Fishmeal and Oil Manufacturers Association [IFOMA], 2001; Turchini et al., 2010). Due to the declining availability of fishmeal and fish oil, their contents in feed are reduced (Hardy, 2010) and substituted by a variety of alternative feed ingredients. As changes in fish diet ingredients can alter the gut microbiota of fish species, it is important to evaluate the impact of these new diets with lower fish-meal and -oil contents on the composition of the gut microbial communities for reared fish species (for a review see Ringø et al., 2016).
In Atlantic salmon (S. salar), a carnivorous fish with significant economic value in European aquaculture (FAO, 2004), the effect of the alternative aquafeed ingredients on the gut microbiota have been evaluated previously and, in some cases, it was revealed that changes associated with intestinal disorders and slower growth performance, were related to the fishmeal diets (e.g., Green et al., 2013; Navarrete et al., 2013; Schmidt et al., 2016; Gajardo et al., 2017; Booman et al., 2018; Egerton et al., 2020). These studies, however, have focused mainly on alternative protein sources and on juveniles and adult stages.
Although feed is considered as the main factor that affect the gut bacterial communities in fish species, data from previous studies have also shown variations in gut microbial communities across development stages which seem to be affected not only by the provided feed but also from the microbial communities of the rearing environment (Bakke et al., 2013, 2015; Stephens et al., 2016; Dehler et al., 2017; Egerton et al., 2018). For example, recent work by Minich et al. (2020) recognizes the strong association between the build environment, i.e., tank biofilm and water from the hatchery installation, and Atlantic salmon mucosal microbiota. In a different salmonid species (rainbow trout), gut microbiota was detectable before first feeding commenced, potentially due to contact with the surrounding water and yok sac digestion, indicating that gut microbiota establishment initiates at first feeding and that diet-type affect the bacterial composition (Ingerslev et al., 2014a,b).
Moreover, it has been reported, that fish egg fragments are consumed from the newly hatched larvae, and their microbiome can affect gut microbiota colonization in fish species (Olafsen, 1984; Beveridge et al., 1991; Nikouli et al., 2019). The significant stage of the mouth opening, in aspect of larval microbiota manipulation, have been also recognized in shrimp larvae by Wang et al. (2020) in aquaculture conditions. In addition, evidence also suggest that as early life stages are more prone in environmental/climate changes, then probably is more crucial to study the microbiome shaping on these stages (Lowe et al., 2021). On the other hand, studies have shown that host development considered to had greater effect than hatching environment on the gut microbiota colonization and succession (Califano et al., 2017; Nikouli et al., 2019; Xiao et al., 2021).
Apart from a few studies, which have investigated the gut microbial communities in early life stages of Atlantic salmon (Llewellyn et al., 2016; Dehler et al., 2017; Lokesh et al., 2019), there is a knowledge gap on the effects of a different dietary lipid source on the gut microbiota in early life stages of this fish species, as only Clarkson et al. (2017) have partially investigated the impact of fish oil replacement by vegetable oils during a dietary experiment in diploid and triploid populations of Atlantic salmon. The objective of the present study was to evaluate the influence of total replacement of fish oil with a blend of terrestrial alternative oils (rapeseed, linseed and palm oils) on the intestinal microbiota of first feeding Atlantic salmon. We also characterized the bacterial communities of the rearing environment to determine their contribution in the early colonization and the succession of the fish intestines.
Materials and Methods
Experimental Design and Sampling
The study was carried out within the Norwegian animal welfare act guidelines, in accordance with EU regulation (EC Directive 2010/63/EU), approved by the Animal Ethics and Welfare Committee of the Norwegian University of Science and Technology (case number 16/10070). The experiment was conducted at the Ervik hatchery (Frøya, Norway) as described previously in Jin et al. (2019). Briefly, a fast-growing Atlantic salmon aquaculture strain was cultivated from fertilized eggs until 93 days post first feeding (dpff). The two diets used in this study, FD (fish oil diet) and VD (blend of rapeseed, linseed and palm oils diet), were formulated to cover the fish nutritional requirements. Apart from the lipid source, the rest of the feed components were identical in the two diets (see Supplementary Table 1).
Each dietary treatment was tested in duplicated groups of 200 Atlantic salmon individuals (0.23 g ± 0.03/fish). On sampling days (0, 35, 65 and 93 dpff) 10 fish from each tank were randomly collected and sacrificed by immersion in 40 mg/L Benzocaine (BENZOAK VET, ACD Pharmaceuticals AS, Oslo, Norway). Furthermore, duplicate samples of rearing water (100 ml/tank) were collected and filtered through 0.2 μm membrane filters (GTTP, Millipore, United States) using a low (<1,500 mmHg) vacuum apparatus. For gut microbiota analysis, hindguts were removed by aseptic dissection and rinsed with ultra-pure water. Moreover, 10 fertilized eggs (EG), 10 yolk sac larvae (YS), and 0.25 g of the provided feeds were sampled in order to assess the contribution of their microbiota on the colonization of Atlantic salmon gut.
DNA Extraction and Sequencing
DNA was isolated from Atlantic salmon (eggs/yolk sac larvae/hindguts) and environmental (water/diets) samples by using the QIAGEN QIAamp DNA Mini Kit (Qiagen, Hilden, Germany) following the manufacturer’s protocol “DNA Purification from Tissues.” Bacterial communities were characterized by 16S rRNA amplicon sequencing. All samples analyzed individually and pooled prior the 16S rRNA analysis as follow: (a) DNA extracts from 5 individual fish samples (eggs, yolk sac larvae and hindguts) were pooled, resulting in two pooled samples from each time point/fish tank (Supplementary Table 2) and (b) the DNA from the rearing water samples were pooled, resulting in 1 water sample per replicate tank (“STW”—initial stock tank, “FW”—rearing water from fish oil (FD) group and “VW”—rearing water from vegetable oil (VD) treatment.
PCR amplification and sequencing was performed at MRDNA Ltd.1 (Shallowater, TX, United States) facilities on a MiSeq using paired end reads (2 × 300 bp) following the manufacturer’s guidelines. A total of 37 samples (representing 30 pooled fish samples, 5 pooled water samples and 2 feed samples) was used in the final amplicon library. The 16S rRNA gene V3-V4 variable region PCR primers S-D-Bact-0341-b-S-17 and S-D-Bact-115 0785-a-A-21 (Klindworth et al., 2013) with barcodes on the forward primer (Supplementary Table 3) were used in a 30 cycle PCR using the HotStarTaq Plus Master Mix Kit (Qiagen, United States) under the following conditions: 94°C for 3 min, followed by 30 cycles of 94°C for 30 s, 53°C for 40 s, and 72°C for 1 min, after which a final elongation step at 72°C for 5 min was performed. After amplification, PCR products were checked in 2% agarose gel to determine the success of amplification and the relative intensity of bands. After that, the samples were pooled together in equal proportions based on their molecular weight and DNA concentrations. Pooled samples were purified using calibrated Ampure XP beads. Then the pooled and purified PCR product was used to prepare illumina DNA library.
Data Analysis
Sequencing raw data were processed with the MOTHUR software (version 1.40.5) (Schloss et al., 2009, 2011) and the operational taxonomic units (OTUs) were classified with the SILVA database release 132 (Quast et al., 2013; Yilmaz et al., 2014) following the methodology described in Nikouli et al. (2018). Identification of closest relative of the Most abundant OTUs was performed with Nucleotide Blast2. Raw sequence data from this study have been submitted to the Sequence Read Archive3 with BioProject accession number PRJNA520982. Statistical analysis and graphical illustrations were performed in the Palaeontological STudies (PAST) software (Hammer et al., 2001) and in the R Studio platform Version 1.1.419 (RStudio Team, 2020), with 3.4.3 R version and enveomics.R package, Version 1.2.0 (Rodriguez-R and Konstantinidis, 2016).
Results
Fish Growth Performance
The growth performance of the fish was evaluated throughout the experiment (see Supplementary Table 4 and Supplementary Figure 1) and at none of the sampling points the mean wet weight or total length differed significantly across replicate tanks or between dietary treatments (FD and VD) (p > 0.05; Supplementary Figure 1). The initial (D0) mean weight was 0.23 ± 0.03 g (±SD). After 93 days (D93) the final mean weight was 4.58 ± 1.74 g for the group FD and 4.54 ± 1.78 g for the group VD. Regarding total mean length, the initial (D0) was 29.9 ± 1.6 cm which increased to 76.0 ± 8.9 cm and 73.8 ± 9.2 cm at D93 for the groups FD and VD, respectively.
Bacterial Diversity
The analysis of the 16S rRNA sequencing data revealed a total of 4,548 unique OTUs, with the rarefaction curves (Supplementary Figure 2) and the OTUs richness coverage based on the Chao1 index (Supplementary Table 5) indicating satisfactory sequencing depth. Diversity was considerable higher for rearing water (STW, FW, VW) than gut and diet samples, both in terms of OTU richness (Table 1) and evenness (Supplementary Table 5).
Taxonomic classification showed the presence of 21 bacterial phyla (Figure 1 and Supplementary Figure 3). OTUs which were not classified to known bacterial phyla were only 3.0% of the relative abundance and are assigned as “Bacteria_unclassified.” Proteobacteria (37.2%), Firmicutes (23.9%), Actinobacteria (18.8%), and Bacteroidetes (12.8%) were the dominant bacterial phyla in the dataset. The remaining 18 phyla (Planctomycetes, Verrucomicrobia, Patescibacteria, Dependentiae, Acidobacteria, Gemmatimonadetes, Fusobacteria, Cyanobacteria, Deinococcus-Thermus, Fibrobacteres, Armatimonadetes, Nitrospirae, Spirochaetes, Elusimicrobia, Omnitrophicaeota, Tenericutes, Chloroflexi, and Kiritimatiellaeota) were present with relative abundance ≤ 2%.
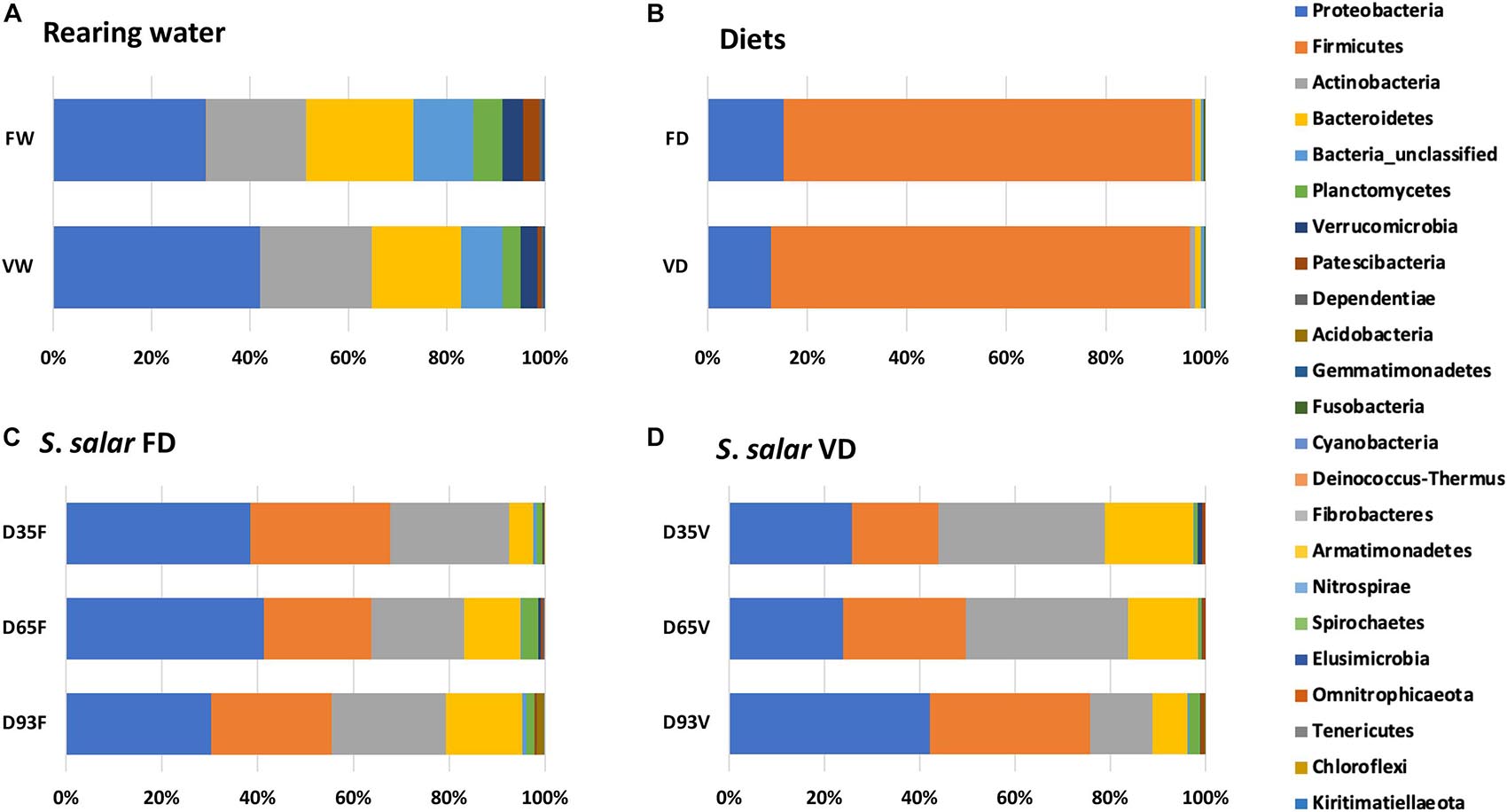
Figure 1. Phylum composition of microbiota from rearing water (A), diets (B) and hindgut of S. salar samples in Fish oil “FD” (C) and vegetable oil “VD” (D) dietary treatments, at D35, D65, and D93 post first feeding.
S. salar Microbiota
Comparing Atlantic salmon microbiota between the different life stages, fertilized eggs (EG) had the highest observed and estimated (Chao1) OTU richness (172 ± 100 and 222 ± 114, respectively). At the yolk sac stage (YS), the OTU richness decreased to 87 ± 0.7 and increased again at first feeding (D0). After that, OTU richness was on the same level until D93 when it decreased (Table 1). Proteobacteria was the dominant bacterial phylum in the samples, mainly due to γ- and β-Proteobacteria (Supplementary Figure 4). β-Proteobacteria was the dominant subphylum in pre-feeding stages (EG, YS, D0), with representatives mainly from the Burkholderiaceae and Chitinibacteraceae families (Supplementary Figure 5). However, in fertilized eggs (EG), OTUs representing β-Proteobacteriales were classified only at class level (44.1% of the total reads). γ-Proteobacteria dominated the period with active feeding (D35—D93) in both dietary treatments, with Pseudomonadaceae, Xanthomonadaceae, Vibrionaceae, Enterobacteriaceae, Moraxellaceae, and Aeromonadaceae as the most abundant families. However, their relative abundances differed between the two dietary treatments (Supplementary Figure 6). Actinobacteria, the dominant bacterial phylum at the late stages (D35 and D65) in vegetable oil dietary group (VD), was due to the high relative abundance of mainly Propionibacteriales, Corynebacteriales, and Micrococcales representatives. The presence of Firmicutes and Bacteroidetes was due to the classes Bacilli and Bacteroidia.
Microbial Communities in Diets and Rearing Water
The bacterial communities in feed samples, consisted almost entirely of Firmicutes (relative abundance of 84.2 and 82.1% in FD and VD, respectively; Figure 1). The Firmicutes were affiliated to the Lactobacillaceae (38.5 and 36.6% in FD and VD, respectively) and Leuconostocaceae families (37.9 and 38.8% in FD and VD, respectively). The rearing water samples (VW, FW, WST) contained mainly Proteobacteria, Actinobacteria and Bacteroidetes species, with Burkholderiaceae (β-Proteobacteria), Sporichthyaceae (Actinobacteria) and Chitinophagaceae (Bacteroidetes) as the most abundant families (Figure 1). In contrast to the experimental diets, Firmicutes in water samples were detected in relative abundance ≤ 1%.
Similarities Between Bacterial Communities
Statistical analysis revealed no significant differences (Tukey’s test, p > 0.05, Supplementary Table 6) in the bacterial community composition of the Atlantic salmon samples between the pre-feeding stages (EG, YS, D0). However, EG and D0 samples differed significantly from those taken during the feeding period (D35—D93) in both dietary treatments, with stage D35 in VD group as the only exception. YS bacterial communities differed significantly (p < 0.05) with the bacterial communities only at D93 in both dietary groups (FD and VD). The gut microbiota of the host did not reveal significant differences between the two dietary groups for the different stages (p > 0.05), again with stage D35 in VD group as the only exception (Supplementary Table 6).
Further comparison of the bacterial community composition of Atlantic salmon hindguts, based on a Bray–Curtis distance matrix (Figure 2), showed a clear separation between bacterial communities in gut and bacterial communities of the rearing environment (water and diets). Moreover, the bacterial communities of the host were more similar with respect to life stages than to the diet treatments (Figure 2 and Supplementary Figure 7), and this is also indicated through the similarity percentages analysis (SIMPER) based on Bray–Curtis distance. According to the results of the analysis the average dissimilarity among the groups of the same life stages was 76.0%, whereas the average dissimilarity within groups of the same dietary treatment was 78.5% (FD) and 83.6% (VD).
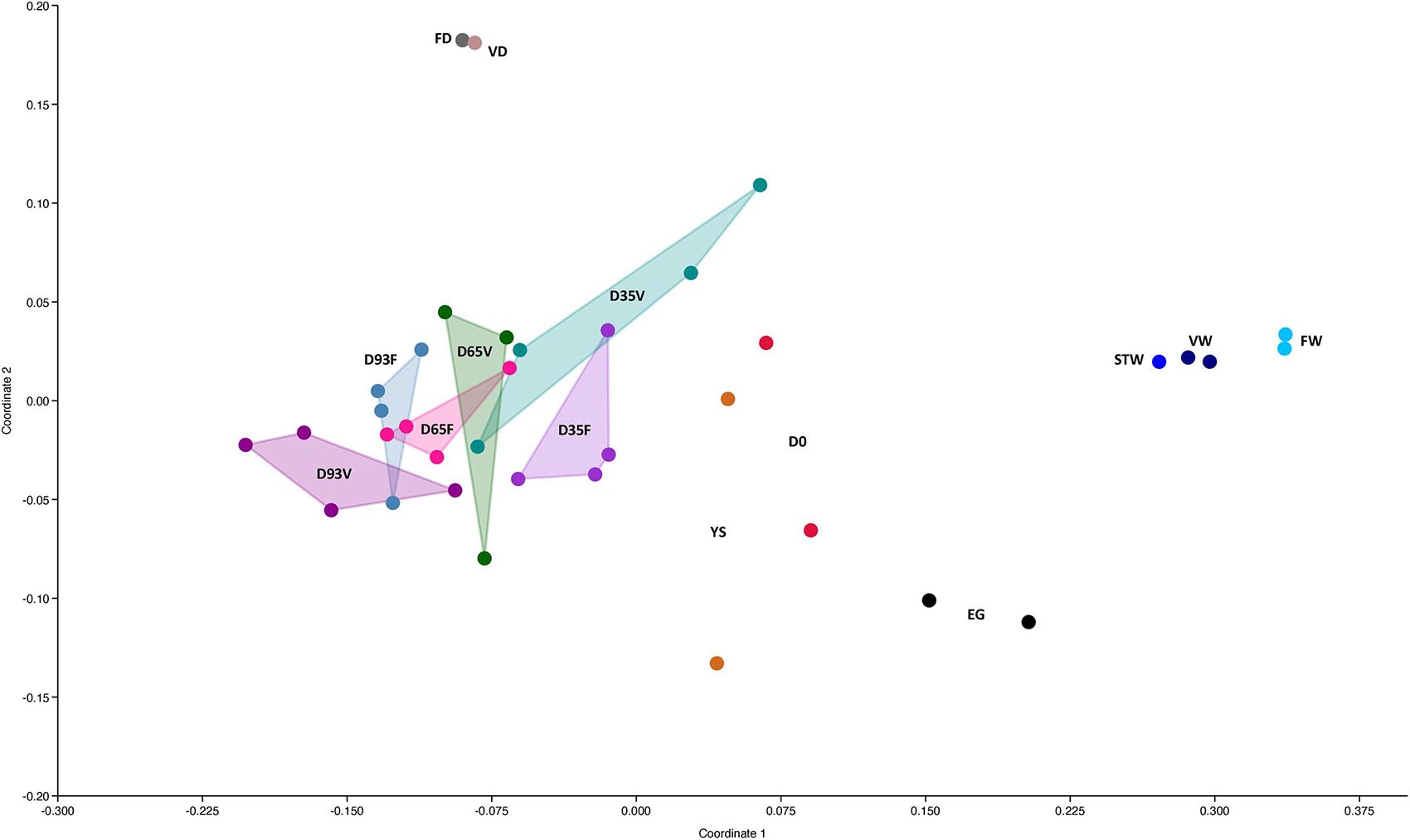
Figure 2. Non-metric multidimensional scaling (nMDS) plot for all the bacterial communities of all sample categories based on Bray-Curtis distances. Pre-feding stages: Eggs (EG), yolk sac larvae (YS) and “D0”. Feeding stages of (a) fish oil group (FD): D35F, D65F, and D93F and (b) vegetable oil group (VD): D35V, D65V, and D93V. Rearing water: Pre-feeding tank water (STW), Fish oil group rearing water (FW), vegetable oil group (VW). Feed: vegetable oil feed (VD) and fish oil feed (FD). D, Day.
Common and Unique OTUs
Overall, only 2.3% of the OTUs were found in all sample types (rearing water, diets, pre- and after first feeding hindguts). 75.4% of OTUs occurred only in water samples (Figure 3). From the 1,004 OTUs detected in total in Atlantic salmon samples, 423 OTUs (9.3% of the OTUs) were unique in that type of samples. The majority of them (343 OTUs) were unique in the host at the active feeding stages, whereas 13 OTUs were shared among all samples independent of life stage or diet treatment.
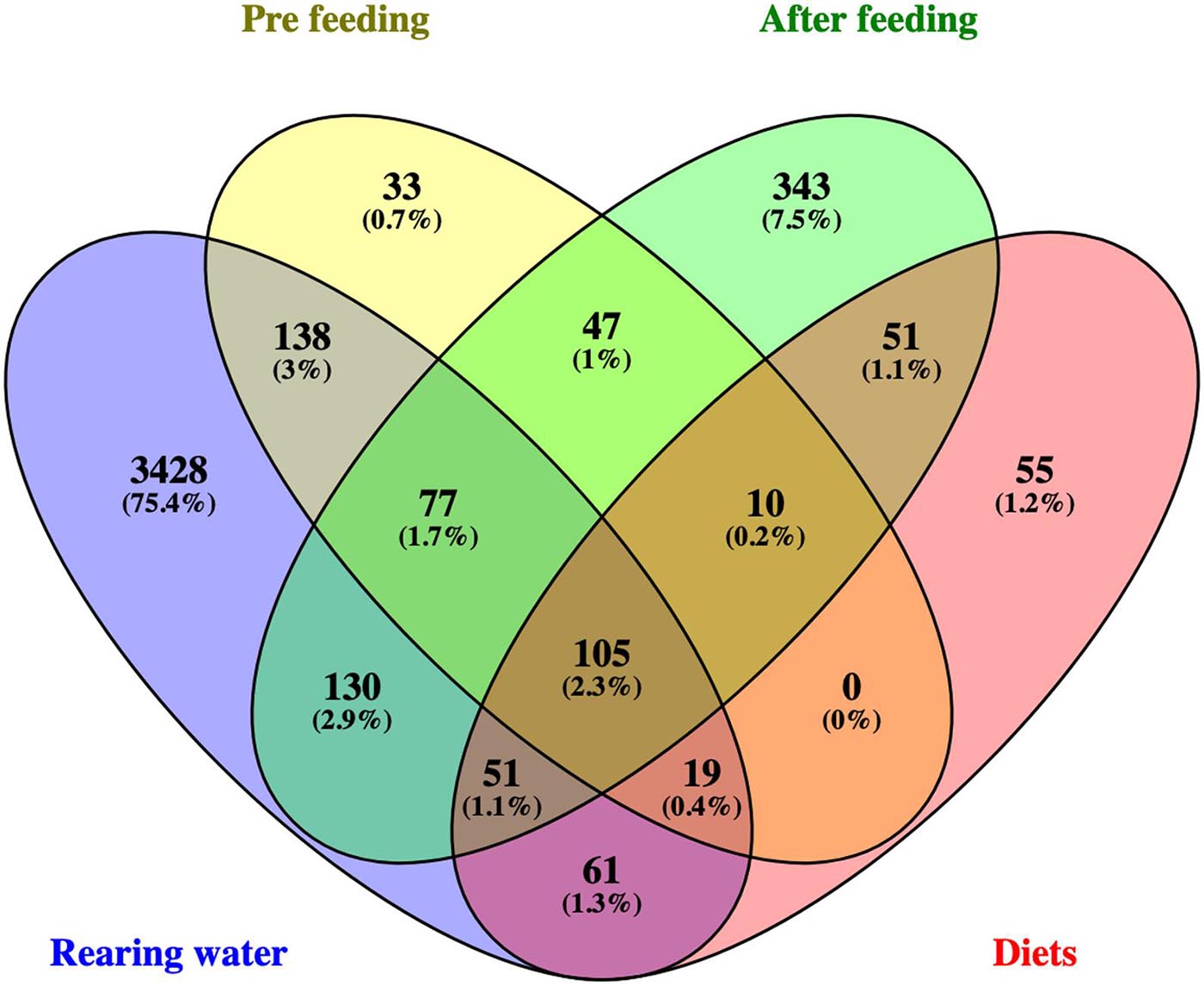
Figure 3. Venn diagram demonstrating the number of the shared and unique operational taxonomic units (OTU) and their percentage of the total library, between S. salar samples [pre-feeding (EG,YS, D0) and post first feeding stages (D35, D65, D93 in both diets)], diets (FD and VD) and rearing water sample (STW, FW, VW).
Discussion
In the present study, we evaluated the influence of dietary administration of two different lipid sources (fish oil and vegetable oil) on the gut bacterial communities of first feeding Atlantic salmon. Moreover, we characterized the bacterial communities from the rearing environment (rearing water and feeds) and the epibiotas of fertilized eggs and yolk sac larvae to determine their contribution in the bacterial colonization and succession of the gut. Previous studies suggest that the bacterial communities of the rearing environment, mainly from the rearing water and the feed, are important sources for community assembly of the intestinal microbiota of fish (Hansen and Olafsen, 1999; Nayak, 2010; McDonald et al., 2012; Scott et al., 2013; Bolnick et al., 2014; Eichmiller et al., 2016; Kashinskaya et al., 2018). For example, Schmidt et al. (2016), reported a significant effect on intestinal microbial communities in postsmolt Atlantic salmon following replacement of dietary fishmeal with plant ingredients. However, the results in the present study suggest that substitution of fish oil by vegetable oils did not significantly affect the composition of intestinal microbial communities in the same host species.
Furthermore, the results of the present study indicate little overlap between the bacterial communities of the host with that of the rearing environment (water and feed), whereas the life stage appeared to be the main factor affecting the structure of gut microbiota. These results are in agreement with previous findings from Llewellyn et al. (2016), who studied 96 wild-caught individuals of Atlantic salmon with different age and habitats and observed grouping of their intestinal bacterial communities based on the lifecycle stage. In addition, Lokesh et al. (2019), reported stage specific microbial enrichment in intestinal mucosa of the same host species (samples from embryonic stages up to 80 weeks post hatch). Similar stage specific signatures have also been reported across development in Sparus aurata (Nikouli et al., 2019), Danio rerio (Stephens et al., 2016), and Gadus morhua (Bakke et al., 2015) suporting further that the life stage seems to be the primary force shaping gut microbiota in juveniles’ stages of fish. The change in microbiota with life stage can be due to both host-microbe (e.g., development in morphology and immune system) and microbe-microbe interactions (mutualism, commensalism and competition). The significance of these factors is, however, still not known.
Proteobacteria, Firmicutes, Actinobacteria, and Bacteroidetes were the dominant bacterial phyla detected in the host samples for both dietary treatments in our study. These bacterial phyla seem to characterize the bacterial communities in individuals of Atlantic salmon at the freshwater life cycle stages (Llewellyn et al., 2016). These bacterial phyla are also commonly found in the gut bacterial communities of both saltwater and freshwater fish species (Hansen and Olafsen, 1999; Nayak, 2010; McDonald et al., 2012; Navarrete et al., 2013; Bolnick et al., 2014; Kormas et al., 2014; Llewellyn et al., 2016; Stephens et al., 2016; Dehler et al., 2017; Tarnecki et al., 2017; Booman et al., 2018; Lokesh et al., 2019; Nikouli et al., 2018, 2019).
Despite the fact that the two experimental feeds contained almost entirely Firmicutes, the increase in relative abundance of Firmicutes in samples after the onset of feeding was not solely due to feed specific OTUs. It should also be noted that 26.4% of the bacterial representatives detected on fertilized eggs (EG) were not detected in the water of the incubation tank (WST). This support the view that the microbial communities of fish eggs may be vertically transmitted from their parents or horizontally from their breading tank (Hansen and Olafsen, 1989; Nikouli et al., 2019).
In agreement with previous studies (Schmidt et al., 2016; Lokesh et al., 2019; Nikouli et al., 2019) the observed species richness in water samples was always an order of magnitude higher than the richness of the host samples. Bacterial communities in rearing water did not show major shifts during the experiment. OTU0001 dominated at all time points, with closest relative the bacterial species Polynucleobacter necessaries. This species is commonly found in freshwater samples and it can contribute to the catabolism of urea and reduction of nitrate (Boscaro et al., 2013). The dominant bacterial species in Atlantic salmon samples are related with bacterial species from various habitats. The dominant OTU on fertilized eggs (OTU0011) was classified within the Methylotenera genus (β-Proteobacteria) and has previously been detected in fertilized eggs of the same host species by Lokesh et al. (2019). This genus consists of methylotrophic species that use methylamine as sole carbon, energy and nitrogen source (Kalyuzhnaya et al., 2006) and seem to be associated with RAS systems (Minich et al., 2020). The dominant OTU at the YS stage (OTU0013), seems to be related with Delftia acidovorans (β-Proteobacteria). Species of the genus Delftia are obligate anaerobes, organotrophic and non-fermentative organisms (Wen et al., 1999). They have previously been detected in the gut of healthy individuals of Epinephelus coioides (Sun et al., 2009), Oncorhynchus mykiss (Navarrete et al., 2012) and Sparus aurata (Kormas et al., 2014; Nikouli et al., 2018) and S. salar (Gajardo et al., 2016).
Just before onset on feeding (D0), the dominant OTU (OT U0009) showed similarities with the species Iodobacter fluviatilis of the Chitinibacteraceae (β-Proteobacteria) family. Species of this genus have been recorded mainly in sediment and water samples (Ryall and Moss, 1975; Wynn-Williams, 1983; Logan, 1989). Their presence on fish skin (Oncorhynchus mykiss and Salmo trutta) has been associated with skin lesions (Carbajal-González et al., 2011). However, they have previously been detected in high relative abundance in healthy Coreius guichenoti individuals (Li et al., 2016) whereas the present study reports the presence of this bacterial species in Atlantic salmon gut microbiota for the first time.
After first feeding, although not statistically significant differences were found between the bacterial communities in the hindgut samples of the different life stages, each stage was characterized by different dominant OTUs. Moreover, gut bacterial communities differed also between dietary treatments regarding their dominant bacterial species (OTU). Chitinibacteraceae, the dominant bacterial family on D0 (with relative abundance 32.3%), was detected in ∼50× lower relative abundance (≤0.6%) in the rest of the samples. At D35 and D65 in FD treatment, the dominant OTUs (OTU0017 and OTU0070, classified as Pseudomonas viridiflava and Janthinobacterium agaricidamnosum, respectively), are described as plant (Alivizatos, 1986; Alimi et al., 2011; Taylor et al., 2011; Sarris et al., 2012) and mushroom pathogens (Lincoln et al., 1999; Graupner et al., 2015). According to recent findings, Janthinobacterium lividum (β-Proteobacteria) exhibits antimicrobial activity against multidrug resistant bacteria of clinical and environmental origin, such as Enterococci and Enterobacteriaceae (Baricz et al., 2018). Its presence in the gastrointestinal bacterial communities of Atlantic salmon, may have probiotic activity.
At D35 and D65, samples from the VD dietary treatment, were dominated by OTU0005, with closest relative Cloacibacterium normanense (Bacteroidetes). This OTU was also dominant at D93 in FD treatment. According to the literature, this species is frequently present in sewage treatment plants (Benedict and Carlson, 1971; Güde, 1980) where it contributes in the decomposition of complex organic compounds (Bernardet et al., 2002). Similar processes may take place in the intestinal system of Atlantic salmon at D35V, D65V, and D93F. The dominant OTU at D93 (OTU0004), also dominant in both provided feeds (FD, VD), was affiliated with Weissella cibaria (Firmicutes). This bacterial species belongs to the lactic acid bacteria, and has antimicrobial activity in the intestinal system of other fish species (Mouriño et al., 2016). Other Weissella spp. have been found in gut of Oncorynchus mykiss (Lyons et al., 2017; Mortezaei et al., 2020) and Atlantic salmon (Reveco et al., 2014; Godoy et al., 2015; Lokesh et al., 2019). It is worth noting that beside OTU0004, also OTU0013 and OTU0017 are associated with probiotic bacterial species (detected in all time points studied here, from EG to D93, independently of the dietary treatment). This observation suggests a co-evolutionary relationship of these bacterial species with the host studied here, and a possible specialized function in the hosts intestinal system.
Conclusion
The present study evaluated the effect of total fish oil replacement by a blend of terrestrial vegetable oils (rapeseed, linseed and palm oils) in the feed on the colonization and the bacterial succession in first feeding of Atlantic salmon, up to 93 days dpff. We demonstrated that feeding on either fish oil or terrestrial vegetable oil diets, did not result in significant differences in the intestinal gut microbiota and growth performance parameters (wet weight and total length). On the contrary, the composition of gut microbiota changed with age, and each stage was characterized by different dominant bacteria. These OTUs are related to species that may have probiotic activity to the host. Finally, this study revealed the occurrence of a core microbiota independent of the studied life stages and diet. These findings indicate that total fish oil replacement by terrestrial vegetable oils is feasible and can lead in low cost formulated feeds. Future work should aim on understanding the functional role of the detected core community which could lead in further feed, growth performance and host health optimization.
Data Availability Statement
The datasets presented in this study can be found in online repositories. The names of the repository/repositories and accession number(s) can be found below: https://www.ncbi.nlm.nih.gov/, PRJNA520982.
Ethics Statement
The animal study was reviewed and approved by the Animal Ethics and Welfare Committee of the Norwegian University of Science and Technology (case no. 16/10070). The study was carried out within the Norwegian Animal Welfare Act guidelines, in accordance with EU regulation (EC Directive 2010/63/EU).
Author Contributions
EN, KK, YO, IB, and OV: methodology. EN: formal analysis. EN and KK: data curation and writing—original draft preparation. EN, KK, YJ, YO, IB, and OV: writing—review and editing. OV: supervision. All authors contributed to the article and approved the submitted version.
Funding
EN was awarded with a visiting Ph.D. student fellowship by State Scholarships Foundation/IKY-Greece under the program “Scholarships of IKY in the Marine and Inland Management of Water Resources” and was co-funded by EEA Grants—Financial Mechanism 2009–2014 (85%) and the General Secretariat for Investments and Development (15%). YJ internal IBI funding, China.
Conflict of Interest
The authors declare that the research was conducted in the absence of any commercial or financial relationships that could be construed as a potential conflict of interest.
Acknowledgments
We would like to thank Jostein Ervik for rearing the fish, AquaGen AS for providing the fish, and Mari-Ann Østensen for assistance with the sampling and for her valuable suggestions during the planning and development of this study.
Supplementary Material
The Supplementary Material for this article can be found online at: https://www.frontiersin.org/articles/10.3389/fmars.2021.665576/full#supplementary-material
Footnotes
References
Alimi, M., Rahimian, H., Hasanzadeh, N., Taghinasab, M., Ahmadikhah, A., Heydari, A., et al. (2011). First detection of Pseudomonas viridiflava, the causal agent of blossom blight in apple by using specific designed primers. Afr. J. Microbiol. Res. 5, 4708–4713.
Alivizatos, A. S. (1986). Tomato pith necrosis caused by Pseudomonas viridiflava. Annales de l’Institut Phytopathologique Benaki. 15, 41–47.
Bakke, I., Coward, E., Andersen, T., and Vadstein, O. (2015). Selection in the host structures the microbiota associated with developing cod larvae (Gadus morhua). Environ. Microbiol. 17, 3914–3924. doi: 10.1111/1462-2920.12888
Bakke, I., Skjermo, J., Vo, T. A., and Vadstein, O. (2013). Live feed is not a major determinant of the microbiota associated with cod larvae (Gadus morhua). Environ. Microbiol. Rep. 5, 537–548. doi: 10.1111/1758-2229.12042
Baricz, A., Teban, A., Chiriac, C. M., Szekeres, E., Farkas, A., Nica, M., et al. (2018). Investigating the potential use of an Antarctic variant of Janthinobacterium lividum for tackling antimicrobial resistance in a One Health approach. Sci. Rep. 8:15272.
Benedict, R. G., and Carlson, D. A. (1971). Aerobic heterotrophic bacteria in activated sludge. Water Res. 5, 1023–1030. doi: 10.1016/0043-1354(71)90036-4
Bernardet, J.-F., Nakagawa, Y., and Holmes, B. Subcommittee on the taxonomy of Flavobacterium and Cytophaga-like bacteria of the international committee on systematics of prokaryotes (2002). Proposed minimal standards for describing new taxa of the family Flavobacteriaceae and emended description of the family. Int. J. Syst. Evol. Microbiol. 52, 1049–1070. doi: 10.1099/00207713-52-3-1049
Beveridge, M. C. M., Sikdar, P. K., Frerichs, G. N., and Millar, S. (1991). The ingestion of bacteria in suspension by the common carp Cyprinus carpio L. J. Fish Biol. 39, 825–831. doi: 10.1111/j.1095-8649.1991.tb04412.x
Bolnick, D. I., Snowberg, L. K., Hirsch, P. E., Lauber, C. L., Knight, R., Caporaso, J. G., et al. (2014). Individuals’ diet diversity influences gut microbial diversity in two freshwater fish (threespine stickleback and Eurasian perch). Ecol. Lett. 17, 979–987. doi: 10.1111/ele.12301
Booman, M., Forster, I., Vederas, J. C., Groman, D. B., and Jones, S. R. M. (2018). Soybean meal-induced enteritis in Atlantic salmon (Salmo salar) and Chinook salmon (Oncorhynchus tshawytscha) but not in pink salmon (O. gorbuscha). Aquaculture 483, 238–243. doi: 10.1016/j.aquaculture.2017.10.025
Boscaro, V., Felletti, M., Vannini, C., Ackerman, M. S., Chain, P. S. G., Malfatti, S., et al. (2013). Polynucleobacter necessarius, a model for genome reduction in both free-living and symbiotic bacteria. Proc. Natl. Acad. Sci. U.S.A. 110, 18590–18595. doi: 10.1073/pnas.1316687110
Califano, G., Castanho, S., Soares, F., Ribeiro, L., Cox, C. J., Mata, L., et al. (2017). Molecular taxonomic profiling of bacterial communities in a gilthead sea bream (Sparus aurata) hatchery. Front. Microbiol. 8:204. doi: 10.3389/fmicb.2017.00204
Carbajal-González, M. T., Fregeneda-Grandes, J. M., Suárez-Ramos, S., Rodríguez Cadenas, F., and Aller-Gancedo, J. M. (2011). Bacterial skin flora variation and in vitro inhibitory activity against Saprolegnia parasitica in brown and rainbow trout. Dis. Aquat. Organ. 96, 125–135. doi: 10.3354/dao02391
Clarkson, M., Migaud, H., Metochis, C., Vera, L. M., Leeming, D., Tocher, D. R., et al. (2017). Early nutritional intervention can improve utilisation of vegetable-based diets in diploid and triploid Atlantic salmon (Salmo salar L.). Br. J. Nutr. 118, 17–29. doi: 10.1017/s0007114517001842
Dehler, C. E., Secombes, C. J., and Martin, S. A. M. (2017). Environmental and physiological factors shape the gut microbiota of Atlantic salmon parr (Salmo salar L.). Aquaculture 467, 149–157. doi: 10.1016/j.aquaculture.2016.07.017
Egerton, S., Culloty, S., Whooley, J., Stanton, C., and Ross, R. P. (2018). The gut microbiota of Marine fish. Front. Microbiol. 9:873. doi: 10.3389/fmicb.2018.00873
Egerton, S., Wan, A., Murphy, K., Collins, F., Ahern, G., Sugrue, I., et al. (2020). Replacing fishmeal with plant protein in Atlantic salmon (Salmo salar) diets by supplementation with fish protein hydrolysate. Sci. Rep. 10:4194. doi: 10.1038/s41598-020-60325-7
Eichmiller, J. J., Hamilton, M. J., Staley, C., Sadowsky, M. J., and Sorensen, P. W. (2016). Environment shapes the fecal microbiome of invasive carp species. Microbiome 4:44.
FAO (2004). Cultured Aquatic Species Information Programme. Salmo salar. Cultured Aquatic Species Information Programme. Rome: FAO Fisheries and Aquaculture Division. Text by Jones, M.
Gajardo, K., Jaramillo-Torres, A., Kortner, T. M., Merrifield, D. L., Tinsley, J., Bakke, A. M., et al. (2017). Alternative protein sources in the diet modulate microbiota and functionality in the distal intestine of Atlantic Salmon (Salmo salar). Appl. Environ. Microbiol. 83:e02615-16. doi: 10.1128/AEM.02615-16
Gajardo, K., Rodiles, A., Kortner, T. M., Krogdahl, Å., Bakke, A. M., Merrifield, D. L., et al. (2016). A high-resolution map of the gut microbiota in Atlantic salmon (Salmo salar): a basis for comparative gut microbial research. Sci. Rep. 6:30893.
Godoy, F. A., Miranda, C. D., Wittwer, G. D., Aranda, C. P., and Calderón, R. (2015). High variability of levels of Aliivibrio and lactic acid bacteria in the intestinal microbiota of farmed Atlantic salmon Salmo salar L. Ann. Microbiol. 65, 2343–2353. doi: 10.1007/s13213-015-1076-3
Graupner, K., Lackner, G., and Hertweck, C. (2015). Genome sequence of mushroom soft-rot pathogen Janthinobacterium agaricidamnosum. Genome Announc 3:e00277-15. doi: 10.1128/genomeA.00277-15
Green, T. J., Smullen, R., and Barnes, A. C. (2013). Dietary soybean protein concentrate-induced intestinal disorder in marine farmed Atlantic salmon, Salmo salar is associated with alterations in gut microbiota. Vet. Microbiol. 166, 286–292. doi: 10.1016/j.vetmic.2013.05.009
Güde, H. (1980). Occurrence of Cytophagas in sewage plants. Appl. Environ. Microbiol. 39, 756–763. doi: 10.1128/aem.39.4.756-763.1980
Hammer, O., Harper, D. A. T., and Ryan, P. D. (2001). PAST: paleontological statistics software package for education and data analysis. Palaeontol. Electronica 4:9.
Hansen, G. H., and Olafsen, J. A. (1989). Bacterial colonization of Cod (Gadus morhua L.) and Halibut (Hippoglossus hippoglossus) eggs in marine aquaculture. Appl. Environ. Microbiol. 55, 1435–1446. doi: 10.1128/aem.55.6.1435-1446.1989
Hansen, G. H., and Olafsen, J. A. (1999). Bacterial interactions in early life stages of marine cold water fish. Microb. Ecol. 38, 1–26. doi: 10.1007/s002489900158
Hardy, R. W. (2010). Utilization of plant proteins in fish diets: effects of global demand and supplies of fishmeal. Aquac. Res. 41, 770–776. doi: 10.1111/j.1365-2109.2009.02349.x
Ingerslev, H. C., Jørgensen, L. V. G., Strude, M. L., Larsen, N., Dalsgaard, I., and Boye, M. (2014a). The development of the gut microbiota in rainbow trout is affected by first feeding and diet type. Aquaculture 424, 24–34. doi: 10.1016/j.aquaculture.2013.12.032
Ingerslev, H. C., Strude, M. L., Jørgensen, L. V. G., Dalsgaard, I., Boye, M., and Madsen, L. (2014b). Diet type dictates the gut microbiota and the immune response against Yersinia ruckeri in rainbow trout (Oncorhynchus mykiss). Fish Shellfish Immunol. 40, 624–633. doi: 10.1016/j.fsi.2014.08.021
International Fishmeal and Oil Manufacturers Association [IFOMA] (2001). Advantages of Using Fishmeal in Animal Feeds. Sociedad Nacional de Pesqueria. Available online at: http://fis.com/snp/ (accessed October 11, 2020).
Jin, Y., Olsen, R. E., Østensen, M.-A., Gillard, G. B., Li, K., Harvey, T. N., et al. (2019). Transcriptional regulation of lipid metabolism when salmon fry switches from endogenous to exogenous feeding. Aquaculture 503, 422–429. doi: 10.1016/j.aquaculture.2018.12.089
Kalyuzhnaya, M. G., Bowerman, S., Lara, J. C., Lidstrom, M. E., and Chistoserdova, L. (2006). Methylotenera mobilis gen. nov., sp. nov., an obligately methylamine-utilizing bacterium within the family Methylophilaceae. Int. J. Syst. Evol. Microbiol. 56, 2819–2823. doi: 10.1099/ijs.0.64191-0
Kashinskaya, E. N., Simonov, E. P., Kabilov, M. R., Izvekova, G. I., Andree, K. B., and Solovyev, M. M. (2018). Diet and other environmental factors shape the bacterial communities of fish gut in an eutrophic lake. J. Appl. Microbiol. 125, 1626–1641. doi: 10.1111/jam.14064
Klindworth, A., Pruesse, E., Schweer, T., Peplies, J., Quast, C., Horn, M., et al. (2013). Evaluation of general 16S ribosomal RNA gene PCR primers for classical and next-generation sequencing-based diversity studies. Nucleic Acids Res. 41:e1. doi: 10.1093/nar/gks808
Kormas, K. A., Meziti, A., Mente, E., and Frentzos, A. (2014). Dietary differences are reflected on the gut prokaryotic community structure of wild and commercially reared sea bream (Sparus aurata). MicrobiologyOpen 3, 718–728. doi: 10.1002/mbo3.202
Li, T., Long, M., Ji, C., Shen, Z., Gatesoupe, F.-J., Zhang, X., et al. (2016). Alterations of the gut microbiome of largemouth bronze gudgeon (Coreius guichenoti) suffering from furunculosis. Sci. Rep. 6:30606.
Lincoln, S. P., Fermor, T. R., and Tindall, B. J. (1999). Janthinobacterium agaricidamnosum sp. nov., a soft rot pathogen of Agaricus bisporus. Int. J. Syst. Bacteriol. 49, 1577–1589. doi: 10.1099/00207713-49-4-1577
Llewellyn, M. S., McGinnity, P., Dionne, M., Letourneau, J., Thonier, F., Carvalho, G. R., et al. (2016). The biogeography of the atlantic salmon (Salmo salar) gut microbiome. ISME J. 10, 1280–1284. doi: 10.1038/ismej.2015.189
Logan, N. A. (1989). Numerical taxonomy of violet-pigmented, gram-negative bacteria and description of Iodobacter fluviatile gen. nov., comb. nov. Int. J. Syst. Evol. Microbiol. 39, 450–456. doi: 10.1099/00207713-39-4-450
Lokesh, J., Kiron, V., Sipkema, D., Fernandes, J. M. O., and Moum, T. (2019). Succession of embryonic and the intestinal bacterial communities of Atlantic salmon (Salmo salar) reveals stage-specific microbial signatures. MicrobiologyOpen 8:e00672. doi: 10.1002/mbo3.672
Lowe, W. H., Martin, T. E., Skelly, D. K., and Woods, H. A. (2021). Metamorphosis in an era of increasing climate variability. Trends Ecol. Evol. 36, 360–375. doi: 10.1016/j.tree.2020.11.012
Lyons, P. P., Turnbull, J. F., Dawson, K. A., and Crumlish, M. (2017). Effects of low-level dietary microalgae supplementation on the distal intestinal microbiome of farmed rainbow trout Oncorhynchus mykiss (Walbaum). Aquac. Res. 48, 2438–2452. doi: 10.1111/are.13080
McDonald, R., Schreier, H. J., and Watts, J. E. M. (2012). Phylogenetic analysis of microbial communities in different regions of the gastrointestinal tract in Panaque nigrolineatus, a wood-eating fish. PLoS One 7:e48018. doi: 10.1371/journal.pone.0048018
Minich, J. J., Poore, G. D., Jantawongsri, K., Johnston, C., Bowie, K., Bowman, J., et al. (2020). Microbial ecology of Atlantic salmon (Salmo salar) hatcheries: impacts of the built environment on fish mucosal microbiota. Appl. Environ. Microbiol. 86, e411–e420. doi: 10.1128/AEM.00411-20
Mortezaei, F., Royan, M., Allaf Noveirian, H., Babakhani, A., Alaie Kordghashlaghi, H., and Balcázar, J. L. (2020). In vitro assessment of potential probiotic characteristics of indigenous Lactococcus lactis and Weissella oryzae isolates from rainbow trout (Oncorhynchus mykiss Walbaum). J. Appl. Microbiol. 129, 1004–1019. doi: 10.1111/jam.14652
Mouriño, J. L. P., Pereira, G., Vieira, F., Jatobá, A. B., Ushizima, T. T., Silva, B. C., et al. (2016). Isolation of probiotic bacteria from the hybrid South American catfish Pseudoplatystoma reticulatum × Pseudoplatystoma corruscans (Siluriformes: Pimelodidae): a haematological approach. Aquac. Rep. 3, 166–171. doi: 10.1016/j.aqrep.2016.03.001
Navarrete, P., Fuentes, P., De la Fuente, L., Barros, L., Magne, F., Opazo, R., et al. (2013). Short-term effects of dietary soybean meal and lactic acid bacteria on the intestinal morphology and microbiota of Atlantic salmon (Salmo salar). Aquac. Nutr. 19, 827–836. doi: 10.1111/anu.12047
Navarrete, P., Magne, F., Araneda, C., Fuentes, P., Barros, L., Opazo, R., et al. (2012). PCR-TTGE analysis of 16S rRNA from rainbow trout (Oncorhynchus mykiss) gut microbiota reveals host-specific communities of active bacteria. PLoS One 7:e31335. doi: 10.1371/journal.pone.0031335
Nayak, S. K. (2010). Role of gastrointestinal microbiota in fish. Aquac. Res. 41, 1553–1573. doi: 10.1111/j.1365-2109.2010.02546.x
Nikouli, E., Meziti, A., Antonopoulou, E., Mente, E., and Kormas, K. A. (2018). Gut bacterial communities in geographically distant populations of Farmed Sea Bream (Sparus aurata) and Sea Bass (Dicentrarchus labrax). Microorganisms. 6:92. doi: 10.3390/microorganisms6030092
Nikouli, E., Meziti, A., Antonopoulou, E., Mente, E., and Kormas, K. A. (2019). Host-associated bacterial succession during the early embryonic stages and first feeding in Farmed Gilthead Sea Bream (Sparus aurata). Genes 10:483. doi: 10.3390/genes10070483
Olafsen, J. A. (1984). “Ingestion of bacteria by cod (Gadus morhua L.) larvae,” in The Propagation of Cod Gadus morhua L, eds E. DahI, D. S. Danielssen, E. Moksness, and P. Solemdal (Bergen: Institute of Marine Research), 627–643.
Quast, C., Pruesse, E., Yilmaz, P., Gerken, J., Schweer, T., Yarza, P., et al. (2013). The SILVA ribosomal RNA gene database project: improved data processing and web-based tools. Nucleic Acids Res. 41, D590–D596.
Reveco, F. E., Øverland, M., Romarheim, O. H., and Mydland, L. T. (2014). Intestinal bacterial community structure differs between healthy and inflamed intestines in Atlantic salmon (Salmo salar L.). Aquaculture 42, 262–269. doi: 10.1016/j.aquaculture.2013.11.007
Ringø, E., Zhou, Z., Vecino, J. L. G., Wadsworth, S., Romero, J., and Krogdahl, Å, et al. (2016). Effect of dietary components on the gut microbiota of aquatic animals. A never-ending story? Aquac. Nutr. 22, 219–282. doi: 10.1111/anu.12346
Rodriguez-R, L. M., and Konstantinidis, K. T. (2016). A collection of functions for microbial ecology and other applications of genomics and metagenomics. Companion package for the Enveomics Collection. PeerJ. Prepr. 4:e1900v1.
Ryall, C., and Moss, M. O. (1975). Selective media for the enumeration of Chromobacterium spp. in soil and water. J. Appl. Bacteriol. 38, 53–59. doi: 10.1111/j.1365-2672.1975.tb00500.x
Sarris, P. F., Trantas, E. A., Mpalantinaki, E., Ververidis, F., and Goumas, D. E. (2012). Pseudomonas viridiflava, a multi host plant pathogen with significant genetic variation at the molecular level. PLoS One 7:e36090. doi: 10.1371/journal.pone.0036090
Schloss, P. D., Gevers, D., and Westcott, S. L. (2011). Reducing the effects of PCR amplification and sequencing artifacts on 16S rRNA-based studies. PLoS One 6:e27310. doi: 10.1371/journal.pone.0027310
Schloss, P. D., Westcott, S. L., Ryabin, T., Hall, J. R., Hartmann, M., Hollister, E. B., et al. (2009). Introducing mothur: open-source, platform-independent, community-supported software for describing and comparing microbial communities. Appl. Environ. Microbiol. 75, 7537–7541. doi: 10.1128/aem.01541-09
Schmidt, V., Amaral-Zettler, L., Davidson, J., Summerfelt, S., and Good, C. (2016). Influence of fishmeal-free diets on microbial communities in Atlantic Salmon (Salmo salar) recirculation aquaculture systems. Appl. Environ. Microbiol. 82, 4470–4481. doi: 10.1128/aem.00902-16
Scott, K. P., Gratz, S. W., Sheridan, P. O., Flint, H. J., and Duncan, S. H. (2013). The influence of diet on the gut microbiota. Pharmacol. Res. 69, 52–60.
Stephens, W. Z., Burns, A. R., Stagaman, K., Wong, S., Rawls, J. F., Guillemin, K., et al. (2016). The composition of the zebrafish intestinal microbial community varies across development. ISME J. 10, 644–654. doi: 10.1038/ismej.2015.140
Sun, Y., Yang, H., Ling, Z., Chang, J., and Ye, J. (2009). Gut microbiota of fast and slow growing grouper. Afr. J. Microbiol. Res. 3, 713–720.
Tarnecki, A. M., Burgos, F. A., Ray, C. L., and Arias, C. R. (2017). Fish intestinal microbiome: diversity and symbiosis unravelled by metagenomics. J. Appl. Microbiol. 123, 2–17. doi: 10.1111/jam.13415
Taylor, R. K., Romberg, M. K., and Alexander, B. J. R. (2011). A bacterial disease of hellebore caused by Pseudomonas viridiflava in New Zealand. Australas. Plant Dis. Notes 6, 28–29. doi: 10.1007/s13314-011-0010-1
Turchini, G. M., Ng, W.-K., and Tocher, D. R. (2010). Fish Oil Replacement and Alternative Lipid Sources in Aquaculture Feeds. Boca Raton, FL: CRC Press.
Wang, Y., Wang, K., Huang, L., Dong, P., Wang, S., Chen, H., et al. (2020). Fine-scale succession patterns and assembly mechanisms of bacterial community of Litopenaeus vannamei larvae across the developmental cycle. Microbiome 8:106.
Wen, A., Fegan, M., Hayward, C., Chakraborty, S., and Sly, L. I. (1999). Phylogenetic relationships among members of the Comamonadaceae, and description of Delftia acidovorans (den Dooren de Jong 1926 and Tamaoka et al. 1987) gen. nov., comb. nov. Int. J. Syst. Bacteriol. 49, 567–576. doi: 10.1099/00207713-49-2-567
Wynn-Williams, D. D. (1983). Distribution and characteristics of Chromobacterium in the maritime and sub-antarctic. Polar Biol. 2, 101–108. doi: 10.1007/bf00303175
Xiao, F., Zhu, W., Yu, Y., He, Z., Wu, B., Wang, C., et al. (2021). Host development overwhelms environmental dispersal in governing the ecological succession of zebrafish gut microbiota. NPJ Biofilms Microbiome. 7:5.
Keywords: Salmo salar, larvae, gut microbiota, dietary intervention, fish oil replacement
Citation: Nikouli E, Kormas KA, Jin Y, Olsen Y, Bakke I and Vadstein O (2021) Dietary Lipid Effects on Gut Microbiota of First Feeding Atlantic Salmon (Salmo salar L.). Front. Mar. Sci. 8:665576. doi: 10.3389/fmars.2021.665576
Received: 08 February 2021; Accepted: 21 April 2021;
Published: 20 May 2021.
Edited by:
Fotini Kokou, Wageningen University and Research, NetherlandsReviewed by:
Christina Pavloudi, Hellenic Centre for Marine Research (HCMR), GreeceShruti Gupta, LetSea, Norway
Copyright © 2021 Nikouli, Kormas, Jin, Olsen, Bakke and Vadstein. This is an open-access article distributed under the terms of the Creative Commons Attribution License (CC BY). The use, distribution or reproduction in other forums is permitted, provided the original author(s) and the copyright owner(s) are credited and that the original publication in this journal is cited, in accordance with accepted academic practice. No use, distribution or reproduction is permitted which does not comply with these terms.
*Correspondence: Eleni Nikouli, elnikoul@uth.gr; Olav Vadstein, olav.vadstein@ntnu.no