- 1School of Natural Resources and Environment, University of Florida, Gainesville, FL, United States
- 2Soil and Water Sciences Department, University of Florida, Gainesville, FL, United States
- 3Nature Coast Biological Station, Institute of Food and Agriculture Sciences, University of Florida, Cedar Key, FL, United States
- 4College of Marine Science, University of South Florida, St. Petersburg, FL, United States
Growing fragments of corals in nurseries and outplanting them to supplement declining natural populations have gained significant traction worldwide. In the Caribbean, for example, this approach provides colonies of Acropora cervicornis with minimal impacts to existing wild colonies. Given the impetus to scale up production to augment limited natural recovery, managers and researchers should consider how the design and location of the nurseries affect the growth of different genotypes of corals and the effort required for maintenance. To elucidate such influences, we grew fragments of different genotypes (five varieties) on differing structures (trees and frames) at two depths (6–8 and 16–18 m). The sum of the lengths of all branches or total linear extensions (TLEs) and accumulation of biofouling were measured over 198 days from May to December 2016 to assess the growth of fragments and the effort required to maintain nurseries. TLEs for all fragments increased linearly throughout the incubation period. Mean daily incremental growth rates varied among the genotypes, with one genotype growing significantly faster than all others, two genotypes growing at intermediate rates, and two genotypes growing more slowly. Mean daily incremental growth rates were higher for all genotypes suspended from vertical frames at both sites, and mean daily incremental growth rates were higher for all fragments held on both types of nurseries in deeper water. If linear growth continued, a fragment of the fastest growing genotype held on a frame in deeper water was estimated to increase the sum of the length of all its branches by an average of 88 cm y–1, which was over two times higher than the estimated mean annual growth rate for a fragment of the slowest growing genotype held on a tree in shallow water. Nurseries in deeper water had significantly less biofouling and appeared to be buffered against daily fluctuations in temperature. Overall, the results demonstrated that increased production and reduced maintenance can result from considering the genotype of fragments to be cultured and the design and location of nurseries.
Introduction
Historically, the staghorn coral Acropora cervicornis was a dominant foundation species that provided much of the rugosity and structural complexity on Caribbean coral reefs (Gilmore and Hall, 1976; Tunnicliffe, 1981; Aronson and Precht, 1997; Alvarez-Filip et al., 2009). This species dominated forereef and shallow spur and groove areas throughout much of the Caribbean because of its rapid growth rates and capacity for asexual reproduction (Tunnicliffe, 1981; Aronson and Precht, 1997). However, the abundance of staghorn corals has declined substantially throughout the Caribbean, with some areas losing more than 97% of historical cover over the past five decades (Acropora Biological Review Team, 2005). This precipitous decline in Caribbean acroporids resulted from white band disease (WBD), which was first observed in 1976 (Gladfelter, 1982), followed by cascading effects on food webs arising from the demise of the long-spined sea urchin Diadema antillarum in the early 1980s and damage caused by hurricanes (Hughes and Connell, 1999; Williams and Miller, 2005). Although modest recovery of staghorn corals has been reported in a few areas of the Caribbean, a rapid natural recovery has been inhibited by the persistence of WBD, more frequent and severe bleaching events, and competition with macroalgae due to insufficient grazing by the reduced numbers of D. antillarum (Aronson and Precht, 2001; Precht et al., 2002).
In an attempt to enhance remaining wild populations and regenerate degraded reefs, acroporid nurseries have proliferated throughout the Caribbean in the last two decades (Edwards and Gomez, 2007; Johnson et al., 2011; National Marine Fisheries Service, 2015). Initial nurseries in the early 2000s employed fragments of coral attached to concrete blocks or polypropylene lines (Johnson et al., 2011; Young and Schopmeyer, 2012). New techniques reduced mortality and improved growth rates by placing nurseries in sheltered environments and raising corals above the substrate (Bowden-Kerby, 2001; Bowden-Kerby et al., 2005; Griffin et al., 2012). Nurseries that held fragments in the water column minimized direct predation by corallivorous snails, Coralliophila abbreviata, and fireworms, Hermodice carunculata and increased access to food (Young and Schopmeyer, 2012). Reducing the damage to coral tissue caused by predation also mitigated transmission of WBD and other waterborne pathogens (Sussman et al., 2003; Gignoux-Wolfsohn et al., 2012). Today, identifying and propagating genetically distinct lineages of corals has become standard practice for nurseries (Johnson et al., 2011). Furthermore, nurseries now produce multiple fragments of coral for outplanting from small portions of wild colonies, and they act as a repository of local genets in the event donor colonies are lost (Schopmeyer et al., 2012). Overall, enhancing genetic diversity in outplants represents a means of improving spatial connectivity that is a critical component of regional restoration (Lirman and Schopmeyer, 2016). At the regional scale, staghorn coral populations have become severely isolated, so propagation of multiple genets improves the likelihood of restoring the successful in situ sexual reproduction that fostered genetic diversity in historical populations (Drury et al., 2017).
Nurseries for corals serve as crucial intermediary steps between collection of fragments from wild colonies and outplanting (Johnson et al., 2011). Despite the increased use of nurseries in the Caribbean, there is currently a dearth of information regarding the influences of genotypes, designs of nurseries, and depth of deployment on the growth of the fragments and the need for maintenance (Young and Schopmeyer, 2012; Schopmeyer et al., 2017). For example, PVC trees have been one of the most popular structures for suspending coral fragments in the water column (Nedimyer et al., 2011). However, restoration after the grounding of the T/V Magara in Puerto Rico suggested that planar, vertical frames placed in deeper water (15–18 m) offered significant advantages (Griffin et al., 2012). Specifically, the fragments on frames in deeper water grew significantly faster and the frames accumulated biofouling at a slower rate than had been reported elsewhere (Quinn and Kojis, 2006; Lohr et al., 2015; Schopmeyer et al., 2017). In addition, experimental work off Little Cayman Island indicated that splitting coral fragments could increase the numbers available for outplanting dramatically; however, limited replication in that study precluded evaluation of variation in growth rates among genotypes (Lohr et al., 2015).
Although information can be compared across studies, to our knowledge, a simultaneous comparison of growth rates for different genotypes attached to nurseries with different designs that were deployed at different depths has not been reported. This study used a field experiment conducted off Little Cayman Island to address this gap in knowledge and inform best practices for culturing fragments of A. cervicornis.
Materials and Methods
Experimental Design: Sites, Nurseries, and Genotypes
Nurseries were deployed over the course of 2 days at the end of May, and measurements were collected for 198 days from June 2016 to December 2016 at two sites along the northern coast of Little Cayman Island (Figures 1A,B). The shallow site was located approximately 150 m from the shoreline in 6–8 m of water on a hardpan plain between the fringing backreef and the beginning of the spur and groove formation (Figure 1C). This site was characterized by sparse, small coral heads and gorgonians, and it lacked high densities of scleractinian corals or large sponges. Mixed schools of ocean surgeonfish, Acanthurus tractus, and doctorfish, Acanthurus chirurgus, frequently grazed on the nurseries. The deep site was established approximately 100 m north of the shallow site in 16–18 m of water (Figure 1C). This site was located in 50 m of sand and rubble at the deeper terminus of a shallow groove in a spur and groove formation. This sandy area was bordered by reef spurs to the east and west and a sandy plain to the north. The adjacent spurs were dominated by corals in the genera Orbicella, Pseudodiploria, and Porites, with Diploria and Agaricia also found in relatively high abundance. The area of sand and rubble was mostly barren, with occasional small fish in the genus Labridae found near the substrate. Several fish species were seen along the reef spurs, and large schools of creole wrasse, Clepticus parrae, were observed in the nearby water column.
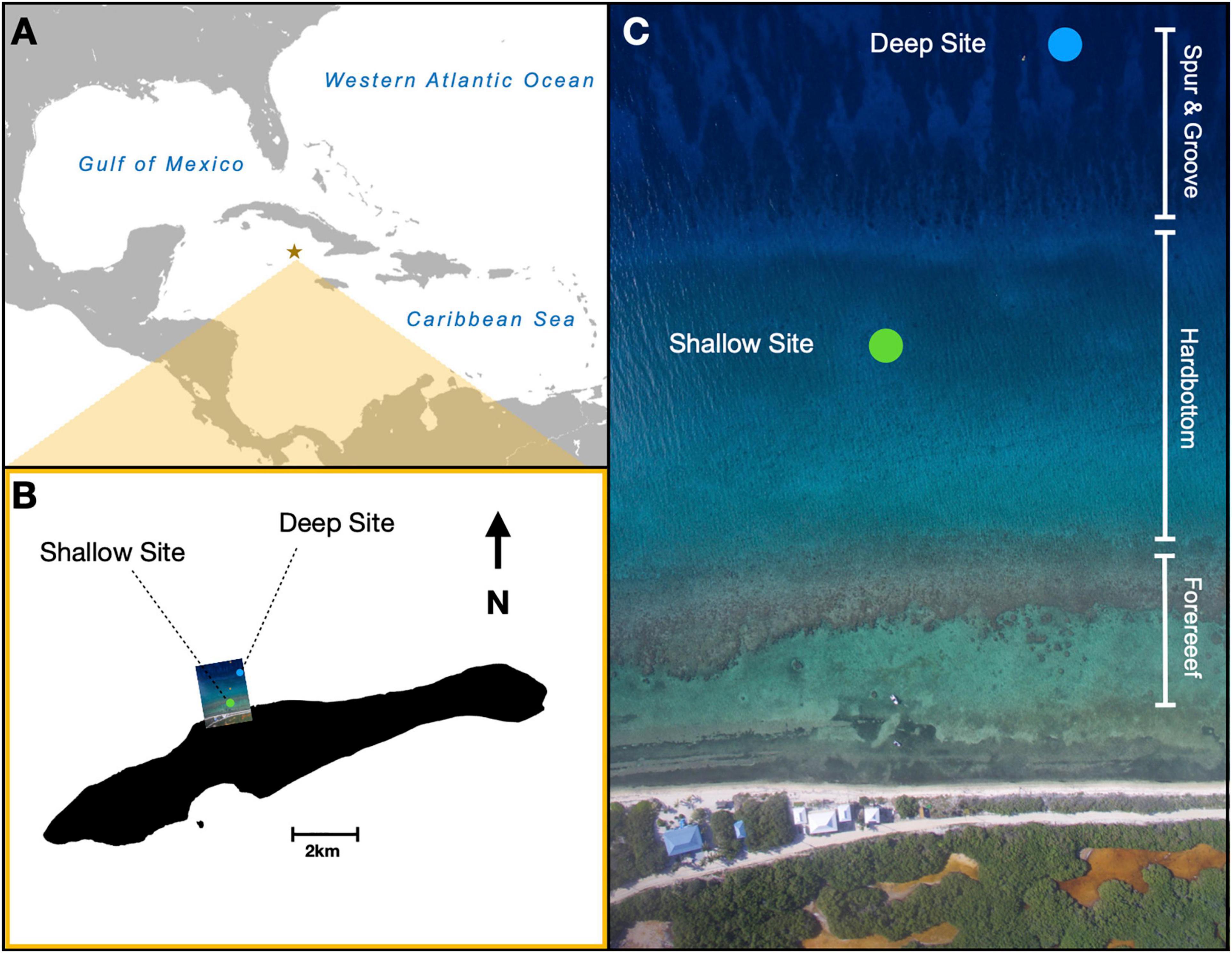
Figure 1. Locations of nurseries for staghorn coral Acropora cervicornis examined in this study. Nursery sites were in the (A) central Caribbean on (B) the north side of Little Cayman Island. (C) Arial imagery shows the specific locations of the shallow (6–8 m) nursery site located in hardbottom habitat and deep (15–18 m) nursery site located on sand in between spur and groove reef.
Two types of nurseries were used: trees and frames. Trees were 2 m in height by 1 m wide, with a single vertical column kept upright by a buoy and nine horizontal PVC branches from which the corals were suspended (Figure 2A). Similar nurseries have been deployed throughout the Caribbean (Johnson et al., 2011; Nedimyer et al., 2011). Frames were PVC rectangles anchored at two points and buoyed at the two opposing points so that the frame remained perpendicular to the substrate (Figure 2B). Each frame was 3.0 m wide by 1.5 m tall, with a center brace and five lines of 400-lb test monofilament strung horizontally. Originally, this design was employed as nurseries for staghorn corals in Puerto Rico (Griffin et al., 2012).
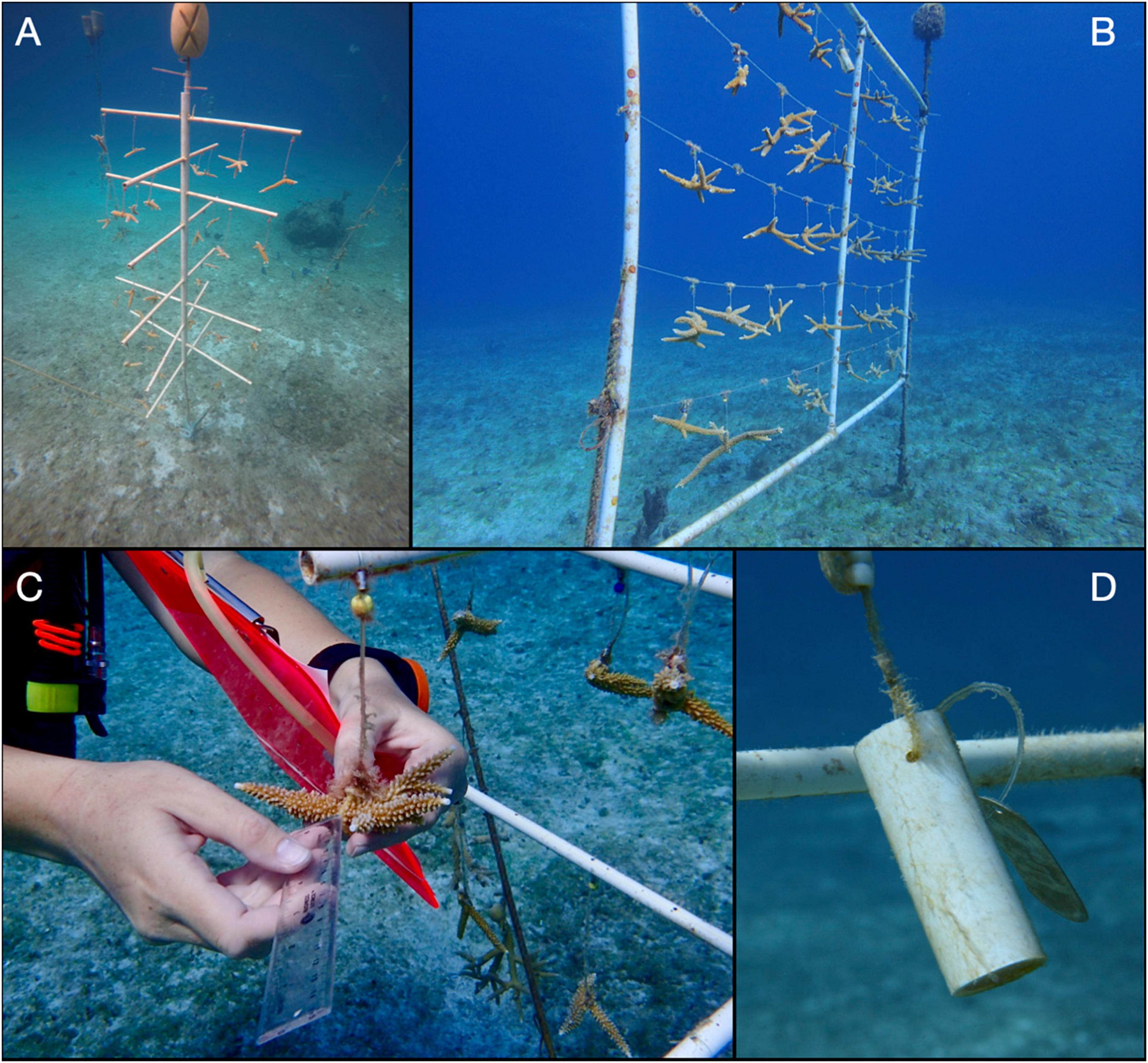
Figure 2. Digital images of nurseries and data collection in this study. Two types of nurseries were used: (A) a tree that comprised a vertical column and nine horizontal PVC branches or a (B) frame that comprised PVC in a vertical rectangle with monofilament strung horizontally. (C) Total linear extensions of coral fragments measured by divers. Accumulation of biofouling was quantified on (D) 4-cm-long piece of PVC attached to the nurseries.
Three replicate nurseries of each design were placed at both sites (total number of nurseries = 12). Stainless steel pins were used to attach trees and frames to the hardbottom substrate at the shallow site (Figure 2A). Concrete blocks partially buried in sand anchored trees and frames at the deep site (Figure 2B). Nurseries at the shallow site were placed at least 20 m away from any pre-existing structures, and each nursery at both sites was at least 5 m away from its neighbors.
Each nursery was populated with 36 fragments of staghorn coral from five genotypes previously identified as genetically distinct (Drury et al., 2017) over 2 days in late May 2016. Genotypes were identified by colored beads, with nine yellow, nine red, nine green, five black, and four blue fragments attached to each structure. Fewer black and blue genotypes were used due to the limited number of fragments available. On trees, four haphazardly selected coral fragments were hung from each of the nine branches (n = 36 fragments). On frames, the 36 coral fragments were haphazardly distributed among the 50 possible attachment sites (i.e., 10 potential sites across each of the five horizontal lines). All 432 fragments of coral were attached using 100-lb test monofilament line and aluminum crimps (Figure 2C). Fragments were selected to be similar in size as determined by summing the lengths of all their branches, i.e., measuring total linear extensions (TLEs). The mean and standard error of initial measurements was 12.1 ± 4.1 cm.
Each nursery also had three, 4-cm-long pieces of PVC pipe attached in the same way as fragments of coral (Figure 2D). Each piece of PVC was weighed to the nearest 0.01 g before deployment and identified by a uniquely numbered metal tag so that changes in weight due to biofouling could be determined.
Data Collection and Maintenance
Growth of coral fragments was quantified by measuring the lengths of all branches comprising each fragment to the nearest 0.5 cm and summing those measurements to yield TLEs (Figure 2C). Measurements were taken in situ by SCUBA divers monthly from June 1 through August 2016, and subsequently, every other month through December 15, 2016, for a total of five sets of measurements. Data sheets included TLEs from the previous dive to minimize measurement error and prompt a search for broken branches should there be a reduction or no change in a TLE.
Temperature was sampled contemporaneously at the shallow and deep sites with Onset HOBO® Pendant Temperature/Light 64K data loggers. Readings were recorded every 30 min for the duration of the study. Data loggers were downloaded in situ every 2–3 months using an Onset HOBO Waterproof Shuttle.
In August 2016, the pieces of PVC that had been deployed in June were removed and placed into individual Ziploc® bags to minimize the loss of accumulated biofouling during handling and transportation. In the laboratory, each section was weighed to the nearest 0.01 g after 8 h of drying in ambient conditions.
Maintenance was performed twice monthly if weather permitted. Divers used cloth gloves to remove fouling organisms from all nursery structures, with the exception of the pieces of PVC deployed to accumulate biofouling.
Analysis of Data
The TLEs were analyzed in two ways. First, TLEs for replicate fragments of coral in each of the 20 different combinations of genotype, depth, and type of nursery were regressed against days since installation to determine if growth remained linear. Second, daily incremental growth rates were derived from measurements of TLEs by taking the difference between two successive measurements and dividing it by the number of days between them. Data for fragments that showed loss of skeletal material were omitted, which accounted for less than 5% of all coral fragments. The resulting growth rates were analyzed with a permutation analysis of variance (PERMANOVA) computed in PRIMER 6 (Anderson et al., 2008). The PERMANOVA treated genotype (yellow, red, green, black, or blue), depth (shallow or deep), and type of nursery (tree or frame) as fixed factors. Three nested (i.e., random) factors accounted for the presence of repeated measures of individual fragments of coral: (1) the three replicate nurseries installed at each depth, (2) each individual fragment of coral hung from every nursery, and (3) the number of days since installation for each observation. The hierarchical portion of the analysis had replicate nested in the interaction of the three fixed factors, individual coral fragments nested in replicates, and days of observation nested in individuals. Where appropriate, additional pairwise, permutation analyses were performed to identify significant differences among levels of fixed factors or their interactions.
High-resolution temperature data (30-min intervals) were used to identify consistent differences between the shallow and deep sites that may have influenced growth rates. A faulty recorder resulted in temperature data being available only after July 30. The data were used to calculate mean daily temperature over the intervals between measurements of TLEs, and the resulting data were analyzed using a paired t-test. The high-resolution data were also examined to identify the frequency and duration of potential thermal stress based on a 31°C threshold drawn from the literature (Shinn, 1966; Acropora Biological Review Team, 2005; Manzello et al., 2007).
Amounts of accumulated biofouling were used to characterize the need for maintenance of nurseries. Amounts of accumulated biofouling at the shallow and deep sites were calculated as the difference between the weights of the pieces of PVC after and before being deployed on nurseries. These differences were analyzed with a Welch’s t-test that accounted for unequal variances.
Results
All 20 regressions of TLEs against days since installation were significant at p < 0.001, and they indicated that growth rates remained relatively constant (Supplementary Figure 1). In general, TLEs for individual fragments became more variable as the experiment progressed. Out of the 10 steepest slopes, which corresponded to the highest growth rates, 80% involved the yellow, black, or blue genotypes; 60% involved fragments on frames; and 80% involved fragments held in deeper water.
These trends were confirmed by the PERMANOVA, which showed that mean daily incremental growth rates varied significantly among replicate nurseries (Table 1). Among the 60 replicate nurseries, mean daily incremental growth rates ranged from 0.07 to 0.28 cm day–1 (Supplementary Figure 2). Out of the 20 highest growth rates, 90% involved the yellow, black, or green genotypes; 55% involved fragments held on frames; and 85% involved fragments held in deeper water. These results highlighted fine-scale variation, which should be considered when culturing fragments of coral. Although variation among replicate nurseries provided some useful and useable insights, the other F-ratios accounted for this variation and provided highly relevant insights. Therefore, the significant effects of genotype, depth, and type of nursery were investigated (Table 1; please see pp. 46–48 in Anderson et al., 2008).
Regardless of the type of nursery or site where they were located, genotypes grew at significantly different rates (Figure 3A). Post hoc tests indicated that the yellow genotype had the highest daily incremental growth rate (mean ± standard error = 0.21 ± 0.006 cm day–1), red and blue genotypes grew slowest (0.13 ± 0.005 and 0.11 ± 0.005 cm day–1, respectively), and black and green genotypes displayed intermediate daily incremental growth rates (0.17 ± 0.005 and 0.16 ± 0.005 cm day–1, respectively). Depth and type of nursery also had significant effects on growth rates. Fragments at the deep site (0.18 ± 0.004 cm day–1) grew significantly faster than fragments held at the shallow site (0.13 ± 0.003 cm day–1; Figure 3B). Although the difference was less pronounced, growth rates were also significantly higher for fragments on frames (0.17 cm day–1 ± 0.004 SE) compared to fragments on trees (0.15 cm day–1 ± 0.003; Figure 3C).
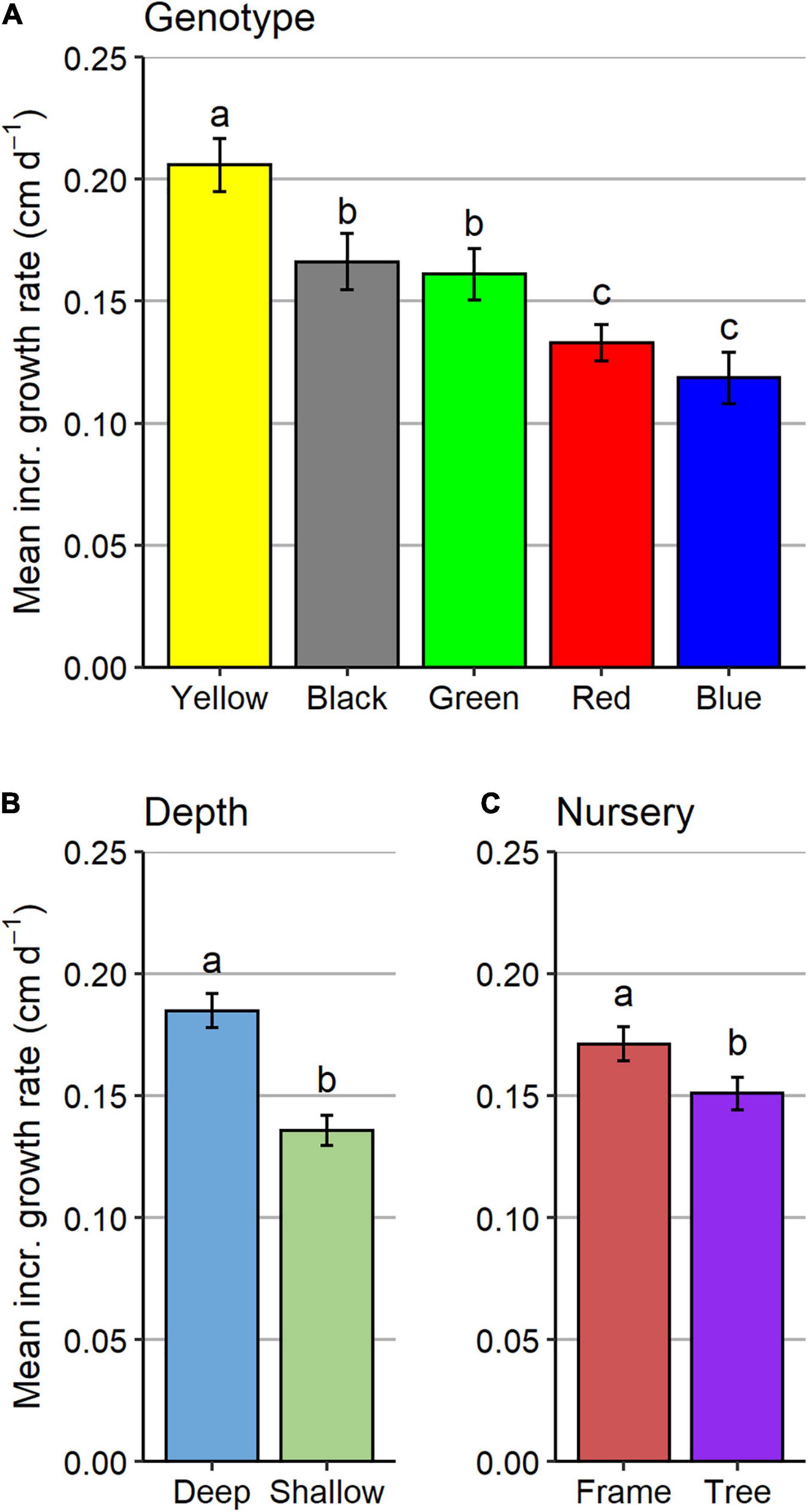
Figure 3. Mean incremental growth rates ± standard errors (SE) for (A) different genotypes, (B) depths, and (C) nurseries. Incr., incremental.
Growth rates at the two sites may have been affected by water temperatures, which did differ. Mean daily water temperatures at the shallow site were significantly higher than those at the deep site (t138 = 10.12, p < 0.001). However, the mean values differed by ∼0.1°C (mean ± standard error of 29.7 ± 0.08°C for the shallow site and 29.6 ± 0.08°C for the deep site), so average temperatures may not have been the most relevant factor. Further evidence that the shallow site experienced warmer temperatures was derived from the data collected every 30 min, particularly during the warmer months of July–September (Figure 4). During these months, temperatures surpassed 31°C at the shallow site on 28 days, which included two runs of 12 and 13 consecutive days, respectively (Figure 4A). In contrast, temperatures at the deep site surpassed 31°C only once (Figure 4B) over the same time period. In addition, the peak daily temperature was greater than 1°C warmer at the shallow site on 5 days in August (Figure 4C). Despite relatively high temperatures, bleaching was not observed for any of the fragments at either site.
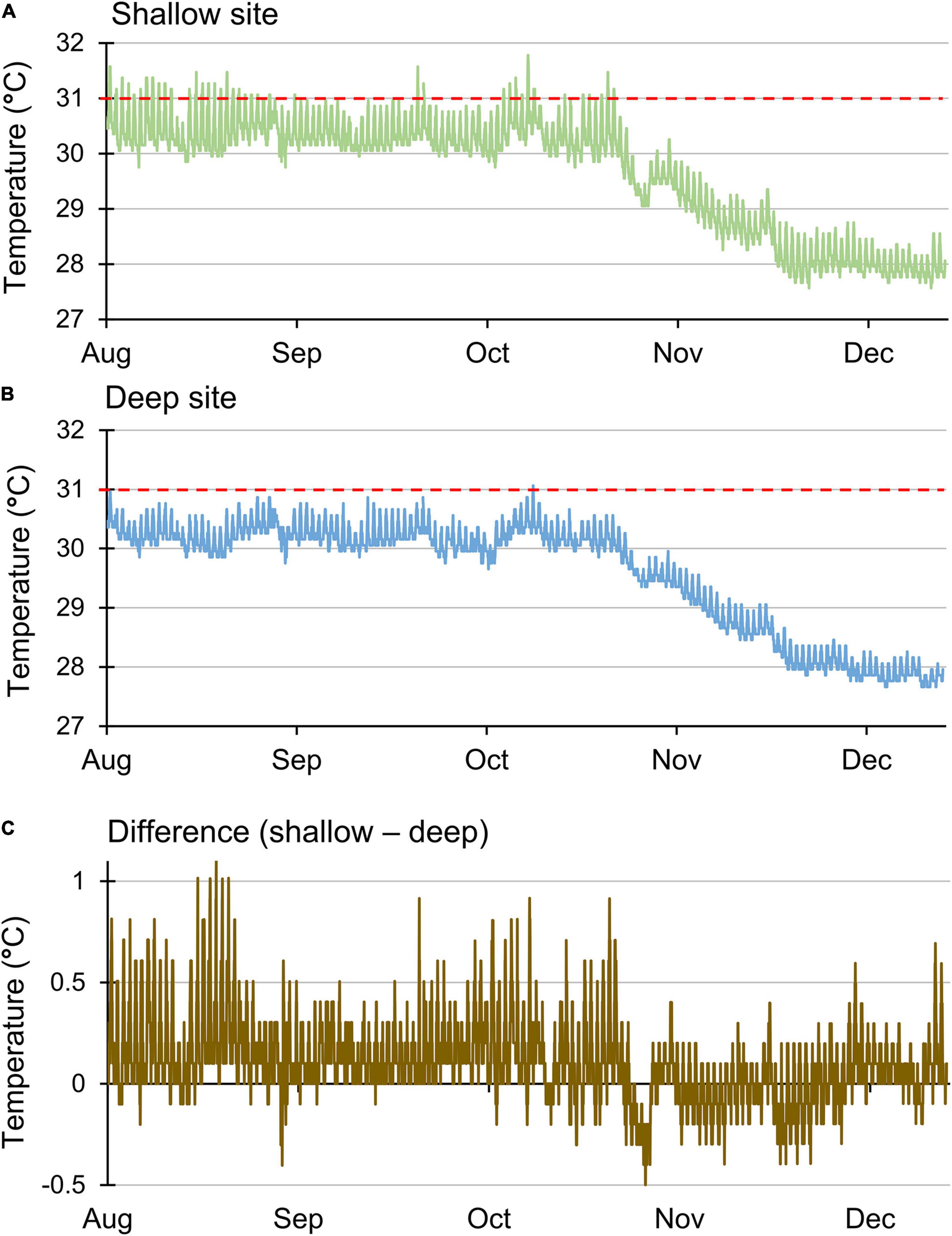
Figure 4. Sea water temperature recorded every 30 min located at a (A) shallow site (6–8 m), (B) deep site (15–18 m), and (C) differences between those temperatures. Red line indicates the 31°C threshold for stress.
Algae accumulated on 12 pieces of PVC at the shallow site and 12 pieces of PVC at the deep site for 3 months. Upon collection, algal biomass on pieces from the shallow site were significantly higher (t8 = 4.86, p = 0.001), with mean dry weights ± standard errors being 1.03 ± 0.15 g for the shallow site and 0.28 ± 0.02 g for the deep site.
Discussion
To our knowledge, this study is the first to simultaneously examine the influences of genotype, design of nurseries, and depth on the growth rates of fragments of staghorn coral, along with efforts to evaluate potential temperature stress, rates of biofouling, and time required for maintenance. We recorded significantly higher mean daily incremental growth rates for fragments of the yellow genotype (24% higher than the next fastest growing genotype and 74% higher than the slowest growing genotype), fragments held at the deep site (36% higher than fragments held at the shallow site), and fragments held on frames (13% higher than fragments on trees). Temperature data suggested that nurseries at the shallow site were exposed to potentially stressful sea surface temperatures during warmer months, which correlated with a 3.5 × higher rate of accumulation of algal biomass at the shallow site. In our experience, less biofouling at the deeper site led to less time expended on maintenance. Collectively, these results point to the value of considering multiple factors when culturing coral fragments to support restoration of coral reefs.
Out of all the influences examined, genotype yielded the greatest variation in growth rates of fragments of coral, with the fastest growing genotype extending at a rate that was nearly twice that of the slowest growing genotype. In addition, these differences in growth rates remained consistent regardless of which depth or type of nursery was employed. Differences in growth rates among genotypes were expected because they had been reported in other studies using nursery-reared corals held in the water column (Lohr et al., 2015; O’Donnell et al., 2017).
Since the goal of coral nursery propagation is to provide genetically diverse and robust coral populations that ultimately survive and thrive after outplanting (Baums et al., 2010), growth rates should not be the only consideration. Slower growing corals may have desirable characteristics, such as thermal tolerance (Jones and Berkelmans, 2010) or disease resistance (Hunt and Sharp, 2014). Such characteristics and survival rates have not been evaluated for staghorn coral off Little Cayman Island; therefore, we support the recommendation made by Shearer et al. (2009), regarding the value of culturing numerous genotypes to establish and maintain sufficient genetic diversity. In summary, further work off Little Cayman Island should track survival and growth after outplanting for more than five genotypes.
Three key and potentially interrelated findings from this study were the higher mean daily incremental growth rates recorded for fragments of coral held at the deep site, the reduced amount of biofouling at the deep site, and the potential for less thermal stress at the deep site. For example, linear extrapolation of growth rates at the deeper site yielded mean growth rates of 66 cm y–1. Enhanced growth may have resulted from less biofouling, with approximately 70% less biofouling accumulating at the deep site (Figure 5). The enhanced growth and reduced biofouling also may have resulted from consistently cooler temperatures at the deep site. In terms of enhanced growth, fragments of coral may have experienced reduced thermal stress as evidenced by fewer maximum daily temperatures above 31°C, although bleaching, evidence of severe thermal stress, was never observed. The cooler temperatures may also have combined with lower levels of light at the deep site to inhibit the growth of algae, which may have promoted more rapid growth of coral fragments and reduced the time required for maintenance of nurseries. These results aligned with similar observations of lower maintenance and higher growth rates reported for fragments of coral held on frames in deep water during preparations for restoring damage from the grounding of the T/V Magara (Griffin et al., 2012). However, the results contradicted previous reports of higher linear extension rates at shallower depths (Wellington and Glynn, 1983; Gladfelter, 1984; Huston, 1985), which were attributed to increased exposure to light. Furthermore, thresholds for optimal growth and onset of stress are likely to vary among locations and genotypes (Glynn, 1990; Knowlton et al., 1992; Edmunds, 1994; Rowan et al., 1997; Berkelmans, 2002; Manzello et al., 2007). In summary, our findings regarding the potential benefits of siting nurseries in deeper water require further evaluation that includes cultured fragments of coral at multiple sites and multiple depths.
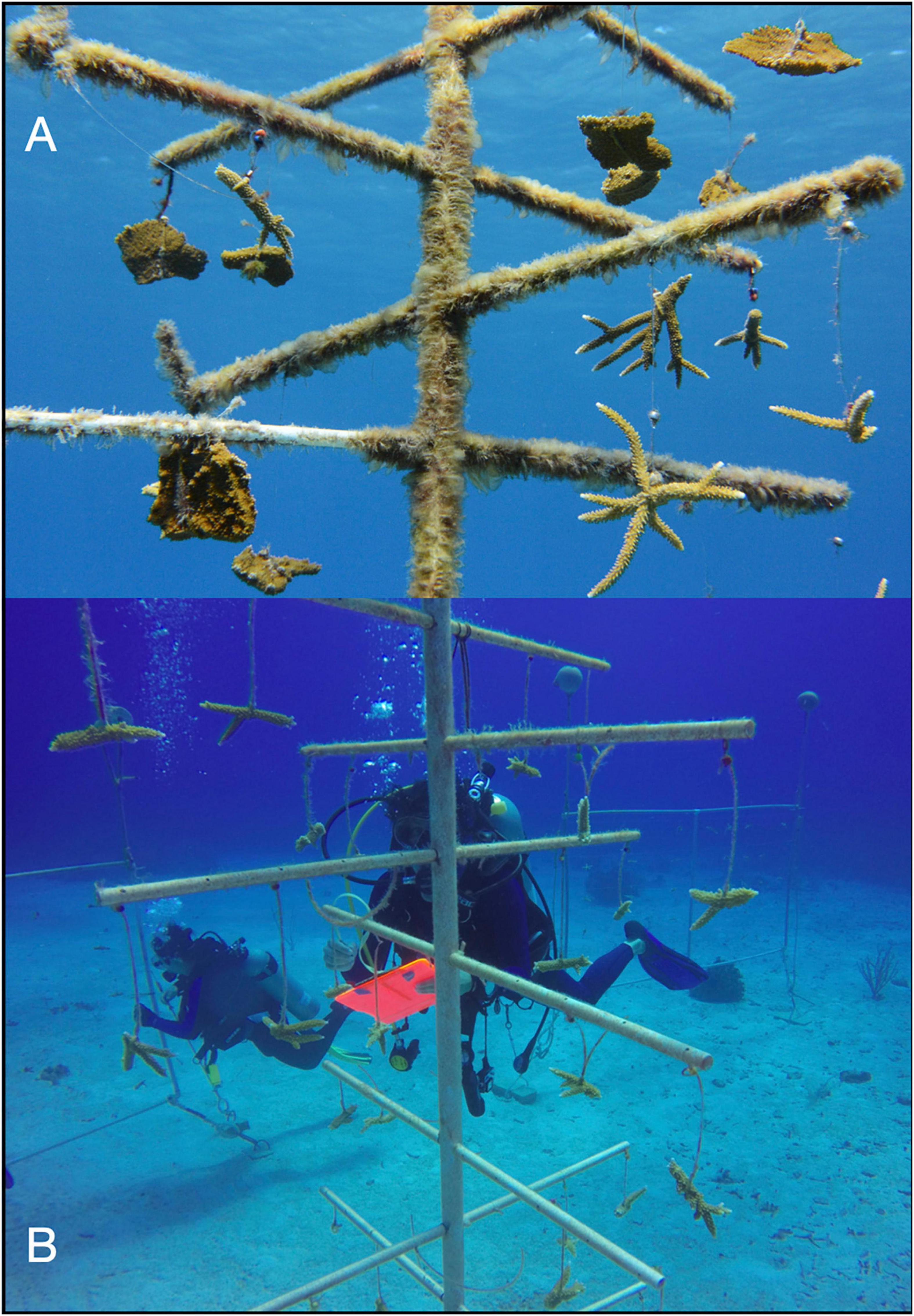
Figure 5. Accumulation of biofouling on (A) a nursery at the shallow site and (B) a nursery at the deep site after approximately 1 month.
The design of nurseries also influenced growth rates, the efficiency of data collection, and maintenance. In this study, even the slowest growing genotypes exhibited growth rates that were markedly higher than many corals grown in nurseries that were closer to the bottom (O’Donnell et al., 2017; Schopmeyer et al., 2017), which indicated the value of suspending fragments in the water column. Furthermore, fragments of coral on frames had slightly higher incremental growth rates, and the planar arrangement of the corals may have reduced competition for food among adjacent fragments because all fragments experienced more similar exposures to currents that carried food. In fact, previous work with trees indicated that higher densities of corals within a nursery restricted growth (O’Donnell et al., 2017). Beyond enhanced growth rates, the planar structure of frames offered practical advantages, with divers reporting less incidental contact with fragments of coral and less interference when two divers worked on a single nursery (Supplementary Video 1). Such advantages should be particularly valuable to operations that employ less experienced divers because skilled divers could measure corals and volunteers could focus on maintenance without undue interference.
Additional support for the value of deploying frames and establishing nurseries in deeper water was provided by the effects of a severe winter storm that occurred a month after this study was completed. The storm dislodged two of the three trees and several large coral fragments from the remaining tree at the shallow site. In comparison, only one frame at the shallow site suffered minor damage and no fragments were lost or damaged. The frames included two points of attachment to the substrate and two floats, which appeared to provide redundancy and resistance to high energy events. Although frames proved beneficial, depth provided protection to both types of nurseries, with none of the structures at the deep site being affected even though it was only 100 m away from the shallow site.
Results from this study have informed practices undertaken by staff of the Central Caribbean Marine Institute on Little Cayman Island. By 2018, the shallow nursery had been retired, the deep nursery was expanded (Supplementary Video 2), additional deep nursery sites were planned, and trees were being replaced by frames. The quantitative and qualitative lessons from this work should be applicable to other efforts to grow and outplant coral fragments and to the more challenging tasks of culturing sufficient numbers of corals that will survive and thrive after outplanting (Rinkevich, 2014; Lirman and Schopmeyer, 2016).
Data Availability Statement
The raw data supporting the conclusions of this article will be made available by the authors, without undue reservation.
Author Contributions
PM and TF conceived the study. PM performed field and laboratory work and drafted the manuscript. HH contributed to field work. PM, CJ, and HH performed analyses and produced figures. PM, HH, CJ, and TF interpreted the data. CJ, HH, and TF contributed to revisions of the manuscript. TF contributed funding. All authors approved the submitted version.
Funding
Support for this work came from the School of Natural Resources and the Environment at the University of Florida. The Central Caribbean Marine Institute funded fieldwork.
Conflict of Interest
The authors declare that the research was conducted in the absence of any commercial or financial relationships that could be construed as a potential conflict of interest.
Acknowledgments
We would like to thank the Central Caribbean Marine Institute, its staff, and volunteers for countless hours of fieldwork and field accommodations. We would also like to thank Sean Griffin from National Oceanic and Atmospheric Administration for input on the design and deployment of frames. This work was conducted with permission from the Cayman Islands Department of Environment.
Supplementary Material
The Supplementary Material for this article can be found online at: https://www.frontiersin.org/articles/10.3389/fmars.2021.670474/full#supplementary-material
Supplementary Figure 1 | Regressions of total linear extension on days since installation. Data for trees (gray squares) are offset by 1 day to allow data for frames (black circles) to be visible. All regressions are significant at p < 0.001. Statistics for regressions: Yellow genotype on frames at the shallow site: y = 0.21x + 13.80, R2 = 0.648; yellow genotype on trees at the shallow site: y = 0.16x + 12.20, R2 = 0.643; yellow genotype on frames at the deep site: y = 0.26x + 9.87, R2 = 0.822; yellow genotype on trees at the deep site: y = 0.25x + 9.78, R2 = 0.784; black genotype on frames at the shallow site: y = 0.15x + 10.50, R2 = 0.789; black genotype on trees at the shallow site: y = 0.14x + 11.48, R2 = 0.659; black genotype on frames at the deep site: y = 0.22x + 9.47, R2 = 0.818; black genotype on trees at the deep site: y = 0.21x + 9.46, R2 = 0.817; green genotype on frames at the shallow site: y = 0.20x + 9.47, R2 = 0.813; green genotype on trees at the shallow site: y = 0.13x + 10.23, R2 = 0.638; green genotype on frames at the deep site: y = 0.22x + 7.95, R2 = 0.758; green genotype on trees at the deep site: y = 0.20x + 6.75, R2 = 0.776; red genotype on frames at the shallow site: y = 0.13x + 10.63, R2 = 0.683; red genotype on trees at the shallow site: y = 0.13x + 12.62, R2 = 0.673; red genotype on frames at the deep site: y = 0.18x + 11.23, R2 = 0.749; red genotype on trees at the deep site: y = 0.16x + 13.33, R2 = 0.692; blue genotype on frames at the shallow site: y = 0.08x + 5.99, R2 = 0.760; blue genotype on trees at the shallow site: y = 0.11x + 11.92, R2 = 0.388; blue genotype on frames at the deep site: y = 0.15x + 6.90, R2 = 0.741; blue genotype on trees at the deep site: y = 0.17x + 10.60, R2 = 0.747.
Supplementary Figure 2 | Mean incremental growth rates ± standard errors (SE) for each replicate of each genotype suspended from the two nurseries at the two depths.
Supplementary Video 1 | Video of divers cleaning frame and trees at the deep site. Note that two divers can work simultaneously on the frame structure, while the tree is limited to a single diver.
Supplementary Video 2 | Video of the CCMI deep site expansion in 2018. New frames have been added and trees are being phased out. These structures contain over 500 linear meters of coral fragments available for outplanting.
References
Acropora Biological Review Team (2005). Atlantic Acropora Status Review Document. St. Petersburg, FL: National Marine Fisheries Service.
Alvarez-Filip, L., Dulvy, N. K., Gill, J. A., Côté, I. M., and Watkinson, A. R. (2009). Flattening of Caribbean coral reefs: region-wide declines in architectural complexity. Proc. R. Soc. B Biol. Sci. 276, 3019–3025. doi: 10.1098/rspb.2009.0339
Anderson, M. J., Gorley, R. N., and Clarke, K. R. (2008). PERMANOVA+ for PRIMER: Guide to Software and Statistical Methods. PRIMER-E: Plymouth.
Aronson, R., and Precht, W. (1997). Stasis, biological disturbance, and community structure of a Holocene coral reef. Paleobiology 23, 326–346. doi: 10.1017/S0094837300019710
Aronson, R. B., and Precht, W. F. (2001). White-band disease and the changing face of Caribbean coral reefs. Hydrobiologia 460, 25–38. doi: 10.1023/A:1013103928980
Baums, I. B., Johnson, M. E., Devlin-Durante, M. K., and Miller, M. W. (2010). Host population genetic structure and zooxanthellae diversity of two reef-building coral species along the Florida Reef Tract and wider Caribbean. Coral Reefs 29:835–842. doi: 10.1007/s00338-010-0645-y
Berkelmans, R. (2002). Time-integrated thermal bleaching thresholds of reefs and their variation on the Great Barrier Reef. Mar. Ecol. Prog. Ser. 229, 73–82. doi: 10.3354/meps229073
Bowden-Kerby, A. (2001). Low-tech coral reef restoration methods modeled after natural fragmentation processes. Bull. Mar. Sci. 69, 915–931.
Bowden-Kerby, A., Quinn, N., Stennet, M., and Mejia, A. (2005). Acropora Cervicornis Restoration to Support Coral Reef Conservation in the Caribbean. Noaa Coastal Zone 05. New Orleans, Louisiana: Coral Gardens Initiative, Counterpart International.
Drury, C., Schopmeyer, S., Goergen, E., Bartels, E., Nedimyer, K., Johnson, M., et al. (2017). Genomic patterns in Acropora cervicornis show extensive population structure and variable genetic diversity. Ecol. Evol. 7, 6188–6200. doi: 10.1002/ece3.3184
Edmunds, P. (1994). Evidence that reef-wide patterns of coral bleaching may be the result of the distribution of bleaching-susceptible clones. Mar. Biol. 121, 137–142. doi: 10.1007/bf00349482
Edwards, A. J., and Gomez, E. D. (2007). Reef Restoration Concepts and Guidelines: Making Sensible Management Choices in the Face of Uncertainty. St. Lucia, Australia: The Coral Reef Targeted Research & Capacity Building for Management Program.
Gignoux-Wolfsohn, S., Marks, C. J., and Vollmer, S. V. (2012). White band disease transmission in the threatened coral, Acropora cervicornis. Sci. Rep. 2:804. doi: 10.1038/srep00804
Gilmore, M., and Hall, B. (1976). Life history, growth habits, and constructional roles of Acropora cervicornis in the patch reef environment. J. Sediment. Petrol. 46, 519–522. doi: 10.1306/212f6fd7-2b24-11d7-8648000102c1865d
Gladfelter, E. H. (1984). Skeletal development in Acropora cervicornis. Coral Reefs 3, 51–57. doi: 10.1007/BF00306140
Gladfelter, W. B. (1982). White-band disease in Acropora palmata: implications for the structure and growth of shallow reefs. Bull. Mar. Sci. 32, 639–643.
Glynn, P. W. (1990). Coral mortality and disturbances to coral reefs in the tropical eastern Pacific. Elsev. Oceanogr. Serie. 52, 55–126. doi: 10.1016/s0422-9894(08)70033-3
Griffin, S., Spathias, H., Moore, T., Baums, I., and Griffin, B. A. (2012). “Scaling up Acropora nurseries in the Caribbean and improving techniques” in D. Yellowlees, T. P. Hughes. (Eds) Proceedings of the 12th International Coral Reef Symposium. (Townsville: ARC Centre of Excellence for Coral Reef Studies). 9–13.
Hughes, T. P., and Connell, J. H. (1999). Multiple stressors on coral reefs: a long-term perspective. Limnol. Oceanogr. 44, 932–940. doi: 10.4319/lo.1999.44.3_part_2.0932
Hunt, J., and Sharp, W. (2014). Developing a Comprehensive Strategy for Coral Restoration for Florida. State Wildlife Grant Award t-32-r 1169: Final Report. Tallahassee, Florida Fish and Wildlife Conservation Commission.
Huston, M. (1985). Variation in coral growth rates with depth at Discovery Bay, Jamaica. Coral Reefs 4, 19–25. doi: 10.1007/BF00302200
Johnson, M. E., Lustic, C., Bartels, E., Baums, I. B., Gilliam, D. S., Larson, L., et al. (2011). Caribbean Acropora Restoration Guide: Best Practices For Propagation And Population Enhancement. Arlington, VA: The Nature Conservancy.
Jones, A., and Berkelmans, R. (2010). Potential costs of acclimatization to a warmer climate: growth of a reef coral with heat tolerant vs. sensitive symbiont types. PLoS One 5:e10437. doi: 10.1371/journal.pone.0010437
Knowlton, N., Weil, E., Weight, L. A., and Guzmán, H. M. (1992). Sibling species in Montastraea annularis, coral bleaching, and the coral climate record. Science 255, 330–333. doi: 10.1126/science.255.5042.330
Lirman, D., and Schopmeyer, S. (2016). Ecological solutions to reef degradation: optimizing coral reef restoration in the Caribbean and Western Atlantic. Peerj 4:e2597.
Lohr, K., Bejarano, S., Lirman, D., Schopmeyer, S., and Manfrino, C. (2015). Optimizing the productivity of a coral nursery focused on staghorn coral Acropora cervicornis. Endanger. Species Res. 27, 243–250. doi: 10.3354/esr00667
Manzello, D. P., Berkelmans, R., and Hendee, J. C. (2007). Coral bleaching indices and thresholds for the Florida Reef Tract, Bahamas, and St. Croix, US Virgin Islands. Mar. Pollut. Bull. 54, 1923–1931. doi: 10.1016/j.marpolbul.2007.08.009
National Marine Fisheries Service (2015). Recovery Plan for Elkhorn Coral (Acropora palmata) and Staghorn Coral (A. cervicornis). St. Petersburg, FL: National Marine Fisheries Service.
Nedimyer, K., Gaines, K., and Roach, S. (2011). Coral tree nursery: an innovative approach to growing corals in an ocean-based field nursery. AACL Bioflux 4, 442–446.
O’Donnell, K. E., Lohr, K. E., Bartels, E., and Patterson, J. T. (2017). Evaluation of staghorn coral (Acropora cervicornis, Lamarck 1816) production techniques in an ocean-based nursery with consideration of coral genotype. J. Exp. Mar. Biol. Ecol. 487, 53–58. doi: 10.1016/j.jembe.2016.11.013
Precht, W., Bruckner, A., Aronson, R., and Bruckner, R. (2002). Endangered acroporid corals of the Caribbean. Coral Reefs 21, 41–42. doi: 10.1007/s00338-001-0209-2
Quinn, N. J., and Kojis, B. L. (2006). Patterns of sexual recruitment of acroporid coral populations on the West Fore Reef at Discovery Bay, Jamaica. Rev. Biol. Trop. 53, 83–89. doi: 10.15517/rbt.v53i1.26623
Rinkevich, B. (2014). Rebuilding coral reefs: does active reef restoration lead to sustainable reefs? Curr. Opin. Eniron. Sustain. 7:28–36. doi: 10.1016/j.cosust.2013.11.018
Rowan, R., Knowlton, N., Baker, A., and Jara, J. (1997). Landscape ecology of algal symbionts creates variation in episodes of coral bleaching. Nature 388:40843. doi: 10.1038/40843
Schopmeyer, S. A., Lirman, D., Bartels, E., Byrne, J., Gilliam, D. S., Hunt, J., et al. (2012). In situ coral nurseries serve as genetic repositories for coral reef restoration after an extreme cold−water event. Restor. Ecol. 20, 696–703. doi: 10.1111/j.1526-100x.2011.00836.x
Schopmeyer, S. A., Lirman, D., Bartels, E., Gilliam, D. S., Goergen, E. A., Griffin, S. P., et al. (2017). Regional restoration benchmarks for Acropora cervicornis. Coral Reefs 36, 1047–1057. doi: 10.1007/s00338-017-1596-3
Shearer, T. L., Porto, I., and Zubillaga, A. L. (2009). Restoration of coral populations in light of genetic diversity estimates. Coral Reefs 28, 727–733. doi: 10.1007/s00338009-0520-x
Shinn, E. (1966). Coral growth-rate, an environmental indicator. J. Paleontol. 40, 233–240. doi: 10.2307/1301658
Sussman, M., Loya, Y., Fine, M., and Rosenberg, E. (2003). The marine fireworm Hermodice carunculata is a winter reservoir and spring−summer vector for the coral−bleaching pathogen Vibrio shiloi. Environ. Microbiol. 5, 250–255. doi: 10.1046/j.1462-2920.2003.00424.x
Tunnicliffe, V. (1981). Breakage and propagation of the stony coral Acropora cervicornis. Proc. Natl. Acad. Sci. U. S. A. 78, 2427–2431. doi: 10.1073/pnas.78.4.2427
Wellington, G. M., and Glynn, P. W. (1983). Environmental influences on skeletal banding in eastern Pacific (Panama) corals. Coral Reefs 1, 215–222. doi: 10.1007/bf00304418
Williams, D. E., and Miller, M. W. (2005). Coral disease outbreak: pattern, prevalence and transmission in Acropora cervicornis. Mar. Ecol. Prog. Ser. 301, 119–128. doi: 10.3354/meps301119
Keywords: coral restoration, staghorn coral, nursery trees, nursery frames, temperature, biofouling, coral propagation
Citation: Maneval P, Jacoby CA, Harris HE and Frazer TK (2021) Genotype, Nursery Design, and Depth Influence the Growth of Acropora cervicornis Fragments. Front. Mar. Sci. 8:670474. doi: 10.3389/fmars.2021.670474
Received: 21 February 2021; Accepted: 10 May 2021;
Published: 04 June 2021.
Edited by:
Baruch Rinkevich, Israel Oceanographic and Limnological Research, IsraelReviewed by:
Kátia Cristina Cruz Capel, University of São Paulo, BrazilDouglas Fenner, Independent researcher, Pago Pago, American Samoa
Copyright © 2021 Maneval, Jacoby, Harris and Frazer. This is an open-access article distributed under the terms of the Creative Commons Attribution License (CC BY). The use, distribution or reproduction in other forums is permitted, provided the original author(s) and the copyright owner(s) are credited and that the original publication in this journal is cited, in accordance with accepted academic practice. No use, distribution or reproduction is permitted which does not comply with these terms.
*Correspondence: Paul Maneval, cGF1bC5tYW5ldmFsQG5vYWEuZ292