- 1Southwest Fisheries Science Center, National Marine Fisheries Service, National Oceanic and Atmospheric Administration, Santa Cruz, CA, United States
- 2Fisheries Collaborative Program, Institute of Marine Sciences, University of California, Santa Cruz, Santa Cruz, CA, United States
- 3Department of Ecology and Evolutionary Biology, University of California, Santa Cruz, Santa Cruz, CA, United States
- 4Department of Environmental Studies, University of California, Santa Cruz, Santa Cruz, CA, United States
- 5Department of Ocean Sciences, University of California, Santa Cruz, Santa Cruz, CA, United States
- 6Institute of Marine Sciences, University of California, Santa Cruz, Santa Cruz, CA, United States
- 7California Sea Grant, Scripps Institution of Oceanography, University of California, San Diego, La Jolla, CA, United States
Understanding and anticipating the effects of climate change on fisheries social-ecological systems (FSESs) is central to proactive fisheries management in a changing global climate. With fisheries management increasingly striving to consider interactions and feedbacks among people, targeted species, and the broader ecological and human communities, fisheries managers and participants need tools to help them assess these complex systems. We developed a new climate vulnerability assessment framework for analyzing the impacts of a climate-induced trend or event on a FSES. The framework divides the FSES into four interrelated and interacting domains: Ecological Community, Fished Species, Fishery, and Human Community. The framework provides a systematic approach to account for indirect as well as direct effects, links among subsystems, and multiple climate change-induced stressors. We demonstrate the framework’s utility by applying it to three case studies: the effects of a marine heatwave on the Dungeness crab FSES, the effects of a marine heatwave on the red sea urchin FSES, and the effects of long-term climate trends on North Pacific albacore. We found that the effects of a climatic trend or event on a FSES are often indirect and can trigger diverse and important feedbacks. These examples also showed that the climatic trend or event may cause changes in the temporal and spatial distribution of fishing effort and fished species that have a more significant impact on the FSES than changes to species abundance per se. Unlike other climate vulnerability assessment frameworks and applications, ours is designed to enable consideration of the range of feedbacks within and among both the ecological and human communities. As such, it is a valuable tool to guide the holistic examination and assessment of potential impacts to FSESs.
Introduction
Anthropogenic climate change is rapidly altering the world’s oceans through both long-term directional trends and increased frequency of extreme or anomalous events (Cheng et al., 2019; IPCC, 2019), with significant impacts on fisheries (Free et al., 2019; Lotze et al., 2019). Natural climatic trends and variability also impact fisheries. As such, it is crucial to understand and anticipate the effects of climatic trends and extreme events on fisheries social-ecological systems (FSESs). Ecosystem-based fisheries management (EBFM), which considers interactions and feedbacks among people, targeted fish stocks, the broader ecological and human communities, and the environment (Link and Browman, 2014; NOAA, 2016) must account for climate change (NOAA, 2016). With fisheries management increasingly striving for an EBFM approach (Townsend et al., 2019), fisheries managers need tools that incorporate climate change and consider numerous interactions and feedbacks in these complex systems.
Climate vulnerability assessments (CVAs) are commonly used to evaluate the potential effects of climate change on a system. In this manuscript, the term climate change includes naturally driven variability along with the dominant, rapid, anthropogenic driven change. Vulnerability assessments are a well-established tool for evaluating issues such as food security and natural disasters (Füssel and Klein, 2006). CVAs, which grew out of climate impact assessments, use existing biophysical and social scientific information to guide and evaluate strategic policy options, with the primary goal of reducing risk related to climate change (Rothman and Robinson, 1997; Füssel and Klein, 2006). CVAs and climate impact assessments range from simple to complex, quantitative to qualitative, science-motivated to policy-motivated, and may span varying spatial and temporal scales (Rothman and Robinson, 1997). The CVA definition in the Third Assessment Report of the Intergovernmental Panel on Climate Change (IPCC, 2001) is the most widely used and considers both external changes (exposure) and internal vulnerabilities to those changes (sensitivity and adaptive capacity) when attempting to predict impacts (Füssel and Klein, 2006).
There are three general types of fisheries CVA (Table 1): those that focus on the ecological components of the system, those that focus on the social components (human dimensions) of the system, and those that address both the ecological and social components of the system. Given the diverse and sometimes distinct ways that the natural and social sciences analyze interconnected systems, constructing and developing CVAs that address both the ecological and social components of ecosystems can be particularly challenging (Füssel and Klein, 2006). However, as with EBFM, considering interactions within and across both subsystems is essential for designing management that can achieve desired goals while avoiding undesirable outcomes (Pomeroy et al., 2018).
Previous fisheries-oriented CVAs have focused on several different climate impacts of varying scale and scope (Table 1). While these CVAs provide valuable approaches and insights, recent climatic events have produced outcomes that would have been difficult to anticipate using these frameworks. In particular, those developed to date lack a generalizable framework that accounts and allows for cascading (indirect) impacts on the broader ecological and social systems, multiple components in both the ecological and social systems (e.g., different fishing sectors and target species’ life stages), and feedbacks among components. For example, CVAs typically scrutinize the direct effects of climate change on a focal fished species or fishery. However, outcomes in FSESs following recent climate events have been driven largely by indirect effects of climate change on other system components that impact the fished species and fishery through interactions or regulatory changes. In environmentally-driven systems such as FSESs, indirect effects may become increasingly important or dominant with increasing environmental change (Rothman and Robinson, 1997). There are also gaps in the literature examining the interaction between the social and ecological subsystems (Salgueiro-Otero and Ojea, 2020).
Here we propose a CVA framework that aims to more comprehensively identify potential climate change impacts on complex FSESs. This framework does not seek to quantify risk per se, but rather to identify potentially important risks, interactions, and impacts. Considering systems both within and beyond a focal fishery, it is relevant to both EBFM and broader ecosystem-based management. The proposed framework has several similarities to existing CVAs. Users can assess multiple aspects of climate beyond temperature changes and use the framework to attempt to link ecological and human subsystems in a single assessment. Moreover, it uses a classical CVA scheme by assessing the exposure and sensitivity of a component, and then considering its adaptive capacity to determine vulnerability or impact. However, it also—and uniquely—considers these elements across multiple variables and several components within four domains (Ecological Community, Fished Species, Fishery, and Human Community) and then links these elements together in a network to identify a set of outcomes. This framework can accommodate both long-term climate trends and extreme events and users can apply it both retrospectively, to ascertain how past events affected a FSES, and prospectively, to identify potential impacts of a future event. As with any complex system, users will need to make judgments about bounding the system to capture its most salient features, so that the analysis does not become unwieldy. While it will be impossible to precisely and completely capture all components and anticipate all outcomes in a prospective case, a transdisciplinary team with diverse expertise (e.g., managers; economists; fishery participants; community members; physical, biological, and social scientists) should be able to map the more likely potential outcomes and use this framework to identify knowledge gaps and potentially overlooked, consequential pathways. This ability to construct and evaluate a broad range of potential outcomes systematically makes this framework unique. It provides a map of the system, key linkages, and issues to consider when planning, implementing and evaluating policy alternatives with respect to climate change effects, adaptation, and mitigation. Ideally, such an approach can better enable fishery participants, managers, and communities to anticipate, prepare for, avoid, and/or mitigate adverse impacts while enhancing positive outcomes.
Below we describe the framework, its rationale, and the types of information it uses. We then apply the framework to three FSES case studies, two retrospective and one prospective. We show that many of the effects observed in the retrospective case studies were indirect and complex, and thus, a traditional CVA would have failed to capture the observed impacts. We conclude by summarizing the strengths and weaknesses of our framework relative to the existing literature and providing recommendations to potential users.
Materials and Methods
The Framework
Our CVA framework (Figure 1) (“framework”) examines a climatic Trend or Event and its effects on a FSES. The Trend or Event is a change in the abiotic environment resulting from long-term climate change or an abrupt climate-driven disturbance to the physical system (e.g., increased water temperature, low dissolved oxygen, and altered currents). The framework divides the FSES into four interacting domains (Ecological Community, Fished Species, Fishery, and Human Community), which comprise the ecological and human subsystems. Box 1 provides definitions of the framework terms. The Fished Species and the Fishery encompass the focal species (finfish, invertebrates, or other harvested taxa) and the people who target the species, respectively. The Ecological Community encompasses the network of other species with which the Fished Species, Fishery, and Human Community interact directly or indirectly. The Human Community encompasses the larger network of people and institutions with which the Fishery interacts directly or indirectly.
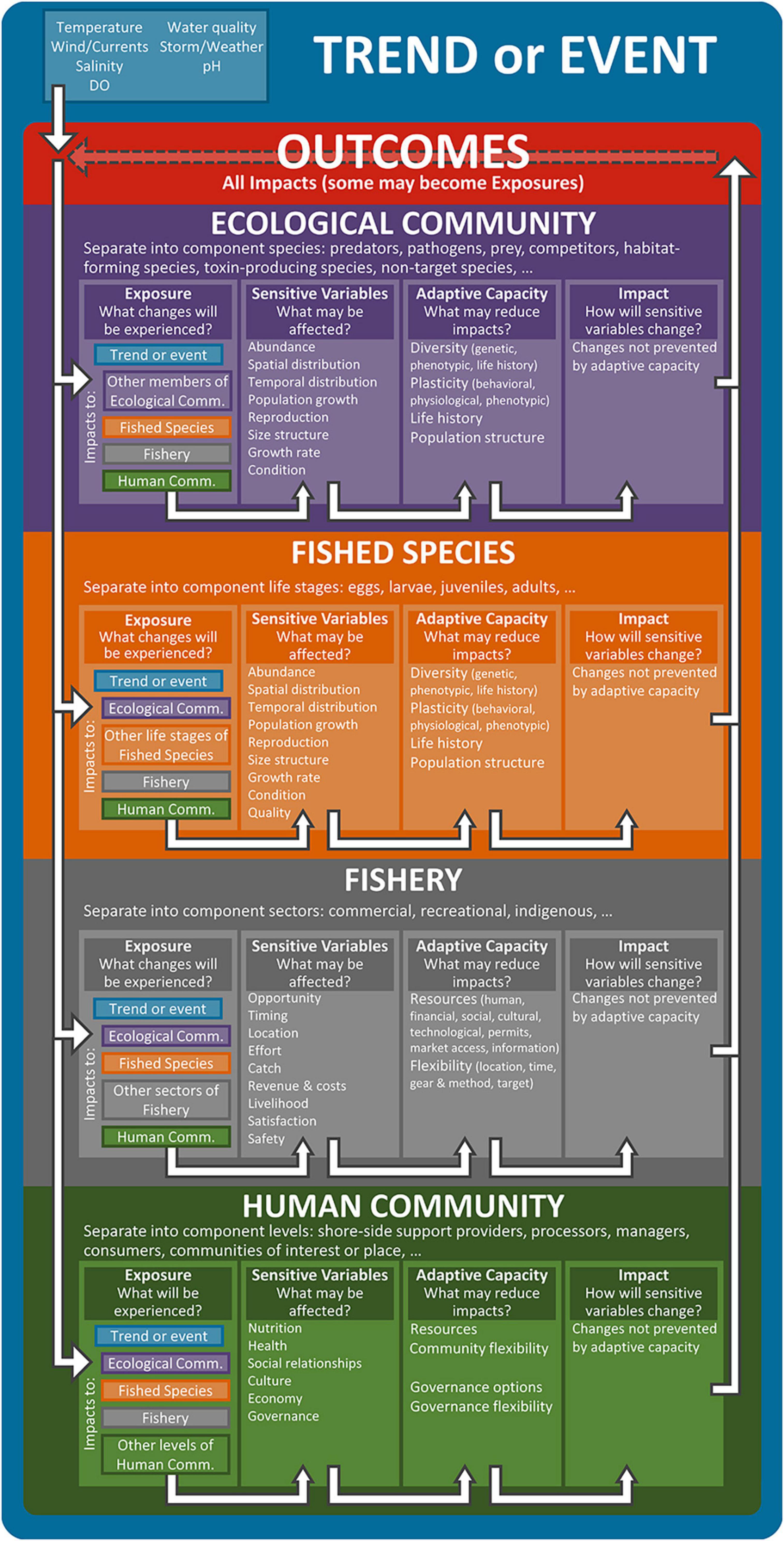
Figure 1. Diagram of the framework. The Trend or Event (blue frame) is the ultimate driver of the observed or possible outcomes. It is displayed at the top to indicate it is the initial exposure and also forms a frame around the entire system to indicate it is the ultimate driver of the analysis. The four larger vertically stacked boxes (purple, orange, gray, and green) represent the four domains (Ecological Community, Fished Species, Fishery, and Human Community). Each domain can have multiple components, which are listed under the relevant domain. Within each domain, there are four aspects (Exposure, Sensitive Variables, Adaptive Capacity, and Impact), each with a list of items to consider when applying the framework (see Box 1 for definitions of these terms). Impacts may be perceived as positive, negative, or neutral. The white arrows represent the work and information flow. The arrow pointing downward from the Trend or Event into Outcomes represents the Trend or Event as causing the initial exposure(s). The descending, right-angled arrows on the left side of the figure represent that outcomes (including the initial abiotic effects of the Trend or Event) can become exposures to one or multiple domains. After the initial consideration of an exposure to a domain (color-coded in the figure to represent their source), the team then considers variables sensitive to the exposure, then adaptive capacity, and finally impacts. The sets of arrows in each domain connecting one aspect to the next representing this process. The set of ascending, right-angled arrows on the right side of the figure represents that the impacts on each domain are outcomes for the system. The dashed, black, right-to-left arrow in the Outcomes box represents that any of the impacts may become exposures to a domain.
BOX 1. Definitions of terms in the proposed framework.
Trend or event: Changes in the abiotic environment. This includes changes in the mean, variance, extremes, and other properties of abiotic variables. These changes may vary in duration, magnitude, and spatial and temporal extent. Examples include hurricanes, hypoxia events, marine heat waves, and long-term gradual warming. Variables that may be impacted include temperature, dissolved oxygen, pH, winds and currents.
Each biotic/human component in the system falls within one of four domains:
Ecological community: A group of species (biotic community) which interact directly or indirectly with the Fished Species, Fishery, or Human Community of interest.
Components include species that may be more than one interaction removed from the Fished Species or Fishery (see section “The Framework” for details). Examples include predators of the Fished Species, predators of those predators, species caught as bycatch in the Fishery, habitat-forming species, and toxin-producing species (e.g., harmful algal species).
Variables that may be impacted include, for example, population abundance or biomass, spatial and temporal distribution, population growth rate, and size structure.
Fished Species: The focal organism(s) of the Fishery.
Components include different life stages of the organism(s) (e.g., larval, juvenile, and adult).
Variables that may be impacted are the same as those for the Ecological Community and include, for example, population abundance, spatial and temporal distribution, population growth rate, size structure, reproductive output, physiological condition, and quality for market.
Fishery: The people, vessels, equipment, gear, and practices of those fishing for the Fished Species.
Components include different sectors of the fishery, such as commercial, recreational, and subsistence, tribal and non-tribal, large- and small-scale operations.
Variables that may be impacted include, for example, fishing opportunity, timing, catch, effort, revenue, and safety.
Human community: A group of people connected by living in the same place, engagement in a common activity, and/or common interest, whose members interact directly or indirectly with the Fishery of interest or the other domains.
Components include different levels of the shoreside system that are one or more interactions removed from the Fishery (see section “The Framework” for details), such as shore-side support providers, processors, managers, consumers, local communities.
Examples of variables that may be impacted include, for example, access to seafood, economic activity and viability, governance, social relationships and institutions, physical and mental health, and behavior.
For each component of the system, the framework considers:
Exposure: Changes experienced directly or indirectly as a result of the Trend or Event. This includes changes to abiotic variables and variables from other components of the system that are impacted by the Trend or Event.
Sensitive variables: The subset of variables that exposures may affect and the extent to which a given exposure affects them.
Adaptive capacity: Properties that influence whether and to what extent an exposure has an impact on a sensitive variable.
Impact: The nature and extent to which an exposure will affect a variable. An exposure will impact a variable if the variable is both sensitive to it and adaptive capacity does not prevent the impacts. There is no value assigned a priori in using ‘impacts’; they may be positive, neutral, or negative depending on one’s perspective and position in the system.
Each domain may be separated into multiple components. For example, components of the Fished Species might include multiple life stages (e.g., larval, juvenile, and adult), and components of the Fishery might include multiple sectors (e.g., commercial, recreational, and subsistence). Components of the Ecological and Human Communities include species and groups of people that may be more than one step (interaction) removed from the Fished Species and Fishery. For example, if the focal species is sardine, the Ecological Community may include predators such as Chinook salmon and the predators of salmon such as sea lions. The Human Community may include processors, retailers, and consumers in the seafood supply chain, along with managers and those who provide fishery-support infrastructure, goods, and services.
The framework structure analyzes four aspects for each of the above components: exposure, sensitive variables, adaptive capacity, and impact. Exposure initially encompasses the abiotic changes associated with the Trend or Event. As the analysis proceeds, other impacts may become part of exposure (see below). The Sensitive Variables are attributes of a component that the exposures may impact. For a Fishery component, such variables might include catch, fishing opportunity, fishing location, revenue, and/or satisfaction. We identify and provide a reasonably comprehensive but non-exhaustive list of domain-specific variables to consider (Supplementary Materials). Variables that are sensitive will be a subset of all possible variables within a given component. Adaptive capacity encompasses those properties of the component that may mediate the effect of exposure on a variable. Impact is how an exposure is expected to affect a sensitive variable when adaptive capacity does not prevent the change. Impacts could be perceived as positive, negative, or neutral depending on one’s position in and perspective on the system.
For exposures to impact a variable, the variable must be both sensitive and lack sufficient adaptive capacity. Adaptive capacity determines which and in what ways sensitive variables are impacted. For example, a Fished Species with a broad thermal performance curve might be insensitive to temperature change, so moderate warming may not impact its abundance, distribution, growth rate, or other variables. A species with a narrow thermal performance curve might be sensitive to temperature change, but if the species is able to shift its distribution (through propagule dispersal or behavioral plasticity) or if individuals vary widely in their thermal optima (phenotypic or genetic diversity), this adaptive capacity may prevent long-term impacts to abundance, although impacts to spatial distribution may occur.
For the Fished Species and the Ecological Community, adaptive capacity includes genetic diversity among individuals, plasticity within individuals, population structure, and life history. Diversity and plasticity may allow for adaptation and acclimatization, respectively, to changing biotic or abiotic conditions. Population structure (including meta-population structure) may buffer against localized changes or disturbances through rescue effects. A species’ life history also may have attributes which buffer against spatially or temporally unfavorable periods for growth or reproduction. For the Fishery, adaptive capacity includes participants’ access to financial, social, technological, and other forms of capital that afford access to alternative fisheries or sources of livelihood and well-being or otherwise buffer adverse impacts. It also includes flexibility, that is, the resources and willingness to shift among locations, times, gear and methods used, and/or species targeted. For the Human Community, adaptive capacity likewise includes access to diverse forms of capital (e.g., financial, human, and social) and the flexibility to use them to make short- and/or long-term adjustments to changing circumstances.
An important feature of this framework is impacts from one component may become exposures for other components in the same or another domain. For example, low dissolved oxygen resulting from a Trend or Event might reduce the abundance of the Fished Species, which in turn becomes an exposure for the Fishery. The catch and revenue of commercial fishery participants may be sensitive to this reduced abundance, and if they lack alternative fishery options (adaptive capacity), may be impacted adversely. The loss of revenue might then have various impacts in the Human Community such as reduced demand for support goods and services and increased need for social services. This framework allows for linkages among all domains and components, cascading effects, and feedbacks (e.g., impacts to an Ecological Community component can directly affect a Fishery component, and vice versa). Therefore, exposure encompasses both the direct and indirect effects of the Trend or Event.
Application of the Framework
Use of the framework begins with a transdisciplinary team (people with diverse professional, experiential, or subject expertise or knowledge) identifying the Trend or Event for which they will evaluate impacts and selecting a focal Fished Species or Fishery. Depending on their focus, the team could alternatively select a focal Ecological or Human Community. The team will use their multidisciplinary knowledge, available literature and data, input from non-team member stakeholders, and step-by-step consideration of the system to determine which components in each domain to include (vs. ignore or assume negligible). Information sources include historic events, connections to and events in related systems, predicted outcomes from projection models, and potential changes to other components (e.g., range shifts in potential bycatch species, temporal shifts in other fisheries). The team should draw on as many resources as possible, however, data gaps likely will exist, requiring the team to decide whether sufficient information exists to draw conclusions.
To demonstrate the application of this framework, we first performed a retrospective analysis of how the North Pacific Marine Heatwave of 2014–2016 impacted two fishery systems. We then performed a prospective analysis of a third fishery system to demonstrate how the framework may be used where the Trend or Event has not yet occurred. Our core team consisted of academic and management agency ecological and social scientists from the fields of conservation biology, fisheries oceanography, marine ecology, marine social science, marine policy, and molecular ecology/conservation genetics. Information for the applications came from multiple sources including a systematic literature review of scientific articles, scientific reports, news reports, and management reports; team members’ participatory and non-participatory observation of management forums and processes; team members’ knowledge based on recent and ongoing research in these systems; discussions with other scientists working on these or related subjects; and outreach and engagement with active participants in the fisheries and associated human communities.
The team held regular meetings over the course of a year. The team explicitly examined each potential variable in each domain to determine if it was sensitive and had exposure to an outcome. While all team members contributed to the analysis of each domain of the system, the social scientists focused on variables within the Human Community and Fishery while the natural scientists focused on variables within the Ecological Community and Fished Species. At each meeting, we discussed how the variables interacted or potentially could have interacted within and across domains.
For each of our case studies, we first describe the physical changes associated with the Trend or Event. We then provide a description of the ecological and social subsystems and their component variables, focusing on those most impacted or with the greatest potential to be impacted. We then present the impacts of the Trend or Event as determined by our analysis, identifying each of the variables considered in bold and noting the exposures it is sensitive to, relevant adaptive capacity, and the ultimate impact on the variable.
Results
Retrospective Case Studies
The North Pacific Marine Heatwave
The 2014–2016 North Pacific Marine Heatwave (MHW) was one of the largest reported ocean warming events on record (Category 3 MHW) (Hobday et al., 2018), persisting for 711 days with temperatures reaching 3 standard deviations above average in the northeast Pacific (Bond et al., 2015). The event arose from a confluence of the 2014–2015 warm anomaly, termed “the Blob” (Bond et al., 2015), and the 2015–2016 El Niño Southern Oscillation (ENSO) event. Anomalously warm sea surface temperatures (SSTs) began in the Gulf of Alaska in November 2013, appeared along the United States West Coast in 2014, and persisted through 2015 (Di Lorenzo and Mantua, 2016; Gentemann et al., 2017). For example, in mid-September 2014, SSTs 32 km off Newport, Oregon increased by 7°C in the span of 1 h and remained warm until June 2015 (Peterson et al., 2015). By the fall of 2014, the upper 50 m of the ocean in southern California was 5°C above average (Zaba and Rudnick, 2016). This coastwide warming was the result of persistent atmospheric pressure systems that modified surface wind and weather patterns (Di Lorenzo and Mantua, 2016) and reduced heat loss from the ocean to the atmosphere (Bond et al., 2015; Zaba and Rudnick, 2016). In the California Current System (CCS), the 2014–2015 warm anomaly was most intense at the surface (0–150 m), but was extended to greater depths by the 2015–2016 ENSO event. ENSO events typically weaken winds favorable for upwelling in the CCS, thereby reducing the vertical flux of nutrients to sunlit surface waters (McPhaden et al., 1998; Alexander et al., 2002). They then modify the interior physical state of the northeastern Pacific, resulting in warm SSTs, deepening of the thermocline, increased southerly source waters (high salinity and nutrient/oxygen depleted), increased sea surface height, and decreased geostrophic flow (Chavez et al., 2002; Checkley and Barth, 2009). These conditions accentuated the extreme warming and associated physical changes of the 2014–2015 anomaly, allowing them to continue into 2016 and extending them to depths up to 500 m (Jacox et al., 2016; Rudnick et al., 2017).
Several of the physical and biogeochemical changes brought about by the MHW were relevant and potentially consequential for species inhabiting the CCS. First, anomalously warm temperatures can affect the physiology of marine ectotherms (Pörtner and Farrell, 2008). Second, altered horizontal advection can affect larval dispersal pathways (Cowen and Sponaugle, 2009) and the distribution of planktonic organisms (Bi et al., 2011). Third, increased stratification can have consequences for the vertical distribution of organisms and resulting predator-prey interactions (Ahlstrom, 1959; Munk et al., 1989). Fourth, although coastwide upwelling was close to average during the MHW (Leising et al., 2015; McClatchie et al., 2016) and anomalously strong in some regions (Jacox et al., 2016; Frischknecht et al., 2017), upwelled water was relatively low in nitrate (Jacox et al., 2018b), leading to reduced primary productivity. Fifth, the warm water pool associated with the MHW compressed cold-water habitat further inshore, leading to the redistribution of many species (Santora et al., 2020). Lastly, there was decreased frontal activity in the central and southern CCS and an associated reduction in primary production (Kahru et al., 2018). These oceanographic changes had substantial repercussions for organisms living in the CCS (Cavole et al., 2016), including the FSESs described in our first two case studies.
California Dungeness Crab FSES
Background
Dungeness crab (Metacarcinus [Cancer] magister) are found from Santa Barbara, California to the Pribilof Islands, Alaska (Rasmuson, 2013). Adult crabs inhabit coastal soft-bottom habitats at depths of 1–230 m (Jensen, 1995), have an estimated lifespan of 8–10 years (Gutermuth and Armstrong, 1989), and produce planktotrophic pelagic larvae. Larval settlement and recruitment to the fishery 4–5 years later is strongly influenced by oceanographic conditions (Shanks, 2013). Juveniles remain in nearshore waters until age 2, then migrate offshore to join adult populations (Collier, 1983; Stevens et al., 1984). Benthic adult crabs are opportunistic feeders on fish, crustaceans and, most commonly, bivalves (Butler, 1954; Gotshall, 1977; Stevens et al., 1984; Lawton and Elner, 1985).
In recent years, California’s commercial Dungeness crab fishery has been among the state’s top fisheries in terms of landings and ex-vessel (dockside) value. In 2018, the fishery accounted for 10.5% of landings and 34.6% of ex-vessel value [18.8 million lbs (8.5 million kg) and $63.6 million, respectively] of statewide commercial fishery activity. Central and northern California fishery participants and communities have become increasingly dependent on Dungeness crab as other fisheries (particularly groundfish and salmon) have declined or become increasingly variable (Pomeroy et al., 2010). Dungeness crabs are caught using baited pot (trap) gear in coastal waters from Crescent City to the Morro Bay-Avila area (Juhasz and Kalvass, 2011) in state and federal waters up to about 73 m (40 fathoms) deep (CDFW, 2019a). Primary management authority lies with the California Legislature, with additional authority delegated to the California Department of Fish and Wildlife (CDFW) to better enable it to respond to emerging management concerns. The Pacific States Marine Fisheries Commission oversees a Tri-State Dungeness Crab Committee that facilitates interstate management cooperation and consultation. Management measures include a minimum size requirement, a ban on the harvest of female crabs, and a 7.5-month fishing season (central management area: November – June, northern management area: December – July) to protect molting animals. A limited entry program was implemented in 1995, with a tiered trap limit program implemented in 2013. Since 1994, the timing of California’s Northern Management Area opener has been subject to the results of pre-season quality testing for meat content, consistent with quality testing in Oregon and Washington under the Tri-State program.
Effort and catch are highly concentrated in the first 4–8 weeks of the season. Fishermen land their catch at ports from the Morro Bay area to Crescent City, with some selling live crab off the boat to consumers but most selling to dockside buyers (first receivers). The catch then may be trucked live to seafood markets (to be sold live, fresh cooked, or cooked and frozen) or processed and distributed domestically and internationally (Pomeroy et al., 2010). As of the early 2000s, about half the catch was processed by a handful of larger seafood businesses (Hackett et al., 2003), although domestic and international markets have varied and changed since then. In addition to buyers, the Human Community includes fishery-support providers (supplying goods and services to support fishing and the seafood supply chain) along with resource managers, shoreside communities, and seafood consumers. The Dungeness crab fishery is part of a larger social system of fishing and seafood production related, in part, to the seasonality of different fisheries and the various fishery combinations that participants pursue (Pomeroy et al., 2010, 2018).
Effects of the marine heatwave
Elevated water temperatures associated with the MHW did not affect the distribution or abundance of adult Dungeness crab, owing to their relatively high temperature tolerance (Des Voigne, 1973). However, the MHW did significantly impact the Fishery and the associated Human Community via two distinct sets of indirect effects on the Ecological Community and associated management responses. Our framework allows us to map these pathways through different elements of the Dungeness crab social-ecological system (Figure 2).
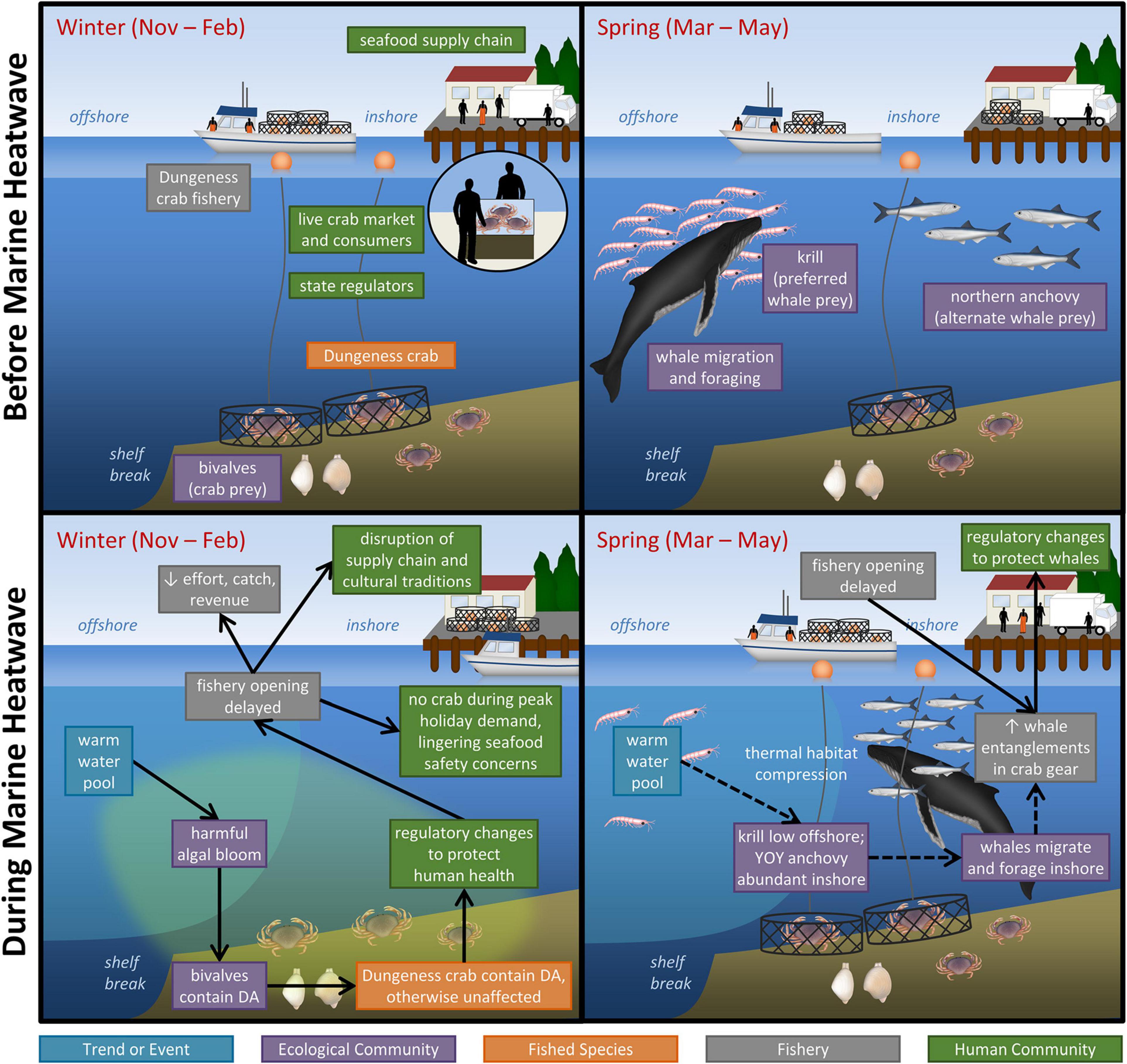
Figure 2. Impacts of the North Pacific Marine Heatwave (MHW) on the California commercial Dungeness crab fishery system. Boxes are color-coded by domain and indicate the major system components (Before Marine Heatwave) and impacts of the MHW (During Marine Heatwave). Arrows connect exposures and impacts. Dashed (vs. solid) arrows indicate the two pathways by which the MHW impacted the fishery system.
First, exposure to nutrient conditions resulting from the MHW (availability of nitrogen and depletion of silicate) affected the abundance and physiological condition of the diatom Pseudo-nitzschia, leading to a harmful algal bloom (HAB) (Ryan et al., 2017). The HAB consisted of greatly increased Pseudo-nitzschia biomass and production of domoic acid (DA). Many species, including bivalves (which consume plankton), and Dungeness crab (which consume bivalves), were exposed to increased DA levels. The condition of both bivalves (in the Ecological Community) and crabs (the Fished Species) was impacted, as they accumulated DA in their tissues through trophic transfer (McCabe et al., 2016). The presence of DA in Dungeness crabs did not negatively affect them physiologically or demographically, however, crab quality (safety to consumers and saleability) was impacted. Specifically, DA levels in Dungeness crab were found to exceed “action levels” established by the United States Food and Drug Administration (30 ppm in Dungeness crab viscera, 20 ppm in the meat) for protecting seafood consumer health. A potent neurotoxin, DA can cause amnesic shellfish poisoning (ASP) in birds and mammals (including humans), with symptoms including vomiting and diarrhea, disorientation, seizure, short-term memory loss and, in extreme cases, death (Pulido, 2008).
Governance, a management component of the Human Community, was sensitive to changes in crab quality, and specifically to toxin levels. Acting on the recommendation of the California Department of Public Health (CDPH), the CDFW took regulatory action, delaying the 2015–2016 season opener up to 7 months in some areas until DA in crab dropped to acceptable levels. Sensitive to these regulations, the timing of the Fishery shifted and compressed from Nov-Feb to March–May. Many Fishery variables including effort, catch, and revenue declined as a result. For the season, both participation and landings declined, with 9.3–13.4% fewer permittees making at least one landing and 25-28% fewer lbs landed than in the previous two and one following seasons (Juhasz and Pomeroy, 2017). Ex-vessel (dockside) prices also fell by 9.6% or more (Mao and Jardine, 2020), and as of early February 2016, ex-vessel revenues to the fishery were estimated at $48.3 million less than the annual average of the same period for the previous five seasons (Gov. Brown letter to Secretary of Commerce Pritzker, February 09, 2016). With captains and crew counting on a timely and productive season to sustain their livelihood, the season delay caused some acute economic hardship.
These impacts to the Fishery in turn had impacts on the economics, social relations, and mental well-being of fishermen, the shoreside seafood supply and support system, and associated central and northern California port communities (Pomeroy et al., 2018; Ritzman et al., 2018). The financial impact on captains and crew prompted community fundraising efforts to provide short-term assistance. Changes in the timing and location of the fishery in turn impacted shoreside seafood unloading, handling, processing, and distribution to consumers. In particular, the temporal shift disrupted the fishery’s ability to meet peak demand during the winter holiday season as well as a strong international market. The delayed start of the fishery also disrupted the larger seasonal pattern of fisheries and associated shoreside support, as spring typically signals the wind-down of crabbing and gearing up for salmon fishing.
The social, economic, and cultural well-being of California communities and consumers was also impacted by the change in season timing and crab quality. For many central and northern California communities, the preparation, sharing, and consumption of Dungeness crab is a cultural tradition for the late fall/winter holidays as well as community gatherings and fundraising events (e.g., “crab feeds”). Although consumer prices did not change once crab became available (Mao and Jardine, 2020), consumers faced a supply shortage during this culturally-important time period, and once the fishery opened, had lingering concern about seafood safety. The closures also reduced the availability of Dungeness crab as a source of nutrition, high in protein and minerals and low in fat.
The MHW impacted the Dungeness crab fishery a second way when the offshore warm water pool associated with the MHW compressed the nearshore cold-water habitat. Members of the Ecological Community exposed to this habitat compression responded with shifts in abundance and spatial distribution: krill decreased in abundance at the shelf break, and young-of-the-year anchovy increased in abundance nearshore (Santora et al., 2020). Both krill and anchovy are prey for humpback whales. Humpback whales are a non-target species of the Dungeness crab fishery that can become entangled in crab trap lines. The whales, having behavioral plasticity in their prey base and foraging location (adaptive capacity), shifted their spatial distribution to more inshore locations during their period of migration through the area (March–May) (Wells et al., 2017; Santora et al., 2020).
Interaction of the first pathway (impacts to fishery timing) with the second (impacts to whale spatial distribution) led to a spatial and temporal overlap in peak whale activity and peak fishing activity. This resulted in perhaps the greatest impact on the fishery, which was a marked increase in whale entanglements in crab gear, a significant monetary and public relations cost to the fishery. Prior to the HAB event, amid growing concern about increases in whale entanglements, the California Dungeness Crab Fishing Gear Working Group, composed of state and federal agencies, fishermen, and NGOs, had developed best practices for the fleet to reduce marine life entanglement (California Dungeness Crab Fishing Gear Working Group, 2016). However, this was insufficient to alter or dampen the pulse of fishing activity and entanglements that occurred once DA levels dropped and the fishery opened. Fishermen who had invested substantial time and money in preparing their gear, vessel, and equipment were eager to get back to work, recoup their investments, and earn their livelihood. The heightened risk of springtime whale entanglements prompted further governance action to close the fishery 2.5–3 months early the following season (i.e., 2016–2017). At the same time, the Working Group developed a Risk Assessment and Mitigation Program (RAMP) to identify and assess entanglement risk and determine the need for management measures to reduce that risk. However, in October 2017, the Center for Biological Diversity sued CDFW and in March 2019 the parties reached a legally binding settlement requiring assessment of entanglement risk at regular intervals throughout the season, with provisions to close the season as early as mid-April. The terms of the settlement provide a mechanism for collective monitoring and deliberate decision-making, which can facilitate adaptation in the Fishery and associated Human Community to changing conditions. However, heightened concerns about whale entanglements and early closures as well as intensified scrutiny by environmental NGOs have adversely affected fishermen’s and crab handlers’ psychological well-being (health) and their expectations and ability to earn their livelihood from the fishery.
Our focus on the impacts of the MHW on California’s commercial Dungeness crab fishery highlights the importance of considering a diversity of climate change impacts, both direct and indirect, on the fishery system. In this case study, impacts to the Fishery and Human Community were due not to changes in the abundance or distribution of Dungeness crab, but were the result of regulatory actions by the state, first to delay the opening of the season and then to open the season by area, in order to protect public health from toxins in the crab. CVA approaches focused exclusively on direct impacts to the Fished Species would not have captured this. This case study also illustrates the critical importance of linkages and feedbacks among ecological (Ecological Community and Fished Species) and social (Fishery and Human Community) domains. In particular, the use of the framework here brings into sharp relief the complexity — and potential for unintended consequences — of managing for both ecological and human health.
Northern California Red Sea Urchin FSES
Background
Red sea urchins (Mesocentrotus franciscanus) inhabit shallow rocky reefs, typically at depths of 5–20 m. In northern California, red urchins are abundant on reefs that support their primary food source, bull kelp, Nereocystis luetkeana. Urchins passively feed on blades of bull kelp that fall to the benthos as “drift,” and shift to active grazing on kelp, other macroalgae, and invertebrates when either drift kelp availability or their predators are reduced (Cowen, 1983; Harrold and Reed, 1985). Their primary competitor is the purple sea urchin, Strongylocentrotus purpuratus. Although purple sea urchins are also harvested for food, their smaller size makes them less desirable (CDFW, 2019b). In northern California, the sunflower star, Pycnopodia helianthoides, is the primary predator of both red and purple urchins. Red sea urchins are broadcast spawners with a planktotrophic larval stage. Larvae settle and find refuge from predation in structurally-complex microhabitats and mature within a few years. Adult red urchins have low mortality (Ebert and Russell, 1993; Ebert, 1996) and the ability to live for many decades (Ebert and Southon, 2003).
The commercial red sea urchin fishery began as an open access fishery in California in the early 1970s. Following rapid growth, the state instituted a permit requirement in 1985, followed by restricted access in 1989 (CDFW, 2019c). In 2015–2016, the state issued about 300 urchin dive permits, 150 of which accounted for the vast majority of landings. Management measures also include minimum size rules and weekend closures during part of the year. The northern California fishery (north of San Francisco Bay) accounted for 31.3% (3.7 million lbs and 1.7 million kg) of statewide commercial red sea urchin landings in 2014 (CDFW, 2019c). Fort Bragg (Noyo Harbor), where the two primary urchin processors for this region are located, is the center of this activity and accounts for the vast majority of northern California urchin landings (Pomeroy et al., 2010). Statewide, red urchin is consistently one of California’s top fisheries by volume (CDFW, 2019c).
Historically, divers using surface-supply air (“hookah”) and hand rakes have fished for urchin at depths of 3–15 m from small vessels (6–9 m length), departing and returning to port daily. Divers evaluate urchin size and gonad quality (the only part of the urchin that is consumed) while underwater. The divers collect the selected urchins in a mesh bag, which a tender (crew) hoists aboard the fishing vessel. The price paid to the diver for live urchins, how the urchin roe (uni) is processed, and where the roe is distributed depends on the quality of the gonad (Kato and Schroeter, 1985). Although the vast majority of the exports are sent to Japan, there is a growing market for urchins in the United States, especially in California (Sun and Chiang, 2015; Sonu, 2017). While some of the product is sold live, much of it is sold fresh following highly specialized, labor-intensive processing, which occurs at or near ports where the catch is landed. In northern California, these ports are located in small isolated communities where both fishermen and processors reside.
Effects of the marine heatwave
The 2014–2016 MHW had severe negative impacts on the red sea urchin fishery in northern California, which included sharp declines in catch and revenue (Rogers-Bennett and Catton, 2019). Application of our framework suggests that these declines resulted from a suite of indirect effects on the shallow rocky reef ecosystem that supports red sea urchins, rather than direct effects on the abundance of red sea urchins. The effects of the MHW were indirect and came about through two interacting pathways affecting the Ecological Community (Figure 3).
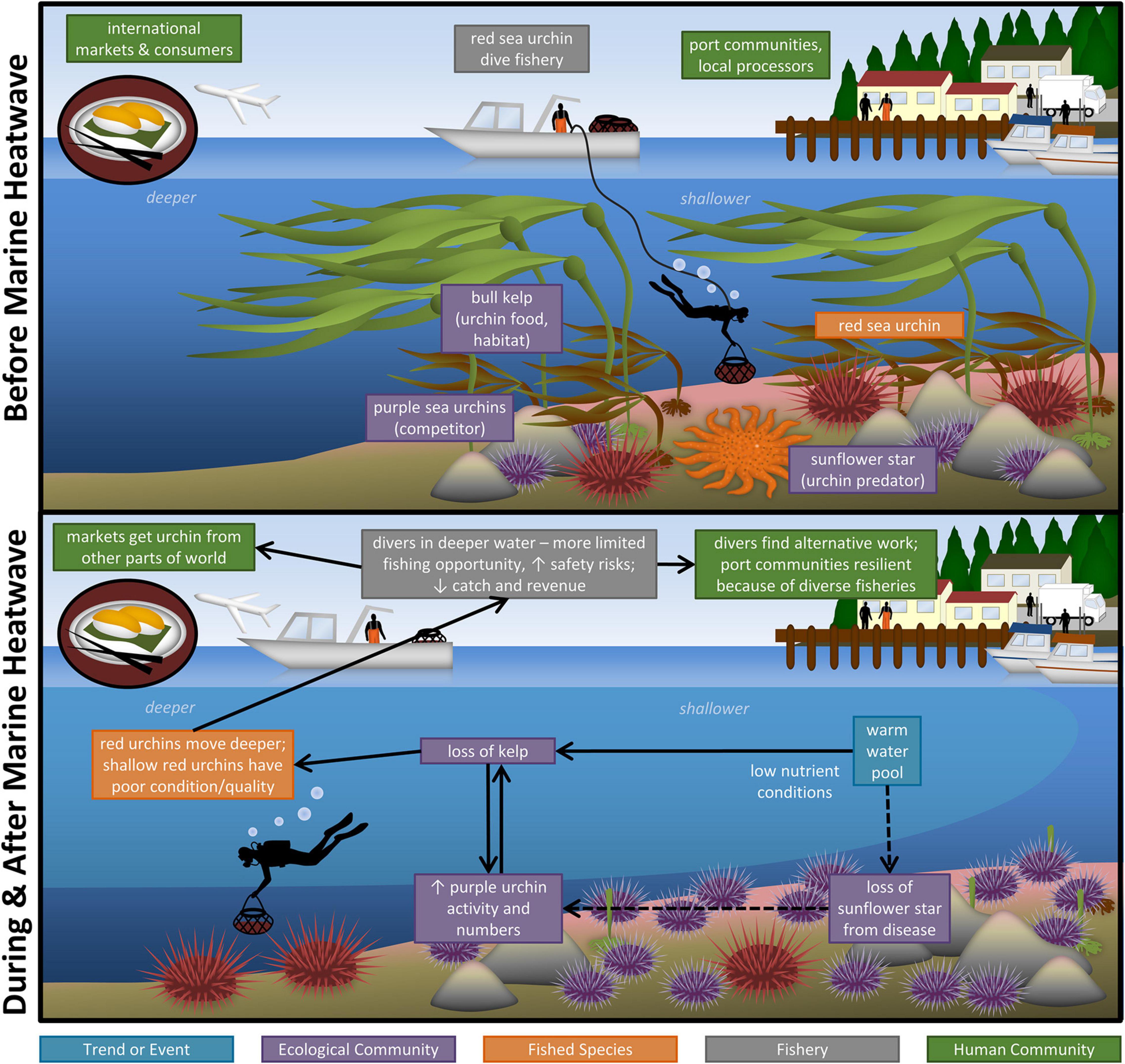
Figure 3. Impacts of the North Pacific Marine Heatwave (MHW) on the northern California commercial red sea urchin fishery system. Boxes are color coded by domain and indicate the major system components (Before Marine Heatwave) and impacts of the HWM (During and After Marine Heatwave). Arrows connect exposures and impacts. Dashed (vs. solid) arrows indicate the two pathways by which the MHW impacted the fishery system.
First, there is evidence, albeit equivocal, that increased water temperatures facilitated the sea star wasting disease epizootic in the northeast Pacific, leading to high rates of mortality and dramatic declines in abundance of the sunflower star (Hewson et al., 2018; Harvell et al., 2019). Sunflower stars had little resistance to the disease and became functionally extirpated throughout large expanses of the red urchin’s range, showing little sign of recovery. Loss of their primary predator and the lack of alternative predators of large urchins in northern California (e.g., fish, lobsters, and otters), resulted in a trophic release of both red and purple urchins. No increased prevalence of disease in the urchins themselves was observed during the MHW.
Second, nutrient (nitrate) levels are inversely related to water temperature, and temperatures during the MHW exceeded the threshold at which nitrate levels become insufficient for kelp production (Zimmerman and Kremer, 1984; García-Reyes et al., 2014; Jacox et al., 2018a). Warming of the upper 150 m of the water column also increased stratification and suppressed nutrient concentrations despite normal upwelling indices (McClatchie et al., 2016). These low nutrient conditions resulted in a decline in bull kelp productivity and the availability of drift kelp, the main prey of red and purple urchins.
These two pathways impacted the behavior and abundance of the red sea urchin’s primary competitor, the purple urchin. Exposure to reduced availability of prey, combined with reduced predator abundance led to a shift in purple sea urchins’ foraging behavior, from a passive detritivore restricted to cracks and crevices to an active grazer, denuding the reef of almost all macroalgae (Rogers-Bennett and Catton, 2019; McPherson et al., 2021). This behavioral response was also accompanied by a numerical response: overgrazing by purple sea urchins led to shifts in the macroalgal assemblage from one dominated by kelps and foliose algae to one dominated by grazing-resistant crustose (and erect) coralline algae (CCA). CCA is a cue for larval urchin settlement, generating a numerical positive feedback. In addition, increased rates of purple urchin larval recruitment in 2014 and 2015 corresponded with increased water temperatures associated with the MHW (Okamoto et al., 2020). The behavioral response and persistent numerical increase in purple urchins (Baskett and Salomon, 2010; Karatayev and Baskett, 2020; Okamoto et al., 2020) led to further declines in kelp abundance and a widespread shift from kelp forests to “urchin barrens.” Kelp forests and urchin barrens are classic examples of alternative ecosystem states (Filbee-Dexter and Scheibling, 2014; Ling et al., 2015), and kelp forest ecosystem shifts have been associated with MHWs around the world (Wernberg et al., 2013, 2016; Smale, 2020). Since bull kelp has an annual life history and the spores are not persistent, populations exhibit little adaptive capacity and recovery is inhibited by high rates of herbivory by purple urchins, which can persist at high densities without starving for many years.
With respect to the red urchin itself, exposure to declines in their prey (i.e., the loss of kelp and other macroalgae resulting from low nutrients and overconsumption by competing purple urchins) had several impacts. Like purple urchins, red urchins have an uncanny ability to resist dying from starvation (Ebert, 1967; Pearse, 1970), an adaptive capacity that prevented declines in abundance and size of the urchins. However, lack of food inhibits energy allocation toward reproduction and gonad development. As a result, northern California red urchins experienced a widespread decline in gonad condition (volume, color, and other characteristics), and thus quality (desirability and market value). Water temperatures above the upper threshold for gametogenesis [e.g., 17°C for purple sea urchins; (Cochran and Engelmann, 1975)] may also have inhibited gonad production. Otherwise, there is little evidence of a direct physiological or population effect of the increased water temperatures associated with the MHW on red urchins.
The spatial distribution of red urchins was also impacted by loss of their primary food resource. The mobility (Mattison et al., 1976) and generalist diet (Briscoe and Sebens, 1988; Hughes A. D. et al., 2012) of red urchins allows populations to track local food availability and avoid areas overgrazed by purple urchins. During the MHW, red urchins redistributed from shallow (3–15 m) to deeper (20–30 m) depths, where purple urchins are less abundant and alternative prey resources, e.g., subtidal barnacles, are more available (Trumper, pers. comm.). Only at these deeper depths did red urchins have gonads of harvestable quality (Trumper, pers. comm).
These impacts to red urchins devastated the northern California fishery. The movement of red urchins to greater depths greatly reduced the amount of time divers could spend harvesting underwater each day, affecting fishing opportunity and location. It also affected safety by greatly increasing divers’ risk of accidents and decompression sickness. In combination with the widespread decline in sea urchin gonad condition and quality, this led to a dramatic decline in catch and revenue. Prior to and at the onset of the MHW (2010–2014), Northern California’s annual red sea urchin landings consistently ranged between 3 and 4 million lbs (0.9–1.4 million kg), but declined sharply from 2015 to 2019, with a concomitant decline in ex-vessel (dockside) value. In 2016 and 2017, ex-vessel revenue in the fishery’s Northern Management Zone fell by 76 and 78%, respectively compared to the previous 5-year average.
Although the dive fishery is highly specialized, it nonetheless has long-term adaptive capacity and resilience. As urchins shifted to deeper depths, precluding diving using surface-supplied air, some divers continued to fish using SCUBA gear. Others shifted to different locations (e.g., Southern California, Alaska) and/or fisheries (e.g., blackcod, sea cucumber, salmon, and nearshore rockfish) or left fishing for other work (reduced effort) not impacted by the MHW. These changes were contingent on environmental, regulatory, and market conditions, and provided urchin fishermen have the resources needed to pursue these options. Some divers intend to return to the fishery as soon as the species becomes marketable again, provided they have the financial, social, and technological resources to do so - and a buyer for the catch. The red urchin itself is also resilient over the long-term. Once their food source recovers, the large number of surviving red urchins should rapidly redistribute to shallower depths and reallocate energy to gonad development, thus recovering their market value (Teck et al., 2018).
While changes in the Fished Species population did not have an impact on the health or nutrition of the Human Community, the associated port community was affected through economic impacts to the shoreside fishery and seafood support system. With the sharp reduction in local urchin harvest, processors had limited access to alternative sources of urchin (which must be processed within hours of being brought to port). Processing employees had to find work in other fisheries or sectors of the economy. Some had the adaptive capacity to do so, as Fort Bragg has a diversity of fisheries (groundfish, salmon, and crab) and a stable tourism industry, affording a mix of opportunities for the labor force (Juntz, pers. Comm.). However, while this flexibility serves the shoreside support system during smaller seasonal disruptions, as typically occur in spring when storms sometimes remove the kelp or make diving particularly dangerous, the damage caused by the 2014–2016 MHW is ongoing. Urchin processors, whose operations are highly specialized and lack the flexibility to process alternative species, suffered economic impacts including forced downsizing and liquidation of some assets. Meanwhile, the supply chain disruption has been exacerbated by divers with reduced catches choosing to sell directly to farmers markets or restaurants, bypassing processors and wholesalers altogether. Impacts on consumers in California and abroad were minimal because of the availability of alternative sources of urchin and overall, initial impacts to the Human Community were marginal but likely will increase as the duration of this event continues.
These impacts, in turn, prompted a request by the state Governor for, and the United States Secretary of Commerce approving, a federal fishery disaster declaration for the 2016–2017 Northern California red sea urchin fishery. In 2020, the United States Congress appropriated $3.3 million in disaster relief assistance. The proposed spending plan for those funds includes using a portion of the funds to pay fishermen to assist with efforts to remove purple urchins to better enable kelp restoration1. However, this governance response was insufficient and followed long after the impacts of the event.
Like the Dungeness crab fishery, the detrimental effects of the MHW on the northern California red sea urchin fishery did not result from direct (e.g., physiological and demographic) impacts to the urchin itself, but from several indirect ecological interactions that affected urchin condition and led to declines in market value. Interestingly, the characteristics of adaptive capacity shared between purple and red urchins (resistance to starvation, generalist diet, and long lifespan) contribute both to the persistence of the current ecological impacts to the Fishery and the long term persistence of the Fished Species. Furthermore, the opportunity and flexibility of urchin fishermen to move to alternative fisheries or local sources of income revealed their adaptive capacity and potential resilience to wait out the recovery of the condition of the urchin. In contrast, urchin processors’ operations and markets are highly specialized, limiting adaptive capacity, and making them more vulnerable to the impacts of the MHW. This case study also underscores the importance of the spatial scale of an assessment. While upper-ocean warming during the MHW was generally similar in northern, central, and southern California, the ecological manifestations and fishery repercussions were markedly different. For a variety of reasons (e.g., species of kelp, alternative sea urchin predators), the kelp forest ecosystem and sea urchin fishery in southern California did not experience the ecological responses that undermined the northern California fishery.
Prospective Case Study
North Pacific Albacore Tuna FSES
Background
North Pacific albacore tuna (“albacore”) spawn in the western and central North Pacific (10–25°N; 120°E–155°W) from March-September, with peak spawning in March–April (Chen et al., 2010). Some larvae are likely advected westward into the southern limb of the Kuroshio Current where they grow quickly and by age 2 are capable of swimming vast distances (Suda, 1966). A portion of the juvenile population (ages 2–4) then utilizes the North Pacific Transition Zone (NPTZ) and North Pacific Current (NPC) to migrate eastward to their summer feeding grounds in the CCS (Polovina et al., 2001, 2017). Seasonal and interannual variability in the latitude of the NPTZ and NPC (Ayers and Lozier, 2010; Sydeman et al., 2011) may impact albacore migration pathways, the proportion of fish that reach the CCS, and residence time in the CCS. In the CCS, albacore feed on young-of-the-year northern anchovy, Pacific saury, North Pacific hake, Pacific sardine, and rockfishes, as well as cephalopods, euphausiids, and myctophids, with a high proportion of their energy intake coming from finfish such as northern anchovy and Pacific saury (Glaser, 2010, 2011; Glaser et al., 2015). Albacore concentrate their feeding activity at fronts (Nieto et al., 2017; Snyder et al., 2017; Xu et al., 2017), which have high prey concentrations (Franks, 1992; Sato et al., 2018) and allow albacore to increase their foraging efficiency through behavioral thermoregulation (Bakun, 2006). Albacore prefer SSTs between 11 and 20°C, below which they are unable to maintain optimal body temperature, and above which they incur high behavioral and metabolic costs (Snyder, 2016). Juvenile albacore are most abundant in the CCS from June through September. A portion then migrate offshore to overwinter in warmer waters of the central Pacific, while others may be largely resident off the Baja Peninsula throughout the year (Childers et al., 2011; Snyder, 2016). Albacore reach maturity by age 5 or 6 (83–93 cm fork length) (Wells et al., 2013; ISC, 2017) after which they migrate to the subtropical western and central North Pacific where they remain (Otsu and Uchida, 1964; Laurs and Lynn, 1977; Ichinokawa et al., 2008).
In the tropical and subtropical North Pacific, albacore are targeted by 35 different commercial fisheries defined by gear type, region, season, and participating nation (Teo et al., 2020). The Albacore Working Group of the International Scientific Committee conducts periodic assessments of North Pacific albacore to estimate biomass and status, and to provide scientific advice to regional fisheries managers. Since 2005, the United States West Coast fishery for albacore, which uses troll and pole-and-line gear, has been managed under the West Coast Highly Migratory Species (HMS) Fishery Management Plan (FMP) developed by the Pacific Fisheries Management Council2. Participation in this fishery requires a permit and catch documentation, but is open access, with no limit on the number of participants or the catch. Since 1981, the United States–Canada Albacore Treaty has allowed each country’s fishing vessels to fish in the partner country’s waters seaward of 12 miles (∼20 km) from shore and to land catch at specified ports. For the period 2010–2018, the United States commercial troll and pole-and-line fishery for albacore ranked third in ex-vessel value in Oregon and Washington, following Dungeness crab and pink shrimp (Frawley et al., 2020). Further, albacore is the most active HMS fishery along the United States West Coast in terms of landings (7,583 round mt in 2019), number of participating vessels (539 in 2019) and ex-vessel revenue ($27.8 million in 2019)3. North Pacific albacore biomass has remained relatively stable over recent years and population assessments suggest the species is not overfished (ISC, 2017, 2020), however, the availability of albacore to the United States fishery varies, and oceanographic conditions are believed to be the primary driver (Phillips et al., 2014).
Fishing for albacore by United States vessels typically occurs from late June through October, with peak catches occurring in August and September. Typically, albacore fishermen troll for albacore at the surface in “clear-warm-blue” water early in the season and then use a combination of live bait and iron/jigs later in the season (Nancy Fitzpatrick, pers. comm.). Fishermen selectively fish at coastal thermal fronts detected using satellite sensors (Watson et al., 2018). Most fish caught are in the 15–25 lbs (∼7–11 kg) range, but at times smaller albacore (“peanuts”) in the 6–10 lbs (3-4.5 kg) range are caught. The catch is landed at ports along the Washington, Oregon, and northern California coast, then shipped overseas or processed locally for domestic markets. Albacore fishermen sell their catch either direct-to-consumer, to smaller integrated businesses (e.g., micro-canners), or to larger canning and other processing operations. Like many other fishermen, albacore fishermen do not rely solely on albacore for their livelihood (Frawley et al., 2020). Rather, they have a portfolio of fisheries, participating in each based on resource seasonality, availability, and regulations among other factors. Many United States commercial troll and pole-and-line fishermen also participate in the United States West Coast salmon troll fisheries, which runs from late spring through summer; the Dungeness crab fishery, which runs from late fall through early spring (see above); and to a lesser extent, groundfish (largely sablefish or blackcod), which can be fished year-round (Radtke and Davis, 2000). With some overlap of the salmon and albacore fishing seasons, fishermen typically target salmon earlier and then switch to albacore, deciding whether and when to switch contingent on the availability and profitability of the different species, among other factors.
Projected climate change manifestations in the CCS in relation to albacore
Several predicted oceanographic changes in the CCS are relevant to albacore and the fishery. Global Climate Models (GCMs) project maximum monthly mean SST will increase ∼3°C by the year 2095 (Alexander et al., 2018). How coastal wind-driven upwelling in the CCS will change is more uncertain. Some studies suggest that upwelling will increase, potentially offsetting increased surface heating (Bakun, 1990; Bakun et al., 2015). Others predict increased (decreased) upwelling in the northern (southern) areas of upwelling systems (Rykaczewski et al., 2015). GCM projections unequivocally show a poleward shift in westerly winds (Yin, 2005; Bengtsson et al., 2006; Archer and Caldeira, 2008) which will shift the North Pacific Current (NPC) to more northern latitudes (Toste et al., 2019). A more northern NPC bifurcation results in a greater proportion of cooler subarctic water enriched in nutrients and oxygen propagating downstream into the CCS (Chelton et al., 1982; Lynn and Simpson, 1987) and may increase ecosystem productivity in the northern CCS (Parrish et al., 2000; Sydeman et al., 2011). Across the CCS, increased temperatures and stratification are projected to lead to ocean deoxygenation (Levin, 2018; Howard et al., 2020), although the impacts may not be uniform along the coast (Cheresh and Fiechter, 2020). The interplay between future ocean warming, increased stratification, coastal upwelling, and changes in source water contributions and biogeochemistry, is complex and many uncertainties exist as to how this will impact ecosystem structure and function. Further, it is unclear how the presence of coastal thermal fronts will change, but if the 2014–2016 MHW is analogous to the impacts of climate change, then it is possible that thermal fronts will be reduced (Kahru et al., 2018).
We consider how these long-term Trends in climate will directly and indirectly affect the Ecological Community, Fished Species, Fishery, and Human Community of the CCS North Pacific albacore fishery system (Figure 4). As there are few direct studies on the impacts of anthropogenic climate change on albacore in this region (Hazen et al., 2013; Christian and Holmes, 2016), we sourced our information through discussions with experts and stakeholders as well as through a literature review of contemporary albacore ecology, fisheries oceanography, stock assessments, and social-ecological studies.
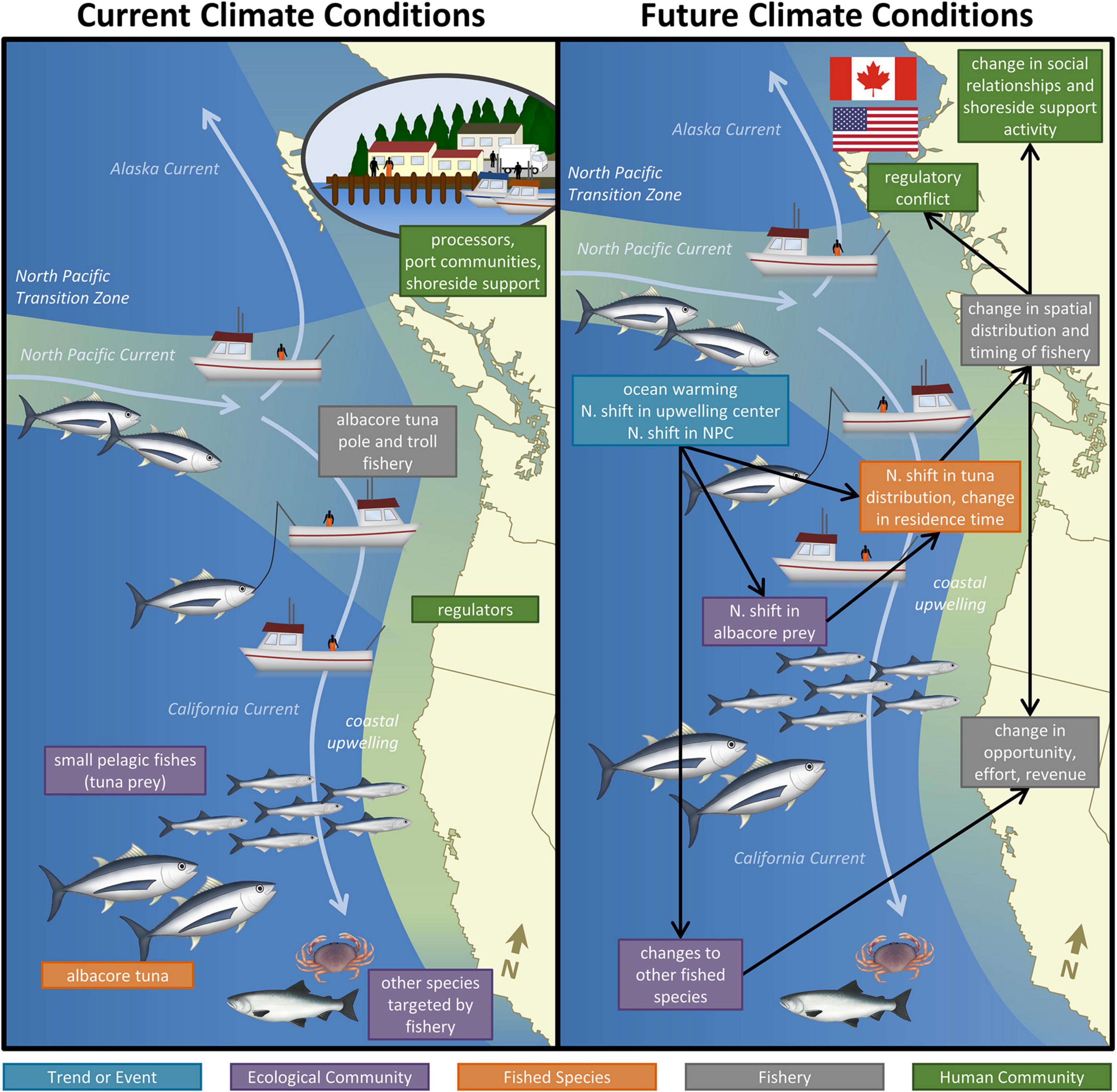
Figure 4. Potential impacts of the climate change on the United States West Coast albacore tuna fishery system. Boxes are color coded by domain and indicate the major system components (Current Climate Conditions) and impacts of climate change (Future Climate Conditions). Black arrows connect exposures and impacts.
Effects of climate change
Within the Ecological Community, the spatial distribution and abundance of albacore prey species are likely to be sensitive to changing temperatures, upwelling dynamics, and ocean biogeochemistry. For example, coastal pelagic species such as northern anchovy, a key prey species of albacore (Glaser, 2010), exhibit large fluctuations in abundance (Baumgartner, 1992; McClatchie et al., 2017) and are highly sensitive to changes in the thermal environment (Checkley Jr et al., 2000; Lindegren et al., 2013). Increasing ocean temperatures and northward shifts in upwelling favorable winds (Rykaczewski et al., 2015) are anticipated to shift sardine and anchovy farther north (Checkley et al., 2017), along with other key albacore prey such as juvenile rockfish and Pacific hake (Auth et al., 2017; Morley et al., 2018; Malick et al., 2020). Less known is how a warmer CCS will shift the distribution of other albacore prey such as Pacific saury, euphausiids, amphipods, and cephalopods. Increases in ecosystem productivity correlated with northward shifts in the NPC (Sydeman et al., 2011) may increase the abundance of key albacore prey species in the CCS. Ocean deoxygenation is expected to decrease the abundance of the mesopelagic (mid-water) prey community in the CCS (Koslow et al., 2011, 2013, 2019), but also move these species closer to the surface where they may be more available to visual predators such as albacore (Netburn and Koslow, 2015).
The changing ocean environment will likely also impact other species albacore fishermen target (e.g., salmon and Dungeness crab). Although we do not explore these changes here, how these species and their FSESs will change needs to be considered when trying to assess the full impact of climate change on the albacore fishery. As albacore have few predators and the Fishery has a very low rate of bycatch (non-target species), these components were not considered further. While albacore share the CCS with many competitors for the same prey types, there is considerable uncertainty as to whether competition limits albacore distribution and abundance (Koehn et al., 2016), and we therefore did not consider competitors further.
In terms of the Fished Species, several exposures are predicted to result in northward shifts in the spatial distribution of albacore. First, albacore are sensitive to the changes in the thermal environment, and will likely move to stay within their preferred temperature range (11–20°C). Albacore distribution models predict large-scale northward shifts, with declining occurrences off California and increasing occurrences off British Columbia and Alaska (Hazen et al., 2013; Phillips et al., 2014; Christian and Holmes, 2016). Second, albacore may track northward shifts in the distribution and productivity of their prey. Since albacore are opportunistic predators (Glaser, 2010, 2011; Glaser et al., 2015) feeding on >580 distinct taxa (Hardy et al., pers. comm.), it is unlikely their distribution will change in response to a particular prey taxon, however, they may be sensitive to large-scale shifts in the prey community. The relative importance of temperature and prey availability for albacore is unclear and is an active area of research (Future Seas, 2021). Third, northward shifts in the NPC (Toste et al., 2019) may shift albacore’s trans-Pacific migration pathways north, leading to fish entering the system at higher latitudes.
Northward NPC shifts may also affect albacore temporal distribution, potentially extending their residence time in the CCS by increasing prey productivity (Sydeman et al., 2011). Changes in the location of fronts, which are preferred feeding locations for albacore, will also affect their local spatial distribution. How the longitudinal distribution of albacore will change in the future is less well known; however, during warm conditions in the past, such as the MHW, albacore have been pressed closer to the North American coast (Frawley et al., 2020). This movement could be the result of habitat compression as discussed in the Dungeness crab case study (Santora et al., 2020), which is a pattern that could continue in the future. Fish condition, in terms of body mass per length, may or may not be impacted; however, some fishermen reported declining fish condition during the MHW (Barbara Muhling, pers. comm.) and this may continue with climate change. Impacts to other variables such as abundance may be buffered by the species’ wide-ranging migratory behavior, broad temperature tolerance, generalist diet, and behavioral plasticity, which are aspects of adaptive capacity.
The location and timing of the Fishery is sensitive to changes in the spatial and temporal distribution of albacore. If albacore forage in the CCS for a longer time period, this may prolong the timing of the Fishery. If albacore are located farther north, then United States vessels that typically operate in northern California, Oregon, and Washington may need to travel farther north to catch albacore. These changes in Fishery location will increase operating costs, potentially decrease revenue, and also affect effort, opportunity, and safety if vessels must travel farther from their home ports. There are differences among commercial fishery participants in their ability and willingness to travel farther offshore, and if tuna were to shift across international boundaries, the ability of fishermen to follow albacore may be limited by regulations in different jurisdictions (governance). Declines in fish condition may also impact revenue.
Since albacore fishermen typically participate in other fisheries, effort in the Fishery is also sensitive to changes in the availability of alternative species to fish both before (e.g., Dungeness crab) and during (e.g., salmon and groundfish) the season. Smaller vessels, which tend to have a more diverse portfolio (Frawley et al., 2020), would be disproportionately affected if losses in the albacore fishery could not be offset by alternative fisheries (e.g., salmon). Such participation in diverse fisheries is an adaptive strategy, so it is important to understand the particular mixes of fisheries that people are engaged in and the circumstances under which they shift among fisheries.
The expected timing and location changes in the fishery also would affect social relationships among fishermen, between fishermen and buyers, and between fishery participants and shoreside communities. Groups of fishermen often have long-standing relationships, through which they share information to help each other locate the fish and address other common needs. Such groups could help fishermen adapt to shifting distribution of the fish - or they could be strained as some fishermen are unwilling or unable to follow the fish beyond the range they typically have fished. Climate change could also cause spatially-variable disruptions to the way in which albacore is bought and sold. For fishermen who handle their own catch through off-the-boat or other direct sales, increased accessibility of albacore can enhance those sales directly by attracting customers with this widely valued species. On the other hand, reduced accessibility can diminish this opportunity for securing a strong customer base. For smaller, locally based seafood handlers, the implications of a shifting albacore resource are similar. In both cases, ties to particular communities suggest they would be particularly sensitive to changing resource availability. Larger canners also may be sensitive to such changes, but typically rely on a larger number and variety of fishing operations and have (access to) larger transportation that afford access to catches landed at more distant ports as well as capacity to distribute finished products. For each of these types of arrangements, changes in where, when, and how the catch is unloaded affect the larger social and economic networks among people, businesses, and communities in the FSES. For example, increasing or decreasing activity at a port would affect demand and support for infrastructure (e.g., berths and hoists) and goods and services (e.g., fuel and ice), with repercussions for relationships and viability as described in the other vignettes.
The bait fishery associated with the late-season albacore fishery also deserves consideration. Opportunity for pole-and-line bait fishing may be impacted by the availability (distribution and abundance) of bait species such as northern anchovy. The condition of albacore when they enter the CCS may also affect gear usage – if albacore are hungrier, then the use of bait may not matter until much later in the season. Changes in gear and related fishing practices, such as a shift away from using live bait, also would impact bait providers, whose presence at ports increases access to bait used in both the albacore and other (e.g., salmon) commercial and recreational fisheries. This could affect those fishery participants and their use of the associated shoreside support system, potentially increasing demand for and use of gear – and participation in other fisheries - that do not use bait. Such a gear change also could affect the timing of activities at shoreside communities, with implications for other, established uses – though these, too, might change.
Shifts in the location of the Fishery also have the potential to lead to regulatory conflict (governance), particularly across international boundaries. The reciprocal fishing agreement between the United States and Canada would be threatened if tensions between these fleets increase due to climate change. Institutional arrangements for natural resource (fishery) governance and seafood handling, distribution, and trade differ among localities, states, and countries. Fishery participants and the Fishery as a whole likely would need to adapt to these different arrangements, learning the rules, acquiring permits, and modifying their practices accordingly. Enhanced coordination across natural resource and food systems institutions within and across jurisdictions would be needed.
Our prospective application of the framework to North Pacific albacore in the CCS shows that there are many uncertainties as to how climate change will specifically impact albacore, but that there will likely be both positive and negative impacts to the species and the United States troll and pole-and-line Fishery. Reducing the uncertainties within the four domains will require additional research. A comprehensive assessment of albacore will require in depth knowledge of climate impacts on albacore prey species, something that can be augmented by other, more quantitative modeling approaches (e.g., Plagányi et al., 2014). We recommend future research on environmental drivers of albacore longitudinal distribution as this will affect the revenue and costs associated with transiting to fishing grounds as well as the safety of fishermen. Further, impacts to alternative species within the albacore portfolio of fisheries (e.g., salmon) should be assessed within the framework to understand the future decisions albacore fishermen will need to make. This case study also highlights how the social dimensions of the framework can be used to anticipate potential interjurisdictional conflicts.
Discussion
We present a framework for evaluating climate change impacts on complex FSESs. The indirect effects and impacts of recent climate events on FSESs, which were mediated through interactions and regulatory changes inspired the framework. The framework divides the FSES into four domains, each of which may contain multiple components. Each component has variables that a climatic Trend or Event may impact directly or indirectly. This framework systematically accounts for feedbacks, links between subsystems and domains, and multiple climate change-induced stressors. The framework is also generalizable in that users can select the temporal and spatial scale of the Trend or Event, whether the analysis is retrospective or prospective, the focal component and domain, and other limits to make analysis as tractable and focused as needed. Through three case studies, we demonstrate the framework’s ability to more thoroughly account for the complex interactions within fishery systems.
The cascade of impacts in our two retrospective case studies shared several commonalities. In both cases, there was no evidence that the increase in temperature directly affected the Fished Species. Rather, the effects on Fished Species were at least three interactions removed from the Trend or Event and occurred via multiple interactions. In both cases, the impact was to the Fished Species’ condition, and thus quality for market and not their abundance. Changes in spatial distribution were important in each (e.g., shift of red urchins to greater depths, shift of whale foraging inshore), however, temporal shifts were more important in the Dungeness crab fishery. Both also involved interactions between “non-adjacent” domains (e.g., whales from the Ecological Community directly affected the Fishery in the Dungeness crab case study). Reductions in fishing opportunity occurred in both cases, but for different reasons: in the case of Dungeness crab, governance action to protect whales and public health; in the case of red urchin, changes to the spatial distribution of the Fished Species and diving safety concerns. Governance played a role in the urchin fishery as well, providing fisherman an alternative target species through a grant program. In both cases, shoreside processors suffered economically, however, the high level of specialization among urchin processors made them particularly sensitive to the exposures. These commonalities illustrate how Trends or Events often affect systems in complex ways, and a framework capable of capturing these complexities is valuable. Whether the observed impacts would have been predicted by a prospective application of the framework to these same systems before the MHW is difficult to say. It undoubtedly would not have predicted the precise events, but may have helped stakeholders envision the broad array of possibilities that an event like the MHW could produce, and potentially enable a smoother response.
Comparing our prospective case study of the albacore fishery system to our retrospective case studies reveals several similarities and differences. Like urchin, albacore are generalists, but may be susceptible to shifts in their overall prey base. Some albacore buyers and processors, like urchin processors, are specialized, but like many crab processors, may have greater capacity to adapt to changing fishery dynamics. In contrast to Dungeness crab and red urchin, changes in temperature may directly affect the temporal and spatial distribution of albacore, and Fishery participants are relatively flexible, participating in multiple fisheries throughout the year and shifting among them as ecological, regulatory, and socioeconomic conditions require. They also are relatively mobile especially compared to urchin divers. Among key factors in that mobility is the management of the fishery under a West Coast-wide FMP without state-specific measures. The interjurisdictional management of the fishery (across national boundaries) adds complexity distinct from the other two cases. Finally, the strong interactions between both the ecological and human dimensions of the albacore fishery with salmon, Dungeness crab, and groundfish FSESs highlights the need for assessments that integrate multiple FSESs simultaneously. Although beyond the scope of this paper, this framework could accommodate complex interdependencies of multiple Fisheries and Human Communities.
Had the FSES CVAs (or locally relevant analogs) listed in Table 1 been applied to our three case studies, eight of them would have used a predefined set of measurable environmental and social metrics to produce an index of vulnerability to the whole system, three are not applicable to systems this size, while two (Pecl et al., 2009; Chavez et al., 2017) have systems more in the vein of our methodology but take a broader and less step-wise approach than our framework. Thus, we think these other CVAs would be unlikely to anticipate the complex interactions that occurred in these case studies, while some even lack the capacity to capture such interactions. However, these previous FSES CVAs are the conceptual inspiration for our framework. The four aspects (Exposure, Sensitivity, Adaptive Capacity, and Impact) are derived from the most commonly used system (IPCC, 2001) as seen in many FSES CVAs (e.g., Grafton, 2010; Mamauag et al., 2013; Ekstrom et al., 2015). While our framework employs similar terms to many CVAs, we use some in slightly different ways. Sensitivity often means the degree to which something can be affected, whereas in our framework we use the term Sensitive Variables to note component attributes that an Exposure could impact. The main deviation from previous CVA methods is that our framework links together individual component variables and looks at them as a network of interactions that results in a set of outcomes. This change necessitates using the concept of Impacts as opposed to the more traditional “vulnerability,” which is often used in respect to the entire system. Additionally, this means we do not report a vulnerability score, but rather a list of impacts. As such, it may be tempting to classify this framework as a Climate Impact Assessment rather than a CVA. However, other than sharing the use of a term, our framework has key characteristics of a CVA in that it considers adaptability of the system, integrates social and ecological systems, and acknowledges uncertainty. As such, our framework is largely in line with a CVA approach.
While this work develops a CVA-type framework, there are alternatives for assessing the threat of climate change on fishery systems. Examples include simple analytical models (Cisneros-Mata et al., 2019), complex models combined with professional judgment [IFRAME (Zhang et al., 2011)], ensembles of fisheries models linked to climate change models [fish-MIP (Tittensor et al., 2018) or models of intermediate complexity (MICE) (Plagányi et al., 2014)], and Ecological Risk Assessment for the Effects of Fishing (Hobday et al., 2011). As these methods are more quantitative and require modeling system interactions, they may not be applicable in more data poor systems or system domains, and may have a limited ability to capture complex or novel climate-driven interactions. Our framework, in contrast, allows stakeholders to map out a broad set of potential outcomes and thus be better prepared to react to future events. This is a unique contribution of the framework in the area of climate adaptation and mitigation.
Our framework has some conceptual and methodological overlap with other frameworks in the SES literature. For instance, the “Resource system,” “Resource units,” “Users,” and “Governance” of Ostrom (2009) framework are partially analogous to our Ecological Community, Fished Species, Fishery, and Human Community, although the variables in Ostrom’s framework are how they relate to the resource differ from ours. In Ostrom’s framework, “Resource system” combines components and variables from our Ecological Community and Human Community, and our Human Community includes more than just Governance. The Driver, Pressure, State, Impact, Response (DPSIR) framework (Carr et al., 2007) has some similarities to our framework in that it links a series of interactions affecting the system to a social response, which can then in turn alter the interactions. A highly conceptual framework in the SES field, Turners Vulnerability Framework, (Turner et al., 2003) is not readily comparable to our framework, but our framework meets all 14 criteria for assessments it outlines.
When using this framework, teams should consider the following recommendations and limitations. While the team should be transdisciplinary, outcomes may be specific to certain components in the system and certain types of actors. Hence, the team should engage diverse types of knowledge and perspectives, including from fishery participants, community members, scientists, managers from relevant agencies (e.g., public health and port districts), and other stakeholders or interested parties. The application of the framework can happen over an extended period of time or in a compressed process involving, for example, a set of workshops. The team should constrain the system scope to only include the relevant components and variables which will prevent the project from becoming unwieldy. To reduce the scope, the team may choose one focal domain, or a focal component of a domain, and only consider elements or interactions which will affect that domain or component. The team should use literature, reports, local knowledge, and information from similar systems to consider what potential impacts the assessed Trend or Event might cause. The team should also limit the analysis time horizon; the selected Trend or Event will often inform this selection. Even with reasonable constraints on the system, a high level of complexity is likely and teams should attempt to follow the framework structure, dissecting the system into its components to ease the analysis.
Particularly when used prospectively, the team must consider inherent challenges of predicting the future. It is usually possible to construct a retrospective causal story, but predicting a causal story may be impossible with the information available. Even a well-qualified team will inevitably miss interactions or include ones that are irrelevant. As what interactions to include and their strength are uncertain, the team may wish to construct multiple scenarios with differing interactions in an effort to explore alternative future outcomes. We must also consider that some apparent impacts to systems might be inherently unpredictable, either because they are random or chaotic. Certainly, a broader consideration of potential impacts may reduce the likelihood of surprises, but in situations of high uncertainty, one should always be wary of the “unknown unknowns” that may compromise even the most carefully constructed forecasts. The team should realize the inherent uncertainty in this type of analysis and prepare for the outcomes they predict but not consider them inevitable. As always, communication of uncertainty to managers is key.
As teams use the framework and share their work with others within the FSES, the information can be vetted, edited, and augmented, with emphasis on areas of particular concern or opportunity, while also being cognizant of the potential for shifting baselines. Additionally, this type of assessment can identify knowledge gaps across the domains of the system, which could direct future research. The framework—and the approach for its use—is adaptable and applicable to a variety of systems. Applying the framework to more systems will benefit its development, help evaluate its broader utility, and help develop a set of best practices, including how to constrain its extent, select a focal domain or component, and select variables. Finally, as the framework and best practices become more refined, future work might investigate the addition of scoring metrics either for likelihood or severity of impacts on the focal domain. We encourage others to deploy our framework in their systems of concern to map out the potential impacts of climate change.
Data Availability Statement
The original contributions generated for this study are included in the article/Supplementary Material, further inquiries can be directed to the corresponding author.
Ethics Statement
Written informed consent was obtained from the individual(s) for the publication of any potentially identifiable images or data included in this article.
Author Contributions
PD designed the initial version of Figure 1. TR designed and drew Figures 2–4. All authors contributed to the framework design, the research for the application of the framework, and the writing of the manuscript.
Funding
MM was supported by the National Science Foundation Graduate Student Research Program (DGE1339067). Support for MC was provided by the Partnership for Interdisciplinary Studies of Coastal Oceans, a Long-Term Ecological Consortium (contribution number 518) funded by the David and Lucile Packard Foundation and the Gordon and Betty Moore Foundation. Support for AB was provided by the California Sea Grant Graduate Research Fellowship (NA18OAR4170073). Support for CP was provided by the California Sea Grant Program and the National Sea Grant College Program, NOAA, United States Department of Commerce. The Lenfest Oceans program and NOAA High Performance Computing and Communications (HPCC) supported TR during this project. Support for PD was provided by the NOAA NMFS.
Conflict of Interest
The authors declare that the research was conducted in the absence of any commercial or financial relationships that could be construed as a potential conflict of interest.
Acknowledgments
We would like to thank the California fishermen, seafood dealers, and seafood processors we reached out to as part of this effort. We would also like to thank Debbie Aseltine-Neilson, Marta Ballasteros, Nancy Fitzpatrick, Natasha Hardy, Bob Juntz, Nate Mantua, Barbara Muhling, Pete Nelson, Will Satterthwaite, Cameron Speir, Tom Trumper, and the Future Seas team for valuable input into this project.
Supplementary Material
The Supplementary Material for this article can be found online at: https://www.frontiersin.org/articles/10.3389/fmars.2021.678099/full#supplementary-material
Footnotes
- ^ https://nrm.dfg.ca.gov/FileHandler.ashx?DocumentID=178446&inline, accessed 9/22/20.
- ^ The West Coast HMS FMP includes Pacific tunas, swordfish, sharks, and billfish.
- ^ https://www.pcouncil.org/summaries-of-commercial-fishery-catch-revenue-and-effort-pacfin-data/
References
Ahlstrom, E. H. (1959). Vertical distribution of pelagic fish eggs and larvae off California and Baja California. Fish. Bull. 60, 107–146.
Alexander, M. A., Blade, I., Newman, M., Lanzante, J. R., Lau, N.-C., and Scott, J. D. (2002). The Atmospheric Bridge: The influence of ENSO teleconnections on air–sea interaction over the global oceans. J. Clim. 15:27.
Alexander, M. A., Scott, J. D., Friedland, K. D., Mills, K. E., Nye, J. A., Pershing, A. J., et al. (2018). Projected sea surface temperatures over the 21st century: Changes in the mean, variability and extremes for large marine ecosystem regions of Northern Oceans. Elem. Sci. Anth. 6:9.
Allison, E. H., Perry, A. L., Badjeck, M.-C., Neil Adger, W., Brown, K., Conway, D., et al. (2009). Vulnerability of national economies to the impacts of climate change on fisheries. Fish. Fish. 10, 173–196.
Archer, C. L., and Caldeira, K. (2008). Historical trends in the jet streams. Geophys. Res. Lett. 35:7.
Auth, T. D., Daly, E. A., Brodeur, R. D., and Fisher, J. L. (2017). Phenological and distributional shifts in ichthyoplankton associated with recent warming in the northeast Pacific Ocean. Glob. Change Biol. 24, 259–272.
Ayers, J. M., and Lozier, M. S. (2010). Physical controls on the seasonal migration of the North Pacific transition zone chlorophyll front. J. Geophys. Res. Oceans 2010:115.
Bakun, A. (1990). Global climate change and intensification of coastal ocean upwelling. Science 247, 198–201.
Bakun, A. (2006). Fronts and eddies as key structures in the habitat of marine fish larvae: opportunity, adaptive response and competitive advantage. Sci. Mar. 70:35.
Bakun, A., Black, B. A., Bograd, S. J., Garcia-Reyes, M., Miller, A. J., Rykaczewski, R. R., et al. (2015). Anticipated effects of climate change on coastal upwelling ecosystems. Curr. Clim. Change Rep. 1, 85–93.
Barange, M., Merino, G., Blanchard, J. L., Scholtens, J., Harle, J., Allison, E. H., et al. (2014). Impacts of climate change on marine ecosystem production in societies dependent on fisheries. Nat. Clim. Change 4, 211–216.
Baskett, M. L., and Salomon, A. K. (2010). Recruitment facilitation can drive alternative states on temperate reefs. Ecology 91, 1763–1773. doi: 10.1890/09-0515.1
Baumgartner, T. (1992). Reconstruction of the history of the Pacific sardine and northern anchovy populations over the past two millennia from sediments of the Santa Barbara basin, California. CalCOFI Rep. 33, 24–40.
Bell, J. D., Johnson, J. E., Ganachaud, A. S., Gehrke, P. C., Hobday, A. J., Hoegh-Guldberg, O., et al. (2011). Vulnerability of tropical Pacific fisheries and aquaculture to climate change: summary for Pacific island countries and territories. Noumea, New Caledonia : Secretariat of the Pacific Community.
Bengtsson, L., Hodges, K. I., and Roeckner, E. (2006). Storm tracks and climate change. J. Clim. 19, 3518–3543.
Bi, H., Peterson, W. T., and Strub, P. T. (2011). Transport and coastal zooplankton communities in the northern California Current system. Geophys. Res. Lett. 38:8.
Blasiak, R., Spijkers, J., Tokunaga, K., Pittman, J., Yagi, N., and Österblom, H. (2017). Climate change and marine fisheries: Least developed countries top global index of vulnerability. PLoS One 12:e0179632. doi: 10.1371/journal.pone.0179632
Bond, N. A., Cronin, M. F., Freeland, H., and Mantua, N. (2015). Causes and impacts of the 2014 warm anomaly in the NE Pacific. Geophys. Res. Lett. 42, 3414–3420. doi: 10.1002/2015gl063306
Briscoe, C. S., and Sebens, K. P. (1988). Omnivory in Strongylocentrotus droebachiensis (Müller) (Echinodermata: Echinoidea): predation on subtidal mussels. J. Exp. Mar. Biol. Ecol. 115, 1–24.
Butler, T. (1954). Food of the commercial crab in the Queen Charlotte Islands region. Fish. Res. Board Can. Prog. Rep. Pac. Coast Stn. Rep. 1954, 3–5.
California Dungeness Crab Fishing Gear Working Group (2016). 2016-17 Best Practices Guide to Minimize Whale Entanglement Risk. California: California Dungeness Crab Fishing Gear Working Group
Carr, E. R., Wingard, P. M., Yorty, S. C., Thompson, M. C., Jensen, N. K., and Roberson, J. (2007). Applying DPSIR to sustainable development. Int. J. Sustain. Dev. World Ecol. 14, 543–555.
Cavole, L., Demko, A., Diner, R., Giddings, A., Koester, I., Pagniello, C., et al. (2016). Biological impacts of the 2013–2015 warm-water anomaly in the Northeast Pacific: Winners, losers, and the future. Oceanography 2016:29.
CDFW (2019a). Dungeness Crab, Metacarcinus magister, Enhanced Status Report. California: California Department of Fish and Wildlife.
CDFW (2019b). Invertebrates of Interest: Sea Urchin. California: California Department of Fish and Wildlife
CDFW (2019c). Red Sea Urchin, Mesocentrotus franciscanus, Enhanced Status Report. California: California Department of Fish and Wildlife.
Chavez, F. P., Pennington, J. T., Castro, C. G., Ryan, J. P., Michisaki, R. P., Schlining, B., et al. (2002). Biological and chemical consequences of the 1997–1998 El Niño in central California waters. Prog. Oceanogr. 54, 205–232.
Chavez, F. P., Costello, C., Aseltine-Neilson, D., Doremus, H., Field, J. C., Gaines, S. D., et al. (2017). Readying California fisheries for climate change. Oakland: Extension Publications.
Checkley, D. M., Asch, R. G., and Rykaczewski, R. R. (2017). Climate, Anchovy, and Sardine. Annu. Rev. Mar. Sci. 9, 469–493.
Checkley, D. M., and Barth, J. A. (2009). Patterns and processes in the California Current System. Prog. Oceanogr. 83, 49–64.
Checkley, D. M. Jr., Dotson, R. C., and Griffith, D. A. (2000). Continuous, underway sampling of eggs of Pacific sardine (Sardinops sagax) and northern anchovy (Engraulis mordax) in spring 1996 and 1997 off southern and central California. Deep Sea Res. Part II Top. Stud. Oceanogr. 47, 1139–1155. doi: 10.1016/s0967-0645(99)00139-3
Chelton, D. B., Bernal, P., and McGowan, J. A. (1982). Large-scale interannual physical and biological interaction in the California Current. J. Mar. Res. 40, 1095–1125.
Chen, K.-S., Crone, P., and Hsu, C.-C. (2010). Reproductive biology of albacore Thunnus alalunga. J. Fish Biol. 77, 119–136.
Cheng, L., Abraham, J., Hausfather, Z., and Trenberth, K. E. (2019). How fast are the oceans warming? Science 363, 128–129.
Cheresh, J., and Fiechter, J. (2020). Physical and biogeochemical drivers of alongshore pH and oxygen variability in the California Current System. Geophys. Res. Lett. 2020:47.
Childers, J., Snyder, S., and Kohin, S. (2011). Migration and behavior of juvenile North Pacific albacore (Thunnus alalunga): North Pacific albacore migration and behavior. Fish. Oceanogr. 20, 157–173.
Christian, J. R., and Holmes, J. (2016). Changes in albacore tuna habitat in the northeast Pacific Ocean under anthropogenic warming. Fish. Oceanogr. 25, 544–554.
Cinner, J. E., McClanahan, T. R., Graham, N. A. J., Daw, T. M., Maina, J., Stead, S. M., et al. (2012). Vulnerability of coastal communities to key impacts of climate change on coral reef fisheries. Glob. Environ. Change 22, 12–20.
Cisneros-Mata, M. A., Mangin, T., Bone, J., Rodriguez, L., Smith, S. L., and Gaines, S. D. (2019). Fisheries governance in the face of climate change: Assessment of policy reform implications for Mexican fisheries. PLoS One 14:e0222317. doi: 10.1371/journal.pone.0222317
Cochran, R. C., and Engelmann, F. (1975). Environmental regulation of the annual reproductive season of Strongylocentrotus purpuratus (Stimpson). Biol. Bull. 148, 393–401.
Colburn, L. L., Jepson, M., Weng, C., Seara, T., Weiss, J., and Hare, J. A. (2016). Indicators of climate change and social vulnerability in fishing dependent communities along the Eastern and Gulf Coasts of the United States. Mar. Policy 74, 323–333.
Collier, P. C. (1983). Movement and growth of post-larval Dungeness crabs, Cancer magister, in the San Francisco area [Marine areas, California]. California: California Department of Fish and Game
Cowen, R. K. (1983). The effects of sheephead (Semicossyphus pulcher) predation on red sea urchin (Strongylocentrotus franciscanus) populations: An experimental analysis. Oecologia 58, 249–255.
Cowen, R. K., and Sponaugle, S. (2009). Larval Dispersal and Marine Population Connectivity. Annu. Rev. Mar. Sci. 1, 443–466.
Crozier, L. G., McClure, M. M., Beechie, T., Bograd, S. J., Boughton, D. A., Carr, M., et al. (2019). Climate vulnerability assessment for Pacific salmon and steelhead in the California Current Large Marine Ecosystem. PLoS One 14:e0217711. doi: 10.1371/journal.pone.0217711
Des Voigne, D. M. (1973). The Influence of Temperature and Photoperiod Upon the Dungeness Crab, Cancer Magister. Ph. D. thesis, University of Washington, Seattle.
Di Lorenzo, E., and Mantua, N. (2016). Multi-year persistence of the 2014/15 North Pacific marine heatwave. Nat. Clim. Change 6, 1042–1047.
Ding, Q., Chen, X., Hilborn, R., and Chen, Y. (2017). Vulnerability to impacts of climate change on marine fisheries and food security. Mar. Policy 83, 55–61.
Ebert, T. (1996). Adaptive aspects of phenotypic plasticity in echinoderms. Oceanol. Acta 19, 347–355.
Ebert, T. A. (1967). Negative growth and longevity in the purple sea urchin Strongylocentrotus purpuratus (Stimpson). Science 157, 557–558.
Ebert, T. A., and Russell, M. P. (1993). Growth and mortality of subtidal red sea urchins (Strongylocentrotus franciscanus) at San Nicolas Island, California, USA: problems with models. Mar. Biol. 117, 79–89.
Ebert, T. A., and Southon, J. R. (2003). Red sea urchins (Strongylocentrotus franciscanus) can live over 100 years: confirmation with A-bomb 14carbon. Fish. Bull. 101, 915–922.
Ekstrom, J. A., Suatoni, L., Cooley, S. R., Pendleton, L. H., Waldbusser, G. G., Cinner, J. E., et al. (2015). Vulnerability and adaptation of US shellfisheries to ocean acidification. Nat. Clim. Change 5, 207–214.
Filbee-Dexter, K., and Scheibling, R. E. (2014). Sea urchin barrens as alternative stable states of collapsed kelp ecosystems. Mar. Ecol. Prog. Ser. 495, 1–25.
Franks, P. J. (1992). Sink or swim: Accumulation of biomass at fronts. Mar. Ecol. Prog. Ser. Oldendorf 82, 1–12. doi: 10.3354/meps082001
Frawley, T. H., Muhling, B. A., Brodie, S., Fisher, M. C., Tommasi, D., Le Fol, G., et al. (2020). Changes to the structure and function of an albacore fishery reveal shifting social-ecological realities for Pacific Northwest fishermen. Fish. Fish. 2020:8g.
Free, C. M., Thorson, J. T., Pinsky, M. L., Oken, K. L., Wiedenmann, J., and Jensen, O. P. (2019). Impacts of historical warming on marine fisheries production. Science 363, 979–983.
Frischknecht, M., Münnich, M., and Gruber, N. (2017). Local atmospheric forcing driving an unexpected California Current System response during the 2015-2016 El Niño. Geophys. Res. Lett. 44, 304–311.
Füssel, H.-M., and Klein, R. J. T. (2006). Climate change vulnerability assessments: An evolution of conceptual thinking. Clim. Change 75, 301–329.
Future Seas (2021). A Physic-to-fisheries Management Strategy Evaluation for the California Current System. Available online at: future-seas.com (Accessed January 12, 2021).
García-Reyes, M., Largier, J. L., and Sydeman, W. J. (2014). Synoptic-scale upwelling indices and predictions of phyto- and zooplankton populations. Prog. Oceanogr. 120, 177–188.
Gentemann, C. L., Fewings, M. R., and García-Reyes, M. (2017). Satellite sea surface temperatures along the West Coast of the United States during the 2014-2016 northeast Pacific marine heat wave: Coastal SSTs During “the Blob.”. Geophys. Res. Lett. 44, 312–319.
Glaser, S. (2010). Interdecadal variability in predator–prey interactions of juvenile North Pacific albacore in the California Current System. Mar. Ecol. Prog. Ser. 414, 209–221.
Glaser, S. M. (2011). Do albacore exert top-down pressure on northern anchovy? Estimating anchovy mortality as a result of predation by juvenile north pacific albacore in the California current system: Albacore predation on anchovy. Fish. Oceanogr. 20, 242–257.
Glaser, S. M., Waechter, K. E., and Bransome, N. C. (2015). Through the stomach of a predator: Regional patterns of forage in the diet of albacore tuna in the California Current System and metrics needed for ecosystem-based management. J. Mar. Syst. 146, 38–49.
Gotshall, D. W. (1977). Stomach contents of northern California Dungeness crabs. Cancer magister. Calif. Fish Game 63, 43–51.
Grafton, R. Q. (2010). Adaptation to climate change in marine capture fisheries. Mar. Policy 34, 606–615.
Gutermuth, F. B., and Armstrong, D. A. (1989). Temperature-dependent metabolic response of juvenile Dungeness crab Cancer magister Dana: Ecological implications for estuarine and coastal populations. J. Exp. Mar. Biol. Ecol. 126, 135–144.
Hackett, S., Dewees, C. M., Hankin, D., Krachey, M., and Sortais, K. (2003). An economic overview of Dungeness crab (Cancer magister) processing in California. CalCOFI Rep. 44, 86–93.
Hare, J. A., Morrison, W. E., Nelson, M. W., Stachura, M. M., Teeters, E. J., Griffis, R. B., et al. (2016). A Vulnerability Assessment of Fish and Invertebrates to Climate Change on the Northeast U.S. Continental Shelf. PLoS One 11, e0146756. doi: 10.1371/journal.pone.0146756
Harrold, C., and Reed, D. C. (1985). Food availability, sea urchin grazing, and kelp forest community structure. Ecology 66, 1160–1169.
Harvell, C. D., Montecino-Latorre, D., Caldwell, J. M., Burt, J. M., Bosley, K., Keller, A., et al. (2019). Disease epidemic and a marine heat wave are associated with the continental-scale collapse of a pivotal predator (Pycnopodia helianthoides). Sci. Adv. 5:eaau7042.
Hazen, E. L., Jorgensen, S., Rykaczewski, R. R., Bograd, S. J., Foley, D. G., Jonsen, I. D., et al. (2013). Predicted habitat shifts of Pacific top predators in a changing climate. Nat. Clim. Change 3, 234–238.
Hewson, I., Bistolas, K. S. I., Quijano Cardé, E. M., Button, J. B., Foster, P. J., Flanzenbaum, J. M., et al. (2018). Investigating the Complex Association Between Viral Ecology, Environment, and Northeast Pacific Sea Star Wasting. Front. Mar. Sci. 5:77. doi: 10.3389/fmars.2018.00077
Himes-Cornell, A., and Kasperski, S. (2015). Assessing climate change vulnerability in Alaska’s fishing communities. Fish. Res. 162, 1–11.
Hobday, A. J., Smith, A. D. M., Stobutzki, I. C., Bulman, C., Daley, R., Dambacher, J. M., et al. (2011). Ecological risk assessment for the effects of fishing. Fish. Res. 108, 372–384.
Hobday, A., Oliver, E., Sen Gupta, A., Benthuysen, J., Burrows, M., Donat, M., et al. (2018). Categorizing and Naming Marine Heatwaves. Oceanography 2018:31.
Howard, E. M., Frenzel, H., Kessouri, F., Renault, L., Bianchi, D., McWilliams, J. C., et al. (2020). Attributing Causes of Future Climate Change in the California Current System With Multimodel Downscaling. Glob. Biogeochem. Cycles 34:e2020GB006646.
Hughes, A. D., Brunner, L., Cook, E. J., Kelly, M. S., and Wilson, B. (2012). Echinoderms Display Morphological and Behavioural Phenotypic Plasticity in Response to Their Trophic Environment. PLoS One 7:e41243. doi: 10.1371/journal.pone.0041243
Hughes, S., Yau, A., Max, L., Petrovic, N., Davenport, F., Marshall, M., et al. (2012). A framework to assess national level vulnerability from the perspective of food security: The case of coral reef fisheries. Environ. Sci. Policy 23, 95–108.
Hutto, S. V., Higgason, K. D., Kershner, J. M., Reynier, W. A., and Gregg, D. S. (2015). Climate Change Vulnerability Assessment for the North-Central California Coast and Ocean. Marine Sanctuaries Conservation Series ONMS-15-02. Marine Sanctuaries Conservation Series ONMS-15-02. U.S. Department of Commerce, National Oceanic and Atmospheric AdministrationNational Ocean Service. Silver Spring, MD: Office of National Marine Sanctuaries.
Ichinokawa, M., Coan, A. L. Jr., and Takeuchi, Y. (2008). Transoceanic migration rates of young North Pacific albacore, Thunnus alalunga, from conventional tagging data. Can. J. Fish. Aquat. Sci. 65, 1681–1691.
IPCC (2001). Climate change 2001: Impacts, Adaptation, and Vulnerability. New York, NY: Cambridge University Press.
IPCC (2019). Summary for Policymakers. In: IPCC Special Report on the Ocean and Cryosphere in a Changing Climate. Rome:IPPC
Jacinto, M. R., Songcuan, A. J. G., Yip, G. V., and Santos, M. D. (2015). Development and application of the fisheries vulnerability assessment tool (Fish Vool) to tuna and sardine sectors in the Philippines. Fish. Res. 161, 174–181. doi: 10.1016/j.fishres.2014.07.007
Jacox, M. G., Alexander, M. A., Mantua, N. J., Scott, J. D., Hervieux, G., Webb, R. S., et al. (2018a). Forcing of multiyear extreme ocean temperatures that impact California Current living marine resources in 2016. Bull. Am. Meteorol. Soc. 99, S27–S33.
Jacox, M. G., Edwards, C. A., Hazen, E. L., and Bograd, S. J. (2018b). Coastal Upwelling Revisited: Ekman, Bakun, and Improved Upwelling Indices for the U.S. West Coast. J. Geophys. Res. Oceans 123, 7332–7350. doi: 10.1029/2018jc014187
Jacox, M. G., Hazen, E. L., Zaba, K. D., Rudnick, D. L., Edwards, C. A., Moore, A. M., et al. (2016). Impacts of the 2015-2016 El Niño on the California Current System: Early assessment and comparison to past events: 2015-2016 El Niño Impact in the CCS. Geophys. Res. Lett. 43, 7072–7080.
Jensen, G. C. (1995). Pacific Coast crabs and shrimps. Available online at: http://agris.fao.org/agris-search/search.do?recordID=US9743074 (accessed July 29, 2019).
Johnson, J. E., and Welch, D. J. (2010). Marine fisheries management in a changing climate: A review of vulnerability and future options. Rev. Fish. Sci. 18, 106–124. doi: 10.1080/10641260903434557
Jones, M. C., and Cheung, W. W. L. (2017). Using fuzzy logic to determine the vulnerability of marine species to climate change. Glob. Change Biol. 24, e719–e731.
Juhasz, C., and Kalvass, P. (2011). Dungeness crab, Metacarcinus magister. California Department of Fish and Game. Available online at: https://nrm.dfg.ca.gov/FileHandler.ashx?DocumentID=65494&inline$^{∗}$ (accessed September 22, 2020).
Juhasz, C., and Pomeroy, C. (2017). Dungeness Crab Fishery Season Updates: 2016-17. Ukiah, CA: Presentation to the California Dungeness Crab Task Force.
Kahru, M., Jacox, M. G., and Ohman, M. D. (2018). CCE1: Decrease in the frequency of oceanic fronts and surface chlorophyll concentration in the California Current System during the 2014–2016 northeast Pacific warm anomalies. Deep Sea Res. Part Oceanogr. Res. Pap. 140, 4–13. doi: 10.1016/j.dsr.2018.04.007
Karatayev, V. A., and Baskett, M. L. (2020). At what spatial scales are alternative stable states relevant in highly interconnected ecosystems? Ecology 101:e02930.
Kato, S., and Schroeter, S. C. (1985). Biology of the red sea urchin, Strongylocentrotus franciscanus, and its fishery in California. Mar. Fish. Rev. 47, 1–20.
Koehn, L. E., Essington, T. E., Marshall, K. N., Kaplan, I. C., Sydeman, W. J., Szoboszlai, A. I., et al. (2016). Developing a high taxonomic resolution food web model to assess the functional role of forage fish in the California Current ecosystem. Ecol. Model. 335, 87–100. doi: 10.1016/j.ecolmodel.2016.05.010
Koslow, A. J., Goericke, R., and Watson, W. (2013). Fish assemblages in the Southern California Current: relationships with climate, 1951–2008. Fish. Oceanogr. 22, 207–219. doi: 10.1111/fog.12018
Koslow, J. A., Davison, P., Ferrer, E., Jiménez Rosenberg, S. P. A., Aceves-Medina, G., and Watson, W. (2019). The evolving response of mesopelagic fishes to declining midwater oxygen concentrations in the southern and central California Current. ICES J. Mar. Sci. 76, 626–638. doi: 10.1093/icesjms/fsy154
Koslow, J. A., Goericke, R., Lara-Lopez, A., and Watson, W. (2011). Impact of declining intermediate-water oxygen on deepwater fishes in the California Current. Mar. Ecol. Prog. Ser. 436, 207–218. doi: 10.3354/meps09270
Laurs, R. M., and Lynn, R. J. (1977). Seasonal migration of North Pacific Albacore, Thunnus alalunga, into North American coastal waters: Distribution, relative abundance, and association with transition zone waters. Fish. Bull. 75, 795–822.
Lawton, P., and Elner, R. W. (1985). Feeding in relation to morphometrics within the genus Cancer: evolutionary and ecological considerations. in Proceedings of the symposium on Dungeness crab biology and management. Alaska Sea Grant Rep. 1985, 357–379.
Leising, A. W., Schroeder, I. D., Bograd, S. J., Abell, J., Durazo, R., Gaxiola-Castro, G., et al. (2015). State of the California Current 2014–15: Impacts of the warm-water “Blob.” Reports 56, 39.
Levin, L. A. (2018). Manifestation, drivers, and emergence of open ocean deoxygenation. Annu. Rev. Mar. Sci. 10, 229–260. doi: 10.1146/annurev-marine-121916-063359
Lindegren, M., Checkley, D. M., Rouyer, T., MacCall, A. D., and Stenseth, N. C. (2013). Climate, fishing, and fluctuations of sardine and anchovy in the California Current. Proc. Natl. Acad. Sci. 110, 13672–13677. doi: 10.1073/pnas.1305733110
Ling, S., Scheibling, R., Rassweiler, A., Johnson, C., Shears, N., Connell, S., et al. (2015). Global regime shift dynamics of catastrophic sea urchin overgrazing. Philos. Trans. R. Soc. B Biol. Sci. 370:20130269. doi: 10.1098/rstb.2013.0269
Link, J. S., and Browman, H. I. (2014). Integrating what? Levels of marine ecosystem-based assessment and management. ICES J. Mar. Sci. 71, 1170–1173. doi: 10.1093/icesjms/fsu026
Lotze, H. K., Tittensor, D. P., Bryndum-Buchholz, A., Eddy, T. D., Cheung, W. W. L., Galbraith, E. D., et al. (2019). Global ensemble projections reveal trophic amplification of ocean biomass declines with climate change. Proc. Natl. Acad. Sci. 116, 12907–12912.
Lynn, R. J., and Simpson, J. J. (1987). The California Current System: The seasonal variability of its physical characteristics. J. Geophys. Res. Oceans 92, 12947–12966. doi: 10.1029/JC092iC12p12947
Maina, J., Kithiia, J., Cinner, J., Neale, E., Noble, S., Charles, D., et al. (2015). Integrating social–ecological vulnerability assessments with climate forecasts to improve local climate adaptation planning for coral reef fisheries in Papua New Guinea. Reg. Environ. Change 16, 881–891. doi: 10.1007/s10113-015-0807-0
Malick, M. J., Hunsicker, M. E., Haltuch, M. A., Parker-Stetter, S. L., Berger, A. M., and Marshall, K. N. (2020). Relationships between temperature and Pacific hake distribution vary across latitude and life-history stage. Mar. Ecol. Prog. Ser. 639, 185–197. doi: 10.3354/meps13286
Mamauag, S. S., Aliño, P. M., Martinez, R. J. S., Muallil, R. N., Doctor, M. V. A., Dizon, E. C., et al. (2013). A framework for vulnerability assessment of coastal fisheries ecosystems to climate change—Tool for understanding resilience of fisheries (VA–TURF). Fish. Res. 147, 381–393. doi: 10.1016/j.fishres.2013.07.007
Mao, J., and Jardine, S. L. (2020). Market impacts of a toxic algae event: the case of California Dungeness crab. Mar. Resour. Econ. 35, 1–20.
Mattison, J., Trent, J., Shanks, A., Akin, T., and Pearse, J. (1976). Movement and feeding activity of red sea urchins (Strongylocentrotus franciscanus) adjacent to a kelp forest. Mar. Biol. 39, 25–30.
McCabe, R. M., Hickey, B. M., Kudela, R. M., Lefebvre, K. A., Adams, N. G., Bill, B. D., et al. (2016). An unprecedented coastwide toxic algal bloom linked to anomalous ocean conditions. Geophys. Res. Lett. 2016:43.
McClatchie, S., Hendy, I., Thompson, A., and Watson, W. (2017). Collapse and recovery of forage fish populations prior to commercial exploitation. Geophys. Res. Lett. 44, 1877–1885. doi: 10.1002/2016gl071751
McClatchie, S., Jacox, M. G., Ohman, M. D., and Sala, L. M. (2016). State of the California current 2015–16: Comparisons with the 1997–98 El Niño. Calif. Coop. Ocean. Fish. Invest. Rep. 57, 5–61.
McPhaden, M. J., Busalacchi, A. J., and Cheney, R. (1998). The Tropical Ocean-Global Atmosphere observing system: A decade of progress. J. Geophys. Res. 1998:72.
McPherson, M. L., Finger, D. J. I., Houskeeper, H. F., Bell, T. W., Carr, M. H., Rogers-Bennett, L., et al. (2021). Large-scale shift in the structure of a kelp forest ecosystem co-occurs with an epizootic and marine heatwave. Nat. - Commun. Biol. 2021:4. doi: 10.1038/s42003-021-01827-6
Monnereau, I., Mahon, R., McConney, P., Nurse, L., Turner, R., and Vallès, H. (2017). The impact of methodological choices on the outcome of national-level climate change vulnerability assessments: An example from the global fisheries sector. Fish Fish 18, 717–731. doi: 10.1111/faf.12199
Morley, J. W., Selden, R. L., Latour, R. J., Frölicher, T. L., Seagraves, R. J., and Pinsky, M. L. (2018). Projecting shifts in thermal habitat for 686 species on the North American continental shelf. PLoS One 13:e0196127. doi: 10.1371/journal.pone.0196127
Morrison, W. E., Nelson, M. W., Howard, J. F., Teeters, E. J., Hare, J. A., Griffis, R. B., et al. (2015). Methodology for assessing the vulnerability of marine fish and shellfish species to a changing climate. NOAA technical memorandum NMFS-OSF. 2015:2.
Munk, P., Kiørboe, T., and Christensen, V. (1989). Vertical migrations of herring, Clupea harengus, larvae in relation to light and prey distribution. Environ. Biol. Fishes 26, 87–96.
Netburn, A. N., and Koslow, J. A. (2015). Dissolved oxygen as a constraint on daytime deep scattering layer depth in the southern California current ecosystem. Deep Sea Res. Part Oceanogr. Res. Pap. 104, 149–158. doi: 10.1016/j.dsr.2015.06.006
Nieto, K., Xu, Y., Teo, S. L. H., McClatchie, S., and Holmes, J. (2017). How important are coastal fronts to albacore tuna (Thunnus alalunga) habitat in the Northeast Pacific Ocean? Prog. Oceanogr. 150, 62–71. doi: 10.1016/j.pocean.2015.05.004
NOAA (2016). Ecosystem-Based Fisheries Management Policy of the National Marine Fisheries Service National Oceanic and Atmospheric Administration. Silver Spring: NOAA.
Okamoto, D. K., Schroeter, S., and Reed, D. C. (2020). Effects of Ocean Climate on Spatiotemporal Variation in Sea Urchin Settlement and Recruitment. Ecology 2020:387282.
Okey, T. A., Agbayani, S., and Alidina, H. M. (2015). Mapping ecological vulnerability to recent climate change in Canada’s Pacific marine ecosystems. Ocean Coast. Manag. 106, 35–48. doi: 10.1016/j.ocecoaman.2015.01.009
Ostrom, E. (2009). A General Framework for Analyzing Sustainability of Social-Ecological Systems. Science 325, 419–422. doi: 10.1126/science.1172133
Otsu, T., and Uchida, R. N. (1964). Model of the migration of albacore in the North Pacific Ocean. Fish. Bull. Fish Wildl. Serv. 63:33.
Parrish, R., Schwing, F. B., and Mendelssohn, R. (2000). Mid-latitude wind stress: The energy source for climatic shifts in the North Pacific Ocean. Fish. Oceanogr. 9, 224–238. doi: 10.1046/j.1365-2419.2000.00136.x
Pearse, J. S. (1970). North WJ. Marine waste disposal and sea urchin ecology. Annu Rep Kelp Habit Imp Proj 1969-1970 Calif Inst Tech Pasadena. Apendex 1970, 1–87.
Pecl, G. Australia and Department of Climate Change (2009). The east coast Tasmanian rock lobster fishery: vulnerability to climate change impacts and adaptation response options. Canberra: Dept. of Climate Change.
Perry, R. I. (2011). Potential impacts of climate change on marine wild capture fisheries: An update. J. Agric. Sci. 149, 63–75. doi: 10.1017/s0021859610000961
Peterson, W., Robert, M., and Bond, N. (2015). The warm Blob continues to dominate the ecosystem of the northern California Current. Sidney: PICES Press.
Phillips, A. J., Ciannelli, L., Brodeur, R. D., Pearcy, W. G., and Childers, J. (2014). Spatio-temporal associations of albacore CPUEs in the Nort heastern Pacific with regional SST and climate environmental variables. ICES J. Mar. Sci. 71, 1717–1727. doi: 10.1093/icesjms/fst238
Plagányi, ÉE., Punt, A. E., Hillary, R., Morello, E. B., Thébaud, O., Hutton, T., et al. (2014). Multispecies fisheries management and conservation: Tactical applications using models of intermediate complexity. Fish Fish 15, 1–22. doi: 10.1111/j.1467-2979.2012.00488.x
Polovina, J. J., Howell, E. A., Kobayashi, D. R., and Seki, M. P. (2017). The transition zone chlorophyll front updated: advances from a decade of research. Prog. Oceanogr. 150, 79–85. doi: 10.1016/j.pocean.2015.01.006
Polovina, J. J., Howell, E., Kobayashi, D. R., and Seki, M. P. (2001). The transition zone chlorophyll front, a dynamic global feature defining migration and forage habitat for marine resources. Prog. Oceanogr. 49, 469–483. doi: 10.1016/s0079-6611(01)00036-2
Pomeroy, C., Aseltine-Neilson, D., Georgilas, N., and Bartling, R. (2018). Socioeconomic guidance for the California Marine Life Management Act Amended Master Plan. California: California Sea Grant and NOAA Fisheries Southwest Fisheries Science Center.
Pomeroy, C., Thomson, C., and Stevens, M. (2010). California’s north coast fishing communities: Historical perspective and recent trends. La Jolla, CA: California Sea Grant and NOAA Fisheries Southwest Fisheries Science Center.
Pörtner, H. O., and Farrell, A. P. (2008). Physiology and Climate Change. Sci. New Ser. 322, 690–692. doi: 10.1126/science.1163156
Radtke, H. D., and Davis, S. W. (2000). Description of the US West Coast commercial fishing fleet and seafood processors. Gladstone Ore Pac. States Mar. Fish. Comm. 2000:6.
Rasmuson, L. K. (2013). The Biology, Ecology and Fishery of the Dungeness crab, Cancer magister. Adv. Mar. Biol. 2013, 95–148. doi: 10.1016/B978-0-12-410498-3.00003-3
Ritzman, J., Brodbeck, A., Brostrom, S., McGrew, S., Dreyer, S., Klinger, T., et al. (2018). Economic and sociocultural impacts of fisheries closures in two fishing-dependent communities following the massive 2015 U.S. West Coast harmful algal bloom. Harmful Algae 80, 35–45. doi: 10.1016/j.hal.2018.09.002
Rogers-Bennett, L., and Catton, C. A. (2019). Marine heat wave and multiple stressors tip bull kelp forest to sea urchin barrens. Sci. Rep. 9:15050.
Rothman, D. S., and Robinson, J. B. (1997). Growing pains: A conceptual framework for considering integrated assessments. Environ. Monit. Assess. 46, 23–43.
Rudnick, D. L., Zaba, K. D., Todd, R. E., and Davis, R. E. (2017). A climatology of the California Current System from a network of underwater gliders. Prog. Oceanogr. 154, 64–106. doi: 10.1016/j.pocean.2017.03.002
Ryan, J. P., Kudela, R. M., Birch, J. M., Blum, M., Bowers, H. A., Chavez, F. P., et al. (2017). Causality of an extreme harmful algal bloom in Monterey Bay, California, during the 2014–2016 northeast Pacific warm anomaly. Geophys. Res. Lett. 44, 5571–5579. doi: 10.1002/2017gl072637
Rykaczewski, R. R., Dunne, J. P., Sydeman, W. J., García-Reyes, M., Black, B. A., and Bograd, S. J. (2015). Poleward displacement of coastal upwelling-favorable winds in the ocean’s eastern boundary currents through the 21st century. Geophys. Res. Lett. 42, 6424–6431. doi: 10.1002/2015gl064694
Sainsbury, N. C., Genner, M. J., Saville, G. R., Pinnegar, J. K., O’Neill, C. K., Simpson, S. D., et al. (2018). Changing storminess and global capture fisheries. Nat. Clim. Change 8, 648–650.
Salgueiro-Otero, D., and Ojea, E. (2020). A better understanding of social-ecological systems is needed for adapting fisheries to climate change. Mar. Policy 122:104123. doi: 10.1016/j.marpol.2020.104123
Santora, J. A., Mantua, N. J., Schroeder, I. D., Field, J. C., Hazen, E. L., Bograd, S. J., et al. (2020). Habitat compression and ecosystem shifts as potential links between marine heatwave and record whale entanglements. Nat. Commun. 11:536.
Sato, M., Barth, J. A., Benoit-Bird, K. J., Pierce, S. D., Cowles, T. J., Brodeur, R. D., et al. (2018). Coastal upwelling fronts as a boundary for planktivorous fish distributions. Mar. Ecol. Prog. Ser. 595, 171–186. doi: 10.3354/meps12553
Shanks, A. L. (2013). Atmospheric forcing drives recruitment variation in the Dungeness crab (Cancer magister), revisited. Fish. Oceanogr. 22, 263–272.
Silva, M. R. O., Pennino, M. G., and Lopes, P. F. M. (2019). Social-ecological trends: Managing the vulnerability of coastal fishing communities. Ecol. Soc. 24:art4.
Snyder, S., Franks, P. J. S., Talley, L. D., Xu, Y., and Kohin, S. (2017). Crossing the line: Tunas actively exploit submesoscale fronts to enhance foraging success: Tunas actively exploit submesoscale fronts. Limnol. Oceanogr. Lett. 2, 187–194. doi: 10.1002/lol2.10049
Snyder, S. M. (2016). Navigating a Seascape: Physiological and Environmental Motivations Behind Juvenile North Pacific Albacore Movement Patterns. Ph. D. Thesis, University of California, San Diego, La Jolla.
Sonu, S. C. (2017). Sea urchin supply, demand, and market of Japan. Silver Spring: US Dept Commer. NOAA NMFS West Coast Reg.
Stevens, B. G., Armstrong, D. A., and Hoe, J. C. (1984). Diel activity of an estuarine population of dungeness crabs, Cancer magister, in relation to feeding and environmental factors. J. Crust. Biol. 4:15.
Stortini, C. H., Shackell, N. L., Tyedmers, P., and Beazley, K. (2015). Assessing marine species vulnerability to projected warming on the Scotian Shelf, Canada. ICES J. Mar. Sci. J. Cons. 72, 1731–1743. doi: 10.1093/icesjms/fsv022
Suda, A. (1966). Catch variations in the North Pacific albacore VI. The speculation about influence of fisheries on the catch and abundance of the albacore in the north-west Pacific by use of some simplified mathematical models (continued paper-I). Rep. Nankai Reg. Fish Res. Lab. 24, 1–14.
Sun, J., and Chiang, F.-S. (2015). Use and exploitation of sea urchins. Echinoderm. Aquac. 2015, 25–45. doi: 10.1002/9781119005810.ch2
Sydeman, W. J., Thompson, S. A., Field, J. C., Peterson, W. T., Tanasichuk, R. W., Freeland, H. J., et al. (2011). Does positioning of the North Pacific Current affect downstream ecosystem productivity? Geophys. Res. Lett. 38, 59.
Teck, S. J., Lorda, J., Shears, N. T., Ben-Horin, T., Toseland, R. E., Rathbone, S. T., et al. (2018). Quality of a fished resource: Assessing spatial and temporal dynamics. PLoS One 13:e0196864. doi: 10.1371/journal.pone.0196864
Teo, S. L., Piner, K. R., Lee, H., and Kuriyama, P. T. (2020). Development of a Preliminary Model for the 2020 North Pacific Albacore Tuna Stock Assessment. La Jolla, CA: NOAA Fisheries Southwest Fisheries Science Center.
Tittensor, D. P., Eddy, T. D., Lotze, H. K., Galbraith, E. D., Cheung, W., Barange, M., et al. (2018). A protocol for the intercomparison of marine fishery and ecosystem models: Fish-MIP v1.0. Geosci. Model Dev. 11, 1421–1442.
Toste, R., de Freitas Assad, L. P., and Landau, L. (2019). Changes in the North Pacific Current divergence and California Current transport based on HadGEM2-ES CMIP5 projections to the end of the century. Deep Sea Res. Part II Top. Stud. Oceanogr. 169:104641. doi: 10.1016/j.dsr2.2019.104641
Townsend, H., Harvey, C. J., deReynier, Y., Davis, D., Zador, S. G., Gaichas, S., et al. (2019). Progress on implementing ecosystem-based fisheries management in the United States through the use of ecosystem models and analysis. Front. Mar. Sci. 6:641. doi: 10.3389/fmars.2019.00641
Turner, B. L., Kasperson, R. E., Matson, P. A., McCarthy, J. J., Corell, R. W., Christensen, L., et al. (2003). A framework for vulnerability analysis in sustainability science. Proc. Natl. Acad. Sci. 100, 8074–8079.
Wabnitz, C. C. C., Lam, V. W. Y., Reygondeau, G., Teh, L. C. L., Al-Abdulrazzak, D., Khalfallah, M., et al. (2018). Climate change impacts on marine biodiversity, fisheries and society in the Arabian Gulf. PLoS One 13:e0194537. doi: 10.1371/journal.pone.0194537
Watson, J. R., Fuller, E. C., Castruccio, F. S., and Samhouri, J. F. (2018). Fishermen follow fine-scale physical ocean features for finance. Front. Mar. Sci. 5:46.
Wells, B. K., Goericke, R., Suryan, R. M., Parrish, J., Dolliver, J., Loredo, S., et al. (2017). State of the California Current 2016-17: Still anything but “normal” in the north. California Cooperat. Oceanic Fish. Investig. Rep. 58:55.
Wells, R. D., Kohin, S., Teo, S. L., Snodgrass, O. E., and Uosaki, K. (2013). Age and growth of North Pacific albacore (Thunnus alalunga): implications for stock assessment. Fish. Res. 147, 55–62.
Wernberg, T., Bennett, S., Babcock, R. C., De Bettignies, T., Cure, K., Depczynski, M., et al. (2016). Climate-driven regime shift of a temperate marine ecosystem. Science 353, 169–172. doi: 10.1126/science.aad8745
Wernberg, T., Smale, D. A., Tuya, F., Thomsen, M. S., Langlois, T. J., De Bettignies, T., et al. (2013). An extreme climatic event alters marine ecosystem structure in a global biodiversity hotspot. Nat. Clim. Change 3, 78–82. doi: 10.1038/nclimate1627
Xu, Y., Nieto, K., Teo, S. L. H., McClatchie, S., and Holmes, J. (2017). Influence of fronts on the spatial distribution of albacore tuna (Thunnus alalunga) in the Northeast Pacific over the past 30 years (1982–2011). Prog. Oceanogr. 150, 72–78. doi: 10.1016/j.pocean.2015.04.013
Yin, J. H. (2005). A consistent poleward shift of the storm tracks in simulations of 21st century climate. Geophys. Res. Lett. 2005:32.
Zaba, K. D., and Rudnick, D. L. (2016). The 2014–2015 warming anomaly in the Southern California Current System observed by underwater gliders. Geophys. Res. Lett. 43, 1241–1248. doi: 10.1002/2015gl067550
Zhang, C. I., Hollowed, A. B., Lee, J.-B., and Kim, D.-H. (2011). An IFRAME approach for assessing impacts of climate change on fisheries. ICES J. Mar. Sci. 68, 1318–1328. doi: 10.1093/icesjms/fsr073
Keywords: climate change, ecosystem-based fisheries management, marine heatwave, conceptual model, human dimensions, fishing communities, ecological communities
Citation: Dudley PN, Rogers TL, Morales MM, Stoltz AD, Sheridan CJ, Beulke AK, Pomeroy C and Carr MH (2021) A More Comprehensive Climate Vulnerability Assessment Framework for Fisheries Social-Ecological Systems. Front. Mar. Sci. 8:678099. doi: 10.3389/fmars.2021.678099
Received: 08 March 2021; Accepted: 13 May 2021;
Published: 14 June 2021.
Edited by:
Paul E. Renaud, Akvaplan-niva, NorwayReviewed by:
Charlotte Teresa Weber, Akvaplan-niva, NorwayJörn Oliver Schmidt, International Council for the Exploration of the Sea (ICES), Denmark
Copyright © 2021 Dudley, Rogers, Morales, Stoltz, Sheridan, Beulke, Pomeroy and Carr. This is an open-access article distributed under the terms of the Creative Commons Attribution License (CC BY). The use, distribution or reproduction in other forums is permitted, provided the original author(s) and the copyright owner(s) are credited and that the original publication in this journal is cited, in accordance with accepted academic practice. No use, distribution or reproduction is permitted which does not comply with these terms.
*Correspondence: Peter N. Dudley, cGV0ZXIuZHVkbGV5QG5vYWEuZ292