Trichome Lengths of the Heterocystous N2-Fixing Cyanobacteria in the Tropical Marginal Seas of the Western North Pacific
- 1Department of Oceanography, National Sun Yat-sen University, Kaohsiung, Taiwan
- 2Department of Ocean and Earth Sciences, Old Dominion University, Norfolk, VA, United States
- 3Kushiro Field Station, Fisheries Resources Institute, Japan Fisheries Research and Education Agency, Kushiro, Japan
- 4Institute of Plant and Microbial Biology, Academia Sinica, Taipei, Taiwan
Calothrix rhizosoleniae and Richelia intracellularis are heterocystous cyanobacteria found in the tropical oceans. C. rhizosoleniae commonly live epiphytically on diatom genera Chaetoceros (C-C) and Bacteriastrum (B-C) while R. intracellularis live endosymbiotically within Rhizosolenia (R-R), Guinardia (G-R), and Hemiaulus (H-R); although, they occasionally live freely (FL-C and FL-R). Both species have much shorter trichomes than the other marine filamentous cyanobacteria such as Trichodesmium spp. and Anabaena gerdii. We investigated the trichome lengths of C. rhizosoleniae and R. intracellularis in the South China Sea (SCS) and the Philippine Sea (PS) between 2006 and 2014. On average, H-R had the shortest trichome lengths (3.5 cells/trichome), followed by B-C and C-C (4.9–5.2 cells/trichome) and FL-C (5.9 cells/trichome), and R-R, G-R, and FL-R had the longest trichome lengths (7.4–8.3 cells/trichome). Field results showed the trichome lengths of C-C and B-C did not vary seasonally or regionally. However, FL-C and H-R from the SCS and during the cool season had longer trichomes, where/when the ambient nutrient concentrations were higher. R-R, G-R, and FL-R also showed regional and seasonal variations in trichome length. Ultrastructural analysis found no gas vesicles within the C. rhizosoleniae cells to assist in buoyancy regulation. Results suggest that the trichome lengths of C. rhizosoleniae and R. intracellularis might be regulated by their diatom hosts’ symbiotic styles and by ambient nutrients. Short trichome length might help C. rhizosoleniae and R. intracellularis to stay in the euphotic zone regardless as to whether they are free-living or symbiotic.
Introduction
Marine cyanobacterial dinitrogen (N2) fixation plays a substantial role in global biogeochemical nitrogen (N) and carbon cycling. Nitrogen fixers (diazotrophs) transform N2 into bioavailable ammonium (NH4+), which can promote primary and secondary production (Carpenter et al., 1999; Sohm et al., 2011) and support large non-diazotrophic diatoms in oligotrophic seawater (Chen et al., 2011). Diazotrophic cyanobacteria are photoautotrophic, or symbiotic with photoautotrophs, and reside in the upper euphotic zone of the ocean (Carpenter and Capone, 2008). They are classified into several phylogenetic groups that include heterocystous (heterocyst-forming) filamentous (e.g., Richelia intracellularis, Calothrix rhizosoleniae, and Anabaena gerdii), non-heterocystous filamentous (Trichodesmium spp.), and unicellular cyanobacteria (Candidatus Atelocyanobacterium thalassa, Crocosphaera watsonii, and Cyanothece spp.) (Carpenter and Janson, 2001; Capone et al., 2005; Foster et al., 2007). While the unicellular representatives can be abundant in both coastal and oligotrophic regions, filamentous groups often occur primarily in oligotrophic oceanic regions and riverine plume-influenced mesohaline waters (Foster et al., 2007; Subramaniam et al., 2008; Grosse et al., 2010; Turk et al., 2011; Chen et al., 2014; Shiozaki et al., 2018, 2020; Mulholland et al., 2019).
Calothrix rhizosoleniae (hereafter Calothrix, unless noted) and Richelia intracellularis (hereafter Richelia) are commonly observed in tropical and subtropical ocean regions and marginal seas (Kimor et al., 1992; Carpenter et al., 1999; Gómez et al., 2005; Bar Zeev et al., 2008; Tuo et al., 2017). Both Calothrix and Richelia are most often observed in symbiotic relationships with certain diatom species and only occasionally observed free-living (FL) outside of a host (Karsten, 1907; Gómez et al., 2005; White et al., 2007; Foster et al., 2011; Tuo et al., 2017). These diatom diazotroph associations/assemblages (DDAs) are thought to contribute substantial fixed N to oceanic regions where they occur (Mague et al., 1974; Carpenter et al., 1999; Bar Zeev et al., 2008; Grosse et al., 2010).
Calothrix and Richelia have different styles of symbiosis. Calothrix is often epiphytic with chain-forming Chaetoceros compressus and Bacteriastrum spp. (both belong to Family Chaetocerotaceae) while Richelia most often occurs as an endosymbiont with Rhizosolenia clevei, Guinardia cylindrus (both belong to Family Rhizosoleniaceae), and Hemiaulus spp. (Family Hemiaulaceae) (Sundström, 1984; Gómez et al., 2005; Tuo et al., 2017). More precisely, symbiotic Richelia filaments dwell inside the cytosol of Hemiaulus hosts or dwell in the periplasmic space between the plasmalemma and frustule of Rhizosolenia hosts (Villareal, 1990; Caputo et al., 2019; Nieves-Morión et al., 2020). The actual location of symbiotic Richelia inside the Guinardia hosts is still unknown. It is also unknown whether the Richelia associated with different host diatoms are distinct species or strains (Sundström, 1984; Janson et al., 1999; Foster and Zehr, 2006). Hemiaulus-associated Richelia (H-R) is considered an obligate symbiont due to the fact that its smaller genome (3.2 Mbp) lacks many N-associated metabolic pathways, e.g., glutamine 2-oxoglutarate aminotransferase (GOGAT) and transporters for NH4+, nitrate (NO3–), nitrite (NO2–), and urea (Hilton et al., 2013; Hilton, 2014). The larger genome (5.4 Mbp) of Rhizosolenia-associated Richelia (R-R) also lacks the combined-N transporters (Hilton, 2014; Nieves-Morión et al., 2020). Both the nifH gene analysis and morphological examination of FL-Richelia (FL-R) from field samples suggest that FL filaments originate from Rhizosolenia rather than Hemiaulus (Zhang et al., 2011; Tuo et al., 2017). However, little is known about the genome of FL-R or Guinardia-associated Richelia (G-R). In contrast, Calothrix is considered a facultative symbiont or an opportunist. Its genome (6.0 Mbp) is more similar to those of FL heterocystous cyanobacterial species (Hilton et al., 2013; Nieves-Morión et al., 2020), suggesting its ability to live freely (FL-C) in nature. In the marginal seas of the low-latitude western North Pacific, FL-C comprised about 20–40% of overall Calothrix abundance [FL-C plus Chaetoceros- (C-C) and Bacteriastrum-associated Calothrix (B-C), 48–698 × 103 filaments m–2] (Tuo et al., 2017). FL-R were occasionally found in the upper water column of the tropical western North Pacific and their abundance (1–29 × 103 filaments m–2) was much lower than symbiotic Richelia (403–1,703 × 103 filaments m–2) (Tuo et al., 2017). It has been suggested that FL-R are not viable due to their lack of gas vesicles to help them regulate their buoyancy (Janson et al., 1995). However, to date, little is known about how cellular ultrastructure affects the buoyancy regulation of Calothrix or whether their symbiotic behavior offer these organisms the ability to stay in the upper water column in the absence of cellular architecture facilitating buoyancy.
It is interesting that both Calothrix and Richelia have much shorter trichome lengths than other marine filamentous cyanobacteria. According to previous studies from different regions, symbiotic Calothrix filaments have no more than 14 cells per trichome (vegetative cells plus one terminal heterocyst) whereas symbiotic Richelia have no more than 21 cells per trichome (Schmidt, 1901; Foster and Zehr, 2006; Tuo et al., 2017). Little is known about the trichome lengths of FL-C and FL-R. In contrast, a filament of Trichodesmium may contain more than 100 cells per trichome (Capone et al., 1997; Luo et al., 2012) and a filament of A. gerdii, a sparsely distributed heterocystous species, may contain more than 300 cells per trichome (Carpenter and Janson, 2001). These field observations give rise to a question about the trichome length of Calothrix and Richelia: were the trichome lengths different among the symbionts associated with distinct diatom hosts?
The northern South China Sea (SCS) and the western Philippine Sea (PS), two large and connected marginal seas of the western North Pacific Ocean, harbor diverse N2-fixing cyanobacteria (Chen et al., 2014, 2019; Shiozaki et al., 2014; Tuo et al., 2017). Both regions are influenced by the Kuroshio western boundary current, in which Trichodesmium, Calothrix, and Richelia are prevalent (Chen et al., 2008; Shiozaki et al., 2014, 2015; Tuo et al., 2014). The Kuroshio originates along the eastern coast of Luzon and flows north across the Luzon Strait where it exchanges waters with the SCS before meandering along the eastern coast of Taiwan and meeting the continental slope of the East China Sea (Figure 1; Caruso et al., 2006; Jan et al., 2015). The monsoonal circulation of the SCS results in seasonal variations in the velocity, width, and path of the Kuroshio at the northwestern boundary of the PS (Jan et al., 2015), as well as stronger Kuroshio intrusion from the PS into the SCS during the northeast monsoon (NEM) (Liang et al., 2003). During the NEM, the SCS has cool surface seawater temperatures (SST) and the circulation in the basin is cyclonic, while during the southwest monsoon (SWM), SST is warm and the circulation is anticyclonic in the SCS (Liu et al., 2002). Although monsoon-driven seasonal changes in SST, surface photosynthetically active radiation (SPAR), and stratification index (SI) are observed, the SCS and the PS are both oligotrophic year-round. However, the nutrient distributions in the upper water column differ somewhat between the regions; the SCS generally has shallower nitracline (DN) and phosphacline depths (DP) than the PS, suggesting a potential for higher fluxes of nitrate and phosphate from the depth (Chen et al., 2008; Tuo et al., 2017). The two marginal seas provide opportunities to examine whether the trichome length of each distinct host-associated or FL Calothrix or Richelia was different as the filaments habited in specific regions and seasons.
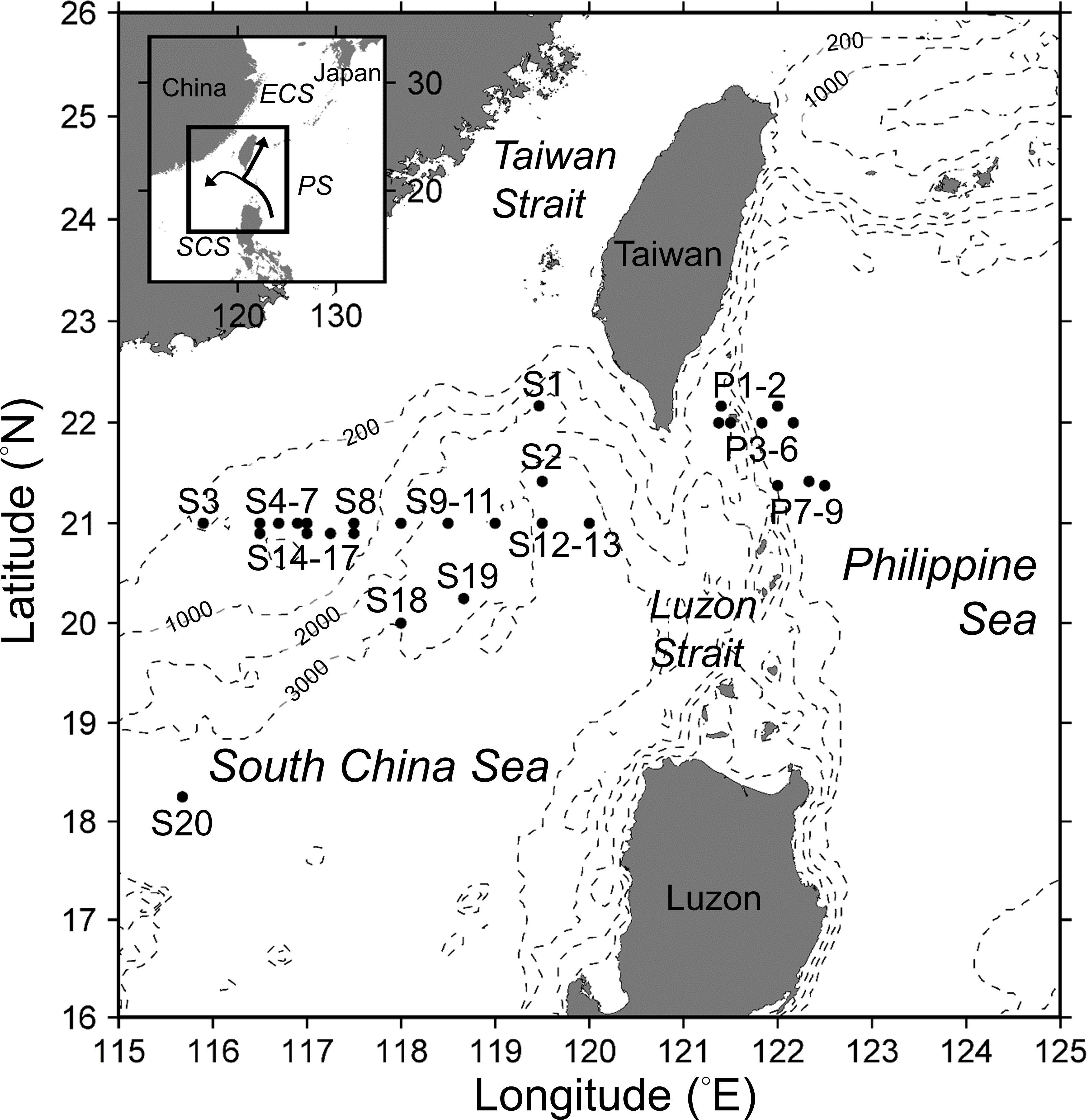
Figure 1. Bottom topography (hatched lines) and locations of sampling stations in the South China Sea (stations S1 to S20) and the Philippine Sea (stations P1 to P9). The arrows in the inset map indicate the path (in the PS) and intrusion (into the SCS) of the Kuroshio (after Caruso et al., 2006).
In this study, we evaluated how trichome length and intracellular characteristics contribute to the distribution of Calothrix and Richelia in the field. Specifically, we (1) examined whether the trichome lengths were different among the symbionts associated with distinct diatom hosts (and their FL counterparts) and (2) examined whether the trichome length for each Calothrix/Richelia symbiont was different between the regions and between the seasons. We also used laboratory cultures of an FL-C strain isolated from the SCS seawater to examine whether C. rhizosoleniae has gas vesicles and other intracellular structures.
Materials and Methods
Sampling Strategy
Field samples were collected during 20 cruises between 2006 and 2014 aboard the R/V Ocean Researcher I (OR1) and the R/V Ocean Researcher III (OR3) (Table 1). The cruises were conducted seasonally during the warm SWM (from April to October, 14 cruises) and the cool NEM (from mid-December to January, 6 cruises) seasons (Table 1). Samples were collected from 1 to 11 stations per cruise to investigate trichome lengths of Calothrix and Richelia and environmental parameters (Figure 1 and Table 1). In total, 83 stations were sampled from 29 discrete locations (some stations were revisited) over the 9-year period, and these were categorized into four region–season combinations: SCS-SWM, SCS-NEM, PS-SWM, and PS-NEM. At each station, water samples were collected from seven to nine discrete depths between 5 and 200 m using 20-L Go-Flo bottles attached to a rosette equipped with a Seabird SBE 9 conductivity–temperature–depth sensor and an underwater photosynthetically active radiation (PAR) sensor (OSP200L, Biospherical).
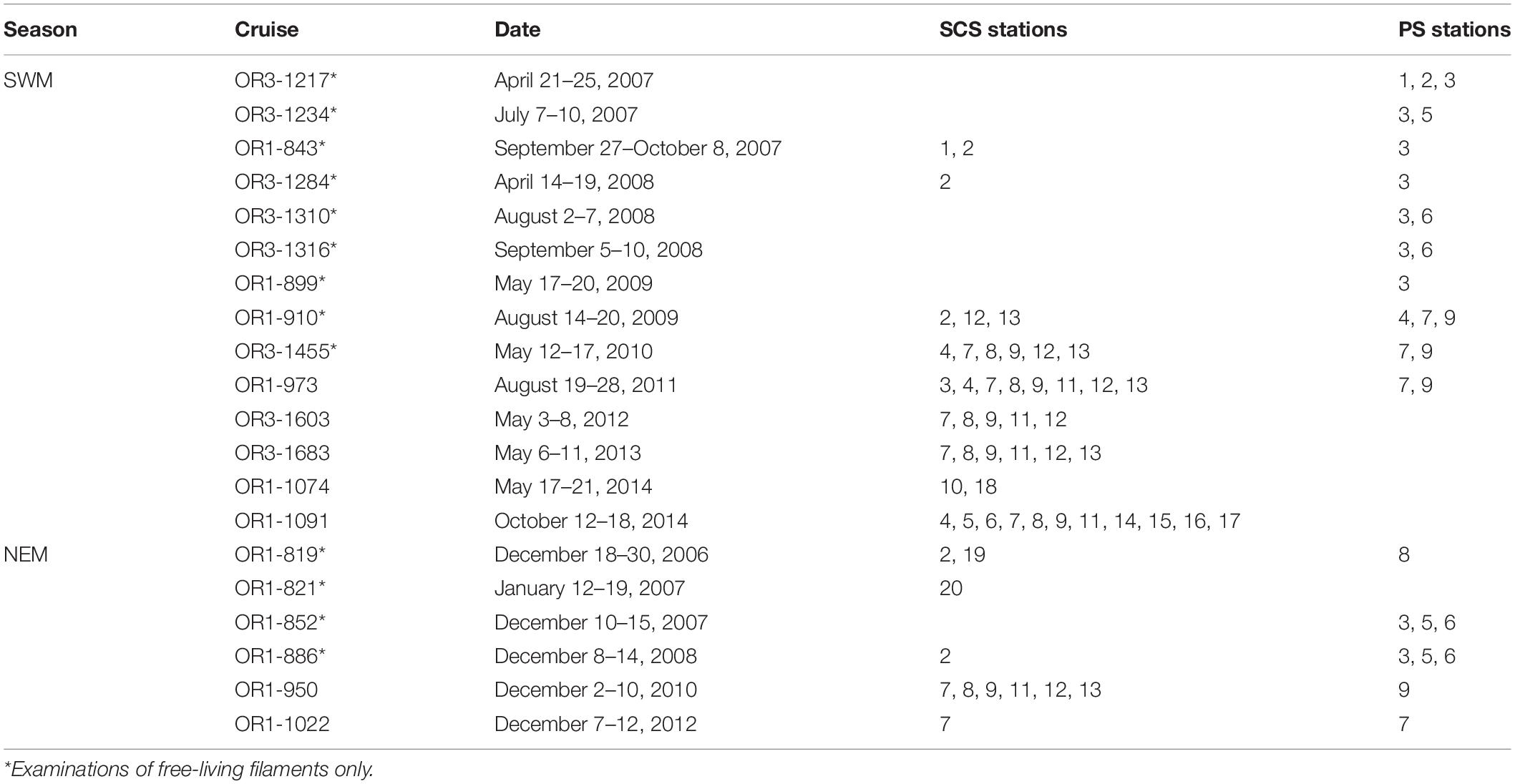
Table 1. Sampling stations occupied during 20 cruises in the SCS and the PS (see Figure 1 for station locations).
Trichome Length Examination on Richelia and Calothrix
Samples collected to identify Richelia and Calothrix and to measure their trichome lengths were prepared as described in Tuo et al. (2017). Richelia and Calothrix, showing an orange-yellow fluorescence, were identified at ×400 magnification with Zeiss epi-fluorescence microscopes equipped filter set of blue excitation and long pass emission (BP 450–490, FT 510, LP 520). Bright field was also used to distinguish symbionts from FL and to identify their host diatom species.
In total, 12,254 filaments of Richelia and 2,697 filaments of Calothrix, in free-living (176 filaments of FL-R and 1,206 filaments of FL-C) or symbiotic forms (12,078 filaments of Richelia and 1,491 filaments of Calothrix), were examined and their trichome lengths recorded. The morphological examination of 1,382 FL filaments (FL-R and FL-C) was found at the 83 stations sampled during the 20 cruises. A total of 13,569 symbiont filaments (H-R, R-R, G-R, C-C, and B-C) were examined from 43 of the 83 stations (from seven of the 20 cruises, conducted between December 2010 and October 2014) (Table 1). The general morphological descriptions and the abundances of each DDA and FL were published in Tuo et al. (2017).
Environmental Parameters
At each station, the euphotic depth (DE, m), surface PAR influx (SPAR, E m–2 day–1), stratification index (SI, kg m–4), mixed-layer depth (DM, m), NO3– plus NO2– (N+N), soluble reactive phosphorus (phosphate, P), and chlorophyll a (mg m–3) concentrations were calculated or measured following Tuo et al. (2017). To better assess the total nutrient inventory in the upper water column at each station, in addition to surface concentrations (NS and PS, nM), depth-integrated nutrient inventories (NI and PI) and the nutricline depths (DN and DP) were calculated for each nutrient (N+N or P). Depth-integrated inventories (NI and PI, mmol m–2) were calculated by trapezoidal integration from the surface to 100 m depth. The nutricline is defined as the seawater layer where the greatest change in the nutrient concentration occurs with depth. Based on the definition, the nutricline depth for each nutrient (DN and DP) was found from the sampling depths as the depth at which the nutrient gradient increased significantly (57 ± 52 nM m–1 for N+N and 4 ± 5 nM m–1 for P) in the upper water column. The data for some environmental parameters, including SPAR, SST, SI, DM, surface salinity (Sal), NS, PS, surface chlorophyll a (Chl), and depth of chlorophyll a maximum (DCM) were previously published in Tuo et al. (2017).
Isolation and Identification of FL-C Strain
A unicyanobacterial strain of FL C. rhizosoleniae was isolated from the surface water in the SCS collected at 21°25′N, 119°28′E on December 10, 2007 (cruise OR1-852) and enriched with nitrate (10 μM final concentration). After a 1-week incubation in the nitrate-enriched natural seawater, cells were transferred into new polycarbonate (PC) bottles and placed near the window at room temperature (around 19–28°C) in the laboratory. Brownish-yellow clumps appeared at the bottom of the bottles after 3 weeks, and these were transferred into a new PC bottle containing YBCII culture medium (Chen et al., 1996) using a pneumatic micropipette under a Zeiss inverted microscope. Solitary filaments were visible in the bottle a few days later, and these were isolated individually, rinsed several times with YBCII medium, and then transferred to a sterilized Pyrex flask with a cotton stopper containing YBCII medium. A stir bar was placed inside the flask, and the flask was placed on a magnetic stir plate to keep the filaments in suspension. The unicyanobacterial culture was then incubated in a light and temperature-controlled incubator at an illumination intensity of 100–120 μmol quanta m–2 s–1 on a 12:12-h light:dark cycle at 28°C.
Both molecular phylogenetic and morphological examinations were done to identify the strain of isolated FL-C. Phylogenetic analysis based on nifH gene sequence (Foster and Zehr, 2006) was applied with several minor modifications (Taniuchi et al., 2012). The nifH primers and nested polymerase chain reaction protocol followed Zehr and Turner (2001). Cloned deoxyribonucleic acid (DNA) fragments were sequenced by Tri-I Biotech (Taipei, Taiwan). The sequences were aligned by ClustalW program of DDBJ1. A phylogenetic tree was then constructed via neighbor-joining by ClustalW. Distance matrix of deduced amino acid sequences of nifH were calculated with Kimura’s model. The bootstrapped value was based on 1,000 iterations to test for robustness. For the phylogenetic analysis, the nifH genes of Trichodesmium sp. IMS 101 and Trichodesmium thiebautii were used as the outgroups. The identity with close strains was calculated by the identity matrix of BioEdit 4.0 (Hall, 1999). The nifH sequences determined in the study have been submitted in the GenBank database2 under Accession No.: KF284162. Morphological observations included size of heterocysts, the size of heterocysts relative to adjacent vegetative cells, and the tapering of filaments, to distinguish Calothrix from Richelia (Tuo et al., 2017).
Ultrastructural Analysis on Cultured FL-C
To verify whether gas vesicles were present in cells of Calothrix, ultrathin sections of cells were examined using a transmission electron microscope (TEM) on April 7, 2015. The examined filaments, harvested from the laboratory cultures in March 2015, were first transferred to 2.5% glutaraldehyde fixative (in YBCII), then postfixed using 1% osmium tetroxide, dehydrated in an acetone series, embedded in Spurr’s resin (Spurr, 1969), and sectioned with an ultramicrotome (EM UC7, Leica). Ultrathin sections were double stained with uranyl acetate and lead citrate (Reynolds, 1963) and observed under TEM (Tecnai G2 Spirit TWIN, FEI) at 80 kV.
Statistical Analysis
To compare the trichome lengths among the seven types of Richelia and Calothrix (H-R, R-R, G-R, FL-R, B-C, C-C, and FL-C), one-way analysis of variance (ANOVA) and a post hoc Tukey-Kramer test with 0.05 of significance level were used. Before the ANOVA, data from the four region-season classifications were combined for each type, respectively. Differences in trichome lengths of Calothrix and Richelia and in hydrographic parameters and biogeochemical parameters between regions (SCS vs. PS) and seasons (NEM vs. SWM) were compared using two-way ANOVA. For the unbalanced datasets, a Type II sum-of-square method was applied for the ANOVA. If the interaction effect between region and season was significant (p < 0.05), then a post hoc Tukey-Kramer test was performed to investigate the difference among the four region–season classifications (SCS-NEM, SCS-SWM, PS-NEM, and PS-SWM). All the data were presented as mean ± 1 standard deviation. SAS program (SAS Institute) was used for all statistical procedures.
Results
Trichome Lengths of Richelia and Calothrix in the Field
The combined field data showed that trichome lengths were different among the different symbiotic styles of Calothrix and Richelia (Figures 2A–G). On average, H-R had the shortest trichome length (3.5 ± 0.9 cells), followed by B-C (4.9 ± 1.3 cells) and C-C (5.2 ± 1.4 cells), and R-R (7.4 ± 2.2 cells) and G-R (8.0 ± 1.7 cells) had the longest trichome length (p < 0.05). The differences between B-C and C-C and between R-R and G-R were not significant (p > 0.05). FL-C showed longer average trichome length (5.9 ± 1.9 cells) than its symbiotic counterparts B-C and C-C (p < 0.05). FL-R also showed longer trichome length (8.3 ± 2.7 cells) than its symbiotic counterpart R-R (p < 0.05); however, it showed comparable (p > 0.05) trichome lengths with G-R. The FL cyanobacteria were able to develop longer trichomes than their symbiotic counterparts, according to the maximum observed cells per trichome (22 cells for FL-R and 19 cells for FL-C) (Figures 2B–G).
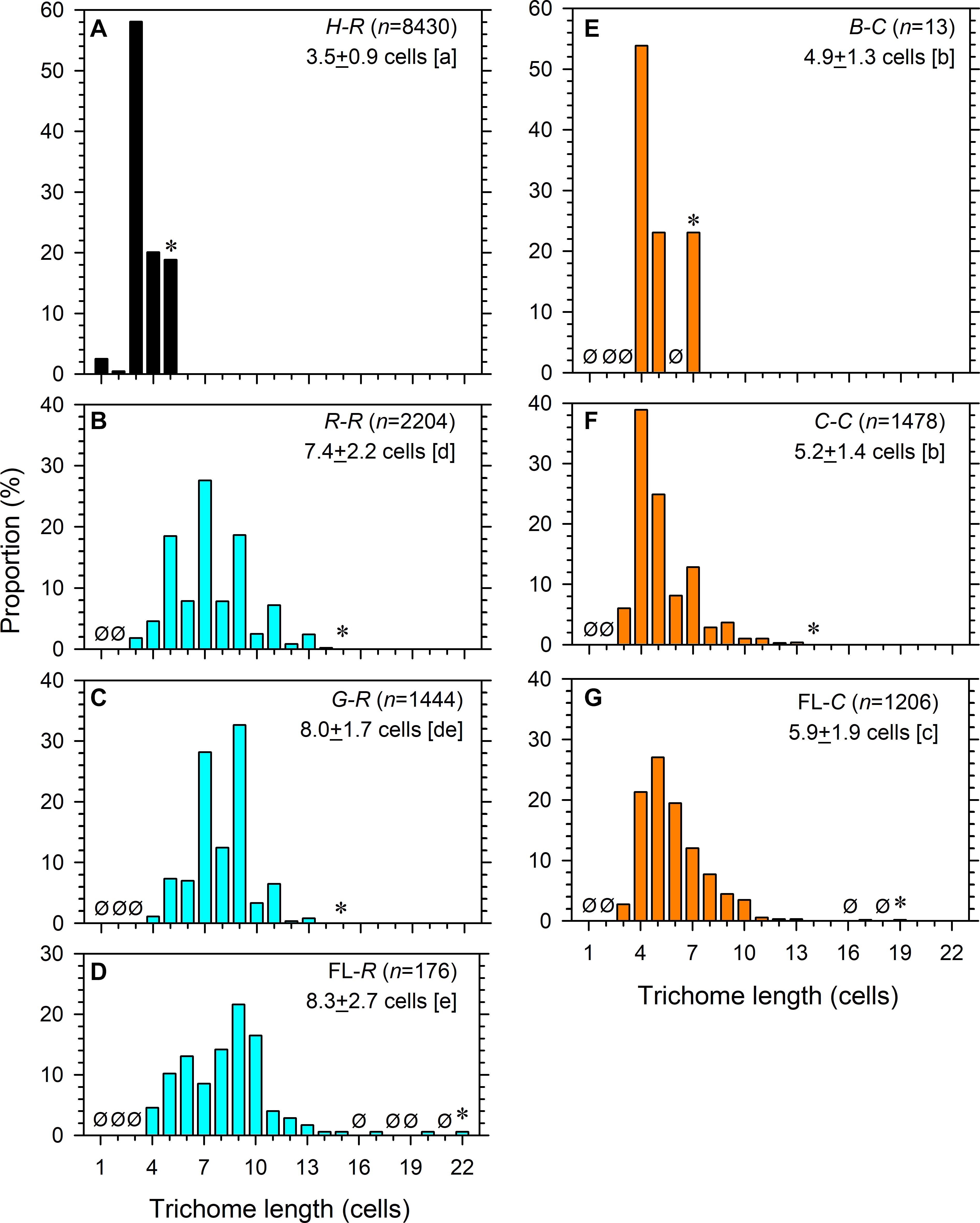
Figure 2. Trichome lengths of (A) internal and (B,C) partial symbionts Richelia and (D) free-living Richelia, as well as (E,F) external symbionts Calothrix and (G) free-living Calothrix. Ø indicates none observed at the length. Asterisk indicates the maximal length observed. n, number of filaments examined. The mean ± 1 standard deviation of length is noted. Different lowercase letters in the brackets refer to significant difference (p < 0.05) of the mean after ANOVA (F6, 14,944 = 3,645, p < 0.0001) and Tukey-Kramer test.
Among the host-associated and FL filaments, some of them showed significant seasonal and/or regional differences in their average trichome length, although the difference was only around 1 cell per trichome (Table 2). H-R had longer trichomes during the NEM than the SWM in both regions and had longer trichomes in the SCS than in the PS during both seasons (p < 0.05) (Table 2 and Supplementary Figure 1). The trichome length of R-R was longer in the SCS than the PS during the SWM and was longer during the NEM than during the SWM in the PS (p < 0.05) (Table 2 and Supplementary Figure 2). Similarly, the trichome length of G-R was also longer in the SCS than the PS during the SWM (p < 0.05) (Table 2 and Supplementary Figure 3). However, FL-R had longer trichomes in the SCS than the PS during both seasons (p < 0.05), but the seasonal differences were not significant (p > 0.05) (Table 2 and Supplementary Figure 4). On the other hand, the symbiotic Calothrix samples did not show regional and seasonal differences. Specifically, the trichome length of C-C was comparable (p > 0.05) between the regions and/or between the seasons (Table 2 and Supplementary Figure 5). The data of B-C were too few for statistical tests (Table 2 and Supplementary Figure 6). However, FL-C had longer trichomes during the NEM than the SWM in both regions and had longer trichomes in the SCS than the PS during both seasons (p < 0.05) (Table 2 and Supplementary Figure 7).
Hydrographic and Biogeochemical Properties in the Study Areas
Hydrographic characteristics, including SPAR, SST, SI, and DM, differed significantly (p < 0.05) between seasons in both the SCS and the PS. The first three parameters were higher during the SWM than during the NEM. SST was also higher in the PS than the SCS during both seasons (p < 0.05). On the other hand, DM was deeper during the NEM than during the SWM in both regions (p < 0.01) and was deeper in the PS than the SCS during both seasons (p < 0.05). The SI was higher in the SCS than the PS during the SWM (p < 0.05), but during the NEM, it was comparable between the regions (p > 0.05) (Table 3).
The SCS had significantly higher NI and PI (p < 0.01) than the PS during both seasons. The SCS also had significantly (p < 0.05) higher NS and PS than the PS during the NEM. However, there were no differences in NS and PS between the two regions during the SWM (Table 3). Both DN and DP were deeper in the PS than the SCS during both seasons (p < 0.01). In the SCS, NS, and PS were significantly (p < 0.05) higher during the NEM than the SWM. In contrast, seasonal variations in NS and PS in the PS were not significant (p > 0.05) (Table 3). Significant differences in DCM were observed seasonally or regionally (p < 0.01). Seasonal differences in Chl were also observed in the SCS but not in the PS. Ratios of N:P (NS:PS and NI:PI) were consistently below the Redfield ratio of 16 (Redfield, 1958), suggesting that phytoplankton growth was limited by N in both regions (Table 3).
In summary, the SCS-NEM stations had the lowest SST, the highest NS, PS, and Chl, and the shallowest DCM. On the other hand, there were significant regional differences in nutrient concentrations; i.e., higher NI and PI and shallower DN and DP in the SCS than the PS (Table 3).
Identification of the Cultured FL-C
The isolated FL-C strain, M15, was positively identified as C. rhizosoleniae according to phylogenetic (Figure 3) and morphological examinations (Figure 4). Phylogenetic trees reconstructed from nifH-deduced amino acid sequences revealed that the isolated strain was included in the het-3 clade (Figure 3) that contained C. rhizosoleniae strain SC01, which was isolated from the North Pacific (Foster and Zehr, 2006). In the analysis of nifH DNA sequence, the strain showed high identity to C. rhizosoleniae SC01 (het-3) (99.6–100%). In contrast, its identity to Richelia associated with Rhizosolenia (het-1) or Hemiaulus (het-2) was ≤91.3% (Supplementary Table 1). Morphologically, a filament of the isolate consisted of several vegetative cells followed by one basal heterocyst covered with thin hyaline sheath and lacked hair formation (Figures 4A,B). The trichome length in maximum and in minimum recorded two and 114 cells, respectively. The heterocyst was often smaller (Figures 4C,D) or equal in diameter to its adjacent vegetative cell (Figures 4A,B,E,F). Most of the newly divided filaments had slightly tapering ends and sometimes empty sheaths near their ends (Figures 4A–C). Some longer filaments tapered in the center and had a developing rounded end-cell at its opposite end suggesting the filament was getting ready to divide (Figures 4D,E). The narrowest cell at the middle part would become a necridium (Figure 4E) from which a filament would divide into the longer mother filament the shorter hormogonium.
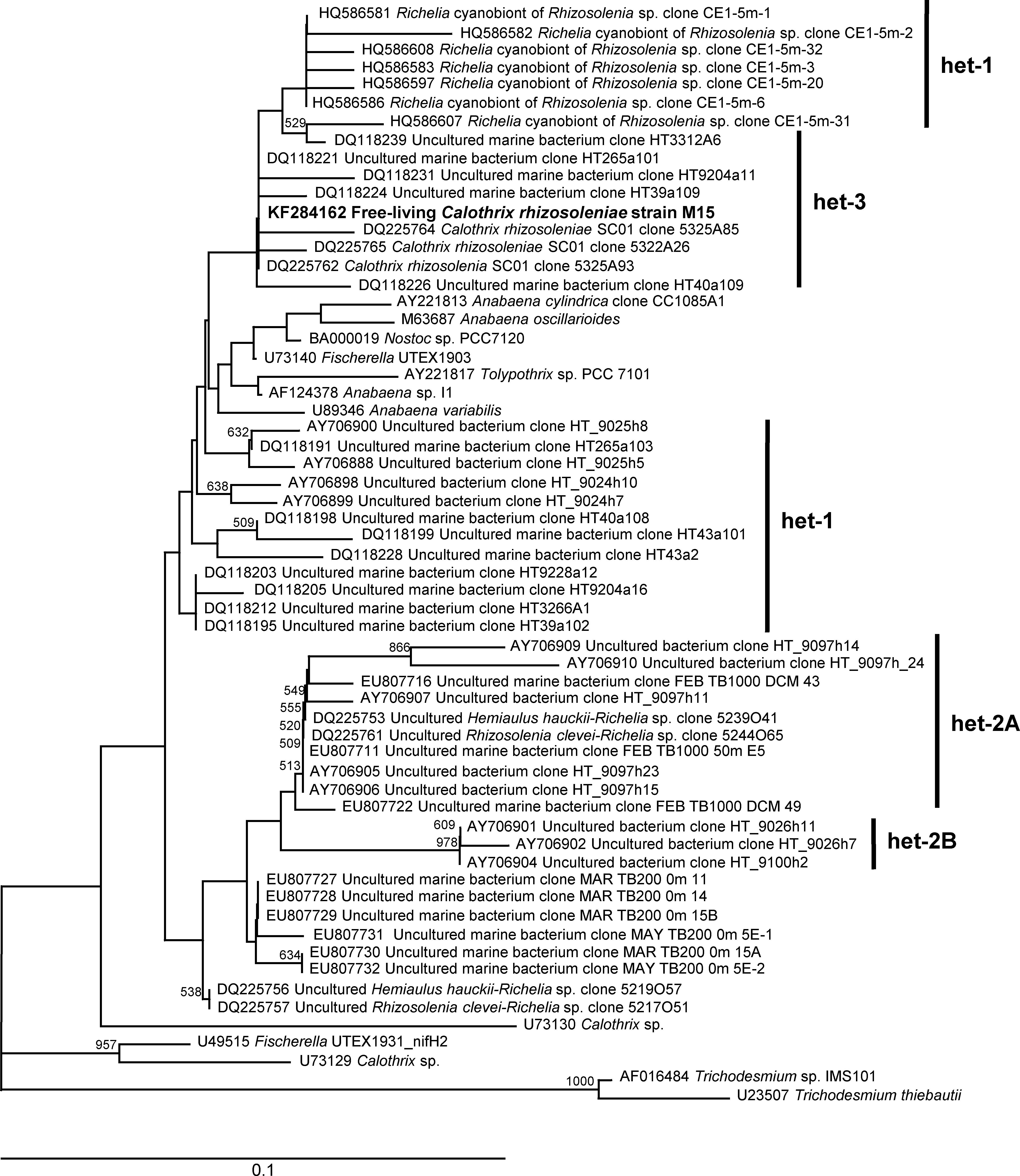
Figure 3. Neighbor-joining phylogenetic tree for partial nifH amino acid sequences. The sequence obtained from Calothrix rhizosoleniae strain M15 (KF284162) is indicated in boldface. The bootstrap values (>500) are indicated at the nodes. The scale bar represents 0.1 substitutions per site, and the nifH genes of Trichodesmium sp. IMS 101 (AF016484) and Trichodesmium thiebautii (U23507) were used as the outgroups.
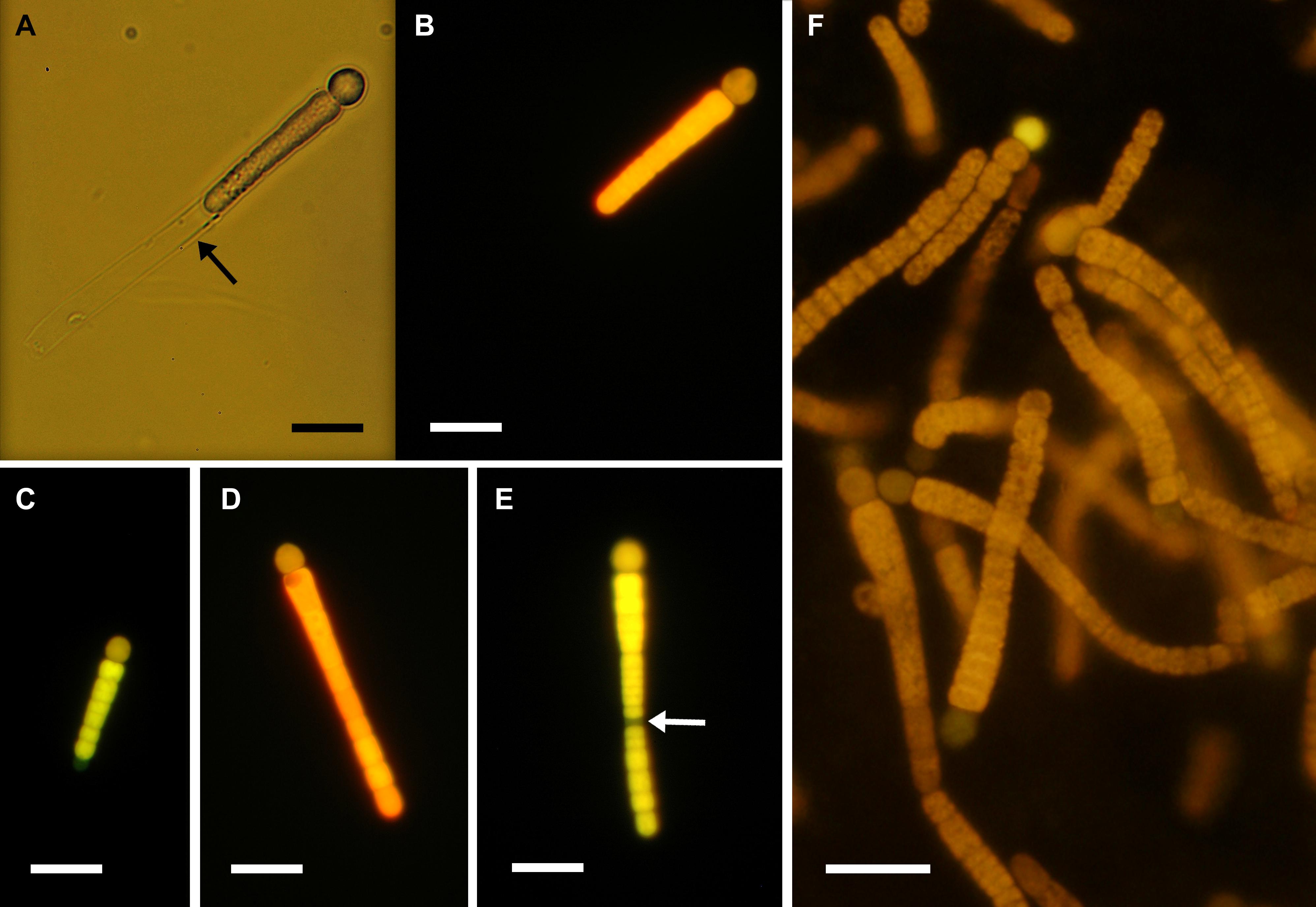
Figure 4. (A–E) Solitary or (F) clumping filaments of Calothrix rhizosoleniae strain M15 under (A) bright field and (B–F) epi-fluorescence microscopy. Black and white arrows indicate empty sheath and necridium, respectively. Scale bar: 10 μm.
Ultrastructure of Cultured FL-C
The images of thin sections showed no gas vesicles in either vegetative cells or heterocysts in the cultured FL-C (Figure 5). The photosynthetic thylakoids were more abundant and denser in vegetative cells (Figures 5D,E) but distributed sparsely in heterocysts (Figures 5B,C) and necridia (Figure 5A and Supplementary Figure 8). The difference in thylakoid abundance among vegetative cells, heterocysts, and necridia was apparent from epifluorescence microscopy, i.e., vegetative cells often had brighter fluorescence than heterocysts and necridia (Figures 4B–F). Carboxysomes, cyanophycin granules, osmiophilic granules, and electron-transparent areas were found in both heterocysts and vegetative cells (Figure 5).
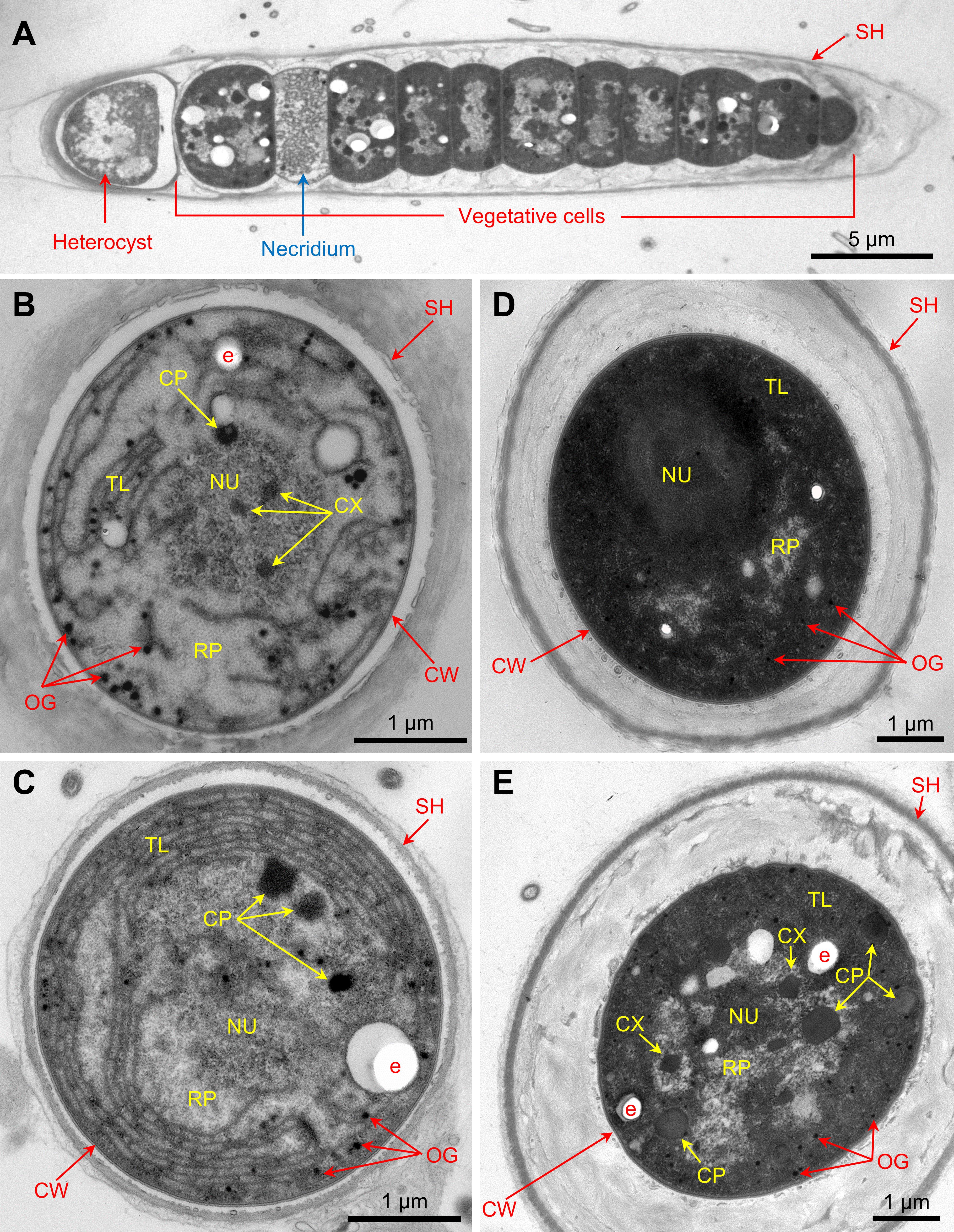
Figure 5. Thin sections of (A) a filament, (B,C) heterocysts, and (D,E) vegetative cells of the FL-C strain. SH, sheath; CW, cell wall; TL, thylakoids; RP, riboplasm; NU, nucleoid; CP, cyanophycin granule; CX, carboxysome; OG, osmiophilic granule; e, electron-transparent area.
Discussion
Different Trichome Lengths Among Host-Specific Symbionts
The field results showed that the trichome lengths were different among the three types of Richelia/Calothrix symbionts, classified by Nieves-Morión et al. (2020). The internal symbiont (H-R) had the shortest trichome lengths, followed by the external symbiont (Calothrix). The partial/periplasmic symbiont (R-R, FL-R, and perhaps G-R) had the longest trichome lengths (Figure 2). According to a coarse-grained cell flux model of the Hemiaulus-Richelia symbiosis (CFM-DDA), the Hemiaulus host provided its symbiont with two-thirds of its fixed carbon (C) requirement (Inomura et al., 2020). Because the Hemiaulus host can provide such a large proportion of their fixed C requirement, an H-R symbiont may not need to maintain as many vegetative cells and so form shorter trichomes (Sundström, 1984; Villareal, 1994; Foster and Zehr, 2006; Foster et al., 2007; Bar Zeev et al., 2008; Lyimo, 2011; Hilton et al., 2013), consistent with our field observations (Table 4). In addition, a reduction in H-R symbiont vegetative cells/trichome length may facilitate their dwelling in the compact cytoplasm of their Hemiaulus hosts (Caputo et al., 2019). On the other hand, external symbionts (C-C and B-C) (Lemmermann, 1905; Janson et al., 1999; Foster and Zehr, 2006) and periplasmic symbionts (R-R) (Schmidt, 1901; Janson et al., 1999; Zehr et al., 2007; Bar Zeev et al., 2008; Kulkarni et al., 2010; Padmakumar et al., 2010; Lyimo, 2011; Jabir et al., 2013; Madhu et al., 2013; Table 4) may require more vegetative cells to satisfy their fixed C demand as their symbiotic locations lead to less-efficient exchange of molecules (e.g., fixed C and N) than internal symbionts. The comparable trichome lengths between R-R and G-R (Figures 2B,C) suggest that G-R is also a periplasmic symbiont. The fact that both FL-R and FL-C showed longer trichomes (both average and maximum trichome lengths) than their symbiotic counterparts (R-R and C-C) (Figure 2) was also predicted by the CFM-DDA (Inomura et al., 2020). This study reports the first quantification of G-R, FL-R, B-C, and FL-C trichome lengths from natural waters. For H-R, R-R, and C-C, our observations of trichome length from the SCS and PS were comparable with those reported from other oceanic regions (Table 4). In a previous global model study, the C biomass of Calothrix and Richelia were calculated by using a fixed number of vegetative cells per trichome (trichome length minus 1) without considering variations in trichome length for symbionts associated with different diatom hosts (Luo et al., 2012). Our results suggest that trichome length varies based on the nature of the DDA, and this should be considered in future models.
The exceptionally short trichome length of H-R observed in this study (Figure 2A) was similar to an earlier study (Table 4; Foster et al., 2007). The short trichomes of H-R are thought to be strongly manipulated or restricted by their hosts; they have an extremely slimmed genome and are located in the host’s cytosol (Hilton et al., 2013; Caputo et al., 2019). Unlike Calothrix, which is considered a facultative symbiont or an opportunist, H-R is unable to live alone without its host. The limited field data from this study suggests that all of the observed FL-R filaments were similar in length to those associated with Rhizosolenia and Guinardia. In a previous study, it was found that none of the FL-R filaments were derived from Hemiaulus (Tuo et al., 2017). Rhizosolenia-associated Richelia may be able to live outside of its host for at least a short time because its genome is larger than that of Hemiaulus-associated Richelia, probably of metabolic necessity since its symbiotic location is just outside the host’s cytosol (Villareal, 1990; Hilton, 2014; Caputo et al., 2019). Microscopic evidence from field samples have shown Richelia filaments escaping from moribund or dead Rhizosolenia hosts by direct penetration through the frustule or attached on the plasmalemma of Rhizosolenia lacking a frustule (Villareal, 1989; Gómez et al., 2005) supporting this assertion.
Although the average trichome lengths between FL and symbiotic Calothrix were comparable, the maximum lengths of the FL-C trichomes were much longer than B-C and C-C (Figures 2E–G), implying that Bacteriastrum and Chaetoceros hosts prefer their epiphytic symbionts to be short. In addition, with only a single heterocyst, a long filament might not be as beneficial to their host in providing fixed N (Foster et al., 2011). As an epiphyte, a long filament might also increase N loss to the surrounding seawater due to the inefficiency of a long-trichome N transport. In addition, longer filaments have more vegetative cells thereby increasing the N demand of the cyanobacterial symbiont itself. Overall, shorter Calothrix seemed to benefit their Chaetoceros and Bacteriastrum hosts by not weighing them down and providing better transfer efficiency for the recently fixed N.
Spatiotemporal Variations of Trichome Lengths
Field results showed significant regional and seasonal differences in the trichome lengths of H-R and FL-C; they were longer in the SCS than the PS and longer during the NEM than the SWM. Similarly, FL-R trichomes were longer in the SCS than in the PS during both seasons, whereas R-R and G-R trichomes were longer in the SCS than the PS only during the SWM. The trichomes of R-R and G-R were also longer during the NEM than the SWM in the PS and the SCS, respectively. Such spatiotemporal differences in trichome lengths were accompanied by variations in nutrient supplies among regions and seasons. Both higher nutrient inventories (NI and PI) and shallower nutriclines (DN and DP) were observed in the SCS than in the PS during both seasons. In the SCS, higher surface nutrient concentrations (NS and PS) seemed related to longer trichome lengths during the NEM than the SWM. In the PS, although NS and PS were seasonally comparable, relatively high nutrient fluxes, reflected in the lower SI and deeper DM, may have contributed to the longer trichome lengths during the NEM. Furthermore, higher Chl and shallower DCM also reflected the relatively high nutrient supplies in the SCS and during the NEM. The correlation matrix also showed positive relationships between trichome length of H-R and nutrients (N+N or P); however, the correlation coefficients were very low (r < 0.10) (Supplementary Table 2). Like nutrients, certain physical variables including SST and SPAR also showed significant regional and seasonal differences (Table 2). The correlation matrix also showed negative relationships between trichome lengths of H-R, R-R, and FL-C and temperature (Supplementary Table 2). However, temperature (or SST) was usually highly correlated with nutrients (Supplementary Table 3; Tuo et al., 2017). Nutrient stress has been considered a cause of short trichome length in cyanobacteria (Smith and Gilbert, 1995). For example, when a freshwater cyanobacterium Oscillatoria agardhii was under a period of P limitation, its cell phosphorus content declined and its trichomes became progressively shorter (Gibson and Stevens, 1979). Our results imply that higher nutrient concentrations (especially P) might support the production of longer trichomes of both Richelia and Calothrix.
In contrast to the other associations and free-living Richelia and Calothrix, C-C had comparable trichome lengths regardless of the region or season. Unlike endosymbiotic Richelia, which inhabit space inside the host cells, C-C attach to the hosts’ apertures and are oriented transversely to the long axis of the chains (Gómez et al., 2005; Tuo et al., 2014). As a facultative symbiont or an opportunist, a filament of C-C could easily detach from its host and lengthen, especially under replete nutrient conditions (Tuo et al., 2017). The clear spatiotemporal length variations of the FL-C trichome relative to C-C and B-C are reflected in Table 4.
It should be noticed that regional and/or seasonal differences in the mean trichome length was around one vegetative cell for most types of Calothrix (∼1.0 cells) and Richelia (0.3–1.7 cells), except FL-C and G-R (Table 2). The larger difference for G-R (4.1 cells) was likely due to the very small sample size at the SCS-NEM stations (n = 2). When ignoring the SCS-NEM sample, the regional difference during the SWM for G-R was also around one cell. On the other hand, FL-C showed larger spatiotemporal differences (0.5–3.2 cells), implying stronger influences from ambient nutrient availability. However, the small variation in symbiotic Calothrix and Richelia trichome length (i.e., one cell) implies that nutrient availability in this study area was not a primary control on trichome length in the SCS and PS.
Lack of Gas Vesicles in C. rhizosoleniae Cells
Gas vesicles are common cellular components in freshwater species of heterocystous cyanobacteria such as Dolichospermum spp., Aphanizomenon spp., Nostoc spp., and Calothrix epiphytica, which usually have elongated trichomes with tens to hundreds of cells per trichome (West and West, 1897; Walsby, 1994; Wacklin et al., 2009). We found that C. rhizosoleniae lacks gas vesicles (Figure 5), similar to Richelia, suggesting poor buoyancy regulation or an inclination to sink (Janson et al., 1995). The lack of gas vesicles in cultured C. rhizosoleniae might have been the result of acclimation to cultivation; cultures were isolated in December 2007 and harvested for TEM in March 2015. To support our ultrathin section results (Figure 5), we also examined gas vesicle structural proteins (Gvp) in the genomes of C. rhizosoleniae, R. intracellularis, and other representative filamentous cyanobacteria to those of Dolichospermum flos-aquae CCAP 1403/13F (GenBank: U17109.1). The similarity comparisons showed that C. rhizosoleniae (SC01, a FL strain isolated from Chaetoceros hosts), R. intracellularis (RC01, associated with Rhizosolenia hosts), and certain species of Calothrix lacked the sequences of the structural protein (GvpA), large outer surface protein (GvpC), and putative gas vesicle protein (GvpN, GvpA/J, GvpK, GvpF/L, GvpG, GvpV, and GvpW) (Table 5 and Supplementary Tables 4, 5). While GvpP was found and is also a putative protein related to gas vesicles, it showed no obvious amino acid identity to the other gvp gene products (especially GvpA) (Li and Cannon, 1998). Thus, the genomic information supported the observation that C. rhizosoleniae and R. intracellularis cells lacked gas vesicles. Without the ability to produce gas vesicles, cyanobacterial species must regulate their buoyancy by turgor collapse and accumulation of condensed carbohydrate (Reynolds et al., 1987), and/or they might decrease their filament volume by shortening trichome length to control their buoyancy/sinking, if the Stokes’ Law worked for them.
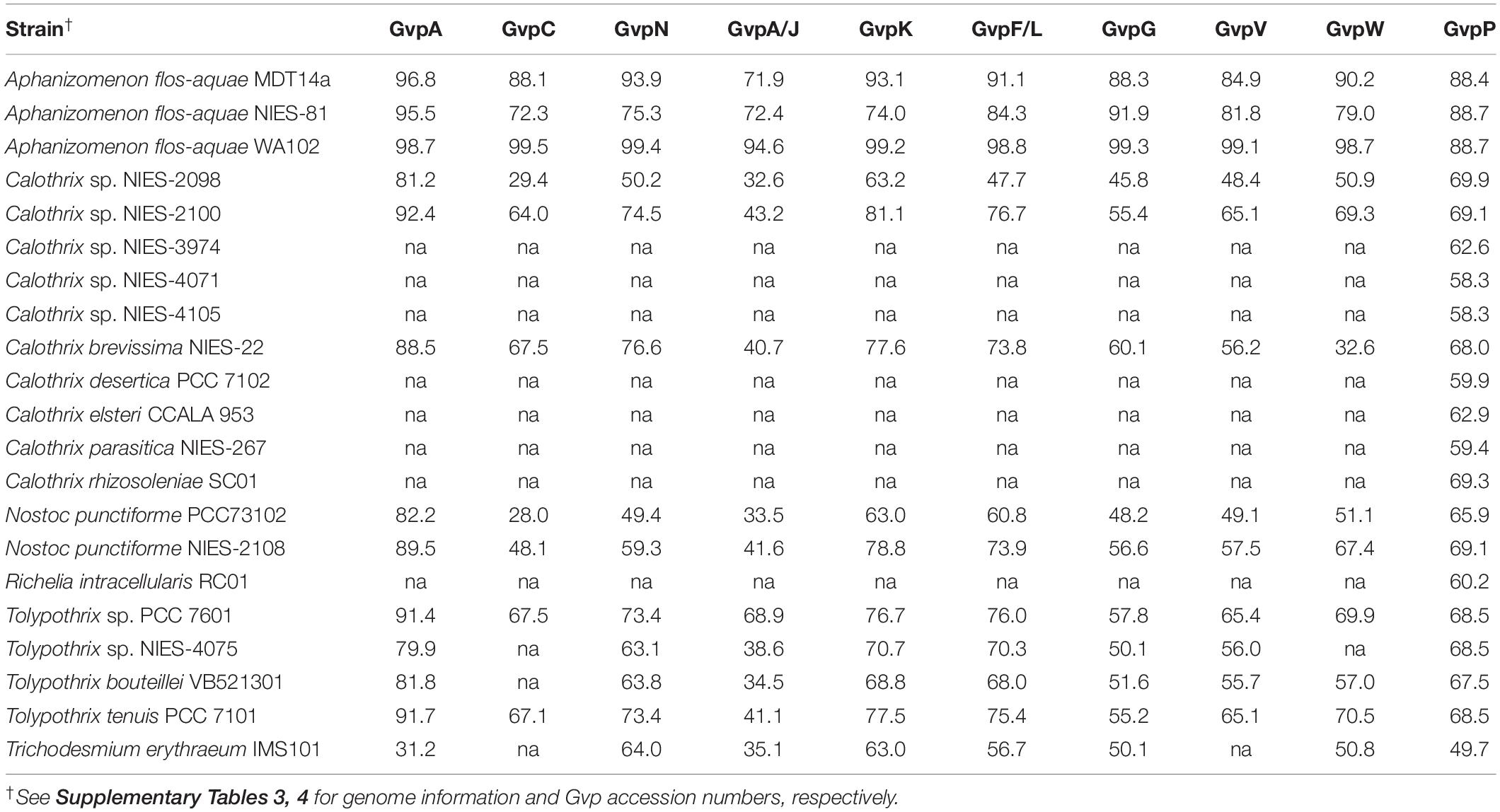
Table 5. Similarities of gas vesicle structural proteins (Gvp) between cyanobacterial diazotrophs and Dolichospermum flos-aquae CCAP 1403/13F (GenBank: U17109.1) by BLASTp.
Summary
The trichome length of diatom host-associated symbionts may be controlled by their hosts/symbiotic styles. On average, the internal Richelia symbiont (H-R) produced the shortest trichomes, followed by the external Calothrix symbionts (C-C and B-C); the periplasmic Richelia symbionts (R-R and perhaps G-R) produced the longest trichomes. The trichome lengths of FL-C and FL-R were comparable with their symbiotic counterparts C-C and R-R, respectively. Regional and/or seasonal differences in trichome length were observed for various types of Richelia and FL-C. However, the difference in length was only about one cell per trichome. Both Richelia and Calothrix (rhizosoleniae) may have limited buoyancy regulation due to their lack of gas vesicles and the ability to produce them. This may require them to maintain shorter trichome lengths unlike other filamentous cyanobacterial species that have gas vacuoles.
Data Availability Statement
The datasets presented in this study can be found in online repositories. The names of the repository/repositories and accession number(s) can be found in the article/Supplementary Material.
Author Contributions
ST and YLLC conceived the idea. ST, MRM, and YLLC wrote the manuscript with contributions from YT. ST, YT, WNJ, and YHL performed the experiments and created the figures and tables. ST, MRM, YLLC, YT, and HYC revised the manuscript. All authors reviewed the manuscript.
Funding
This research was in part supported by the Taiwan Ministry of Science and Technology (formerly, National Science Council) through the grants NSC (94-2611-M-110-009, 95-2611-M-110-002, 95-2611-M-110-004, 96-2628-M-110-005, 97-2628-M-110-002, 98-2628-M-110-002, 99-2611-M-110-015, 100-2611-M-110-001, 101-2611-M-110-001, and 102-2611-M-110-001); MOST (103-2611-M-110-001, 104-2611-M-110-005, 104-2811-M-110-009, and 108-2917-I-564-017), and in part from the Asia-Pacific Ocean Research Center, National Sun Yat-sen University, Kaohsiung, Taiwan.
Conflict of Interest
The authors declarethat the research was conducted in the absence of any commercial or financial relationships that could be construed as a potential conflict of interest.
Acknowledgments
We thank Mei-Ling Tsai for the DNA cloning, Takuhei Shiozaki, Hsiao-Hsueh Shen, Yuan Ju Liao, Tze Ching Yong, Chien-Chih Huang, Bo-Ruei Huang, Yi-Xain Liu, Lian-Fen Jian, Hong Jun Ma, Jiunn Lin Tzeng, Hanlin Lai, Tien Yi Chen, and Che-Chen Chuang, as well as the captains, crews, and technicians on R/Vs Ocean Researcher I and III for assistance in field collections.
Supplementary Material
The Supplementary Material for this article can be found online at: https://www.frontiersin.org/articles/10.3389/fmars.2021.678607/full#supplementary-material
Footnotes
References
Bar Zeev, E., Yogev, T., Man-Aharonovich, D., Kress, N., Herut, B., Béja, O., et al. (2008). Seasonal dynamics of the endosymbiotic, nitrogen-fixing cyanobacterium Richelia intracellularis in the eastern Mediterranean Sea. ISME J. 2, 911–923. doi: 10.1038/ismej.2008.56
Capone, D. G., Burns, J. A., Montoya, J. P., Subramaniam, A., Mahaffey, C., Gunderson, T., et al. (2005). Nitrogen fixation by Trichodesmium spp.: an important source of new nitrogen to the tropical and subtropical North Atlantic Ocean. Glob. Biogeochem. Cycles 19:GB2024.
Capone, D. G., Zehr, J. P., Paerl, R. W., Bergman, B., and Carpenter, E. J. (1997). Trichodesmium, a globally significant marine cyanobacterium. Science 276, 1221–1229. doi: 10.1126/science.276.5316.1221
Caputo, A., Nylander, J. A., and Foster, R. A. (2019). The genetic diversity and evolution of diatom-diazotroph associations highlights traits favoring symbiont integration. FEMS Microbiol. Lett. 366:fny297.
Carpenter, E. J., and Capone, D. G. (2008). “Dinitrogen fixation,” in Nitrogen in the Marine Environment, eds D. G. Capone, M. R. Mulholland, and E. J. Carpenter (Amsterdam: Elsevier), 141–198.
Carpenter, E. J., and Janson, S. (2001). Anabaena gerdii sp. nov., a new planktonic filamentous cyanobacterium from the South Pacific Ocean and Arabian Sea. Phycologia 40, 105–110.
Carpenter, E. J., Montoya, J. P., Burns, J., Mulholland, M. R., Subramaniam, A., and Capone, D. G. (1999). Extensive bloom of a N2-fixing diatom/cyanobacterial association in the tropical Atlantic Ocean. Mar. Ecol. Prog. Ser. 185, 273–283. doi: 10.3354/meps185273
Caruso, M. J., Gawarkiewicz, G. G., and Beardsley, R. C. (2006). Interannual variability of the Kuroshio Intrusion in the South China Sea. J. Oceanogr. 62, 559–575. doi: 10.1007/s10872-006-0076-0
Chen, T. Y., Chen, Y. L. L., Sheu, D. S., Chen, H. Y., Lin, Y. H., and Shiozaki, T. (2019). Community and abundance of heterotrophic diazotrophs in the northern South China Sea: revealing the potential importance of a new alphaproteobacterium in N2 fixation. Deep Sea Res. I 143, 104–114. doi: 10.1016/j.dsr.2018.11.006
Chen, Y. B., Zehr, J. P., and Mellon, M. (1996). Growth and nitrogen fixation of the diazotrophic filamentous nonheterocystous cyanobacterium Trichodesmium sp. IMS 101 in defined media: evidence for a circadian rhythm. J. Phycol. 32, 916–923. doi: 10.1111/j.0022-3646.1996.00916.x
Chen, Y. L. L., Chen, H. Y., Lin, Y. H., Yong, T. C., Taniuchi, Y., and Tuo, S. (2014). The relative contributions of unicellular and filamentous diazotrophs to N2 fixation in the South China Sea and the upstream Kuroshio. Deep Sea Res. I 85, 56–71. doi: 10.1016/j.dsr.2013.11.006
Chen, Y. L. L., Chen, H. Y., Tuo, S., and Ohki, K. (2008). Seasonal dynamics of new production from Trichodesmium N2 fixation and nitrate uptake in the upstream Kuroshio and South China Sea basin. Limnol. Oceanogr. 53, 1705–1721. doi: 10.4319/lo.2008.53.5.1705
Chen, Y. L. L., Tuo, S.-H., and Chen, H. Y. (2011). Co-occurrence and transfer of fixed nitrogen from Trichodesmium spp. to diatoms in the low-latitude Kuroshio Current in the NW Pacific. Mar. Ecol. Prog. Ser. 421, 25–38. doi: 10.3354/meps08908
Foster, R. A., Goebel, N. L., and Zehr, J. P. (2010). Isolation of Calothrix rhizosoleniae (Cyanobacteria) strain SC01 from Chaetoceros (Bacillariophyta) spp. diatoms of the subtropical North Pacific Ocean. J. Phycol. 46, 1028–1037. doi: 10.1111/j.1529-8817.2010.00885.x
Foster, R. A., Kuypers, M. M., Vagner, T., Paerl, R. W., Musat, N., and Zehr, J. P. (2011). Nitrogen fixation and transfer in open ocean diatom-cyanobacterial symbioses. ISME J. 5, 1484–1493. doi: 10.1038/ismej.2011.26
Foster, R. A., Subramaniam, A., Mahaffey, C., Carpenter, E. J., Capone, D. G., and Zehr, J. P. (2007). Influence of the Amazon River plume on distributions of free-living and symbiotic cyanobacteria in the western tropical north Atlantic Ocean. Limnol. Oceanogr. 52, 517–532. doi: 10.4319/lo.2007.52.2.0517
Foster, R. A., and Zehr, J. P. (2006). Characterization of diatom-cyanobacteria symbioses on the basis of nifH, hetR and 16S rRNA sequences. Environ. Microbiol. 8, 1913–1925. doi: 10.1111/j.1462-2920.2006.01068.x
Gibson, C. E., and Stevens, R. J. (1979). Changes in phytoplankton physiology and morphology in response to dissolved nutrients in Lough Neagh, N. Ireland. Freshw. Biol. 9, 105–109. doi: 10.1111/j.1365-2427.1979.tb01494.x
Gómez, F., Furuya, K., and Takeda, S. (2005). Distribution of the cyanobacterium Richelia intracellularis as an epiphyte of the diatom Chaetoceros compressus in the western Pacific Ocean. J. Plankton. Res. 27, 323–330. doi: 10.1093/plankt/fbi007
Grosse, J., Bombar, D., Doan, H. N., Nguyen, L. N., and Voss, M. (2010). The Mekong River plume fuels nitrogen fixation and determines phytoplankton species distribution in the South China Sea during low and high discharge season. Limnol. Oceanogr. 55, 1668–1680. doi: 10.4319/lo.2010.55.4.1668
Hall, T. A. (1999). BioEdit: a user-friendly biological sequence alignment editor and analysis program for Windows 95/98/NT. Nucleic Acids Symp. Ser. 41, 95–98.
Hilton, J. A. (2014). Ecology and Evolution of Diatom-Associated Cyanobacteria Through Genetic Analyses. PhD Thesis. Santa Cruz, CA: University of California Santa Cruz.
Hilton, J. A., Foster, R. A., Tripp, H. J., Carter, B. J., Zehr, J. P., and Villareal, T. A. (2013). Genomic deletions disrupt nitrogen metabolism pathways of a cyanobacterial diatom symbiont. Nat. Commun. 4:1767.
Inomura, K., Follett, C. L., Masuda, T., Eichner, M., Prášil, O., and Deutsch, C. (2020). Carbon transfer from the host diatom enables fast growth and high rate of N2 fixation by symbiotic heterocystous cyanobacteria. Plants 9:192. doi: 10.3390/plants9020192
Jabir, T., Dhanya, V., Jesmi, Y., Prabhakaran, M. P., Saravanane, N., Gupta, G. V. M., et al. (2013). Occurrence and distribution of a diatom-diazotrophic cyanobacteria association during a Trichodesmium bloom in the southeastern Arabian Sea. Int. J. Oceanogr. 2013:350594. doi: 10.1155/2013/350594
Jan, S., Yang, Y. J., Wang, J., Mensah, V., Kuo, T. H., Chiou, M. D., et al. (2015). Large variability of the Kuroshio at 23.75°N east of Taiwan. J. Geophys. Res. Oceans 120, 1825–1840. doi: 10.1002/2014jc010614
Janson, S., Rai, A. N., and Bergman, B. (1995). Intracellular cyanobiont Richelia intracellularis: ultrastructure and immuno-localisation of phycoerythrin, nitrogenase, Rubisco and glutamine synthetase. Mar. Biol. 124, 1–8. doi: 10.1007/bf00349140
Janson, S., Wouters, J., Bergman, B., and Carpenter, E. J. (1999). Host specificity in the Richelia-diatom symbiosis revealed by hetR gene sequence analysis. Environ. Microbiol. 1, 431–438. doi: 10.1046/j.1462-2920.1999.00053.x
Karsten, G. (1907). “Das indische Phytoplankton nach dem Material der deutschen Tiefsee-Expedition 1898-1899,” in Wissenschaftliche Ergehniss der deutschen Tiefsee-Expedition auf dem Dampfer “Valdivia” 1898-1899, ed. C. Chun (Jena: Gustav Fischer Verlag), 221–548.
Kimor, B., Gordon, N., and Neori, A. (1992). Symbiotic associations among the microplankton in oligotrophic marine environments, with special reference to the Gulf of Aqaba, Red Sea. J. Plankton. Res. 14, 1217–1231. doi: 10.1093/plankt/14.9.1217
Kulkarni, V. V., Chitari, R. R., Narale, D. D., Patil, J. S., and Anil, A. C. (2010). Occurrence of cyanobacteria-diatom symbiosis in the Bay of Bengal: implications in biogeochemistry. Curr. Sci. 99, 736–737.
Lemmermann, E. (1905). Die Algenflora der Sandwich Inseln. Ergebnisse einer Reise nach dem Pacific, H. Schauinsland 1896/97. Bot. Jahrb. Syst., Pflanzengesch. Pflanzengeogr. 34, 607–633.
Li, N., and Cannon, M. C. (1998). Gas vesicle genes identified in Bacillus megaterium and functional expression in Escherichia coli. J. Bacteriol. 180, 2450–2458. doi: 10.1128/jb.180.9.2450-2458.1998
Liang, W. D., Tang, T. Y., Yang, Y. J., Ko, M. T., and Chuang, W. S. (2003). Upper-ocean currents around Taiwan. Deep Sea Res. II 50, 1085–1105. doi: 10.1016/s0967-0645(03)00011-0
Liu, K. K., Chao, S. Y., Shaw, P. T., Gong, G. C., Chen, C. C., and Tang, T. Y. (2002). Monsoon-forced chlorophyll distribution and primary production in the South China Sea: observations and a numerical study. Deep Sea Res. I 49, 1387–1412. doi: 10.1016/s0967-0637(02)00035-3
Luo, Y.-W., Doney, S. C., Anderson, L. A., Benavides, M., Berman-Frank, I., Bode, A., et al. (2012). Database of diazotrophs in global ocean: abundance, biomass and nitrogen fixation rates. Earth Syst. Sci. Data 4, 7–73. doi: 10.5194/essd-4-47-2012
Lyimo, T. J. (2011). Distribution and abundance of the cyanobacterium Richelia intracellularis in the coastal waters of Tanzania. J. Ecol. Nat. Environ. 3, 85–94.
Madhu, N. V., Meenu, P., Ullas, N., Ashwini, R., and Rehitha, T. V. (2013). Occurrence of cyanobacteria (Richelia intracellularis)-diatom (Rhizosolenia hebetata) consortium in the Palk Bay, southeast coast of India. Indian J. Mar. Sci. 42, 453–457.
Mague, T. H., Weare, N. M., and Holm-Hansen, O. (1974). Nitrogen fixation in the north Pacific Ocean. Mar. Biol. 24, 109–119. doi: 10.1007/bf00389344
Mulholland, M. R., Bernhardt, P. W., Widner, B. N., Selden, C. R., Chappell, P. D., Clayton, S., et al. (2019). High rates of N2 fixation in temperate, western North Atlantic coastal waters expand the realm of marine diazotrophy. Glob. Biogeochem. Cycles 33, 826–840. doi: 10.1029/2018GB006130
Nieves-Morión, M., Flores, E., and Foster, R. A. (2020). Predicting substrate exchange in marine diatom-heterocystous cyanobacteria symbioses. Environ. Microbiol. 22, 2027–2052. doi: 10.1111/1462-2920.15013
Padmakumar, K. B., Menon, N. R., and Sanjeevan, V. N. (2010). Occurrence of endosymbiont Richelia intracellularis (Cyanophyta) within the diatom Rhizosolenia hebetata in Northern Arabian Sea. Int. J. Biodivers. Conserv. 2, 70–74.
Redfield, A. C. (1958). The biological control of chemical factors in the enviroment. Am. Sci. 46, 205–221.
Reynolds, C. S., Oliver, R. L., and Walsby, A. E. (1987). Cyanobacterial dominance: the role of buoyancy regulation in dynamic lake environments. N. Z. J. Mar. Freshw. Res. 21, 379–390. doi: 10.1080/00288330.1987.9516234
Reynolds, E. S. (1963). The use of lead citrate at high pH as an electron-opaque stain in electron microscopy. J. Cell. Biol. 17, 208–212. doi: 10.1083/jcb.17.1.208
Schmidt, J. (1901). Über Richelia intracellularis, eine neue in Plankton-Diatomeen lebende Alge. Hedwigia 40, 112–115.
Shiozaki, T., Chen, Y. L. L., Lin, Y. H., Taniuchi, Y., Sheu, D. S., Furuya, K., et al. (2014). Seasonal variations of unicellular diazotroph groups A and B, and Trichodesmium in the northern South China Sea and neighboring upstream Kuroshio Current. Cont. Shelf Res. 80, 20–31. doi: 10.1016/j.csr.2014.02.015
Shiozaki, T., Fujiwara, A., Ijichi, M., Harada, N., Nishino, S., Nishi, S., et al. (2018). Diazotroph community structure and the role of nitrogen fixation in the nitrogen cycle in the Chukchi Sea (western Arctic Ocean). Limnol. Oceanogr. 63, 2191–2205. doi: 10.1002/lno.10933
Shiozaki, T., Fujiwara, A., Inomura, K., Hirose, Y., Hashihama, F., and Harada, N. (2020). Biological nitrogen fixation detected under Antarctic sea ice. Nat. Geosci. 13, 729–732. doi: 10.1038/s41561-020-00651-7
Shiozaki, T., Takeda, S., Itoh, S., Liu, X., Hashihama, F., and Furuya, K. (2015). Why is Trichodesmium abundant in the Kuroshio? Biogeosciences 12, 6931–6943. doi: 10.5194/bg-12-6931-2015
Smith, A. D., and Gilbert, J. J. (1995). Spatial and temporal variability in filament length of a toxic cyanobacterium (Anabaena affinis). Freshw. Biol. 33, 1–11. doi: 10.1111/j.1365-2427.1995.tb00381.x
Sohm, J. A., Subramaniam, A., Gunderson, T. E., Carpenter, E. J., and Capone, D. G. (2011). Nitrogen fixation by Trichodesmium spp. and unicellular diazotrophs in the North Pacific Subtropical Gyre. J. Geophys. Res. 116:G03002.
Spurr, A. R. (1969). A low-viscosity epoxy resin embedding medium for electron microscopy. J. Ultrastruct. Res. 26, 31–43. doi: 10.1016/s0022-5320(69)90033-1
Subramaniam, A., Yager, P. L., Carpenter, E. J., Mahaffey, C., Björkman, K., Cooley, S., et al. (2008). Amazon River enhances diazotrophy and carbon sequestration in the tropical North Atlantic Ocean. Proc. Natl. Acad. Sci. U.S.A. 105, 10460–10465. doi: 10.1073/pnas.0710279105
Sundström, B. G. (1984). Observations on Rhizosolenia clevei Ostenfeld (Bacillariophyceae) and Richelia intracellularis Schmidt (Cyanophyceae). Bot. Mar. 27, 345–356.
Taniuchi, Y., Chen, Y. L. L., Chen, H. Y., Tsai, M. L., and Ohki, K. (2012). Isolation and characterization of the unicellular diazotrophic cyanobacterium Group C TW3 from the tropical western Pacific Ocean. Environ. Microbiol. 14, 641–654. doi: 10.1111/j.1462-2920.2011.02606.x
Tuo, S., Chen, Y. L. L., and Chen, H. Y. (2014). Low nitrate availability promotes diatom diazotroph associations in the marginal seas of the western Pacific. Aquat. Microb. Ecol. 73, 135–150. doi: 10.3354/ame01715
Tuo, S., Chen, Y. L. L., Chen, H. Y., and Chen, T. Y. (2017). Free-living heterocystous cyanobacteria in the tropical marginal seas of the western North Pacific. J. Plankton. Res. 39, 404–422. doi: 10.1093/plankt/fbx023
Turk, K. A., Rees, A. P., Zehr, J. P., Pereira, N., Swift, P., Selley, R., et al. (2011). Nitrogen fixation and nitrogenase (nifH) expression in tropical waters of the eastern North Atlantic. ISME J. 5, 1201–1212. doi: 10.1038/ismej.2010.205
Villareal, T. A. (1989). Division cycles in the nitrogen-fixing Rhizosolenia (Bacillariophyceae)-Richelia (Nostocaceae) symbiosis. Eur. J. Phycol. 24, 357–365. doi: 10.1080/00071618900650371
Villareal, T. A. (1990). Laboratory culture and preliminary characterization of the nitrogen-fixing Rhizosolenia-Richelia symbiosis. Mar. Ecol. 11, 117–132. doi: 10.1111/j.1439-0485.1990.tb00233.x
Villareal, T. A. (1994). Widespread occurrence of the Hemiaulus-cyanobacterial symbiosis in the southwest North Atlantic Ocean. Bull. Mar. Sci. 54, 1–7.
Wacklin, P., Hoffmann, L., and Komárek, J. (2009). Nomenclatural validation of the genetically revised cyanobacterial genus Dolichospermum (Ralfs ex Bornet et Flahault) comb. nova. Fottea 9, 59–64. doi: 10.5507/fot.2009.005
Walsby, A. E. (1994). Gas vesicles. Microbiol. Mol. Biol. Rev. 58, 94–144. doi: 10.1128/mmbr.58.1.94-144.1994
West, W., and West, G. S. (1897). Welwitsch’s African freshwater algae. J. Bot. Br. For. 35, 235–243.
White, A. E., Prahl, F. G., Letelier, R. M., and Popp, B. N. (2007). Summer surface waters in the Gulf of California: prime habitat for biological N2 fixation. Glob. Biogeochem. Cycles 21:GB2017.
Zehr, J., and Turner, P. (2001). “Nitrogen fixation: nitrogenase genes and gene expression,” in Methods in Microbiology, ed. J. H. Paul (St. Petersburg, FL: Academic Press), 271–286. doi: 10.1016/s0580-9517(01)30049-1
Zehr, J. P., Montoya, J. P., Jenkins, B. D., Hewson, I., Mondragon, E., Short, C. M., et al. (2007). Experiments linking nitrogenase gene expression to nitrogen fixation in the North Pacific subtropical gyre. Limnol. Oceanogr. 52, 169–183. doi: 10.4319/lo.2007.52.1.0169
Keywords: Calothrix rhizosoleniae, Richelia intracellularis, diatom diazotroph associations, free-living Richelia and Calothrix, South China Sea, Philippine Sea, gas vesicles
Citation: Tuo S, Mulholland MR, Taniuchi Y, Chen HY, Jane WN, Lin YH and Chen YLL (2021) Trichome Lengths of the Heterocystous N2-Fixing Cyanobacteria in the Tropical Marginal Seas of the Western North Pacific. Front. Mar. Sci. 8:678607. doi: 10.3389/fmars.2021.678607
Received: 10 March 2021; Accepted: 08 June 2021;
Published: 12 July 2021.
Edited by:
Bangqin Huang, Xiamen University, ChinaReviewed by:
Yehui Tan, South China Sea Institute of Oceanology (CAS), ChinaZuozhu Wen, Xiamen University, China
Copyright © 2021 Tuo, Mulholland, Taniuchi, Chen, Jane, Lin and Chen. This is an open-access article distributed under the terms of the Creative Commons Attribution License (CC BY). The use, distribution or reproduction in other forums is permitted, provided the original author(s) and the copyright owner(s) are credited and that the original publication in this journal is cited, in accordance with accepted academic practice. No use, distribution or reproduction is permitted which does not comply with these terms.
*Correspondence: Sing-how Tuo, singhow.tuo@gmail.com