Evaluation of the Anti-fouling Efficacy of Bacillus licheniformis Extracts Under Environmental and Natural Conditions
- 1Departamento de Microbioloxía e Parasitoloxía, Facultade de Bioloxía-CIBUS, Universidade de Santiago de Compostela, Santiago de Compostela, Spain
- 2AquaBioTech Group, Mosta, Malta
- 3School of Zoology, George S. Wise Faculty of Life Sciences, Tel Aviv University, Tel Aviv, Israel
There is an increasing interest in developing innovative coatings and testing natural products with anti-fouling activity to substitute current highly toxic biocides that have a harmful impact on marine organisms. Bacillus licheniformis species have shown different anti-biofilm and anti-fouling activities in vitro, but so far, its efficacy in field trials has not been tested. For this purpose, the capacity of different extracts of B. licheniformis NCTC 10341T to prevent micro and macro-fouling was first tested in vitro. The methanol cell extract (MCE) inhibited bacterial biofilm formation without significantly affecting planktonic growth and displayed a significant efficacy to prevent larval settlement of the macro-fouler Bugula neritina in vitro without inducing lethality. Additionally, the MCE presented low toxicity against the non-target species Artemia salina. The B. licheniformis MCE was then incorporated in a self-polishing paint at 2 and 5% w/w and tested in a static immersion experiment in the Gulf of Aqaba (northern Red Sea) for 180 days. Fouling coverage decreased by 30% in the 5% MCE-treated panels in comparison with the control panels. Differences in the anti-biofilm activity of the extracts depending on the culture medium highlight the importance of the strict control of culture conditions for the production of biomass with stable bioactive activity. The results indicate the potential of B. licheniformis NCTC 10341T crude extracts for environmentally friendly anti-fouling applications, although a deeper characterization of the bioactive compounds present in the B. licheniformis MCE and its mode of action is required to allow strict control of the activity of the extracts to achieve large-scale industrial production.
Introduction
Biofouling is a complex process in which aquatic microorganisms (bacteria, diatoms, microalgae, and fungi) as well as soft (macroalgae) and hard (mussel and cyprids) macro-organisms and their products adhere and encrust a submerged surface. This natural process can be divided into two steps: (1) the micro-fouling process, in which the microorganisms attach and form a biofilm; and (2) the macro-fouling process, in which the macro-organisms adhere to the initial microbial biofilm. In this process, the initial colonizers are required to produce specific physical and chemical cues in order to attract the macro-foulers (Tait et al., 2005; Tait and Havenhand, 2013), although in some cases the biofilm may exert an inhibitory effect (Dobretsov and Rittschof, 2020) and some macrofouling organisms can settle without any interaction with microbial biofilms (Freire et al., 2019).
Biofilm formation and biofouling accumulation cause a high negative impact on the marine industry and in water purification systems, constituting a global economic problem and a health hazard issue (Yebra et al., 2004). Microfouling is also involved in microbially-induced corrosion (MIC), which causes an enormous economic impact in different industrial sectors (Jia et al., 2019). Anti-fouling coatings traditionally incorporated different highly toxic biocides such as tributyltin (TBT), which was subsequently banned because of its adverse environmental effects on the aquatic ecosystems resulting from its endocrine disruption properties and its accumulation in the sediments (Gibbs and Bryan, 2009). Nevertheless, highly toxic biocides are still being used in anti-fouling applications, despite their impact on marine organisms. For example, copper exposure induces interferences with fishes’ chemoreception and olfaction (Ten Hallers-Tjabbes and Walmsley, 2010), and 4,5-dichloro-2-n-octyl-4-isothiazolin-3-one, also known as DCOIT, displays endocrine-disrupting and reproductive-damaging effects at environmentally worst-case concentrations (Chen and Lam, 2017). Anti-fouling paint formulations are often composed of inorganic biocides (typically cuprous oxide) and one or more organic or organo-metallic co-biocides, which boost the biocidal efficacy of the paint (Hellio and Yebra, 2009), but these compounds may also pose important risks to aquatic ecosystems (Martins et al., 2017). Therefore, efforts have been made to control biofouling by developing alternative environmentally friendly strategies that could either substitute or boost the activity of currently used, toxic biocides. Different strategies are being explored, including the use of non-bionic anti-fouling technologies (Tian et al., 2021) and the application of bioinspired strategies, such as bioinspired polymeric anti-fouling coatings, and biomimetic surface microtopographies (Chen et al., 2021; Tian et al., 2021). The simultaneous use of multiple anti-fouling approaches that act synergistically has been proposed in order to reduce the toxicity and increase the effectiveness of the anti-fouling coatings (Xie et al., 2020).
Due to the need for eco-friendly compounds and strategies to substitute the traditional highly toxic anti-fouling biocides, current efforts are also focused on the development of innovative coatings and the examination of the effectiveness of novel natural products with anti-fouling activity (e.g., Maréchal and Hellio, 2009; Nir and Reches, 2016; Chen and Qian, 2017; Chen et al., 2021). Polymers and nanoparticles (Kavouras et al., 2019; Gutner-Hoch et al., 2018; Gu et al., 2020), but also enzymes and peptides have been applied for anti-fouling purposes at laboratory scale. However, their high costs and problematic production prevented large-scale testing and application (Ma et al., 2018; Chen et al., 2021). The incorporation of natural functional compounds into the matrix of the coatings has also been explored, although only a few of them have been tested under natural conditions. Promising activities have been found in different marine macroalgae such as Delisea pulchra (Dworjanyn et al., 2006), Dictyota menstrualis (Schmitt et al., 1998), and Fucus vesiculosus (Brock et al., 2007). However, the limited supply of bioactive compounds from marine macro-organisms and variations in the bioactivity in the harvested biomass constitute severe obstacles for their application at a large scale.
Natural anti-fouling compounds of bacterial origin present important advantages compared to those obtained from higher organisms and have been proposed as one of the best possible alternative approaches to replace current biocides in paints (Dobretsov et al., 2013b; Wang et al., 2017; Adnan et al., 2018; Liu et al., 2020). In fact, half of the anti-fouling compounds described in the last 5 years were isolated from marine-derived microorganisms (Liu et al., 2020). Microorganisms can be easily produced at a low cost and the production of bioactive compounds can be easily modulated through the control of cultivation parameters (Rajarajan et al., 2013). An excellent example of an anti-fouling compound of bacterial origin is butenolide, derived from a marine Streptomyces strain, which shows effective inhibition against major fouling species, short half-life, and low toxicity against non-target organisms (Chen et al., 2017; Liu et al., 2020). Although the identification of the active molecules is compulsory for the industrial application of the anti-fouling compounds, the use of microbial extracts with anti-fouling activity may represent an interesting alternative to the use of purified compounds, presenting a lower production cost and the possibility of having different bioactive compounds in the same extract that could act synergically on different targets. Microbial extracts with anti-fouling activity have been identified (Armstrong et al., 2000; Peppiatt et al., 2000) and even tested successfully under field conditions (Kharchenko et al., 2020). Live cells or spores have been also successfully incorporated into anti-fouling coatings (Gatenholm et al., 1995; Eduok et al., 2015). Nevertheless, in most cases, the microbial strains and extracts are selected based on antimicrobial or biocide activity (Armstrong et al., 2000; Peppiatt et al., 2000; Gao et al., 2014; Kharchenko et al., 2020), and few candidates are available that have been selected based on specific anti-biofilm or anti-fouling activity, presenting a low toxicity profile.
Several species of the genus Bacillus, a group of Gram-positive, spore-forming rods belonging to the phylum Firmicutes, have been reported as an important source of anti-fouling compounds (Peppiatt et al., 2000; Ortega-Morales et al., 2008), including biosurfactants (Rivardo et al., 2009; Alemán-Vega et al., 2020), bacteriocins (Palanichamy and Subramanian, 2017), enzymes (Shah et al., 2010), and fatty acids with anti-diatom adhesion activity (Xin et al., 2016). Among these, Bacillus licheniformis, a species with multiple biological activities which has already been included in commercial products for human health, veterinary applications, and aquaculture (Muras et al., 2021b), has been proposed as a good candidate for anti-fouling applications due to the presence of multiple biochemical activities. B. licheniformis marine strain EI-34-6 produces an extracellular nuclease, NucB, that exhibits high anti-biofilm, as well as dispersing activities since it can digest extracellular DNA, which features a broad functionality in the structural integrity of biofilms (Nijland et al., 2010). The use of this enzyme to prevent and disrupt biofilm development has been patented (Burgess et al., 2017). Similarly, a lipopeptide produced by B. licheniformis strain V9T14 inhibits biofilm formation by the human pathogens Escherichia coli and Staphylococcus aureus (Rivardo et al., 2009). Additionally, an exopolysaccharide obtained from a B. licheniformis strain isolated from the marine sponge Spongia officinalis and a chitinase from a strain isolated from spoilt vegetables were found to reduce biofilm development in a wide range of bacteria (Sayem et al., 2011; Muslim et al., 2016). An extracellular protein from the tropical marine B. licheniformis strain D1 not only diminished biofilm formation but also dispersed pre-formed biofilms of the yeast Candida albicans, and the bacteria Pseudomonas aeruginosa and Bacillus pumilus (Dusane et al., 2013). Also, the production of lichenysin, a biosurfactant with notable anti-adhesion activity against the pathogenic strains S. aureus and C. albicans, was described for B. licheniformis strain AL1.1 (Coronel-León et al., 2016). Regarding anti-fouling activity, a paint including extracts of culture supernatants of B. licheniformis MAI-11-01-CP inhibited algal attachment and reduced surface coverage by algal spores (Burgess et al., 2003). Moreover, spores of B. licheniformis have been included in a coating to inhibit marine microbial-induced corrosion, based on the production of extracellular polymeric antibiotic substances such as γ-polyglutamate (γ-PGM) (Eduok et al., 2015). Beyond anti-fouling activity, B. licheniformis can grow in different types of industrial wastes due to its high production of numerous enzymes (Vasconcellos et al., 2009; Mao et al., 2013), having the potential to contribute to the zero-emission concept. In conclusion, B. licheniformis strains exhibit a plethora of biological activities and a great potential for large-scale production of bioactive compounds and native or recombinant enzymes with promising biotechnological applications (Muras et al., 2021b), nevertheless, these capacities have been tested so far only in vitro, and the anti-fouling activity under the much more complex natural conditions remains unknown.
The presence of multiple antibiofilm and anti-fouling compounds with complementary activities in B. licheniformis strains and the increasing interest in the search for new eco-friendly anti-fouling compounds intrigued us to test the capacity of extracts of the type strain B. licheniformis NCTC 10341T to prevent fouling under natural conditions. The strain was selected in a preliminary screening experiment in which the anti-biofilm activity of several compounds and bacterial strains against marine bacteria was tested (Muras et al., 2021a). The use of extracts was preferred due to lower production cost, and to take profit of the probable presence of multiple active compounds in the same extract. In a preliminary phase, and in order to select the most suitable extract for field trials, the anti-biofilm activity of different B. licheniformis extracts was assessed using different marine bacteria selected as models for marine biofilm formation and measured using the real-time equipment xCELLigence® System RTCA DP (Agilent Technologies Inc., Santa Clara, CA, United States) (Muras et al., 2021a). Furthermore, the anti-macrofouling efficacy against model organisms, including the bryozoan Bugula neritina and the barnacle Amphibalanus amphitrite, was examined. The toxicity of the bacterial extract was tested against nauplii of the brine shrimp Artemia salina, a non-target organism. The B. licheniformis NCTC 10341T methanol cell extract (MCE), presenting the highest in vitro activity, was then incorporated in a solvent-based self-polishing standard test product paint at 2 and 5% w/w and tested in the field (Eilat, Northern Red Sea) for 180 days. The results indicate the feasibility of using B. licheniformis NCTC 10341T methanol extract as a booster biocide for environmentally friendly anti-fouling applications.
Materials and Methods
Bacterial Strains and Growth Conditions
Bacillus licheniformis NCTC 10341T obtained from the Spanish Collection of Type Cultures (CECT20T) was cultured in Luria-Bertani (LB) medium at 37°C. The biofilm-forming marine strains Vibrio aestuarianus CECT625, Vibrio tubiashii CECT631, Pseudoalteromonas flavipulchra and Pseudoalteromonas maricaloris were routinely cultured at 22°C on Marine Agar/Broth (MA/MB, Pronadisa, Laboratorios Conda S.A., Madrid, Spain) and/or Tryptone Soya Agar/Broth (TSA/TSB; Panreac Química SLU, Barcelona, Spain).
The acyl-homoserine lactones (AHL) biosensor strains Chromobacterium subtsugae CV026 (formerly C. violaceum CV026, McClean et al., 1997; Harrison and Soby, 2020) and VIR07 (Morohoshi et al., 2008) were used to detect quorum quenching (QQ) activity in solid-plate assays as explained below. These strains were routinely cultured on LB medium supplemented with kanamycin (50 μg/ml) at 30°C.
Cell Extract Preparation
Two biomass extracts (aqueous and methanol cell extracts) and one from the supernatant of cultures after cell removal (ethyl acetate extract), were obtained from B. licheniformis NCTC10341T in order to select the best candidate for field tests. Aqueous cell extract (ACE) was obtained from the biomass of a 15 ml shaken culture in LB, which was centrifuged for 5 min at 2000 × g. The pellet was washed with 15 ml of phosphate-buffered saline (PBS) pH 6.5, resuspended in 1.5 ml of the same buffer, sonicated for 5 min on ice, and centrifuged at 16000 × g for 30 min at 4°C. The ACE obtained in this way was filtered through a 0.22 μm and stored at 4°C. The MCE was obtained in the same way, but the biomass was resuspended in methanol. After clarification by centrifugation, the solvent was evaporated to dryness in a rotary evaporator system at 40°C and resuspended in 1.5 ml PBS pH 6.5. Cell-free spent culture medium (CFSM) was extracted twice with ethyl acetate (1:1), the solvent was evaporated to dryness in a rotary evaporator system at 40°C and resuspended in 1.5 ml PBS pH 6.5. These different extracts were prepared with samples taken at different cultivation times (24 h, 72 h, 5 days, and 7 days). Stationary phase was achieved by days 5–7 in LB (Supplementary Figure 2).
Further experiments were performed with extracts obtained from 1 L of LB culture medium inoculated with 1 ml of an overnight culture of B. licheniformis and incubated at 37°C with shaking for 7 days. Biomass was concentrated by centrifugation to prepare the aqueous and methanol cell extracts, as explained above. Extracts were freeze-dried to obtain the dry weight. In order to further characterize the activity present in the extracts, an aliquot of the MCE was evaporated, resuspended in PBS buffer, and extracted twice with dichloromethane (MCE-DM). An aliquot of the ACE was extracted twice with ethyl acetate (ACE-EA). Both extracts were evaporated and resuspended in PBS buffer.
Biofilm Quantification Using the xCELLigence® System
Tryptone Soya Broth (TSB), 24-h cultures of the marine biofilm-forming bacteria V. aestuarianus CECT625, V. tubiashii CECT631, P. flavipulchra, and P. maricaloris were adjusted to 0.05 OD600 with fresh TSB. Then, 180 μl of each culture were added into E-Plates 16 (Agilent Technologies Inc., Santa Clara, CA, United States) and supplemented with 20 μl of B. licheniformis extracts. The system was incubated at 22°C for 44 h. As controls, the un-inoculated TSB medium and the culture of biosensor biofilm-forming bacteria with 20 μl of PBS were used (Muras et al., 2021a). Experiments were performed in triplicate.
Simultaneously, the effect of extracts on planktonic growth was investigated by measuring bacterial growth measured at 600 nm. 24-h TSB cultures were adjusted to 0.05 OD600 as explained above, mixed well, and added into a 96-well microtiter plate. A volume of 20 μl of the anti-fouling extract was added into 180 μl of the bacterial inoculum, while 20 μl of PBS were added in the control wells. The microtiter plate was cultured at 22°C for 44 h. After the incubation period, the bacterial growth was estimated by measuring the optical density (600 nm) after mixing with a pipette. Experiments were performed in triplicate.
AHL-Degradation Assay
Five hundred microliters of 24 h B. licheniformis NCTC 10341T cultures in LB were centrifuged, and pellets were washed with PBS pH 6.5 and resuspended in another 500 μl of the same buffer. These cell suspensions were used for live-cell AHL degradation assay by adding either N-hexanoyl-L-homoserine lactone (C6-HSL) or N-decanoyl-L-homoserine lactone (C10-HSL) (Sigma-Aldrich, final concentration 10 μM) and incubating for 24 h at 22°C. In order to reveal the integrity of the AHL after incubation, 100 μl of the supernatant obtained after centrifugation of the degradation mixture were placed in wells made on an LB plate overlaid with a 1/100 dilution of an overnight culture of C. violaceum CV026 or C. violaceum VIR07 in soft LB (0.8% agar) for the detection of C6-HSL and C10-HSL, respectively. The plates were incubated for 24 h at 30°C. The degradation of AHLs is revealed by the absence of the halo of the purple pigment violacein around the wells (McClean et al., 1997). PBS pH 6.5 plus the corresponding AHL (10 μM) was used as control (Muras et al., 2018).
Effect of Culture Medium on Microfouling Activity
To determine if the anti-biofilm activity of B. licheniformis NCTC 10341T or its productivity could be improved according to the medium composition, flasks with 250 ml of four different culture media were inoculated with an overnight culture of B. licheniformis and grown for 7 days at 37°C with shaking. The composition of the culture media is shown in Supplementary Table 1. LB culture medium was used as a reference. Two of the culture media were constituted by adding more tryptone (medium 1) or Na-glutamate (medium 3) as carbon and nitrogen sources, and the other two included either glucose or sucrose as carbon source and ammonia as nitrogen source (media 2 and 4, Supplementary Table 1). The daily growth was monitored by optical density (OD 600 nm), the productivity, measured as lyophilized biomass, and their anti-biofilm activity as MCE against P. maricaloris were compared.
Bugula neritina Settlement Inhibition Test
Larvae of the bryozoan B. neritina were used for anti-fouling efficacy assays following Gutner-Hoch et al. (2018). B. neritina colonies were collected from ropes and boats mooring from Gzira’s shores, Malta (35°54′N 14°30′E) from November 2015 to June 2016 and transported to the laboratory in seawater containers. Animals were gently cleaned to remove algae, debris, and other organisms and kept in 0.45 μm filtered seawater (FSW) with gentle aeration in the dark at ambient temperature (15–22°C) for a minimum of 12 h. The larval release was induced by exposing the adults to a strong light (>6000 lux) for 1 to 2 h. Then the larvae were attracted to a localized area by lighting a corner of the tank with a flashlight and subsequently collected with a Pasteur pipette. The collected larvae were immediately used in bioassays.
A series of five concentrations of B. licheniformis MCE and copper pyrithione (CuPT, CAS: 154592-20-8) were prepared with 0.45 μm FSW (from 17.8 to 90 mg/l). A control group was prepared with FSW. 24-well tissue culture plates were filled with test solutions, and 10 larvae were added to each well (four replicate wells for each treatment group, N = 40) and incubated at 20°C in an LMSTM 80 incubator. Settlement and mortalities were recorded after 24 and 48 h using an Optika Stereomicroscope (SZP-10).
Amphibalanus amphitrite Settlement Inhibition Test
Amphibalanus amphitrite cyprids were used to assess the anti-fouling efficacy of the MCE against encrusting calcareous macro-foulers. Adult barnacles were collected from Gzira shores, Malta Central Mediterranean Sea (35°54′N 14°30′E), in the intertidal zone in February 2015. In the laboratory, they were glued to fiberglass plates with epoxy coral glue cement (D-D Aquascape) and maintained with moderate to strong aeration in ∼ 10 L aquaria filled with 0.45 μm FSW (20–25°C, pH ∼ 8.2 and salinity of 35 ± 2‰) and with a light intensity around 3000 lux. The barnacles were daily fed with 2–5 ml of concentrated A. salina nauplii and the unicellular algae Tetraselmis chuii, and Thalassiosira pseudonana (2 × 105 cells/ml). A 50% water exchange was performed twice a week.
In order to release nauplii, a dozen barnacles were exposed to bright light for 1 h. The released nauplii were attracted to a localized light source such as a flashlight, collected with a Pasteur pipette and transferred to a 1 L beaker filled with 0.45 μm FSW with 20 mg/L penicillin (CAS: 69578) and 30 mg/L streptomycin (CAS: 3810740) (Rittschof et al., 1992) as well as 25 ml of 2 × 105 of T. chuii and T. pseudonana cultures. The nauplii were kept at 25 ± 1°C with gentle aeration and a 12 h/12 h photoperiod at 3000 lux without food renewal. After 4–5 days, nauplii developed into cyprids and were collected manually with a Pasteur pipette or by filtration through a tier of 300, 230, and 169 μm plankton nettings. The nauplii were retained in the 300 μm netting, while the cyprids were retained on the 230 and 169 μm nettings.
The cyprids were placed in autoclaved FSW and kept at 8 ± 2°C for 3–6 days following (Rittschof et al., 1992). A stock solution of 1000 mg/L of the B. licheniformis MCE was made with FSW and sonicated for 15 min (Ultrasonic batch Wiselclean®). The stock solution was diluted 9 times by a factor of 1.2 down to 190 mg/L. Two sets of control groups and serial dilutions of CuPT with FSW were prepared. 12-well tissue culture plates were filled with test solutions, and 10 cyprid larvae were added to each well (four replicate wells for each treatment group, N = 40). The plates were incubated at 28°C for 48 h in an LMSTM 80 incubator. A gentle current was induced in each well by pumping and releasing seawater with a 0.2 ml Pasteur pipette to determine the number of unattached or settled cyprids using an Optika Stereomicroscope (SZP-10) after 24 and 48 h.
Artemia salina Toxicity Tests
The toxicity tests were carried out on the non-target species A. salina nauplii (II-III stage). Half a teaspoon of dried cysts (Varicon Aqua Solutions Ltd.) was introduced into a beaker containing 125 ml of 0.45 μm FSW (35 ± 2‰). Following 24–30 h of incubation at 25°C (LMSTM 80 incubator) under vigorous aeration and continuous illumination, 10 hatched nauplii were collected with a Pasteur Pipette and transferred to each well of 24-well plates (three replicate wells for each treatment group, N = 30) filled with test solutions (B. licheniformis MCE and CuPT) and incubated at 25°C for 24 h (LMSTM 80 incubator). Nauplii were observed under a stereoscopic microscope after 24 h, and the mortality was defined as lack of motility for 10 s. A control group was prepared with FSW. The median lethal concentration (LC50) was calculated using mortality rates after 24 h of exposure of the Artemia nauplii.
Static Immersion Field Test
The field test was conducted as a static immersion experiment in a reef area across from the Interuniversity Institute for Marine Sciences in Eilat (IUI), Gulf of Aqaba, northern Red Sea, Israel (29.501451° N, 34.916285° E) from June to December 2016, for a total of 180 days.
To test its anti-fouling capabilities, the B. licheniformis NCTC 10341T MCE was mixed at 2 and 5% w/w into tested standard self-polishing coating formulation (Test product), provided by JOTUN S.A.,1 containing no biocides. These paint-formulations were applied on 7 × 10 cm PVC plates, using a paint applicator with a 400 μm gap size. Coating formulations containing no biocide were used as a negative control and two sets of positive controls were prepared: zinc pyrithione (ZnPT, Zinc OmadineTM, Lonza Ltd. CAS: 13463-41-7) and SEA-NINETM 211N (Dichlorooctylisothiazolinone - DCOIT at 30% with xylene, CAS: 64359-81-5, DOW Inc.) incorporated at 2%.
The field-trial methodology has been adapted considering the standard guidelines in term of panel sizes, data analysis and immersion length (ASTM D3623-78a, 2020 and ASTM D6990-20, 2020). In order to install the experimental panels, two rectangular stainless-steel frames were deployed, and two PVC boards were attached by four steel bars at their corners, leaving a space of 0.2 m in between to form a box-like structure (1.5 × 1.5 × 0.2 m), with a board-faces attached on each one. Before deployment, both the steel and PVC components were coated with commercial anti-fouling paint. The PVC provided surfaces onto which the test panels were attached. The installations were secured by ropes attached to 90 Kg sinkers at a depth of 8–9 m and a marker buoy above (4–5 m below the seawater surface). The PVC test panels were individually labeled, and each attached to the boards by two stainless steel bolts. Monthly monitoring of the fouling on the panels was carried out by underwater photography using a Canon PowerShot D30 waterproof camera. Few hard-fouling organisms settled during the trial on all panels, including on the control group and therefore they were not identified since they were not considered representative. Seawater temperature was obtained from the meteorological data collected by the Israeli National Monitoring Program at the Gulf of Aqaba found at http://www.meteo-tech.co.il/eilat-yam/eilat_en.asp. The image processing program ImageJ (V.1.50) (Rasband, 1997-2018) was used to determine the percentage of fouling on each panel.
Data Analysis
The median efficacy concentration EC50 (with 95% confidence interval) for settlement inhibition of barnacle cyprids and bryozoan larvae and the median lethal concentration LC50 (with 95% confidence interval) of Artemia nauplii were assessed by applying the statistically best-fitted dose-response model using the drc package (Ritz et al., 2015) of the open software R (R Core Team, 2017). E/LC50 were estimated after correction in the controls following Abbott’s formula (Finney, 1971) using dose responses curves through non-regression analysis, and the models displaying the lowest Akaike Information Criterion (AIC) were selected and fitted. Settlement inhibition EC50 and LC50 toxicity values were compared with a state-of-the-art copper-based biofilm inhibitor. Before analysis, the different data sets were checked for normality and equality of variance using the Shapiro-Wilk and the Levene test, respectively. Whenever both assumptions were met, data were analyzed by one-way ANOVA followed by Tukey HSD post hoc test. Data were analyzed by the non-parametric Kruskal-Wallis test followed by the Dunn’s test whenever either of the assumptions was not met. Differences were considered significant at p < 0.05.
A linear mixed-effects model with nested random effects (month of exposure) and Bonferroni corrections, followed by a Tukey post hoc analysis, was used to assess differences between the different treatment percentages fouling cover in the field test. All statistical analysis were performed using the software R (R Core Team, 2017).
Results
Biofilm Inhibition by B. licheniformis Extracts
Two Vibrio strains (V. aestuarianus and V. tubiashii) and two Pseudoalteromonas strains (P. flavipulchra and P. maricaloris) were selected to test the biofilm inhibitory activity of different B. licheniformis extracts. These marine strains were chosen based on their ability to form robust biofilms as detected by the xCELLigence real-time biofilm monitoring system, presenting different sensitivity to quorum sensing (QS) inhibitors and being able to form biofilm in a wide range of salinities (Muras et al., 2021a).
Three types of extracts were prepared using B. licheniformis samples taken at different times in the bacterial growth curve (days 1, 3, 5, and 7): the ACE, the MCE, and the CFSM. Important biofilm inhibitory activity was found, mainly in the MCE. In general, the biofilm inhibitory activity increased with cultivation time, with the highest inhibition being recorded for stationary-phase extracts (days 5–7) in most cases (Supplementary Figure 1). In this preliminary experiment, important biofilm inhibitory activity was found in the 7-day MCE against P. maricaloris (83.62 ± 23.59%), P. flavipulchra (26.38 ± 5.25%), and V. tubiashii (60.86 ± 26.17%). A strong inhibitory activity against P. maricaloris biofilm formation was also observed in the ACE (71.25 ± 14.83%), but no activity was found in the CFSM from B. licheniformis for any of the indicator strains (Supplementary Figure 1).
Based on the positive results obtained for the MCE of B. licheniformis against these marine micro-foulers, the activity of an MCE obtained from a large volume (1 liter) 7-days culture was evaluated at two concentrations: 0.35 and 3.5 mg/ml (Figure 1A). The highest inhibitory effect of the MCE was observed on P. flavipulchra (74.04 ± 22.24%) and P. maricaloris (86.27 ± 0.71%) biofilms at the highest concentration tested (3.5 mg/ml). The high sensitivity of P. maricaloris biofilm observed in the preliminary experiments (Supplementary Figure 1) was confirmed since the lower MCE concentration (0.35 mg/ml) also inhibited biofilm formation in this strain after 24 h and 48 h (55.04 ± 8.42% and 28.16 ± 5.40% inhibition, respectively) (Figure 1A). On the contrary, only a weak reduction of biofilm was observed for V. aestuarianus (21.51 ± 4.12%) and V. tubiashii (18.96 ± 6.06%) 24-h biofilms. Measuring the effect of the B. licheniformis MCE on planktonic growth revealed that the high biofilm inhibition observed for P. maricaloris was accompanied by a strong growth inhibition in the planktonic cells (Figure 1B). On the contrary, the strong biofilm inhibition observed for P. flavipulchra at a concentration of 3.5 mg/ml was not reflected in a proportional inhibition in planktonic growth, indicating that a specific anti-biofilm activity against this species is present in the MCE of B. licheniformis.
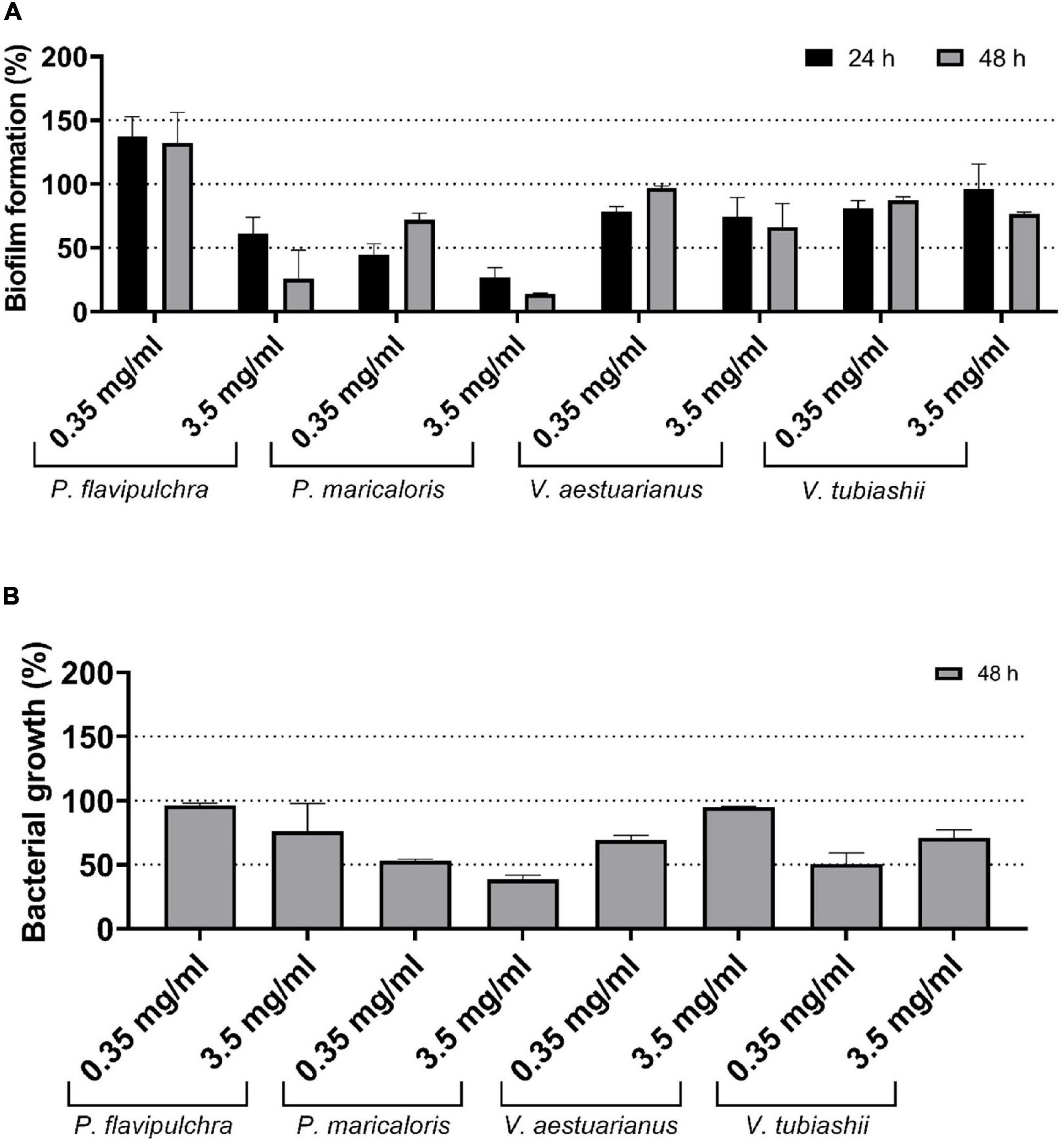
Figure 1. Effect of the methanol cell extract (MCE) of Bacillus licheniformis NCTC 10341T at final concentration of 0.35 and 3.5 mg/ml on biofilm formation (A) and planktonic growth (B) of the biofilm-forming marine bacteria Pseudoalteromonas flavipulchra, Pseudoalteromonas maricaloris, Vibrio aestuarianus, and Vibrio tubiashii. Biofilms were incubated for 24 h (black) and 48 h (gray) and measured with the xCELLigence real-time monitoring system. Planktonic growth was measured as optical density at 600 nm of homogenized cultures after 48 h. Values are shown as percentage in comparison with untreated controls (n = 3).
Preliminary Characterization and Medium Optimization
In order to further characterize the observed anti-biofilm activity, different extracts from a higher volume, 7-days culture of B. licheniformis grown at 37°C in LB were prepared. MCE and ACE were extracted with dichloromethane (MCE-DM) and ethyl acetate (ACE-EA), respectively, and were tested against the biofilm formed by P. maricaloris measured in the xCELLigence system® (Figure 2A). Similar inhibitory activities on P. maricaloris biofilm were observed for MCE-DM (76.79%) and ACE-EA (71.2%). The ethyl-acetate extraction of the aqueous extract significantly increased the anti-biofilm activity (Figure 2A). None of these extracts inhibited the planktonic growth of P. maricaloris (Figure 2B). Interestingly, the planktonic growth inhibitory activity observed for the MCE (15.18%) disappeared in the MCE-DM extract (Figure 2B) while presenting even higher biofilm inhibitory activity (Figure 2A).
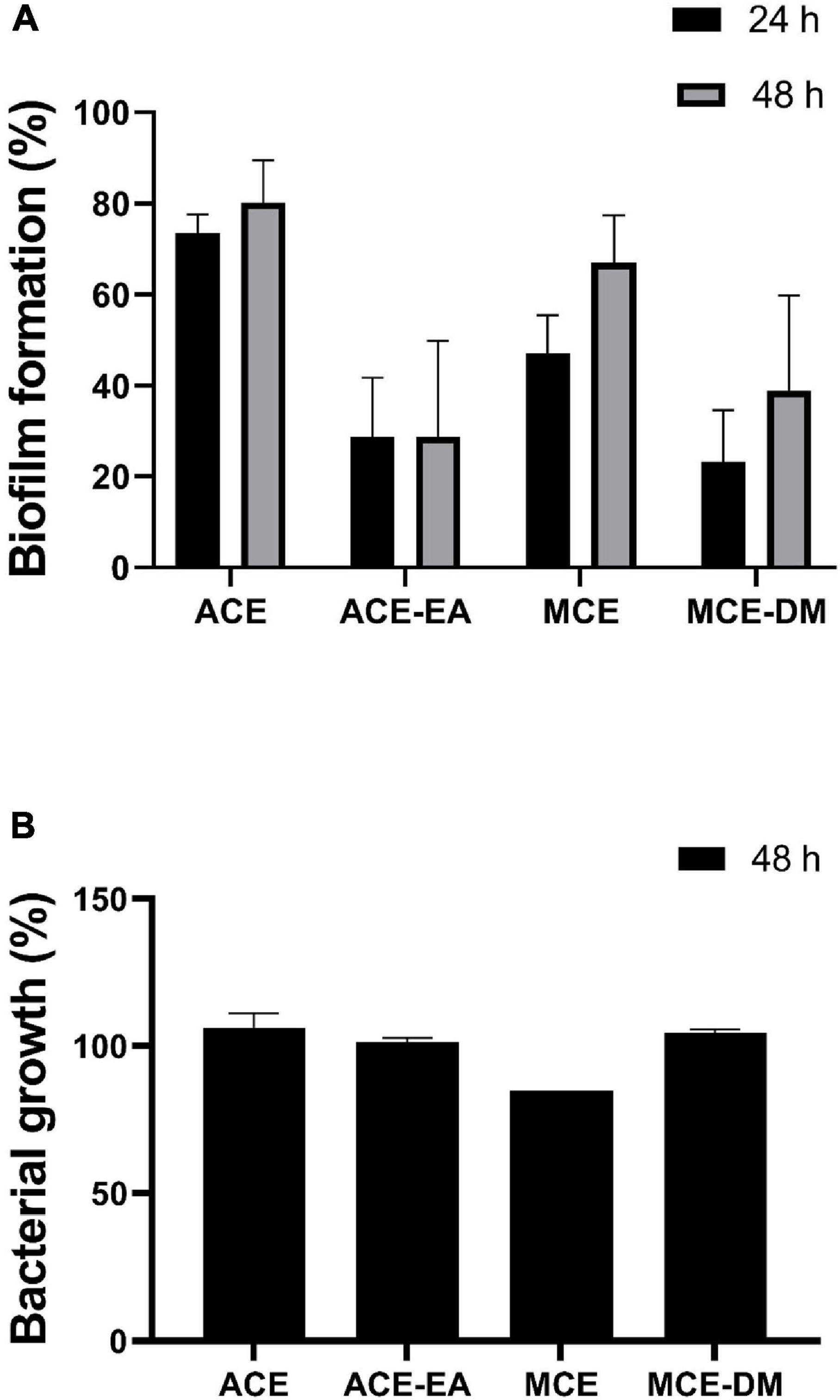
Figure 2. Effect of different cell extracts of 7-day cultures of Bacillus licheniformis NCTC 10341T on biofilm formation (A) and planktonic growth (B) of Pseudoalteromonas maricaloris. The effect of methanol cell extract (MCE), aqueous cell extract (ACE), and MCE extracted with dichloromethane (MCE-DM), and ACE extracted with ethyl acetate (ACE-EA) on biofilm formation was evaluated after 24 and 48 h with the xCELLigence real-time monitoring system. Planktonic growth was measured as optical density at 600 nm of homogenized cultures after 48 h. All extracts were assayed at 0.35 mg/ml. Values are shown as a percentage in comparison with untreated controls (n = 3).
Since the capacity to degrade QS signals, a process known as QQ, is a common activity among Bacillus spp. (Dong et al., 2002), and in order to ascertain if this activity could be responsible for the observed biofilm inhibitory activity, the capacity of live cells to inactivate the QS signals C6 and C10-HSL using the engineered C. violaceum-based bioassay was evaluated. However, neither C6 nor C10-HSL activity was quenched by B. licheniformis live cells (data not shown).
Four semi-defined culture media were prepared, containing an extra source of amino acids (media 1 and 3) or sugars and ammonium (media 2 and 4) as carbon and nitrogen sources (Supplementary Table 1), to evaluate the effect of culture medium on the observed anti-microfouling activity, and LB culture medium was used as a reference. The optical density data (OD600 nm) showed that LB culture produced the highest growth in all cases (Supplementary Figure 2). Differences in biomass yield among the culture media were lower when the dry-weight of the biomass was measured after 7 days, with values ranging between 1.71 and 2.03 mg/ml (Supplementary Figure 3). The LB culture medium also produced the biomass with the highest biofilm inhibitory activity against the biofilm formed by P. maricaloris, reaching an inhibition of 44.15–57.95% for a concentration of 0.35 mg/ml of MCE and 70.82–85.11% for a concentration of 3.5 mg/ml after 24 and 48 h, respectively (Supplementary Figure 4).
Anti-macrofouling and Toxicity Properties of the MCE of B. licheniformis
Following the determination of successful biofilm inhibitory properties, the MCE was tested for anti-macro-fouling activity against two model target species: the soft bryozoan B. neritina, and the encrusting barnacle A. amphitrite. The MCE extract displayed significant efficacy against B. neritina larval settlement with an EC 50 of 37.39 mg/L (95% CI = 28.43–46.35 mg/L) (Figure 3A). Contrary to state-of-the-art anti-fouling biocides, the MCE efficacy did not seem to solely rely on toxicity mechanisms as the mortality rate was lower than the settlement inhibition rate (Figure 3B). After 48 h of exposure, the larvae that did not settle were either alive and still swimming or dead. The LC50 was calculated at 49.22 mg/L (95% CI = 37.53–60.90 mg/L). Since an unusually high proportion of bryozoan larvae still swimming was observed in the cultures treated with the B. licheniformis MCE more than 24 h after larval release, the proportion of larvae still swimming after 24 h of exposure was calculated and compared in the different treatment groups, including the control group (Figure 4). Statistical analysis revealed a significant difference in the percentage of swimming larvae at 60 mg/L in comparison to the control (Kruskal-Wallis, p = 0.045, Dunn’s post hoc test, p = 0.027), indicating the MCE extract was able to prevent B. neritina larval settlement in a dose-dependent manner without inducing lethality.
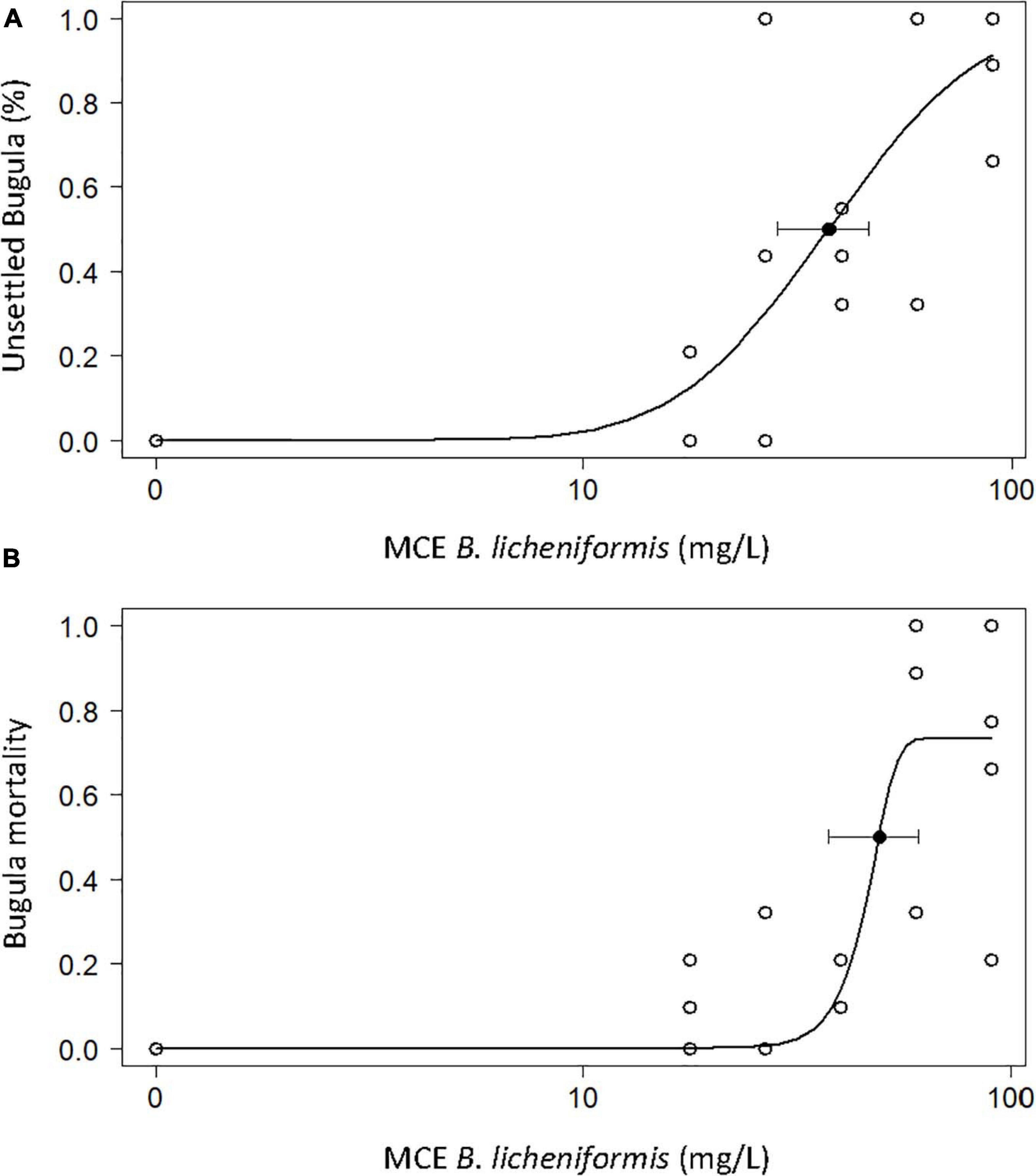
Figure 3. Percentage of larvae of the bryozoan Bugula neritina larvae not settled (A) and dead (B) after exposure to the methanol cell extract (MCE) of B. licheniformis for 48 h (n = 4 × 10). E/LC50 represented by black filled dots. EC50 = 37.39 mg/L (95% CI = 28.43–46.35 mg/L). LC50 = 49.22 mg/L (95% CI = 37.53–60.90 mg/L).
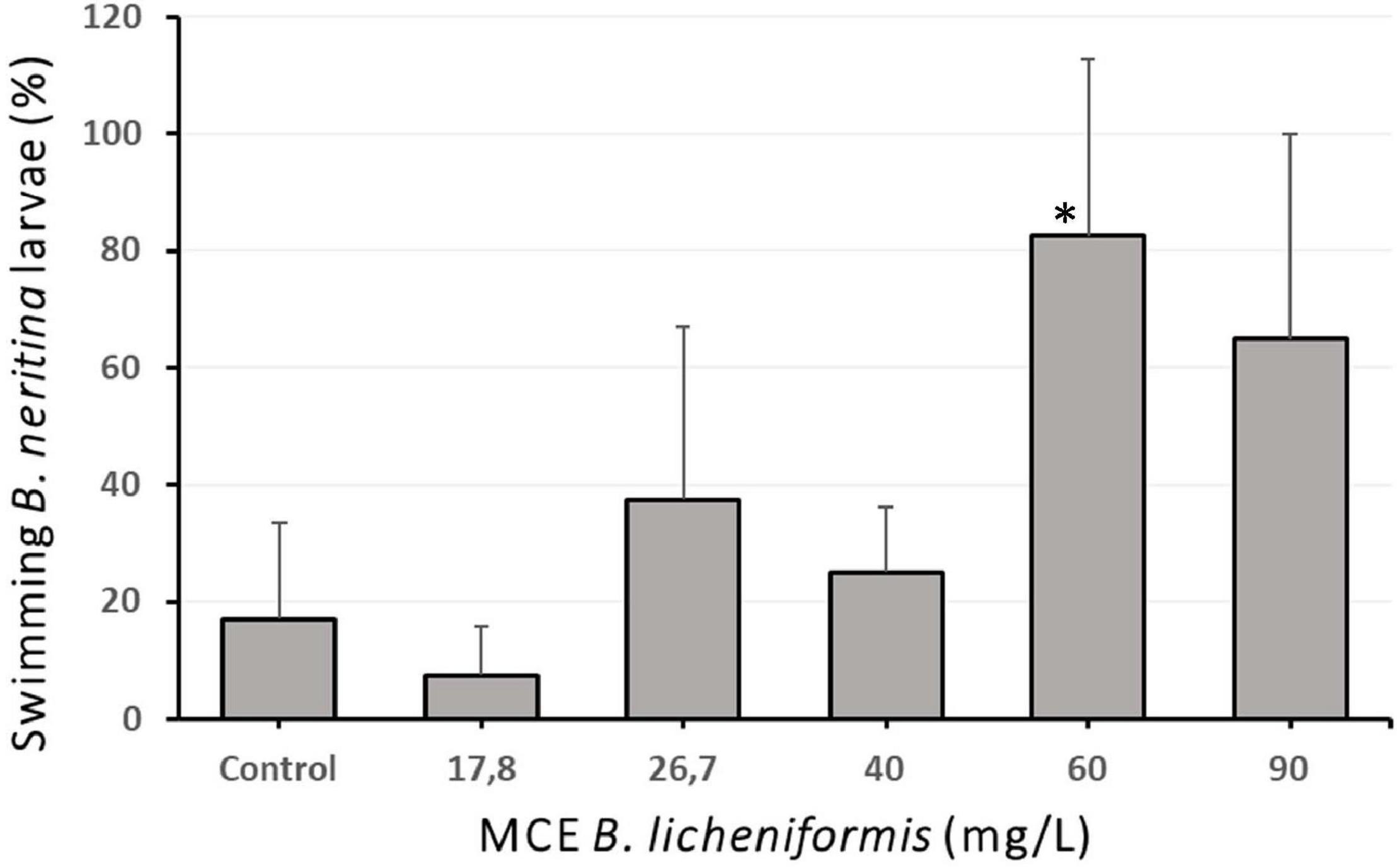
Figure 4. Percentage of swimming and settled larvae of the bryozoan Bugula neritina 24 h after exposure to the methanol cell extract (MCE) of Bacillus licheniformis (N = 4 × 10). Error bars represent standard deviations of the mean. ∗indicates significant differences with the control group (Kruskal-Wallis, p = 0.045. Dunn’s post hoc test, p = 0.027).
In the bioassay with larvae of the barnacle A. amphitrite, the control group achieved an average settlement of 63.4 ± 9.4%, while at the highest MCE concentrations tested, settlement averages of 27.5 ± 9.6 and 21.7 ± 26.5% were achieved at 830 and 1000 mg/L, respectively (data not shown). Overall, the B. licheniformis MCE displayed low anti-fouling efficacy properties to inhibit the settlement of the barnacle A. amphitrite, with an EC 50 of 733.60 mg/L (95% CI = 539.24–927.96) (Figure 5).
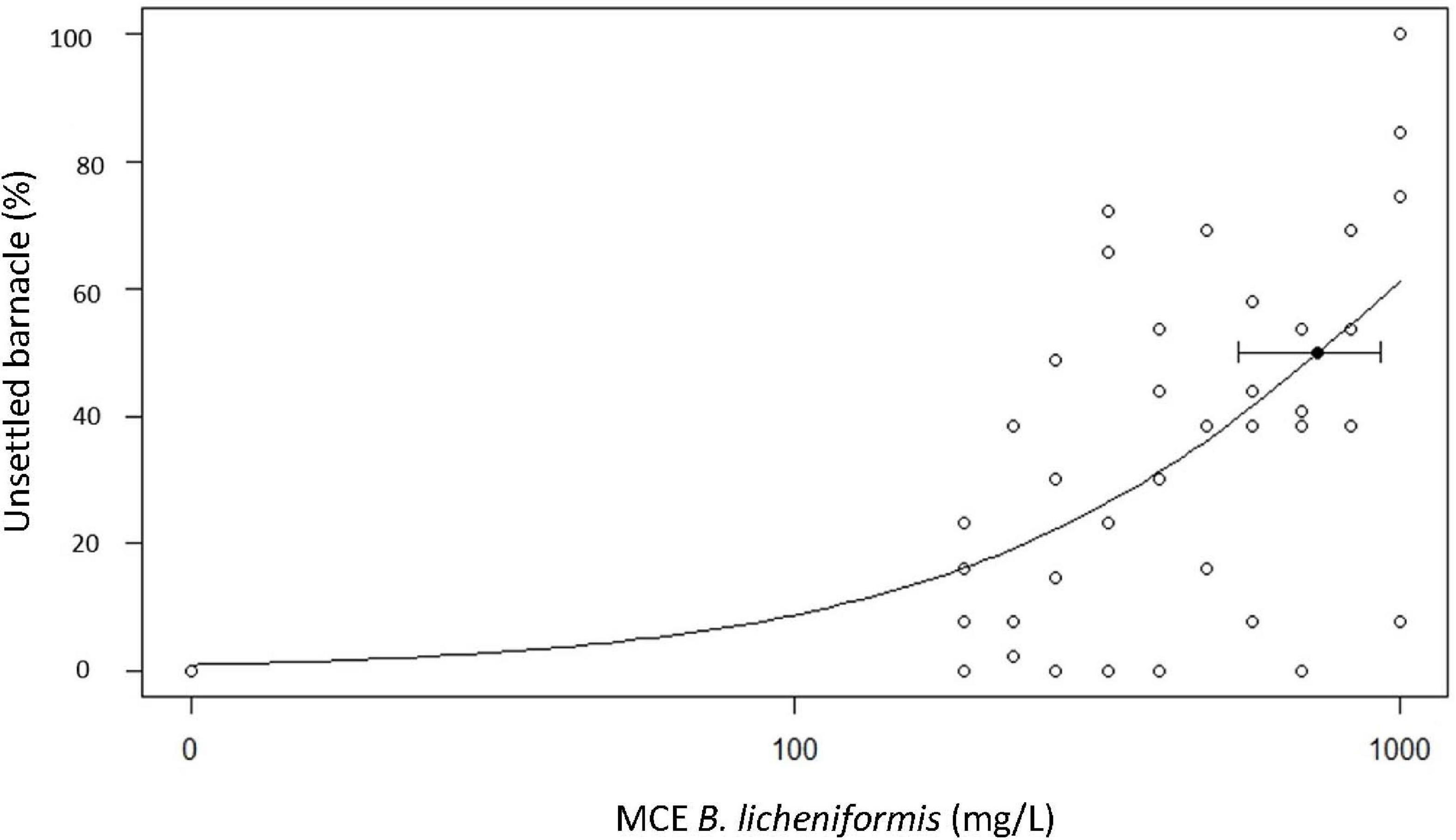
Figure 5. Percentage of cyprid barnacles Amphibalanus amphitrite not settled after exposure to a dilution series of the methanol cell extract (MCE) of Bacillus licheniformis. Median Efficacy Concentration EC50 plotted with 95% Confidence Intervals. EC50 = 733.60 mg/L (95% CI = 539.24–927.96).
In the toxicity trials with A. salina, the B. licheniformis MCE induced less than 10% mortality at 1000 mg/L (Supplementary Figure 5), indicating that it is not toxic to the brine shrimp A. salina. Toxicity assays against the marine microalgae Tetraselmis suecica, Nannochloropsis gaditana, and Porphyridium cruentum showed no toxicity of the MCE (data not shown).
Comparing the anti-fouling efficacy of MCE B. licheniformis with the state-of-the-art biofilm inhibitor CuPT (Table 1), it was observed that the efficacy of the bacterial extract is 220 times lower against the settlement of the soft bryozoan B. neritina and 22,925 times lower against the settlement of the encrusting barnacle A. amphitrite compared to the biocide of reference. On the other hand, the toxicity to the non-target species A. salina is more than 4 × 105 times lower with the MCE extract than CuPT, indicating that it could constitute an environmentally friendly alternative to state-of-the-art anti-fouling biocides.
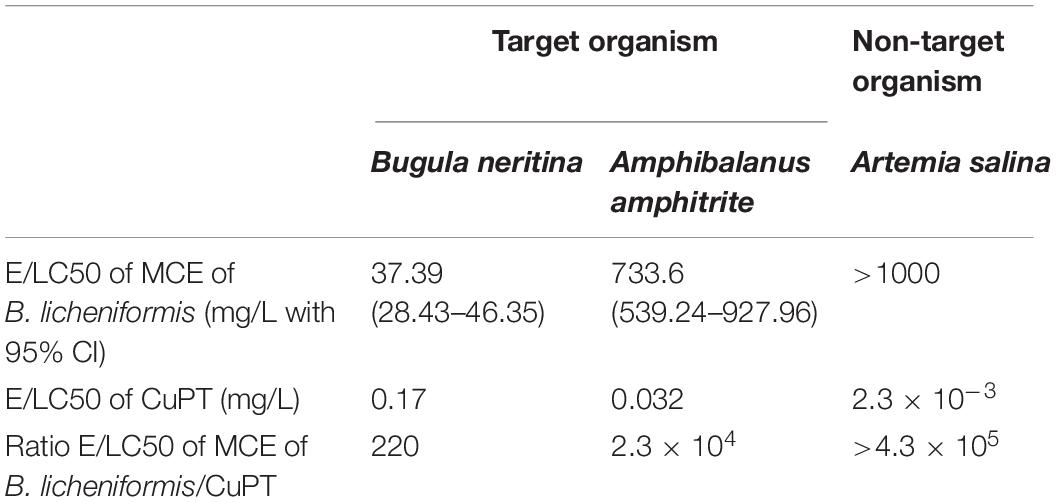
Table 1. Comparison of anti-fouling efficacy (EC50) of B. licheniformis methanol cell extract (MCE) and CuPT against larvae of the bryozoan Bugula neritina and the barnacle Amphibalanus amphitrite and toxicity (LC50) to the brine shrimp Artemia salina.
Anti-fouling Efficacy of the MCE of B. licheniformis in Field Tests
Following the promising results in the bioassays with bacteria and soft fouling species, the MCE was incorporated at 2 and 5% w/w in a solvent-based self-polishing standard test product and immersed in the Red Sea for 6 months. Monthly photographs of the panels revealed a steady growth of soft fouling organisms during the trial (Figure 6). Differences in the color of the panels are due to differences in the color of the matrix. Although the color of the substrate has been described to influence the settlement of micro and macro-foulers in swallow waters (Dobretsov et al., 2013a), this effect can be considered negligible in our case, since the panels were submerged at a depth of 6–8 m. Fouling organisms colonized over 40% of the control panel surface after 132 days of immersion and up to an average of 47.6 ± 11.8% after 180 days (Figure 7). The positive control with ZnPT at 2% maintained low fouling coverage throughout the experiment up to 2.47 ± 1.14% while treatment with 2% SEA-NINETM accumulated 10.46 ± 2.85% fouling coverage after 180 days of exposure (data not shown). In the 2% w/w treatment group, biofouling organisms colonized on average 40.1 ± 3% of the panels after 180 days of immersion, while in the 5% w/w treatment group, 32.7 ± 4% of the panel surface was colonized. The B. licheniformis MCE inhibited the growth of biofouling organisms in a concentration-dependent manner, as observed in the laboratory bioassays. The coefficient of variations in the treatment groups was calculated around 12% (data not shown), indicating consistency and that the formulation was stable during the immersion trial. Statistical analysis revealed significant differences between the different treatment groups and the control (linear mixed effect models with nested random effect, p = 0.036). Post hoc analysis indicated the fouling coverage percentage on the coating with B. licheniformis at 5% was significantly lower than the control group (Tukey, p = 0.027). Nevertheless, the inhibitory activity was not continuous since, at 5% w/w, biofouling growth almost stopped in the second half of the trial (Figure 7), whereas biofouling organisms continued to colonize the control coatings steadily, indicating that the MCE extract might have started to leach at the coating’s surface from the third month onward.
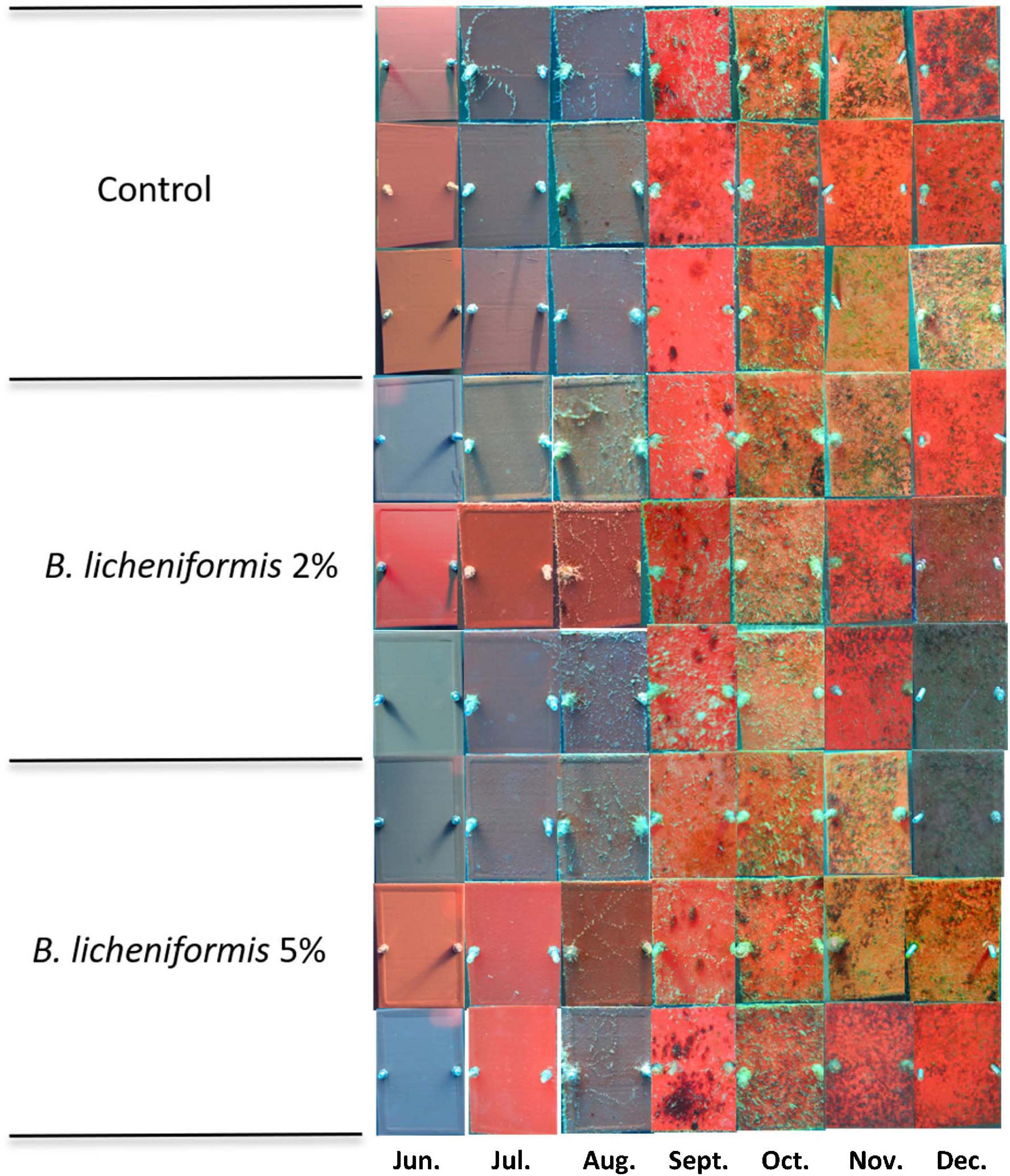
Figure 6. Panels of the static immersion experiment featuring fouling growth from June to December 2016 in the Red Sea (29.501451°N, 34.916285°E) photographed monthly starting from day 0. The Bacillus licheniformis methanol cell extract (MCE) was incorporated at 2 and 5% w/w in a solvent-based self-polishing coating test product from Jotun without biocides. Differences in the color of the panels are due to differences in the color of the matrix.
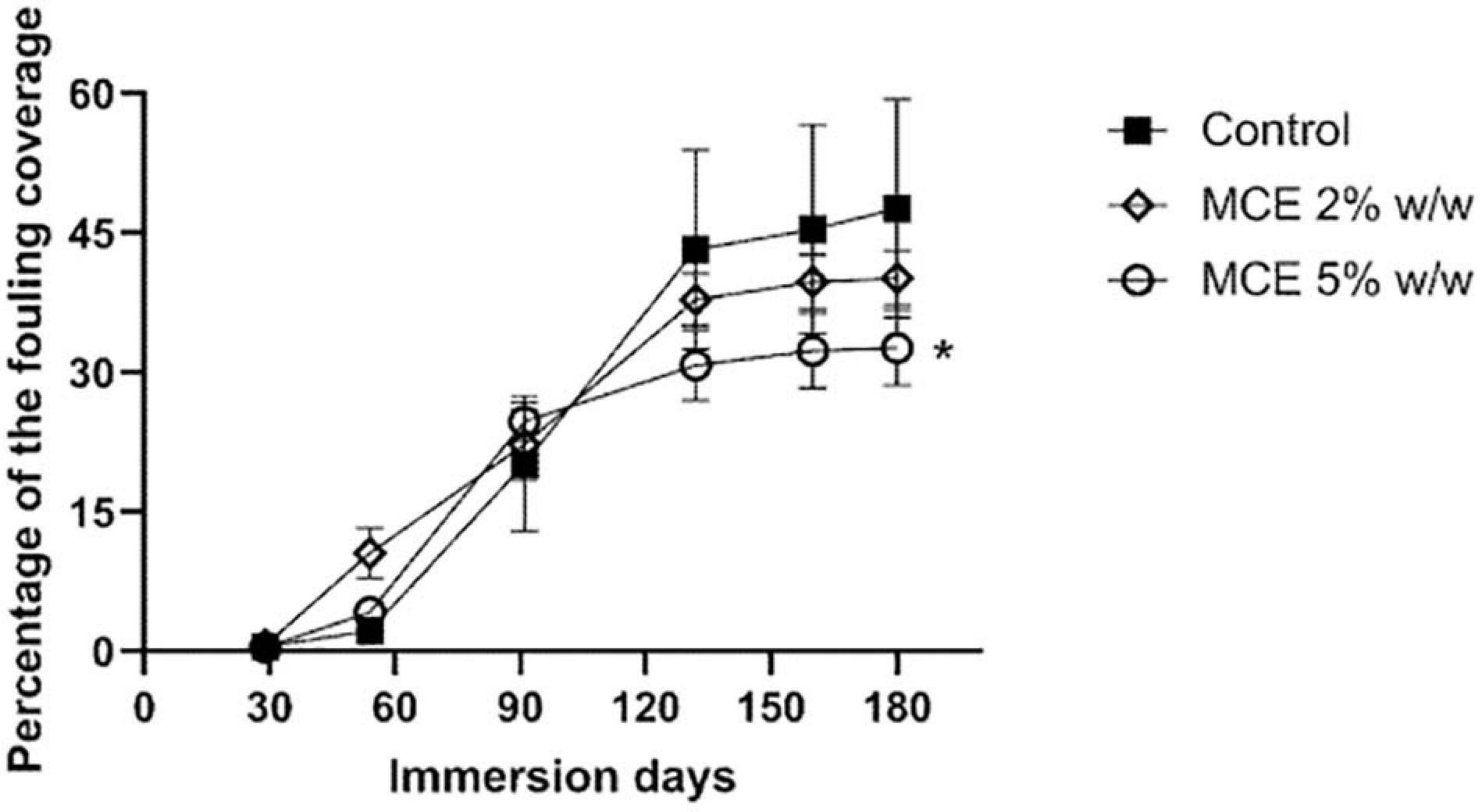
Figure 7. Average percentage fouling coverage on panels coated with a control solvent-based self-polishing coating (n = 12, black squares) and with the methanol cell extract (MCE) of B. licheniformis at 2% (open rhombus) and 5% (open circles) w/w (n = 3) submerged in the Red Sea for 180 days (June–December 2016). Error bars represent standard deviations of the mean. * indicates statistical significance between a treatment and the control group (linear mixed-effects model with nested random effects, Bonferroni correction, Tukey post hoc analysis, p < 0.05).
Discussion
Because of the complexity of the micro and macro-organisms involved in marine biofouling, it is accepted that no single environmental-friendly compound could inhibit the attachment of all of them. Therefore, a wide range of test organisms has been used in in vitro anti-fouling tests (Briand, 2009) but the real effectivity of an anti-fouling compound can only be assessed in field trials. The use of microbial extracts containing multiple bioactive substances may constitute a cost-effective, eco-friendly alternative to the use of chemically synthesized biocides (Adnan et al., 2018; Kharchenko et al., 2020; Liu et al., 2020). The possibility of fine-tuning the production of anti-fouling compounds through cultivation techniques (Rajarajan et al., 2013), the stability of the production and the ease of cultivation represent clear advantages of microorganisms as a source of anti-fouling compounds in comparison to higher organisms. In the present study, the ability of a methanolic extract of B. licheniformis NCTC 10341T biomass to prevent biofouling was assessed under natural conditions, after being selected in laboratory assays. In order to select the most promising extract, the inhibition of the biofilm formation by four marine bacteria biofilm-forming strains (Muras et al., 2021a) was assessed. To avoid the handicaps of the standard end-point techniques (Pantanella et al., 2013), the online measuring equipment xCELLigenceR® System RTCA DP (Agilent Technologies Inc., Santa Clara, CA, United States) was used as a screening method for the identification of new anti-microfouling compounds (Muras et al., 2021a). Both aqueous and methanol cell extracts from B. licheniformis NCTC 10341T presented biofilm inhibitory activity, although this activity was species-specific. The anti-biofilm activity observed in MCE showed different effects depending on the test microorganism, the concentration of the bioactive compound, and the culture method. The most sensitive micro-fouler was P. maricaloris, for which biofilm was reduced by 55% after 24 h and by 28.97% after 48 h when the MCE was added at 0.35 mg/ml. No biofilm inhibition could be identified in the cell-free spend medium from B. licheniformis NCTC 10341T culture, while in previous studies, the presence of different compounds identified in cell-free supernatants of different B. licheniformis strains such as polysaccharides, lipopeptides, and antimicrobial peptides was reported to reduce the biofilm formation of a range of both Gram-negative and Gram-positive bacteria (Rivardo et al., 2009; Sayem et al., 2011; Dusane et al., 2013). These results confirm that the anti-fouling activity found in B. licheniformis is highly variable among strains and may also depend on the culture conditions, as demonstrated by the differences in activity observed for the B. licheniformis MCE obtained with different culture media (Supplementary Figure 2). Nevertheless, the activity retrieved in the MCE obtained in LB medium was constant, indicating the feasibility of producing cell extracts with a stable biofouling activity when the culture conditions are properly controlled. Due to its wide enzymatic arsenal, B. licheniformis has been cultured on different industrial wastes (Vasconcellos et al., 2009; Mao et al., 2013), a possibility that should be explored to further reduce the cost of production of anti-fouling extracts. Nevertheless, the identification of the compounds responsible for the observed anti-fouling activities of the extract is a necessary step in order to be able to strictly monitor the activity of the anti-fouling microbial extracts produced at an industrial scale and before any microbial extract can be commercialized for anti-fouling applications (Rittschof et al., 2015).
Gram-negative bacteria are predominant in marine environments, including biofilms (Cicirelli et al., 2014; Muras et al., 2018; Antunes et al., 2019). Many Gram-negatives produce QS signals known as N-Acyl homoserine lactones (AHLs) that are involved in the process of biofilm formation and have been therefore identified as targets for the development of anti-fouling compounds (Chen and Qian, 2017; Antunes et al., 2019). The interception of this type of QS signals has been proposed as a novel anti-fouling strategy, since the cues produced by bacterial biofilms are important for the attachment of macro-foulers (Dobretsov et al., 2007, 2011). The use of QQ strategies for the control of biofilm formation has been demonstrated to be successful not only for membrane bioreactors operating at under high organic load conditions (Soler et al., 2018) and environmental biofilms (Huang et al., 2019) but also for the clinical ones (Mayer et al., 2018; Muras et al., 2020). Although different Bacillus species have been reported to degrade QS signals, B. licheniformis NCTC 10341T could not block either the short-chain C6-HSL or the long-chain C10-HSL, at least in the conditions tested. It should be noted that, although the production of AHLs is highly dependent on culture conditions, the capacity to degrade these signals seems to be expressed independently of the culture conditions, and in many cases, the activity is expressed constitutively (Romero et al., 2012; Muras et al., 2018). In this sense, the anti-biofilm activity of sponge-associated B. licheniformis strains was not caused by the competition with the QS signals (Sayem et al., 2011). On the contrary, the B. licheniformis DHAB1 strain isolated from healthy Indian white shrimps (Fenneropanaeus indicus) showed anti-biofilm activity against Vibrio sp. that has been attributed to the production of the AHL-lactonase AiiA (Vinoj et al., 2014). These results further evidence the wide metabolic variability among B. licheniformis strains and the importance of strain selection for the success of microbial-based anti-fouling technologies.
Different types of cell extracts from B. licheniformis were tested against the biofilm formed by P. maricaloris to obtain more information on the biochemical nature of the compound responsible for the biofilm inhibitory activity. The MCE-DM (76.79%) and ACE-EA (71.2%) presented the highest anti-biofilm activity. Both extracts were obtained using fewer polar solvents than methanol or water since ethyl acetate is a polar aprotic solvent and dichloromethane is highly non-polar. Hence, these results seem to suggest that the molecule responsible for the anti-biofilm activity of B. licheniformis could be a non-polar substance since the highest anti-biofilm activity was found in the MCE-DM, the less polar extract. More detailed studies, including further fractionation of the MCE and assessment of the activity on other macro-foulers are necessary to characterize further the molecule(s) responsible for the anti-biofilm activity of the extracts of B. licheniformis. Moreover, the chemical elucidation of the structure of the active compounds is an unavoidable requirement for allowing the quality control of the production of extracts at an industrial scale. However, we can discard molecules such as enzymes or proteins that have been previously identified as sources of anti-fouling activity in B. licheniformis strains (Nijland et al., 2010; Dusane et al., 2013; Muslim et al., 2016; Burgess et al., 2017).
The identification of bacterial extracts with multiple inhibitory activities is of special interest due to the complex nature of the fouling process. Therefore, the MCE extract, presenting the highest anti-biofilm activity, was further tested in vitro using larvae of the soft bryozoan B. neritina and the barnacle A. amphitrite. The settlement of the larvae of the soft bryozoan B. neritina is dependent on endogenous and exogenous factors, but under optimal conditions, 50% of larvae are able to settle within 2 h when appropriate settlement cues are present (Keough, 1978). We provide evidence that, after short exposition times, the B. licheniformis MCE prevents B. neritina larvae settlement at low concentrations, with an EC50 of 37.90 mg/L, without inducing mortality, in contrast with the effect of commercial anti-fouling biocides such as copper pyrithione, which usually causes the death of larvae within 24 h. The lethality observed at the end of the 48 h bioassay probably reflects the experimental conditions with a small volume of water. In the field, we can hypothesize that upon coming in contact with a coating leaching the B. licheniformis inhibitory compound, the larvae would swim away and seek an alternative settlement surface, as reported for natural deterrents produced by invertebrates and other fouling repellents (Krug, 2006; Xie et al., 2020). Although other commercial compounds such as the QS-blockers triclosan and furanone can inhibit B. neritina larval settlement (Dobretsov et al., 2007), they also exhibit toxicity, and therefore, their use should be limited (Rasch et al., 2004; Dann and Hontela, 2011). The unique, anti-macrofouling efficacy of B. licheniformis against B. neritina could be explained by the important role of bacterial cues for settlement in this species. B. neritina settlement directly correlates with bacteria and diatoms densities in biofilms (Dahms et al., 2004). The species settles better on biofilms of various bacteria species but is also an indiscriminate colonizer and can attach to certain monospecific bacterial films (Dahms et al., 2004). Since filtered natural seawater was used in our experiments, the observed settlement delays may indicate that B. licheniformis could inhibit the activity of a wide variety of Bacterial species, therefore blocking the production of bacterial settlement cues. Many sessile invertebrates, such as coral or tubeworm larvae (Dobretsov and Rittschof, 2020), require external cues to settle, which may also be inhibited by the MCE of B. licheniformis. The lack of activity against A. amphitrite larvae, A. salina nauplii and three different microalgal species: T. suecica, N. gaditana, and P. cruentum suggests a specificity of action and low toxicity of the anti-fouling compounds present in the B. licheniformis MCE. It should be noted that the crude extract of a Bacillus cereus strain with anti-fouling potential presented an EC50 value of 290.6 mg/L against the microalga Skeletonema costatum (Gao et al., 2014) while the B. licheniformis MCE showed no toxicity against Artemia nauplii and microalgae at concentrations as high as 1000 mg/L. Dozens of biocides are currently used in anti-fouling products and their toxicity effects on marine ecosystems are not fully understood. Martins et al. (2017) derived Predicted No Effect Concentrations (PNECs) values for a wide range of biocides, including CuPT, and PNECs Acute seawater values reported are often below 1 × 10–2 μg/L (CuPT, ZnPT, DCOIT, Diuron, and Irgarol), except for Chlorothalonil and Dichlofluanid (0.11 and 0.2 μg/L, respectively). Our preliminary results indicate that the B. licheniformis extract would display low toxicity effects on marine ecosystems. However, additional testing with diverse models from different trophic levels should be performed in order to validate this theory. Further experiments with other macro-foulers are also required to determine the mode of action and to identify the active compounds present in the extract that are responsible for the observed activity. Despite the interest of the positive results obtained with the MCE of B. licheniformis, and the economic advantages of using bacterial extracts instead of purified compounds, the identification of the active substances is pivotal in order to assess the stability of the activity in the biomass produced as well as to fulfill the regulatory requirements (Rittschof et al., 2015; McNeil, 2018; Tian et al., 2021).
The field tests demonstrated that the MCE from B. licheniformis NCTC 10341T can be successfully incorporated in a standard self-polishing coating formulation and retain its anti-fouling properties for up to 6 months. Coatings with 5% w/w MCE of B. licheniformis NCTC 10341T displayed a significant and consistent reduction of fouling growth during the 6 months’ trial that was evident only after the third month, with a reduction of the fouling coverage by 30% compared to the control group. This reduction in fouling coverage is low in comparison with other biocides such as ZnPT or SEA-NINETM, which achieved more than 90% reduction in the same experiment, corroborating results from laboratory experiments against macro-foulers such as the barnacle A. amphitrite. For this reason, the B. licheniformis NCTC 10341T MCE should be explored as a booster biocide, in conjunction with a biocide with a broad-spectrum anti-fouling activity able to prevent the settlement of hard-encrusting macro-fouler organisms. The incorporation of 1% butanol extract of Pseudoalteromonas piscicida in paints also inhibited macrofouling and reduced microfouling 1.5–2.5-fold in the coating/seawater interface in a 3 months experiment, with important differences being found in the activity depending on the organic extract assayed (Kharchenko et al., 2020). Further purification/concentration of the B. licheniformis MCE extract may therefore result in higher effectivity. The fouling coverage’s progression on the coatings with the B. licheniformis NCTC 10341T extract suggests that the coating formulation needs further research to adjust the leaching rate, as previously demonstrated for the modulation of leaching rate of biocidal tannins extracted from the mangrove Rizophora apiculata (Noor Idora et al., 2015). It must be noted that despite the field trial was performed in a period of the year characterized by massive reproduction of various invertebrates (June–December) and included a mild upwelling event that occurs in September, fouling pressure of the Red Sea is low since its seawater represents an oligotrophic environment. Few hard-fouling organisms settled during the trial on all panels, including on the control group. Field trials in different geographic locations with a higher fouling pressure, with barnacles as dominant species, such as the Atlantic or the Pacific Ocean, might yield different results. Moreover, longer exposition experiments are required to assess the long-term anti-fouling effect (Azevedo et al., 2020).
This study demonstrated an environmentally friendly anti-fouling activity in B. licheniformis MCE, specifically targeting biofilm and preventing soft bryozoan larvae settlement, with low toxicity impacts, in contrast with current commercial biocides that display a broad activity spectrum but also harm non-target organisms in the marine environment. B. licheniformis NCTC 10341T represents an eco-friendly solution for biofilm and soft-fouler settlement prevention and could be used as a booster biocide. Despite the economic advantages of using cell extracts, further analysis on the mechanisms of action of this cell extract in biofilm inhibition is required, since the possibility that different molecules are responsible for the observed inhibitory activities against micro and macro-foulers cannot be disregarded. Moreover, in the view that differences in the activity were observed depending on the culture medium and conditions used, further optimization experiments would be required in order to maximize the activity in the extracts. Also, improving its incorporation in the anti-fouling coating formulations is necessary to optimize and stabilize the extract’s leaching rate.
Data Availability Statement
The raw data supporting the conclusions of this article will be made available by the authors, without undue reservation.
Author Contributions
AM, SL, CM, TT, and RW performed the experiments and data analysis. AM and SL wrote the first draft of the manuscript. AO and YB contributed to conception and design of the study and supervised the experiments. All authors contributed to manuscript revision, read, and approved the submitted version.
Funding
This work was supported by the European Union under Grant FP7-OCEAN-2013 612717 (Low-toxic cost-efficient environment-friendly anti-fouling materials). AM was supported by a predoctoral fellowship from the Consellería de Cultura, Educación e Ordenación Universitaria, Xunta de Galicia (ED481A-2015/311). CM was supported by a post-doctoral fellowship from Xunta de Galicia (IN606B-2019/010).
Conflict of Interest
SL and TT were employed by Merchant AquaBioTech Group.
The remaining authors declare that the research was conducted in the absence of any commercial or financial relationships that could be construed as a potential conflict of interest.
Publisher’s Note
All claims expressed in this article are solely those of the authors and do not necessarily represent those of their affiliated organizations, or those of the publisher, the editors and the reviewers. Any product that may be evaluated in this article, or claim that may be made by its manufacturer, is not guaranteed or endorsed by the publisher.
Acknowledgments
We would like to thank Juan Barja from Universidade de Santiago de Compostela for kindly providing us with P. flavipulchra and P. maricaloris strains used in this study. We would like to thank Paul Williams from the University of Nottingham and Tomohiro Morohoshi from Utsunomiya University for kindly providing us with the Chromobacterium violaceum CV026 and VIR07 biosensor strains, respectively. We would like to thank Jotun S. A. for providing the benchmarked coatings and Smallmatek lda. for applying the coatings on PVC plates. We would also like to thank Manuela Maranhão and Inês Pimparel for the collection and husbandry of the marine organisms. We thank the Interuniversity Institute for Marine Sciences in Eilat (IUI) for logistic support and kind hospitality and T. Shaler for assisting in the anti-fouling assays. The collection of animals complied with a permit issued by the Israel Nature and National Parks Protection Authority.
Supplementary Material
The Supplementary Material for this article can be found online at: https://www.frontiersin.org/articles/10.3389/fmars.2021.711108/full#supplementary-material
Footnotes
References
Adnan, M., Alshammari, E., Patel, M., Amir Ashraf, S., Khan, S., and Hadi, S. (2018). Significance and potential of marine microbial natural bioactive compounds against biofilms/biofouling: necessity for green chemistry. PeerJ 6:e5049. doi: 10.7717/peerj.5049
Alemán-Vega, M., Sánchez-Lozano, I., Hernández-Guerrero, C. J., Hellio, C., and Quintana, E. T. (2020). Exploring antifouling activity of biosurfactants producing marine bacteria isolated from Gulf of California. Int. J. Mol. Sci. 21:6068. doi: 10.3390/ijms21176068
Antunes, J., Leão, P., and Vasconcelos, V. (2019). Marine biofilms: diversity of communities and of chemical cues. Environ. Microbiol. Rep. 11, 287–305. doi: 10.1111/1758-2229.12694
Armstrong, E., Boyd, K. G., Pisacane, A., Peppiatt, C. J., and Burgess, J. G. (2000). Marine microbial natural products in antifouling coatings. Biofouling 16, 215–224. doi: 10.1080/08927010009378446
ASTM D3623-78a. (2020). Standard Test Method for Testing Antifouling Panels in Shallow Submergence. West Conshohocken: ASTM International.
ASTM D6990-20. (2020). Standard Practice for Evaluating Biofouling Resistance and Physical Performance of Marine Coating Systems. West Conshohocken: ASTM International.
Azevedo, J., Antunes, J. T., Machado, A. M., Vasconcelos, V., Leão, P. N., and Froufe, E. (2020). Monitoring of biofouling communities in a Portuguese port using a combined morphological and metabarcoding approach. Sci. Rep. 10:13461. doi: 10.1038/s41598-020-70307-4
Briand, J. F. (2009). Marine anti-fouling laboratory bioassays: an overview of their diversity. Biofouling 4, 297–311. doi: 10.1080/08927010902745316
Brock, E., Nylund, G. M., and Pavia, H. (2007). Chemical inhibition of barnacle larval settlement by the brown alga Fucus vesiculosus. Mar. Ecol. Prog. Ser. 337, 165–174. doi: 10.3354/meps337165
Burgess, J. G., Boyd, K. G., Amstrong, E., Jiang, Z., Yan, L., Berggren, M., et al. (2003). The development of a marine natural product-based anti-fouling paint. Biofouling 19, 197–205. doi: 10.1080/0892701031000061778
Burgess, J. G., Hall, M. J., and Nijland, R. (2017). Compounds and Methods for Biofilm Disruption and Prevention. Patent US9675736.
Chen, L., Duan, Y., Cui, M., Huang, R., Su, R., Qi, W., et al. (2021). Biomimetic surface coatings for marine antifouling: natural antifoulants, synthetic polymers and surface microtopography. Sci. Total Environ. 766:144469. doi: 10.1016/j.scitotenv.2020.144469
Chen, L., and Lam, C. J. (2017). SeaNine 211 as anti-fouling biocide: a coastal pollutant of emerging concern. J. Environ. Sci. 61, 68–79. doi: 10.1016/j.jes.2017.03.040
Chen, L., and Qian, P. Y. (2017). Review of molecular mechanisms of anti-fouling compounds: an update since 2012. Mar. Drugs 15:264. doi: 10.3390/md15090264
Chen, L., Xia, C., and Qian, P. Y. (2017). Optimization of anti-fouling coatings incorporating butenolide, a potent anti-fouling agent via field and laboratory tests. Prog. Org. Coat. 109, 22–29. doi: 10.1016/j.porgcoat.2017.04.014
Cicirelli, E. M., Williamson, H., Tait, K., and Fuqua, C. (2014). “Acylated homoserine lactone signaling in marine bacterial systems,” in Chemical Communication Among Bacteria, eds S. C. Winans and B. L. Bassler (Washington: ASM Press), 251–272. doi: 10.1128/9781555815578.ch16
Coronel-León, J., Marqués, A. M., Bastida, J., and Manresa, A. (2016). Optimizing the production of the biosurfactant lichenysin and its application in biofilm control. J. Appl. Microbiol. 120, 99–111. doi: 10.1111/jam.12992
Dahms, H. U., Dobretsov, S., and Qian, P. Y. (2004). The effect of bacterial and diatom biofilms on the settlement of the bryozoan Bugula neritina. J. Exp. Mar. Biol. Ecol. 313, 191–209. doi: 10.1016/j.jembe.2004.08.005
Dann, A. B., and Hontela, A. (2011). Triclosan: environmental exposure, toxicity and mechanisms of action. J. Appl. Toxicol. 31, 285–311. doi: 10.1002/jat.1660
Dobretsov, S., Abed, R. M., and Voolstra, C. R. (2013a). The effect of surface colour on the formation of marine micro and macrofouling communities. Biofouling 29, 617–627. doi: 10.1080/08927014.2013.784279
Dobretsov, S., Dahms, H. U., Yili, H., Wahl, M., and Qian, P. Y. (2007). The effect of quorum sensing blockers on the formation of marine microbial communities and larval attachment. FEMS Microbiol. Ecol. 60, 177–188. doi: 10.1111/j.1574-6941.2007.00285.x
Dobretsov, S., Raeid, M. M. A., and Teplitski, M. (2013b). Minireview: inhibition of biofouling by marine microorganisms. Biofouling 29, 423–441. doi: 10.1080/08927014.2013.776042
Dobretsov, S., and Rittschof, D. (2020). Love at first taste: induction of larval settlement by marine microbes. Int. J. Mol. Sci. 21:731. doi: 10.3390/ijms21030731
Dobretsov, S., Teplitski, M., Bayer, M., Gunasekera, S., Proksch, P., and Paul, V. J. (2011). Inhibition of marine biofouling by bacterial quorum sensing inhibitors. Biofouling 27, 893–905. doi: 10.1080/08927014.2011.609616
Dong, Y. H., Gusti, A. R., Zhang, Q., Xu, J. L., and Zhang, L. H. (2002). Identification of quorum-quenching N-acyl homoserine lactonases from Bacillus species. Appl. Environ. Microbiol. 68, 1754–1759. doi: 10.1128/AEM.68.4.1754-1759.2002
Dusane, D. H., Damare, S. R., Nancharaiah, Y. V., Ramaiah, N., Venugopalan, V. P., Kumar, A. R., et al. (2013). Disruption of microbial biofilm by an extracellular protein isolated from epibiotic tropical marine strain of Bacillus licheniformis. PLoS One 8:e64501. doi: 10.1371/journal.pone.0064501
Dworjanyn, S. A., de Nys, R., and Steinberg, P. D. (2006). Chemically mediated anti-fouling in the red alga Delisea pulchra. Mar. Ecol. Prog. Ser. 318, 153–163. doi: 10.3354/meps318153
Eduok, U., Suleiman, R., Gittens, J., Khaled, M., Smith, T. J., Akid, R., et al. (2015). Anticorrosion/anti-fouling properties of bacterial spore-loaded sol–gel type coating for mild steel in saline marine condition: a case of thermophilic strain of Bacillus licheniformis. RSC Adv. 5, 93818–93830. doi: 10.1039/C5RA16494J
Freire, I., Gutner-Hoch, E., Muras, A., Benayahu, Y., and Otero, A. (2019). The effect of bacteria on planula-larvae settlement and metamorphosis in the octocoral Rhytisma fulvum fulvum. PLoS One 14:e0223214. doi: 10.1371/journal.pone.0223214
Gao, M., Wang, K., Su, R., Su, R., Li, X., and Lu, W. (2014). Antifouling potential of bacteria isolated from a marine biofilm. J. Ocean Univ. China 13, 799–804. doi: 10.1007/s11802-014-2469-9
Gatenholm, P., Holmström, C., Maki, J. S., and Kjelleberg, S. (1995). Toward biological antifouling surface coatings: marine bacteria immobilized in hydrogel inhibit barnacle larvae. Biofouling 8, 293–301. doi: 10.1080/08927019509378282
Gibbs, P. E., and Bryan, G. W. (2009). Reproductive failure in populations of the dog-whelk, Nucella lapillus, caused by imposex induced by tributyltin from anti-fouling paints. J. Mar. Biol. Ass. U. K. 66, 767–777. doi: 10.1017/S0025315400048414
Gu, Y., Yu, L., Mou, J., Wu, D., Xu, M., Zhou, P., et al. (2020). Research Strategies to Develop Environmentally Friendly Marine Antifouling Coatings. Mar. Drugs 18:371. doi: 10.3390/md18070371
Gutner-Hoch, E., Martins, R., Oliveira, T., Maia, F., Soares, A. M. V. M., Loureiro, S., et al. (2018). Antimicrofouling efficacy of innovative inorganic nanomaterials loaded with booster biocides. J. Mar. Sci. Eng. 6:6. doi: 10.3390/jmse6010006
Harrison, A. M., and Soby, S. D. (2020). Reclassification of Chromobacterium violaceum ATCC 31532 and its quorum biosensor mutant CV026 to Chromobacterium subtsugae. AMB Exp. 10:202. doi: 10.1186/s13568-020-01140-1
Hellio, C., and Yebra, D. M. (2009). Advances in Marine Antifouling Coatings and Technologies. Cornwall: CRC Press.
Huang, S., Bergonzi, C., Schwab, M., Elias, M., and Randall, E. H. (2019). Evaluation of biological and enzymatic quorum quencher coating additives to reduce biocorrosion of steel. PLoS One 14:e0217059. doi: 10.1371/journal.pone.0217059
Jia, R., Unsal, T., Xu, D., Lekbach, Y., and Gu, T. (2019). Microbiologically influenced corrosion and current mitigation strategies: a state of the art review. Int. Biodeterior. Biodegradation 137, 42–58. doi: 10.1016/j.ibiod.2018.11.007
Kavouras, P., Trompeta, A. F., Larroze, S., Maranhão, M., Teixeira, T., Beltri, M., et al. (2019). Correlation of mechanical properties with anti-fouling efficacy of coatings containing loaded microcapsules. Prog. Org. Coatings 136:105249. doi: 10.1016/j.porgcoat.2019.105249
Keough, M. J. (1978). Dispersal of the bryozoan Bugula Neritina and effects of adults on newly metamorphosed juveniles. Mar. Ecol. Prog. Ser. 57, 163–171. doi: 10.3354/meps057163
Kharchenko, U., Beleneva, I., and Karpov, V. (2020). Antifouling and anticorrosion potential of Pseudoalteromonas piscicida extract. J. Coat. Technol. Res. 17, 887–896. doi: 10.1007/s11998-019-00308-0
Krug, P. J. (2006). “Defense of benthic invertebrates against surface colonization by larvae: a chemical arms race,” in Antifouling Compounds. Marine Molecular Biotechnology, Vol. 42, eds N. Fusetani and A. S. Clare (Berlin: Springer).
Liu, L. L., Wu, C. H., and Qian, P. Y. (2020). Marine natural products as anti-fouling molecules - a mini-review (2014-2020). Biofouling 36, 1210–1226. doi: 10.1080/08927014.2020.1864343
Ma, W., Panecka, M., Tufenkji, N., and Rahaman, M. S. (2018). Bacteriophage-based strategies for biofouling control and ultrafiltration: in situ biofouling mitigation, biocidal additives and biofilm cleanser. J. Colloid Interface Sci. 523, 254–265. doi: 10.1016/j.jcis.2018.03.105
Mao, X., Liu, P., He, S., Xie, J., Kan, F., Yu, C., et al. (2013). Antioxidant properties of bio-active substances from shrimp head fermented by Bacillus licheniformis OPL-007. Appl. Biochem. Biotechnol. 171, 1240–1252. doi: 10.1007/s12010-013-0217-z
Maréchal, J. P., and Hellio, C. (2009). Challenges for the development of new non-toxic antifouling solutions. Int. J. Mol. Sci. 10, 4623–4637. doi: 10.3390/ijms10114623
Martins, S. E., Fillmann, G., Lillicrap, A., and Thomas, K. V. (2017). Review: ecotoxicity of organic and organo-metallic antifouling co-biocides and implications for environmental hazard and risk assessments in aquatic ecosystems. Biofouling 34, 34–52. doi: 10.1080/08927014.2017.1404036
Mayer, C., Muras, A., Romero, M., Rumbo-Leal, S., López-Días, M., Tomás, M., et al. (2018). Multiple quorum quenching enzymes are active in the nosocomial pathogen Acinetobacter baumannii ATCC17979. Front. Cell Infect. Microbiol. 8:310. doi: 10.3389/fcimb.2018.00310
McClean, K. H., Winson, M. K., Fish, L., Taylor, A., Chhabra, S. R., Cámara, M., et al. (1997). Quorum sensing and Chromobacterium violaceum: exploitation of violacein production and inhibition for the detection of N-acylhomoserine lactones. Microbiology 143, 3703–3711. doi: 10.1099/00221287-143-12-3703
McNeil, E. M. (2018). Antifouling: regulation of biocides in the UK before and after Brexit. Mar. Policy 92, 58–60. doi: 10.1016/j.marpol.2018.02.015
Morohoshi, T., Kato, M., Fukamachi, K., Kato, N., and Ikeda, T. (2008). N-acylhomoserine lactone regulates violacein production in Chromobacterium violaceum type strain ATCC 12472. FEMS Microbiol. Lett. 279, 124–130. doi: 10.1111/j.1574-6968.2007.01016.x
Muras, A., López-Pérez, M., Mayer, C., Parga, A., Amaro-Blanco, J., and Otero, A. (2018). High prevalence of quorum-sensing and quorum-quenching activity among cultivable bacteria and metagenomic sequences in the Mediterranean Sea. Genes 9:100. doi: 10.3390/genes9020100
Muras, A., Otero-Casal, P., Blanc, V., and Otero, A. (2020). Acyl homoserine lactone-mediated quorum sensing in the oral cavity: a paradigm revisited. Sci. Rep. 10:9800. doi: 10.1038/s41598-020-66704-4
Muras, A., Parga, A., Mayer, C., and Otero, A. (2021a). Use of quorum sensing inhibition strategies to control microfouling. Mar. Drugs 19:74. doi: 10.3390/md19020074
Muras, A., Romero, M., Mayer, C., and Otero, A. (2021b). Biotechnological applications of Bacillus licheniformis. Crit. Rev. Biotechnol. 41, 609–627. doi: 10.1080/07388551.2021.1873239
Muslim, S. N., Al-Kadmy, I. M. S., Hussein, N. H., Mohammed Ali, A. N., Taha, B. M., Aziz, S. N., et al. (2016). Chitosanase purified from bacterial isolate Bacillus licheniformis of ruined vegetables displays broad spectrum biofilm inhibition. Microb. Pathogen 100, 257–262. doi: 10.1016/j.micpath.2016.10.001
Nijland, N., Hall, M. J., and Burgess, J. G. (2010). Dispersal of biofilms by secreted, matrix degrading, bacterial DNase. PLoS One 5:e15668. doi: 10.1371/journal.pone.0015668
Nir, S., and Reches, M. (2016). Bio-inspired anti-fouling approaches: the quest towards non-toxic and non-biocidal material. Curr. Opin. Biotechnol. 39, 48–55. doi: 10.1016/j.copbio.2015.12.012
Noor Idora, M. S., Ferry, M., Wan Nik, W. B., and Jasnizat, S. (2015). Evaluation of tannin from Rhizophora apiculata as natural antifouling agents in epoxy paint for marine application. Progr. Organ. Coat. 81, 125–131. doi: 10.1016/j.porgcoat.2014.12.012
Ortega-Morales, B. O., Chan-Bacab, M. J., Miranda-Tello, E., Fardau, M. L., Carrero, J. C., and Stein, T. (2008). Anti-fouling activity of sessile bacilli derived from marine surfaces. J. Ind. Microbiol. Biotechnol. 35, 9–15. doi: 10.1007/s10295-007-0260-2
Palanichamy, S., and Subramanian, G. (2017). Anti-fouling properties of marine bacteriocin incorporated epoxybased paint. Prog. Org. Coat. 103, 33–39. doi: 10.1016/j.porgcoat.2016.11.020
Pantanella, F., Valenti, P., Natalizi, T., Passeri, D., and Berlutti, F. (2013). Analytical techniques to study microbial biofilm on abiotic surfaces: pros and cons of the main techniques currently in use. Ann. Ig. 25, 31–42. doi: 10.7416/ai.2013.1904
Peppiatt, C. J., Armstrong, E., Pisacane, A., and Burgess, J. G. (2000). Antibacterial activity of resin based coatings containing marine microbial extracts. Biofouling 16, 225–234. doi: 10.1080/08927010009378447
R Core Team. (2017). R: A Language and Environment for Statistical Computing. Vienna: R Foundation for Statistical Computing.
Rajarajan, N., Ward, A. C., Burgess, J. G., and Glassey, J. (2013). Use of physiological information and process optimization enhances production of extracellular nuclease by a marine strain of Bacillus licheniformis. Biores. Technol. 130, 152–158. doi: 10.1016/j.biortech.2012.12.064
Rasch, M., Buch, C., Austin, B., Slierendrecht, W. J., Ekmann, S. K., Larsen, J. L., et al. (2004). An inhibitor of bacterial quorum sensing reduces mortalities caused by vibriosis in rainbow trout (Oncorhynchus mykiss, Walbaum). System. Appl. Microbiol. 27, 350–359. doi: 10.1078/0723-2020-00268
Rittschof, D., Chai, C., Teo, S. L. M., and Maki, J. S. (2015). Fouling and its next generation management: a perspective. J. Agric. Mar. Sci. 20, 16–23. doi: 10.24200/10.24200/jams.vol26iss1pp1-12
Rittschof, D., Clare, A. S., Gerhart, D. J., Mary, S. A., and Bonaventura, J. (1992). Barnacle in vitro assays for biologically active substances: toxicity and settlement inhibition assays using mass cultured Amphibalanus amphitrite amphitrite darwin. Biofouling 6, 115–122. doi: 10.1080/08927019209386217
Ritz, C., Baty, F., Streibig, J. C., and Gerhard, D. (2015). Dose-response analysis using R. PLoS One 10:e0146021. doi: 10.1371/journal.pone.0146021
Rivardo, F., Turner, R. J., Allegrone, G., Ceri, H., and Martinotti, M. G. (2009). Anti-adhesion activity of two biosurfactants produced by Bacillus spp. prevents biofilm formation of human bacterial pathogens. Appl. Microbiol. Biotechnol. 83, 541–553. doi: 10.1007/s00253-009-1987-7
Romero, M., Martin-Cuadrado, A. B., and Otero, A. (2012). Determination of whether quorum quenching is a common activity in marine bacteria by analysis of cultivable bacteria and metagenomic sequences. Appl. Environ. Microbiol. 78, 6345–6348. doi: 10.1128/AEM.01266-12
Sayem, S. A., Manzo, E., Ciavatta, L., Tramice, A., Cordone, A., Zanfardino, A., et al. (2011). Anti-biofilm activity of an exopolysaccharide from a sponge-associated strain of Bacillus licheniformis. Microb. Cell Fact. 10:74. doi: 10.1186/1475-2859-10-74
Schmitt, T. M., Lindquist, N., and Hay, M. E. (1998). Seaweed secondary metabolites as antifoulants: effects of Dictyota spp. diterpenes on survivorship, settlement, and development of marine invertebrate larvae. Chemoecology 8, 125–131. doi: 10.1007/s000490050017
Shah, K., Mody, K., Keshri, J., and Jha, B. (2010). Purification and characterization of a solvent, detergent and oxidizing agent tolerant protease from Bacillus cereus isolated from the Gulf of Khambhat. J. Mol. Catal. B Enzym. 67, 85–91. doi: 10.1016/j.molcatb.2010.07.010
Soler, A., Arregui, L., Arroyo, M., Mendoza, J. A., Muras, A., Álvarez, C., et al. (2018). Quorum sensing versus quorum quenching bacterial isolates obtained from MBR plants treating leachates from municipal solid waste. Int. J. Environ. Res. Public Health 15:1019. doi: 10.3390/ijerph15051019
Tait, K., and Havenhand, J. (2013). Investigating a possible role for the bacterial signal molecules N-acylhomoserine lactones in Balanus improvises cyprid settlement. Mol. Ecol. 22, 2588–2602. doi: 10.1111/mec.12273
Tait, K., Joint, I., Daykin, M., Milton, D. L., Williams, P., and Cámara, M. (2005). Disruption of quorum sensing in seawater abolishes attraction of zoospores of the green alga Ulva to bacterial biofilms. Environ. Microbiol. 7, 229–240. doi: 10.1111/j.1462-2920.2004.00706.x
Ten Hallers-Tjabbes, C. C., and Walmsley, S. (2010). “Consequences of anti-fouling systems – an environmental perspective,” in Biofouling ed. S. Dürr and J.C. Thomason (Oxford: Blackwell Publishing Ltd.), 243–251. doi: 10.1002/9781444315462.ch17
Tian, L., Yin, Y., Bing, W., and Jin, E. (2021). Antifouling technology trends in marine environmental protection. J. Bionic Eng. 18, 239–263. doi: 10.1007/s42235-021-0017-z
Vasconcellos, S. P., Cereda, M. P., Cagnon, J. R., Foglio, M. A., Rodrigues, R. A., Manfio, G. P., et al. (2009). In vitro degradation of linamarin by microorganisms isolated from cassava wastewater treatment lagoons. Braz. J. Microbiol. 40, 879–883. doi: 10.1590/S1517-838220090004000019
Vinoj, G., Vaseeharan, B., Thomas, S., Spiers, A. J., and Shanthi, S. (2014). Quorum quenching activity of the AHL-lactonase from Bacillus licheniformis DAHB1 inhibits Vibrio biofilm formation in vitro and reduces shrimp intestinal colonization and mortality. Mar. Biotechnol. 16, 707–715. doi: 10.1007/s10126-014-9585-9
Wang, K.-L., Wu, Z.-H., Wang, Y., Wang, C.-Y., and Xu, Y. (2017). Mini-Review: antifouling natural products from marine microorganisms and their synthetic analogs. Mar. Drugs 15:266. doi: 10.3390/md15090266
Xie, C., Guo, H., Zhao, W., and Zhang, L. (2020). Environmentally friendly marine antifouling coating based on a synergistic strategy. Langmuir 36, 2396–2402. doi: 10.1021/acs.langmuir.9b03764
Xin, X., Huang, G., Zhou, X., Sun, W., Jin, C., Jiang, W., et al. (2016). Potential anti-fouling compounds with antidiatom adhesion activities from the sponge-associated bacteria, Bacillus pumilus. J. Adhes. Sci. Technol. 31, 1028–1043. doi: 10.1080/01694243.2016.1242219
Keywords: Bacillus licheniformis, anti-fouling, natural products, micro-fouling, macro-fouling, biofilm inhibition, bacterial extracts
Citation: Muras A, Larroze S, Mayer C, Teixeira T, Wengier R, Benayahu Y and Otero A (2021) Evaluation of the Anti-fouling Efficacy of Bacillus licheniformis Extracts Under Environmental and Natural Conditions. Front. Mar. Sci. 8:711108. doi: 10.3389/fmars.2021.711108
Received: 17 May 2021; Accepted: 12 July 2021;
Published: 13 August 2021.
Edited by:
Carla C. C. R. de Carvalho, Universidade de Lisboa, PortugalReviewed by:
Jorge T. Antunes, University of Porto, PortugalSteve Flint, Massey University, New Zealand
Copyright © 2021 Muras, Larroze, Mayer, Teixeira, Wengier, Benayahu and Otero. This is an open-access article distributed under the terms of the Creative Commons Attribution License (CC BY). The use, distribution or reproduction in other forums is permitted, provided the original author(s) and the copyright owner(s) are credited and that the original publication in this journal is cited, in accordance with accepted academic practice. No use, distribution or reproduction is permitted which does not comply with these terms.
*Correspondence: Ana Otero, anamaria.otero@usc.es
†Present address: Celia Mayer, Instituto de Investigación Sanitaria de Santiago de Compostela (IDIS), Santiago de Compostela, Spain; National Biofilms Innovations Centre, Biodiscovery Institute and School of Life Sciences, University of Nottingham, Nottingham, United Kingdom