- 1State-Province Joint Engineering Laboratory of Marine Bioproducts and Technology, College of Ocean and Earth Sciences, Xiamen University, Xiamen, China
- 2Fujian Provincial Key Laboratory of Ophthalmology and Visual Science, School of Medicine, Xiamen University, Xiamen, China
Endocannabinoids play important roles in the functioning of various physiological systems in humans and non-mammalian animals, including invertebrates. However, information concerning their roles in physiological functions in members of the phylum Mollusca is scarce. Here the hypothesis that the endocannabinoids are involved in mediating settlement of marine invertebrates was tested. Two endocannabinoids [N-arachidonoyl ethanolamide (AEA) and 2-arachidonoyl glycerol (2-AG)], and two endocannabinoid-like lipids [N-Oleoylethanolamide (OEA) and N-Palmitoylethanolamide (PEA)] were detected in the green mussel Perna viridis. In particular, 2-AG was present at significantly higher levels in unattached P. viridis compared with attached mussels. The in vivo level of 2-AG was inversely correlated with the attachment activity of P. viridis. Furthermore, exposure to synthetic 2-AG inhibited attachment of P. viridis in a reversible manner. Transcriptomic analysis suggested that up-regulation of 2-AG synthase (Phospholipase C-β, PLC-β) and down-regulation of its degrading enzyme (Monoacylglycerol lipase, MAGL) resulted in higher levels of 2-AG in unattached mussels. A putative mechanism for the negative regulation of mussel attachment by 2-AG is proposed that involves a Ca2+- Nitric oxide (NO)- cyclic guanosine monophosphate (cGMP) pathway. This study broadens our understanding of the evolution and roles of the endocannabinoid system in animals, and reveals an endogenous regulatory cue for mussel attachment.
Introduction
The plant Cannabis sativa has been used by humans for centuries, and is currently the most widely used illicit drug in many countries (Hall et al., 1999; Salhab, 2017). Its use is controversial due to its psychological effects on humans (Bloomfield et al., 2011; Bridgeman and Abazia, 2017). The major psychoactive component of cannabis is Δ9-tetrahydrocannabinol (THC) (Gaoni and Mechoulam, 1964; Wachtel et al., 2002). Studies on the mechanism of action of THC led to the discovery of cannabinoid receptors (Matsuda et al., 1990; Munro et al., 1993; Howlett et al., 2002), which in turn led to the discovery of natural endogenous cannabinoids (Devane et al., 1992; Mechoulam et al., 1995; Mechoulam and Hanuš, 2000). The two most well-studied endocannabinoids are the lipids, N-arachidonoyl ethanolamine (AEA or anandamide) and 2-arachidonoylglycerol (2-AG). The endocannabinoid system has been found to play important roles in the functioning of various physiological systems in humans including the nervous system, the immune system and the cardiovascular system (Montecucco and Marzo, 2012; Cabral et al., 2015; Zou and Kumar, 2018). Interestingly, the endocannabinoid system is also present in non-mammalian animals, including invertebrates (Salzet and Stefano, 2002; McPartland et al., 2006; Silver, 2019; Clarke et al., 2021). This system also occurs in Hydra (De Petrocellis et al., 1999), the earliest animal described to date to have evolved a neural network. Many other invertebrates such as the sea urchin Paracentrotus lividus, the mollusc Mytilus galloprovincialis, and the annelid Hirudo medicinalis were also found to contain endocannabinoids (Thalheim, 1997; Sepe et al., 1998; Matias et al., 2001).
Endocannabinoids are thought to play several roles in invertebrates, including control of reproduction, control of feeding behavior, and neuroimmune modulation (Salzet and Stefano, 2002; Silver, 2019; Clarke et al., 2021). For benthic invertebrates like hydra and mussels, attachment is a critical stage in their natural lifestyle that is important for successful reproduction and dispersal to new habitats. However, the molecular mechanisms that regulate attachment in benthic invertebrates are poorly understood. Investigations into the physiological role of endocannabinoids in invertebrates may provide insights into the functions of small endogenous molecules and lead to a deeper understanding of the evolutionary origins of endocannabinoid systems in higher vertebrates and mammals (Salzet and Stefano, 2002).
Mussels are an important group of bivalves from both ecological and economic perspectives. Mussels such as the blue mussel, Mytilus edulis, are an important food resource. Mussel beds, formed by dense aggregations of mussels can be very large and are found in intertidal zones, at deep-sea hydrothermal vents, and at cold seeps (Commito et al., 2008, 2018; Turnipseed et al., 2010). Mussel beds also provide an important habitat for other organisms and have been recognized as ecosystem engineers (Commito et al., 2008, 2018). In contrast, some invasive mussel species such as the zebra mussel Dreissena polymorpha have negative impacts on aquatic ecosystems (Karatayev et al., 2015). Mussels are one of the most problematic macrofoulers. They can settle on submerged artificial surfaces such as ship hulls by producing proteinaceous byssal threads. Mussel fouling is particularly troublesome when it is responsible for clogging water pipelines in power plants and water treatment plants (Hosler, 2011; Prescott et al., 2013). To address this serious issue, current antifouling technology commonly uses toxicants that can have adverse effects on the marine environment.
In the search for antifoulants with reduced environmental damage, Angarano et al. (2009) found that exposure to synthetic AEA inhibited zebra mussel byssal attachment in a non-toxic manner. AEA and endocannabinoid-like compounds such as PEA (an endogenous lipid known to mimic several effects of endocannabinoids) have previously been detected in lipid extracts of the mussel M. galloprovincialis (Sepe et al., 1998). It has been speculated that exogenous AEA and 2-AG may stimulate nitric oxide (NO) release from immunocytes of M. edulis tissues (Stefano et al., 1996, 2000). However, whether the endocannabinoids naturally present in the mussels are involved in the mediation of byssal attachment is unknown. Based on the previous findings of Sepe et al. (1998) and Angarano et al. (2009), it is reasonable to hypothesize that the endocannabinoids may play a role in regulation of mussel attachment.
The green mussel Perna viridis is widely distributed as a native species in the Asia-Pacific and Indo-Pacific waters and is an invasive species in Australia, the Caribbean, North America and South America (Benson, 2001; Baker et al., 2007; Stafford et al., 2007; Donaghy and Volety, 2011). This species is a valuable source of seafood in Asia. It is also notorious for fouling power plant pipes, ship hulls, and other submerged artificial structures in large clusters and is used as a model species in antifouling research (Benson, 2001; Yaqin et al., 2014; Basu et al., 2020).
In the natural environment, mussel attachment (which is achieved by byssus threads) can be broken by storms and other physical disturbances (Lee et al., 1990). After detaching from their substratum, the juvenile and adult mussels tend to crawl across surfaces using their foot and reattach to new locations by producing new byssus threads (Kavouras and Maki, 2003; Rajagopal et al., 2005; South et al., 2021). During preliminary studies, we noticed that when attached green mussels of P. viridis were cut from their byssus and subsequently cultured in seawater for 24 h, most mussels would reattach by producing new byssus, but there were some individuals that did not secrete any byssus threads and remained unattached. This led to the hypothesis that there may be naturally occurring substance(s) in mussels that mediate the reattachment process and that these mediator(s) may be present in different amounts in the reattached mussels compared to the unattached mussels. We further hypothesized that endocannabinoids (or endocannabinoid-like lipids) could function as such mediator(s) in P. viridis. The aims of this study were to test our hypotheses, to improve our understanding of the molecular mechanism underlying mussel attachment, and to provide underpinning knowledge which may be useful in developing environmentally friendly methods for the control of mussel fouling. We measured the levels of endocannabinoids and endocannabinoid-like compounds in the reattached and unattached P. viridis, examined the change in the content of 2-AG in P. viridis during its attachment, and investigated the effect of exogenous 2-AG on mussel attachment. Furthermore, comparative transcriptomic analysis of attached and unattached mussels was performed to provide possible clues to the regulation mechanism of 2-AG on mussel attachment.
Materials and Methods
Mussels
Specimens of the green mussel P. viridis (shell length, 2.1 ± 0.3 cm) were collected from submerged ropes on a fish farm near Dadeng Island, Fujian Province, P.R. China (24°34′N, 118°22′E), during March 2017. In the laboratory, the mussels were held without food in an aquarium with aerated natural seawater (collected from the cost of Xiamen, Fujian Province, P.R. China) for 1–2 days prior to use for assays.
Analysis of Endocannabinoids and Endocannabinoid-Like Lipids in Attached and Unattached P. viridis
Green mussels attached with byssus were chosen and their byssus was gently cut off with sharp scissors. Eight of these mussels were randomly chosen and immediately dissected (referred to as Group I). Their shell free tissues were weighed, frozen in liquid nitrogen and stored at –80°C until use for extraction of endogenous cannabinoids. Meanwhile, the other mussels with removed byssus were placed into 12-well plates, with one mussel in each well containing 5 mL 0.22 μm filtered seawater (FSW). After 24 h, all mussels were still alive. Most mussels attached to the surface of wells by secreting byssal threads. A few mussels did not secrete the byssus and remained unattached. Eight mussels were randomly selected from the attached mussels (referred to as Group Π), and another eight mussels were randomly selected from the unattached mussels (referred to as Group Ш). For each group, there were eight replicates, with one mussel considered as a replicate. The mussels were dissected and their shell-free tissues were weighed, frozen in liquid nitrogen and stored at –80°C until use for extraction. A schematic illustration of the sampling for extraction is shown in Figure 1A.
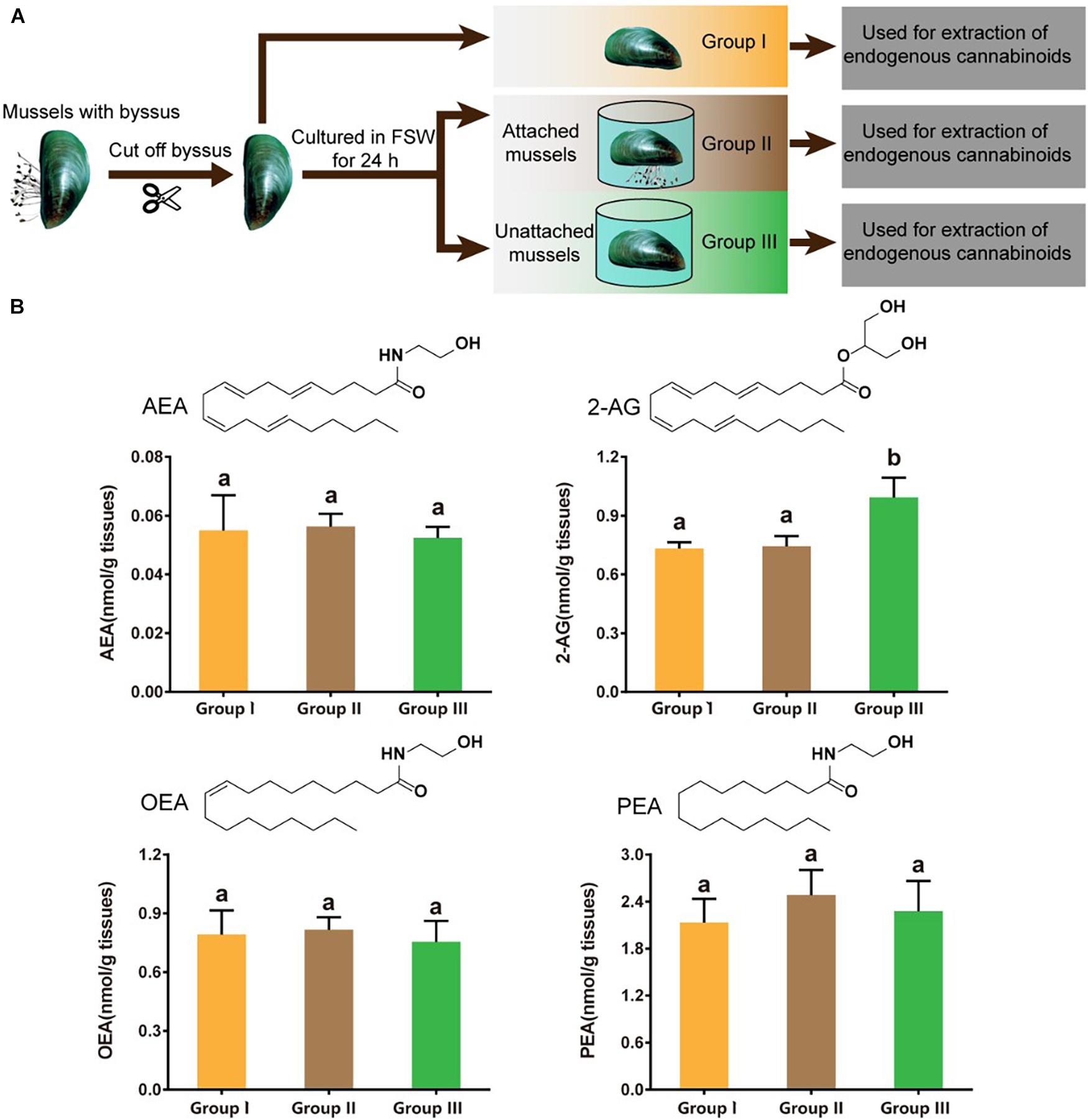
Figure 1. 2-AG is present at significantly higher levels in unattached P. viridis. (A) Schematic illustration of the sampling for extraction of endogenous cannabinoids. (B) Comparison of amounts of AEA, 2-AG, OEA, and PEA in three groups of P. viridis. Group I: Mussels with the byssus removed. Group Π: Mussels that had the byssus removed and that were then incubated in FSW for 24 h and reattached. Group Ш: Mussels with byssus removed, then incubated in FSW for 24 h and which remained unattached. Data shown are means + SE of replicates (n = 8). Different letters above columns indicate significant differences among groups (P < 0.05).
The endocannabinoids and endocannabinoid-like lipids were extracted from mussel tissue and analyzed with liquid chromatography–mass spectrometry (LC-MS/MS) by the procedure of Yang et al. (2015) with minor modifications. Briefly, each batch of shell-free mussel tissue from the groups I, II, and III was homogenized, and added to a glass tube. Then, 1 mL solution with 1 nmol heptadecanoyl ethanolamide (C17:1 FAE, as internal standard) in methanol and 1 mL double distilled H2O were added into each tube, which was subsequently vortexed for 10 s. Then 4 mL chloroform was added into each tube and the tubes were vortexed again for 2 min. After centrifugation at 1,000 × g for 10 min at 4°C, the lipid layer was concentrated by nitrogen flow and subjected to column chromatography using silica gel eluted with the mixed solvent chloroform/methanol (9: 1, V/V). The eluate was dried by nitrogen flow and reconstituted into 0.1 mL chloroform/methanol mixture (1: 3, V/V), and 15 μL of the solution was used for LC-MS/MS.
The analyses were conducted using an Agilent 1100 G1316A HPLC system (Agilent, Shanghai, China) coupled with Mass Spectrometry 3200 Q TRAP (Applied Biosystems, Foster City, CA, United States). An Agilent Eclipse C18 (250 mm × 4.6 mm, 5 μm) column was used and the column temperature was kept at 30°C. The mobile phase for HPLC analysis consisted of methanol and ultra-pure water. The gradient elution, at a flow rate of 1 mL/min, was as follows: 85% methanol for the first 3 min, followed by a linear gradient from 85 to 100% methanol for 2 min and then 100% methanol for 10 min. The elution condition was finally returned to 85% methanol before the next run. The mass spectra were acquired by ion detection at APCI + -MRM mode. Here two endocannabinoids AEA and 2-AG, and two endocannabinoid-like lipids PEA and OEA were analyzed. The molecular ions were monitored at m/z 300.20/62.00 for PEA, m/z 326.10/62.00 for OEA, m/z 348.00/62.00 for AEA, m/z 379.10/287.10 for 2-AG, and m/z 313.10/62.00 for C17:1 FAE.
Change in the Content of 2-AG in P. viridis During Its Attachment
Since the amount of 2-AG was significantly different between the attached and unattached mussels 24 h after having the byssus removed, to further understand how 2-AG is involved in the attachment of P. viridis, the change in 2-AG content in mussels over a 24 h period of incubation was measured. Six hundred green mussels were detached by cutting the byssus threads and immediately put into 12-well plates, with one mussel in each well containing 5 mL FSW. These were separated into two groups (A and B, each group with 300 mussels), with group A for observing the reattachment of mussels, and the group B for measuring the change in 2-AG content in mussels during reattachment. During the 24 h incubation period, for group A, the number of the mussels that had reattached was counted at 0.167, 0.5, 1, 1.5, 2, 4, 6, 12, and 24 h after being put into the wells. Three replicates were set up, with each replicate including 100 mussels. The new attachment rate of the mussels at each time point was calculated by formula (1). At each time point, for group B, three mussels (with one mussel as a replicate) were randomly selected, frozen in liquid nitrogen and stored at −80°C until used for extraction of 2-AG. The extraction and LC-MS/MS analysis of 2-AG were performed as described above.
where Rt: the new attachment rate of mussels at certain time point; At: the number of attached mussels at a certain time point; and t: time points (t0 = 0, t1 = 0.167, t2 = 0.5, t3 = 1, t4 = 1.5, t5 = 2, t6 = 4, t7 = 6, t8 = 12, and t9 = 24 h).
Effect of Exogenous 2-AG on Attachment of P. viridis
To further investigate the effect of 2-AG on attachment of P. viridis, mussels were exposed to synthetic 2-AG and their response observed. This attachment assay was performed following the method described in Angarano et al. (2009) with minor modification. The tested mussels were detached by removing their byssus. The 2-AG (Sigma-Aldrich, United States) was dissolved in dimethyl-sulfoxide (DMSO). A total of 25 μL 2-AG solution and 4.975 mL FSW were added into each well (12-well plates), after which one mussel was put into each well. The final test concentrations of 2-AG were 10, 20, 50, and 100 μM. The FSW control and the solvent control (0.5% DMSO in FSW, V/V) were used in this experiment. There were three replicates for each treatment. Each replicate included 12 mussels, with one mussel in one well of a 12-well plate. After a 24 h exposure period, the numbers of mussels attached, or alive but unattached were counted. To test whether 2-AG had a residual effect on P. viridis, after the above 24 h exposure period, the attached mussels were gently detached by cutting off the byssus threads with sharp scissors, and washed with FSW. The living but unattached mussels were also washed with FSW. Then, these mussels were put into new 12-well plates, with each well containing 5 mL FSW and one mussel. After 24 h of the post-exposure incubation, the numbers of mussels attached or alive but unattached were counted. The number of byssus threads produced by each mussel was also counted (Winkle, 1970; Rajagopal et al., 2003). No dead mussels were observed in this bioassay. The viability of mussels was tested following Matthews and McMahon (1999).
RNA Extraction and Transcriptome Sequencing
To investigate the events that occur upon detachment, we sequenced transcripts of P. viridis under two states: individuals of P. viridis that attached at 24 h after being detached by removing their byssus threads (AT group), and mussels that remained unattached at 24 h after being detached by removing their byssus threads (UAT group). Each group had three replicates, each replicate containing RNA from three mussels. Total RNA was extracted from shell-free tissues of mussels from the AT and UAT groups using TRIzol® Reagent (Invitrogen, Carlsbad, CA, United States) following the manufacturer’s instructions. The purity of the RNA was checked using a NanoDrop 2,000 spectrophotometer (Thermo-Fisher Scientific, Waltham, MA, United States). The concentration of RNA was then measured using Qubit® RNA Assay Kit by a Qubit® 2.0 Fluorometer (Life Technologies, Carlsbad, CA, United States). The integrity of RNA was assessed using the RNA Nano 6000 Assay Kit of the Agilent Bioanalyzer 2,100 system (Agilent Technologies, Santa Clara, CA, United States). The cDNA library construction and transcriptome sequencing were performed by Novogene Bioinformatics Technology Co., Ltd. (Beijing, China). Sequencing libraries were generated using NEBNext® UltraTM RNA Library Prep Kit for Illumina® (New England Biolabs, Ipswich, MA, United States) following the manufacturer’s instructions. Sequencing was carried out using an Illumina Hiseq 4000 platform.
Transcriptome Assembly and Annotation
Raw reads generated using the Illumina Hiseq 4000 platform were filtered to remove adaptors, reads with unknown bases (N) > 10%, and low-quality reads (those consisting of more than 50% bases whose quality scores were ≤ 20) to achieve clean reads. The clean reads were used for de novo assembly of the transcriptome using Trinity (Grabherr et al., 2011). All of the unigenes were annotated against several databases: Nr (NCBI non-redundant protein sequences) and Swiss-Prot using diamond v0.8.22 with e-value = 1e-5 (Buchfink et al., 2015); KOG (Eukaryotic Orthologous Groups) with e-value = 1e-3; Nt (NCBI non-redundant nucleotide sequences) using NCBI blast v2.2.28 + with e-value = 1e-5 (Altschul et al., 1997); KO (KEGG Ortholog database) using KAAS r140224 with e-value = 1e-10 (Moriya et al., 2007); and Gene Ontology (GO) using Blast2GO with e-value = 1e-6 (Stefan et al., 2008).
Analysis of Differentially Expressed Genes (DEGs)
The clean reads were mapped to unigenes using RSEM (Li and Dewey, 2011), and the expression levels of unigenes were calculated using the Fragments Per Kilobase per Million reads (FPKM) method (Trapnell et al., 2010). DEGs between the AT and UAT groups were identified using DESeq v1.10.1 (Anders and Huber, 2010). Genes with p-value ≤ 0.05 and the absolute value of log2 (fold change) ≥ 1 were considered differentially expressed. GO enrichment analysis of DEGs was performed using GOseq v1.10.0 (Young et al., 2010). Kyoto Encyclopedia of Genes and Genomes (KEGG) pathway analysis of DEGs was performed using KOBAS v2.0.12 (Mao et al., 2005). GO terms and KEGG pathways with p-value ≤ 0.05 were considered to be significantly enriched.
Validation of Differentially Expressed Genes by Quantitative Real-Time PCR (qRT-PCR)
To confirm the transcriptome results, qRT-PCR analysis was performed for six unigenes (Supplementary Table 1). FP1V-1, FP1V-2, and FP4V-1 are foot proteins involved in mussel attachment. PLC-β and MAGL are important enzymes in metabolism of 2-AG. PKA is involved in the retrograde endocannabinoid signaling pathway. To generate single-stranded cDNA, 1 μg of total RNA sample was reverse-transcribed using the PrimeScriptTM RT reagent Kit (TaKaRa, Shanghai, China). qRT-PCR was performed on the Applied Biosystems 7500 Real-Time PCR System (Applied Biosystems, Foster City, CA, United States) with a FastStart Universal SYBR® Green Master Kit (Roche, Mannheim, Germany) following the manufacturer’s instructions. The qRT-PCR program was as follows: one cycle of 95°C for 5 min; and 40 cycles of 95°C for 20 s, 58°C for 20 s, and 72°C for 30 s; followed by a melting curve analysis. The qRT-PCR reactions were performed in triplicate for each gene in each sample and there were three biological replicates. The relative gene expression levels were calculated by the 2–△△CT method and normalized to the expression of 18S rRNA. The gene-specific primers used in the qRT-PCR were designed using Primer premier v5.0 (Supplementary Table 1).
Statistical Analysis
The results were analyzed with SPSS 18.0 software. In the assay measuring the levels of endocannabinoids and endocannabinoid-like lipids in mussels and the assay testing exogenous 2-AG on mussel attachment, one-way analysis of variance (ANOVA) was performed with Tukey’s post hoc test for analysis of significant differences between groups or treatments. In the qRT-PCR assay, Student’s t-test was used to evaluate the statistical significance between two data sets. The concentration of 2-AG that inhibited attachment by 50% relative to the control (EC50) was calculated using GraphPad Prism software, Version 6.01.
Results
AEA, 2-AG, OEA, and PEA in Attached and Unattached P. viridis
AEA, 2-AG, OEA, and PEA were all detected in P. viridis, with PEA being most abundant (Figure 1B). In the mussels of group I that had just had their byssus threads cut off, AEA, 2-AG, OEA, and PEA were present at the following concentrations (means ± SE): 0.055 ± 0.012, 0.732 ± 0.032, 0.773 ± 0.122, and 2.131 ± 0.304 nmol/g, respectively, indicating the background levels of these lipids in normal P. viridis. At 24 h after removing the byssus, most mussels reattached by secreting new byssus threads and these mussels (group Π) showed similar amounts of these four compounds as in the mussels of group I. However, in the mussels that remained unattached 24 h after their byssus was removed (group Ш), the amount of 2-AG was significantly higher than in the groups I (P = 0.032) and Π (P = 0.042), although for AEA, OEA, and PEA, there was no significant difference between these three groups (Figure 1B). This suggests that 2-AG may play a role in mediating mussel attachment.
2-AG Levels in P. viridis Changed During Attachment
As shown in Figure 2, during 1 h after the byssus was removed, there was an increase in reattachment activity, and the amount of 2-AG decreased. The highest new attachment rate was found at 1 h. It is noteworthy that the lowest level of 2-AG was also found at 1 h. For the 1–2 h period, the reattachment activity decreased, but the amount of 2-AG increased. At 2 h, 79.0% of the mussels had reattached, and following this the reattachment activity remained low. Correspondingly, 2-AG levels remained stable level after 2 h. The finding that the amount of 2-AG decreased when P. viridis was active at attachment was consistent with the finding that attached P. viridis contained a lower level of 2-AG compared to unattached organisms (Figure 1B).
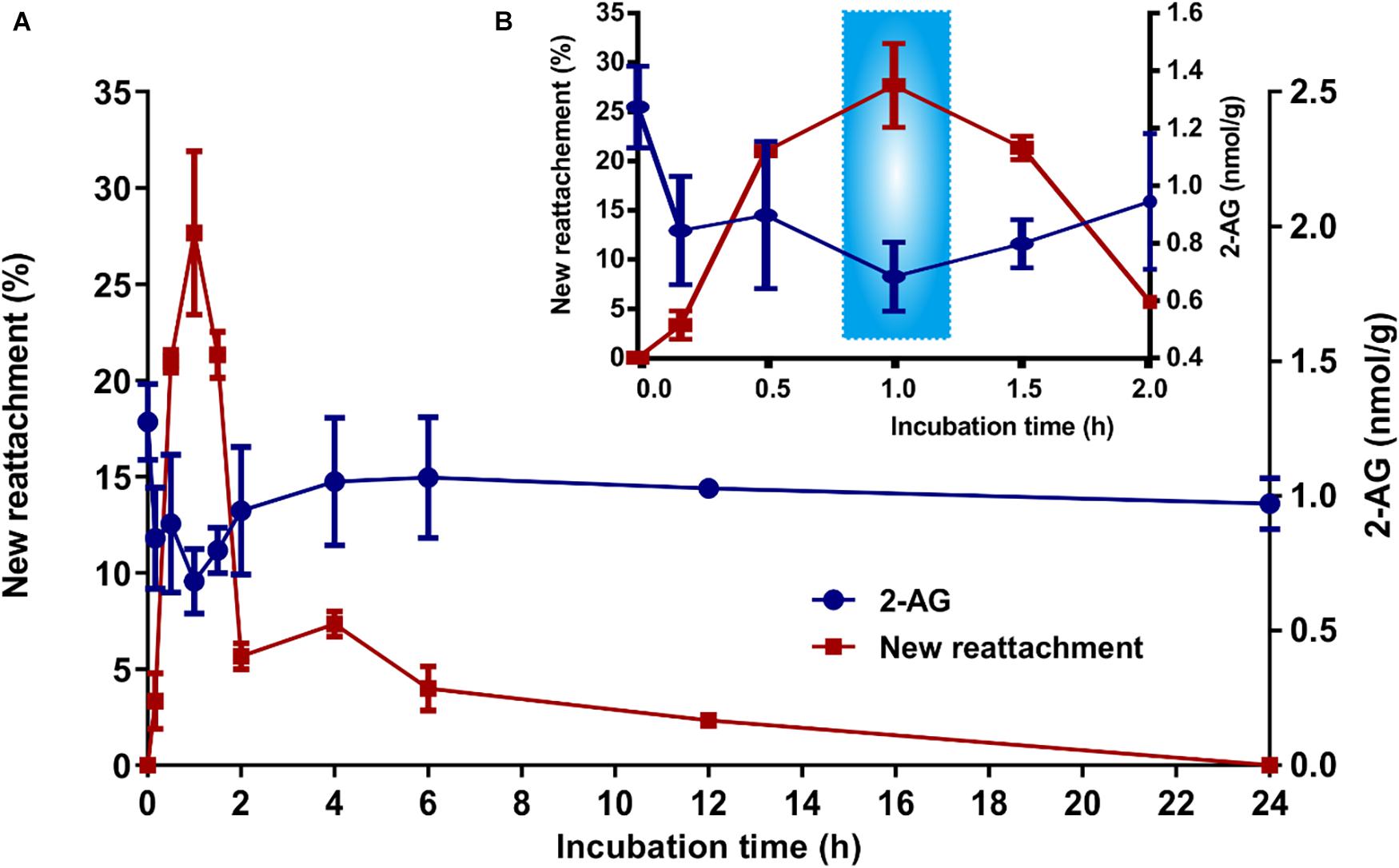
Figure 2. Changes in the content of 2-AG in P. viridis during attachment. The level of 2-AG in P. viridis is inversely correlated with reattachment activity. (A) Changes in P. viridis reattachment and content of 2-AG during the first 24 h of incubation after removal of the byssus. (B) The enlarged view during the first 2 h. Data shown are means ± SE of replicates (n = 3).
Effect of Exogenous 2-AG on Attachment of P. viridis
Attachment of P. viridis was significantly inhibited in the presence of exogenous 2-AG at a concentration of 100 μM when compared to the solvent control (P = 0.003, Figure 3A). The EC50 value of 2-AG for mussel attachment was 80.67 μM. Although there was no significant difference in attachment percentage between the treatment of 50 μM 2-AG and the solvent control (P > 0.5), the byssus thread production of P. viridis decreased significantly in the treatment of 50 μM 2-AG when compared with the solvent control (P = 0.004, Figure 3B), indicating an inhibitory effect on byssus thread production at this concentration. The EC50 value of 2-AG for byssus thread production was 46.29 μM. Exposure to 2-AG had no obvious residual effect on mussel attachment, because when 2-AG was removed, almost all mussels in each treatment group attached during the 24 h post-exposure period, with the percentage of attached mussels not significantly different from the control (Figure 3C). A similar effect was observed for byssus thread production (Figure 3D). This suggests that 2-AG inhibits attachment and byssus thread production in P. viridis in a non-toxic manner (which is consistent with the observation that there was no mussel mortality over both the exposure and post-exposure periods in all treatments), and that this inhibitory effect is reversible.
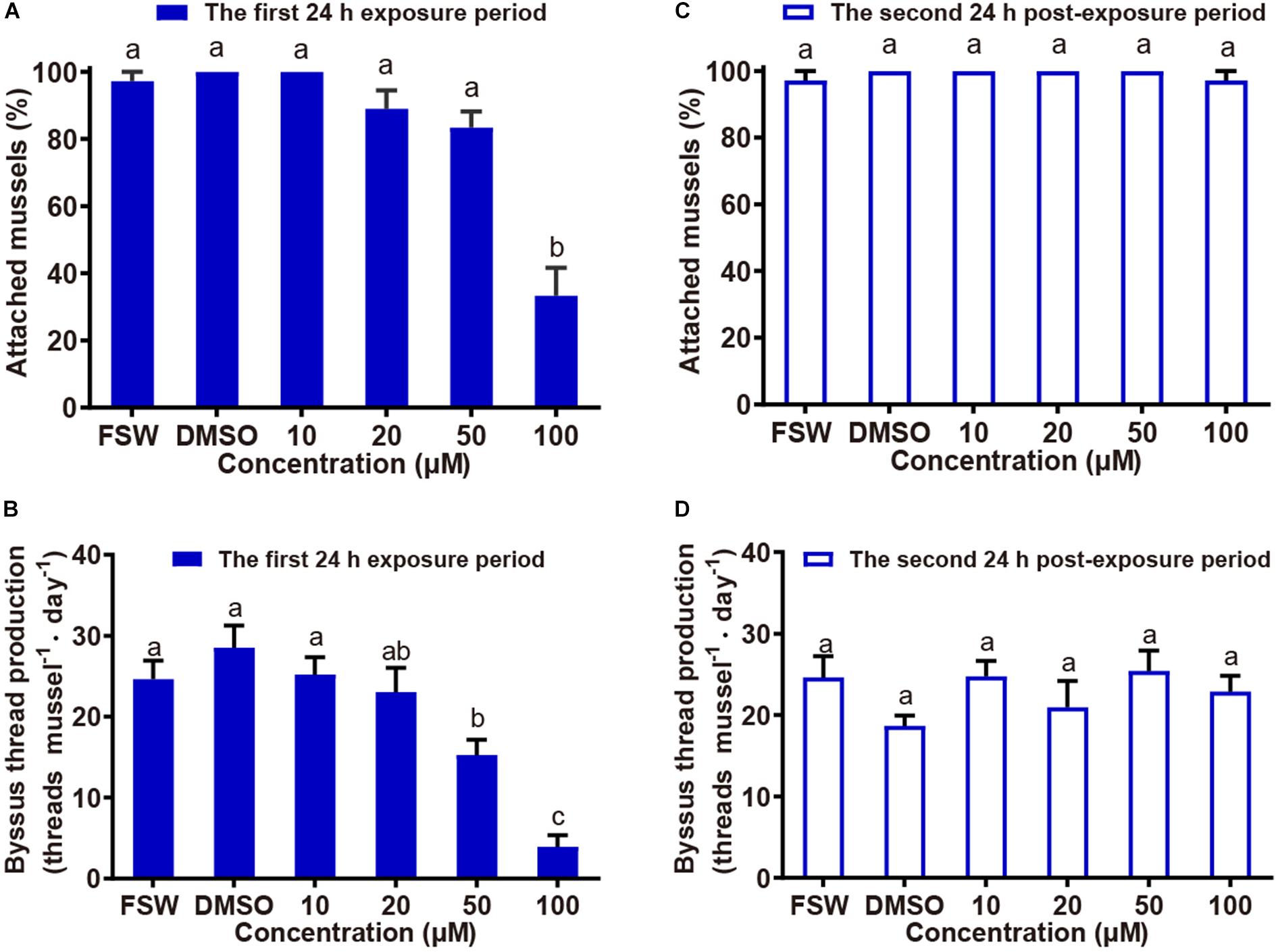
Figure 3. Effect of exogenous 2-AG on attachment of P. viridis. Attachment and byssus thread production of mussels following exposure to 2-AG for a 24 h period (exposure effect) and in the absence of 2-AG in the subsequent 24 h post-exposure period (residual effect). FSW: The FSW control. DMSO: The solvent only control (0.5% DMSO in FSW). Different letters above columns indicate significant differences (P < 0.05). Data shown are means + SE of replicates (n = 3 for attachment percentage in (A,C); n = 36 for the number of byssus threads in (B,D).
Transcriptomic Analysis of Attached and Unattached P. viridis
The results of transcriptome sequencing and assembly are shown in Supplementary Tables 2, 3. All of the RNA-seq data have been deposited in the NCBI Short Read Archive (SRA) under accession number PRJNA601624. For functional annotation, all unigenes were annotated against databases including Nr, Swiss-Prot, Nt, KOG, KO and GO, with 68,446 unigenes annotated (Supplementary Figure 1). Specifically, the genes involved in endocannabinoid signaling were found to be present in P. viridis (Table 1). The genes encoding enzymes for 2-AG synthesis (PLC-β and diacylglycerol lipase, DAGL) and degradation (MAGL), enzymes for AEA, OEA, and PEA synthesis (N-acyl phosphatidylethanolamine phospholipase D, NAPE-PLD) and their degradation (Fatty acid amide hydrolase, FAAH and N-Acylethanolamine acid amidase, NAAA) were annotated here. The genes for proteins involved in the retrograde endocannabinoid signaling pathway, including metabotropic glutamate receptors (mGluRs), α-amino-3-hydroxy-5-methyl-4-isoxazolepropionic acid receptor (also known as AMPA receptor, AMPAR), Voltage-gated calcium channels (VGCC), γ-Aminobutyric acid type A (GABAA), Mitogen-activated protein kinase (MAPK), Protein kinase A (PKA), and Protein kinase C (PKC) were also annotated. Although the expression of two well-known vertebrate cannabinoid receptors 1 and 2 (CB1 and CB2, two G-protein-coupled receptors) was not found in the present transcriptomic data of P. viridis, the genes for TRPV1 and PPARα, which can also interact with endocannabinoid or endocannabinoid-like compounds, and the gene for CNRIP1 (or CRIP1), which is a regulator of CB1 signaling, were found among the annotated genes (Table 1). These findings, combined with the above discovery of the presence of endocannabinoids in P. viridis, indicate the presence of an endocannabinoid system in P. viridis.
In total, 3,380 differentially expressed genes (DEGs) were identified between attached and unattached P. viridis, including 1,637 up-regulated DEGs and 1,743 down-regulated DEGs in the unattached group compared with the attached group (Figures 4A,B). The results of GO and KEGG enrichment analyses of DEGs are shown in Supplementary Figure 2. Particular attention was paid to DEGs between attached and unattached P. viridis that were related to the metabolism and action of 2-AG (Table 1). As shown in Table 1, the expression level of PLC-β which is involved in synthesis of 2-AG, was significantly up-regulated (P = 0.001178), and the transcription level of MAGL which is involved in degradation of 2-AG, was down-regulated (P = 0.077479). This indicates a possible mechanism for why unattached mussels had higher levels of 2-AG than attached mussels. Furthermore, it was noteworthy that there were three DEGs (mGluRs, AMPAR, and VGCC) enriched in the pathway of retrograde endocannabinoid signaling in the unattached P. viridis (Table 1).
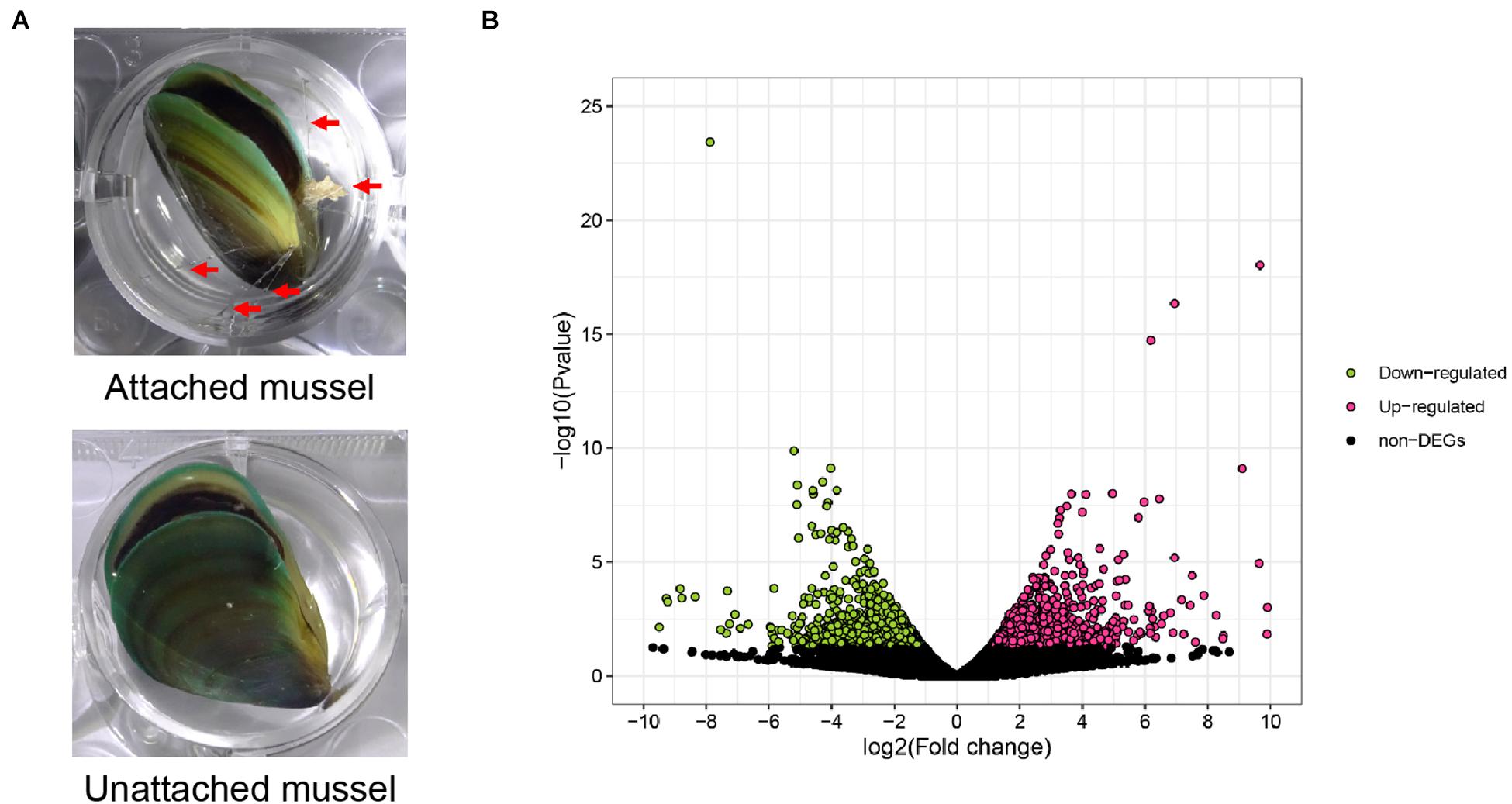
Figure 4. Transcriptomic analysis of attached and unattached P. viridis. (A) The mussels attached 24 h after byssus thread removal (AT group for transcriptome analysis) and the mussels unattached 24 h after byssus thread removal (UAT group for transcriptome). Red arrows indicate byssus attachment sites. (B) Volcano plots of differentially expressed genes between AT and UAT mussels. Red and green spots indicate the up-regulated expressed genes and the down-regulated expressed genes between the AT and UAT groups, respectively. Black spots indicate genes that were not differentially expressed.
Validation of the transcriptome data using qRT-PCR was performed with six unigenes [FP1V-1 (Foot protein 1 variant), FP1V-2, FP4V-1, MAGL, PLC-β, and PKA]. As shown in Supplementary Figure 3, the transcription profiles of all six selected unigenes as determined by qRT-PCR were consistent with those determined by RNA-seq, confirming the reliability and reproducibility of the transcriptome data.
Discussion
Mussel attachment is critical for the formation of mussel beds, thus underpinning the propagation and persistence of mussel population in the marine ecosystem (Commito et al., 2014). Many chemical cues, including exogenous factors (or environmental chemical cues, such as metabolites from conspecifics and bacteria) and endogenous factors (such as catecholamines, serotonin, and acetylcholine) have been suggested to promote mussel attachment (Li et al., 2014; Morello and Yund, 2016; Joyce and Vogeler, 2018). Most of these studies on endogenous cues used exposure assays with synthetic compounds to confirm the effect of chemical cues on mussel attachment. However, little information is available concerning the relationship between the level of endogenous cues in the mussels and their attachment activity. Here, the strong inverse relationship found between the levels of 2-AG in P. viridis and its attachment behavior suggests that 2-AG may function as a negative regulator of mussel attachment. Interestingly, an inverse correlation between the in vivo level of 2-AG and animal behavior has also been reported in mice (Chevalier et al., 2020), wherein the effect is manifested as a depressive-like behavior.
The understanding of negative cues of mussel attachment is far less advanced than the study of inducing cues. The exposure assay carried out here found that by adding exogenous 2-AG, attachment of P. viridis could be inhibited, demonstrating the negative effect of 2-AG on mussel attachment. Consistent with these findings are the observations that structural analogs of 2-AG, including AEA, linoleyl ethanolamide, and noladin ether, inhibit byssal attachment in a different subclass of mussel (in Dreissena polymorpha) (Angarano et al., 2009), indicating that endocannabinoids might also inhibit attachment in other mussels.
Transcriptomic sequence data indicated the existence of several endocannabinoid synthesizing and degrading enzymes in P. viridis. There are very few studies of endocannabinoid metabolic enzymes in molluscs, only the FAAH-like enzymatic activity has been reported in Mytilus galloprovincialis (Sepe et al., 1998). This study confirmed the presence of the gene for FAAH in molluscs. Furthermore, the present transcriptomic analysis on metabolic enzymes suggests a possible underlying mechanism for the higher level of 2-AG in unattached mussels. Expression of PLC-β was significantly up-regulated in unattached mussels. PLC-β plays a pivotal role in catalyzing the hydrolysis of phosphatidylinositol 4,5-bisphosphate (PIP2) to yield inositol trisphosphate (IP3) and diacylglycerol (DAG) (Kadamur and Ross, 2013). DAG can be further hydrolyzed by DAGL to produce 2-AG (Kano et al., 2009). In other words, PLC-β is important for the synthesis of 2-AG. In contrast, expression of MAGL was down-regulated in unattached mussels (with a P-value of 0.077479, near to 0.05). MAGL is an important enzyme in degradation of 2-AG to arachidonic acid (AA) and glycerol (Lambert et al., 2010; Savinainen et al., 2012). We therefore suggest that the up-regulation of the synthase and down-regulation of the degrading enzyme for 2-AG results in increased concentrations of 2-AG in unattached mussels.
The discovery of the endocannabinoid system in mammals also raises interest in its evolutionary origins (Elphick and Egertova, 2001). The endocannabinoid system is generally suggested to consist of endocannabinoids, enzymes responsible for their synthesis and degradation, and cannabinoid receptors (Buznikov et al., 2010). The endocannabinoids (such as AEA and 2-AG) and their metabolic enzymes have been found in various species of non-mammalian vertebrates and invertebrates, indicating they are phylogenetically widespread (Soderstrom, 2009; Elphick, 2012). For cannabinoid receptors, genes encoding the mammalian CB1/CB2 receptors have been identified in non-mammalian vertebrates (Elphick and Egertová, 2009; Elphick, 2012), the urochordate Ciona intestinalis (Elphick et al., 2003), and the cephalochordate Branchiostoma floridae (Elphick, 2007) (the two invertebrate species are closely related to the vertebrates). However, CB1/CB2 receptor-like genes have not been found in non-chordate invertebrates. Consistent with this, the present transcriptome sequencing of the mussel P. viridis did not reveal any CB1/CB2 genes. Nevertheless, investigations using radiolabeled cannabinoids revealed the presence of specific binding sites for cannabinoids in non-chordate invertebrate species such as the sea urchin Strongylocentrotus purpuratus (Chang et al., 1993), the leech Hirudo medicinalis (Stefano et al., 1997), the mussel M. edulis (Stefano et al., 1996), and the cnidarian Hydra vulgaris (De Petrocellis et al., 1999), indicating the existence of non-CB1/CB2-type cannabinoid receptors. As Elphick and Egertova (2001) suggested, not all cannabinoid receptors are necessarily orthologs of vertebrate CB1/CB2 genes.
Phylogenetic analysis of the relationship of vertebrate CB receptors with other GPCRs suggested that CB receptors, lysophospholipid receptors, melanocortin receptors and adenosine receptors have evolved from a common ancestral gene (Elphick and Egertova, 2001). Yin et al. (2009) found that AEA and 2-AG showed weak agonist activity at the lysophospholipid S1P1 receptor in Mus musculus. Here the finding of the genes for the S1P receptor and adenosine receptor in P. viridis implied the possibility of these receptors as primitive cannabinoid receptors in mussels, although this would need to be verified experimentally. In addition, recent studies have found that besides CB1 and CB2, other proteins including PPARα and TRPV1 can be activated by endocannabinoid or endocannabinoid-like compounds (Muller et al., 2019). PPARα was found to bind with OEA and then activate the downstream transcriptional activity in Mus musculus (Sun et al., 2007). Yuan and Burrell (2013) discovered that by activating TRPV-type receptors, 2-AG mediates retrograde synaptic signaling in the nervous system of the leech H. medicinalis. The present finding of the gene for TRPV1 in P. viridis suggests the possibility of 2-AG binding with TRPV1 to mediate mussel attachment.
It has been reported that endocannabinoids serve as retrograde messengers in vertebrates (Kreitzer and Regehr, 2002; Ohno-Shosaku et al., 2012). Released endocannabinoids can activate the receptors at presynaptic terminals and suppress the release of neurotransmitters by inhibiting Ca2+ channels (Twitchell et al., 1997; Di Marzo, 1998; Diana and Marty, 2004). The CB1 receptor has been reported to be able to suppress Ca2+ influx via VGCC (Zou and Kumar, 2018). Ca2+ has been previously suggested to play an important role in settlement of marine invertebrates, including the barnacle Balanus amphitrite (Clare, 1996), the sea urchin Strongylocentrotus purpuratus (Amador-Cano et al., 2006), and the oyster Ostrea edulis (Smyth et al., 2018). The suppression of Ca2+ influx by receptor activation and the significant down-regulation of VGCC in unattached P. viridis could lower intracellular Ca2+ in unattached P. viridis. Ca2+ regulates NO synthase (Schmidt et al., 1993). Furthermore, significant differences in expression of the NO synthase gene were found between attached and unattached P. viridis (down-regulation in unattached mussels, Table 1). NO synthase can convert L-arginine to NO, a diffusible gaseous signaling molecule in many organisms (Tejero and Stuehr, 2013). It is noteworthy that the NO signaling system has been previously reported to regulate settlement of many marine invertebrates (Bishop and Brandhorst, 2003; Say and Degnan, 2020; Zhu et al., 2020). In the barnacle B. amphitrite and the sponge Amphimedon queenslandica, it was suggested that NO regulates their settlement through cGMP signaling, in which NO activates Guanylate cyclase (GC); GC is converted to Guanosine-5′-triphosphate (GTP) and then to the important second messenger cGMP, which mediates downstream effectors (Lucas et al., 2000; Zhang et al., 2012; Sahoo and Khandeparker, 2018; Say and Degnan, 2020). The NO-cGMP pathway may also play a regulatory role in settlement of P. viridis. Based on the above analysis, one possible explanation for the observed inhibition of attachment is suggested (Figure 5).
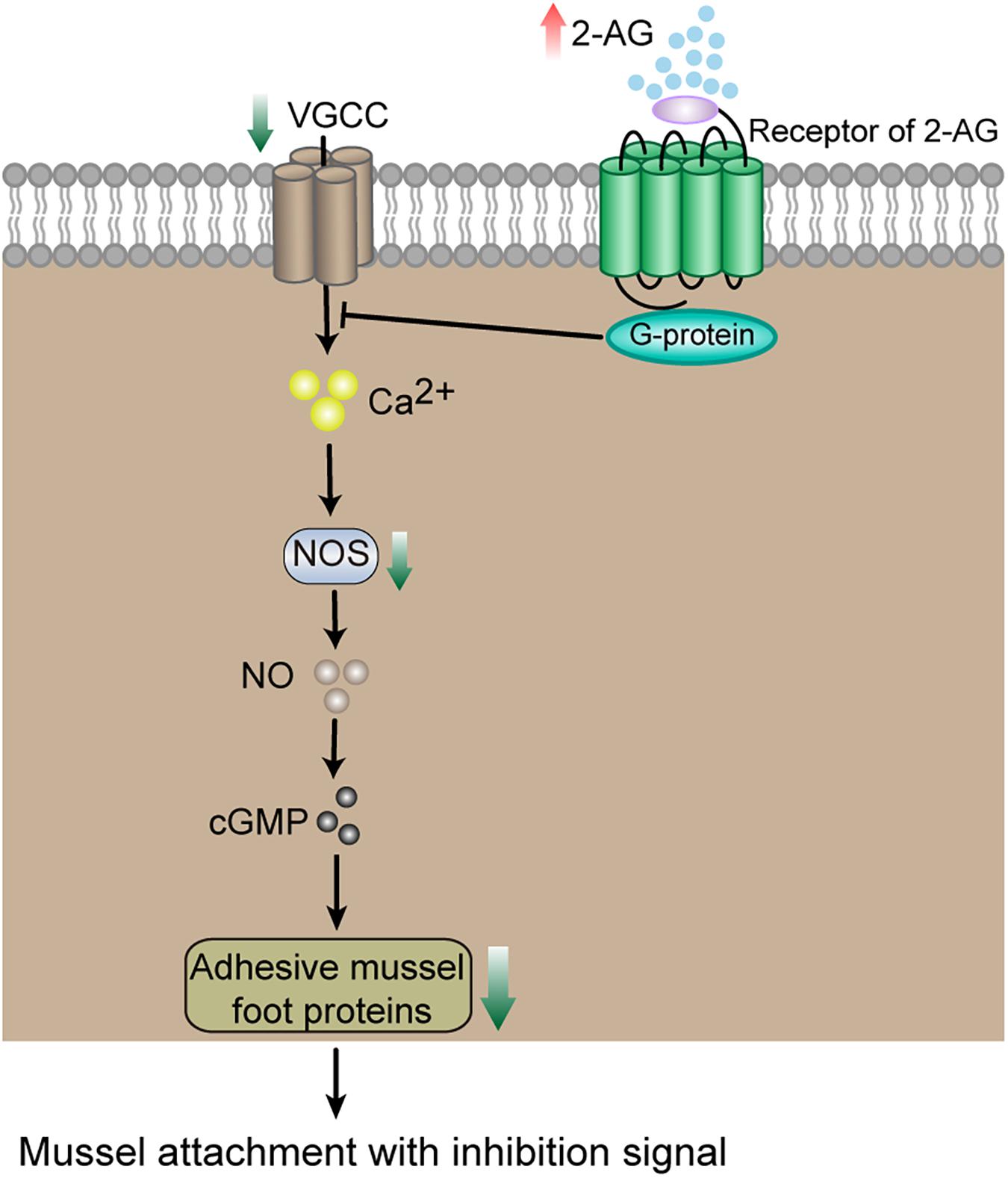
Figure 5. A suggested pathway for the inhibitory effect of 2-AG on mussel attachment based on transcriptomic data. Red arrows indicate higher levels. Green arrows indicate lower levels.
The occurrence of the endocannabinoid system has been suggested in different phyla of animals from Hydra to humans, indicating its fundamental importance to animals (Elphick and Egertova, 2001; Soderstrom, 2009). Investigation of this system in invertebrates may therefore shed light on important questions such as how the endocannabinoid system evolved, the roles of this system are in controlling behavior in non-mammalian animals, and what clues it can provide for the neurophysiology of humans (Salzet and Stefano, 2002). As the review by Elphick (2012) pointed out, there has been relatively little investigation of the endocannabinoid system in molluscs. The present work suggests the existence of a functional endocannabinoid system in P. viridis, which provides a basis for deeper investigation. For example, the question of whether endocannabinods are also involved in regulating settlement in other marine benthic invertebrates.
Conclusion
The present results suggest that the endocannabinoid system might be present in P. viridis and in particular, 2-AG is an endogenous cue for mediating attachment of P. viridis. The up-regulation of the synthase (PLC-β) and down-regulation of the degrading enzyme (MAGL) for 2-AG resulted in a higher level of 2-AG in unattached mussels. A potential mechanism for the negative regulation of 2-AG on mussel attachment via the Ca2+-NO-cGMP pathway is proposed. Our study reveals an endogenous cue for mediating mussel attachment, thereby enhancing our understanding of the attachment mechanism of mussels. The findings herein may also be of relevance for controlled enhancement of settlement, for example in aquaculture of mussels, and for the prevention of attachment, for example in the development of new antifouling strategies.
Data Availability Statement
The datasets presented in this study can be found in online repositories. The names of the repository/repositories and accession number(s) can be found in the article/Supplementary Material.
Author Contributions
QD, Y-QS, and D-QF designed the research. QD, Z-XW, Y-QS, and PS conducted the experiments. Z-WW and YQ performed the data analysis. QD, Z-XW, and D-QF wrote the manuscript. C-HK and D-QF supervised the project and edited the manuscript. All authors contributed to the article and approved the submitted version.
Funding
This research was supported by the Chinese Ministry of Science and Technology through the National Key Research and Development Program of China under Grant 2018YFC1407505, the Youth Innovation Fund Project of Xiamen under Grant 3502Z20206053, and the Key Laboratory of Marine Ecological Monitoring and Restoration Technology, Ministry of Nature Resources under Grant 2020ZX01.
Conflict of Interest
The authors declare that the research was conducted in the absence of any commercial or financial relationships that could be construed as a potential conflict of interest.
Publisher’s Note
All claims expressed in this article are solely those of the authors and do not necessarily represent those of their affiliated organizations, or those of the publisher, the editors and the reviewers. Any product that may be evaluated in this article, or claim that may be made by its manufacturer, is not guaranteed or endorsed by the publisher.
Supplementary Material
The Supplementary Material for this article can be found online at: https://www.frontiersin.org/articles/10.3389/fmars.2021.719781/full#supplementary-material
Abbreviations
2-AG, 2-Arachidonoyl glycerol; AEA, N-arachidonoyl ethanolamide; AMPAR, α-Amino-3-hydroxy-5-methyl-4-isoxazolepropionic acid receptor; cGMP, Cyclic guanosine monophosphate; CNRIP1, Cannabinoid receptor-interacting protein 1; DAG, Diacylglycerol; DEG, Differentially expressed genes; DMSO, Dimethyl sulfoxide; FAAH, Fatty acid amide hydrolase; FP1V, Foot protein 1 variant; FPKM, Fragments Per kb per Million reads; FSW, Filtered seawater; GABAA, γ-Aminobutyric acid type A; GPCR, G Protein-Coupled Receptor; GTP, Guanosine-5 ′ -triphosphate; KEGG, Kyoto Encyclopedia of Genes and Genomes; LC-MS, Liquid chromatography–mass spectrometry; MAGL, Monoacylglycerol lipase; MAPK, Mitogen-activated protein kinase; mGluR, Metabotropic glutamate receptor; NAAA, N-Acylethanolamine Acid Amidase; NAPE-PLD, N-acyl phosphatidylethanolamine phospholipase D; NO, Nitric oxide; PEA, N-Palmitoylethanolamide; PKA, Protein kinase A; PKC, Protein kinase C; PLC- β, Phospholipase C β; PPAR α, Peroxisome proliferator-activated receptor alpha; S1P, Sphingosine 1-phosphate; THC, Δ 9-tetrahydrocannabinol; TRPV1, Transient receptor potential cation channel, subfamily V, member 1; VGCC, Voltage-gated calcium channels.
References
Altschul, S. F., Madden, T. L., Schäffer, A. A., Zhang, J., Zhang, Z., Webb, M., et al. (1997). Gapped BLAST and PSI-BLAST: a new generation of protein database search programs. Nucleic Acids Res. 25, 3389–3402. doi: 10.1093/nar/25.17.3389
Amador-Cano, G., Carpizo-Ituarte, E., and Cristino-Jorge, D. (2006). Role of protein kinase C, G-protein coupled receptors, and calcium flux during metamorphosis of the Sea Urchin Strongylocentrotus purpuratus. Biol. Bull. 210, 121–131. doi: 10.2307/4134601
Anders, S., and Huber, W. (2010). Differential expression analysis for sequence count data. Genome Biol. 11, 106–110.
Angarano, M. B., Mcmahon, R. F., and Schetz, J. A. (2009). Cannabinoids inhibit zebra mussel (Dreissena polymorpha) byssal attachment: a potentially green antifouling technology. Biofouling 25, 127–138. doi: 10.1080/08927010802592743
Baker, P., Fajans, J. S., Arnold, W. S., Ingrao, D. A., Marelli, D. C., and Baker, S. M. (2007). Range and dispersal of a tropical marine invader, the Asian green mussel, Perna viridis, in subtropical waters of the southeastern United States. J. Shellfish. Res. 26, 345–355. doi: 10.2983/0730-8000(2007)26[345:radoat]2.0.co;2
Basu, S., Hanh, B. M., Chua, J. I., Daniel, D., Ismail, M. H., Marchioro, M., et al. (2020). Green biolubricant infused slippery surfaces to combat marine biofouling. J. Colloid. Interface Sci. 568, 185–197. doi: 10.1016/j.jcis.2020.02.049
Benson, A. J. (2001). Establishment of the green mussel, Perna viridis (Linnaeus 1758)(Mollusca: Mytilidae) on the west coast of Florida. J. Shellfish. Res. 20, 21–29.
Bishop, C. D., and Brandhorst, B. P. (2003). On nitric oxide signaling, metamorphosis, and the evolution of biphasic life cycles. Evol. Dev. 5, 542–550. doi: 10.1046/j.1525-142x.2003.03059.x
Bloomfield, M. A. P., Ashok, A. H., Volkow, N. D., and Howes, O. D. (2011). The effects of Δ9-tetrahydrocannabinol on the dopamine system. Nature 539, 369–377.
Bridgeman, M. B., and Abazia, D. T. (2017). Medicinal cannabis: history, pharmacology, and implications for the acute care setting. Pharm. Ther. 42:180.
Buchfink, B., Xie, C., and Huson, D. H. (2015). Fast and sensitive protein alignment using DIAMOND. Nat. Methods 12, 59–60. doi: 10.1038/nmeth.3176
Buznikov, G. A., Nikitina, L. A., Bezuglov, V. V., Francisco, M. E. Y., Boysen, G., Obispo-Peak, I. N., et al. (2010). A putative ‘pre-nervous’ endocannabinoid system in early echinoderm development. Dev. Neurosci. 32, 1–18. doi: 10.1159/000235758
Cabral, G. A., Ferreira, G. A., and Jamerson, M. J. (2015). Endocannabinoids and the immune system in health and disease. Handb. Exp. Pharmacol. 231, 185–211. doi: 10.1007/978-3-319-20825-1_6
Chang, M. C., Berkery, D., Schuel, R., Laychock, S. G., Zimmerman, A. M., Zimmerman, S., et al. (1993). Evidence for a cannabinoid receptor in sea urchin sperm and its role in blockade of the acrosome reaction. Mol. Reprod. Dev. 36, 507–516. doi: 10.1002/mrd.1080360416
Chevalier, G., Siopi, E., Guenin-Macé, L., Pascal, M., Laval, T., Rifflet, A., et al. (2020). Effect of gut microbiota on depressive-like behaviors in mice is mediated by the endocannabinoid system. Nat. Commun. 11, 1–15.
Clare, A. S. (1996). Signal transduction in barnacle settlement: calcium re-visited. Biofouling 10, 141–159. doi: 10.1080/08927019609386276
Clarke, T. L., Johnson, R. L., Simone, J. J., and Carlone, R. L. (2021). The endocannabinoid system and invertebrate neurodevelopment and regeneration. Int. J. Mol. Sci. 22:2103. doi: 10.3390/ijms22042103
Commito, J. A., Commito, A. E., Platt, R. V., Grupe, B. M., Piniak, W. E. D., Gownaris, N. J., et al. (2014). Recruitment facilitation and spatial pattern formation in soft-bottom mussel beds. Ecosphere 5, 1–26. doi: 10.1007/s10152-002-0102-6
Commito, J. A., Como, S., Grupe, B. M., and Dow, W. E. (2008). Species diversity in the soft-bottom intertidal zone: biogenic structure, sediment, and macrofauna across mussel bed spatial scales. J. Exp. Mar. Biol. Ecol. 366, 70–81. doi: 10.1016/j.jembe.2008.07.010
Commito, J. A., Jones, B. R., Jones, M. A., Winders, S. E., and Como, S. (2018). What happens after mussels die? Biogenic legacy effects on community structure and ecosystem processes. J. Exp. Mar. Biol. Ecol. 506, 30–41. doi: 10.1016/j.jembe.2018.05.004
De Petrocellis, L., Melck, D., Bisogno, T., Milone, A., and Di Marzo, V. (1999). Finding of the endocannabinoid signalling system in Hydra, a very primitive organism: possible role in the feeding response. Neuroscience 92, 377–387. doi: 10.1016/s0306-4522(98)00749-0
Devane, W., Hanus, L., Breuer, A., Pertwee, R., Stevenson, L., Griffin, G., et al. (1992). Isolation and structure of a brain constituent that binds to the cannabinoid receptor. Science 258, 1946–1949. doi: 10.1126/science.1470919
Di Marzo, V. (1998). ‘Endocannabinoids’ and other fatty acid derivatives with cannabimimetic properties: biochemistry and possible physiopathological relevance. Biochim. Biophys. Acta 1392, 153–175. doi: 10.1016/s0005-2760(98)00042-3
Diana, M. A., and Marty, A. (2004). Endocannabinoid-mediated short-term synaptic plasticity: depolarization-induced suppression of inhibition (DSI) and depolarization-induced suppression of excitation (DSE). Br. J. Pharmacol. 142, 9–19. doi: 10.1038/sj.bjp.0705726
Donaghy, L., and Volety, A. K. (2011). Functional and metabolic characterization of hemocytes of the green mussel, Perna viridis: in vitro impacts of temperature. Fish. Shellfish. Immunol. 31, 808–814.
Elphick, M. R. (2007). BfCBR: a cannabinoid receptor ortholog in the cephalochordate Branchiostoma floridae (Amphioxus). Gene 399, 65–71. doi: 10.1016/j.gene.2007.04.025
Elphick, M. R. (2012). The evolution and comparative neurobiology of endocannabinoid signalling. Philos. Trans. R. Soc. Lond. B Biol. Sci. 367, 3201–3215. doi: 10.1098/rstb.2011.0394
Elphick, M. R., and Egertova, M. (2001). The neurobiology and evolution of cannabinoid signalling. Philos. Trans. R. Soc. Lond. B Biol. Sci. 356, 381–408. doi: 10.1098/rstb.2000.0787
Elphick, M. R., and Egertová, M. (2009). “Cannabinoid receptor genetics and evolution,” in The Cannabinoid Receptors, ed. P. H. Reggio (New York: Humana Press), 123–149. doi: 10.1007/978-1-59745-503-9_5
Elphick, M. R., Satou, Y., and Satoh, N. (2003). The invertebrate ancestry of endocannabinoid signalling: an orthologue of vertebrate cannabinoid receptors in the urochordate Ciona intestinalis. Gene. 302, 95–101. doi: 10.1016/s0378-1119(02)01094-6
Gaoni, Y., and Mechoulam, R. (1964). Isolation, structure, and partial synthesis of an active constituent of hashish. J. Am. Chem. Soc. 86, 1646–1647. doi: 10.1021/ja01062a046
Grabherr, M. G., Haas, B. J., Yassour, M., Levin, J. Z., Thompson, D. A., Amit, I., et al. (2011). Full-length transcriptome assembly from RNA-Seq data without a reference genome. Nat. Biotechnol. 29, 644–652. doi: 10.1038/nbt.1883
Hall, W., Johnston, L., and Donnelly, N. (1999). “Epidemiology of cannabis use and its consequences,” in The Health Effects of Cannabis, eds H. Kalant and W. Corrigal (Toronto: Addiction and Mental Health), 71–125.
Hosler, D. M. (2011). Early detection of dreissenid species: zebra/Quagga mussels in water systems. Aquat. Invasions 6, 217–222. doi: 10.3391/ai.2011.6.2.10
Howlett, A. C., Barth, F., Bonner, T. I., Cabral, G., Casellas, P., Devane, W. A., et al. (2002). International union of pharmacology. XXVII. Classification of cannabinoid receptors. Pharmacol. Rev. 54, 161–202. doi: 10.1124/pr.54.2.161
Joyce, A., and Vogeler, S. (2018). Molluscan bivalve settlement and metamorphosis: neuroendocrine inducers and morphogenetic responses. Aquaculture 487, 64–82. doi: 10.1016/j.aquaculture.2018.01.002
Kano, M., Ohno-Shosaku, T., Hashimotodani, Y., Uchigashima, M., and Watanabe, M. (2009). Endocannabinoid-mediated control of synaptic transmission. Physiol. Rev. 89, 309–380. doi: 10.1152/physrev.00019.2008
Karatayev, A. Y., Burlakova, L. E., and Padilla, D. K. (2015). Zebra versus quagga mussels: a review of their spread, population dynamics, and ecosystem impacts. Hydrobiologia 746, 97–112. doi: 10.1007/s10750-014-1901-x
Kavouras, J., and Maki, J. (2003). The effects of natural biofilms on the reattachment of young adult zebra mussels to artificial substrata. Biofouling 19, 247–256. doi: 10.1080/0892701032000077149
Kreitzer, A. C., and Regehr, W. G. (2002). Retrograde signaling by endocannabinoids. Curr. Opin. Neurol. 12, 324–330. doi: 10.1016/s0959-4388(02)00328-8
Lambert, D. M., Wouters, J., and Labar, G. (2010). A review on the monoacylglycerol lipase: at the interface between fat and endocannabinoid signalling. Curr. Med. Chem. 17, 2588–2607. doi: 10.2174/092986710791859414
Lee, C. Y., Lim, S. S. L., and Owen, M. D. (1990). The rate and strength of byssal reattachment by blue mussels (Mytilus edulis L.). Can. J. Zool. 68, 2005–2009. doi: 10.1139/z90-282
Li, B., and Dewey, C. N. (2011). RSEM: accurate transcript quantification from RNA-Seq data with or without a reference genome. BMC Bioinform. 12:323.
Li, Y. F., Guo, X. P., Yang, J. L., Liang, X., Bao, W. Y., Shen, P. J., et al. (2014). Effects of bacterial biofilms on settlement of plantigrades of the mussel Mytilus coruscus. Aquaculture 433, 434–441. doi: 10.1016/j.aquaculture.2014.06.031
Lucas, K. A., Pitari, G. M., Kazerounian, S., Ruiz-Stewart, I., Park, J., Schulz, S., et al. (2000). Guanylyl cyclases and signaling by cyclic GMP. Pharmacol. Rev. 52, 375–414.
Mao, X., Cai, T., Olyarchuk, J. G., and Wei, L. (2005). Automated genome annotation and pathway identification using the KEGG Orthology (KO) as a controlled vocabulary. Bioinformatics 21, 3787–3793. doi: 10.1093/bioinformatics/bti430
Matias, I., Bisogno, T., Melck, D., Vandenbulcke, F., and Salzet, M. (2001). Evidence for an endocannabinoid system in the central nervous system of the leech Hirudo medicinalis. Mol. Brain Res. 87, 145–159. doi: 10.1016/s0169-328x(00)00290-4
Matsuda, L. A., Lolait, S. J., Brownstein, M. J., Young, A. C., and Bonner, T. I. J. N. (1990). Structure of a cannabinoid receptor and functional expression of the cloned cDNA. Nature 346, 561–564. doi: 10.1038/346561a0
Matthews, M. A., and McMahon, R. F. (1999). Effects of temperature and temperature acclimation on survival of zebra mussels (Dreissena polymorpha) and Asian clams (Corbicula fluminea) under extreme hypoxia. J. Mollus. Stud. 65, 317–325. doi: 10.1093/mollus/65.3.317
McPartland, J. M., Agraval, J., Gleeson, D., Heasman, K., and Glass, M. (2006). Cannabinoid receptors in invertebrates. J. Evolution. Biol. 19, 366–373. doi: 10.1111/j.1420-9101.2005.01028.x
Mechoulam, R., Ben-Shabat, S., Hanus, L., Ligumsky, M., and Compton, D. R. (1995). Identification of an endogenous 2-monoglyceride, present in canine gut, that binds to cannabinoid receptors. Biochem. Pharmacol. 50, 83–90. doi: 10.1016/0006-2952(95)00109-d
Mechoulam, R., and Hanuš, L. (2000). A historical overview of chemical research on cannabinoids. Chem. Phys. Lipids 108, 1–13. doi: 10.1016/s0009-3084(00)00184-5
Montecucco, F., and Marzo, V. D. (2012). At the heart of the matter: the endocannabinoid system in cardiovascular function and dysfunction. Trends Pharmacol. Sci. 33, 331–340. doi: 10.1016/j.tips.2012.03.002
Morello, S. L., and Yund, P. O. (2016). Response of competent blue mussel (Mytilus edulis) larvae to positive and negative settlement cues. J. Exp. Mar. Biol. Ecol. 480, 8–16. doi: 10.1016/j.jembe.2016.03.019
Moriya, Y., Itoh, M., Okuda, S., Yoshizawa, A. C., and Kanehisa, M. (2007). KAAS: an automatic genome annotation and pathway reconstruction server. Nucleic Acids Res. 35, W182–W185.
Muller, C., Morales, P., and Reggio, P. H. (2019). Cannabinoid ligands targeting TRP channels. Front. Mol. Neurosci. 11:487.
Munro, S., Thomas, K. L., and Abu-Shaar, M. (1993). Molecular characterization of a peripheral receptor for cannabinoids. Nature 365, 61–65. doi: 10.1038/365061a0
Ohno-Shosaku, T., Tanimura, A., Hashimotodani, Y., and Kano, M. (2012). Endocannabinoids and retrograde modulation of synaptic transmission. Neuroscientist 18, 119–132. doi: 10.1177/1073858410397377
Prescott, T., Claudi, R., and Prescott, K. (2013). “Impact of dreissenid mussels on the infrastructure of dams and hydroelectric power plants,” in Quagga and Zebra Mussels: Biology, Impacts, and Control, 2nd Edn, eds T. F. Nalepa and D. W. Schloesser (Boca Raton: CRC Press), 244–257.
Rajagopal, S., van der Velde, G., van der Gaag, M., and Jenner, H. A. (2005). Byssal detachment underestimates tolerance of mussels to toxic compounds. Mar. Pollut. Bull. 50, 20–29. doi: 10.1016/j.marpolbul.2004.08.015
Rajagopal, S., Venugopalan, V. P., Velde, G. V. D., and Jenner, H. A. (2003). Response of fouling brown mussel, Perna perna (L.), to chlorine. Arch. Environ. Con. Tox. 44, 0369–0376.
Sahoo, G., and Khandeparker, L. (2018). Nitric Oxide-Serotonin interplay in the cyprid metamorphosis of Balanus amphitrite (Cirripedia, Thoracica). Int. Biodeter. Biodegr. 127, 95–103. doi: 10.1016/j.ibiod.2017.11.018
Salhab, A. (2017). Embattled cannabis: pharmacological, medical, recreational, and adverse effects aspects. J. Subst. Use. 22, 236–239. doi: 10.3109/14659891.2016.1149237
Salzet, M., and Stefano, G. B. (2002). The endocannabinoid system in invertebrates. Prostag. Leukot. Ess. 66, 353–361. doi: 10.1054/plef.2001.0347
Savinainen, J. R., Saario, S. M., and Laitinen, J. T. (2012). The serine hydrolases MAGL, ABHD6 and ABHD12 as guardians of 2-arachidonoylglycerol signalling through cannabinoid receptors. Acta Physiol. 204, 267–276. doi: 10.1111/j.1748-1716.2011.02280.x
Say, T. E., and Degnan, S. M. (2020). Molecular and behavioural evidence that interdependent photo-and chemosensory systems regulate larval settlement in a marine sponge. Mol. Ecol. 29, 247–261. doi: 10.1111/mec.15318
Schmidt, H. H., Lohmann, S. M., and Walter, U. (1993). The nitric oxide and cGMP signal transduction system: regulation and mechanism of action. BBA Mol. Cell. Res. 1178, 153–175. doi: 10.1016/0167-4889(93)90006-b
Sepe, N., De Petrocellis, L., Montanaro, F., Cimino, G., and Di Marzo, V. (1998). Bioactive long chain N-acylethanolamines in five species of edible bivalve molluscs: possible implications for mollusc physiology and sea food industry. Biochim. Biophys. Acta 1389, 101–111. doi: 10.1016/s0005-2760(97)00132-x
Smyth, D., Mahon, A. M., Roberts, D., and Kregting, L. (2018). Settlement of Ostrea edulis is determined by the availability of hard substrata rather than by its nature: implications for stock recovery and restoration of the European oyster. Aquat. Conserv. 28, 662–671. doi: 10.1002/aqc.2876
Soderstrom, K. (2009). “Lessons from nonmammalian species,” in Behavioral Neurobiology of the Endocannabinoid System. Current Topics in Behavioral Neurosciences, Vol. 1, eds D. Kendall and S. Alexander (Heidelberg: Springer-Verlag), 173–198. doi: 10.1007/978-3-540-88955-7_7
South, P. M., Floerl, O., and Jeffs, A. G. (2021). Immersion can trigger detachment of juvenile mussels. Aquaculture 538:736548. doi: 10.1016/j.aquaculture.2021.736548
Stafford, H., Willan, R. C., and Neil, K. M. (2007). The invasive Asian green mussel, Perna viridis (Linnaeus, 1758)(Bivalvia: Mytilidae), breeds in trinity inlet, tropical northern Australia. Molluscan. Res. 27, 105–109.
Stefan, G., Miguel, G. G. J., Javier, T., Williams, T. D., Nagaraj, S. H., José, N. M., et al. (2008). High-throughput functional annotation and data mining with the Blast2GO suite. Nucleic Acids Res. 36, 3420–3435. doi: 10.1093/nar/gkn176
Stefano, G. B., Bilfinger, T. V., Rialas, C. M., and Deutsch, D. G. (2000). 2-arachidonyl-glycerol stimulates nitric oxide release from human immune and vascular tissues and invertebrate immunocytes by cannabinoid receptor 1. Pharmacol. Res. 42, 317–322. doi: 10.1006/phrs.2000.0702
Stefano, G. B., Liu, Y., and Goligorsky, M. S. (1996). Cannabinoid receptors are coupled to nitric oxide release in invertebrate immunocytes, microglia, and human monocytes. J. Biol. Chem. 271, 19238–19242. doi: 10.1074/jbc.271.32.19238
Stefano, G. B., Salzet, B., and Salzet, M. (1997). Identification and characterization of the leech CNS cannabinoid receptor: coupling to nitric oxide release. Brain Res. 753, 219–224. doi: 10.1016/s0006-8993(96)01484-9
Sun, Y., Alexander, S. P. H., Garle, M. J., Gibson, C. L., Hewitt, K., Murphy, S. P., et al. (2007). Cannabinoid activation of PPARα; a novel neuroprotective mechanism. Br. J. Pharmacol. 152:734.
Tejero, J., and Stuehr, D. (2013). Tetrahydrobiopterin in nitric oxide synthase. IUBMB Life 65, 358–365.
Thalheim, B. (1997). Occurence and metabolism of anandamide and related acyl-ethanolamides in ovaries of the sea urchin Paracentrotus lividus. Biochim. Biophys. Acta 1345, 338–348. doi: 10.1016/s0005-2760(97)00009-x
Trapnell, C., Williams, B. A., Pertea, G., Mortazavi, A., Kwan, G., van Baren, M. J., et al. (2010). Transcript assembly and quantification by RNA-Seq reveals unannotated transcripts and isoform switching during cell differentiation. Nat. Biotechnol. 28, 511–515. doi: 10.1038/nbt.1621
Turnipseed, M., Knick, K. E., Lipcius, R. N., Dreyer, J., and Dover, C. L. V. (2010). Diversity in mussel beds at deep-sea hydrothermal vents and cold seeps. Ecol. Lett. 6, 518–523. doi: 10.1046/j.1461-0248.2003.00465.x
Twitchell, W., Brown, S., and Mackie, K. (1997). Cannabinoids inhibit N- and P/Q-type calcium channels in cultured rat hippocampal neurons. J. Neurophysiol. 78, 43–50. doi: 10.1152/jn.1997.78.1.43
Wachtel, S., Elsohly, M., Ross, S., Ambre, J., and Wit, H. D. (2002). Comparison of the subjective effects of Δ9-tetrahydrocannabinol and marijuana in humans. Psychopharmacology 161, 331–339. doi: 10.1007/s00213-002-1033-2
Winkle, W. V. (1970). Effect of environmental factors on byssal thread formation. Mar. Biol. 7, 143–148. doi: 10.1007/bf00354918
Yang, L., Li, L., Chen, L., Li, Y., Chen, H., Li, Y., et al. (2015). Potential analgesic effects of a novel N-acylethanolamine acid amidase inhibitor F96 through PPAR-α. Sci. Rep. 5:13565.
Yaqin, K., Tresnati, J., Rape, R. A., and Aslam, M. (2014). The use of Byssogenesis of green mussel, Perna viridis, as a biomarker in laboratory study. Curr. Nutr. Food. Sci. 10, 100–106. doi: 10.2174/1573401310666140306225434
Yin, H., Chu, A., Li, W., Wang, B., Shelton, F., Otero, F., et al. (2009). Lipid G protein-coupled receptor ligand identification using β-arrestin PathHunterTM assay. J. Biol. Chem. 284, 12328–12338. doi: 10.1074/jbc.m806516200
Young, M. D., Wakefield, M. J., Smyth, G. K., and Oshlack, A. (2010). Gene ontology analysis for RNA-seq: accounting for selection bias. Genome Biol. 11:R14.
Yuan, S., and Burrell, B. D. (2013). Endocannabinoid-dependent long-term depression in a nociceptive synapse requires coordinated presynaptic and postsynaptic transcription and translation. J. Neurosci. 33, 4349–4358. doi: 10.1523/jneurosci.3922-12.2013
Zhang, Y., He, L. S., Zhang, G., Xu, Y., Lee, O. O., Matsumura, K., et al. (2012). The regulatory role of the NO/cGMP signal transduction cascade during larval attachment and metamorphosis of the barnacle Balanus (=Amphibalanus) amphitrite. J. Exp. Biol. 215, 3813–3822.
Zhu, Y. T., Zhang, Y., Liu, Y. Z., Li, Y. F., Yoshida, A., Osatomi, K., et al. (2020). Nitric oxide negatively regulates larval metamorphosis in hard-shelled mussel (Mytilus coruscus). Front. Mar. Sci. 7:356.
Keywords: endocannabinoid, 2-AG, mussel, Perna viridis, attachment
Citation: Dai Q, Wang Z-X, Sheng Y-Q, Wu Z-W, Qiu Y, Su P, Ke C-H and Feng D-Q (2021) 2-Arachidonoylglycerol as an Endogenous Cue Negatively Regulates Attachment of the Mussel Perna viridis. Front. Mar. Sci. 8:719781. doi: 10.3389/fmars.2021.719781
Received: 03 June 2021; Accepted: 12 August 2021;
Published: 01 September 2021.
Edited by:
Gary H. Dickinson, The College of New Jersey, United StatesReviewed by:
Qiong Shi, Beijing Genomics Institute (BGI), ChinaJin-Long Yang, Shanghai Ocean University, China
Copyright © 2021 Dai, Wang, Sheng, Wu, Qiu, Su, Ke and Feng. This is an open-access article distributed under the terms of the Creative Commons Attribution License (CC BY). The use, distribution or reproduction in other forums is permitted, provided the original author(s) and the copyright owner(s) are credited and that the original publication in this journal is cited, in accordance with accepted academic practice. No use, distribution or reproduction is permitted which does not comply with these terms.
*Correspondence: Dan-Qing Feng, ZHFmZW5nQHhtdS5lZHUuY24=
†These authors have contributed equally to this work