- 1Guangdong Provincial Key Laboratory of Water Quality Improvement and Ecological Restoration for Watersheds, Institute of Environmental and Ecological Engineering, Guangdong University of Technology, Guangzhou, China
- 2Southern Marine Science and Engineering Guangdong Laboratory, Guangzhou, China
- 3Department of Estuarine and Delta Systems, Royal Netherlands Institute for Sea Research, Utrecht University, Yerseke, Netherlands
- 4Department of Physical Geography, Faculty of Geosciences, Utrecht University, Utrecht, Netherlands
Coastal wetlands such as salt marshes have been increasingly valued for their capacity to buffer global climate change effects, yet their long-term persistence is threatened by environmental changes. Whereas, previous studies largely focused on lateral erosion risk induced by stressors like sea level rise, it remains poorly understood of the response of lateral expansion to changing environments. Seedling establishment is a key process governing lateral marsh expansion as seen in many coastal regions such as Europe and East Asia. Here, we evaluate mechanistically the response of seed bank dynamics to changing physical disturbance at tidal flats, using the globally common coastal foundation plant, cordgrass as a model. We conducted a large-scale field study in an estuary in Northwest Europe, where seed bank dynamics of cordgrass in the tidal flats was determined and linked to in situ hydrodynamics and sediment dynamics. The results revealed that wave disturbance reduced the persistence of seeds on the surface, whereas amplified sediment disturbance lowered the persistence of both surface and buried seeds. Overall, this indicates that increasing storminess and associated sediment variability under climate change threatens seed bank persistence in tidal flats, and hence need urgently be incorporated into models for long-term bio-geomorphological development of vegetated coastal ecosystems. The knowledge gained here provides a basis for more accurate predictions on how climatically driven environmental changes may alter the fitness, resilience and persistence of coastal foundation plants, with significant implications for nature-based solutions with coastal vegetation to mitigate climate change effects.
Introduction
Vegetated wetlands (e.g., salt marshes, seagrasses, and mangroves) are among the most ecological and socio-economically valuable systems on the globe (Costanza et al., 1997). Over the last decades, there has been increasing recognition of the coastal defenses value of vegetation due to their efficacy in wave attenuation and sediment stabilization (Gedan et al., 2011; Temmerman et al., 2013; Moller et al., 2014; Zhu et al., 2020b). This initiated a paradigm shift to a more ecosystem-based flood protection using vegetated foreshores in the face of climate change (Cheong et al., 2013; Temmerman et al., 2013). Given a rising sea level and increasing storminess as predicted for many parts of the world (Donat et al., 2011; Young et al., 2011) and the vulnerability of coastal wetlands to environmental variations like sea level rise (Kirwan and Temmerman, 2009; Kirwan and Megonigal, 2013) and waves (Callaghan et al., 2010; Marani et al., 2011), it raises uncertainties in the stability and long-term persistence of coastal wetlands.
Seedling establishment is a crucial process of vegetation recovery seen in many meso- and macrotidal marsh ecosystems around the world such as in Europe and East Asia (Gray et al., 1991; Temmerman et al., 2007; Strong and Ayres, 2013; Liu et al., 2017). Unfortunately, seedling recruitment often forms a bottleneck, as waves and associated sediment dynamics often impose difficulties for seedling survival (e.g., Bouma et al., 2009; Friess et al., 2012; Balke et al., 2013) and seed persistence (Groenendijk, 1986; Marion and Orth, 2012; Zhu et al., 2014). Although, the minimal period that seed banks need to persist can be short, to cover the period between when seeds become available (fall) and seedlings start to emerge (spring), seed persistence (i.e., seed retention and preservation of viability) during this short period is vital. Seed bank formation in the coastal sediment matrix can be facilitated by sediment mixing induced by hydrodynamics coupled with ecosystem engineering of benthic fauna (Delefosse and Kristensen, 2012; Blackburn and Orth, 2013; Zhu et al., 2016a). However, seed dislodgment due to sediment erosion serves as a major source for seed loss of coastal foundation species at tidal flats (Groenendijk, 1986; Marion and Orth, 2012; Zhu et al., 2014), in which wave action plays a critical role in remobilizing the initially deposited seeds (Chang et al., 2008; Koch et al., 2010; Zhu et al., 2020a). A flume study on marsh plant seeds showed that wave-generated orbital water movement is necessary to dislodge and move seeds from the bottom to the water column where they can be exported by current velocity, whereas even high currents have a very limited ability to export waterlogged seeds in the absence of waves (Chang et al., 2008). A recent field study found that seed removal from tidal flat surface increased with wave-induced bed shear stress (Zhu et al., 2020a). When seeds germinate, they can be more easily lifted off by currents because of enhanced buoyancy. A recent flume study of Spartina seeds shows that seed removal from the surface increased non-linearly with germination stage and current velocity (Zhao et al., 2021).
Although, climate-change intensified hydrodynamic disturbance and associated sediment disturbance at tidal flats (e.g., the removal of sediment particles) may be expected to reduce seed bank persistence of coastal foundation plants, with far-reaching consequences for long-term ecosystem stability and functions, this is lacking from current biogeomorphic models. One of the keystone coastal plant engineers is cordgrass (Spartina spp.), which globally defines and stabilizes the shoreline of many temperate marshes (Strong and Ayres, 2009, 2013; Bortolus et al., 2019). Seed colonization plays a vital role in its natural (re-) establishment and range expansion, as well as the fast spread in many areas where the species has been willingly or accidentally been introduced (Gray et al., 1991; Ayres et al., 2008; Xiao et al., 2009). Seedling establishment of cordgrass occurs mainly in the pioneer zone and adjacent bare mudflats at suitable elevations at the presence of an annually built soil seed bank (Wolters and Bakker, 2002; Xiao et al., 2009). Given the role of cordgrass in enhancing coastal defense as powerful ecosystem engineers and its worldwide distribution, it is highly relevant to study the potential effects of climate-induced shifts in physical disturbance on the fate of its (annual) seed bank.
In this article, we investigate the persistence of cordgrass seed bank at tidal flats under variable hydro-morphodynamic conditions. Since hydrodynamics induced seed dislodgment serves as a major source of seed loss from the local seed bank at tidal flats (Groenendijk, 1986; Marion and Orth, 2012; Zhu et al., 2014), we here focused on the physical presence of seeds in the sediment matrix. Other components of seed persistence that are not directly affected by hydrodynamics, such as seed viability and seed germination, are beyond the scope of this study. Specifically, we examine whether increased physical disturbances decline seed bank persistence of cordgrass at tidal flats. Previous studies showed that the mobility of seeds on tidal flats was primarily determined by wave motion (Chang et al., 2008; Zhu et al., 2020a), and the retention of buried seeds at tidal flats increased with seed burial depth (Marion and Orth, 2012; Zhu et al., 2014). Hence, we hypothesize that stronger waves and greater sediment dynamics erode more seeds from the seed bank at tidal flats. To test these hypotheses, we conducted a large-scale field study in the Scheldt estuary in NW Europe, where seed bank persistence of cordgrass at tidal flats was determined and linked to local wave conditions and sediment dynamics. We aim to drive essential quantitative relationships to enable biogeomorphic modelers to include the potential effects of altered physical disturbance by environmental changes on the resilience and persistence of coastal foundation plants.
Materials and Methods
Study Site
The Scheldt estuary is a macrotidal estuary situated near the border between Netherlands and Belgium (Figure 1). The mean tidal range increases from 3.8 m near the mouth of the estuary to > 5.0 m upstream of the border (Baeyens et al., 1998). It was originally composed of two aligned and interconnected water bodies called Westerschelde and Oosterschelde. Due to land reclamation, the Oosterschelde was progressively separated from the Westerschelde. The pioneer salt marsh vegetation consists mainly of the perennial common cordgrass (Spartina anglica), which was introduced to the Scheldt estuary in the 1920s (Groenendijk, 1986; van der Wal et al., 2008). The study area comprises seven salt marshes with different wave exposure due to their position relative to the prevailing southwesterly winds (Callaghan et al., 2010; Suykerbuyk et al., 2016). Site Paulinapolder (PA), Zuidgros (ZG), Baarland (BA) and Hellegateplder (HE) are in the Westerschelde, whereas Dortsman (DO), Rattekaai (RA) and Zandkreek (ZA) in the Oosterschelde (Table 1 and Figure 1). All the experiments and measurements were conducted in adjacent tidal flats in front of a salt marsh. The elevation of the experimental area was ca. 90 cm above NAP (i.e., Dutch ordinance level, which is close to local mean sea level) for most field sites, while a higher elevation (175 cm NAP) was adopted in two Westerschelde sites ZG & BA, where salt marshes extend less deep. At ZG, we included an additional location with a lower elevation (ca. 90 cm NAP) for direct comparison between the higher and lower elevations (ZGHIGH and ZGLOW). The mean velocity at tidal flats of the four Westerschelde site is less than 0.2 m/s (Callaghan et al., 2010), whereas the maximum velocity at tidal flats of the three Oosterschelde sites is around 0.2 m/s (Suykerbuyk et al., 2016; Table 1). A recent flume study on seed transport of cordgrass seeds shows that current velocity of <0.2 m/s has limited ability to lift-off ungerminated seeds (Zhao et al., 2021).
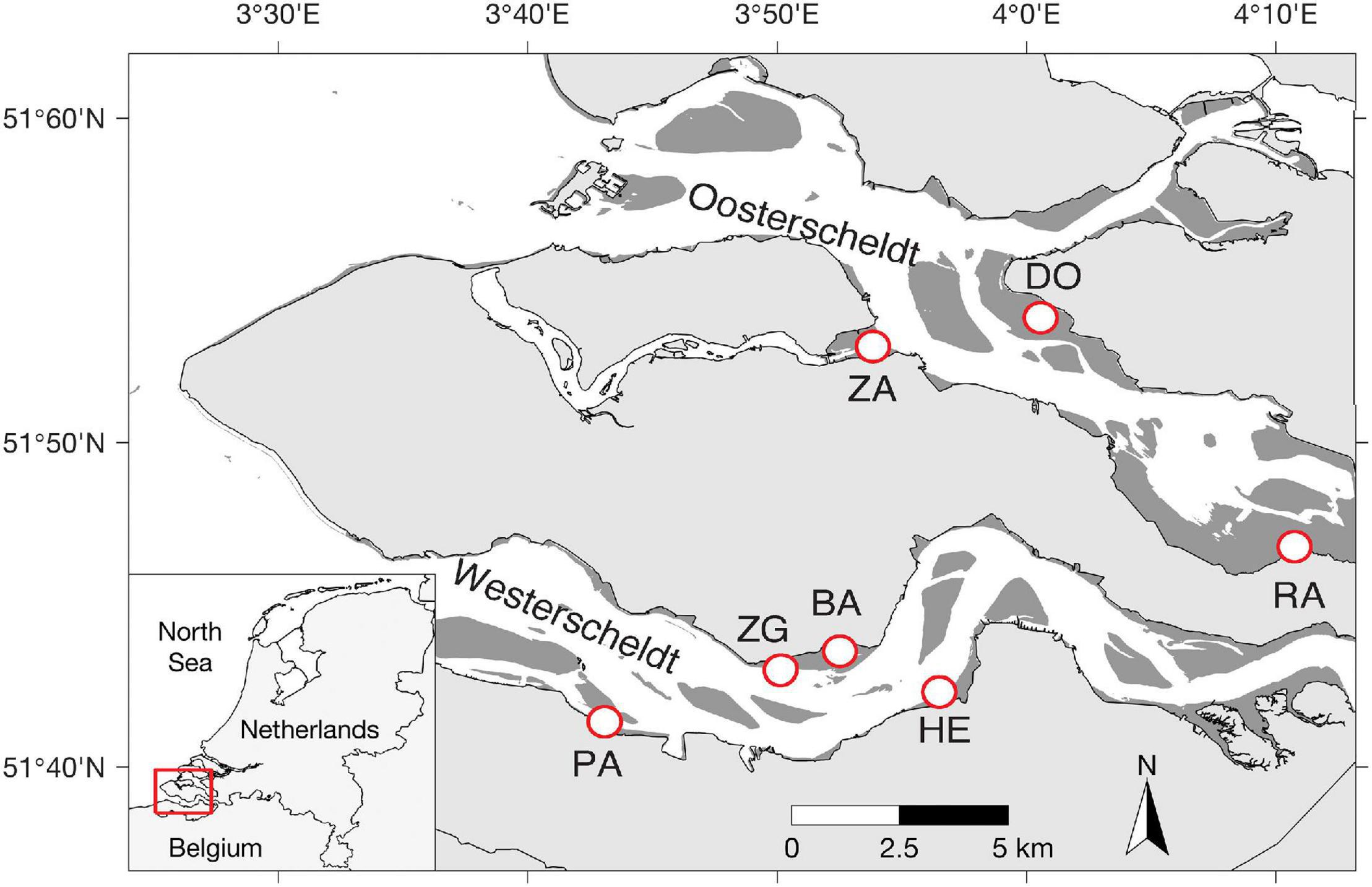
Figure 1. Geographic locations of selected study sites. Site Paulinapolder (PA), Zuidgros (ZG), Baarland (BA), and Hellegateplder (HE) are in the Westerschelde, whereas Dortsman (DO), Rattekaai (RA), and Zandkreek (ZA) in the Oosterschelde (Table 1).
Manipulative Seed Bank Experiment
To examine the relationship between seed bank persistence and physical disturbance, we conducted a manipulative seed bank experiment in all the selected locations. The manipulative seed bank was built by placing pre-prepared layered seed bank cores into the sediment. Seed mimics were also used to detect if any biogenic seed loss (e.g., predation and decomposition) occurred. For each core, five seeds/mimics were placed at the surface and the depth of 1–3 cm (Figure 2A). Layered indicator cores (Marion and Orth, 2012) were employed to estimate sediment disturbance depth (SDD) by placing tracer particles at known depths. Such particles were intended to wash away when momentarily resuspended by wave disturbance, leaving a record of scouring depth (Marion and Orth, 2012). In our experiment, Quartz Flour (manufactured silt, white, d50 = 100 μm) served as tracer particles, which were deployed at 0.5 cm intervals alternating with ambient sediments to make layered cores consisting of 3 tracer layers and 4 sediment layers (Figure 2B).
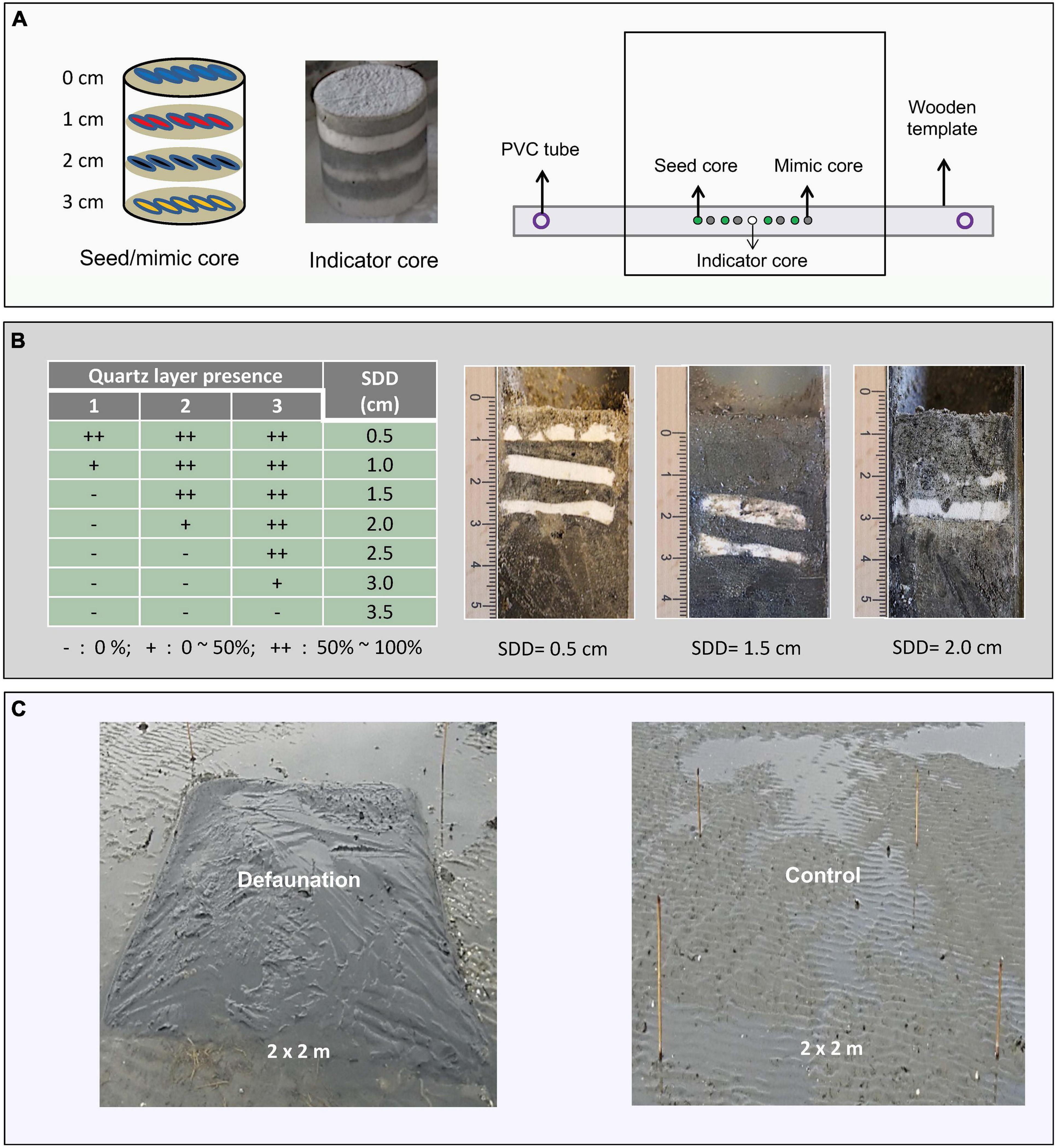
Figure 2. (A) Seed bank core, indicator core, and their deployment in the plots. (B) Scoring rules and examples of sediment disturbance depth (SDD). (C) Defaunated and control plots.
Layered seed/mimic cores were created in 200 ml top-truncated syringes (3.6 cm diameter) with homogenized ambient sediment and color-stained seeds/mimics, using the same methods and mimics (plastic chips of four colors) as described by Zhu et al. (2014). This material was proven to be highly effective in mimicking cordgrass seeds (Zhu et al., 2014). The sowed seeds were first sterilized by freezing them in a −20°C freezer for 2 weeks to prevent seed loss due to germination (Zhu et al., 2016b). Layered indicator cores for sediment disturbance were produced in the same syringes as the seed bank cores. Each layer was made by filling in homogenized tracer particles/ambient sediment to a thickness of 0.5 cm and then sent to the −20°C freezer. While still frozen, a new layer was added to minimize mixing. When all the layers were ready, these cores were taken out of the syringes and preserved in the freezer until use.
In each location, four permanent 2 × 2 m plots, ca. 3 m apart, were established and marked by PVC stakes (Figure 2A). Half of these plots were defaunated to detect the importance and the effects of the benthic animals on seed bank persistence (Figure 2C). The defaunation was implemented in January 2013 by covering the plots with a thick rubber sheets that were buried to a depth of 30 cm along the plot edges (Van Colen et al., 2008). Removing the aboveground rubber materials, the defaunated plots were opened after 12 weeks, which was sufficient long to kill the benthic animals by hypoxia (Van Colen et al., 2008). Seed bank cores and indicator cores were established 4 weeks later when the sediment surface in defaunated plots was leveled by natural physical process. This defaunation method has been proven to greatly lower the abundance of benthic animals; recovery takes at least one summer (Beukema et al., 1999; Van Colen et al., 2008).
In each defaunation/control plot, 4 seed cores, 4 mimic cores, and 1 indicator core were deployed. These cores were located in a line through a wooden template with 9 round holes (40 mm diameter), 15 cm apart. The indicator core was situated in the middle, while the seed cores and mimic cores were alternating each other on both sides at intervals of 15 cm (Figure 2A). All the cores were transported to the field in a foam box with dry ice to keep them frozen, and then placed with their surface level with the surrounding sediment in the method as described in Zhu et al. (2014). Both endpoints of the template possessed a hole that fitted a fixed PVC tube inserted into the soil, to allow accurate re-relocation upon retrieval.
After 8 weeks, all these cores were relocated using the same template and recovered to a depth of 100 mm, through PVC pipes which are larger in diameter (5 cm) than the original cores, to ascertain the whole core is re-sampled even if there is a slight imprecision in relocation. The recovered seed/mimic cores (Depth = 100 mm) were transported to the lab, sieved through a 1 mm sieve to retrieve deployed seeds and mimics, which were classified per layer according to their colors. Many seeds (but no mimics) turned black when retrieved because of the anaerobic condition within the sediment. To identify the stained color of those seeds, they were bathed in fresh water for at least 24 h until their original stain re-appeared (Zhu et al., 2014). The number of recovered seeds or mimics of each color was counted and recorded. Seed persistence (%) was then calculated as the recovered/total deployed.
Upon arrival in the lab, the retrieved indicator cores were stored in a −20°C freezer until processing. While still frozen, they were longitudinally cross-sectioned using a band saw. The frozen smear left by the band saw was removed using a razor. SDD was estimated through a scoring system that examines the presence of Quartz Flour layers in the profile of the half core (scoring rules and examples given in Figure 2B). In total, 30 out of 32 indicator cores were scored, whereas two cores retrieved from one control plot at ZGLOW and one defaunation plot at PA failed due to the damage during processing.
This was implemented in a relative storm-free period (May–July, 2013) to exclude the impacts of severe storms, and to obtain a range of wave conditions including both small and large waves. During this period, the wind speed was usually less than 10 m/s and winds were mostly from Southwest or Southeast (Supplementary Figure 1). The wave forcing and tidal level of each location was measured using pressure sensors (OSSI-010-003C; Ocean Sensor Systems, Inc.) deployed in the experiment zone. The pressure sensors were placed 5 cm above the tidal flat surface. The measuring interval and period were 15 and 7 min, respectively. The wave analysis was based on pressure fluctuations, as measured with a frequency of 5 Hz. The recorded pressure readings were converted to water level fluctuations, which were then corrected by removing erroneous spikes, shifts, corrupted bursts, and low frequency tidal components (c.f. Callaghan et al., 2010; Christianen et al., 2013). From the detrended data, water depth (h) was determined meanwhile wave parameters, e.g., significant wave height (Hs) and wave period (Ts) were calculated based on the linear wave theory (Tucker and Pitt, 2001).
Based on water depth and wave parameters, we calculated the time-averaged bed shear stress induced by waves (τwave) with the same method (Supplementary Methods) as described in previous studies (Callaghan et al., 2010; Zhu et al., 2019, 2020a). τwave is a relevant proxy for the hydrodynamic energy in relation with sediment motion (Callaghan et al., 2010) and seed removal from tidal flat surface (Zhu et al., 2020a). The elevation in the experimental zone was monthly monitored during Mar and July, using a 3D Laser scanner (RIEGL VZ-400), as described in our previous study at the same locations (Zhu et al., 2020a).
Statistics
We first conducted Pairwise Wilcoxon Rank Sum Tests to detect the difference of seed bank persistence between seeds and seed mimics. Since the results showed no significant difference (p = 0.33) between them, these data were pooled together in further analysis. Due to the non-normally distributed data, GLMs (Generalized linear models) were employed to examine the effects of study location, seed burial depth and treatment (defaunated or not) on seed persistence. We specified “binomial” family to the model, given a negative binomial distribution of the data. When necessary, we refitted the model using “quasi-binomial” to account for the over-dispersion. We then calculated the averaged seed persistence for the control and defaunated plots at each location, respectively, and linked it to wave disturbance (τwave) and SDD at that location via linear models. Prior to the analysis, the data of seed persistence was square root transformed to satisfy the requirement of data normality. All the statistical analysis was done in R1, applying a significance level of α = 0.05.
Results
Overview of Physical Conditions at All Study Locations
The selected locations exhibited variable water depth and wave conditions during the experiment (Figure 3 and Table 2). The maximum value of water depth h ranged between 0.9 m (Site BA) and 1.9 m (Site PA). The mean significant wave height Hs, covered a range of 0.03–0.10 m, the range of maximum Hs was between 0.17 and 0.44 m. The range of mean wave period Ts was 2.97 – 9.71 s, and the maximum value range was 47.28 to 410.90 s.
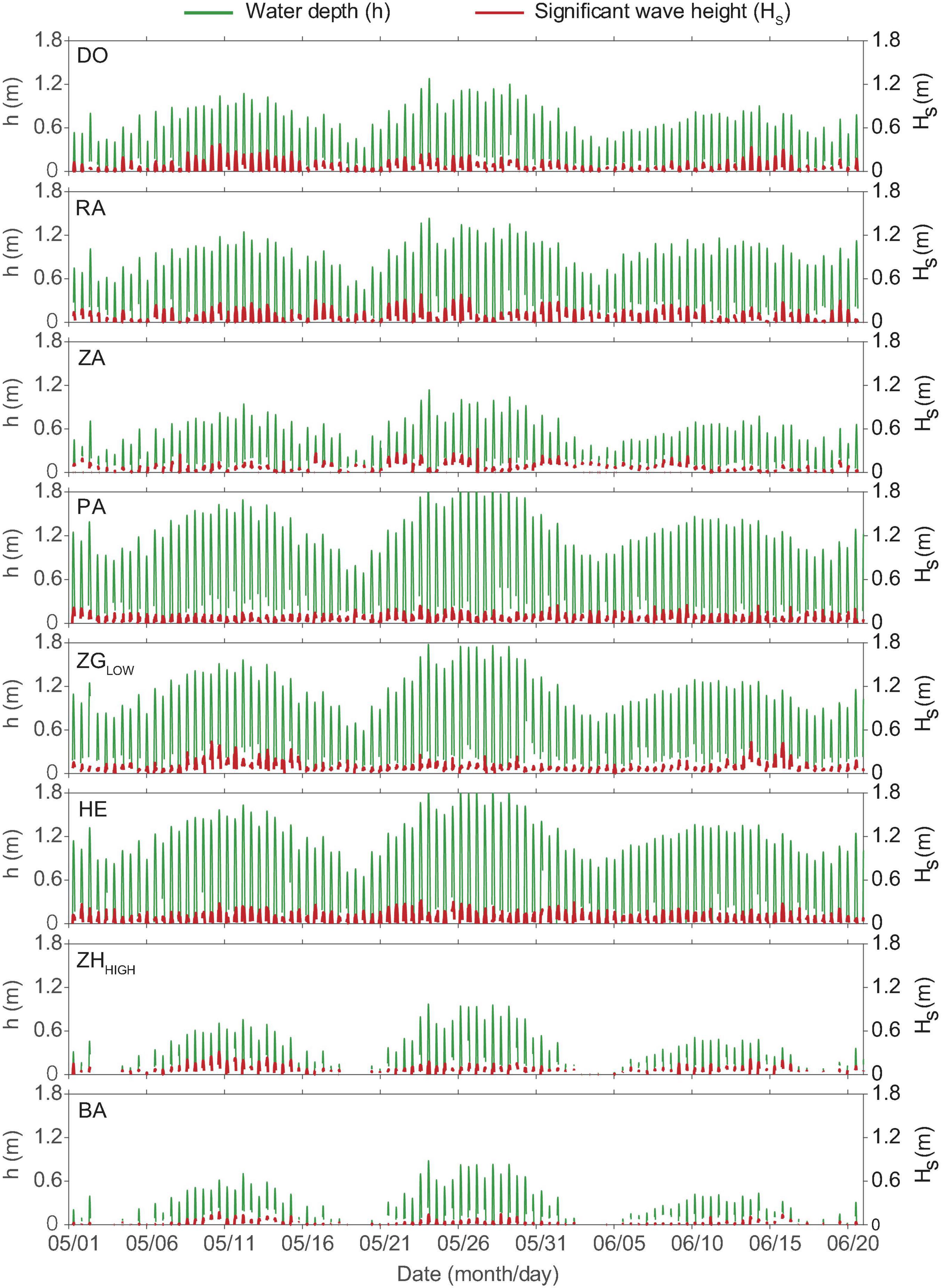
Figure 3. Time-series water depth (h, m) and significant wave heights (Hs, m) at all locations during the experiment. The mean and maximum value of significant wave heights and wave periods are summarized in Table 2.
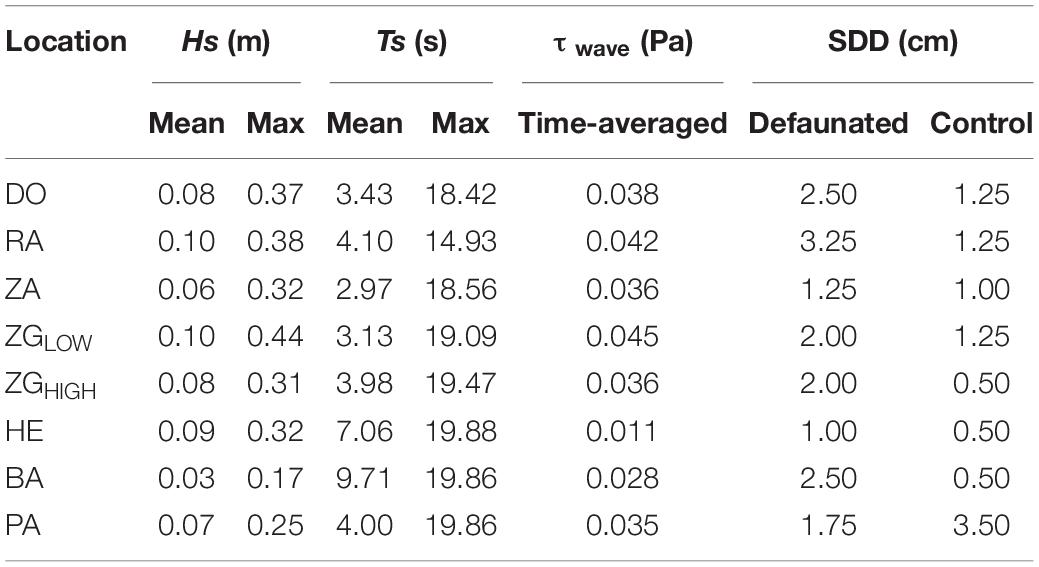
Table 2. Significant wave heights (Hs), wave periods (Ts), wave-induced bed shear stress (τwave, time-averaged), and sediment disturbance depth (SDD) at each study location during the experiment.
Sediment disturbance depth differed between locations as well as between defauated and control plots (Table 2). SDD ranged from 1.00 cm to 3.25 cm for the control plots, whereas it was 0.50 – 3.50 cm for the defaunated plots. The defaunation plots had greater SDD (p < 0.001), suggesting that at the local scale benthic animals may affect seed bank persistence by influencing sediment erodibility to hydrodynamics.
Relationship Between Physical Disturbance and Seed Bank Persistence
Seed persistence at tidal flats was dependent on their vertical positions, and varied between control and defaunated plots, as well as between locations that differed in the strength of wave disturbance and sediment disturbance (Figure 4).
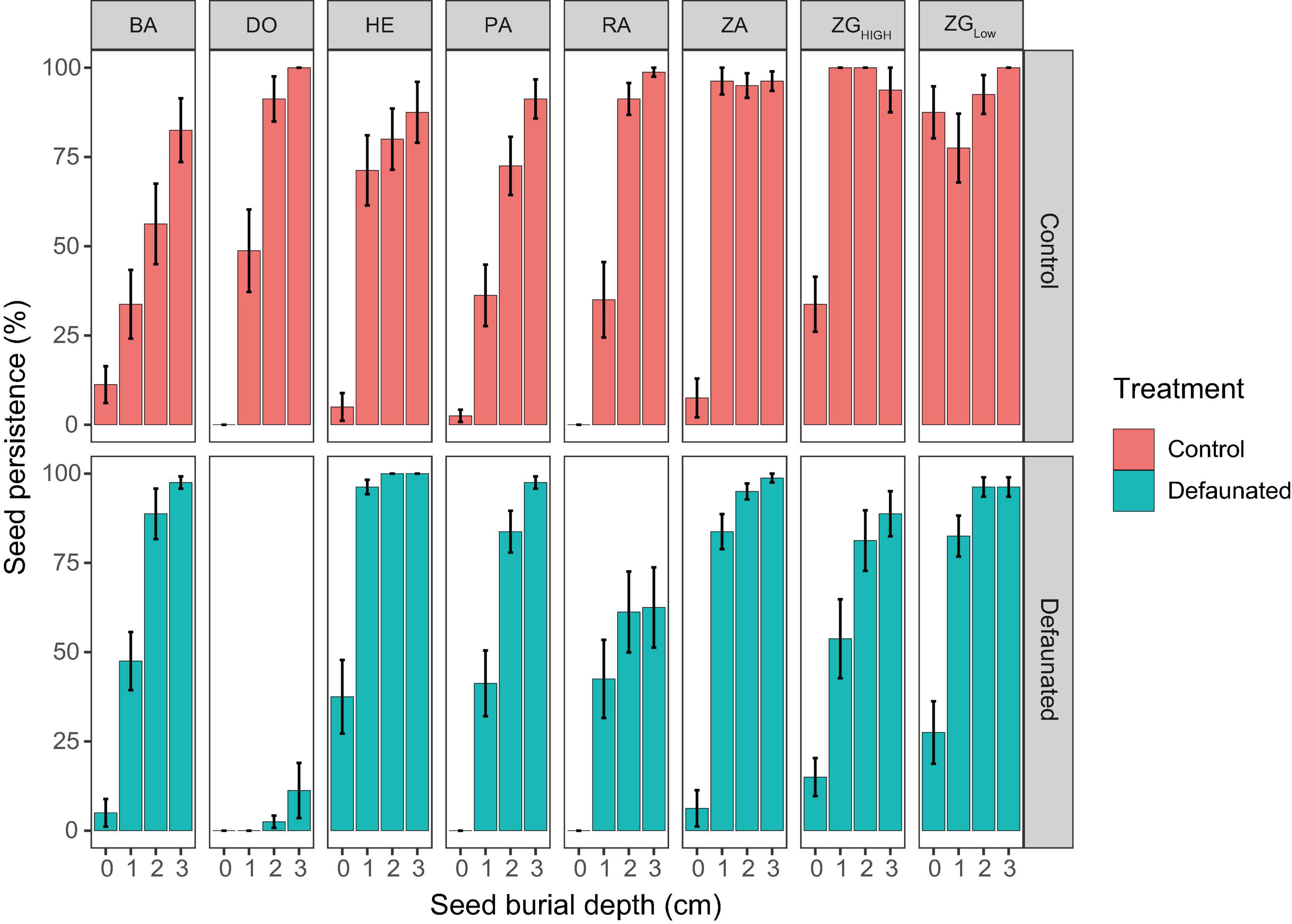
Figure 4. The persistence of the manipulated seed bank at each study location, which was shown for the decaudated treatment (green bars) and the control (red bars), separately.
For the seeds on the surface, their persistence declined with the growth of wave-induced bed shear stress (τwave) and SDD (Figure 5). Such relationship could be described by the function: z = 0.63 − 0.10 x −8.46 y, where x is SDD (cm), and y is wave-induced bed shear stress (Pa). This relationship was not significant when including data from all locations, whereas it became highly significant if the only continuously fast accreting location ZGLOW (ca. 1.2 mm/d, Supplementary Figure 2) was removed from the analysis (Table 3). The fast accretion most likely resulted from the dumping of dredged sediment near this location (Sistermans and Nieuwenhuis, 2019), which might offer the surface seeds a window of opportunity to get sufficiently buried to escape wave disturbance.
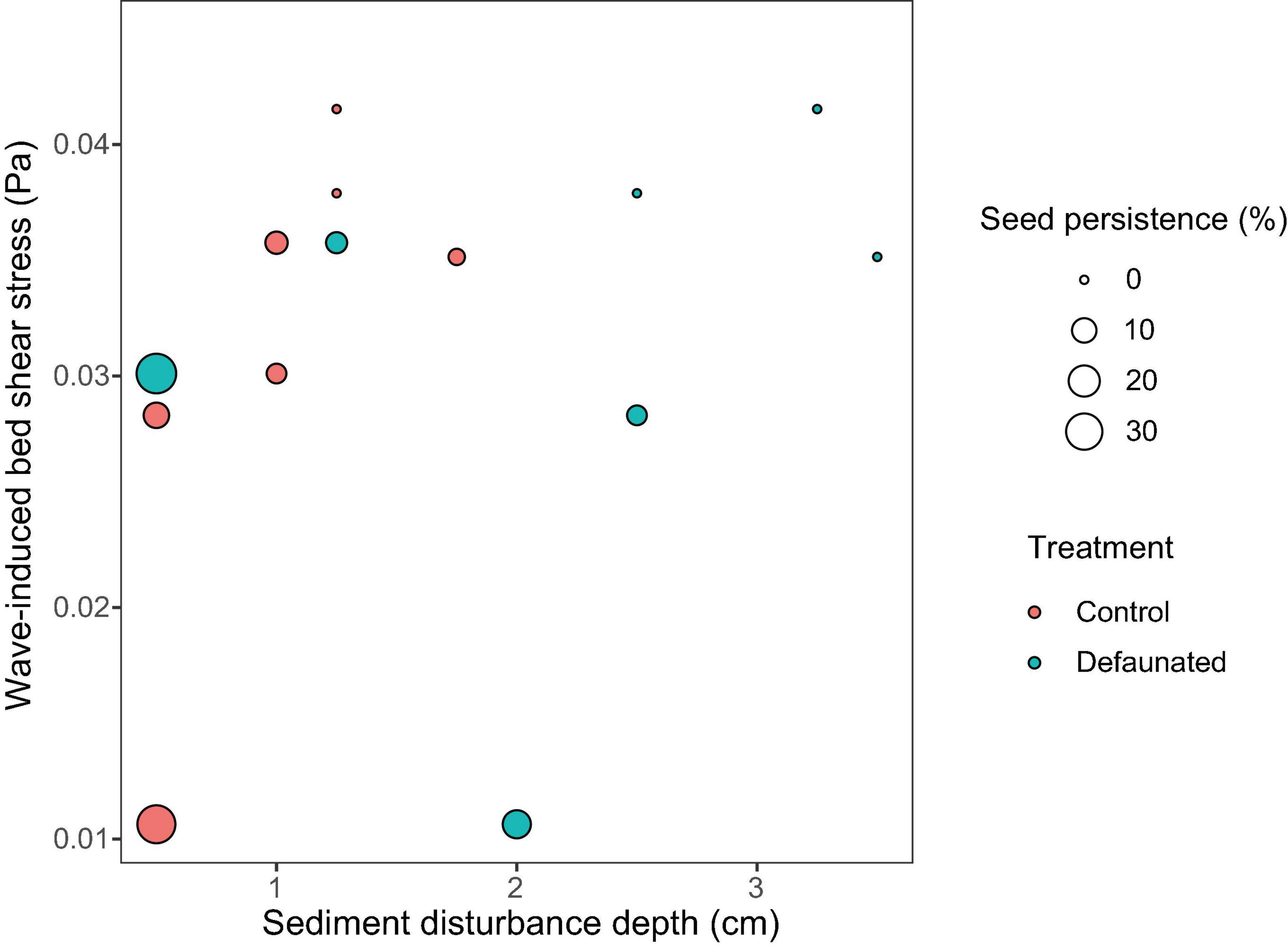
Figure 5. The combined data from the decaudated treatment (green circles) and the control (red circles) showed that, the persistence of seeds on the surface (presented as the size of the circle) decreased with increased wave disturbance and sediment disturbance. Such relationship could be described by the function: z = 0.63 − 0.10 x −8.46 y, where x is sediment disturbance depth (cm), and y is wave-induced bed shear stress (Pa).
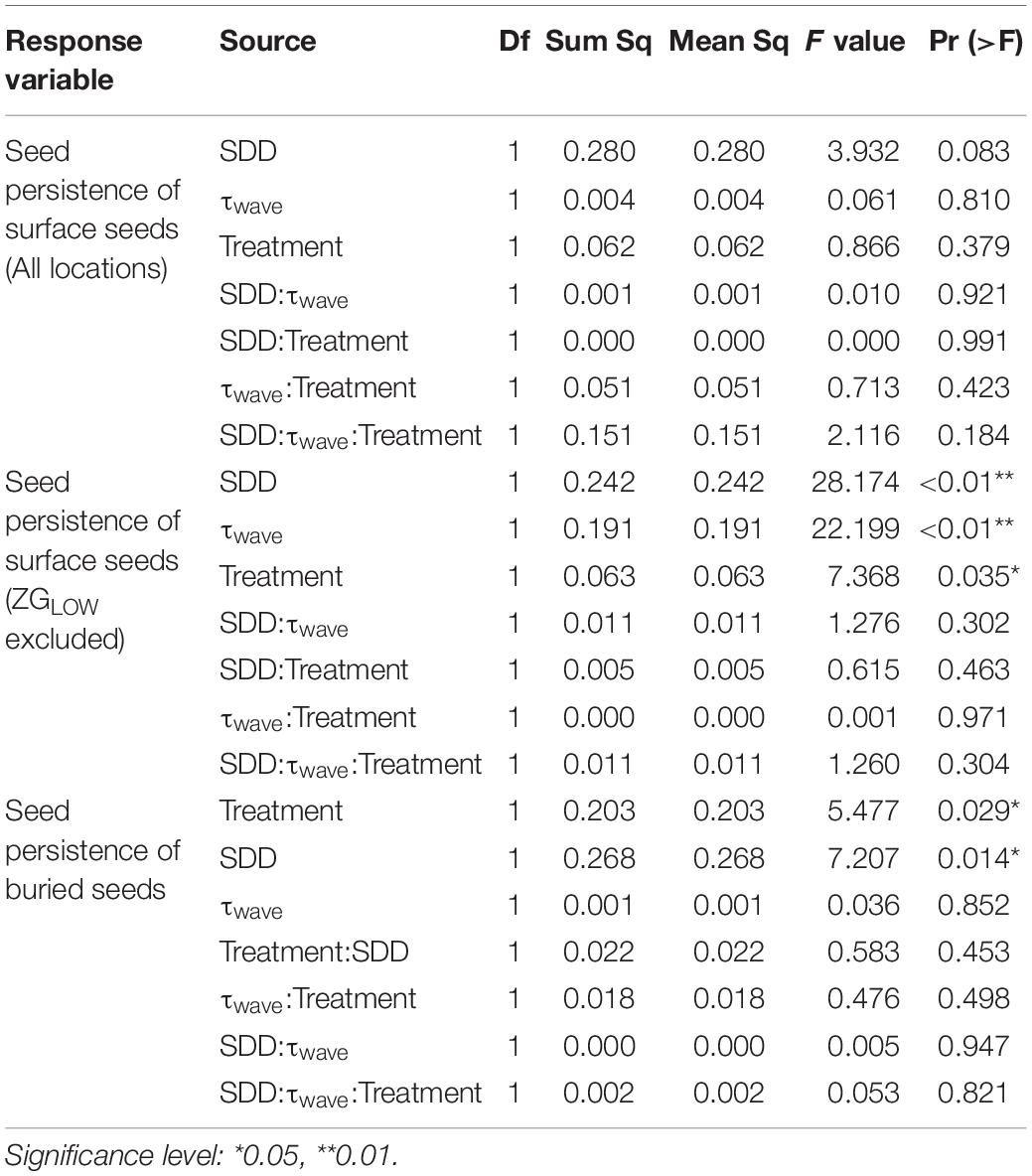
Table 3. ANOVA table for the linear models examining the response of seed persistence to sediment disturbance depth (SDD), wave disturbance (τwave), and treatment (defaunated/control).
When seeds were buried, their persistence decreased significantly with growing SDD, whereas there was no significant effect from wave disturbance (Figure 6A and Table 3). Further analysis revealed that seed persistence was enhanced non-linearly with increasing seed burial depth and the response curve was affected by SDD (Figure 6B). Greater SDD led to lower seed persistence and vice versa.
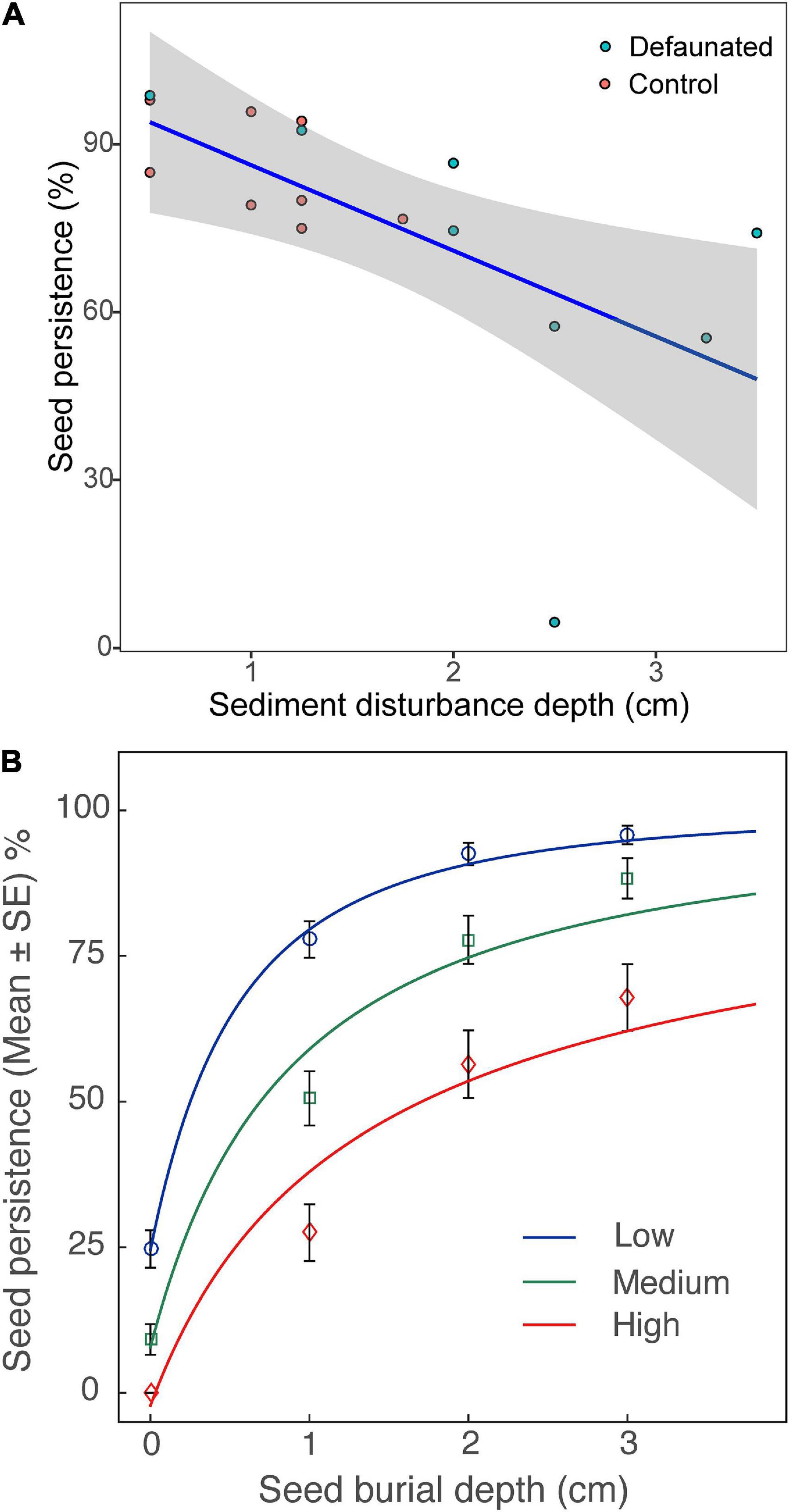
Figure 6. (A) The persistence of the buried seeds decreased with increased sediment disturbance depth (curve fit: y = 1.01 – 0.15x). (B) Effects of sediment disturbance depth (SDD, three levels: low, medium and high) on the persistence of seeds from different burial depths; Low: SDD < = 1 cm, Medium: 1 < SDD < = 2 cm, and High: SDD > 2 cm; the response curves were fit with function: y = 1 − (x + a) ^ b (Zhu et al., 2014).
Additional Pairwise Wilcoxon Rank Sum Tests showed that there was no significant difference of surface seed persistence between the defaunated and control plots (p = 0.09). In contrast, seed persistence of the buried seeds in the defaunated plots was significantly lower (p < 0.001) than that in the control plots, where SDD was generally higher (Figure 6A).
Discussion
Whereas, previous studies and modeling work largely focused on lateral marsh erosion risk induced by stressors like sea level rise, much less is known on the response of lateral marsh expansion to changing environments. Physical disturbance (e.g., waves and sediment mixing) on seedling establishment has been suggested as a main bottleneck for seed-based lateral expansion of coastal marsh vegetation (Lovelock et al., 2010; Friess et al., 2012; Balke et al., 2013). Using cordgrass as an example, this study clearly showed that wave disturbance affected the persistence of seeds on the surface, whereas sediment disturbance decreased the persistence of both surface and buried seeds. Overall, our findings indicate that (i) climate change likely reduce seed bank persistence in coastal wetlands via intensifying wave forcing and associated sediment disturbance and (ii) this process hence needs to be incorporated in biogeomorphic models predicting the future of coastal wetlands.
Seed burial can non-linearly enhance seed persistence. However, the buried seeds would still be turned over by sediment disturbance. Our experiment demonstrated that the quantity of seed loss from the buried seed bank was determined by both SDD and seed burial depth. Greater disturbance depth can turn over the buried seeds that would otherwise remain “safe” under lower disturbance depth. Although, wave disturbance might ease seed burial by promoting sediment mixing (Zhu et al., 2016a), the present study showed that amplified wave disturbance and sediment variability led to increased seed erosion. Deep burial improves seed persistence at tidal flats, yet it can lead to the failure of seed-seedling transition. Seedling emergence of cordgrass was found to decline with increasing seed burial depth (Bouma et al., 2016), as seeds germinated from deep depth are not able to reach the sediment surface (Greve et al., 2005). Hence, successful seed-seedling transition predominantly requires seeds to be positioned in moderate depths that benefit both seed persistence and seedling emergence.
The eroded seeds may be transported seaward to distant places or washed ashore (Huiskes et al., 1995). The landward transported seeds may either be trapped by the standing vegetation (Chang et al., 2008) or add into driftline materials deposited near/on the dike (Wolters and Bakker, 2002). Our results imply that, in coastal habitats with predicted intensifying storminess under global change, more frequent and stronger waves in winter and early spring might lower seed bank formation and persistence in more exposed areas, i.e., the pioneer zone and tidal flats. In contrast, seeds would tend to settle down in more sheltered areas (e.g., within vegetation), where seedling establishment is however less meaningful due to the lack of niches.
Sediment disturbance was primarily driven by hydrodynamics, but can also be shaped by benthos activities. Many benthic organisms (e.g., polychaetes and bivalves) are known to increase local sediment erodibility by bioturbating activities (Widdows and Brinsley, 2002; Meysman et al., 2006; Andersen et al., 2010), while some others (e.g., diatoms) can armor and stabilize the sediment (Stal, 2003). In our large-scale field experiments, the reduction of benthos resulted in overall higher sediment disturbance that caused lower seed persistence. This finding suggests that sediment erodiblity and seed bank mobility may be non-linearly enhanced by intensified wave disturbance, given that benthos abundance in intertidal flats tends to be lower under stronger physical forcing (e.g., Cozzoli et al., 2014).
Coastal vegetated systems like salt marshes are dynamic systems with long-term cyclic alternations between a retreating phase of cliff erosion and an establishment phase by seedling recruitment (van de Koppel et al., 2005; Bouma et al., 2016). Successful seedling establishment requires the presence of window of opportunity, i.e., disturbance free (or low) period (Balke et al., 2011, 2014; Hu et al., 2015). With amplified disturbance characteristics (frequency and magnitude) under climate change, seed colonization potential of coastal vegetated systems may decline due to worsened seed bank formation, and seed bank persistence. Such effect combined with reduced window of opportunity for seedling survival due to amplified physical disturbances can lead to a substantial reduction of regeneration capacity. Consequently, an impaired regeneration capacity might affect long-term vegetation persistence by disabling the shift of available niches from a bare state to a vegetated state, thus “locking” the system to the retreating phase. This will inevitably threaten long-term persistence of valuable coastal wetlands, thereby risking the loss of invaluable ecosystems services such as coastal defense values and carbon sequestration.
Biogeomorphic models are essential tools for predicting long-term evolution and persistence of coastal wetlands. However, in current biogeomorphic models that simulate lateral marsh dynamics, seeds are typically assumed to be present everywhere, with the seedling survival being related to bed-shear-stress (Temmerman et al., 2007; Fagherazzi et al., 2012). The current study clearly showed that seed persistence at tidal flats was dependent on both wave disturbance and sediment disturbance. To enable more accurate simulations of lateral marsh dynamics, the availability of seeds at tidal flats should be calculated as a function of bed shear stress induced by waves and the magnitude of sediment erosion. The quantitative relationship derived from our field measurements (Figure 5) could contribute to the parametrization of such a function.
Conclusion
The current study reveals that stronger wave disturbance and greater sediment disturbance erode more seeds from the seed bank at tidal flats, which advances the understanding of underlying mechanisms governing lateral dynamics of coastal wetlands. Given the critical role of seed colonization in lateral expansion of coastal vegetation and its sensitivity to environmental changes, the knowledge gained here enables more accurate predictions on how climatically driven environmental changes may alter the resilience and persistence of coastal foundation plants, with significant implications for nature-based solutions with coastal vegetation to mitigate climate change.
Data Availability Statement
The raw data supporting the conclusions of this article will be made available by the authors, without undue reservation.
Author Contributions
ZZ designed, carried out the experiment, analyzed the data, and wrote the initial version of the manuscript. All authors contributed to the improvement of the article and approved the submitted version.
Funding
ZZ, QZ, YC, and ZY were supported by the Program for Guangdong Introducing Innovative and Entrepreneurial Teams (2019ZT08L213) and the Key Special Project for Introduced Talents Team of Southern Marine Science and Engineering Guangdong Laboratory (Guangzhou) (GML2019ZD0403). TB was supported by the Perspectief Research Program “All-Risk” (project number P15-21B1), primarily financed by NWO Domain Applied and Engineering Sciences.
Conflict of Interest
The authors declare that the research was conducted in the absence of any commercial or financial relationships that could be construed as a potential conflict of interest.
Publisher’s Note
All claims expressed in this article are solely those of the authors and do not necessarily represent those of their affiliated organizations, or those of the publisher, the editors and the reviewers. Any product that may be evaluated in this article, or claim that may be made by its manufacturer, is not guaranteed or endorsed by the publisher.
Acknowledgments
We thank Lennart van IJzerloo, Jeroen van Dalen, Nanyang Chu, and Tao Hong for their assistances in the field.
Supplementary Material
The Supplementary Material for this article can be found online at: https://www.frontiersin.org/articles/10.3389/fmars.2021.728065/full#supplementary-material
Footnotes
References
Andersen, T. J., Lanuru, M., van Bernem, C., Pejrup, M., and Riethmueller, R. (2010). Erodibility of a mixed mudflat dominated by microphytobenthos and Cerastoderma edule, East Frisian Wadden Sea, Germany. Estuar. Coast. Shelf Sci. 87, 197–206. doi: 10.1016/j.ecss.2009.10.014
Ayres, D. R., Zaremba, K., Sloop, C. M., and Strong, D. R. (2008). Sexual reproduction of cordgrass hybrids (Spartina foliosa x alterniflora) invading tidal marshes in San Francisco Bay. Divers. Distrib. 14, 187–195. doi: 10.1111/j.1472-4642.2007.00414.x
Baeyens, W., van Eck, B., Lambert, C., Wollast, R., and Goeyens, L. (1998). General description of the Scheldt estuary. Hydrobiologia 366, 1–14. doi: 10.1007/978-94-017-3573-5_1
Balke, T., Bouma, T. J., Horstman, E. M., Webb, E. L., Erftemeijer, P. L. A., and Herman, P. M. J. (2011). Windows of opportunity: thresholds to mangrove seedling establishment on tidal flats. Mar. Ecol. Prog. Ser. 440, 1–9. doi: 10.3354/meps09364
Balke, T., Herman, P. M., and Bouma, T. J. (2014). Critical transitions in disturbance-driven ecosystems: identifying Windows of Opportunity for recovery. J. Ecol. 102, 700–708. doi: 10.1111/1365-2745.12241
Balke, T., Webb, E. L., van den Elzen, E., Galli, D., Herman, P. M. J., and Bouma, T. J. (2013). Seedling establishment in a dynamic sedimentary environment: a conceptual framework using mangroves. J. Appl. Ecol. 50, 740–747. doi: 10.1111/1365-2664.12067
Beukema, J. J., Flach, E. C., Dekker, R., and Starink, M. (1999). A long-term study of the recovery of the macrozoobenthos on large defaunated plots on a tidal flat in the Wadden Sea. J. Sea Res. 42, 235–254. doi: 10.1016/s1385-1101(99)00027-1
Blackburn, N. J., and Orth, R. J. (2013). Seed burial in eelgrass Zostera marina: the role of infauna. Mar. Ecol. Prog. Ser. 474, 135–145. doi: 10.3354/meps10103
Bortolus, A., Adam, P., Adams, J. B., Ainouche, M. L., Ayres, D., Bertness, M. D., et al. (2019). Supporting Spartina: interdisciplinary perspective shows Spartina as a distinct solid genus. Ecology 100:e02863.
Bouma, T. J., Friedrichs, M., Klaassen, P., van Wesenbeeck, B. K., Brun, F. G., Temmerman, S., et al. (2009). Effects of shoot stiffness, shoot size and current velocity on scouring sediment from around seedlings and propagules. Mar. Ecol. Prog. Ser. 388, 293–297. doi: 10.3354/meps08130
Bouma, T. J., van Belzen, J., Balke, T., van Dalen, J., Klaassen, P., Hartog, A. M., et al. (2016). Short-term mudflat dynamics drive long-term cyclic salt marsh dynamics. Limnol. Oceanogr. 61, 2261–2275. doi: 10.1002/lno.10374
Callaghan, D. P., Bouma, T. J., Klaassen, P., van der Wal, D., Stive, M. J. F., and Herman, P. M. J. (2010). Hydrodynamic forcing on salt-marsh development: distinguishing the relative importance of waves and tidal flows. Estuar. Coast. Shelf Sci. 89, 73–88. doi: 10.1016/j.ecss.2010.05.013
Chang, E. R., Veeneklaas, R. M., Buitenwerf, R., Bakker, J. P., and Bouma, T. J. (2008). To move or not to move: determinants of seed retention in a tidal marsh. Funct. Ecol. 22, 720–727. doi: 10.1111/j.1365-2435.2008.01434.x
Cheong, S.-M., Silliman, B., Wong, P. P., van Wesenbeeck, B., Kim, C.-K., and Guannel, G. (2013). Coastal adaptation with ecological engineering. Nat. Clim. Chang. 3, 787–791. doi: 10.1038/nclimate1854
Christianen, M. J. A., van Belzen, J., Herman, P. M. J., van Katwijk, M. M., Lamers, L. P. M., van Leent, P. J. M., et al. (2013). Low-Canopy Seagrass Beds Still Provide Important Coastal Protection Services. PLoS One 8:e62413. doi: 10.1371/journal.pone.0062413
Costanza, R., d’Arge, R., de Groot, R., Farberk, S., Grasso, M., Hannon, B., et al. (1997). The value of the world’s ecosystem services and natural capital. Nature 387:253.
Cozzoli, F., Eelkema, M., Bouma, T. J., Ysebaert, T., Escaravage, V., and Herman, P. M. J. (2014). A mixed modeling approach to predict the effect of environmental modification on species distributions. PLoS One 9:e89131. doi: 10.1371/journal.pone.0089131
Delefosse, M., and Kristensen, E. (2012). Burial of Zostera marina seeds in sediment inhabited by three polychaetes: laboratory and field studies. J. Sea Res. 71, 41–49. doi: 10.1016/j.seares.2012.04.006
Donat, M., Renggli, D., Wild, S., Alexander, L., Leckebusch, G., and Ulbrich, U. (2011). Reanalysis suggests long-term upward trends in European storminess since 1871. Geophys. Res. Lett. 38:L14703.
Fagherazzi, S., Kirwan, M. L., Mudd, S. M., Guntenspergen, G. R., Temmerman, S., D’Alpaos, A., et al. (2012). Numerical models of salt marsh evolution: ecological, geomorphic, and climatic factors. Rev. Geophys. 50:RG1002.
Friess, D. A., Krauss, K. W., Horstman, E. M., Balke, T., Bouma, T. J., Galli, D., et al. (2012). Are all intertidal wetlands naturally created equal? Bottlenecks, thresholds and knowledge gaps to mangrove and saltmarsh ecosystems. Biol. Rev. 87, 346–366. doi: 10.1111/j.1469-185x.2011.00198.x
Gedan, K. B., Kirwan, M. L., Wolanski, E., Barbier, E. B., and Silliman, B. R. (2011). The present and future role of coastal wetland vegetation in protecting shorelines: answering recent challenges to the paradigm. Clim. Change 106, 7–29. doi: 10.1007/s10584-010-0003-7
Gray, A. J., Marshall, D. F., and Raybould, A. F. (1991). A century of evolution in Spartina anglica. Adv. Ecol. Res. 21, 1–62. doi: 10.1016/s0065-2504(08)60096-3
Greve, T. M., Krause-Jensen, D., Rasmussen, M. B., and Christensen, P. B. (2005). Means of rapid eelgrass (Zostera marina L.) recolonisation in former dieback areas. Aquat. Bot. 82, 143–156. doi: 10.1016/j.aquabot.2005.03.004
Groenendijk, A. M. (1986). Establishment of a Spartina-anglica population on a tidal mudflat - A field experiment. J. Environ. Manage. 22, 1–12.
Hu, Z., Belzen, J., Wal, D., Balke, T., Wang, Z. B., Stive, M., et al. (2015). Windows of opportunity for salt marsh vegetation establishment on bare tidal flats: the importance of temporal and spatial variability in hydrodynamic forcing. J. Geophys. Res. Biogeosci. 120, 1450–1469. doi: 10.1002/2014jg002870
Huiskes, A. H. L., Koutstaal, B. P., Herman, P. M. J., Beeftink, W. G., Markusse, M. M., and Munck, W. D. (1995). Seed dispersal of halophytes in tidal salt marshes. J. Ecol. 83, 559–567. doi: 10.2307/2261624
Kirwan, M., and Temmerman, S. (2009). Coastal marsh response to historical and future sea-level acceleration. Quat. Sci. Rev. 28, 1801–1808. doi: 10.1016/j.quascirev.2009.02.022
Kirwan, M. L., and Megonigal, J. P. (2013). Tidal wetland stability in the face of human impacts and sea-level rise. Nature 504, 53–60. doi: 10.1038/nature12856
Koch, E. W., Ailstock, M. S., Booth, D. M., Shafer, D. J., and Magoun, A. D. (2010). The role of currents and waves in the dispersal of submersed angiosperm seeds and seedlings. Restor. Ecol. 18, 584–595. doi: 10.1111/j.1526-100x.2010.00698.x
Liu, W., Strong, D. R., Pennings, S. C., and Zhang, Y. (2017). Provenance-by-environment interaction of reproductive traits in the invasion of Spartina alterniflora in China. Ecology 98, 1591–1599. doi: 10.1002/ecy.1815
Lovelock, C., Sorrell, B., Hancock, N., Hua, Q., and Swales, A. (2010). Mangrove Forest and Soil Development on a Rapidly Accreting Shore in New Zealand. Ecosystems 13, 437–451. doi: 10.1007/s10021-010-9329-2
Marani, M., D’Alpaos, A., Lanzoni, S., and Santalucia, M. (2011). Understanding and predicting wave erosion of marsh edges. Geophys. Res. Lett. 38:L21401.
Marion, S. R., and Orth, R. J. (2012). Seedling establishment in eelgrass: seed burial effects on winter losses of developing seedlings. Mar. Ecol. Prog. Ser. 448, 197–207. doi: 10.3354/meps09612
Meysman, F. J. R., Middelburg, J. J., and Heip, C. H. R. (2006). Bioturbation: a fresh look at Darwin’s last idea. Trends Ecol. Evol. 21, 688–695. doi: 10.1016/j.tree.2006.08.002
Moller, I., Kudella, M., Rupprecht, F., Spencer, T., Paul, M., van Wesenbeeck, B. K., et al. (2014). Wave attenuation over coastal salt marshes under storm surge conditions. Nat. Geosci. 7, 727–731. doi: 10.1038/ngeo2251
Sistermans, P., and O. Nieuwenhuis. (2019). Western Scheldt Estuary (The Netherlands). EUROSION Case Study. Available online at: http://databases.eucc-d.de/plugins/projectsdb/project.php?show=380
Stal, L. J. (2003). Microphytobenthos, their extracellular polymeric substances, and the morphogenesis of intertidal sediments. Geomicrobiol. J. 20, 463–478. doi: 10.1080/713851126
Strong, D., and Ayres, D. (2009). Spartina introductions and consequences in salt marshes.) Human impacts on salt marshes: a global perspective. London: University of California Press Ltd.
Strong, D. R., and Ayres, D. R. (2013). Ecological and evolutionary misadventures of Spartina. Annu. Rev. Ecol. Evol. Syst. 44, 389–410. doi: 10.1146/annurev-ecolsys-110512-135803
Suykerbuyk, W., Bouma, T. J., Govers, L. L., Giesen, K., de Jong, D. J., Herman, P., et al. (2016). Surviving in changing seascapes: sediment dynamics as bottleneck for long-term seagrass presence. Ecosystems 19, 296–310. doi: 10.1007/s10021-015-9932-3
Temmerman, S., Bouma, T., Van de Koppel, J., Van der Wal, D., De Vries, M., and Herman, P. (2007). Vegetation causes channel erosion in a tidal landscape. Geology 35, 631–634. doi: 10.1130/g23502a.1
Temmerman, S., Meire, P., Bouma, T. J., Herman, P. M. J., Ysebaert, T., and De Vriend, H. J. (2013). Ecosystem-based coastal defence in the face of global change. Nature 504, 79–83. doi: 10.1038/nature12859
Van Colen, C., Montserrat, F., Vincx, M., Herman, P. M. J., Ysebaert, T., and Degraer, S. (2008). Macrobenthic recovery from hypoxia in an estuarine tidal mudflat. Mar. Ecol. Prog. Ser. 372, 31–42. doi: 10.3354/meps07640
van de Koppel, J., van der Wal, D., Bakker, J. P., and Herman, P. M. (2005). Self-organization and vegetation collapse in salt marsh ecosystems. Am. Nat. 165, E1–E12.
van der Wal, D., Wielemaker-Van den Dool, A., and Herman, P. M. J. (2008). Spatial patterns, rates and mechanisms of saltmarsh cycles (Westerschelde, The Netherlands). Estuar. Coast. Shelf Sci. 76, 357–368. doi: 10.1016/j.ecss.2007.07.017
Widdows, J., and Brinsley, M. (2002). Impact of biotic and abiotic processes on sediment dynamics and the consequences to the structure and functioning of the intertidal zone. J. Sea Res. 48, 143–156. doi: 10.1016/s1385-1101(02)00148-x
Wolters, M., and Bakker, J. P. (2002). Soil seed bank and driftline composition along a successional gradient on a temperate salt marsh. Appl. Veg. Sci. 5, 55–62. doi: 10.1111/j.1654-109x.2002.tb00535.x
Xiao, D., Zhang, L., and Zhu, Z. (2009). A study on seed characteristics and seed bank of Spartina alterniflora at saltmarshes in the Yangtze Estuary, China. Estuar. Coast. Shelf Sci. 83, 105–110. doi: 10.1016/j.ecss.2009.03.024
Young, I., Zieger, S., and Babanin, A. (2011). Global trends in wind speed and wave height. Science 332, 451–455. doi: 10.1126/science.1197219
Zhao, Z., Zhang, L., Li, X., Yuan, L., and Bouma, T. J. (2021). The onset of secondary seed dispersal is controlled by germination-features: a neglected process in sudden saltmarsh establishment. Limnol. Oceanogr. 66, 3070–3084. doi: 10.1002/lno.11860
Zhu, Q., Van Prooijen, B., Maan, D., Wang, Z., Yao, P., Daggers, T., et al. (2019). The heterogeneity of mudflat erodibility. Geomorphology 345:106834. doi: 10.1016/j.geomorph.2019.106834
Zhu, Z., Bouma, T. J., Ysebaert, T., Zhang, L., and Herman, P. M. J. (2014). Seed arrival and persistence at the tidal mudflat: identifying key processes for pioneer seedling establishment in salt marshes. Mar. Ecol. Prog. Ser. 513, 97–109. doi: 10.3354/meps10920
Zhu, Z., van Belzen, J., Hong, T., Kunihiro, T., Ysebaert, T., Herman, P. M. J., et al. (2016b). Sprouting as a gardening strategy to obtain superior supplementary food: evidence from a seed-caching marine worm. Ecology 97, 3278–3284. doi: 10.1002/ecy.1613
Zhu, Z., Cozzoli, F., Chu, N., Salvador, M., Ysebaert, T., Zhang, L., et al. (2016a). Interactive effects between physical forces and ecosystem engineers on seed burial: a case study using Spartina anglica. Oikos 125, 98–106. doi: 10.1111/oik.02340
Zhu, Z., Vuik, V., Visser, P. J., Soens, T., van Wesenbeeck, B., van de Koppel, J., et al. (2020b). Historic storms and the hidden value of coastal wetlands for nature-based flood defence. Nat. Sustain. 3, 853–862. doi: 10.1038/s41893-020-0556-z
Keywords: waves, sediment disturbance, tidal flats, seed bank, persistence
Citation: Zhu Z, Bouma TJ, Zhu Q, Cai Y and Yang Z (2021) Effects of Waves and Sediment Disturbance on Seed Bank Persistence at Tidal Flats. Front. Mar. Sci. 8:728065. doi: 10.3389/fmars.2021.728065
Received: 20 June 2021; Accepted: 01 October 2021;
Published: 20 October 2021.
Edited by:
Zheng Gong, Hohai University, ChinaReviewed by:
Peng Yao, Hohai University, ChinaYining Chen, Second Institute of Oceanography, Ministry of Natural Resources, China
Copyright © 2021 Zhu, Bouma, Zhu, Cai and Yang. This is an open-access article distributed under the terms of the Creative Commons Attribution License (CC BY). The use, distribution or reproduction in other forums is permitted, provided the original author(s) and the copyright owner(s) are credited and that the original publication in this journal is cited, in accordance with accepted academic practice. No use, distribution or reproduction is permitted which does not comply with these terms.
*Correspondence: Zhifeng Yang, emZ5YW5nQGdkdXQuZWR1LmNu