- 1School of the Environment, Geography and Geosciences (SEGG), University of Portsmouth, Portsmouth, United Kingdom
- 2Mediterranean Institute for Advanced Studies (IMEDEA), Spanish National Research Council-University of the Balearic Islands (CSIC-UIB), Esporles, Spain
Seagrass species play a critical role in the mitigation of climate change by acting as valuable carbon sinks and storage sites. Another important ecosystem service of this coastal vegetation is nutrient removal. However, coastal ecosystems are under increasing pressure of global warming and associated establishment of invasive species. To elucidate the respective contributions of seagrass species Posidonia oceanica and Cymodocea nodosa and the non-native macroalga Halimeda incrassata as primary producers and nutrient sinks in coastal habitats we conducted in-situ incubations in the North-western Mediterranean Sea. Measured metabolic activity and nutrient removal as well as calcification rates in these habitats over a 24 h period in spring and summer confirmed that the endemic seagrass P. oceanica represents a valuable ecosystem with high O2 production and considerable carbon capture. The documented regression of P. oceanica meadows with higher temperatures and decline in autotrophy as measured here causes concern for the continuity of ecosystem services rendered by this habitat throughout the Mediterranean Sea with progressing climate warming. In contrast, the enhanced performance of C. nodosa and the calcifying alga H. incrassata with increasing temperatures, under expected rates of future warming is uncertain to mitigate loss of productivity in case of a potential shift in marine vegetation. This could ultimately lead to a decline in ecosystem services, decreased carbon storage and mitigation of climate change. Furthermore, this study provides a first estimate for the growth rate of H. incrassata in the Mediterranean Sea, supporting evidence for the mechanism of its rapid extension.
Introduction
Ever since the 18th century, humanity has caused raised and still increasing emission of greenhouse gases (GHGs), mainly carbon dioxide (CO2) with annual averages of 410 ppm in 2019 (IPCC, 2021) and predicted CO2 levels four times higher compared to pre-industrial (Wigley, 1983) levels (IPCC, 2021). Global warming, including the rise of ocean temperature, is the most direct consequence of increasing CO2 levels in the atmosphere. The ocean plays a relevant role mitigating the CO2 rise in the atmosphere through the absorption of around a third of the anthropogenic emissions (Gruber et al., 2019). This CO2 uptake impacts the chemical balance of seawater by increasing bicarbonate ion concentration (HCO3–) and reducing the availability of carbonate ions (CO23–) by 60% (Feely et al., 2004). This shift in water chemistry results in a decrease of seawater pH, a process referred to as Ocean Acidification (OA; Doney et al., 2009). In fact, sea surface pH is expected to decrease from 0.3 to 0.5 units by the end of this century (Caldeira and Wickett, 2003). Ocean acidification is a global phenomenon, impacting calcification and metabolism of organisms, which has been documented in many marine regions (Kroeker et al., 2013).
The Mediterranean Sea is strongly affected by climate change and is among the marine regions with the highest rates of warming, from two to four times higher than in other ocean regions (Vargas-Yáñez et al., 2010; Burrows et al., 2011). Despite its high productivity, the semi-enclosed Mediterranean Sea is most likely to be more impacted by climate change than other seas (Richon et al., 2019), due to its physical properties with a short mixing and water exchange period of around 70 years (Durrieu de Madron et al., 2011). The Mediterranean Sea is therefore defined as a “hotspot for climate change” (Giorgi, 2006), with additional disturbances interacting in this region, both natural and anthropogenic (Lejeusne et al., 2010). Natural disturbances such as more extreme and frequent meteorological events (Parry, 2000; IPCC, 2013) and anthropogenic disturbances due to progressing urbanization, habitat destruction, pollution, overfishing, and species introduction induce additional pressure on the marine ecosystems (Lejeusne et al., 2010). The intrusion of invasive species into the Mediterranean has dramatically increased due to growing aquatic trade, aquaculture, maritime traffic (Raitsos et al., 2010) and most severe, the opening of the Suez Canal in 1869 and its latest widening in 2015 (Galil et al., 2015). More than 600 alien species have already established in the Mediterranean (Zenetos et al., 2017) and this number is expected to increase by 2050 (Sardain et al., 2019).
Although seagrasses occupy only a 0.1% of the ocean surface and their extension is limited to the coastal regions of temperate, sub- and tropical waters (Kennedy et al., 2010), their communities are among the most productive marine ecosystems (Duarte and Chiscano, 1999), modulate biogeochemical processes (Duarte, 1999), and provide valuable ecosystem functions (Costanza et al., 1997). Seagrass meadows form dense meadows providing food, habitat, and nursery grounds for various species (Duarte, 2002) and contribute to the physical protection of the coastal zone by providing wave attenuation and forming dense rhizomes below-ground stabilizing the sediment, thus preventing coastal erosion (Costanza et al., 1997; Fourqurean et al., 2012). Furthermore, they eliminate nutrients from the water column (Barry et al., 2013; Grall and Chauvaud, 2017), P. oceanica meadows are known to be net sinks for Nitrate and Phosphate (Barrón and Duarte, 2009) and contribute greatly to ecosystem resistance to eutrophication (Duarte, 1995; Lloret et al., 2008). Seagrasses have also demonstrated a high capacity of capturing CO2 (Duarte et al., 2010, 2013), accounting for 20% of the global marine carbon sequestration (Duarte et al., 2013; Duarte and Krause-Jensen, 2017) and are referred to as blue carbon habitats (Macreadie et al., 2019). Seagrass meadows are mainly autotrophic, their productivity exceeds their carbon needs and they can export 24.3% of their production to adjacent ecosystems (Duarte, 2002), or even up to 50–70% (Kennedy et al., 2010). By removing carbon, seagrass canopies also locally mitigate the effects of OA during the daytime, acting as a buffer increasing pH in their canopies (Hendriks et al., 2014).
However, seagrass meadows are susceptible to various threats to their habitat, and face most of the serious threats to marine ecosystems: physical modifications by human activity, decreasing water quality, pressure from invasive species and climate change (Waycott et al., 2009). The Western Mediterranean basin accounts for a total of 510,715 ha of Posidonia oceanica meadows (Telesca et al., 2015), the endemic seagrass species in the Mediterranean Sea, which represents the major coastal ecosystem of the Balearic Islands. Posidonia oceanica is distributed throughout the temperate Mediterranean Sea and is impacted by warming since elevated temperatures cause physiological stress and shoot mortality increases (Diaz-Almela et al., 2007; Marbà and Duarte, 2010). The slow-growing P. oceanica is prone to show a stronger negative response to higher temperatures, due to a low thermal tolerance with optimal temperatures for growth of 15.5–18°C (Olsen et al., 2012), although average optimum temperatures for photosynthesis are typically higher (30–32°C; Lee et al., 2007). More temperature tolerant species such as Cymodocea nodosa, a seagrass of tropical origin has a higher optimal temperature for growth (24.5°C) than the temperate P. oceanica (Olsen et al., 2012) and appears to be better adapted to increasing water temperature (Lee et al., 2007; Olsen et al., 2012; Savva et al., 2018). The tropical green alga Halimeda incrassata was reported for the first time in 2011 in Palma Bay (Balearic Islands), introduced most likely by recreational boats (Alós et al., 2016). The alga found favoring conditions to expand rapidly, forming dense meadows over 41% of the monitored area in Palma Bay between 2011 and 2015 (Alós et al., 2016). Still, to the best of our knowledge, a specific growth rate has not been estimated. Benefitting from increasing water temperatures, H. incrassata is more likely physiologically affected by water with a lower pH and less calcium carbonate available for calcification, than increasing temperatures, although through the link between photosynthesis and energy compensation calcification might not be impacted directly (Hofmann and Bischof, 2014). Gradually increasing temperatures with more frequent heatwaves are expected to severely impact the persistence of temperate species with low thermal tolerance (Short and Neckles, 1999; Jordà et al., 2012). Changes in the composition of marine vegetation and structure of seagrass meadows and hence changes in the metabolic activity of these habitats directly affect biogeochemical processes of the coastal zone, impacting the carbon sequestration capacity and the oxygen dynamics, thereby impairing the functioning of these coastal ecosystems (Sand-Jensen and Borum, 1991; Duarte, 1995). These ecosystems are heavily impacted by global warming and increased presence of exotic species (Han and Liu, 2014) and loss of seagrass meadows increases the risk of erosion of historically deposited carbon, releasing it from the sediment and emitting it to the atmosphere (Marbà et al., 2015).
Here we evaluate how three Mediterranean macrophyte species contribute to biogeochemical processes in the coastal zone by evaluating their metabolic activity during the most productive period of the year. We conduct field incubations to estimate oxygen production, directly linked to carbon consumption, and nutrient use comparing seagrass species P. oceanica and C. nodosa and the non-indigenous calcifying alga H. incrassata. We chose to evaluate two distinct time points during growth season, one in spring and one in summer, with expected differences in productivity peaks between the three species and within their preferred temperature optima. The western Mediterranean Sea shows a strong seasonality in temperature and we chose April, when temperatures start to rise and H. incrassata was observed entering an active state while spring is known as the most productive time for P. oceanica, and July, when temperatures start to reach the annual maxima. Additionally, to estimate a growth rate for the tropical H. incrassata, that can serve as an indicator of the mechanism for its rapid expansion in the Mediterranean Sea it is crucial to include the actual growth period. Possible shifts in community composition favoring species more tolerant to warmer temperatures will change the impact of coastal marine vegetation in the Mediterranean Sea on the carbon cycle and nutrient uptake. Our comparison between three macrophyte species with contrasting future trajectories highlights the importance of these vital coastal ecosystems in climate change mitigation and can help to predict changes we might expect in the future.
Materials and Methods
Study Site
The study site at Punta Negra (39.5266°N, 2.5515°E), a small bay on the south coast of Mallorca (NW Mediterranean, see Supplementary Figure 1) with water depths ranging from 2 to 6 m has no direct inflow of sewage waters. The site represents the predominant coastal setting in the Mediterranean Sea and was chosen because of its accessibility and presence of all three study species. The site is dominated by P. oceanica meadows, with meadows of C. nodosa and H. incrassata present on sand and death matte of P. oceanica. For on-site monitoring, a temperature and light logger (HOBO Pendant UA-002-64; Onset, United States) was installed inside a H. incrassata patch on February 12th, 2019 at 2.8 m depth, recording data hourly. Light intensity (PAR, Table 1) was converted from the HOBOs values in Lux [lum/ft2] to μmol photons/m2/s according to a previous calibration with a LiCOR light sensor (LI-1400, LI-COR Biosciences, United States) under laboratory conditions as photons = 0.1 LUX + 2.9. With the PAR values, the daily light integral (DLI) was calculated in mol photons/m2/d (Table 1). This sensor was left on-site from February to the end of the experiment. Additional HOBO sensors were installed attached to multiparametric sensors measuring watercolumn values in each habitat at max. 1 m distance from the benthic incubations (see “Field Incubations”) for the duration of the incubations. The distance between different macrophyte habitats was between 5 and 10 m.
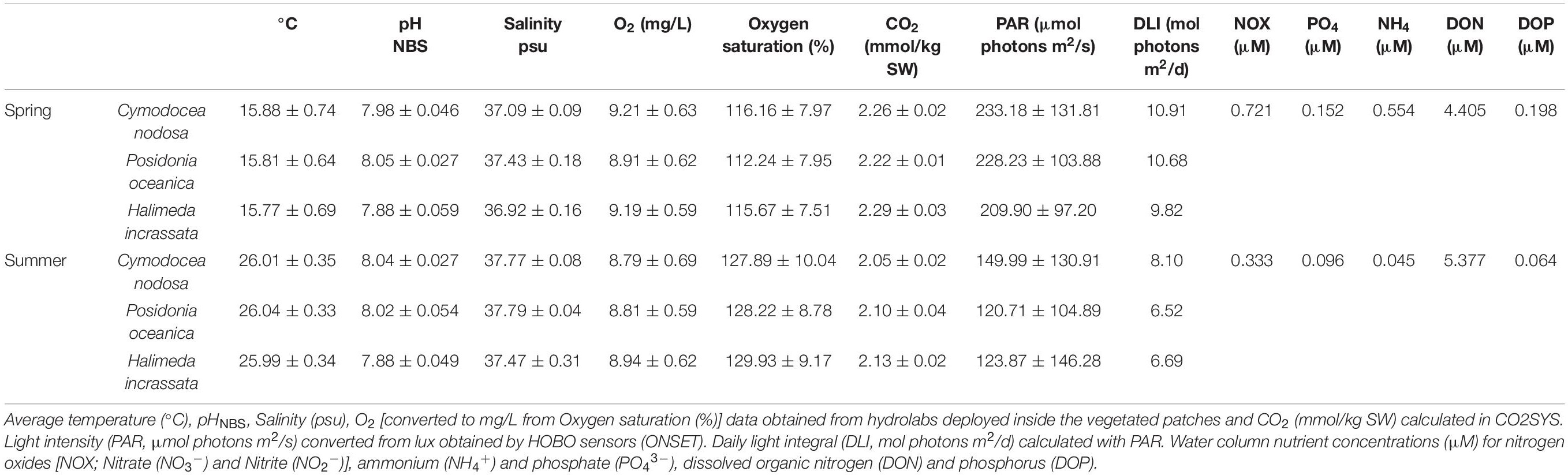
Table 1. Field conditions during incubations at Punta Negra, conducted in April (11–12th) and in July (2–3rd) 2019.
Incremental Growth Rate
The growth rate of H. incrassata was estimated with the Alizarin Red-S dyeing technique (Dustan, 1975; Wefer, 1980; Multer, 1988), adjusted for H. incrassata according to Payri (1988). Two aquaria (92 L Volume) were deployed upside down on two patches of H. incrassata (March 25th to 27th, 2019) and a concentrated solution of Alizarin Red-S was injected at sunrise during two consecutive days, for maximum incorporation during daylight. The aquaria were removed at sunset to ensure recirculating of the water column and prevent hypoxic conditions. This led to an effective staining period of 24 h.
Field Incubations
We targeted the most productive season for seagrass productivity (spring) and the subsequent summer for field incubations to facilitate a comparison between species as Halimeda incrassata is not active during colder periods. During April (11th–12th) and July (2nd–3rd) 2019, we conducted closed in-situ incubations in patches of P. oceanica, C. nodosa, and H. incrassata, each for 24 h. Simultaneously, Hydrolabs (HL4; OTT HydroMet) were installed in each habitat at max. 1 m distance from the incubations, to record temperature, pH, conductivity, depth, and oxygen (O2) to get a record of oxygen dynamics in the water column (Table 1). For additional calculations of metabolism based on open water oxygen profiles measured with the multiparametric sensors, we considered mixing depth and atmosphere-sea interactions according to Cole et al. (2000), using a modification of a program originally written by Coloso et al. (2008), implemented in MATLAB (v.R2010b, the Mathworks Inc.).
For each habitat type, three replicate incubations were set up by SCUBA-divers. PVC-rings (Height: 0.2 m; Radius: 0.09 m) were hammered into the seafloor, allowing for intact patches of macrophytes in the center. Translucent incubation bags (coextruded high barrier multilayer film PA/EVOH/PA/PE), which did not permit gas exchange between the chamber and the water column, with a three-way valve (luer-lock) for sampling were fitted over the rings and fixed with elastic bands. Additionally, planktonic incubations to measure water column metabolic activity in dark and light periods were set up in opaque and translucent glass bottles. Initial samples were collected at sunset from the water column at the start of the incubations for total alkalinity (TA), O2, and nutrients. During subsequent dives at sunrise and again at sunset, samples for TA, O2 and nutrients were taken from all individual incubation bags using 50-ml (polyethylene) acid-washed syringes. Samples taken at sunrise were compared with the first sunset samples to account for the night (period of 11 h in April, 9 h in July), samples at sunset account for the day (period of 13 and 15 h, respectively). O2 samples were fixed immediately on site according to the protocols by Carpenter (1965) and Labasque (2004) and samples for nutrients were filtered over GF/F filters and stored frozen at −20°C until further analysis. TA samples were routinely poisoned with mercury (II) chloride (HgCl2).
Sample Processing and Analyses
Biomass Analysis
After each incubation period, macrophyte material from within the incubation rings was collected. The vegetation was rinsed, dried for 24 h at 60°C to obtain the dry weight and then divided into above and below ground biomass.
Incremental Growth Rate
The site of H. incrassata dyed with Alizarin Red-S was not selected for the incubations but collected separately, 99 days after in-situ staining. Algae were rinsed and placed for 30 min into a 5% Sodium Hypochlorite (NaClO) solution for bleaching. The growth rate was calculated as the ratio of new segments after staining and the sum of all segments (Wefer, 1980). With the days between dyeing H. incrassata until harvesting the biomass, the number of segments produced and the percental increase in segment number per day was calculated.
Analyses of Water Chemistry
Oxygen samples were analyzed by the spectrophotometric Winkler method following recommendations by Labasque (2004) in a spectrometer (Spectronic Helios alpha; ThermoFisher Scientific, United States) at 466 nm and calibrated using a 5-point calibration with Potassium iodide (KIO3) as coloring reagent.
Total Alkalinity was analyzed by open cell potentiometric titration with a Titrando 808 (Metrohm) following the Standard Operation Procedure (SOP) 3b (Dickson et al., 2007). We used certified CO2 seawater reference material (CRM Batch #136) from Prof. A. Dickson at Scripps Institution of Oceanography (United States) to warrant the quality of the analysis, which had a precision of 4.4 μeq/Kg and an accuracy of <1 μeq/Kg.
With TA and in-situ measured values of salinity, temperature and pH, the CO2-concentration was calculated with the CO2SYS software (Pierrot et al., 2006) using pH values in NBS scale (mol/kg H2O), K1 and K2 constants from Mehrbach et al. (1973) refit by Dickson and Millero (1987) and the KSO4 constant after Dickson (1990).
Nutrient samples were analyzed by continuous flow analysis with the Autoanalyser AA3 HR (Seal Analytical, United Kingdom) at the Mediterranean Center for Marine and Environmental Research (CMIMA, Barcelona, Spain).
Metabolism
To evaluate the metabolism of the enclosed community, Net community production (NCP), community respiration (CR), and gross primary production (GPP) were calculated from changes in oxygen concentrations between dark and light periods. The O2− measurements of the planktonic incubations are used to correct the total measured metabolic activity in the benthic incubations for the metabolic activity in the water column. Also, metabolism in the vegetated incubations was corrected for average values from incubations containing only bare sediment, to account for respiration or production by the sediment itself. CR was calculated as the change in O2 overnight and NCP was calculated as the overall change in O2 over the incubation period of 24 h. Under the assumption that CR is equal during night and day, GPP was estimated as the difference between NCP and CR. The rates were then corrected for the hours of day light in situ, to obtain metabolism per time unit (h). Then, NCP, CR, and GPP were corrected for the area and volume of each incubation setup, as estimated by injections of 5-ml of a 0.25 mol/L phosphate solution in each bag after the incubations and calculating its dilution after spectrophotometric determination (Hansen and Koroleff, 1999). Finally, we normalized the results by dry weight of the macrophyte material to obtain a final concentration of O2 in mmol/d/gr. DW.
Calcification Rate
We approached the net calcification rate (g) by applying the alkalinity anomaly technique to derive the net calcification of the community under the assumption that only CaCO3 incorporation or dissolution influences TA (Smith and Key, 1975). The difference between pre- and post-incubation TA equals ΔTA in μmol/kg, which then was converted into mmol/kg. The net calcification rate g (mmol CaCO3/unit time) was then calculated by using following formula:
With ρSW (density of Seawater) as 1.03 kg/m3 and ΔT the time (h) between time periods. We calculated ΔTA for two time periods: ΔTA between initial water samples (sunset) and samples collected at sunrise and between sunrise and samples collected at sunset. After correction for the hours between sampling this permits to investigate whether calcification takes place mostly at night or during the daytime.
Nutrients
Nutrient utilization was evaluated by calculating the changes in nutrient concentration between time periods. Nutrient usage over night was calculated by subtracting the results from samples taken at sunrise from the initial water column samples. Nutrient usage during daylight was calculated by subtracting the nutrient concentration from samples taken at sunset from samples taken at sunrise. Nutrient concentrations in the vegetated incubations were corrected for average values from incubations containing only bare sediment, to account any retention or production by the sediment. A reduction of nutrients in the water column indicates a depletion by the macrophyte, whereas an increase in nutrient concentration within the benthic chamber indicates that the habitat released nutrients.
Statistical Analysis
Two-way ANOVA and Tukey HSD tests were used to distinguish significant differences between macrophyte species and respective incubation setting in spring or summer on the response variables (NCP, CR, GPP, and g). The statistical analyses were conducted in Rstudio (version 1.1.456; RS Team, 2015) using the R platform (R Core Team, 2021).
Results
Field Incubations
There were no strong environmental differences between habitats (Table 1), as water temperature was similar for all locations, with pronounced differences between day and night due to the limited depth and influence of irradiance during the day with temperatures ranging from 16.21 to 17.38°C during the day and 15.09–15.95°C at night in spring and 26.5–27.12°C during the day and between 25.68 and 25.9°C at night and in summer. Salinity values remained similar for all habitats and between seasons, ranging between 36.92 and 37.97 psu.
The growth rate of H. incrassata was of 0.93 newly produced segments per day during 99 days after staining, which accounts for a percental increase in segment number per day of 0.9207. This growth rate does not account for an annual growth rate, but as a mechanism for the establishment and expansion of H. incrassata. The time period investigated was from early spring, when temperatures rose and H. incrassata was observed to enter its active state, to summer, when water temperatures approached annual temperature maxima in the Mediterranean Sea, therefore spanning across the crucial period for the success of colonization of H. incrassata.
Metabolism
Metabolic rates, CR, GPP, and NCP confirmed P. oceanica to be a highly productive habitat (Figure 1), with the highest O2 turnover rates of all habitats. Both, CR [F(2,2) = 28.24] and GPP [F(2,2) = 35.45] are significantly higher than for C. nodosa and H. incrassata (p < 0.05), although NCP showed no significant difference between species (p = 0.445). No significant difference was observed between seasons (p = 0.210 for CR, p = 0.099 for GPP and p = 0.266 for NCP). However, P. oceanica showed increased respiration activity (Δ5.322 mmol O2/d/m2) and increased GPP (Δ3.042 mmol O2/d/m2) in summer and decreased NCP of the habitat (Δ1.657 mmol O2/d/m2). In contrast, C. nodosa and H. incrassata showed increased NCP in summer of Δ10.266 and Δ8.284 mmol O2/d/m2, respectively, due to an increase in GPP (Δ9.311 and Δ12.815 mmol O2/d/m2). Calcification rate for C. nodosa decreased by Δ0.332 mmol O2/d/m2, whereas for H. incrassata CR increased by Δ5.155 mmol O2/d/m2.
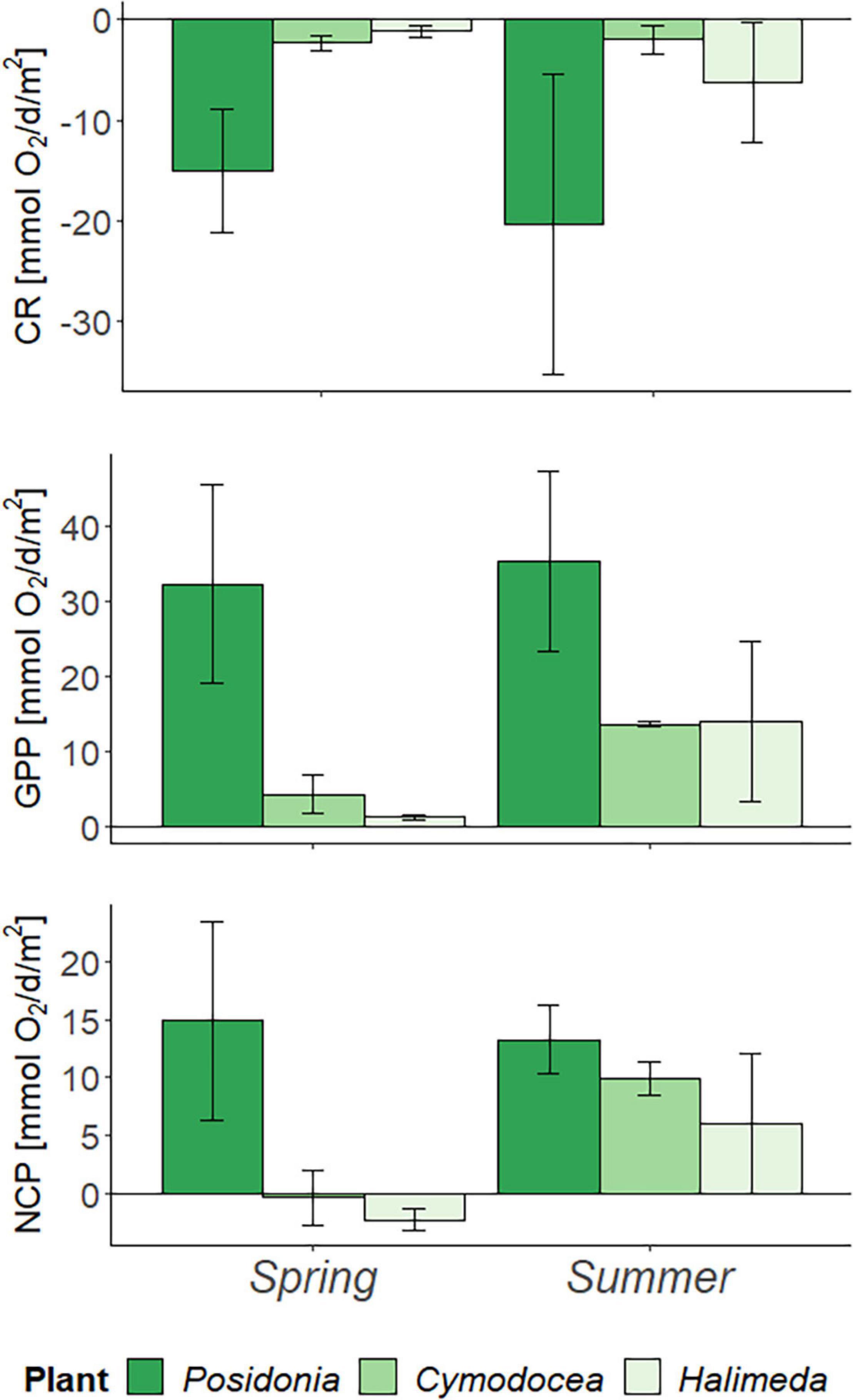
Figure 1. Metabolic rates per day (d) and square meter (m2) in spring (April) and summer (July) 2019 measured during in-situ incubations, corrected for biomass and bare sediment. Community Respiration (CR), Gross Primary Production (GPP), and Net Community Production (NCP) in mmol O2/d/m2, with error bars indicating standard deviation (SD).
A normalization of the results per gram dry weight suggests that in fact C. nodosa has the highest O2 turnover rates per biomass unit (Figure 2). Although no statistical significance between species nor season (p > 0.05) was found, P. oceanica showed less productivity in summer with a decrease in NCP of Δ0.012 mmol O2/d/gr. DW, whereas C. nodosa and H. incrassata showed increasing NCP of Δ0.149 mmol O2/d/gr. DW and Δ0.033 mmol O2/d/gr. DW, respectively. Similar as for the results per m2, CR in C. nodosa decreased in summer (Δ0.077 mmol O2/d/gr. DW) and increased in H. incrassata (Δ0.018 mmol O2/d/gr. DW).
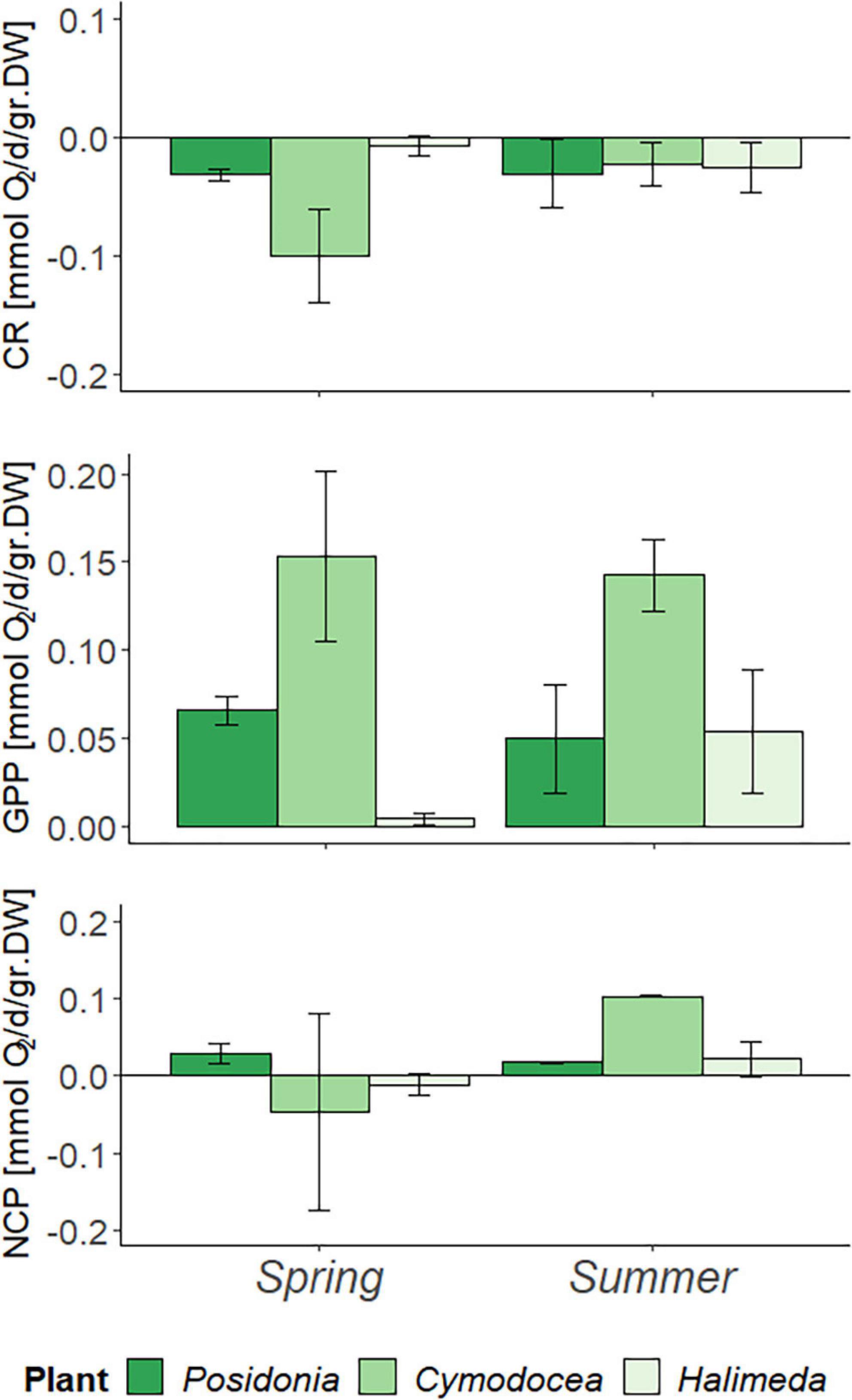
Figure 2. Metabolic rates per day (d) in spring (April) and summer (July) 2019 measured during the in-situ incubations, corrected for biomass (per gram Dry weight; gr. DW) and bare sediment. Community respiration, GPP, and NCP in mmol O2/d/gr. DW, with error bars indicating SD.
Multiparametric Sensors
The whole ecosystem metabolic rates calculated with oxygen data from three multiparametric dataloggers measuring in the water column (Supplementary Figure 2) did not allow for distinction of species-specific productivity even if they were situated in distinct habitats (p > 0.05). The net ecosystem metabolic rates were considerably higher than those obtained from enclosed habitats in the benthic chambers, compared to the adjacent sensor with CR [F(1,2) = 1117 in spring, and F(1,2) = 893.8 in summer, p < 0.001 in both seasons] and GPP [F(1,2) = 266.7, p < 0.01 in spring, F(1,2) = 1149 in summer, p < 0.001]. While whole ecosystem NCP was not different from the incubation values in spring [F(1,2) = 587.0, p = 0.124], in summer a significant increase in whole ecosystem NCP in all habitats [F(1,2) = 61.825, p < 0.05] resulted in rates which were considerably higher than in the benthic chambers [F(1,2) = 1,393, p < 0.001].
Calcification Rate
Calcification rate (g) per time period differed greatly between the incubation bags and there was a high deviation from the averages per habitat (Figure 3). No significant difference was found between species [F(2,13) = 0.857, p = 0.447], nor between seasons [F(1,13) = 0.057, p = 0.814]. Posidonia oceanica showed the highest calcification rates at night, with 0.269 ± 0.56 mmol CaCO3/m2 (here and elsewhere: mean ± SD) in spring and 0.381 ± 0.07 mmol CaCO3/m2 in summer. Calcification seemed to occur mainly during day for both seagrass habitats (0.187 ± 0.17 mmol CaCO3/m2 for C. nodosa in summer), whereas both seagrass habitats seemed to release CaCO3 during night-time (0.291 ± 0.39 mmol CaCO3/m2 in P. oceanica and 0.029 ± 0.01 mmol CaCO3/m2 in C. nodosa).
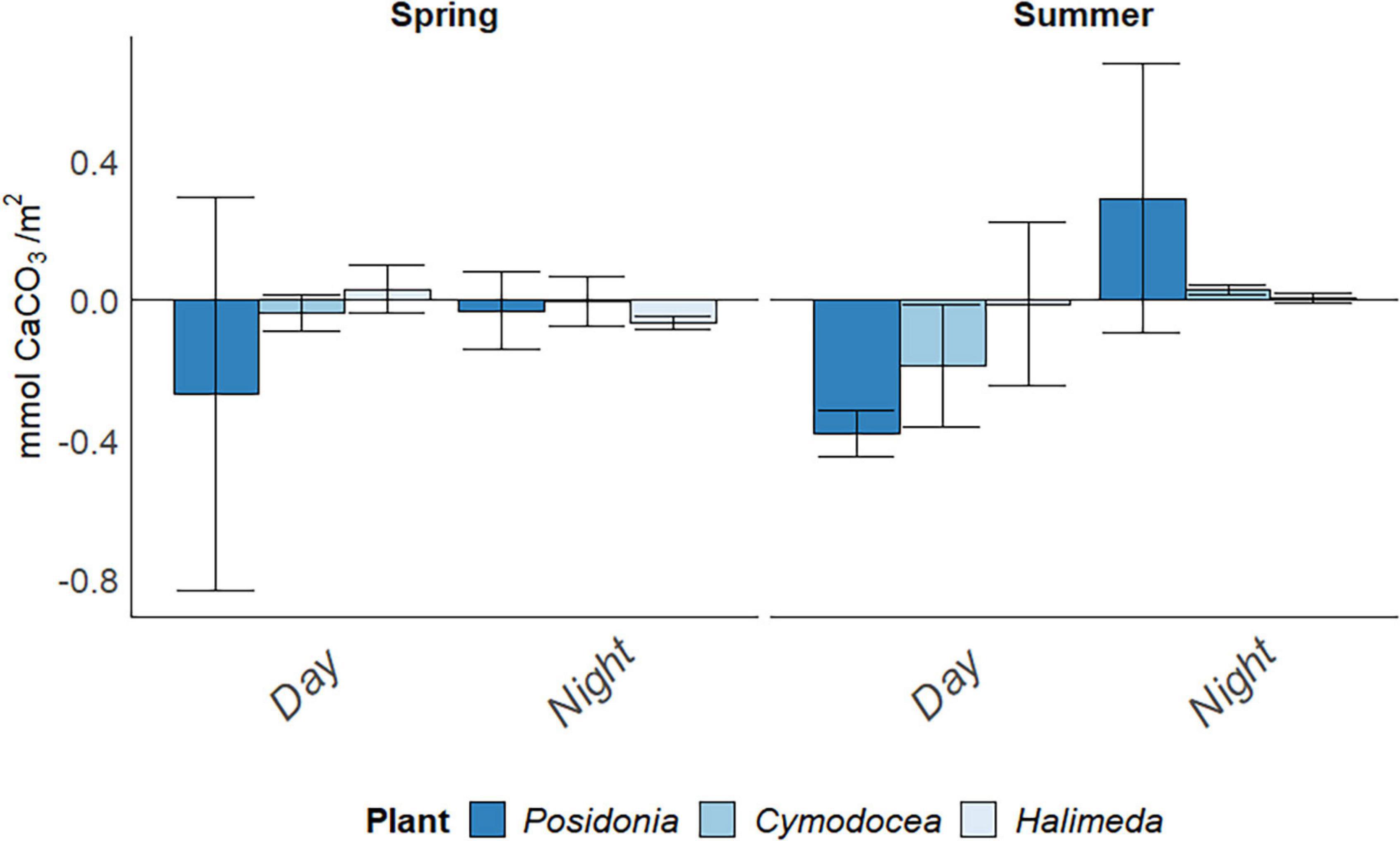
Figure 3. Daily Calcification rate (g) in mmol CaCO3/m2 in spring (April) and summer (July) 2019, divided into day and night periods. Error bars indicating SD.
Nutrients
Water column nutrient concentrations, as a measure for all three macrophyte habitat as the site is shallow and well-mixed, of nitrogen oxides [NOX; Nitrate (NO3–) and Nitrite (NO2–)], ammonium (NH4+) and phosphate (PO43–) were significantly higher (p < 0.05) in spring than in summer (Table 1). The utilization of NOX, phosphate and ammonium within the three different habitats also differed between seasons (Figure 4). In spring (Figure 4A), P. oceanica depleted inorganic nutrients throughout the incubation period, but at higher rates during night (4.055 ± 0.13 μM/m2 for NOX, 0.379 ± 0.41 μM/m2 of phosphate and 2.127 ± 1.01 μM/m2 for ammonium). Cymodocea nodosa showed a depletion of NOX (0.562 ± 0.26 μM/m2) and ammonium (0.372 ± 0.27 μM/m2) during night, but a release at higher rates during day (0.577 ± 0.28, 0.991 ± 0.64 μM/m2, respectively). Halimeda incrassata indicated the highest rates of releasing phosphate during the night (0.421 ± 0.28 μM/m2) and NOX during the day (1.061 ± 0.37 μM/m2), yet depleting ammonium at a considerable rate of 1.095 ± 0.95 μM/m2 during the day. In summer (Figure 4B), P. oceanica showed primarily a depletion of inorganic nutrients throughout the incubation period, yet at higher rates during night (1.593 μM/m2 for NOX and 1.411 μM/m2 for ammonium). Generally, rates for nutrient usage in P. oceanica were lower than in spring, whereas rates for C. nodosa were higher in summer. Cymodocea nodosa, in contrast to spring, showed primarily a depletion of nutrients (0.535 ± 0.48 μM/m2 for NOX and 2.849 ± 1.92 μM/m2 for ammonium) during day and a release during night (0.389 ± 0.46 μM/m2, 2.119 ± 1.81 μM/m2, respectively). Rates of nutrient release in H. incrassata were also lower in summer than in spring.
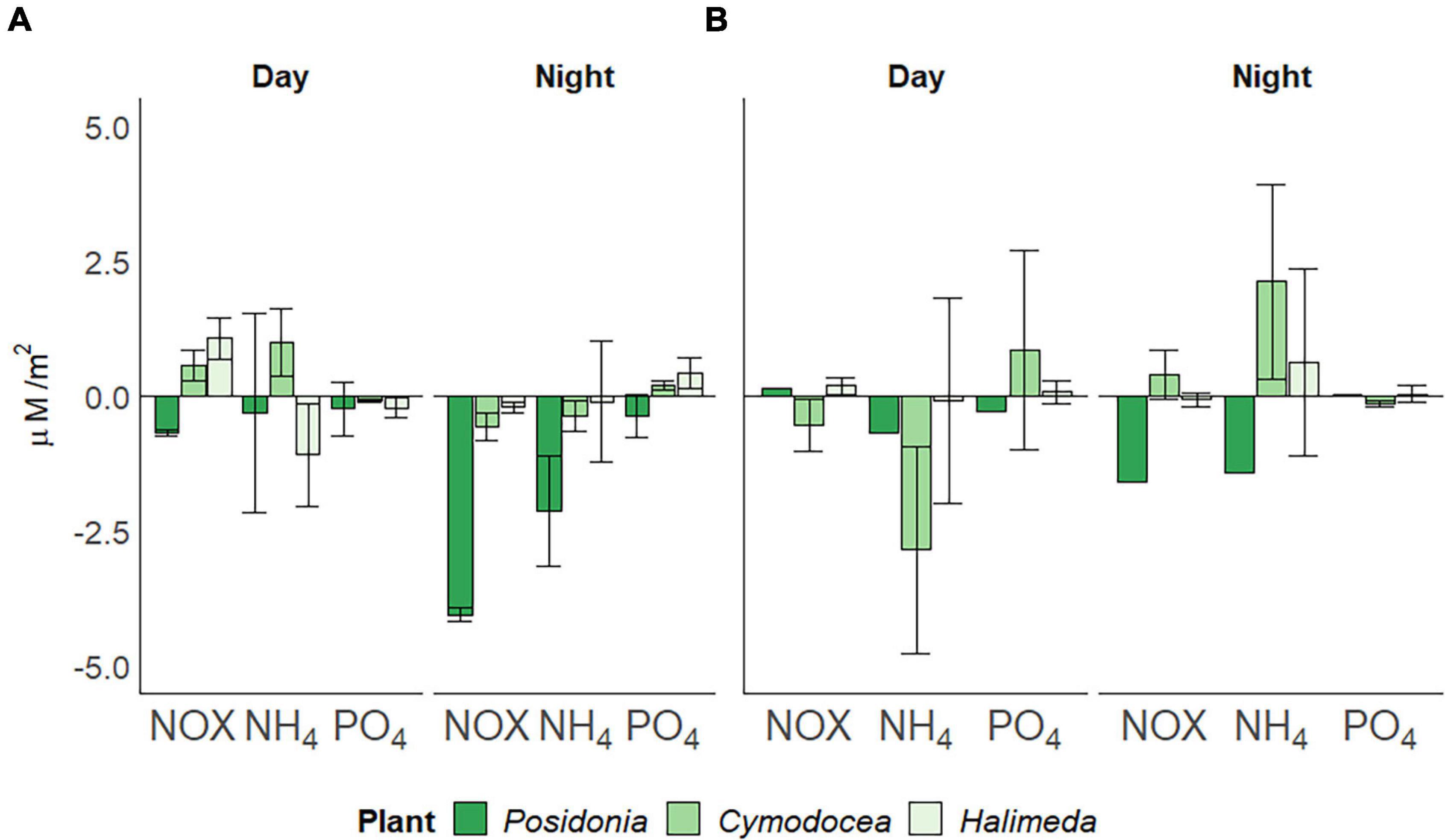
Figure 4. Daily usage of inorganic nutrients per square meter (in μM/m2), divided by day and night periods. Nitrogen oxides [NOX; Nitrate (NO3–) and Nitrite (NO2–)], ammonium (NH4+) and phosphate (PO43–) in (A) spring (April) and (B) summer (July) 2019, corrected for bare sediment. Negative rates indicate depletion, positive rates a release. Standard deviation indicated by error bars.
Dissolved organic nitrogen (DON) in the surrounding water was significantly lower (p < 0.05) in spring than in summer, whereas dissolved organic phosphorus (DOP) was lower (p < 0.05) in summer (Table 1). Highest rates of dissolved organic nutrient usage were observed for P. oceanica (Figure 5), yet differences between the different macrophytes are apparent. In spring (Figure 5A), both seagrass species showed a depletion of DON during day (11.645 ± 17.14 μM/m2 for P. oceanica and 10.413 ± 8.56 μM/m2 for C. nodosa). At night, P. oceanica showed a release of 13.827 ± 9.67 μM/m2, whereas C. nodosa still indicated a depletion of DON (13.369 ± 7.59 μM/m2). The green alga H. incrassata also showed a depletion of DON during night (2.699 ± 3.85 μM/m2), but a release (13.522 ± 3.44 μM/m2) during the day. DOP usage was similar for all three macrophyte, showing primarily a depletion during night but a release during day, with rates higher for both seagrasses (1.339 ± 0.25 μM/m2 for P. oceanica and 1.428 ± 0.24 μM/m2 for C. nodosa) than for H. incrassata (0.872 ± 0.89 μM/m2). In summer (Figure 5B), all three habitats released DON during night (at rates of 18.512 μM/m2 for P. oceanica, 6.469 ± 3.85 μM/m2 for C. nodosa and 8.211 ± 8.59 μM/m2 for H. incrassata) and showed a tendency toward depleting DON during the day (P. oceanica with the highest rate of 3.937 μM/m2). The release of DOP during day was not observed in summer, except for C. nodosa (1.609 ± 2.69 μM/m2).
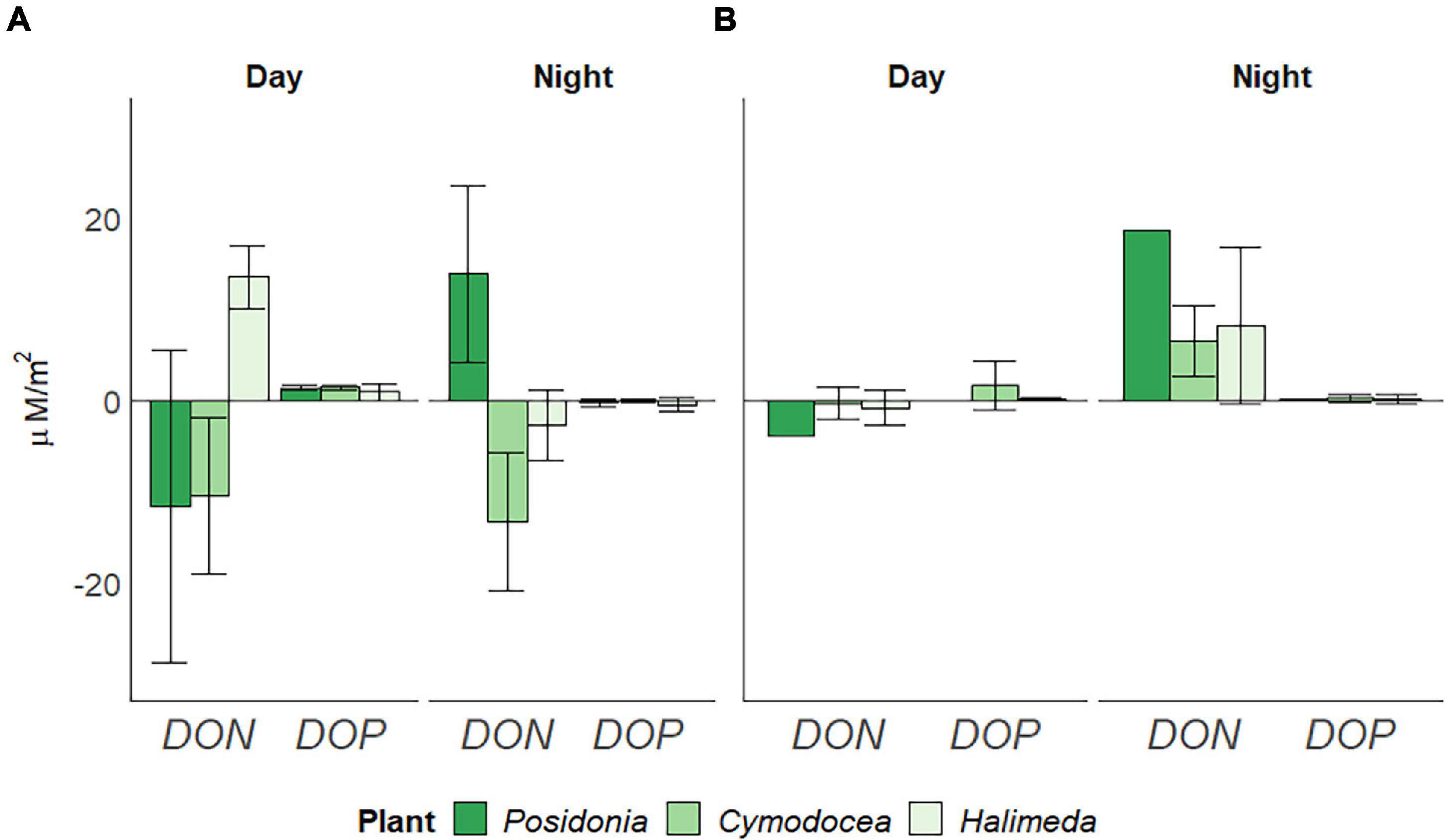
Figure 5. Daily usage of dissolved organic nitrogen (DON) and phosphorus (DOP) per square meter (in μM/m2), divided by day and night periods in (A) spring (April) and (B) summer (July) 2019, corrected for bare sediment. Negative rates indicate depletion, positive rates a release. Standard Deviation indicated by error bars.
Discussion
Marine macrophytes play a foundational role in coastal ecosystem productivity, though not all seagrass and macroalgae taxa have the same potential for eliminating CO2 from the water column. The habitat of P. oceanica is a highly productive coastal ecosystem with a high potential for eliminating CO2 from the water column. Posidonia oceanica’s large biomass and the formation of vast and dense meadows make it an invaluable carbon sink with a high capacity of carbon storage beneath its meadows. Although C. nodosa appears to have a more efficient metabolism when normalized by biomass, the superior biomass and physiology of P. oceanica provides a larger photosynthetic active surface than canopies of C. nodosa. However, the in-situ biomass of the macrophytes was rather low and values for metabolic response rates [>160 mmol O2/d/m2 for GPP and >125 mmol O2/d/m2 CR in P. oceanica meadows (Duarte et al., 2010; Champenois and Borges, 2012; Eggert, 2012); >160 mmol C/d/m2 for GPP and >80 mmol C/d/m2 for CR in C. nodosa after conversion from O2 metabolic rates (Egea et al., 2020)] in previous studies exceeded the values measured in this study. The Mediterranean Sea has experienced a decline in seagrass coverage (Delgado et al., 1999), estimated to be between 13 and 38% of initial coverage of P. oceanica since 1960 (Marbà et al., 2014). Physiological stress due to progressing coastal eutrophication, enhanced organic loading of the water column and respective increased light attenuation are contributing to this decline (Brocke et al., 2015), and in fact, the available underwater light at our study site in Punta Negra was generally lower than in other locations in the Western Mediterranean (González-Correa et al., 2008). Additionally, the intrusion of non-indigenous species like H. incrassata have resulted in higher competition for nutrients and light (Davis and Fourqurean, 2001). Although the temperature measured in summer (25.68–27.12°C) is favorable to the settlement and colonization of H. incrassata, the growth rate of 0.93 new segments per day for the time period investigated is lower than for the congenerous H. tuna in a tropical reef system in Florida, probably related to environmental differences between these studies (Vroom et al., 2003). However, environmental differences to these studies on sites where Halimeda spp. are endemic are apparent and the growth rate for this study might be an underestimation due to its exponential growth pattern. H. incrassata maintains its basic functioning in an inactive state in low temperatures (15.09–17.38°C measured in spring) (Thorhaug, 1976) and benefits from a higher temperature in summer, increasing photosynthesis, and promoting calcification (Campbell et al., 2016). Evidently, we see the expected better metabolic performance of H. incrassata in summer, whereas the water temperature exceeded the preferred growing temperature range for P. oceanica (Olsen et al., 2012), which could reduce its growth to cope with thermal stress (Marín-Guirao et al., 2018). The observed decline in autotrophy in summer is in line with the observations of thermal stress in summer (García et al., 2013), specifically when seawater exceeds 28°C associated with the occurrence of heat waves (Marbà and Duarte, 2010), whereas the more tolerant C. nodosa seems to be less affected. The elevated NCP and decline in CR in summer suggests a resource reallocation toward growth rather than energy storage. Whole ecosystem metabolic rates calculated with oxygen data from the multiparametric sensors showed trends of increased productivity in summer in all habitats. Since the hydrolabs were installed 0.2 m above the seafloor, and the measurement of the oxygen profile in the water column is the net result of mixing of water bodies passing through various benthonic as well as pelagic compartments with lateral water movement the multiparametric sensors represent ecosystem values. The distinction between the three macrophyte species and each habitat can only be made with a system preventing this lateral movement like the benthic chambers. However, P. oceanica is known to export a substantial amount of its productivity toward adjacent ecosystems (Duarte, 2002; Kennedy et al., 2010) and the increase in NCP measured by the multiparametric sensors is possibly due to the export of oxygenated water out of P. oceanica meadows.
Apart from warming, the Mediterranean Sea shows a trend of decreasing pH of −0.0044 ± 0.00006 pH units per year (Flecha et al., 2015). Seagrass species might have the ability to increase productivity with decreasing pH, using CO2 as an alternative carbon source; the photosynthetic activity and function of seagrass meadows causes fluctuations of 0.2–0.7 pH units (0.05–0.19 pH units in this study) on a daily scale, making them a valuable buffer system against OA (Hendriks et al., 2010, 2014). The major source of carbon for H. incrassata is the precipitation of bicarbonate to build up structural components, hence a higher calcification rate that can be observed in summer (Invers et al., 1997, 2001). However, for the vegetated sites with lower shoot density of C. nodosa and H. incrassata, we cannot rule out a substantial contribution of bacterial activity in the sediment to calcification rates, a correction for bare sediment did not lead to reliable results due to the large deviation within incubation settings. Although H. incrassata finds favorable conditions in the study site, its physiology is most likely to be negatively affected by progressing OA due to changes in the water chemistry and further limitation of carbonate (Nelson, 2009; Price et al., 2011; Hofmann and Bischof, 2014). Low water temperatures and elevated levels of CO2 in spring present the additional risk of dissolution, although non-living segments of Halimeda spp. might be resistant to dissolution under elevated CO2 (Peach et al., 2017). However, epiphytic coverage, although not investigated here, and potential nutrient limitations on plant growth cannot be excluded.
Nutrient availability is setting an overall limit for the development of seagrasses (Lucea et al., 2003) and the oligotrophic waters around the Balearic Islands are characterized by enriched nutrient concentrations during spring and low concentrations in summer (Alcoverro et al., 1997, 2001), corresponding with our findings. C:N:P ratios in the water column in our study exceeded the Seagrass Redfield Ratio of 500–550:25–30:1 (Duarte, 1990; Fourqurean et al., 2007). Low ratios in spring are likely to result from increased demand for nutrients due to favorable conditions for growth (Fourqurean et al., 2007), whereas increasing demand for N in summer is likely to lead to P limitation. In addition, our results suggest that the methodology used in this study might have led to P limitation within the incubation bags, most likely due to the limited volume of the incubation chamber (2.27 L for P. oceanica, 1.27 L for C. nodosa, and 1.11 L for H. incrassata). N:P ratios for P. oceanica were considerably higher (5.178 in spring and 3.741 in summer) than reported in previous work (Barrón and Duarte, 2009). Posidonia oceanica represents an important nutrient filter and acts as a net sink of inorganic nutrients, although associated micro- and macroalgae within the meadows contribute to nutrient depletion (Touchette and Burkholder, 2000). Posidonia oceanica depends on bioavailable NOX and ammonium as nitrogen sources and shows net uptake rates in both seasons, whereas it is assumed to be a net source of ammonium and DON on an annual scale (Barrón and Duarte, 2009). Yet, DON and its bioconversion into bioavailable forms by seagrass associated microbiota (Tarquinio et al., 2018) can function as an important alternative nitrogen source for seagrasses (Vonk et al., 2008; Van Engeland et al., 2011). In fact, P. oceanica seems to depend on DON as co-substrate during its optimal growth period in spring, the substantial uptake of DON during the day to meet the demand for biomass production decreases the overall net release of the habitat (Hemminga et al., 1991; Day et al., 2012). A net release of DON of 2.182 μM/m2 per day in spring is far lower than in summer (14.575 μM/m2 per day) and average daily rates based on annual rates (12.055 μM/m2 per day; Barrón and Duarte, 2009). The utilization of DON as co-substrate is hindered in summer due to progressing P-limitation, overall decreased nutrient availability and intensified the competitive pressure by beneficial growth conditions for C. nodosa and H. incrassata. Whereas both tropical species are acting as net nutrient sources in spring, C. nodosa undertakes a shift to a net sink of NOX and ammonium, suggesting the utilization as a source of nitrogen under enhanced growth. H. incrassata presents a net source of inorganic nutrients even under favorable growth conditions in summer and together with a further decline of P. oceanica increases the risk of further eutrophication.
Concluding, temperature and its implications on metabolism is the key driver for the development of biomass of all three macrophytes and therefore for the vegetative structure and carbon sink activity in coastal settings. Yet, the data presented here also confirms the influence of changing environmental parameters between season on the metabolic activity of the macrophytes. A shift toward higher average temperatures as predicted in climate change scenarios (van Vuuren et al., 2011; IPCC, 2013) will lead to unfavorable conditions for P. oceanica, exceeding its temperature optimum and therefore contribute to further regression of P. oceanica (Telesca et al., 2015). Moreover, a rise in SST might facilitate the colonization by sub- and tropical species and ongoing warming facilitates the settlement and expansion of non-indigenous species such as H. incrassata. Furthermore, progressing nutrient limitation in coastal settings and advantages in competition for nutrients, light and space favoring macroalgae contributes to the decline of seagrasses (Van Tussenbroek and Van Dijk, 2007; Thomsen et al., 2009; Han and Liu, 2014). The loss of vital P. oceanica meadows threatens the autochthonous biodiversity, potentially causing a shift in coastal marine vegetation (Cheung et al., 2009; Lejeusne et al., 2010; Burrows et al., 2011). Further regression of P. oceanica and the inherent loss of ecosystem services will severely impact the productivity and biogeochemical cycling of the coastal ecosystem in the Mediterranean Sea.
Data Availability Statement
The raw data supporting the conclusions of this article will be made available by the authors, without undue reservation.
Author Contributions
IH: conception. IH and SF: design of the study. LM: draft the first version of the manuscript. All authors conducted the relevant field work jointly, contributed to data acquisition and sample analysis, revised the manuscript critically, contributed, and had substantial impact on the final draft of the manuscript.
Funding
Funding was provided by projects PRD2018/18, from the Conselleria d’Innovació, Recerca i Turisme of Mallorca (Spain) and RTI2018-095441-B-C21 (SUMAECO) from the Spanish Ministry of Science, Innovation and Universities. SF was supported by a “Margalida Comas” postdoctoral scholarship, funded by the Balearic Islands Government.
Conflict of Interest
The authors declare that the research was conducted in the absence of any commercial or financial relationships that could be construed as a potential conflict of interest.
Publisher’s Note
All claims expressed in this article are solely those of the authors and do not necessarily represent those of their affiliated organizations, or those of the publisher, the editors and the reviewers. Any product that may be evaluated in this article, or claim that may be made by its manufacturer, is not guaranteed or endorsed by the publisher.
Acknowledgments
We would like to thank the Club Náutico Palmanova for allowing boat entrance and the H10 Punta Negra for granting easy access to the study site. We also thank A. Verdu Campillo for assistance in the field. LM would like to personally thank the coordination team and the program IMBRSEA (International Master of Science in Marine Biological Resources, www.imbrsea.eu), in which framework this project was conducted.
Supplementary Material
The Supplementary Material for this article can be found online at: https://www.frontiersin.org/articles/10.3389/fmars.2021.746379/full#supplementary-material
References
Alcoverro, T., Cerbiān, E., and Ballesteros, E. (2001). The photosynthetic capacity of the seagrass Posidonia oceanica: influence of nitrogen and light. J. Exp. Mari. Biol. Ecol. 261, 107–120. doi: 10.1016/S0022-0981(01)00267-2
Alcoverro, T., Romero, J., Duarte, C. M., and López, N. I. (1997). Spatial and temporal variations in nutrient limitation of seagrass Posidonia oceanica growth in the NW mediterranean. Mari. Ecol. Prog. Ser. 146, 155–161. doi: 10.3354/meps146155
Alós, J., Tomas, F., Terrados, J., Verbruggen, H., and Ballesteros, E. (2016). Fast-spreading green beds of recently introduced Halimeda incrassata invade Mallorca island (NW Mediterranean sea). Mari. Ecol. Prog. Ser. 558, 153–158. doi: 10.3354/meps11869
Barrón, C., and Duarte, C. M. (2009). Dissolved organic matter release in a Posidonia oceanica meadow. Mari, Ecol. Progr. Ser. 374, 75–84. doi: 10.3354/meps07715
Barry, S. C., Frazer, T. K., and Jacoby, C. A. (2013). Production and carbonate dynamics of Halimeda incrassata (Ellis) Lamouroux altered by Thalassia testudinum banks and Soland ex König. J. Exp. Mari. Biol. Ecol. 444, 73–80. doi: 10.1016/j.jembe.2013.03.012
Brocke, H. J., Wenzhoefer, F., De Beer, D., Mueller, B., Van Duyl, F. C., and Nugues, M. M. (2015). High dissolved organic carbon release by benthic cyanobacterial mats in a caribbean reef ecosystem. Sci. Rep. 5:8852. doi: 10.1038/srep08852
Burrows, M. T., Schoeman, D. S., Buckley, L. B., Moore, P., Poloczanska, E. S., Brander, K. M., et al. (2011). The pace of shifting climate in marine and terrestrial ecosystems. Science 334, 652–655. doi: 10.1126/science.1210288
Caldeira, K., and Wickett, M. E. (2003). Anthropogenic carbon and ocean pH. Nature 425:365. doi: 10.1038/425365a
Campbell, J. E., Fisch, J., Langdon, C., and Paul, V. J. (2016). Increased temperature mitigates the effects of ocean acidification in calcified green algae (Halimeda spp.). Coral Reefs 35, 357–368. doi: 10.1007/s00338-015-1377-9
Carpenter, J. H. (1965). The chesapeake bay institute technique for the winkler dissolved oxygen method. Limnol. Oceanogr. 10, 141–143. doi: 10.4319/lo.1965.10.1.0141
Champenois, W., and Borges, A. V. (2012). Seasonal and interannual variations of community metabolism rates of a Posidonia oceanica seagrass meadow. Limnol. Oceanogr. 57, 347–361. doi: 10.4319/lo.2012.57.1.0347
Cheung, W. W. L., Lam, V. W. Y., Sarmiento, J. L., Kearney, K., Watson, R., and Pauly, D. (2009). Projecting global marine biodiversity impacts under climate change scenarios. Fish Fish. 10, 235–251. doi: 10.1111/j.1467-2979.2008.00315.x
Cole, J. J., Pace, M. L., Carpenter, S. R., and Kitchell, J. F. (2000). Persistence of net heterotrophy in lakes during nutrient addition and food web manipulations. Limnol. Oceanogr. 45, 1718–1730. doi: 10.4319/lo.2000.45.8.1718
Coloso, J. J., Cole, J. J., Hanson, P. C., and Pace, M. L. (2008). Depth-integrated, continuous estimates of metabolism in a clear-water lake. Can. J. Fish. Aquatic Sci. 65, 712–722. doi: 10.1139/F08-006
Costanza, R., D’Arge, R., De Groot, R., Farber, S., Grasso, M., Hannon, B., et al. (1997). The value of the world’s ecosystem services and natural capital. Nature 387, 253–260. doi: 10.1038/387253a0
Davis, B. C., and Fourqurean, J. W. (2001). Competition between the tropical alga, Halimeda incrassata, and the seagrass, Thalassia testudinum. Aquatic Bot. 71, 217–232. doi: 10.1016/S0304-3770(01)00179-6
Day, J. W. Jr., Kemp, W. M., Yáñez-Arancibia, A., and Crump, B. C. (2012). Estuarine Ecology. Hoboken, NJ: John Wiley & Sons. doi: 10.1002/9781118412787
Delgado, O., Pérez, M., Ruiz, J. M., Ballesteros, E., and Romero, J. (1999). Effects of fish farming activities on seagrass (Posidonia oceanica) beds in a Mediterranean bay: seagrass decline post-disturbance. Oceanol. Acta 22, 110–117. doi: 10.1016/S0399-1784(99)80037-1
Diaz-Almela, E., Marbà, N., and Duarte, C. M. (2007). Consequences of Mediterranean warming events in seagrass (Posidonia oceanica) flowering records. Global Change Biol. 13, 224–235. doi: 10.1111/j.1365-2486.2006.01260.x
Dickson, A. G. (1990). Standard potential of the reaction: AgCl(s) + 12H2(g) = Ag(s) + HCl(aq), and and the standard acidity constant of the ion HSO4− in synthetic sea water from 273.15 to 318.15 K. J. Chem. Thermodyn. 22, 113–127. doi: 10.1016/0021-9614(90)90074-Z
Dickson, A. G., and Millero, F. J. (1987). A comparison of the equilibrium constants for the dissociation of carbonic acid in seawater media. Deep Sea Res. Part A. Oceanogr. Res. Papers 34, 1733–1743. doi: 10.1016/0198-0149(87)90021-5
Dickson, A. G., Sabine, C. L., and Christian, J. R. (eds) (2007). Guide to Best Practices for Ocean CO2 Measurement: (PICES Special Publication 3; IOCCP Report 8). Sidney, BC: North Pacific Marine Science Organization, 191. doi: 10.25607/OBP-1342
Doney, S. C., Fabry, V. J., Feely, R. A., and Kleypas, J. A. (2009). Ocean acidification: the other CO 2 problem. Ann. Rev. Mari. Sci. 1, 169–192. doi: 10.1146/annurev.marine.010908.163834
Duarte, C. M. (1990). Seagrass nutrient content. Mari. Ecol. Prog. Ser. Oldendorf 6, 201–207. doi: 10.3354/meps067201
Duarte, C. M. (1995). Submerged aquatic vegetation in relation to different nutrient regimes. Ophelia 41, 87–112. doi: 10.1080/00785236.1995.10422039
Duarte, C. M. (1999). Seagrass ecology at the turn of the millennium: challenges for the new century. Aquatic Bot. 65, 7–20. doi: 10.1016/S0304-3770(99)00027-3
Duarte, C. M. (2002). The future of seagrass meadows. Environ. Conserv. 29, 192–206. doi: 10.1017/S0376892902000127
Duarte, C. M., and Chiscano, C. L. (1999). Seagrass biomass and production: a reassessment. Aquatic Bot. 65, 159–174. doi: 10.1016/S0304-3770(99)00038-8
Duarte, C. M., and Krause-Jensen, D. (2017). Export from seagrass meadows contributes to marine carbon sequestration. Front. Mari. Sci. 4:13. doi: 10.3389/fmars.2017.00013
Duarte, C. M., Losada, I. J., Hendriks, I. E., Mazarrasa, I., and Marbà, N. (2013). The role of coastal plant communities for climate change mitigation and adaptation. Nat. Clim. Change 3, 961–968. doi: 10.1038/nclimate1970
Duarte, C. M., Marbà, N., Gacia, E., Fourqurean, J. W., Beggins, J., Barrón, C., et al. (2010). Seagrass community metabolism: assessing the carbon sink capacity of seagrass meadows. Global Biogeochem. Cycles 24, 1–8. doi: 10.1029/2010GB003793
Durrieu de Madron, X., Guieu, C., Sempéré, R., Conan, P., Cossa, D., D’Ortenzio, F., et al. (2011). Marine ecosystems’ responses to climatic and anthropogenic forcings in the Mediterranean. Progr. Oceanogr. 91, 97–166. doi: 10.1016/j.pocean.2011.02.003
Dustan, P. (1975). Growth and form in the reef-building coral Montastrea annularis. Mari. Biol. 33, 101–107. doi: 10.1007/BF00390714
Egea, L. G., Jiménez-Ramos, R., Hernández, I., and Brun, F. G. (2020). Differential effects of nutrient enrichment on carbon metabolism and dissolved organic carbon (DOC) fluxes in macrophytic benthic communities. Mari. Environ. Res. 162:105179. doi: 10.1016/j.marenvres.2020.105179
Eggert, A. (2012). “Seaweed responses to temperature,” in Seaweed Biology, eds C. Wiencke and K. Bischof (Berlin: Springer), 47–66.
Feely, R. A., Sabine, C. L., Lee, K., Berelson, W., Kleypas, J., Fabry, V. J., et al. (2004). Impact of anthropogenic CO2 on the CaCO3 system in the oceans. Science 305, 362–366. doi: 10.1126/science.1097329
Flecha, S., Pérez, F. F., García-Lafuente, J., Sammartino, S., Ríos, A. F., and Huertas, I. E. (2015). Trends of pH decrease in the Mediterranean Sea through high frequency observational data: indication of ocean acidification in the basin. Sci. Rep. 5:16770. doi: 10.1038/srep16770
Fourqurean, J. W., Duarte, C. M., Kennedy, H., Marbà, N., Holmer, M., Mateo, M. A., et al. (2012). Seagrass ecosystems as a globally significant carbon stock. Nat. Geosci. 5, 505–509. doi: 10.1038/ngeo1477
Fourqurean, J. W., Marbà, N., Duarte, C. M., Díaz-Almela, E., and Ruiz-Halpern, S. (2007). Spatial and temporal variation in the elemental and stable isotopic content of the seagrasses Posidonia oceanica and Cymodocea nodosa from the Illes Balears. Spain. Marine Biology 151, 219–232. doi: 10.1007/s00227-006-0473-3
Galil, B. S., Boero, F., Fraschetti, S., Piraino, S., Campbell, M. L., Hewitt, C. L., et al. (2015). The enlargement of the suez canal and introduction of non-indigenous species to the Mediterranean sea. Limnol. Oceanogr. Bull. 24, 43–45. doi: 10.1002/lob.10036
García, R., Holmer, M., Duarte, C. M., and Marbà, N. (2013). Global warming enhances sulphide stress in a key seagrass species (NW Mediterranean). Global Change Biol. 19, 3629–3639. doi: 10.1111/gcb.12377
González-Correa, J. M., Torquemada, Y. F., and Sánchez Lizaso, J. L. (2008). Long-term effect of beach replenishment on natural recovery of shallow Posidonia oceanica meadows. Estuat. Coast. Shelf Sci. 76, 834–844. doi: 10.1016/j.ecss.2007.08.012
Grall, J., and Chauvaud, L. (2017). Marine eutrophication and benthos: the need for new approaches and concepts. Global Change Biol. 8, 813–830. doi: 10.1046/j.1365-2486.2002.00519.x
Gruber, N., Clement, D., Carter, B. R., Feely, R. A., van Heuven, S., Hoppema, M., et al. (2019). The oceanic sink for anthropogenic CO2 from 1994 to 2007. Science 363, 1193–1199. doi: 10.1126/science.aau5153
Han, Q., and Liu, D. (2014). Macroalgae blooms and their effects on seagrass ecosystems. J. Ocean Univ. China 13, 791–798. doi: 10.1007/s11802-014-2471-2
Hansen, H. P., and Koroleff, F. (1999). “Determination of nutrients,” in Methods of Seawater Analysis, eds K. Grasshoff, K. Kremling, and M. Ernhardt (Dordrecht: Springer).
Hemminga, M. A., Harrison, P. G., and Van Lent, F. (1991). The balance of nutrient losses and gains in seagrass meadows. Mari. Ecol. Progr. Ser. 71, 85–96.
Hendriks, I. E., Duarte, C. M., and Álvarez, M. (2010). Vulnerability of marine biodiversity to ocean acidification: a meta-analysis. Estua. Coast. Shelf Sci. 86, 157–164. doi: 10.1016/j.ecss.2009.11.022
Hendriks, I. E., Olsen, Y. S., Ramajo, L., Basso, L., Steckbauer, A., Moore, T. S., et al. (2014). Photosynthetic activity buffers ocean acidification in seagrass meadows. Biogeosciences 11, 333–346. doi: 10.5194/bg-11-333-2014
Hofmann, L. C., and Bischof, K. (2014). Ocean acidification effects on calcifying macroalgae. Aquatic Biol. 22, 261–279.
Invers, O., Romero, J., and Pérez, M. (1997). Effects of pH on seagrass photosynthesis: a laboratory and field assessment. Aquatic Bot. 59, 185–194.
Invers, O., Zimmerman, R. C., Alberte, R. S., Perez, M., and Romero, J. (2001). Inorganic carbon sources for seagrass photosynthesis: an experimental evaluation of bicarbonate use in species inhabiting temperate waters. J. Exp. Mari. Biol. Ecol. 265, 203–217.
IPCC (2013). Climate Change 2013: The Physical Science Basis. Contribution of Working Group I to the Fifth Assessment Report of the Intergovernmental Panel on Climate Change, eds T. F. Stocker, D. Qin, G.-K. Plattner, M. Tignor, S. K. Allen, J. Boschung, et al. (Cambridge: Cambridge University Press), 1535. doi: 10.1017/CBO9781107415324
IPCC (2021). “Summary for Policymakers,” in Climate Change 2021: The Physical Science Basis. Contribution of Working Group I to the Sixth Assessment Report of the Intergovernmental Panel on Climate Change, eds V. Masson-Delmotte, P. Zhai, A. Pirani, S. L. Connors, C. Péan, S. Berger, et al. (Cambridge: Cambridge University Press).
Jordà, G., Marbà, N., and Duarte, C. M. (2012). Mediterranean seagrass vulnerable to regional climate warming. Nat. Climate Change 2, 821–824. doi: 10.1038/nclimate1533
Kennedy, H., Beggins, J., Duarte, C. M., Fourqurean, J. W., Holmer, M., Marbá, N., et al. (2010). Seagrass sediments as a global carbon sink: Isotopic constraints. Global Biogeochem. Cycles 24, 1–8. doi: 10.1029/2010GB003848
Kroeker, K. J., Kordas, R. L., Crim, R., Hendriks, I. E., Ramajo, L., Singh, G. S., et al. (2013). Impacts of ocean acidification on marine organisms: quantifying sensitivities and interaction with warming. Global Change Biol. 19, 1884–1896. doi: 10.1111/gcb.12179
Labasque, T. (2004). Spectrophotometric Winkler determination of dissolved oxygen: re-examination of critical factors and reliability. Mari. Chem. 88, 53–60. doi: 10.1016/s0304-4203(04)00058-1
Lee, K. S., Park, S. R., and Kim, Y. K. (2007). Effects of irradiance, temperature, and nutrients on growth dynamics of seagrasses: a review. J. Exp. Mar. Biol. Ecol. 350, 144–175.
Lejeusne, C., Chevaldonné, P., Pergent-Martini, C., Boudouresque, C. F., and Pérez, T. (2010). Climate change effects on a miniature ocean: the highly diverse, highly impacted Mediterranean sea. Trends Ecol. Evolu. 25, 250–260. doi: 10.1016/j.tree.2009.10.009
Lloret, J., Marín, A., and Marín-Guirao, L. (2008). Is coastal lagoon eutrophication likely to be aggravated by global climate change? Estua. Coast. Shelf Sci. 78, 403–412. doi: 10.1016/j.ecss.2008.01.003
Lucea, A., Duarte, C. M., Agustí, S., and Søndergaard, M. (2003). Nutrient (N, P and Si) and carbon partitioning in the stratified NW Mediterranean. J. Sea Res. 49, 157–170.
Macreadie, P. I., Anton, A., Raven, J. A., Beaumont, N., Connolly, R. M., Friess, D. A., et al. (2019). The future of blue carbon science. Nat. Commun. 10, 1–13.
Marbà, N., Arias-Ortiz, A., Masqué, P., Kendrick, G. A., Mazarrasa, I., Bastyan, G. R., et al. (2015). Impact of seagrass loss and subsequent revegetation on carbon sequestration and stocks. J. Ecol. 103, 296–302. doi: 10.1111/1365-2745.12370
Marbà, N., Díaz-Almela, E., and Duarte, C. M. (2014). Mediterranean seagrass (Posidonia oceanica) loss between 1842 and 2009. Biol. Conserv. 176, 183–190. doi: 10.1016/j.biocon.2014.05.024
Marbà, N., and Duarte, C. M. (2010). Mediterranean warming triggers seagrass (Posidonia oceanica) shoot mortality. Global Change Biol. 16, 2366–2375. doi: 10.1111/j.1365-2486.2009.02130.x
Marín-Guirao, L., Bernardeau-Esteller, J., García-Muñoz, R., Ramos, A., Ontoria, Y., Romero, J., et al. (2018). Carbon economy of Mediterranean seagrasses in response to thermal stress. Mari. Pollut. Bull. 135, 617–629.
Mehrbach, C., Culberson, C. H., Hawley, J. E., and Pytkowicx, R. M. (1973). Measurement of the apparent dissociation constants of carbonic acid in seawater at atmospheric pressure. Limnol. Oceanogr. 18, 897–907. doi: 10.4319/lo.1973.18.6.0897
Multer, H. G. (1988). Growth rate, ultrastructure and sediment contribution of Halimeda incrassata and Halimeda monile, Nonsuch and Falmouth Bays, Antigua, W.I. Coral Reefs 6, 179–186.
Nelson, W. A. (2009). Calcified macroalgae–critical to coastal ecosystems and vulnerable to change: a review. Mari. Fresh. Res. 60, 787–801.
Olsen, Y. S., Sánchez-Camacho, M., Marbà, N., and Duarte, C. M. (2012). Mediterranean seagrass growth and demography responses to experimental warming. Estua. Coasts 35, 1205–1213. doi: 10.1007/s12237-012-9521-z
Parry, M. L. (ed.) (2000). Assessment of Potential Effects and Adaptations for Climate Change in Europe: The Europe Acacia Project (a Concerted Action Towards a Comprehensive Climate Impacts and Adaptations Assessment for the European Union): Report of a Concerted Action of the Environment Programme of the Research Directorate General of the Commission of the European Communities. Norwich: University of East Anglia, School of Environmental Sciences, Jackson Environment Institute.
Payri, C. E. (1988). Halimeda contribution to organic and inorganic production in a Tahitian reef system. Coral Reefs 6, 251–262. doi: 10.1007/BF00302021
Peach, K. E., Koch, M. S., Blackwelder, P. L., and Manfrino, C. (2017). Calcification and photophysiology responses to elevated pCO2 in six Halimeda species from contrasting irradiance environments on little cayman island reefs. J. Exp. Mari. Biol. Ecol. 486, 114–126. doi: 10.1016/j.jembe.2016.09.008
Pierrot, D., Lewis, E., and Wallace, D. W. R. (2006). CO2SYS DOS Program Developed for CO2 System Calculations. ORNL/CDIAC-105. Oak Ridge, TN: Carbon Dioxide Information Analysis Center, Oak Ridge National Laboratory, US Department of Energy.
Price, N. N., Hamilton, S. L., Tootell, J. S., and Smith, J. E. (2011). Species-specific consequences of ocean acidification for the calcareous tropical green algae halimeda. Mari. Ecol. Prog. Ser. 440, 67–78. doi: 10.3354/meps09309
Raitsos, D. E., Beaugrand, G., Georgopoulos, D., Zenetos, A., Pancucci-Papadopoulou, A. M., Theocharis, A., et al. (2010). Global climate change amplifies the entry of tropical species into the eastern Mediterranean sea. Limnol. Oceanogr. 55, 1478–1484. doi: 10.4319/lo.2010.55.4.1478
Richon, C., Dutay, J. C., Bopp, L., Le Vu, B., Orr, J. C., Somot, S., et al. (2019). Biogeochemical response of the Mediterranean sea to the transient SRES-A2 climate change scenario. Biogeosciences 16, 135–165. doi: 10.5194/bg-16-135-2019
R Core Team (2021). R: A Language and Environment for Statistical Computing. Vienna: R Foundation for Statistical Computing.
Sand-Jensen, K., and Borum, J. (1991). Interactions among phytoplankton, periphyton, and macrophytes in temperate freshwaters and estuaries. Aquatic Bot. 41, 137–175. doi: 10.1016/0304-3770(91)90042-4
Sardain, A., Sardain, E., and Leung, B. (2019). Global forecasts of shipping traffic and biological invasions to 2050. Nat. Sustainabil. 2, 274–282. doi: 10.1038/s41893-019-0245-y
Savva, I., Bennett, S., Roca, G., Jordà, G., and Marbà, N. (2018). Thermal tolerance of Mediterranean marine macrophytes: vulnerability to global warming. Ecol. Evol. 8, 12032–12043.
Short, F. T., and Neckles, H. A. (1999). The effects of global climate change on seagrasses. Aquatic Bot. 63, 169–196. doi: 10.1016/S0304-3770(98)00117-X
Smith, S. V., and Key, G. S. (1975). Carbon dioxide and metabolism in marine environments. Limnol. Oceanogr. 20, 493–495. doi: 10.4319/lo.1975.20.3.0493
Tarquinio, F., Bourgoure, J., Koenders, A., Laverock, B., Säwström, C., and Hyndes, G. A. (2018). Microorganisms facilitate uptake of dissolved organic nitrogen by seagrass leaves. ISME J. 12, 2796–2800.
Telesca, L., Belluscio, A., Criscoli, A., Ardizzone, G., Apostolaki, E. T., Fraschetti, S., et al. (2015). Seagrass meadows (Posidonia oceanica) distribution and trajectories of change. Sci. Rep. 5, 1–14. doi: 10.1038/srep12505
Thomsen, M. S., Wernberg, T., Tuya, F., and Silliman, B. R. (2009). evidence for impacts of nonindigenous Macroalgae: a meta-analysis of experimental field studies 1. J. Phycol. 45, 812–819.
Touchette, B. W., and Burkholder, J. M. (2000). Review of nitrogen and phosphorus metabolism in seagrasses. J. Exp. Mari. Biol. Ecol. 250, 133–167.
Van Engeland, T., Bouma, T. J., Morris, E. P., Brun, F. G., Peralta, G., Lara, M., et al. (2011). Potential uptake of dissolved organic matter by seagrasses and macroalgae. Mari. Ecol. Prog. Ser. 427, 71–81.
Van Tussenbroek, B. I., and Van Dijk, J. K. (2007). Spatial and temporal variability in biomass and production of Psammophytic halimeda incrassata (bryopsidales, chlorophyta) in a Caribbean reef lagoon 1. J. Phycol. 43, 69–77.
van Vuuren, D. P., Edmonds, J., Kainuma, M., Riahi, K., Thomson, A., Hibbard, K., et al. (2011). The representative concentration pathways: an overview. Clim. Change 109, 5–31. doi: 10.1007/s10584-011-0148-z
Vargas-Yáñez, M., Zunino, P., Benali, A., Delpy, M., Pastre, F., Moya, F., et al. (2010). How much is the western mediterranean really warming and salting? J. Geophys. Res. Oceans 115, 1–12. doi: 10.1029/2009JC005816
Vonk, J. A., Middelburg, J. J., Stapel, J., and Bouma, T. J. (2008). Dissolved organic nitrogen uptake by seagrasses. Limnol. Oceanogr. 53, 542–548.
Vroom, P. S., Smith, C. M., Coyer, J. A., Walters, L. J., Hunter, C. L., Beach, K. S., et al. (2003). Field biology of halimeda tuna (bryopsidales, chlorophyta) across a depth gradient: comparative growth, survivorship, recruitment, and reproduction. Hydrobiologia 501, 149–166. doi: 10.1023/A:1026287816324
Waycott, M., Duarte, C. M., Carruthers, T. J. B., Orth, R. J., Dennison, W. C., Olyarnik, S., et al. (2009). Accelerating loss of seagrasses across the globe threatens coastal ecosystems. Proc. Natl. Acad. Sci. U.S.A. 106, 12377–12381. doi: 10.1073/pnas.0905620106
Wefer, G. (1980). Carbonate production by algae halimeda. Penicillus Padina. Nat. 285, 323–324. doi: 10.1038/285323a0
Wigley, T. M. (1983). The pre-industrial carbon dioxide level. Climatic Change 5, 315–320. doi: 10.1007/BF02423528
Keywords: Posidonia oceanica (L.) Delile, Cymodocea nodosa, Halimeda incrassata, primary production, carbon sink, invasive algae
Citation: Marx L, Flecha S, Wesselmann M, Morell C and Hendriks IE (2021) Marine Macrophytes as Carbon Sinks: Comparison Between Seagrasses and the Non-native Alga Halimeda incrassata in the Western Mediterranean (Mallorca). Front. Mar. Sci. 8:746379. doi: 10.3389/fmars.2021.746379
Received: 23 July 2021; Accepted: 12 October 2021;
Published: 04 November 2021.
Edited by:
Glenn Hyndes, Edith Cowan University, AustraliaReviewed by:
Rene Friedland, Leibniz Institute for Baltic Sea Research (LG), GermanyKenta Watanabe, Port and Airport Research Institute (PARI), Japan
Copyright © 2021 Marx, Flecha, Wesselmann, Morell and Hendriks. This is an open-access article distributed under the terms of the Creative Commons Attribution License (CC BY). The use, distribution or reproduction in other forums is permitted, provided the original author(s) and the copyright owner(s) are credited and that the original publication in this journal is cited, in accordance with accepted academic practice. No use, distribution or reproduction is permitted which does not comply with these terms.
*Correspondence: Iris Eline Hendriks, aXJpc0BpbWVkZWEudWliLWNzaWMuZXM=