Integrating Oceanographic Data and Benthic Community Structure Temporal Series to Assess the Dynamics of a Marginal Reef
- 1Reef Systems Ecology Laboratory, Ecology Post-Graduate Program, Department of Ecology and Zoology, Federal University of Santa Catarina, Florianópolis, Brazil
- 2Reef Systems Ecology and Conservation Laboratory, Marine Biology Department, Fluminense Federal University, Rio de Janeiro, Brazil
- 3Ecology Post-Graduate Program, Department of Ecology and Zoology, Federal University of Santa Catarina, Florianópolis, Brazil
- 4Laboratory of Ocean and Climate Studies, Institute of Oceanography, Federal University of Rio Grande, Rio Grande, Brazil
- 5Center of Physical and Mathematical Sciences, Federal University of Santa Catarina, Florianópolis, Brazil
- 6Coral Vivo Institute, Arraial D’Ajuda, Porto Seguro, Brazil
Reefs are the richest marine ecosystems. Their benthic communities generate structural complexity and participate in nutrient cycles, providing habitat and food for many marine species. These ecosystems have been threatened by local and global anthropogenic impacts and changes in community structure have led to loss of biodiversity, ecosystem function and services worldwide. Most studies about these structural changes have been conducted in Caribbean and Indo-Pacific coral reefs. In the Southwestern Atlantic, where reefs are naturally algae-dominated, these efforts are incipient, especially at oceanic islands where local anthropic impacts tend to be lower, and natural and climate-induced fluctuations might be easily detected. We conducted the first temporal assessment of benthic communities and the influence of oceanographic parameters between 2013 and 2019 in Fernando de Noronha (FNA), the largest Brazilian oceanic archipelago. We annually sampled benthic communities in FNA’s shallow reefs (2–21 m) using photoquadrats, quantified and gathered organisms in major groups according to their functional roles. We also characterized and tested “sea surface temperature,” “marine heatwaves,” “diffuse attenuation coefficient,” and “wave energy” influence for the same period. The most abundant groups were epilithic algal matrix (EAM; mean annual coverage: 23–60%), macroalgae (15–35%) and calcifiers (15–29%), followed by cyanobacteria (1–37%), suspension/filter-feeders (<2%), zoanthids (<1%) and other invertebrates (<0.1%). EAM was negatively correlated with “marine heatwaves” and positively correlated with “wave energy,” while macroalgae and calcifiers showed opposite responses to “marine heatwaves” and “wave energy,” respectively. Cyanobacteria was positively correlated with “marine heatwaves.” The dominance of EAM and macroalgae was already described for reefs along the Brazilian Province and we demonstrated the persistence of this structure over the years in FNA, with the exception of 2019 when there was a substantial increase of cyanobacteria after a strong marine heatwave. Our results suggest a flickering dynamic between EAM and macroalgae, which vary according to the oceanographic conditions, reinforcing its distinct dynamics from most tropical coral reefs. However, the increase of cyanobacteria added to projections of more frequent and stronger marine heatwaves worldwide indicate possible structural changes in this community. Continued monitoring of community and oceanographic drivers is key for better understanding and predicting changes in important marginal reefs.
Introduction
Reefs are the richest marine ecosystems, supporting important primary production in tropical and subtropical shallow waters and a variety of marine species, by providing them food and habitat (Hatcher, 1997; Knowlton et al., 2010). They are often compared to rainforests due to their high structural complexity and biodiversity (Connell, 1978; Reaka-Kudla, 1997). Reefs structure and functioning also provide important ecosystem services for humanity, such as coastal protection from wave action, fish stocks and tourism (Hoegh-Guldberg, 1999; Woodhead et al., 2019). Their complex structure is mostly given by the benthic community, whose sessile organisms provide tridimensionality and are primarily responsible for nutrient cycling (Sousa, 2001; Graham and Nash, 2012; Rogers et al., 2014; Darling et al., 2017). Due to their sessile feature, these organisms are constantly subjected to disturbances, both natural (e.g., predation, seasonal variations on temperature, freshwater discharge, wave action, hurricanes; Putman, 1984) and triggered by local anthropic activities (e.g., pollution, sedimentation, tourism) and global climate change (e.g., rising sea surface temperature, ocean acidification, extreme weather events; Hoegh-Guldberg, 2004, 2010; Fiori and Carcedo, 2013; Halpern et al., 2015). Thus, the reef benthic community structure is primarily shaped by the average setting of environmental conditions, incidences of disturbances, and the organisms’ ability to persist after those disturbances, which results in fluctuations on their abundance and on the performance of their functions (Airoldi, 1998). Sessile reef benthic communities represent, therefore, an important indicator of the status of the reef, since their structure reflects the overall environmental conditions and the influence of disturbances.
Despite their importance, reef ecosystems have been increasingly threatened, especially at coastal zones (França et al., 2020). The synergistic effect of local and global stressors has accelerated and intensified the disturbances, introducing ecosystems to a level of instability beyond natural variation and reducing their recovery interval (Wolkovich et al., 2014; Sully et al., 2019). As a consequence, reef benthic communities have undergone structural changes, in many cases with the expansion of more generalist and opportunistic species (Airoldi, 1998; Brustolin et al., 2019), leading to loss of biodiversity, ecosystem functions and services (Hatcher, 1988; Pandolfi et al., 2003; Alvarez-Filip et al., 2009; Hughes et al., 2010; Halpern et al., 2015). Within this scenario, recording and monitoring reef benthic communities became important tools in order to better understand the mechanism behind natural fluctuations on reef ecosystems (e.g., between years) and to identify the major drivers of these dynamics. With this information, it is possible to comprehend the responses to specific disturbances and how resilient the ecosystem is (Cruz et al., 2014a; Aued et al., 2018), which also provides a basis for potential adaptive management measures (Briske et al., 2017).
Within the scope of reef monitoring, oceanic islands represent important study sites for understanding natural fluctuations and the possible effects of global stressors on benthic communities. Although still affected by global climate change, geographical isolation makes oceanic islands’ ecosystems much less subjected to synergistic effect of local anthropic stressors, and may present features closer to pristine, making natural variations and the effects of global stressors more evident (Friedlander and DeMartini, 2002; Sandin et al., 2008). Potential structural changes in these communities might be related both to seasonal or interannual fluctuations on sea surface temperature, light penetration on the water column and wave action, and to specific disturbances, such as El Niño and marine heatwave events. The thermal stress itself can cause coral bleaching events that, if long-lasting, might lead these organisms to death (Hoegh-Guldberg, 1999). In addition, changes in wind, wave and current trends, triggered by greater energy availability (i.e., heat) for atmospheric and oceanic circulation (Neves and Muehe, 2008; Steffen et al., 2017; Hu et al., 2020), may intensify mechanical disturbances on reefs, as well as sediment resuspension, affecting primary production by increasing light attenuation (Risk and Edinger, 2011).
Overall, processes of disturbances and associated structural changes have been well studied for Caribbean and Indo-Pacific coral reefs (Pratchett et al., 2008; Hughes et al., 2010, 2018a,b; Roff and Mumby, 2012), especially mass coral bleaching events (e.g., Hughes et al., 2018a) and major shifts from coral to macroalgae, referred to as coral-algal phase shifts (Done, 1992; Knowlton, 1992; Hughes, 1994). Thus, macroalgae dominance is frequently related to areas degraded by herbivorous overfishing, eutrophication and coral mortality (Bellwood et al., 2004; Hughes et al., 2007; Mumby and Steneck, 2008). In the Southwest Atlantic, however, reefs are naturally characterized by low coral cover (Leão et al., 2003; Aued et al., 2018), even those geographically isolated and with little human influence. In fact, turf algae and macroalgae are the major components of reef benthic communities across all of the Brazilian Province, including oceanic islands (Pereira-Filho et al., 2011; Fonseca et al., 2012; Longo et al., 2015; Magalhães et al., 2015; Meirelles et al., 2015; Aued et al., 2018; Matheus et al., 2019). This distinct structure is possibly related to the setting of environmental conditions under which these ecosystems have developed over time. Unlike most tropical coral reefs, Southwestern Atlantic reefs developed under high influence of continental runoff, with high sediment and nutrient load, turbid waters and highly variable temperature and salinity, which characterize them as marginal reefs (according to Perry and Larcombe, 2003 definition). It also creates different responses than coral-dominated reefs, such as those related to disturbances and recovery potential (Connell, 1997; Roff and Mumby, 2012; Camp et al., 2018; Banha et al., 2019; Mies et al., 2020).
In spite of their differences from most tropical coral reefs, Southwestern Atlantic reefs, as well as other algae-dominated reefs, represent equally important ecosystems and perform essential ecological processes (Vroom et al., 2006). Yet, not unlike other reef areas, they have undergone exponential and deleterious pressure of unbridled urbanization over the past few years (Maida et al., 1995; Leão and Kikuchi, 2005), as well as coral bleaching episodes after thermal stress, especially El Niño events (Leão et al., 2016; Teixeira et al., 2019). There are even records of structural changes on benthic communities, with replacement of coral cover by zoanthid and macroalgae (Cruz et al., 2014b, 2018), as well as decrease on Sargassum spp. coverage and increase of turf algae (Gorman et al., 2019) at some localities along the Brazilian coast. Additionally, an increase in frequency, duration, intensity and extension of marine heatwaves has been recently reported in the South Atlantic (Rodrigues et al., 2019), and this is very likely to intensify ecosystem damages (Fordyce et al., 2019).
Although there are some temporal studies on reef benthic communities along the Brazilian coast, to date, only spatial or short-term assessments of reef benthic communities in Brazilian oceanic islands are available (Laborel, 1970; de Eston et al., 1986; Pires et al., 1992; Maida et al., 1995; Ferreira et al., 2006, 2012; Krajewski et al., 2010; Krajewski and Floeter, 2011; Pereira-Filho et al., 2011; Amado-Filho et al., 2012; Longo et al., 2015; Magalhães et al., 2015; Meirelles et al., 2015; Aued et al., 2018; Matheus et al., 2019) and the lack of methodological standardization jeopardizes temporal comparisons. Additionally, there is a gap in the understanding of environmental drivers that most influence communities’ structure, which has already been raised by other studies (Francini-Filho et al., 2013). Thus, we conducted the first effort to evaluate the temporal dynamics of reef benthic communities in Fernando de Noronha Archipelago (FNA), the largest Brazilian oceanic archipelago, using a 7-year assessment (2013–2019). We focused on major benthic groups interannual dynamics and investigated the influence of oceanographic parameters, seeking to characterize community structure fluctuations and to identify their main drivers. We also discussed possible future changes in a scenario of increasing climate and oceanographic disturbances, and local anthropogenic stressors.
Materials and Methods
Study Area
Fernando de Noronha is an archipelago formed by a main island and other 20 smaller islands located 200 miles from the Brazilian northeast coast (3.54°S; 32.25°W; Figure 1). With an emerged area of approximately 27 km2 and an insular shelf of 190 km2 and 80 m-depth, it is the largest Brazilian oceanic archipelago and is situated over the eastern extremity of the 75 km-diameter and 4,000 m-depth Fernando de Noronha Ridge (de Almeida, 2006).
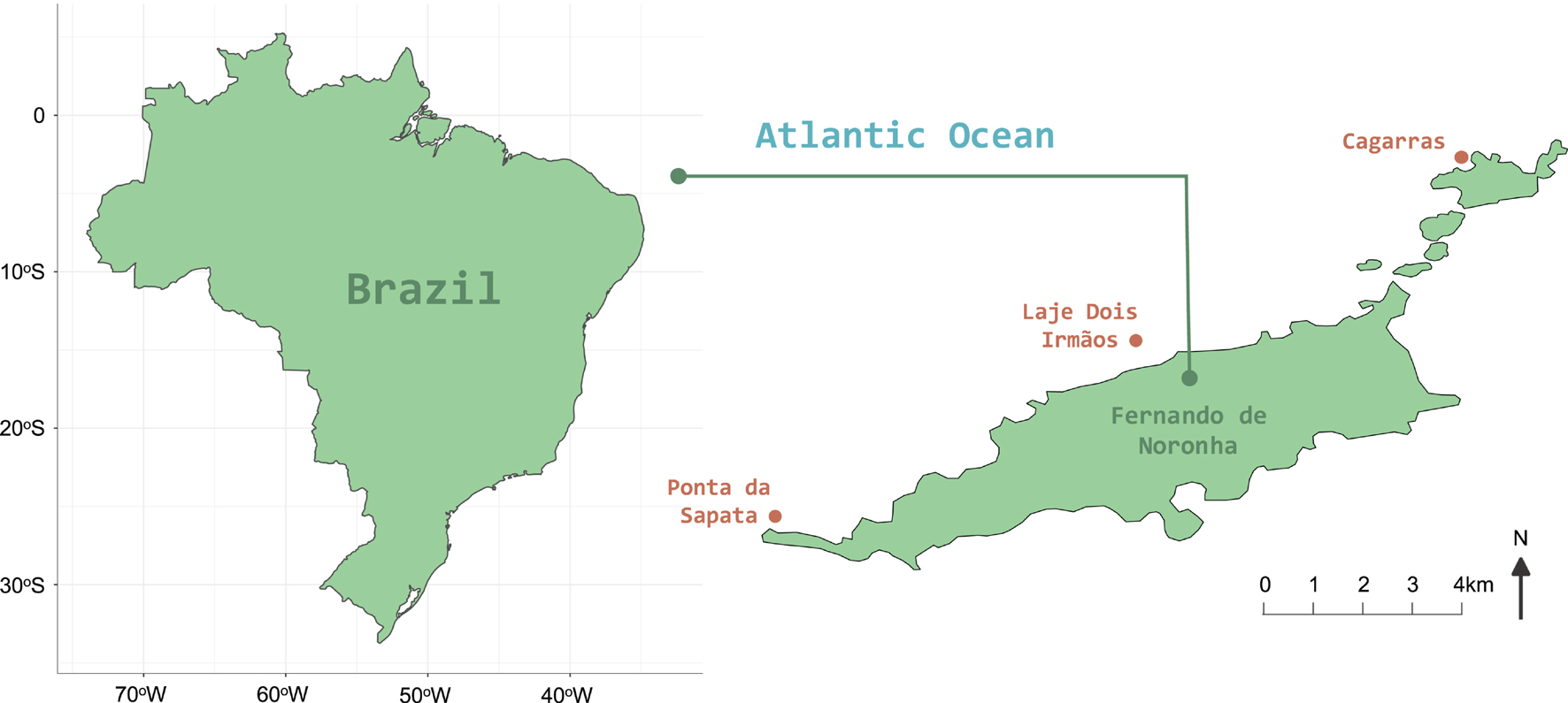
Figure 1. Study area. Location of Fernando de Noronha Archipelago at 200 miles from Brazil’s northeast coast. The three sampling sites detailed in brown were annually sampled in October from 2013 to 2019 in order to assess temporal dynamics of the reef benthic community.
The climate at FNA is tropical/oceanic with strong influence of southeastern trade winds, which makes the southeastern shore exposed to strong winds and high swells throughout the year, especially from April to November. The northwestern shore is protected from these prevailing winds, with low wave exposure from April to November, but it still faces high oceanic swells from November to March (Linsker, 2003). Mean sea surface temperature and salinity around the archipelago are about 27°C and 35.5, respectively (Linsker, 2003), which are influenced by a steep thermocline between 50 and 120 m-depth, by the oligotrophic South Equatorial Current at the surface and the South Equatorial Undercurrent at the subsurface (Travassos et al., 1999), and by occasional upwelling in the region (Matheus et al., 2019). There are only a few freshwater streams in the islands (de Eston et al., 1986).
Most FNA’s reefs occur at depths up to 60 m and are mostly constituted by volcanic rocks, highly exposed to wave action (Krajewski and Floeter, 2011; Matheus et al., 2019). Reef benthic community at depths up to 30 m is mainly composed by turf algae and macroalgae (de Eston et al., 1986; Krajewski and Floeter, 2011; Aued et al., 2018; Matheus et al., 2019), besides high cover of articulated coralline algae and crustose coralline algae (de Eston et al., 1986). Coral cover is usually low (0–5%) at reefs up to 10 m, but it increases at 20–30 m, with 20% cover (Krajewski and Floeter, 2011; Matheus et al., 2019). The most abundant coral is Montastraea cavernosa (de Eston et al., 1986; Pires et al., 1992; Ferreira et al., 2006; Krajewski and Floeter, 2011; Matheus et al., 2019), followed by Siderastrea stellata (at shallower reefs) and Mussismillia hispida (de Eston et al., 1986). From 40 to 50 m, reefs show higher cover of Porifera (Matheus et al., 2019) and there are reports of rhodolith beds all around the insular shelf from 10 to 100 m (Amado-Filho et al., 2012).
Since 1998, 75% of the archipelago is protected by the Fernando de Noronha Marine National Park, which imposes restrictions to certain activities, such as fishing up to 50 m-depth. The remnant area is protected by an Environmental Protection Area, which regulates the sustainable use of local natural resources, land occupation and tourism (Linsker, 2003).
Oceanographic Parameters Acquisition
In order to assess and describe oceanographic dynamics around FNA across the sampling period, we gathered data on “sea surface temperature” (sst 11 μm daytime) and ‘‘diffuse attenuation coefficient’’ (kd at 490 nm) with monthly satellite images estimated for a 4 km resolution global grid (L3 products) from the MODIS-Aqua mission through the OceanColor platform1 (NASA, 2019). Using the Windows Image Manager software (WIM v.11) (Kahru, 2018), we defined a 12 × 12 km-grid with central coordinate at FNA’s main island (3.54°S; 32.25°W) over each image (Supplementary Figure 1). From each grid we extracted monthly data on mean “sea surface temperature” and “diffuse attenuation coefficient” for the available historical series (2003–2020).
We gathered data on ‘‘marine heatwaves’’ (MHW) cumulative intensity for the available historical series (1984–2020) for FNA’s main island coordinate from the online Marine Heatwave Tracker2 (Schlegel, 2018), which categorizes marine heatwave’s intensity according to Hobday et al. (2018) (i.e., absent, moderate, strong, severe, and extreme), with a daily and 0.25° resolution. Finally, we gathered daily data on “wave energy” (WE) for the sampling period (2013–2019) for a 1 × 1°-grid over the archipelago’s northwestern shore from the WAVEWATCH III Global Wave Model dataset at the Coast Watch platform3 (The Wavewatch III Development Group [WW3DG], 2016; Supplementary Figure 2), by squaring “significant wave height,” multiplying it by “wave period” and averaging by month.
Benthic Community Sampling
In order to assess and describe reef benthic community temporal dynamics, we annually sampled the substrate cover from three sites within FNA’s main island using photoquadrats in the month of October, from 2013 to 2019 (Figure 1). The three sites were chosen in order to create spatial representativeness of FNA’s shallow reefs and their communities, and the location at the northwestern shore of the island, as well as the period of the year chosen to perform the samplings, was designed to make the field work safer, considering the lower wave action. At Cagarras (3°48′33.83″S; 32°23′23.54″W) and Ponta da Sapata (3°52′30.65″S; 32°28′27.61″W) we sampled reefs from 2 to 17 m-depth and at Laje Dois Irmãos (3°50′30.25″S; 32°25′51.63″W), which was included in 2014, we sampled reefs from 11 to 21 m-depth. Different depths were included because of different geomorphological shapes at each site. While Cagarras and Ponta da Sapata have shallow reefs, Laje Dois Irmãos has reefs only from 10 m-deep, which provided representativeness of different benthic groups. At each site we traced three to six 20 m-transects in parallel to the coastline and spaced apart by 2 m, and fixed them with rebar to maintain the same sampling area across the years. We sampled 10–11 50 × 50 cm photoquadrats at each transect each year, resulting in a total of 1,040 images across all the years. The variability in the number of transects and photoquadrats is due to the possibility of distributing transects on the reef while keeping their orientation in relation to the coastline and the distance between them, considering the different geomorphological features of each site. Samplings were performed under the activities of Long-Term Ecological Research at Brazilian Oceanic Islands (PELD-ILOC).4
Photoquadrat Processing
Image processing consisted on the identification of major taxonomic and functional benthic groups and on the estimate of their percentage cover in each sampling year. Therefore, we used the Coral Point Count with Excel extensions software (CPCe v. 4.1) (Kohler and Gill, 2006). We performed the analysis by overlaying 50 random points on each image and visually identifying the organisms below each point, gathering them into major groups according to their recognized role in the reef ecosystem: (i) calcifiers (corals, crustose, and articulated coralline algae); (ii) macroalgae; (iii) epilithic algal matrix (EAM—usually referred to as turf algae, it is a matrix of low lying filamentous and coralline algae, sediment and the associated cryptofauna and sessile invertebrates5); (iv) suspension/filter-feeders (sponge and ascidian); (v) cyanobacteria; (vi) zoanthid; and (vii) other invertebrates (starfish, polychaeta and bivalve mollusks) (Littler et al., 1983; Steneck and Dethier, 1994; Connell et al., 2014; Aued et al., 2018). Instead of depending strictly on species detection and loss, this approach focuses on a wider system functioning dynamic (Mouillot et al., 2013), because these major groups enable the detection in advance of structural and functional changes in the ecosystem. For example, the increase in EAM coverage may indicate increased physical disturbances (Littler and Littler, 1980; Cheroske et al., 2000), while the persistence of calcifiers coverage may indicate a more stable environment (Kleypas et al., 1999). Similarly, the increase in macroalgae and cyanobacteria coverages may signal the influence of increasing sea surface temperature (Robarts and Zohary, 1987).
Data Analysis
We first tested the overall benthic community temporal variability by applying a Multivariate Generalized Linear Model (mGLM; R “mvabund” package) with nBoot = 1,000, using all benthic groups percentage cover as response variable, and years as explanatory variable. Then, in order to test which major groups had eventual significant differences between years, we applied a Generalized Additive Mixed Model (GAMM; R “mgcv” package) for each group, using their percentage cover as response variable, years as explanatory variable and sites as random factor. Considering the proportion nature of data, we used a negative binomial distribution when running mGLM and a binomial distribution when running GAMM (Zuur et al., 2009). We also applied a Kruskal-Wallis analysis (KW) with a Tukey post hoc test (R “PMCMRplus” package) for each group in order to test in which years there were eventual significant differences. To illustrate it, we plotted the coverage of each group per year with stacked barplot and with boxplot (R “ggplot2” package). We also plotted frequency histograms for each of the most abundant groups per year.
Lastly, we tested the influence of oceanographic parameters on temporal dynamics of the benthic community structure by applying a Generalized Additive Mixed Model (GAMM; R “mgcv” package) for each of the major benthic groups that presented eventual significant differences between years. We used benthic groups percentage cover as response variable, and sites and other major groups as random factor, in order to isolate the influence of other groups on the response of the interest group. To define the most important explanatory variables, we used a Principal Component Analysis (R “vegan” package). For “sea surface temperature,” “diffuse attenuation coefficient,” and “wave energy” we used the average value of the 2 months prior samplings (August–September), considering that biological communities may present a delayed response to oceanographic conditions. For “marine heatwaves” we used the sum of cumulative intensities of all events between the sampling of interest and the previous sampling, considering the cumulative effect of marine heatwaves on biological communities. After applying these data in the Principal Component Analysis, we selected those variables that were most related to the first axis. These variables were then applied as explanatory variables to each GAMM, which were selected by the lowest AIC. Again, considering the proportion nature of data, we used a binomial distribution when running GAMM, and we applied a cubic regression spline as a smoothing method with knots = 7 (Zuur et al., 2009). We also plotted time series for all explanatory variables to support the models’ interpretation. Analysis and plots were made using R Studio software v. 1.1.453 (R Core Team, 2016).
Results
Oceanographic Setting
The sampling period was characterized by quite stable mean “sea surface temperature,” varying 2.15–2.75°C within each year, usually peaking in April (highest in 2019: 29.21°C) and reaching minimum values in August–September (lowest in 2015: 26.09°C; Figure 2A). However, there was a trend of increase in maximum values from 2014 to 2019 (28.71, 28.84, 29.04, 28.84, 29.09, and 29.21°C) and higher minimum values from 2016 to 2019 than previous years (average of 26.64 and 26.39°C, respectively). Regarding “marine heatwaves,” there are records of two moderate and one strong event just before the 2013 sampling, totalizing 32 days with a cumulative intensity of 24.15°C; two moderate events before the 2014 sampling (10 days and 9.15°C); one strong event before the 2015 sampling (9 days and 8.86°C); five moderate events before the 2016 sampling (50 days and 42.35°C); seven moderate events before the 2017 sampling (98 days and 80.45°C); two moderate events before the 2018 sampling (17 days and 12.25°C); and five moderate and one strong event before the 2019 sampling (128 days and 126.37°C; Figure 2A).
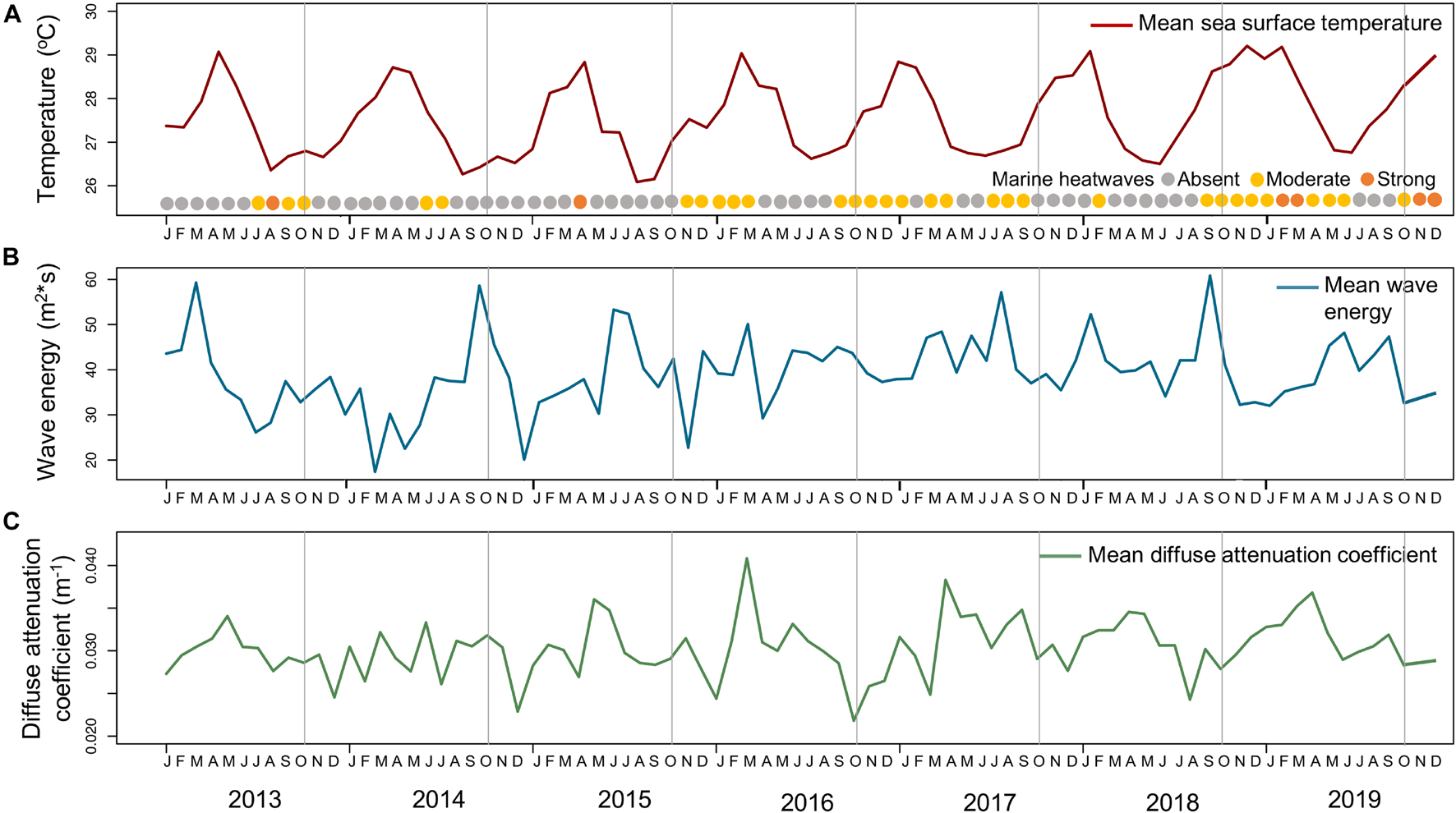
Figure 2. Oceanographic setting. (A) Monthly mean sea surface temperature (solid red line) during the 7-year monitoring in Fernando de Noronha Archipelago. Circles indicate the absence or presence of marine heatwaves per month and their category (gray—absent; yellow—moderate; orange—strong). (B) Monthly mean wave energy (solid blue line) during the 7-year monitoring in Fernando de Noronha Archipelago. (C) Monthly mean diffuse attenuation coefficient (solid green line) during the 7-year monitoring in Fernando de Noronha Archipelago. Sampling months are indicated by vertical solid gray lines.
In terms of mean “wave energy,” values varied 16.16–41.23 m2s within each year, however without a clear pattern (Figure 2B). The lowest “wave energy” was in March 2014 (17.39 m2s) and the highest in November 2018 (60.84 m2s). “Wave energy” also showed higher minimum values from 2017 to 2019 than previous years (average of 34.39 and 23.22 m2s, respectively) and overall monthly mean also higher (41.27 and 37.69 m2s, respectively). Lastly, regarding “diffuse attenuation coefficient,” values varied 0.0085–0.0190 m–1 within each year, peaking in May-June and reaching minimum values in November-December (Figure 2C). Both the lowest and the highest “diffuse attenuation coefficient” were in 2016 (0.0218 and 0.0408 m–1, respectively).
Based on the Principal Component Analysis, we selected “marine heatwaves” and “wave energy” as explanatory variables for the models (Supplementary Figure 3 and Supplementary Table 1). Both variables were mostly related to PC1 (86.98 and 70.56%, respectively), which explained 62.1% of data variation.
Benthic Community Structure and Temporal Dynamics
The most abundant benthic groups across all sampling years were EAM (minimum annual mean and standard deviation 22.72 ± 18.56%—maximum annual mean and standard deviation 60.22 ± 18.97%), macroalgae (14.63 ± 15.32%–34.67 ± 14.24%) and calcifiers (15.39 ± 14.20%–28.56 ± 14.65%), followed by cyanobacteria (0.66 ± 1.64%–37.49 ± 28.42%), suspension/filter-feeders (0.87 ± 2.91%–1.39 ± 3.12%), zoanthid (0 ± 0%–0.90 ± 7.32%) and other invertebrates (0 ± 0%–0.05 ± 0.34%) (Figure 3). Overall benthic community structure was significantly different between years (mGLM: test statistic 19.74, p-value = 0.000999) and it was a result of changes on cover of the three dominant groups (EAM: 11.3%, p-value < 2e-16; macroalgae: 2.23%, p-value < 2e-16; calcifiers: 0.63%, p-value < 2e-16) and cyanobacteria (41.9%, p-value < 2e-16) (Supplementary Table 2). GAMM also indicated eventual significant variations between years for suspension/filter-feeders (0.082%, p-value = 0.0288) and zoanthid (0.025%, p-value < 2e-16), however, Kruskal-Wallis test with Tukey post hoc did not show any differences (Supplementary Figure 4).
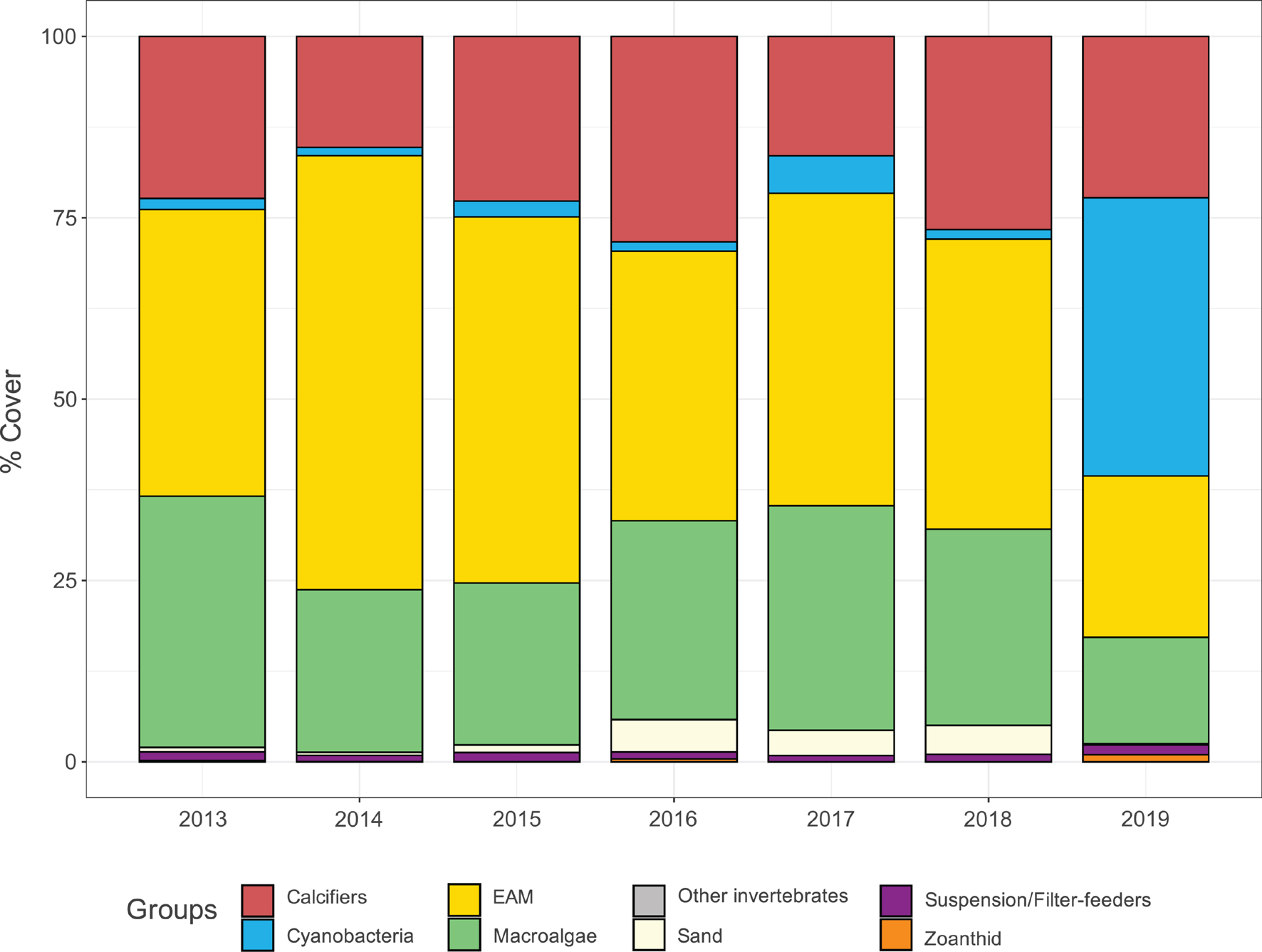
Figure 3. Yearly benthic community structure. Stacked barplot illustrating the yearly substrate cover pooled during the 7-year monitoring in Fernando de Noronha Archipelago in terms of major benthic groups and sand mean percentage cover.
In the first sampling year, EAM and macroalgae showed quite equitable coverages (annual mean and standard deviation 39.54 ± 17.01% and 34.67 ± 14.24%, respectively) (Figure 4). In 2014 EAM reached its highest percentage cover (60.22 ± 18.97%), whereas macroalgae decreased (22.39 ± 17.54%), as well as calcifiers (22.23 ± 12.47% in 2013 and 15.39 ± 14.20% in 2014). In 2015 and 2016, EAM’s coverage decreased (50.64 ± 18.82% and 37.60 ± 17.85%, respectively), while macroalgae and calcifiers increased (macroalgae: 22.60 ± 14.51% and 27.74 ± 18.27%, respectively; calcifiers: 22.82 ± 15.93% and 28.56 ± 14.65%, respectively), highlighting a period (2013–2016) in which these three groups showed opposite trends. From 2017 to 2018, variations in calcifiers’ coverage were accompanied by variations in cyanobacteria’s coverage (calcifiers: 16.52 ± 13% and 26.76 ± 17.66%, respectively; cyanobacteria: 4.89 ± 5.88% and 0.78 ± 2.11%, respectively). In the last sampling year, EAM and macroalgae decreased to their lowest percentage cover (22.72 ± 18.56% and 14.63 ± 15.32%, respectively), while cyanobacteria reached its highest coverage (37.49 ± 28.42%). Indeed, regarding EAM percentage cover, the most different years were 2014, 2015, and 2019, while for macroalgae it was 2019. Although calcifiers have shown temporal fluctuations, none of the years stood out among the others. Finally, regarding cyanobacteria, the most different years were 2017 and 2019 (Figure 4).
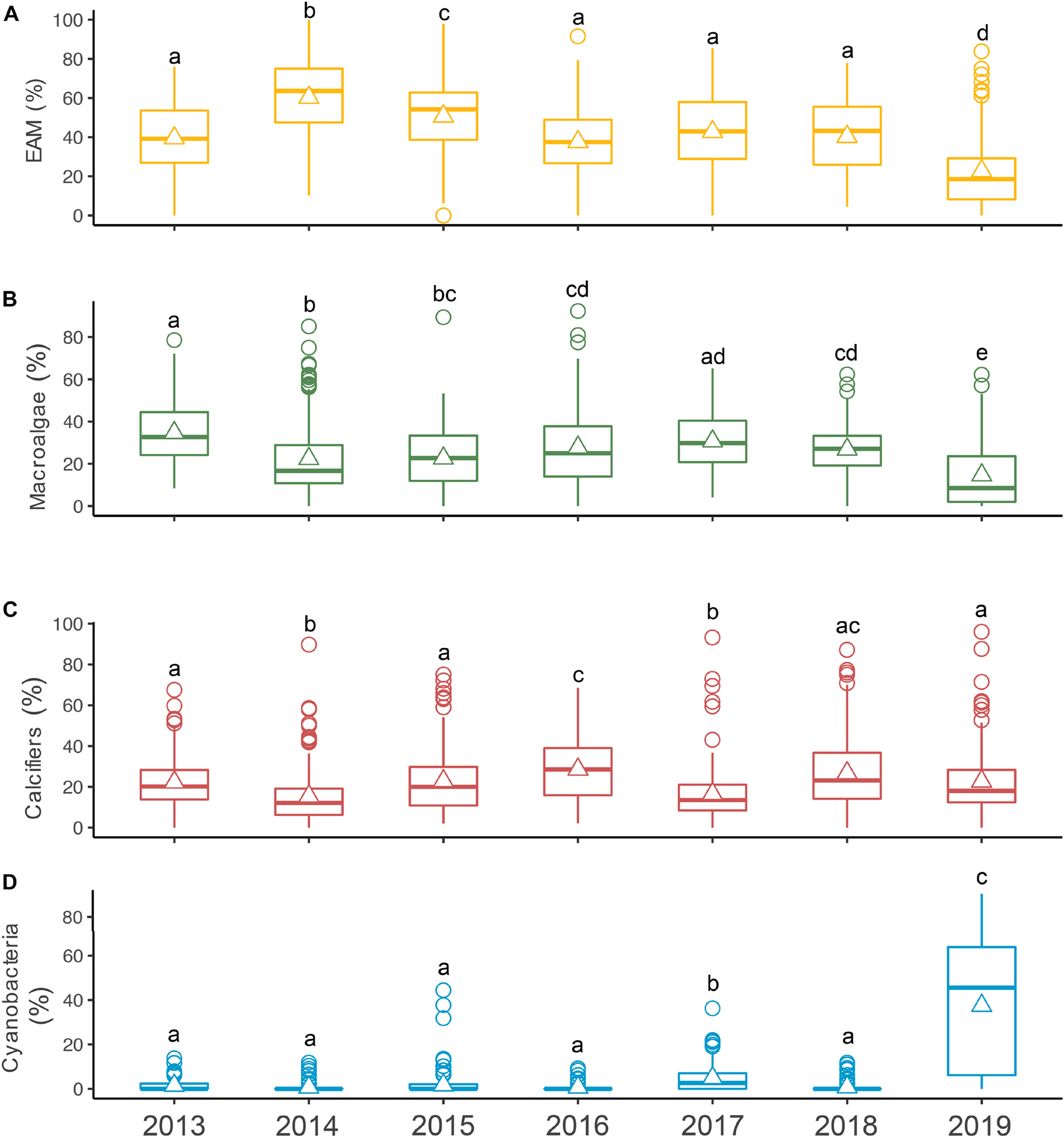
Figure 4. Benthic groups temporal dynamics. Boxplots illustrating temporal dynamics of (A) epilithic algal matrix (EAM; yellow), (B) macroalgae (green), (C) calcifiers (red) and (D) cyanobacteria (blue) during the 7-year monitoring in Fernando de Noronha Archipelago. Horizontal lines indicate median values, triangles indicate mean values and circles indicate outliers. Years within groups sharing the same letter are not significantly different (Kruskal-Wallis test with Tukey post hoc–p < 0.05).
In terms of the most abundant groups’ temporal dynamics, EAM’s coverage was distributed between 20 and 80%, with the exception of 2019, when its percentage cover was concentrated mostly between 0 and 20%. Macroalgae’s coverage was mostly distributed between 0 and 50%, with a higher concentration between 0 and 20% in 2019, while calcifiers’ percentage cover was mostly distributed between 0 and 40% across all sampling years. Regarding cyanobacteria, its coverage was concentrated between 0 and 10%, with the exception of 2019, when it was distributed between 0 and 80% (Figure 5). It is important to highlight the similar lateral displacement on coverages of macroalgae and calcifiers and the opposite trend of EAM’s coverage, especially between 2014 and 2016. It is also important to highlight the lateral displacement of EAM and macroalgae to lower coverages in 2019 and the opposite trend of cyanobacteria’s coverage.
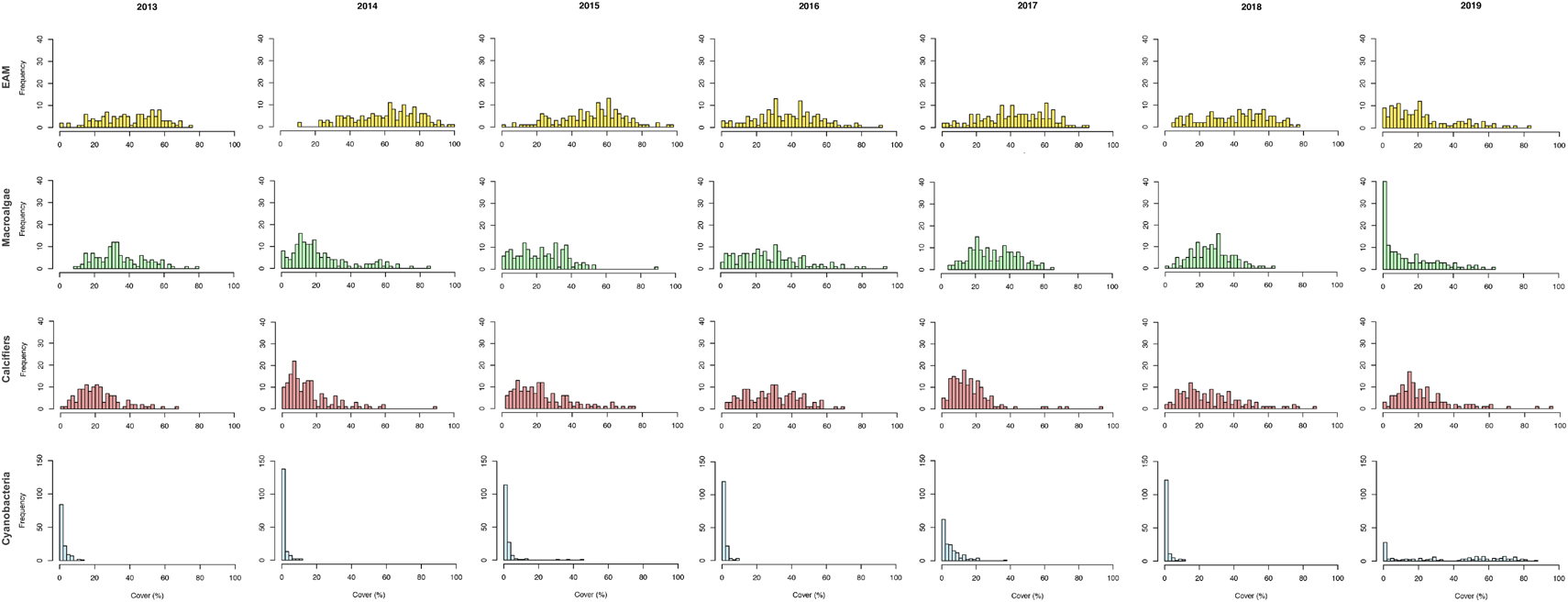
Figure 5. Frequency variation on benthic groups coverage. Frequency histograms of epilithic algal matrix (EAM; yellow), macroalgae (green), calcifiers (red) and cyanobacteria (blue) percentage cover during the 7-year monitoring in Fernando de Noronha Archipelago.
Since EAM, macroalgae, calcifiers, and cyanobacteria were the only groups to present significant differences between years, we investigated the influence of “marine heatwaves” and “wave energy” on each of them. EAM responded negatively to the increase in “marine heatwaves” (p-value = 0.00572) and positively to the increase in “wave energy” (p-value = 6.22e-16), with two optimal intervals and with 24.8% of its variation explained by the model (Figure 6 and Supplementary Table 3). Macroalgae responded positively to the increase in “marine heatwaves” (p-value < 2e-16), with two optimal intervals, however showing a decrease under extreme values of “marine heatwaves”. 10.6% of its variation were explained by the model (Figure 6 and Supplementary Table 3). Calcifiers responded negatively to the increase in “wave energy” (p-value < 2e-16), however with an interval of positive response to intermediate values, with 7.9% of its variation explained by the model (Figure 6 and Supplementary Table 3). Lastly, cyanobacteria responded positively to the increase in “marine heatwaves” (p-value < 2e-16), with 47.3% of its variation explained by the model (Figure 6 and Supplementary Table 3). It is important to highlight that the smoothing argument was significant for all the models (edf > 1), with the exception of EAM (Supplementary Table 3), indicating the non-linear response of the benthic groups to the oceanographic variables.
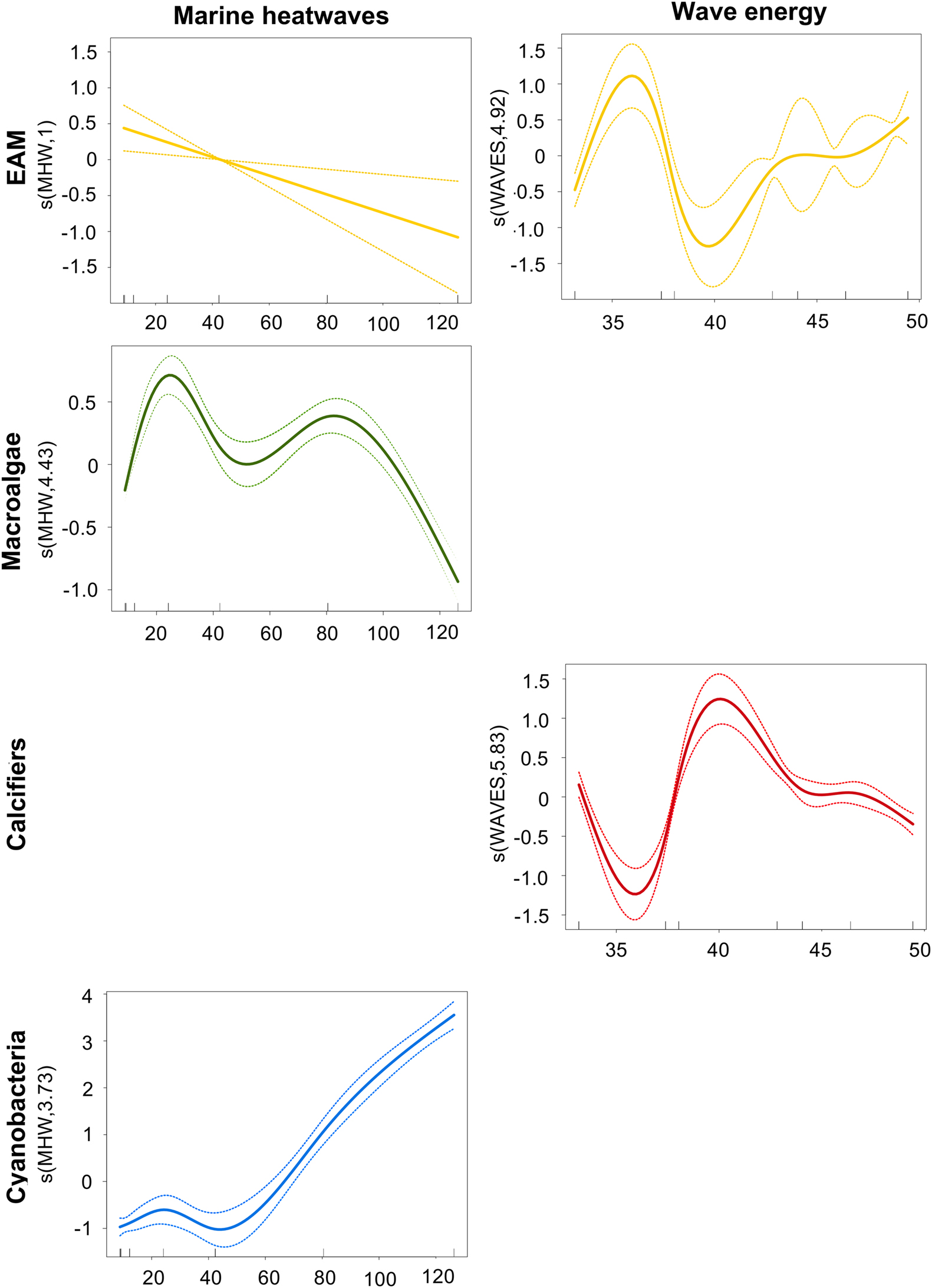
Figure 6. Benthic groups responses to oceanographic parameters. Generalized Additive Mixed Models plots for epilithic algal matrix (EAM; yellow), macroalgae (green), calcifiers (red) and cyanobacteria (blue) indicating their responses to “marine heatwaves” and “wave energy” over the 7-year monitoring in Fernando de Noronha Archipelago. The x axis represents the explanatory variable gradient (interval between the lower and the higher value found for “marine heatwaves” (°C) and “wave energy” (m2s) throughout the sampling period). The y axis represents the smoothed partial effect of each explanatory variable (“marine heatwaves” and “wave energy”) in each benthic group (EAM, macroalgae, calcifiers and cyanobacteria). The value in the parentheses in the y axis is the edf (effective degrees of freedom) and indicates if the benthic group has a linear (edf = 1) or a non-linear (edf > 1) response to the explanatory variable. Solid lines indicate fitted values and dashed lines indicate standard errors.
Discussion
We showed that epilithic algal matrix, macroalgae and calcifiers are the main components of the benthic community in Fernando de Noronha Archipelago’s reefs, followed at lower proportions by cyanobacteria, suspension/filter-feeders, zoanthid and other invertebrates. This structure was quite persistent throughout our 7-year assessment and underwent little changes over most monitoring, represented mainly by fluctuations on EAM, macroalgae, calcifiers, and cyanobacteria. The exception was 2019, when there was a substantial increase of cyanobacteria and a decrease of EAM and macroalgae. Considering that algae-dominated communities tend to be quite variable, changing seasonally or even within and between years (Steneck and Dethier, 1994; Vroom and Braun, 2010), fluctuations observed across the sampling period may reflect the community’s natural dynamics. Yet, significant correlations between major benthic groups and oceanographic parameters suggest that “marine heatwaves” and “wave energy” might be playing important roles in this dynamic, especially regarding cyanobacteria, EAM and macroalgae.
The overall community structure was consistent with the pre-existing information for FNA’s shallow reefs (de Eston et al., 1986; Krajewski and Floeter, 2011; Aued et al., 2018; Matheus et al., 2019). It was also comparable with reefs at similar depths from the other three Brazilian oceanic islands (Pereira-Filho et al., 2011; Fonseca et al., 2012; Longo et al., 2015; Magalhães et al., 2015; Meirelles et al., 2015), especially in terms of high abundance of turf algae (here referred to as EAM) and macroalgae. Indeed, the dominance of these two benthic groups was already described for reef benthic communities from all the Brazilian Province (Aued et al., 2018). Besides the high coverage, EAM seemed to be consistent over time in FNA, with abundance equivalent to or higher than macroalgae and higher than other groups, with the exception of cyanobacteria in 2019 (Figures 3, 4). Also, its fluctuations seemed to be oppositely related to macroalgae’s (2013–2016) and cyanobacteria’s (2019) fluctuations. EAM is known for being tolerant to physical disturbances (Littler and Littler, 1980; Cheroske et al., 2000) and for trapping high amounts of sediment (Airoldi, 1998; Connell et al., 2014). Even little fluctuations of sediment deposition are able to influence turf expansion (Airoldi, 1998). Thus, this group tends to be favored by “wave energy,” which explains its positive correlation with this variable (Figure 6), since it causes sediment resuspension and availability in the water column. It has already been observed at Rocas Atoll, where open pools, with higher hydrodynamics, show higher turf biomass than closed pools (Longo et al., 2015). Indeed, the possible influence of strong wave action and sediment input on high EAM abundance in FNA’s shallow reefs was already raised by previous studies (Krajewski and Floeter, 2011; Matheus et al., 2019), especially considering records of erosive events related to wind, waves and storm action at the archipelago (Castro, 2010). Alternatively, EAM showed a negative correlation with “marine heatwaves,” whereas macroalgae showed a positive correlation (Figure 6). Previous studies have shown that higher temperatures might favor EAM in occupying available substrate (Connell and Russell, 2010). However, macroalgae are also favored by warmer waters (Guimaraens and Coutinho, 1996; Harley et al., 2012; Koch et al., 2013; Ji et al., 2016). Considering that EAM is less competitive than macroalgae (Steneck and Dethier, 1994; O’Brien and Scheibling, 2018), the increase in macroalgae’s coverage may prevent EAM expansion through limiting available substrate. In this case, the negative correlation between EAM and “marine heatwaves” may be an indirect response to the increase of macroalgae with this variable.
Similarly to macroalgae, calcifiers also showed an opposite trend to EAM, both on coverage fluctuations (2013–2016; Figures 3, 4) and on responses to oceanographic variables (Figure 6). Calcifiers benefit from water transparency and light availability (Kleypas et al., 1999), which might explain its negative correlation with “wave energy.” However, this response may also be indirectly related to EAM response to this same variable, especially if we consider that corals and crustose coralline algae have slower responses in terms of coverage change. With higher EAM coverage, for example, calcifiers may be enclosed, reducing its coverage. Calcifiers’ fluctuations were also accompanied by changes in cyanobacteria (2017–2019; Figures 3, 4). This latter group is known for its rapid response to the increase on sea surface temperature (Robarts and Zohary, 1987), rapidly occupying available substrate and overgrowing other organisms (Kelly et al., 2011). Indeed, cyanobacteria was positively correlated to “marine heatwaves” (Figure 6) and showed an increase in 2017 after consecutive moderate events, and a substantial increase in 2019 after the strongest event ever recorded in FNA until that date since 1984 (Marine Heatwave Tracker, 2020). This might explain the changes in calcifiers’ coverage, despite no significative fluctuations in EAM and macroalgae in 2017 and 2018, and also the decrease in coverages of these two groups with extreme values of “marine heatwaves” in 2019.
Based on the oceanographic setting (Figure 2), it was possible to notice that the first year of sampling was a year with lower “wave energy” (Figure 2B) and with two moderate and one strong “marine heatwave” event preceding sampling (Figure 2A), which may have favored the higher coverage of macroalgae (Figures 3, 4). The following years (i.e., 2014 and 2015) were the years with fewer “marine heatwaves” (Figure 2A) and also with higher “wave energy” than 2013 (Figure 2B), which may have influenced the increase of EAM, consequently reducing macroalgae and calcifiers coverage (Figures 3, 4). The five moderate “marine heatwave” events in 2016 (Figure 2A) might have favored the following increase of macroalgae (Figures 3, 4) and even more events in 2017 (Figure 2A) might have induced the increase in cyanobacteria coverage (Figures 3, 4). Similarly to 2014 and 2015, in 2018 there were fewer “marine heatwaves” (Figure 2A), which may have led to cyanobacteria reduction, revealing calcifiers coverage (Figures 3, 4). Finally, 2019 stood out with the highest “marine heatwave” cumulative intensity (Figure 2A), which may have triggered the substantial increase of cyanobacteria in detriment of other groups (Figures 3, 4).
Considering major benthic groups fluctuations and their responses to oceanographic parameters, the overall benthic community dynamics seems to be dictated mainly by changes on EAM and macroalgae coverages, suggesting that Southwestern Atlantic marginal reefs might have a different dynamic than most tropical coral reefs. Instead of having corals and macroalgae as alternative states (Done, 1992; Knowlton, 1992; Hughes, 1994), FNA’s reefs might present EAM and macroalgae as dominant stable states, and fluctuations on community structure may be related to an inherent flickering dynamic between these two groups (Rindi et al., 2017). EAM and macroalgae coverages may be fluctuating according to the setting of oceanographic conditions, however neither of them completely dominate the substrate. This is probably because of the natural variation in oceanographic parameters (i.e., sea surface temperature and wave energy seasonality) and/or even due to the short duration of more intense and specific disturbances (i.e., marine heatwaves). These events might induce changes on the overall community structure, however, do not last long enough or do not occur so often to trigger a permanent change.
Turf-forming algae are less competitive in comparison to macroalgae, however, it is an opportunistic group and is facilitated by macroalgae reduction, rapidly replacing it after disturbances (Steneck and Dethier, 1994; Airoldi, 1998). This trade-off has already been described, with turf algae coverage being shaped by both the macroalgae removal and the abiotic conditions, according to which the system “flickers” between two potential stable states (i.e., turf-dominated and macroalgae-dominated) (Rindi et al., 2017). Nevertheless, the turf-dominated state is more likely to be established when macroalgae removal is higher than 70%, a threshold from which macroalgae loses ability to prevent turf algae propagation (Rindi et al., 2017). Thus, unless there is a substantial reduction on macroalgae coverage and a setting of abiotic conditions to maintain the competitive balance in favor of turf algae, the flickering dynamic is likely to persist (O’Brien and Scheibling, 2018).
Coral reefs all around the globe have experienced structural changes from coral-dominance to algae-dominance. Yet, some studies have also discussed the loss of canopy-forming macroalgae and the expansion of turf-forming algae on marine ecosystems across the globe (Benedetti-Cecchi et al., 2001; Gorgula and Connell, 2004; Connell et al., 2008; Filbee-Dexter and Wernberg, 2018; Gorman et al., 2019; Tebbett and Bellwood, 2019), in most cases linked to increasing anthropic impacts and rising sea surface temperature. These dynamics, together with the temporal stability of turf algae (Gordon et al., 2016) and its high tolerance to disturbances (Littler and Littler, 1980; Airoldi, 1998; Cheroske et al., 2000), suggest a possible rearrangement of reef benthic communities around less complex stable states (i.e., reef flattening) (e.g., Tebbett et al., 2019), including other possible alternative states afterward, such as cyanobacterial mats (e.g., de Bakker et al., 2017). Indeed, proliferation of cyanobacterial mats have been widely reported on reef benthic communities after thermal disturbances (Beltram et al., 2019) and here we show a substantial increase of cyanobacteria in algae-dominated reefs after a very intense marine heatwave.
The flickering dynamics between EAM and macroalgae, on the one hand, signals the persistence of the benthic community structure within the sampling period, but the increase of cyanobacteria, on the other hand, makes room to discuss potential structural changes on FNA’s reef benthic community in a scenario of increasing oceanographic and climate disturbances. More frequent, intense, long-lasting and wider marine heatwaves are expected to occur globally (Oliver et al., 2018, 2019), including the South Atlantic (Rodrigues et al., 2019), with structural consequences for reef environments (Fordyce et al., 2019). Predictions indicate that marine heatwaves are expected to be worse in the tropics, with the first year with permanent events occurring within the next 20 years (Frolicher and Laufkotter, 2018; Oliver et al., 2019). Indeed, 2020 has already stood out among previous years, with the strongest marine heatwave ever recorded in FNA since 1984 (161 days and 188.9°C of cumulative intensity), which was followed by other moderate events (Marine Heatwave Tracker, 2020). Cyanobacteria is very likely to benefit and expand its coverage with these increasing thermal anomalies (Robarts and Zohary, 1987; de Bakker et al., 2017). Macroalgae is also likely to benefit to some extent with higher temperatures, both by the positive effect on its metabolism (Harley et al., 2012; Koch et al., 2013; Ji et al., 2016) and by the predicted decline in fish herbivory (Inagaki et al., 2020). EAM, in turn, might benefit with strong wave energy from seasonal swells (Matheus et al., 2019). However, it is also important to mention other variables that could influence the benthic community, such as nutrients. Although protected by conservation units, FNA is one of the most famous tourist spots in Brazil and, besides its 6,000 habitants, the island receives about 90,000 tourists per year (Matheus et al., 2019), which may enhance nutrient input in the water column from tourist activities and sewage discharge, and add bottom-up sources of variation. More frequent and intense marine heatwaves combined to strong wave energy and possibly enhanced nutrient inputs, thus, may narrow the recovery window for some benthic groups and/or widen favorable condition for others, driving the community toward a tipping point.
Our study provides valuable insights about the structure of the reef benthic community in the largest Brazilian oceanic archipelago, as well as its natural fluctuations, and the oceanographic drivers influencing its dynamics. The study also helps to understand how a marginal reef might respond to different oceanographic conditions, especially in a context of increasingly frequent and intense climate disturbances, providing groundwork for adaptive management and resilience maintenance efforts (Briske et al., 2017). In this case, the ecosystem’s resilience might be assessed through persistence of benthic community structure over time. Our study also stresses the importance of looking to more local extreme climate events, such as marine heatwaves (Fordyce et al., 2019), considering the quite stable mean sea surface temperature (Figure 2A) even in years of strong El-Niño events in the Pacific Ocean (i.e., El-Niño 2015/2016). Although algae dominated reefs are usually associated with degraded environments, they represent ecosystems as important as coral reefs and perform essential ecological processes (Vroom et al., 2006), especially in oligotrophic oceanic waters, contributing to primary production and structural complexity. Besides its rocky origin, high coverages of crustose and articulated coralline algae together with corals make reefs at the FNA an important carbonate system for Tropical Southwestern Atlantic oceanic waters (Matheus et al., 2019). We emphasize the importance of maintaining standardized protocols for monitoring community and oceanographic drivers in order to better understand and predict long-term structural changes at important Southwestern Atlantic marginal reefs, and also the need for taking these ecosystems into account on global reef assessments.
Conclusion
Here we presented the first effort to evaluate the temporal dynamics of reef benthic communities in Fernando de Noronha Archipelago (FNA), the largest Brazilian oceanic archipelago, using a 7-year interannual assessment (2013–2019) of major benthic groups with photoquadrats methodology. We also investigated the influence of oceanographic parameters through satellite and modeling data, seeking to identify the main drivers of this dynamics. We showed that epilithic algal matrix (EAM), macroalgae and calcifiers are the main components of the benthic community in FNA’s reefs, followed at lower proportions by cyanobacteria, suspension/filter-feeders, zoanthid and other invertebrates. We also showed that, besides natural fluctuations, marine heatwaves and wave energy might be playing important roles in the community dynamics. The dominance of EAM and macroalgae was already described for reefs along the Brazilian Province and here we demonstrated the persistence of this structure over the years in FNA, with the exception of 2019, when there was a substantial increase of cyanobacteria after a strong marine heatwave. Our results suggest a flickering dynamic between EAM and macroalgae, which vary according to the oceanographic conditions, reinforcing its distinct dynamics from most tropical coral reefs, with EAM and macroalgae as dominant stable states, instead of corals and macroalgae. However, the increase of cyanobacteria added to projections of more frequent and stronger marine heatwaves worldwide, and to local anthropogenic stressors, indicate possible structural changes in this community. Our study helps to understand how a marginal reef might respond to different oceanographic conditions, especially in a context of increasingly frequent and intense climate disturbances, providing groundwork for adaptive management. We emphasize the importance of continued monitoring of community and oceanographic drivers for better understanding and predicting changes in important Southwestern Atlantic marginal reefs, and also the need for taking these ecosystems into account on global reef assessments.
Data Availability Statement
The raw data supporting the conclusions of this article will be made available by the authors, without undue reservation.
Author Contributions
JB, AA, and BS conceived the study idea. JB and BS organized and participated in samplings. CE contributed with oceanographic parameters acquisition. JB analyzed photoquadrats and cured the data and wrote the original draft of the manuscript. JB and LM-S performed statistical analysis. JB, AA, LM-S, VP, CE, and BS reviewed and edited the manuscript. All authors contributed to the article and approved the submitted version.
Funding
This research was funded by the Long Term Ecological Research of Reef Communities at Oceanic Islands (PELD/ILOC—CNPq 441241/2016-6; CEL Ferreira PI), by the Coordination for the Improvement of Higher Education Personnel (CAPES) through master’s scholarship to JB and VP, and by the Oceanic modeling development focusing on generation of future global climate change scenarios using the global climate model BESM at the continental shelf and coastal zone of Brazil (ModCosta—CAPES 88887.145863/2017-00) through financing JB’s round trip to FNA. AA was supported by a postdoctoral scholarship granted by CNPq/CAPES/FAPs/BC—Fundo Newton—Pesquisa Ecológica de Longa Duração (CNPQ-CAPES-PELD) and LM-S was supported by a postdoctoral grant awarded by CAPES-Brazil (Coordenação de Aperfeiçoamento de Pessoal de Nível Superior).
Conflict of Interest
The authors declare that the research was conducted in the absence of any commercial or financial relationships that could be construed as a potential conflict of interest.
Publisher’s Note
All claims expressed in this article are solely those of the authors and do not necessarily represent those of their affiliated organizations, or those of the publisher, the editors and the reviewers. Any product that may be evaluated in this article, or claim that may be made by its manufacturer, is not guaranteed or endorsed by the publisher.
Acknowledgments
We are grateful to the PELD/ILOC team for financial, logistical and fieldwork support, and to the Fernando de Noronha Archipelago Marine National Park (PARNAM Fernando de Noronha) and Chico Mendes Institute of Biodiversity Conservation (ICMBio) for providing research permits and logistical support. We also wish to thank the Ecology Post-Graduate Program—Federal University of Santa Catarina (PPGECO/UFSC) and Oceanography Post-Graduate Program—Federal University of Santa Catarina (PPGOceanografia/UFSC) members for scientific support. We acknowledge CAPES and ModCosta for financial support. The content of this manuscript has appeared online as part of the Master’s thesis of Julia Biscaia Zamoner (Zamoner, 2018).
Supplementary Material
The Supplementary Material for this article can be found online at: https://www.frontiersin.org/articles/10.3389/fmars.2021.762453/full#supplementary-material
Footnotes
- ^ oceancolor.gsfc.nasa.gov/l3/
- ^ http://www.marineheatwaves.org/tracker.html
- ^ coastwatch.pfeg.noaa.gov/erddap/griddap/NWW3_Global_Best
- ^ https://peldiloc.sites.ufsc.br/
- ^ Since it is not possible to identify each component of this matrix through photoquadrats, we considered it as specific benthic group, but opted for the term EAM instead of turf algae because it better reflects the heterogeneity and complexity of this group (Wilson et al., 2003).
References
Airoldi, L. (1998). Roles of disturbance, sediment stress, and substratum retention on spatial dominance in algal turf. Ecology 79, 2759–2770.
Alvarez-Filip, L., Dulvy, N. K., Gill, J. A., Côté, I. M., and Watkinson, A. R. (2009). Flattening of Caribbean coral reefs: region-wide declines in architectural complexity. Proc. R. Soc. B Biol. Sci. 276, 3019–25. doi: 10.1098/rspb.2009.0339
Amado-Filho, G. M., Pereira-Filho, G. H., Bahia, R. G., Abrantes, D. P., Veras, P. C., and Matheus, Z. (2012). Occurrence and distribution of rhodolith beds on the Fernando de Noronha Archipelago of Brazil. Aquat. Bot. 101, 41–45. doi: 10.1016/j.aquabot.2012.03.016
Aued, A. W., Smith, F., Quimbayo, J. P., Cândido, D. V., Longo, G. O., Ferreira, C. E. L., et al. (2018). Large-scale patterns of benthic marine communities in the Brazilian Province. PLoS One 13:e0198452. doi: 10.1371/journal.pone.0198452
Banha, T. N. S., Capel, K. C. C., Kitahara, M. V., Francini-Filho, R. B., Francini, C. L. B., Sumida, P. Y. G., et al. (2019). Low coral mortality during the most intense bleaching event ever recorded in subtropical Southwestern Atlantic reefs. Coral Reefs 39, 515–521. doi: 10.1007/s00338-019-01856-y
Bellwood, D. R., Hughes, T. P., Folke, C., and Nystrom, M. (2004). Confronting the coral reef crisis. Nature 429, 827–833. doi: 10.1038/nature02691
Beltram, F. L., Lamb, R. W., Smith, F., and Witman, J. D. (2019). Rapid proliferation and impacts of cyanobacterial mats on Galapagos rocky reefs during the 2014–2017 El Niño Southern Oscillation. J. Exp. Mar. Biol. Ecol. 51, 18–26. doi: 10.1016/j.jembe.2019.03.007
Benedetti-Cecchi, L., Pannacciulli, F., Bulleri, F., Moschella, P. S., Airoldi, L., Relini, G., et al. (2001). Predicting the consequences of anthropogenic disturbance: large-scale effects of loss of canopy algae on rocky shores. Mar. Ecol. Progr. Ser. 214, 137–150.
Briske, D. D., Illius, A. W. and Anderies, J. M. (2017). “Nonequilibrium Ecology and Resilience Theory,” in Springer Series on Environmental Management, ed. D. Briske (Cham: Springer), 197–227.
Brustolin, M. C., Nagelkerken, I., Ferreira, C. M., Goldenberg, S. U., Ullah, H., and Fonseca, G. (2019). Future ocean climate homogenizes communities across habitats through diversity loss and rise of generalist species. Glob. Change Biol. 25, 3539–3548. doi: 10.1111/gcb.14745
Camp, E. F., Schoepf, V., Mumby, P. J., Hardtke, L. A., Rodolfo-Metalpa, R., Smith, D. J., et al. (2018). The future of coral reefs subject to rapid climate change: lessons from natural extreme environments. Front. Mar. Sci. 5:4. doi: 10.3389/fmars.2018.00004
Castro, J. W. A. (2010). Ilhas oceânicas da Trindade e Fernando de Noronha, Brasil: uma visão da Geologia Ambiental. Rev. Gestão Costeira Integrada 10, 303–319.
Cheroske, A. G., Williams, S. L., and Carpenter, R. C. (2000). Effects of physical and biological disturbances on algal turfs in Kaneohe Bay, Hawaii. J. Exp. Mar. Biol. Ecol. 248, 1–34.
Connell, S. D., and Russell, B. D. (2010). The direct effects of increasing CO2 and temperature on non-calcifying organisms: increasing the potential for phase shifts in kelp forests. Proc. R. Soc. B Biol. Sci. 277, 1409–15. doi: 10.1098/rspb.2009.2069
Connell, S. D., Foster, M. S., and Airoldi, L. (2014). What are algal turfs? Towards a better description of turfs. Mar. Ecol. Progr. Ser. 495, 299–307. doi: 10.3354/meps10513
Connell, S. D., Russel, B. D., Turner, D. J., Shepherd, S. A., Kildea, T., Miller, D., et al. (2008). Recovering a lost baseline: missing kelp forests from a metropolitan coast. Mar. Ecol. Progr. Ser. 360, 63–72. doi: 10.3354/meps07526
Cruz, I. C. S., Kikuchi, R. K. P., and Creed, J. C. (2014a). Improving the construction of functional models of alternative persistent states in coral reefs using insights from ongoing research programs: a discussion paper. Mar. Environ. Res. 97, 1–9. doi: 10.1016/j.marenvres.2014.01.003
Cruz, I. C. S., Kikuchi, R. K. P., Longo, L. L., and Creed, J. C. (2014b). Evidence of a phase shift to Epizoanthus gabrieli Carlgreen, 1951 (Order Zoanthidea) and loss of coral cover on reefs in the Southwest Atlantic. Mar. Ecol. 36, 318–325. doi: 10.1111/maec.12141
Cruz, I. C. S., Waters, L. G., Kikuchi, R. K. P., Leão, Z. M. A. N., and Turra, A. (2018). Marginal coral reefs show high susceptibility to phase shift. Mar. Pollut. Bull. 135, 551–561. doi: 10.1016/j.marpolbul.2018.07.043
Darling, E. S., Graham, N. A. J., Januchowski-Hartley, F. A., Nash, K. L., Pratchett, M. S., and Wilson, S. K. (2017). Relationships between structural complexity, coral traits, and reef fish assemblages. Coral Reefs 36, 561–575. doi: 10.1007/s00338-017-1539-z
de Almeida, F. F. M. (2006). Ilhas oceânicas e suas relações com a tectônica atlântica. TerrÆ Didaìtica 2, 3–18.
de Bakker, D. M., van Duyl, F. C., Bak, R. P. M., Nugues, M. M., Nieuwland, G., and Meesters, E. H. (2017). 40 Years of benthic community change on the Caribbean reefs of Curaçao and Bonaire: the rise of slimy cyanobacterial mats. Coral Reefs 36, 355–367. doi: 10.1007/s00338-016-1534-9
de Eston, V. R., Migotto, A. E., de Oliveira-Filho, E. C., Rodrigues, S. A., and Freitas, J. C. (1986). Vertical distribution of benthic marine organisms on rocky coasts of the Fernando de Noronha Archipelago (Brazil). Boletim Instituto Oceanograìfico 34, 37–53.
Done, T. J. (1992). Phase shifts in coral reef communities and their ecological significance. Hydrobiologia 247, 121–132.
Ferreira, B. P., Costa, M. B. S. F., Coxey, M. S., Gaspar, A. L. B., Veleda, D., and Araujo, M. (2012). The effects of sea surface temperature anomalies on oceanic coral reef systems in the southwestern tropical Atlantic. Coral Reefs 32, 441–454. doi: 10.1007/s00338-012-0992-y
Ferreira, B. P., Maida, M., Castro, C. B., Pires, D. O., D’amico, T. M., Prates, A. P. L., et al. (2006). The status of coral reefs in Brazil. Int. Coral Reef Symp. 10 Proc. 10th Int. Coral Reef Symp. 1, 1011–1015.
Filbee-Dexter, K., and Wernberg, T. (2018). Rise of Turfs: a new battlefront for globally declining kelp forests. Bioscience 68, 64–76. doi: 10.1093/biosci/bix147
Fiori, S., and Carcedo, M. (2013). “Benthic community and climate change,” in Marine Ecology in a Changing World, eds A. H. Arias and M. C. Menéndez (Boca Raton: Science Publishers), 121–139.
Fonseca, A. C., Villaça, R., and Knoppers, B. (2012). Reef flat community structure of Atol das Rocas, Northeast Brazil and Southwest Atlantic. J. Mar. Biol. 2012:179128. doi: 10.1155/2012/179128
Fordyce, A. J., Ainsworth, T. D., Heron, S. F., and Leggat, W. (2019). Marine Heatwave Hotspots in Coral Reef Environments: physical drivers, ecophysiological outcomes, and impact upon structural complexity. Front. Mar. Sci. 6:498. doi: 10.3389/fmars.2019.00498
França, F. M., Benkwitt, C. E., Peralta, G., Robinson, J. P. W., Graham, N. A. J., Tylianakis, J. M., et al. (2020). Climatic and local stressor interactions threaten tropical forests and coral reefs. Philos. Trans. R. Soc. B Biol. Sci. 375:20190116. doi: 10.1098/rstb.2019.0116
Francini-Filho, R. B., Coni, E. O. C., Meirelles, P. M., Amado-Filho, G. M., Thompson, F. L., Pereira-Filho, G. H., et al. (2013). Dynamics of coral reef benthic assemblages of the Abrolhos bank, Eastern Brazil: inferences on natural and anthropogenic drivers. PLoS One 8:e54260. doi: 10.1371/journal.pone.0054260
Friedlander, A. M., and DeMartini, E. E. (2002). Contrasts in density, size, and biomass of reef fishes between the northwestern and the main Hawaiian islands: the effects of fishing down apex predators. Mar. Ecol. Progr. Ser. 230, 253–264.
Frolicher, T. L., and Laufkotter, C. (2018). Emerging risks from marine heat waves. Nat. Commun. 9:650. doi: 10.1038/s41467-018-03163-6
Gordon, S. E., Goatley, C. H. R., and Bellwood, D. R. (2016). Composition and temporal stability of turf sediments on inner-shelf coral reefs. Mar. Pollut. Bull. 111, 178–183. doi: 10.1016/j.marpolbul.2016.07.013
Gorgula, S. K., and Connell, S. D. (2004). Expansive covers of turf-forming algae on human- dominated coast: the relative effects of increasing nutrient and sediment loads. Mar. Biol. 145, 613–619. doi: 10.1007/s00227-004-1335-5
Gorman, D., Horta, P., Flores, A. A., Turra, A., Berchez, F. A. S., Batista, M. B., et al. (2019). Decadal losses of canopy-forming algae along the warm temperate coastline of Brazil. Glob. Change Biol. 26, 1446–1457. doi: 10.1111/gcb.14956
Graham, N. A. J., and Nash, K. L. (2012). The importance of structural complexity in coral reef ecosystems. Coral Reefs 32, 315–326. doi: 10.1007/s00338-012-0984-y
Guimaraens, M. A., and Coutinho, R. (1996). Spatial and temporal variation of benthic marine algae at the Cabo Frio upwelling region, Rio de Janeiro, Brazil. Aquat. Bot. 52, 283–299.
Halpern, B. S., Frazier, M., Potapenko, J., Casey, K. S., Koenig, K., Longo, C., et al. (2015). Spatial and temporal changes in cumulative human impacts on the world’s ocean. Nat. Commun. 6:7615. doi: 10.1038/ncomms8615
Harley, C. D. G., Anderson, K. M., Demes, K. W., Jorve, J. P., Kordas, R. L., and Coyle, T. A. (2012). Effects of climate changfe on global seaweed communities. J. Phycol. 48, 1064–78. doi: 10.1111/j.1529-8817.2012.01224.x
Hatcher, B. G. (1988). Coral reef primary productivity: a beggar’s banquet. Trends Ecol. Evol. 3, 106–111. doi: 10.1016/0169-5347(88)90117-6
Hatcher, B. G. (1997). Coral reef ecosystems: how much greater is the whole than the sum of the parts? Coral Reefs 16, S77–S91. doi: 10.1007/s003380050244
Hobday, A., Oliver, E., Gupta, A. S., Benthuysen, J., Burrows, M., Donat, M., et al. (2018). Categorizing and naming marine heatwaves. Oceanography 31, 162–173. doi: 10.5670/oceanog.2018.205
Hoegh-Guldberg, O. (1999). Climate change, coral bleaching and the future of the world’s coral reefs. Mar. Freshw. Res. 50, 839–866. doi: 10.1071/mf99078
Hoegh-Guldberg, O. (2004). “Coral reefs and projections of future change,” in Coral Health Disease, eds E. Rosenberg and Y. Loya (Berlin: Springer), 463–484.
Hoegh-Guldberg, O. (2010). Coral reef ecosystems and anthropogenic climate change. Reg. Environ. Change 11, 215–227. doi: 10.1007/s10113-010-0189-2
Hu, S., Sprintall, J., Guan, C., McPhaden, M. J., Wang, F., Hu, D., et al. (2020). Deep-reaching acceleration of global mean ocean circulation over the past two decades. Sci. Adv. 6:eaax7727. doi: 10.1126/sciadv.aax7727
Hughes, T. P. (1994). Catastrophes, phase shift and large scale degradation of a Caribbean coral reef. Science 265, 1547–1551.
Hughes, T. P., Anderson, K. D., Connolly, S. R., Heron, S. F., Kerry, J. T., Lough, J. M., et al. (2018a). Spatial and temporal patterns of mass bleaching of corals in the Anthropocene. Science 359, 80–83. doi: 10.1126/science.aan8048
Hughes, T. P., Kerry, J. T., Baird, A. H., Connolly, S. R., DIetzel, A., Eakin, C. M., et al. (2018b). Global warming transforms coral reef assemblages. Nature 556, 492–496. doi: 10.1038/s41586-018-0041-2
Hughes, T. P., Graham, N. A. J., Jackson, J. B. C., Mumby, P. J., and Steneck, R. S. (2010). Rising to the challenge of sustaining coral reef resilience. Trends Ecol. Evol. 25, 633–642. doi: 10.1016/j.tree.2010.07.011
Hughes, T. P., Rodrigues, M. J., Bellwood, D. R., Ceccarelli, D., Hoegh-Guldberg, O., McCook, L., et al. (2007). Phase shifts, herbivory, and the resilience of coral reefs to climate change. Curr. Biol. 17, 360–365. doi: 10.1016/j.cub.2006.12.049
Inagaki, K. Y., Pennino, M. G., Floeter, S. R., Hay, M. E., and Longo, G. O. (2020). Trophic interactions will expand geographically but be less intense as oceans warm. Glob. Change Biol. 26, 6805–6812. doi: 10.1111/gcb.15346
Ji, Y., Xu, Z., Zou, D., and Gao, K. (2016). Ecophysiological responses of marine macroalgae to climate change factors. J. Appl. Phycol. 28, 2953–2967. doi: 10.1007/s10811-016-0840-5
Kelly, L. W., Barott, K. L., Dinsdale, E., Friedlander, A. M., Nosrat, B., Obura, D., et al. (2011). Black reefs: iron-induced phase shifts on coral reefs. ISME J. 6, 638–649. doi: 10.1038/ismej.2011.114
Kleypas, J. A., McManus, J. W., and Meñez, L. A. B. (1999). Environmental limits to coral reef development: where do we draw the line? Am. Zool. 39, 146–159.
Knowlton, N. (1992). Thresholds and multiple stable states in coral reef community dynamics. Am. Zool. 32, 674–682. doi: 10.1093/icb/32.6.674
Knowlton, N., Brainard, R. E., Fisher, R., Moews, M., Plaisance, L., and Caley, M. J. (2010). “Coral Reef Biodiversity,” in Life in The World’s Oceans, ed. A. D. McIntyre (Hoboken: Blackwell Publishing Ltd), 65–78.
Koch, M., Bowes, G., Ross, C., and Zhang, X. H. (2013). Climate change and ocean acidification effects on seagrasses and marine macroalgae. Glob. Change Biol. 19, 103–132. doi: 10.1111/j.1365-2486.2012.02791.x
Kohler, K. E., and Gill, S. M. (2006). Coral Point Count with Excel extensions (CPCe): a visual basic program for the determination of coral and substrate coverage using random point count methodology. Comp. Geosci. 32, 1259–1269. doi: 10.1016/j.cageo.2005.11.009
Krajewski, J. P., and Floeter, S. R. (2011). Reef fish community structure of the Fernando de Noronha Archipelago (Equatorial Western Atlantic): the influence of exposure and benthic composition. Environ. Biol. Fishes 92, 25–40. doi: 10.1007/s10641-011-9813-3
Krajewski, J. P., Floeter, S. R., Jones, G. P., and Leite, F. P. P. (2010). Patterns of variation in behaviour within and among reef fish species on an isolated tropical island: influence of exposure and substratum. J. Mar. Biol. Assoc. U. K. 91, 1359–1368. doi: 10.1017/s0025315410000111
Laborel, J. L. (1970). Les peuplements de madreporaires des côtes tropicales du Brésil. Abidjan: Ann. Univ. D’Abidjan.
Leão, Z. M. A. N., and Kikuchi, R. K. P. (2005). A relic coral fauna threatened by global changes and human activities, Eastern Brazil. Mar. Pollut. Bull. 51, 599–611. doi: 10.1016/j.marpolbul.2005.04.024
Leão, Z. M. A. N., Kikuchi, R. K. P., and Testa, V. (2003). Corals and coral reefs of Brazil. Latin Am. Coral Reefs 2003, 9–52.
Leão, Z. M. A. N., Kikuchi, R. K. P., Ferreira, B. P., Neves, E. G., Sovierzoski, H. H., Oliveira, M. D. M., et al. (2016). Brazilian coral reefs in a period of global change: a synthesis. Braz. J. Oceanogr. 64, 97–116. doi: 10.1590/s1679-875920160916064sp2
Linsker, R. (2003). Arquipélago de Fernando de Noronha: o paraiìso do vulcão. São Paulo: Terra Virgem.
Littler, M. M., and Littler, D. S. (1980). The evolution of Thallus form and survival strategies in Benthic Marine Macroalgae: field and laboratory tests of a functional form model. Am. Nat. 116, 25–44.
Littler, M. M., Littler, D. S., and Taylor, P. R. (1983). Evolutionary strategies in a tropical barrier reef system: functional form groups of marine macroalgae. J. Phycol. 19, 229–237.
Longo, G. O., Morais, R. A., Martins, C. D. L., Mendes, T. C., Aued, A. W., Cândido, D. V., et al. (2015). Between-habitat variation of benthic cover, reef fish assemblage and feeding pressure on the Benthos at the Only Atoll in South Atlantic: rocas Atoll, NE Brazil. PLoS One 10:e0127176. doi: 10.1371/journal.pone.0127176
Magalhães, G. M., Amado-Filho, G. M., Rosa, M. R., Moura, R. L., Brasileiro, P. S., De Moraes, F. C., et al. (2015). Changes in benthic communities along a 0–60 m depth gradient in the remote St. Peter and St. Paul Archipelago (Mid-Atlantic Ridge, Brazil). Bull. Mar. Sci. 91, 377–396. doi: 10.5343/bms.2014.1044
Maida, M., Ferreira, B. P., and Bellini, C. (1995). Avaliação preliminar do recife da Baiìa do Sueste, Fernando de Noronha, com ênfase nos corais escleractiìneos. Boletim Técnico Cientiìfico Cepene. 3, 37–47.
Marine Heatwave Tracker (2020). Marine Heatwave Tracker. Available Online at: http://www.marineheatwaves.org/tracker.html [Accessed May 15, 2019].
Matheus, Z., Francini-Filho, R. B., Pereira-Filho, G. H., Moraes, F. C., Moura, R. L., Brasileiro, P. S., et al. (2019). Benthic reef assemblages of the Fernando de Noronha Archipelago, tropical South-west Atlantic: effects of depth, wave exposure and cross-shelf positioning. PLoS One 14:e0210664. doi: 10.1371/journal.pone.0210664
Meirelles, P. M., Amado-Filho, G. M., Pereira-Filho, G. H., Pinheiro, H. T., Moura, R. L., Joyeux, J. C., et al. (2015). Baseline Assessment of Mesophotic Reefs of the Vitoìria-Trindade Seamount Chain Based on Water Quality, Microbial Diversity, Benthic Cover and Fish Biomass Data. Plos One 10:e0130084. doi: 10.1371/journal.pone.0130084
Mies, M., Francini-Filho, R. B., Zilberberg, C., Garrido, A. G., Longo, G. O., Laurentino, E., et al. (2020). South Atlantic coral reefs are major global warming refugia and less susceptible to bleaching. Front. Mar. Sci. 7:514. doi: 10.3389/fmars.2020.00514
Mouillot, D., Graham, N. A. J., Villéger, S., Mason, N. W. H., and Bellwood, D. R. (2013). A functional approach reveals community responses to disturbances. Trends Ecol. Evol. 28, 167–177. doi: 10.1016/j.tree.2012.10.004
Mumby, P., and Steneck, R. (2008). Coral reef management and conservation in light of rapidly evolving ecological paradigms. Trends Ecol. Evol. 23, 555–563. doi: 10.1016/j.tree.2008.06.011
NASA (2019). NASA Goddard Space Flight Center, Ocean Ecology Laboratory, Ocean Biology Processing Group. Data from: Moderate-resolution Imaging Spectroradiometer (MODIS) Aqua Ocean Color Data. South Dakota: DAAC.
Neves, C. F., and Muehe, D. (2008). Vulnerabilidade, impactos e adaptaçãão a mudanças do clima: a zona costeira. Parcerias Estratégicas 13, 217–295.
O’Brien, J. M., and Scheibling, R. E. (2018). Turf wars: competition between foundation and turf-forming species on temperate and tropical reefs and its role in regime shifts. Mar. Ecol. Progr. Ser. 590, 1–17. doi: 10.3354/meps12530
Oliver, E. C. J., Burrows, M. T., Donat, M. G., Gupta, A. S., Alexander, L. V., Perkins-Kirkpatrick, S. E., et al. (2019). Projected marine heatwaves in the 21st century and the potential for ecological impact. Front. Mar. Sci. 6:734. doi: 10.3389/fmars.2019.00734
Oliver, E. C. J., Donat, M. G., Burrows, M. T., Moore, P. J., Smale, D. A., Alexander, L. V., et al. (2018). Longer and more frequent marine heatwaves over the past century. Nat. Commun. 9:1324. doi: 10.1038/s41467-018-03732-9
Pandolfi, J. M., Bradbury, R. H., Sala, E., Hughes, T. P., Bjorndal, K. A., Cooke, R. G., et al. (2003). Global Trajectories of the Long-Term Decline of Coral Reef Ecosystems. Science 301, 955–958.
Pereira-Filho, G. H., Amado-Filho, G. M., Guimarães, S. M. P. B., Moura, R. L., Sumida, P. Y. G., Abrantes, D. P., et al. (2011). Reef fish and benthic assemblages of the Trindade and Martin Vaz Island group, Southwestern Atlantic. Braz. J. Oceanogr. 59, 201–212.
Perry, C. T., and Larcombe, P. (2003). Marginal and non-reef-building coral environments. Coral Reefs 22, 427–432. doi: 10.1007/s00338-003-0330-5
Pires, D. O., Castro, C. B., Migotto, A. E., and Marques, A. C. (1992). Cnidaìrios bentônicos do Arquipélago de Fernando de Noronha, Brasil. Bolm. Mus. Nac. Zool. Rio Janeiro 354, 1–21.
Pratchett, M. S., Munday, P. L., Wilson, S. K., Graham, N. A. J., Cinner, J. E., Bellwood, D. R., et al. (2008). Effects of climate-induced coral bleaching on coral-reef fishes - ecological and economic consequences. Oceanogr. Mar. Biol. Ann. Rev. 46, 251–296.
Putman, R. J. (1984). Temporal Change in Community Structure and Function, in Principles of Ecology. Berlin: Springer.
R Core Team (2016). R: A Language Environment for Statistical Computing. Vienna: R Foundation for Statistical Computing.
Reaka-Kudla, M. L. (1997). “The global biodiversity of coral reefs: a comparison with rainforests,” in Biodiversity II: Understanding and protecting our biological resources, ed. M. L. Reaka-Kudla (Washington: National Academy Press), 83–108.
Rindi, L., Bello, M. D., Dai, L., Gore, J., and Benedetti-Cecchi, L. (2017). Direct observation of increasing recovery length before collapse of a marine benthic ecosystem. Nat. Ecol. Evol. 1:153. doi: 10.1038/s41559-017-0153
Risk, M. J., and Edinger, E. (2011). “Impacts of Sediment on Coral Reefs,” in Encyclopedia of Modern Coral Reefs, ed. D. Hopley (Berlin: Springer), 575–586.
Robarts, R. D., and Zohary, T. (1987). Temperature effects on photosynthetic capacity, respiration, and growth rates of bloom-forming cyanobacteria. New Zeal. J. Mar. Freshw. Res. 21, 391–399. doi: 10.1080/00288330.1987.9516235
Rodrigues, R. R., Taschetto, A. S., Gupta, A. S., and Foltz, G. R. (2019). Common cause for severe droughts in South America and marine heatwaves in the South Atlantic. Nat. Geosci. 12, 620–626. doi: 10.1038/s41561-019-0393-8
Roff, G., and Mumby, P. J. (2012). Global disparity in the resilience of coral reefs. Trends Ecol. Evol. 27, 404–413. doi: 10.1016/j.tree.2012.04.007
Rogers, A., Blanchard, J. L., and Mumby, P. J. (2014). Vulnerability of Coral Reef Fisheries to a Loss of Structural Complexity. Curr. Biol. 24, 1000–1005. doi: 10.1016/j.cub.2014.03.026
Sandin, S. A., Smith, J. E., DeMartini, E. E., Dinsdale, E. A., Donner, S. D., Friedlander, A. M., et al. (2008). Baselines and Degradation of Coral Reefs in the Northern Line Islands. PLoS One 3:e1548. doi: 10.1371/journal.pone.0001548
Schlegel, R. W. (2018). Marine Heatwave Tracker: The App to See When and Where Marine Heatwaves are Happening Around the World. Available Online at: http://www.marineheatwaves.org/tracker (accessed May 15, 2019).
Sousa, W. P. (2001). “Natural disturbance and dynamics of marine benthic communities,” in Marine Community Ecology, eds M. D. Bertness, S. D. Gaines, and M. E. Hay (Sunderland: Sinauer Associates), 85–130.
Steffen, W., Hughes, L., Alexander, D., and Rice, M. (2017). Cranking up the Intensity: Climate Change and Extreme Weather Events. Climate Council of Australia Limited.
Steneck, R. S., and Dethier, M. N. (1994). A functional group approach to the structure of algal- dominated communities. Oikos 69, 476–498.
Sully, S., Burkepile, D. E., Donovan, M. K., Hodgson, G., and Van Woesik, R. (2019). A global analysis of coral bleaching over the past two decades. Nat. Commun. 10:1264. doi: 10.1038/s41467-019-09238-2
Tebbett, S. B., and Bellwood, D. R. (2019). Algal turf sediments on coral reefs: what’s known and what’s next. Mar. Pollut. Bull. 149:110542. doi: 10.1016/j.marpolbul.2019.110542
Tebbett, S. B., Streit, R. P., and Bellwood, D. R. (2019). A 3D perspective on sediment accumulation in algal turfs: implications of coral reef flattening. J. Ecol. 108, 70–80. doi: 10.1111/1365-2745.13235
Teixeira, C. D., Leitão, R. L. L., Ribeiro, F. V., Moraes, F. C., Neves, L. M., Bastos, A. C., et al. (2019). Sustained mass coral bleaching (2016–2017) in Brazilian turbid-zone reefs: taxonomic, cross-shelf and habitat-related trends. Coral Reefs 38, 801–813. doi: 10.1007/s00338-019-01789-6
The Wavewatch III Development Group [WW3DG] (2016). User manual and system documentation of WAVEWATCH III version 5.16: Technical Note. Washington: NOAA.
Travassos, P., Hazin, F. H. V., Zagaglia, J. R., Adviìncula, R., and Schober, J. (1999). Thermohaline structure around seamounts and islands off North-Eastern Brazil. Arch. Fish. Mar. Res. 47, 211–222.
Vroom, P. S., and Braun, C. L. (2010). Benthic Composition of a Healthy Subtropical Reef: baseline species-level cover, with an emphasis on algae, in the Northwestern Hawaiian Islands. PLoS One 5:e9733. doi: 10.1371/journal.pone.0009733
Vroom, P. S., Page, K. N., Kenyon, J. C., and Brainard, R. E. (2006). Algae dominated reefs: numerous reports suggest that reefs must be dominated by coral to be healthy, but many thriving reefs depend more on algae. Am. Sci. 94, 430–437.
Wilson, S. K., Bellwood, D. R., Choat, J. H., and Furnas, M. (2003). “Detritus in the epilithic algal matrix and its use by coral reef fishes,” in Oceanography and Marine Biology: an Annyal Review, eds R. N. Gibson and R. J. A. Atkinson (Milton Park: Taylor & Francis), 279–309.
Wolkovich, E. M., Cook, B. I., McLauchlan, K. K., and Davies, T. J. (2014). Temporal ecology in the Anthropocene. Ecol. Lett. 17, 1365–1379. doi: 10.1111/ele.12353
Woodhead, A. J., Hicks, C. C., Norstrom, A. V., Williams, G. J., and Graham, N. A. J. (2019). Coral reef ecosystem services in the Anthropocene. Funct. Ecol. 33, 1023–1034. doi: 10.1111/1365-2435.13331
Zamoner, J. (2018). O papel de parâmetros oceanográficos na dinâmica temporal (2013-2018) da comunidade bentônica recifal do arquipélago de Fernando de Noronha. Ph. D. thesis. Florianópolis: Universidade Federal de Santa Catarina.
Keywords: temporal dynamics, Brazilian reef, turf algae, macroalgae, cyanobacteria, Fernando de Noronha Archipelago, environmental factors, marine heatwaves
Citation: Biscaia Zamoner J, Aued AW, Macedo-Soares LCP, Picolotto VAP, Eiras Garcia CA and Segal B (2021) Integrating Oceanographic Data and Benthic Community Structure Temporal Series to Assess the Dynamics of a Marginal Reef. Front. Mar. Sci. 8:762453. doi: 10.3389/fmars.2021.762453
Received: 21 August 2021; Accepted: 11 October 2021;
Published: 01 November 2021.
Edited by:
Irene D. Alabia, Hokkaido University, JapanReviewed by:
José Lino Vieira De Oliveira Costa, University of Lisbon, PortugalHanzhi Lin, Science Systems and Applications, Inc., United States
Wei-Chuan Chiang, Council of Agriculture, Taiwan
Copyright © 2021 Biscaia Zamoner, Aued, Macedo-Soares, Picolotto, Eiras Garcia and Segal. This is an open-access article distributed under the terms of the Creative Commons Attribution License (CC BY). The use, distribution or reproduction in other forums is permitted, provided the original author(s) and the copyright owner(s) are credited and that the original publication in this journal is cited, in accordance with accepted academic practice. No use, distribution or reproduction is permitted which does not comply with these terms.
*Correspondence: Julia Biscaia Zamoner, jubiscaia.oceano@gmail.com