- 1Department of Ocean Engineering and Marine Sciences, Florida Institute of Technology, Melbourne, FL, United States
- 2St. Johns River Water Management District, Palatka, FL, United States
Organic-rich sediments in estuaries and the coastal ocean are often a product of land clearing, runoff of excess nutrients and other human activities. They can harbor pollutants, oxygen-consuming microbes and toxic hydrogen sulfide (H2S), thereby creating a hostile environment for infauna. In one barrier island lagoon, the Indian River Lagoon (IRL), Florida, layers of organic-rich sediments have increased substantially in thickness and areal extent over the past 60 years. Geochemical properties of these muddy sediments have been described; however, less is known about their habitability. We analyzed infauna and geochemical properties of 102 samples taken during wet and dry seasons at 17 locations spanning 60 km of the lagoon. We quantified infaunal abundance and diversity (Shannon-Wiener, H′) and determined Pearson’s correlation coefficients for effective number of species (ENS = eH′) vs. sediment porosity (ϕ = 0.69–0.95), organic carbon (1–8%), nitrogen (0.1–0.7%), silt + clay (16–99%), porewater H2S (5–3,600 μM), and other environmental variables. Small bivalves accounted for 70% of the organisms collected, followed by gastropods, polychaetes and other biota. The bivalves were predominantly Macoma spp., Mulinia lateralis and Parastarte triquetra with average abundances of 3,896, 2,049, and 926 individuals per m2, respectively. High abundance of some species, such as Macoma, showed that these opportunists had adapted to poor quality sediments. More than two-thirds of the 35 species collected were present at <100 individuals per m2 of sediment. Cluster analysis identified four groups of stations with significantly different geochemical properties. Permutation analyses of variance indicated that the four groups also represented statistically different infaunal communities. Diversity decreased with increasing sediment concentrations of organic carbon, nitrogen and silt + clay; however, community richness at our most prolific station along the perimeter of muddy deposits was ∼7 times lower than found previously in sandy sediments from the IRL. The results identified areas where infaunal communities have experienced the greatest stress due to accumulation of organic-rich sediments. Results from this study help support management plans for remediation of organic-rich mud and improvement of sediment and water quality, especially in areas identified with low ENS.
Introduction
Estuarine eutrophication has long prompted a global call for action by environmental scientists and managers (e.g., Rhyther and Dunstan, 1971; Paerl, 2006). Impacts from eutrophication in estuaries and the coastal ocean include nutrient loading, disruption of marine ecosystems and increases in sediment organic matter (OM) (Zimmerman and Canuel, 2000; Andersen et al., 2006; Lu et al., 2020). Organic-rich sediments harbor contaminants, store nutrients, and create anoxic benthic environments (National Research Council, 1989; Southwell et al., 2010). Increased deposition rates for OM in sediments also alter microbiomes and induce significant shifts in sediment chemistry, including releases of dissolved nutrients to the overlying water column (Cloern, 2001). Management strategies that improve water quality and ecosystem health require a better understanding of connections between environmental variables and biological abundance and diversity.
Human impacts within watersheds, including agriculture, land clearing and urban development, can increase sedimentation rates in estuaries and lead to progressive increases in nutrient loading and eutrophication (Cronin and Vann, 2003). Such impacts create poor water quality, loss of seagrasses, decreased fisheries and increased potential for harmful algal blooms (HABs) and fish kills (Unsworth et al., 2018). Estuaries, especially barrier island lagoons, are subject to these effects because they often have limited exchange with the coastal ocean. Therefore, future management decisions regarding remediation of organic-rich sediments should be based on a detailed understanding of how and why benthic biota respond to these deposits.
Bacterial decomposition of OM in organic-rich sediments creates anoxia and toxic concentrations of dissolved ammonium (NH4+) and hydrogen sulfide (H2S) (Gu et al., 1987; Hitchcock et al., 2010). Exposure to elevated concentrations of NH4+ and H2S greatly shorten times for the onset of adverse health effects and mortality in benthic communities (Kemp et al., 2005; Long and Seitz, 2009). Anoxia or a low concentration of dissolved oxygen (DO) has been linked to reduced growth rates and biomass for many marine organisms; low DO also is lethal to macrofauna when concentrations remain <1.4 mg/L for 1–2 weeks (Josefson and Widbom, 1988). In Chesapeake Bay, increased periods of low DO were strongly and negatively correlated with the integrity of benthic communities (Dauer et al., 2000).
Multiple investigations have identified natural and anthropogenic impacts, including increased OM and sedimentation rates, that alter benthic communities by limiting diversity and allowing more aggressive, opportunistic organisms to excel (Pearson and Rosenberg, 1978; Alongi, 1989; Norkko et al., 2006). As sandy sediment becomes buried and replaced by higher porosity, organic-rich sediment, benthic community abundance and diversity decrease because biota are unable to cope with OM degradation and resulting hypoxia/anoxia (Chou et al., 2004; Hale et al., 2016). Various forms of the Pearson-Rosenberg (P-R) model have shown that decreasing numbers of taxa, abundances of organisms and biomass are functions of increased OM and contaminants (Pearson and Rosenberg, 1978; Thompson and Lowe, 2004; Hyland et al., 2005).
Inputs of OM from rivers have, in some cases, increased macro-faunal abundance; however, when sediment total organic carbon (TOC) exceeded 1.0–1.5%, the number of taxa was reduced (Burd et al., 2008). Numbers of taxa and abundances also decreased when concentrations of sediment total nitrogen (TN) exceeded 0.4% (Burd et al., 2008). One study conducted in a sub-tropical estuary near Hong Kong found that the diversity of the microbenthic communities correlated negatively with TOC, total Kjeldahl nitrogen, NH4+ and total phosphorus (TP) in sediments (Shin et al., 2008). Sediment type and grain size also have been shown to be major factors affecting community diversity and abundance (Lin et al., 2018). For example, Chou et al. (2004) concluded that family richness and abundance decreased in benthic habitats with 10–65% increases in silt + clay.
Our study focused on organic-rich sediments in the Indian River Lagoon (IRL), a barrier island lagoon in Florida. Organic-rich sediments in the IRL typically contain >60% silt + clay, >75% water by weight, >10% OM, and >100 μM dissolved H2S (Trefry and Trocine, 2011; Fox and Trefry, 2018). These sediments release dissolved nutrients that fuel algal blooms (Fox and Trefry, 2018). Along with increased algal blooms, the IRL has experienced reductions in seagrass, decreased water quality and fish kills (Phlips et al., 2015). The goal of this study was to improve our understanding of the link between environmental variables and the abundance and diversity of benthic biota in organic-rich sediments in the IRL. Such knowledge supports management decisions related to successful remediation of fine-grained, organic-rich sediments in the lagoon and elsewhere.
Materials and Methods
Study Area
The IRL is a barrier island lagoon that extends ∼250 km along the central east coast of Florida with a width of 1–8 km, mostly wind-driven currents and limited oceanic exchange via just five inlets to the Atlantic Ocean. Salinities in the IRL typically range from 15 to 40 (Morris and Virnstein, 2004). The average depth of the IRL is ∼1.7 m (Smith, 2007).
Samples were collected from nine areas along ∼60 km of the IRL from Cocoa to Saint Sebastian River (Figure 1). These samples included mouths of tributaries plus areas known from previous studies to have anoxic, organic-rich, muddy sediment. The average water depth for areas with muddy sediments was 2.5 ± 1.0 m with a range of 0.9–4.9 m. These muddy sediments cover an area of <10% of the lagoon; however, they were estimated to be the source of >25% of total dissolved inorganic N and P fluxes to the lagoon (Trefry and Trocine, 2011; Fox and Trefry, 2018; Tetra Tech Inc and Closewaters LLC, 2021).
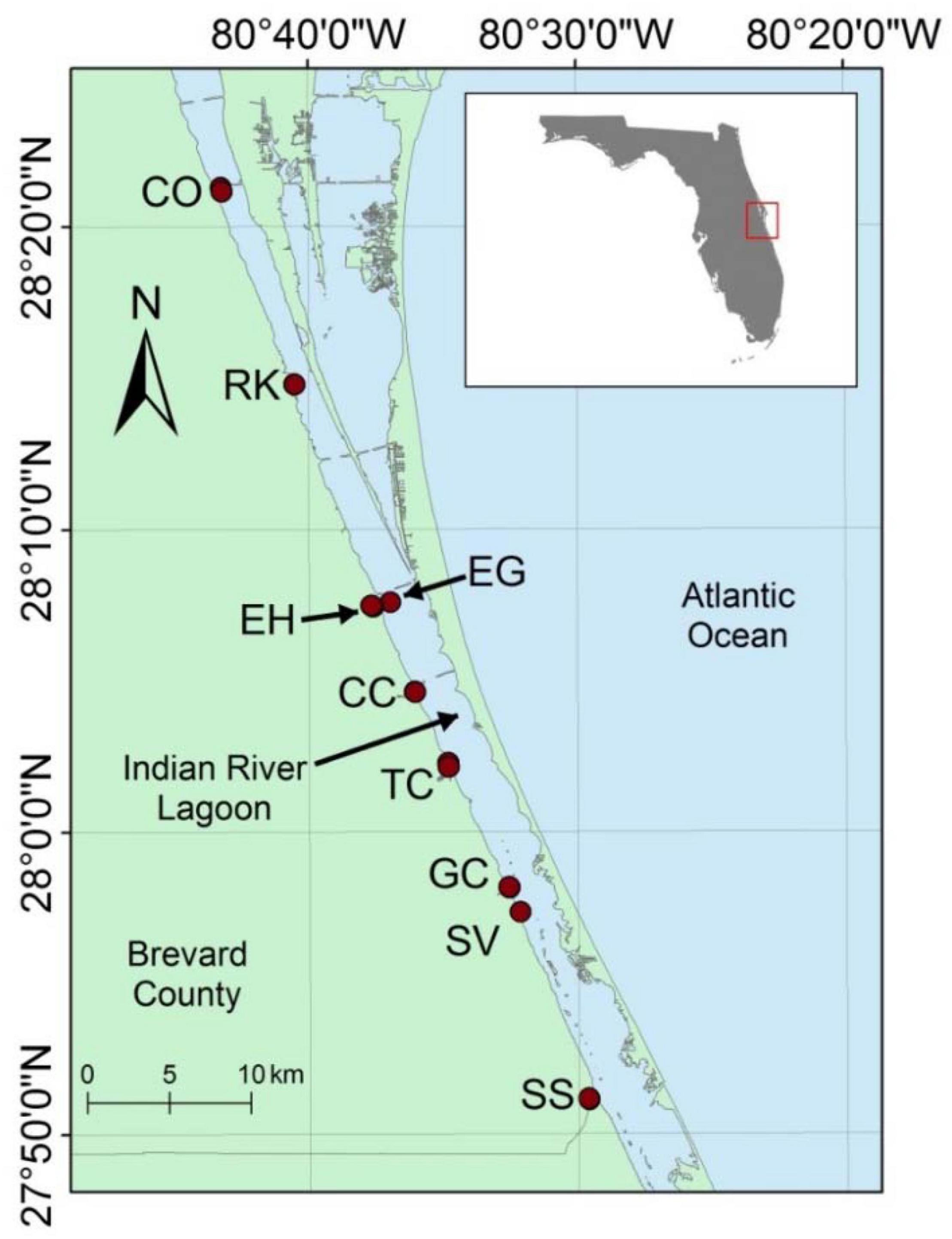
Figure 1. Map showing sampling locations in the Indian River Lagoon (IRL), Florida. From north to south, the stations are as follows: Cocoa (CO), Rockledge (RK), Eau Gallie (EG), Eau Gallie Harbor (EH), Crane Creek (CC), Turkey Creek (TC), Goat Creek (GC), South Valkaria (SV), and Saint Sebastian River (SS). Inset map shows IRL along east central coast of Florida.
Two stations were sampled in each of eight areas of the lagoon, plus one station in South Valkaria, for a total of 17 stations (Figure 1). Station locations identified with a P (e.g., SS-P) were on the perimeter of an organic-rich, muddy deposit where mixing with sand was more likely. Station locations identified with a C (e.g., SS-C) were in the center of muddy deposits that were especially rich in OM and H2S. Therefore, two nearby stations with significantly different sediment textures and compositions were sampled in each area. These two stations had a high likelihood of being colonized by the same species; however, sediment composition for the two stations was typically very different.
Field Sampling
This study was conducted from November 2015 to July 2016. All 17 stations were sampled twice, once when water temperatures averaged 19 ± 3°C (dry season) and once at 29 ± 2°C (wet season). Each pair of stations (e.g., CO-P and CO-C) was sampled on the same day during each sampling period. Continuous water column profiles for temperature, DO, % DO saturation (DOsat), salinity and pH were obtained using a Yellow Springs Instrument 6600 V2.0 Sonde. The sonde was calibrated prior to each day-long sampling trip following manufacturer’s specifications.
Water depths and thicknesses of muddy sediments overlaying sand layers were determined at each station using a marked and capped 4.2-cm diameter polyvinyl chloride pole. The pole was inserted vertically into the water until it touched the surface of the sediment; that depth was recorded to the nearest 0.1 m as the water column depth. Then, the pole was pushed into the muddy sediment until it reached hard bottom (usually sand), with the resulting total depth representing the depth of the water column plus the thickness of muddy sediment. Thicknesses of muddy sediments were determined by subtracting water column depth from total depth.
Triplicate sediment samples were collected at each station using an Ekman grab (15 cm × 15 cm × 19 cm). Sediment from each grab was sieved through a 500-μm, stainless steel mesh and organisms were placed in separate 700-mL containers with water from the sample site. A fourth grab was collected at each station for chemical analysis of the sediment. A clear acrylic tube (7 cm diameter, 24.5 cm long), with holes every 2 cm along its length for subsequent electrode insertion (plugged during deployment), was placed vertically into the center of the fourth grab, then removed and sealed for determination of DO, Eh, and pH. Four 10-mL syringes with the forward ends cut off (1.3 cm diameter, 8 cm long) were used to collect mini-cores from the chemistry grab (Fox and Trefry, 2018). These syringe samples were sealed immediately with parafilm, kept on ice and returned to the laboratory for determination of porewater concentrations of dissolved NH4+ and H2S. Sediment also was collected from the upper 5 cm of the chemistry grab and placed in double Ziploc bags. This sediment was used to determine water content, grain size, loss on ignition at 550°C (LOI), CaCO3, TN, TOC, and porosity. All samples were kept cold (∼4°C) until analyzed or freeze-dried.
Physical and Chemical Analysis of Sediments
Four mini-cores from each site were placed into N2-purged tubes and centrifuged for 7 min to obtain porewater. The supernatant (porewater) was then filtered through Whatman, 0.45-μm polypropylene, membrane filters. The filtered water was immediately analyzed for dissolved H2S by Hach Method #8131 with a precision of 10% relative standard deviation (RSD). Filtered water was stored at 4°C and purged with N2 gas before being analyzed for NH4+ to limit analytical interference from H2S. Concentrations of NH4+ were determined colorometrically using the standard phenate method #4500-NH3 (Clesceri et al., 1989) with an analytical precision of 3% (RSD) and 3% accuracy.
Concentrations of DO in sediments were determined using an OM-4 oxygen microelectrode. Two-point calibration was carried out using air-purged and N2-purged water samples prior to each use of the electrode. Dissolved oxygen concentrations were determined for surface water, the water-sediment interface, and then at 1-mm intervals into the sediment to a depth of 5 mm. Oxygen concentrations were determined prior to Eh and pH measurements to avoid disturbing or aerating the sediment. A depth of 5 mm was selected because DO concentrations were below the detection limit of 0.1% O2 at deeper than 1 mm in all sediments. Eh and pH were then determined every 2 cm in the 15-cm long core using a calibrated Orion 250A meter with Eh (platinum, ±5 mV) and pH (Ag-AgCl, ±0.05 pH units) electrodes. The probes were inserted into pre-cut holes in the tube after removing the tape attached prior to sampling.
Sediment samples from Ziploc bags were freeze-dried in 74-mL vials and water loss (by mass) was determined to calculate porosity (ϕ, the fractional volume of water). Freeze-dried sediments were homogenized and then analyzed using the LOI methods of Heiri et al. (2001) to determine OM after heating to 550°C and CaCO3 content after subsequent heating to 950°C. TOC and TN were determined using homogenized, freeze-dried sediments treated with 10% (v/v) hydrochloric acid to remove carbonate. Organic N was not lost during this process based on our laboratory testing. Analysis for TOC and TN was carried out using a Leco TruMac CNS/NS/Carbon/Nitrogen/Sulfur Analyzer following methods provided by the manufacturer (Version 1.3x, part number 200–753, August 2014). All values obtained for the certified reference material (Leco CRM 502-309) were within the 95% confidence interval (11.98 ± 0.44% TOC and 0.93 ± 0.04% TN). Laboratory precision was 6% for TOC and 2% for TN. Grain size was determined by the method of Folk (1974).
Analysis of Sediment Infauna
Samples of benthic organisms were stored at 4°C and sorted within 72 h of collection using an Olympus SZ Binocular Stereo Zoom Microscope with 7×–40× zoom magnification. Movement differentiated living vs. dead organisms within the 72-h window. Identifications were reported to the family level when genus and species could not be determined; all taxa were used to determine diversity. Abundances were reported as individuals per m2 to facilitate comparisons with other studies. High abundances of tolerant organisms make sediments seem enriched with life, especially in stressed environments; however, this is not necessarily representative of the overall health of a benthic community (Warwick and Clarke, 1995). Therefore, community diversity was determined using the Shannon-Wiener diversity index (H′, Eq. 1). Effective Number of Species (ENS, Eq. 2) was used to express the number of equally occurring individual species needed in a community to produce the observed diversity index; values were reported as number of species instead of an index (Dauby and Hardy, 2012). ENS has been studied extensively for use in ecology; it has advantages over other diversity indices including versatility and direct relationship to species composition (Jost, 2006; Chao et al., 2010). Other diversity indices can be easily transformed into ENS values as shown for H′ (Eq. 2).
Community diversity for each station was calculated by first determining the Shannon-Wiener (H′) diversity index value:
where S refers to the species richness and pi is the fraction of S of a single species (i) to the S of all species recorded. The ENS, also referred to as the Hill number (N1, Hill, 1973), was calculated as:
Number of species per grab was recorded as richness (S). When biological variables such as diversity were subjected to regression analysis with our environmental data, the exponential form, ENS, had the best fit (most R2 ≥0.5). Therefore, ENS values were used in this study as the main biological parameter for identifying relationships between environmental properties and benthic community composition.
Statistical Analysis
Values for geochemical properties of sediments (e.g., TOC) were range standardized within sampling areas by converting the values to a 0–1 scale using:
A resemblance matrix was created from the standardized values using Euclidean distance. Hierarchical cluster analysis was used to explore groups of samples, with similarity profile analysis (SIMPROF) indicating data that could be permuted without any sample moving into a different group (PRIMER 6 and PERMANOVA). Similarities percentage analysis (SIMPER) was used to determine which geochemical parameters contributed to differences among groups.
Uncommon taxa (i.e., fauna present in <5 samples), were culled from the dataset. Faunal abundances were range standardized within sampling areas using Eq. 3 to balance the influence of less and more abundant groups. A permutation analysis of variance (PERMANOVA) that treated the groups identified from geochemical characteristics as a fixed factor was performed on a resemblance matrix created with a Bray-Curtis similarity measure and an arbitrary value of 1 added to eliminate undefined distances. Significantly different groups (p < 0.05) were analyzed with SIMPER to determine the taxa that contributed to statistically significant differences. To further identify relationships between biological and environmental variables, Pearson’s correlation coefficients (r) were used to compare values (e.g., TOC vs. ENS) and tested for significance using a two-sample, two-tailed t-test. Correlations were identified as mild for 0.2 ≤ r < 0.4, moderate for 0.4 ≤ r < 0.6, moderately strong for 0.6 ≤ r < 0.8, strong for 0.8 ≤ r < 0.9, and very strong for ≥0.9.
Results
Sediment Physical and Chemical Variables
Concentrations of physical and chemical variables for the full suite of organic-rich sediments varied widely (Table 1). For example, silt + clay and sand content ranged from 6–99 to 1–84%, respectively. TOC and TN ranged from 1–8 to 0.1–0.7%, respectively. Therefore, environmental characteristics were compared using a hierarchical cluster analysis with SIMPROF. Four groups were identified as statistically different (Figure 2). Groups A and B were strictly center muddy deposits, whereas group C contained only perimeter muddy sand. Group D was the only mixed group with three perimeter deposits and one center deposit (Table 1); the anomalous center deposit contained 63.5% sand. SIMPER indicated that all environmental characteristics contributed to differences among groups and mean values for each parameter provided insight about differences in environmental conditions (Table 1).
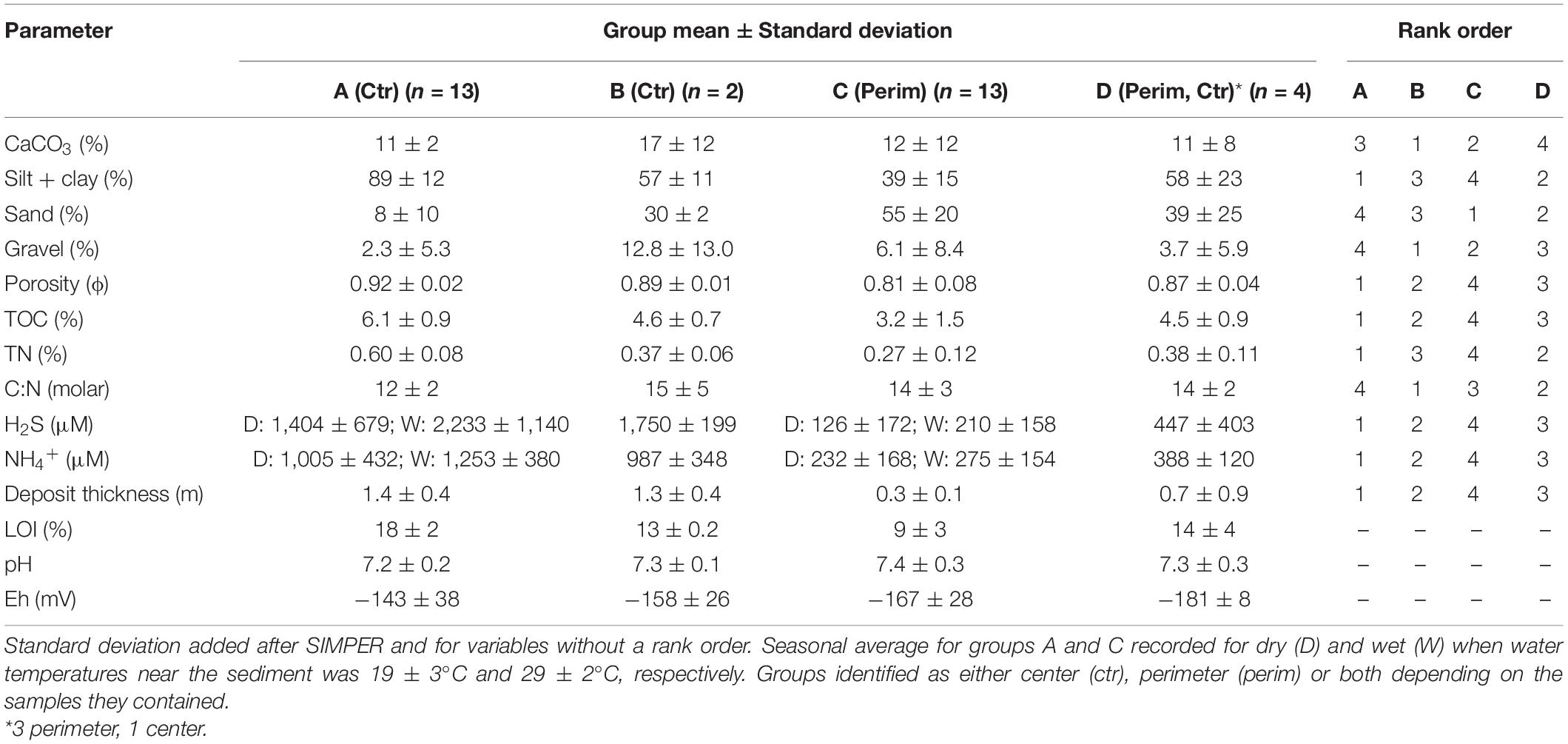
Table 1. Similarities percentage analysis of mean environmental characteristics of the four groups identified from hierarchical cluster analysis with the rank order from highest concentration to lowest for each parameter per group.
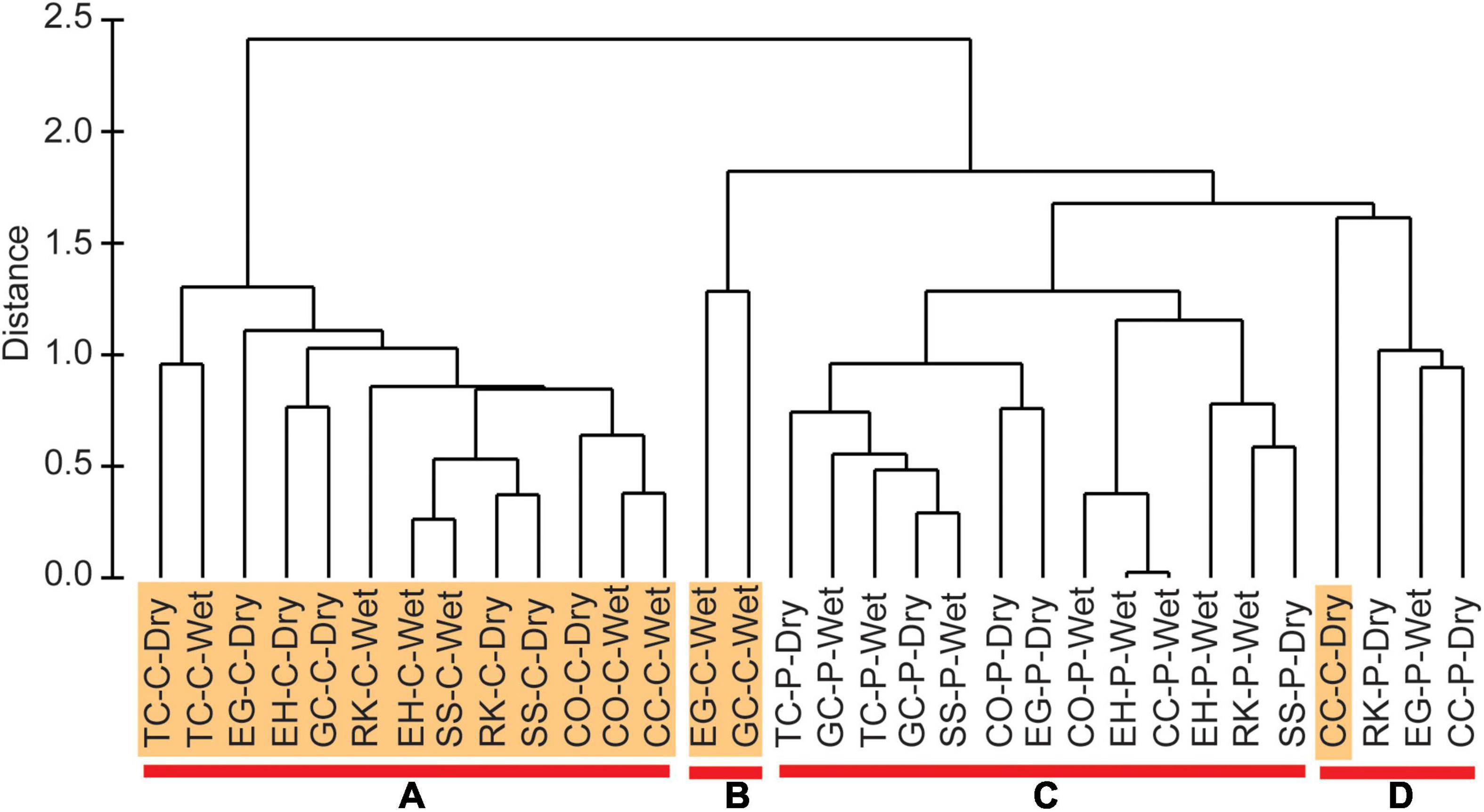
Figure 2. Hierarchical cluster analysis created from range-standardized environmental characteristics and Euclidean distance. Four groups identified as shown by samples above red bars (A–D). Samples from center (C) locations are highlighted, but not perimeter (P) samples. Sampling seasons represented by wet and dry.
Group A with the lowest sand content and highest concentrations of contaminants (i.e., TOC, TN, and H2S) represented sandy mud, center deposits. Group C showed the opposite trend and represented muddy sand, perimeter deposits (Table 1). Samples from center deposits (group A) contained an average of 2.3-fold higher silt + clay contents than perimeter sediments (group C, Table 1). Groups B and D had similar values for porosity, sand and silt + clay that were between values for groups A and C (Table 1).
All sediments were anoxic, even within the top 1 mm. Redox potential (Eh) in the top 8 cm ranged from −43 to −176 mV in more sandy perimeter sediments relative to −98 to −190 mV in muddier center sediments. Negative Eh values showed the range of reducing conditions and supported high porewater concentrations of reduced nitrogen and sulfur as ammonium (NH4+) and hydrogen sulfide (H2S), respectively (Table 1). Average concentrations of porewater NH4+ and H2S were an overall ∼5- to 12-fold higher in the muddy central area sediments than in sandier perimeter deposits (Table 1). Seasonality also had an impact on these concentrations due to increased microbial activity during warmer, wetter summer months. Concentrations of NH4+ and H2S for all sediment porewater averaged ∼20 and ∼60% higher, respectively, during the wet season than the dry season (Table 1). Porewater H2S values correlated strongly with NH4+ (Figure 3A). pH as [H+] was about 37% lower in the center samples due, in part, to higher concentrations of dissolved H2S and HS– (Table 1; Peltzer et al., 2016).
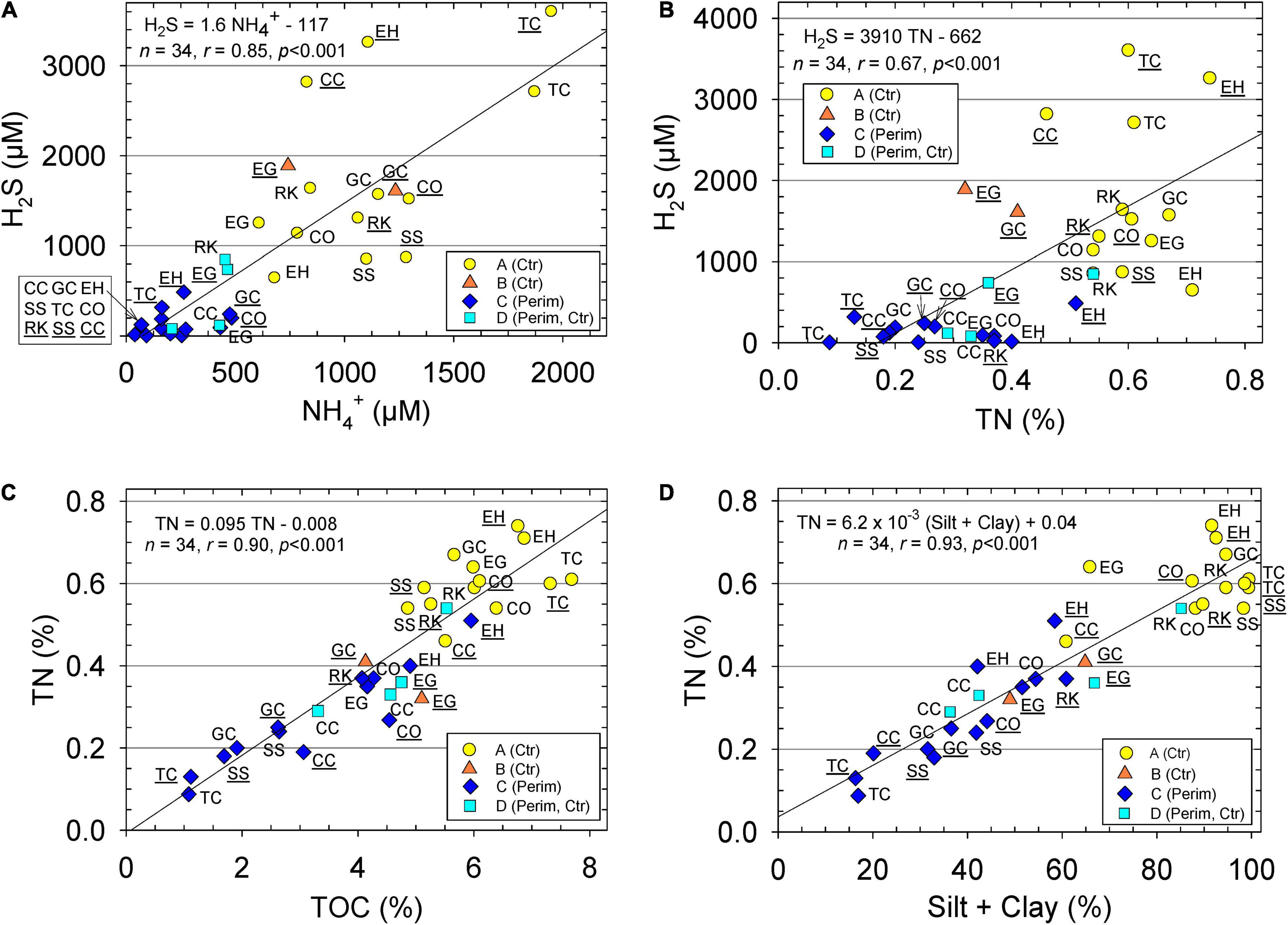
Figure 3. Sediment (A) porewater hydrogen sulfide (H2S) vs. ammonium (NH4+), (B) porewater dissolved H2S vs. sediment total nitrogen (TN), (C) concentrations of TN vs. total organic carbon (TOC) and (D) concentrations of sediment TN vs. silt + clay content. Data for perimeter (Perim) and center (Ctr) deposits of muddy sediment. Equations and lines from linear regression, correlation coefficient (r), number of samples (n), and p statistic. Stations with ID underlined were sampled during wet season and with no underline during dry season. Groups determined from hierarchical cluster analysis are identified with different markers.
Average values for TOC and TN in the top 5 cm of sediment from the center of muddy deposits were 1.9- and 2.2-fold greater, respectively, than for sediments from the sandier perimeter (Table 1). TOC values correlated very strongly with TN for all samples; the molar C/N ratio for all samples averaged 13.0 ± 2.6 and ratios were similar in the perimeter and center sediments (Figure 3C and Table 1). TN correlated positively with H2S and silt + clay with lowest values for perimeter stations (Figures 3B,D). Average CaCO3 content was not statistically different (p = 0.77) in perimeter vs. center locations because sizeable shell hash, when found, was present at both stations in the same area (Table 1).
Variation in Benthic Communities
The presence and abundance of key species varied considerably throughout our study area as well as between perimeter and center deposits. A total of 23,522 individuals and 35 species were collected from all organic-rich sediments (Table 2). The number of individuals ranged from 1 to 1,009 per grab with an average of 231 ± 285 per grab or 9,900 ± 12,300 per m2. The organisms were divided into bivalvia, gastropoda and polychaeta, plus a combined group (others) (Table 2). Bivalvia was the most abundant class followed by gastropoda, others and polychaeta. Ranges in abundances for all four groups were large (Table 3).
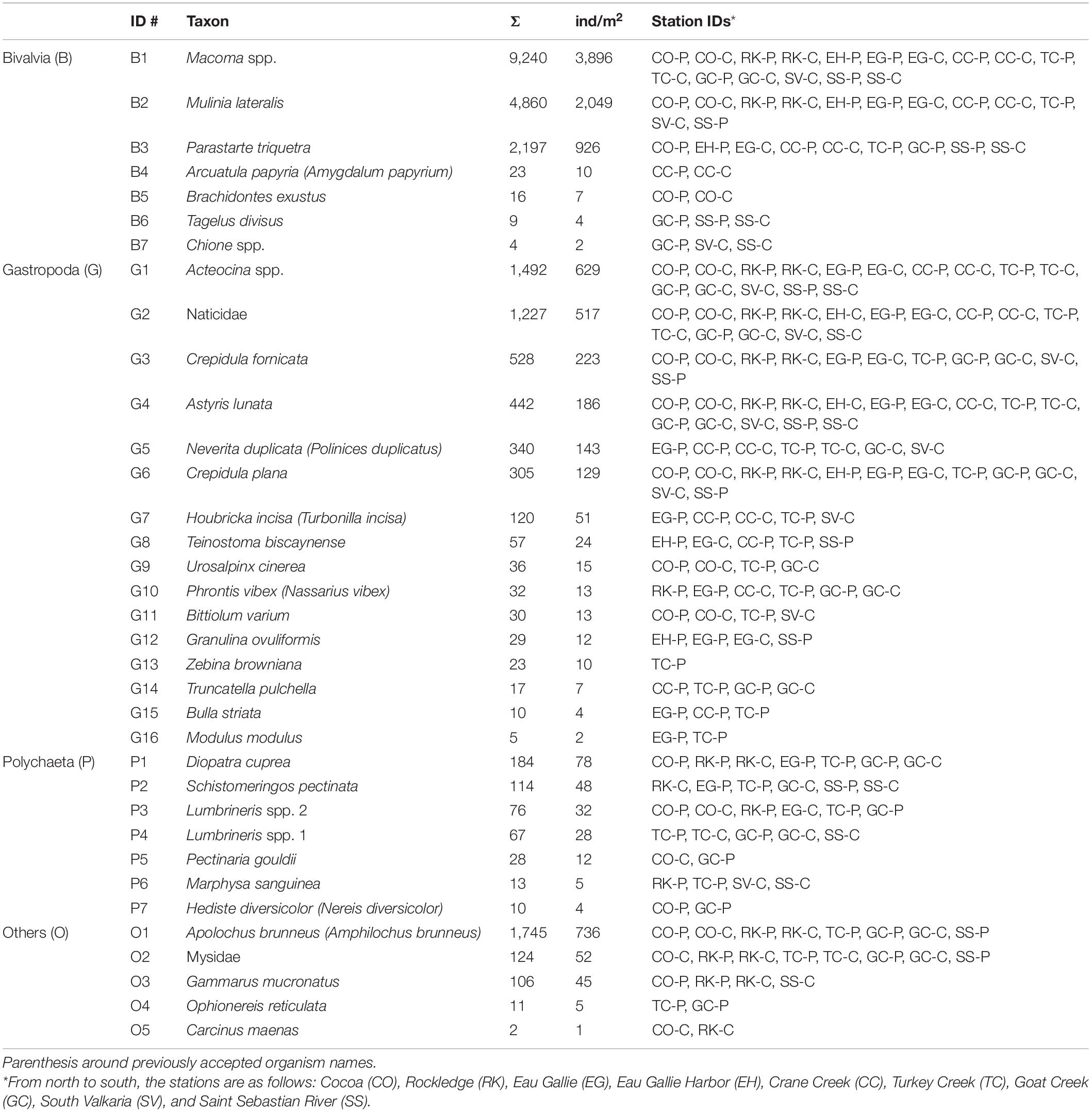
Table 2. Scientific names of organisms collected from Indian River Lagoon, Florida, with identification number (ID), total number of organisms (Σ) from 102 grabs (34 stations × 3 grabs/station × 0.023m2), average abundance per grab as individuals (ind) per m2 and station IDs where each organism was found (P = perimeter, C = center).

Table 3. Average means ± standard deviations and (ranges) for abundances of bivalves, gastropods, polychaetes and others as well as the effective number of species (ENS) for the four groups identified from hierarchical cluster analysis of sediment deposits sampled in triplicate from the Indian River Lagoon, Florida.
A PERMANOVA showed that faunal abundances varied significantly among the four different environmental groups (pseudo-F = 7.29, PERMANOVA P-value = 0.001), and follow-up PERMANOVAs indicated that all four groups were significantly different from each other (P-values < 0.05). SIMPER identified the main taxa comprising these four groups (Table 4). Group A, which is most representative of center deposits, yielded ∼70% Macoma spp. and ∼14% Apolochus brunneus; whereas perimeter deposits, best represented by group C, had a greater mixture of taxa (Table 4).
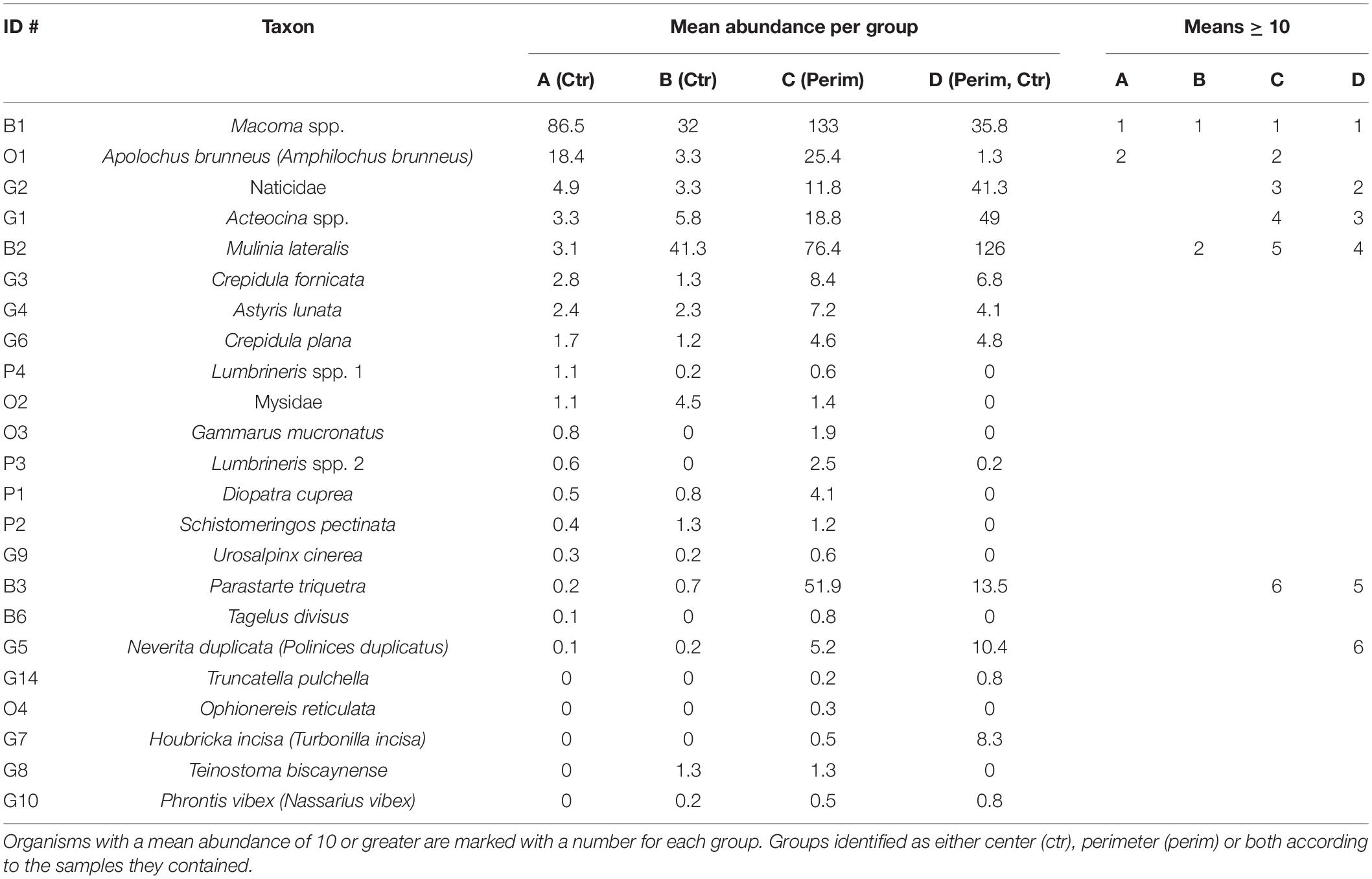
Table 4. Similarities percentage analysis of mean abundances (individuals per grab) for the four groups that were determined from hierarchical cluster analysis.
Just three species, Macoma spp., Mulinia lateralis and Parastarte triquetra, comprised >99% of the bivalves and 70% of all individuals collected (Table 2). These three species showed somewhat different distribution patterns for perimeter vs. center deposits (Table 4). Macoma spp. was ubiquitous (39% of all individuals) and collected from all but one station (Table 2). Abundances of Macoma were 1.5-fold greater in perimeter, sandier sediments (group C) than center, muddy deposits (Table 4, organism B1). The bivalve M. lateralis comprised 20% of all individuals collected and was found at 12 of the 17 stations (Table 2). However, 25-fold more M. lateralis individuals were collected from perimeter deposits and the largest mean concentration was found in group D sediments (Table 4, organism B2). The third most abundant bivalve, P. triquetra (9% of all individuals; Table 2), also was predominantly found in more sandy perimeter deposits from group C (Table 4, organism B3). The other four bivalve species made up <0.4% of all bivalves (Table 2). Collectively, bivalve abundances were ∼3 times greater in the perimeter than the center of mud deposits (Table 3). Bivalvia abundance for all groups also was about 3.5-fold higher than the average for gastropoda (Table 3).
Gastropoda (sea snails and slugs) made up 20% of total abundance and included Acteocina spp., Crepidula fornicata and a gastropod in the family Naticidae (Table 2). Gastropod abundances were ∼4 times higher in the sandier perimeter than muddy center deposits, with the greatest number of gastropods in group D (Table 3). Acteocina spp. was the most abundant gastropod in this study; ∼6-fold more of these organisms were collected from sandier perimeter locations of group C than center deposits of group A (Tables 2, 4, organism G1). A species of Naticidae was almost as abundant as Acteocina spp. and found in 12 of 17 IRL areas (Table 2, organism G2). The abundance of snails from the family Naticidae was 2.4 times higher in perimeters than centers of muddy deposits (Table 4).
Although polychaetes were the least abundant (2%) class of organisms, they were ∼3 times more abundant in perimeter than center deposits with the greatest abundance found in group C (Tables 2, 3). The most abundant polychaetes were Diopatra cuprea, Schistomeringos pectinate and two species of Lumbrineris (spp. 2, 32 individuals per m2; spp.1, 28 individuals per m2) (Table 2). The only polychaete that was more abundant in the central area of the muddy deposits was Lumbrineris spp. 1 (organism P4) with ∼60% of the individuals collected in muddy center deposits of group A (Tables 2, 4).
The others category was made up of four species of malacostracans and a brittle star (class Ophiuroidea). This mixed group made up 8% of all the organisms collected. A. brunneus, an amphipod and the most abundant organism in the others group, was collected at 8 of 17 stations (Table 2). A. brunneus also was the fourth most abundant organism in this study (Table 2, organism O1). About 52% of the A. brunneus were collected from perimeter deposits (group C) relative to 38% from center deposits (group A), with the remainder from groups B and D (Table 4).
Variation in Benthic Communities Related to Environmental Conditions
Pearson’s correlation coefficients were moderate to moderately strong between metrics for biota and environmental data (Table 5). Negative correlations for ENS vs. TOC, TN, LOI, H2S, porosity and silt + clay were determined (Table 5). Thus, higher values for each of these six environmental covariates corresponded with less habitable sediments and lower ENS values. In contrast, positive correlations were obtained for ENS vs. sand content (Table 5). Gastropoda and polychaeta had the most significant correlations with environmental parameters, including a significant positive correlation with % sand. The bivalvia and others groups correlated significantly and positively only with CaCO3 and bottom water temperature, respectively (Table 5).
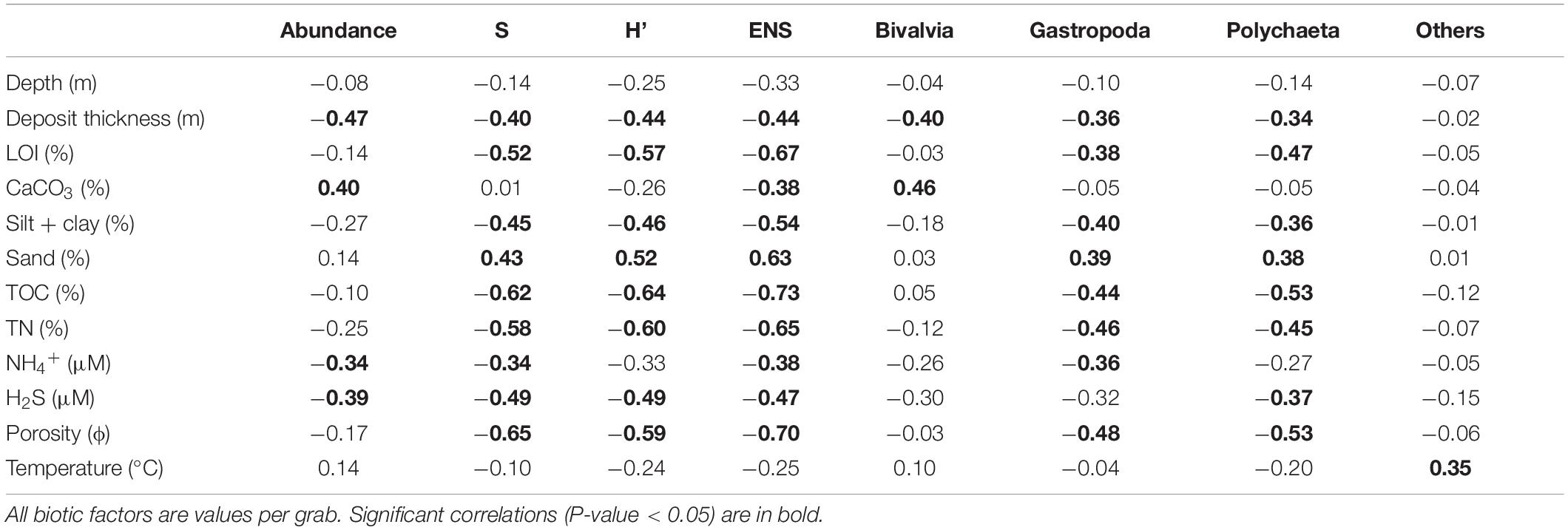
Table 5. Pearson correlation coefficients (r) for significance between biotic and sediment factors for core/grab data from 34 samples collected at 17 locations in the Indian River Lagoon; abundances of organism groups, richness (S), Shannon-Wiener diversity index (H′), and effective number of species (ENS).
We also investigated the significance of individual environmental variables in controlling ENS using correlation plots (Figure 4). The greatest difference for species abundance and diversity was for perimeter sediments from station TC vs. the center deposit at station EH (Figure 4). Station TC-P, with an ENS value of 7–9, had <0.2% TN, <1.5% TOC, and >80% sand. In contrast, station EH-C with an ENS of 1, had >0.7% TN, >6% TOC, and <10% sand (Figures 4A,B,E). Overall, higher ENS values were found where there was lower porosity and more sand (Figures 4C,E). Sediments from the IRL with the highest ENS had a porosity of 0.65–0.75 and 65–89% sand (Figures 4C,E). Sediment samples with >6% TOC, a porosity >0.9, and <30% sand, had ENS values <4 (Figures 4B,C,E).
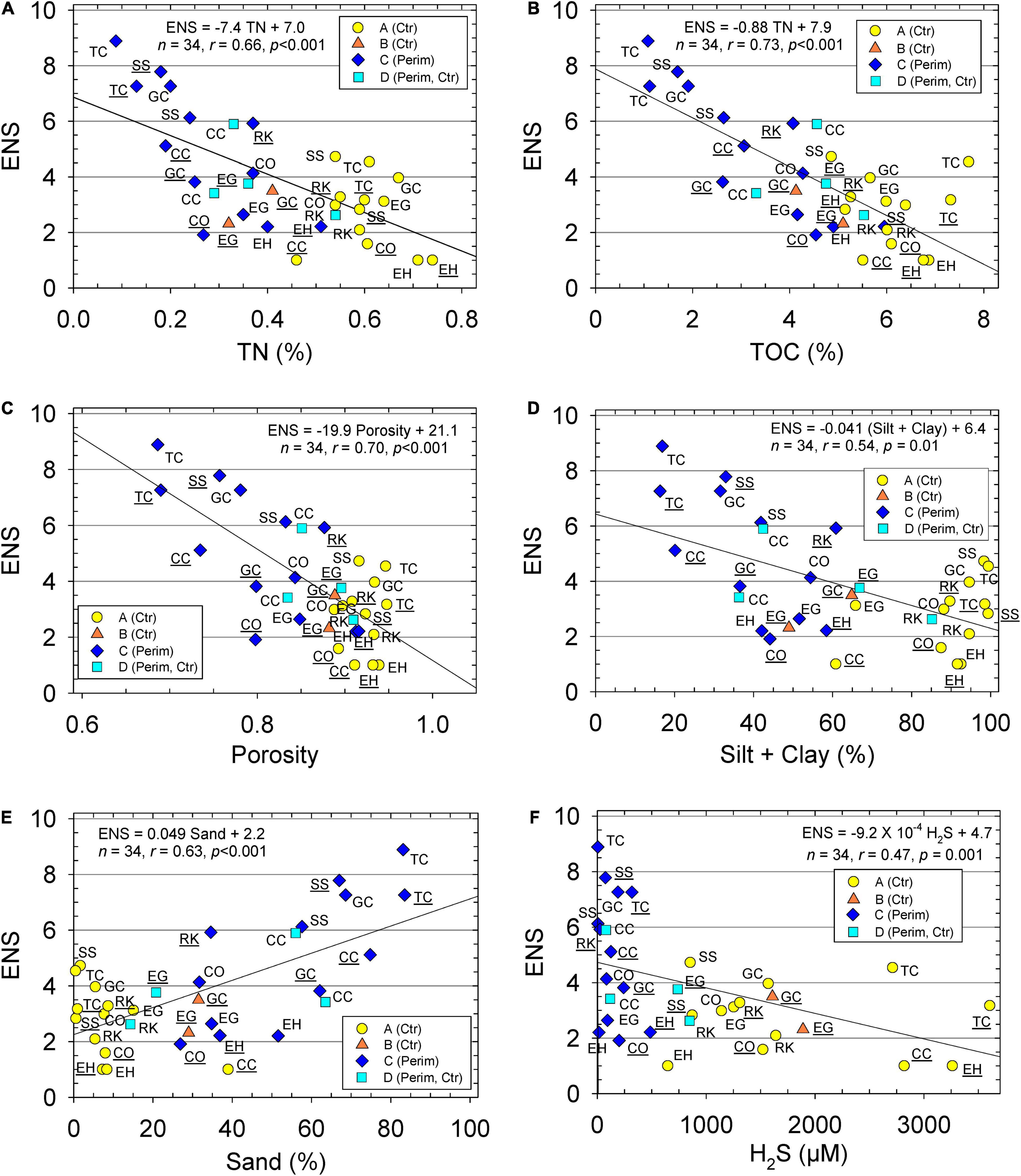
Figure 4. Effective number of species (ENS) vs. (A) sediment total nitrogen (TN), (B) sediment total organic carbon (TOC), (C) porosity, (D) silt + clay, (E) sand, and (F) porewater hydrogen sulfide (H2S). Data for perimeter (Perim) and center (Ctr) deposits of muddy sediment. Equations and lines from linear regression, correlation coefficient (r), number of samples (n), and p statistic. Stations with ID underlined were sampled during wet season and with no underline for dry season. Groups determined from hierarchical cluster analysis are identified with different markers.
As in the P-R model (Pearson and Rosenberg, 1978), data for abundance were plotted as a function of TOC (Figure 5). Lower abundances of polychaeta and gastropoda were found with increasing TOC (Figure 5A). This trend was not observed for the bivalves that had highest abundance at a TOC of 4–7% (Figure 5A). When ENS was plotted vs. TOC ranges, a clear trend of increasing ENS with decreasing TOC was found (Figure 5B). H2S increased with increasing TOC (Figure 5B).
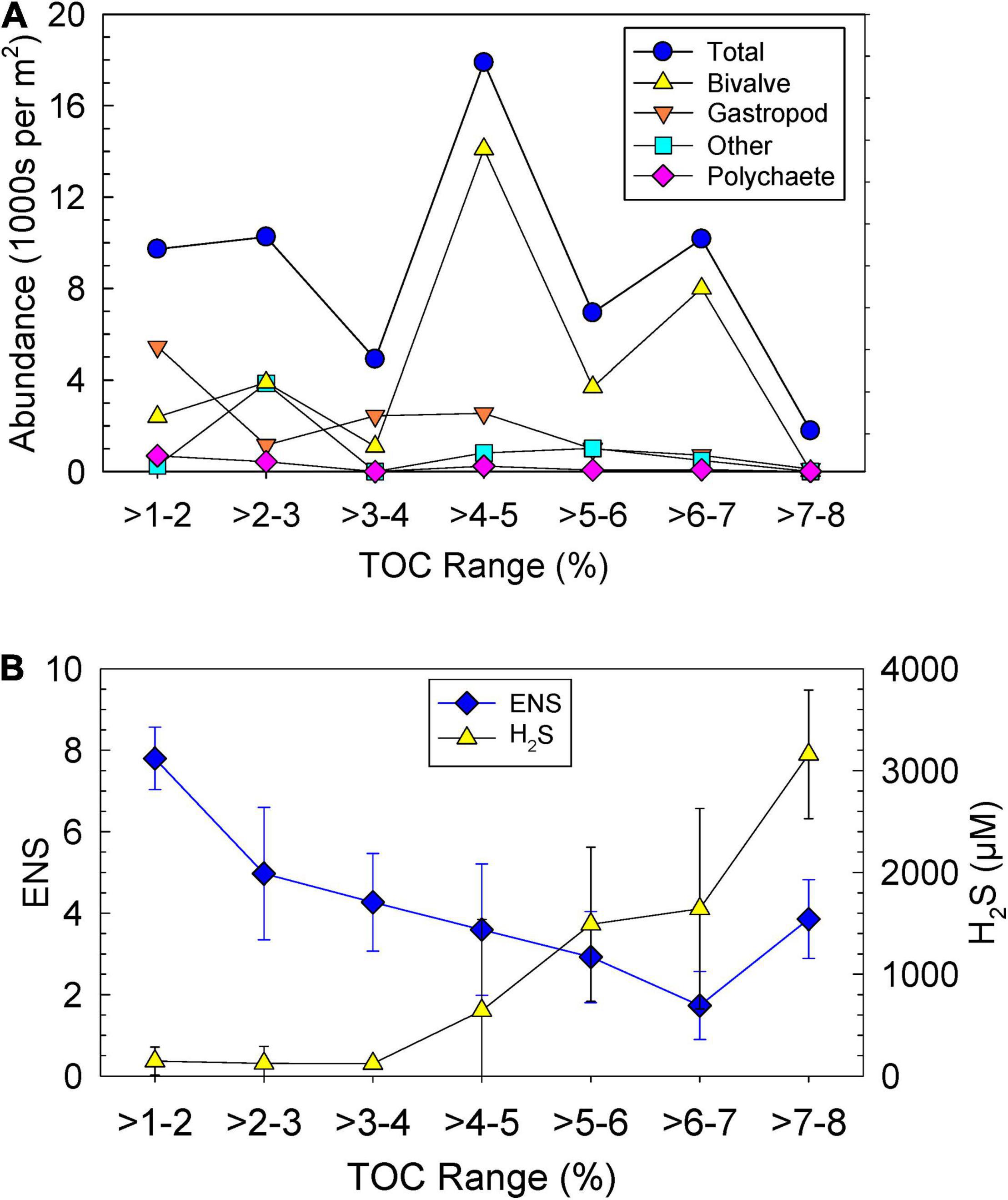
Figure 5. Ranges in sediment total organic carbon (TOC) vs. (A) average abundances of four classes of taxa per m2 and (B) average effective number of species (ENS) and porewater hydrogen sulfide (H2S) with standard deviations.
Discussion
Adaptations Support Opportunistic Organisms in Muddy Indian River Lagoon Sediments
Increased loading of sediment OM can stress benthic infaunal communities (Cloern, 2001). This stress leads to shifts in community structure with opportunists becoming the predominant species amidst the loss of other organisms (Norkko et al., 2006). We can use our data plus global examples, to help improve our understanding of these shifts and identify opportunistic organisms that are adapted to fill the niche of organic-rich sediments.
Similarities percentage analysis showed that Macoma best characterized benthic communities in the areas sampled (Table 4). Macoma spp. was collected from all stations except EH-C, one of the least favorable habitats due to high TOC and TN (Table 2 and Figure 3C). Macoma are well suited for muddy and even anoxic environments due to their long siphons that can extend above the sediment surface (Seitz et al., 2001). Macoma have been reported to live in organic-rich effluent from a wastewater treatment plant (Hummel et al., 2000). Furthermore, Korpanty and Kelley (2014) noted that Macoma were better known as opportunists and capable of living among death assemblages, much like we found in shell hash layers. Therefore, their survival in organic-rich, muddy sediment in the IRL, was consistent with other studies.
Like Macoma spp., M. lateralis also is well adapted and commonly found in high porosity, anoxic, and sulfidic sediments because they can function (feed, digest, and grow) under such conditions with the specialized adaptation of anaerobic glycolysis to produce energy (Shumway et al., 1983). Their average abundance of 2,049 individuals per m2 from our IRL study (Table 2) was much lower than the 74,000 individuals per m2 reported for Hillsborough Bay, Florida, in the mid-1970s (Santos and Simon, 1980). Conditions in Hillsborough Bay, only 10% silt + clay and higher DO in bottom water, created more favorable environments than muddy deposits in the IRL (Santos and Simon, 1980). Unlike Macoma and M. lateralis that survived under poor conditions, P. triquetra have been found in sand or around seagrass beds in high densities (Virnstein et al., 1983). P. triquetra recolonized at a density of 6,600 individuals per m2 in sediments with 90% sand in the adjacent Banana River Lagoon; however, their abundance decreased with decreasing DO and they were not considered opportunists (Grizzle, 1984).
Virnstein et al. (1983) found gastropods at greater densities in seagrass beds in the IRL than on bare, sandy substrate. The more robust gastropod communities in the TC area potentially survived due to proximity to a healthier benthic habitat where seagrass beds were present. TC is the only area that had no single dominant species contributing to the benthic community, but instead a more evenly distributed group of organisms indicating a greater diversity (Supplementary Table 1). Although gastropods made up only 20% of total abundance, they accounted for 46% of all species collected in this study (Table 2). Two of the most abundant gastropods, Acteocina and a snail from the family Naticidae, were much more abundant in sandier perimeter deposits in the IRL, as previously noted by Virnstein and Howard (1987; Table 4). Acteocina spp. have been reported in other lagoons and on mudflats such as the Bolsa Chica Lagoon (California), where ∼4,000 individuals per m2 inhabited sediment with 3–83% sand and 3–12% OM, a habitat more like perimeter deposits in the IRL (Levin et al., 1998; Table 1 where LOI = OM). Much like Acteocina spp., the naticid also has been found in sandy, vegetated areas; however, because of its large foot, it is well suited to traverse soft sediments (Cernohorsky, 1971).
Polychaetes have been used as indicator species for environmental stress in benthic communities because they have a shorter life span and lower tolerance for contaminants (Dean, 2008). Low abundances for polychaetes in our study may highlight the stressful character of anoxic, muddy sediments in the IRL. However, D. cuprea (organism P1), our most abundant polychaete, has adapted by building tubes and completely replacing them within days, making them less likely to become smothered in soft sediments and die (Thomsen and McGlathery, 2005). Mangum et al. (1968) concluded that D. cuprea have an exceptionally low oxygen consumption and their abundance was not found to correlate with grain size. Fast tube building, the ability to survive in fine and course sediments and the capacity to cope with low oxygen concentrations make D. cuprea well suited for anoxic mud.
Unlike D. cuprea, Pectinaria gouldii create a cone-shaped tube from sand grains over a life cycle, not days, and their abundance is dependent on sediment type (Busch and Loveland, 1975). Therefore, they may become more easily buried or have lower preference for settlement in the muddy deposits found in the IRL than D. cuprea (P1). Less sand and higher water content in muddy sediments decreases sediment bulk density and organisms more easily sink into the organic-rich sediments and die. Hinchey et al. (2006) reported that when organisms sink and become buried by sediment beyond a manageable depth, respiration via the oxic water layer above the sediment was inhibited. The only polychaete in our study to be found more commonly in the sandy mud of center deposits was a Lumbrineris spp. Some species of Lumbrineris have been collected in the eastern Mediterranean Sea where they were found surviving in sandy mud habitats like our center deposits (Carrera-Parra et al., 2011).
More amphipods were collected during the wet season, most likely due to seasonal peaks of benthic amphipods as they have an inverse relationship to macrophyte biomass, which declines in hotter, wetter months (Stoner, 1980). A. brunneus, our most abundant amphipod, is typically found in sandy sediments and is exclusively a substrate deposit feeder (Conradi and López-González, 1999; de-la-Ossa-Carretero et al., 2012). However, A. brunneus (organism O1) were roughly equally found in perimeter and center deposits (Table 4). This lesser degree of selectivity may result from an overall greater mobility for amphipods than most organisms in the study; likewise, they could escape anoxic sediments after feeding.
Physical and Chemical Controls on Abundance of Benthic Infauna
The P-R model was formulated to describe benthic community responses to environmental stressors, such as increased OM or contaminants (Pearson and Rosenberg, 1978). This approach can monitor the overall health of an ecosystem because the benthic community is consistently present and can be studied temporally and spatially. Areas experiencing eutrophication also experience hypoxia and increased NH4+ and H2S; therefore, these chemical components have more recently been added to the P-R model (Thompson and Lowe, 2004; Hyland et al., 2005).
All 34 IRL samples had NH4+ values that were less than the acute toxicity level of 1,950 μM (United States Environmental Protection Agency [USEPA], 1989). However, NH4+ concentrations were greater than the USEPA chronic level of 250 μM in 26 of the 34 samples (Figure 3A). The 8 samples with less than chronic values were collected from sandier perimeter sediments. Seventy percent of the 34 sediment samples had H2S values greater than the 4-day tolerance of 176 μM for Macoma (Figure 3A; Caldwell, 1975). Only bivalves and gastropods were found at stations with high concentrations of porewater H2S and low ENS.
Pearson and Rosenberg (1978) also distinguished infauna as opportunists when only a few species contributed to overall abundances in a polluted environment. Only two taxa had a mean abundance >10 in the center deposits in group A with increased contamination (Table 4). In contrast, six taxa from group C had abundances >10. Group A also had the lowest mean sand content at ∼8% and highest TOC (∼6%) and TN (0.6%) relative to ∼55% sand, ∼3% TOC and 0.27% TN in group C, the main group of perimeter samples (Table 1 and Figure 4). One extensive study of TOC vs. species abundance and diversity across 7 coastal regions and over 900 stations around the world typically found higher species richness when sediment TOC values were ∼1% (Hyland et al., 2005). Conversely, lower species richness was found more frequently when sediment TOC values were >3.5% (Hyland et al., 2005). This observation by Hyland et al. (2005) is consistent with our observations that sediments with TOC ≥5.0% had ENS ≤5; whereas sediments with <2% TOC had ENS values >7, with richness as high as 24 species (Figure 4B). Burd et al. (2008) also found species richness declined as sediment TOC and TN increased. Elevated concentrations of TOC and TN yield an anoxic, degraded habitat that is more reducing with elevated concentrations of H2S (Figure 3B). Biota from low ENS sediments were either 100% bivalves or gastropods, but not both. Even at our most prolific site (TC-P-dry), ENS values were ∼7 times lower than found by Mikkelsen et al. (1995) from a previous study of sandy sediments in the IRL.
Hierarchical clustering, along with the SIMPROF, and SIMPER analyses, show how the environmental parameters vary across the groups and in concert with each other (Figure 2 and Table 1). Correlation plots for ENS vs. environmental parameters help validate groupings from the omnibus statistical tests (Figure 4). When samples were grouped by environmental characteristics, the results supported distinct separation of sediments from center and perimeter locations (Figure 2 and Table 1). Groups B and D were similar in geomorphic data; however, there was a large difference in mean concentrations of H2S and NH4+. This difference was observed because group B selected exclusively wet season samples, when temperatures of bottom water were ∼10°C warmer and increased microbial activity increased concentrations of H2S and NH4+ in porewater from organic-rich sediments (Fox and Trefry, 2018). Group D selected samples collected during the cooler, dry season, and had a slightly greater sand concentration, consistent with lower porewater concentrations of H2S and NH4+ compared to group B.
Lower pH in center sediments reflected higher concentrations of dissolved CO2 and H2S. Even though concentrations of H2S varied significantly between warm (wet) and cool (dry) seasons due to differences in temperature of bottom water, no significant differences were found for abundance, richness, diversity and ENS between the two sampling seasons (Table 5). Therefore, a 10°C difference in temperature had no discernible effect on abundance, richness, diversity or ENS at any given station. Overall, ENS was a valuable parameter for showing organismic responses to environmental variables and determining a hierarchy of habitable organic-rich sediments.
Groups C and D, mostly with samples from perimeter deposits, were more representative of transitional organic-rich sediments where more taxa contribute to total abundance (Table 4; Borja et al., 2000; Norkko et al., 2006). When abundance was compared with TOC, the bivalves follow a trend of a tolerant taxa as defined by the enhanced P-R model of Thompson and Lowe (2004); bivalve abundance was high while abundances of other taxa decreased (Figure 5A). In this study, the tolerant organism was Macoma spp. due to its high average abundance of ∼86 individuals per grab in group A with an average TOC of ∼6% (Tables 1, 4). This result was predicted by the enhanced P-R model of Hyland et al. (2005) which showed that increased OM had a negative impact on the overall health of benthic faunal communities. Sediments with more OM are well known to experience increased bacterial decomposition, decreased bottom water DO, and increased concentrations of H2S, making these environments much less habitable for benthic life and more favorable for opportunistic, adapted species (Hansen et al., 2000; Schulz et al., 2000; Schultz and Urban, 2008). These data show that even at low OM content, proximity to muddy sediments can lead to decreased diversity due to potential for mud to mix with sand.
Applications to Lagoon Management
Fine-grained, organic-rich sediments have been accumulating in the IRL since the early 1950s, a consequence of increased human activity (Trefry et al., 1990; Trefry and Trocine, 2011; Fox and Trefry, 2018). These sediments help deplete oxygen in surrounding water, create less habitable benthic environments, store pollutants, and release N and P to the overlying water (Trefry et al., 1990). Although the total area of the lagoon covered with muddy deposits is estimated to be <10%, benthic fluxes of NH4+ and phosphate to the overlying water column from these muddy sediments yield ≥25% of total fluxes of N and P to the IRL (Fox and Trefry, 2018; Tetra Tech Inc and Closewaters LLC, 2021). Dredging has been the primary form of remediation for muddy sediments in the IRL since the late 1990s. Such activity has focused on sediment-filled creeks and harbors to limit sediment transfer to the open lagoon. We believe that results from our study of species abundance and diversity in high-porosity, organic-rich sediments fill an information gap and thereby promote enhanced discussion of alternate forms of sediment remediation.
Our collection of organic-rich sediments from perimeters and centers of muddy deposits along 60 km of IRL provided a representative selection available infauna from sediments with a large range in concentrations of TOC, TN and porewater H2S. Most sediments we sampled had H2S concentrations that exceeded the LC50 values for many infauna. Total abundance was ∼3-fold greater for more sandy perimeter deposits than for more muddy center areas. Richness from sandy sediments adjacent to mud were at least ∼7-fold lower than previously reported for sandy areas in the open IRL (Mikkelsen et al., 1995). Observed higher abundance, yet low diversity from this study, was due to greater abundances of a few bivalves, including the clam Macoma. ENS values from our IRL study ranged from 1 to 9 (i.e., H′ of 0–2.2). Species surviving in these deposits were adapted to survive extreme conditions, most likely by using a siphon as reported for Macoma species and other clams found in the muddy deposits (Hummel et al., 2000; Seitz et al., 2001). Negative correlations were obtained for ENS vs. TOC, TN, porosity, and porewater H2S, whereas a positive correlation was obtained for ENS vs. % sand. Therefore, ENS could be predicted from correlation plots of ENS vs. various environmental variables. Center areas of muddy deposits had the lowest ENS values (<3); ENS increased to ∼9 with decreasing concentrations of TOC, TN, and H2S, plus increasing sand content. These data for environmental and biological variables in muddy IRL sediments can be used to help predict the overall health of the benthic biota and determine where sediment treatment/remediation is needed to improve the overall health of the ecosystem. Post-treatment data also can help assess the success of remediation.
Dredging and capping contaminated sediments have been primary forms of sediment remediation globally for many years (National Research Council, 1989). Leaving contaminated sediments in place is generally more economically viable and often preferred due to concerns for contamination during removal or issues at containment and disposal sites on land. Sediment contaminants in IRL mud are primarily N and P that support large fluxes of dissolved ammonium and phosphate to the overlying water (Fox and Trefry, 2018). From 1998 to 2009, ∼2 million m3 of fine-grained, organic-rich sediment, along with some underlying sand, were dredged from Crane Creek, Turkey Creek and the St. Sebastian River. From 2016 to 2020, ∼600,000 m3 of sediment were dredged from Turkey Creek, the Eau Gallie River and some smaller areas (Fox and Trefry, 2018). All these locations were adjacent to or upstream of the IRL. Removal costs and recovery of dredged spoils have prompted discussion of alternative, in situ treatment of muddy sediment in the IRL. Capping of anoxic, fine-grained, organic-rich sediment with sand in Lake Worth Lagoon (LWL), Florida, has shown positive results (United States Army Corps of Engineers, 2018). Most sites where capping was carried out in LWL were close to shore or in dead-end passages. However, sand capping has been, thus far, deemed successful in LWL.
Sand is clearly a key variable that helps increase ENS in IRL sediments because it promotes decreased H2S in porewater through lower TOC and TN (Figure 4). We now know that mixing of sand with anoxic, fine-grained, organic-rich sediments leads to a significant increase in ENS (Figure 4E). We believe the process of testing sand capping in open water areas should proceed carefully in barrier island lagoons with the best engineering designs and high quality, remote monitoring to track performance over time. Integration of sand capping, coupled with monitoring the boundary between sand and organic-rich sediments, would reduce nutrient loading to the lagoon system and improve water quality and benthic habitat.
Data Availability Statement
The original contributions presented in the study are included in the article/Supplementary Material, further inquiries can be directed to the corresponding author.
Author Contributions
KF and AF: planning, sampling, analysis, data interpretation, and manuscript preparation. JT: planning, sampling, data interpretation, manuscript preparation, and funding. CJ: analysis, data interpretation, and manuscript preparation. All authors contributed to the article and approved the submitted version.
Funding
Funds for this investigation were provided by the St. Johns River Water Management District as part of Contract No. 27815 and the Florida Legislature as part of Department of Environmental Protection Grant Agreement No. 50714-Brevard County Muck Dredging.
Conflict of Interest
The authors declare that the research was conducted in the absence of any commercial or financial relationships that could be construed as a potential conflict of interest.
Publisher’s Note
All claims expressed in this article are solely those of the authors and do not necessarily represent those of their affiliated organizations, or those of the publisher, the editors and the reviewers. Any product that may be evaluated in this article, or claim that may be made by its manufacturer, is not guaranteed or endorsed by the publisher.
Acknowledgments
We greatly appreciate the continued help and support of our laboratory personnel, both past and present, including Bob Trocine, Stacey Fox, and Jessica Voelker. We thank Virginia Barker, Mike McGarry, and Matt Culver (Brevard County Natural Resources Management Department) for their advice and support as well as Glenn Beckett for his assistance and use of his boat for conducting this research. Very helpful reviews from our two reviewers were greatly appreciated.
Supplementary Material
The Supplementary Material for this article can be found online at: https://www.frontiersin.org/articles/10.3389/fmars.2021.768083/full#supplementary-material
References
Alongi, D. M. (1989). Ecology of tropical soft-bottom benthos: a review with emphasis on emerging concepts. Rev. Biol. Trop. 37, 85–100.
Andersen, J. H., Schlüter, L., and Ærtebjerg, G. (2006). Coastal eutrophication: recent developments in definitions and implications for monitoring strategies. J. Plankton Res. 28, 621–628. doi: 10.1093/plankt/fbl001
Borja, A., Franco, J., and Pérez, V. (2000). A marine biotic index to establish the ecological quality of soft-bottom benthos within European estuarine and coastal environments. Mar. Pollut. Bull. 40, 1100–1114. doi: 10.1016/S0025-326X(00)00061-8
Burd, B. J., Macdonald, R. W., Johannessen, S. C., and Van Roodselaar, A. (2008). Responses of subtidal benthos of the Strait of Georgia, British Columbia, Canada to ambient sediment conditions and natural and anthropogenic depositions. Mar. Environ. Res. 66, S62–S79. doi: 10.1016/j.marenvres.2008.08.009
Busch, D. A., and Loveland, R. E. (1975). Tube-worm-sediment relationships in populations of Pectinaria gouldii (Polychaeta: Pectinariidae) from Barnegat Bay, New Jersey, USA. Mar. Biol. 33, 255–264. doi: 10.1007/BF00390930
Caldwell, R. S. (1975). Hydrogen Sulfide Effects on Selected Larval and Adult Marine Invertebrates. Corvallis, OR: Oregon State University. WRRI–31.
Carrera-Parra, L. F., Çinar, M. E., and Dagli, E. (2011). Description of a new species of Lumbrineris (Polychaeta: Lumbrineridae) from the coasts of Turkey (eastern Mediterranean). Mar. Biodiv. 41, 343–347. doi: 10.1007/s12526-010-0074-8
Cernohorsky, W. O. (1971). The family Naticidae (Mollusca: Gastropoda) in the Fiji Islands. Rec. Auckland Instit. Museum 8, 169–208.
Chao, A., Chiu, C. H., and Jost, L. (2010). Phylogenetic diversity measures based on Hill numbers. Philos. Trans. R. Soc. Lond. B Biol. Sci. 365, 3599–3609. doi: 10.1098/rstb.2010.0272
Chou, L. M., Yu, J. Y., and Loh, T. L. (2004). Impacts of sedimentation on soft-bottom benthic communities in the southern islands of Singapore. Hydrobiologia 515, 91–106. doi: 10.1023/B:HYDR.0000027321.23230.2f
Clesceri, S. L., Greenberg, E. A., and Trussel, R. R. (1989). Standard Methods for the Examination of Water and Wastewater. Washington, DC: American Public Health Association.
Cloern, J. E. (2001). Our evolving conceptual model of the coastal eutrophication problem. Mar. Ecol. Prog. Ser. 210, 223–253. doi: 10.3354/meps210223
Conradi, M., and López-González, P. J. (1999). The benthic Gammaridea (Crustacea, Amphipoda) fauna of Algeciras Bay (Strait of Gibraltar): distributional ecology and some biogeographical considerations. Helgol. Mar. Res. 53, 2–8. doi: 10.1007/PL00012134
Cronin, T. M., and Vann, C. D. (2003). The sedimentary record of climatic and anthropogenic influence on the Patuxent estuary and Chesapeake Bay ecosystems. Estuaries 26, 196–209. doi: 10.1007/BF02695962
Dauby, G., and Hardy, O. J. (2012). Sampled-based estimation of diversity sensu stricto by transforming Hurlbert diversities into effective number of species. Ecography 35, 661–672. doi: 10.1111/j.1600-0587.2011.06860.x
Dauer, D. M., Ranasinghe, J. A., and Weisberg, S. B. (2000). Relationships between benthic community condition, water quality, sediment quality, nutrient loads, and land use patterns in Chesapeake Bay. Estuaries 23, 80–96. doi: 10.2307/1353227
Dean, H. K. (2008). The use of polychaetes (Annelida) as indicator species of marine pollution: a review. Rev. Biol. Trop. 56, 11–38.
de-la-Ossa-Carretero, J. A., Del-Pilar-Ruso, Y., Giménez-Casalduero, F., Sánchez-Lizaso, J. L., and Dauvin, J. C. (2012). Sensitivity of amphipods to sewage pollution. Estuar. Coast. Shelf Sci. 96, 129–138. doi: 10.1016/j.ecss.2011.10.020
Folk, R. L. (1974). Petrology of Ssedimentary Rocks. Austin, TX: Hemphill Publishing Company, 33–39.
Fox, A. L., and Trefry, J. H. (2018). Environmental dredging to remove fine-grained, organic-rich sediments and reduce inputs of nitrogen and phosphorus to a subtropical estuary. Mar. Tech. Soc. J. 52, 42–57. doi: 10.4031/mtsj.52.4.3
Grizzle, R. E. (1984). Pollution indicator species of macrobenthos in a coastal lagoon. Mar. Ecol. Prog. Ser. 18, 191–200. doi: 10.3354/meps018191
Gu, D., Iricanin, N., and Trefry, J. H. (1987). The geochemistry of interstitial water for a sediment core from the Indian River Lagoon, Florida. Florida Sci. 50, 99–110.
Hale, S. S., Cicchetti, G., and Deacutis, C. F. (2016). Eutrophication and hypoxia diminish ecosystem functions of benthic communities in a New England estuary. Front. Mar. Sci. 3:249. doi: 10.3389/fmars.2016.00249
Hansen, J. W., Thamdrup, B., and Jørgensen, B. B. (2000). Anoxic incubation of sediment in gas-tight plastic bags: a method for biogeochemical process studies. Mar. Ecol. Prog. Ser. 208, 273–282. doi: 10.3354/meps208273
Heiri, O., Lotter, A. F., and Lemcke, G. (2001). Loss on ignition as a method for estimating organic and carbonate content in sediments: reproducibility and comparability of results. J. Paleolimnol. 25, 101–110. doi: 10.1023/A:1008119611481
Hill, M. (1973). Diversity and evenness: a unifying notation and its consequences. Ecology 54, 427–432.
Hinchey, E. K., Schaffner, L. C., Hoar, C. C., Vogt, B. W., and Batte, L. P. (2006). Responses of estuarine benthic invertebrates to sediment burial: the importance of mobility and adaptation. Hydrobiologia 556, 85–98. doi: 10.1007/s10750-005-1029-0
Hitchcock, J. N., Mitrovic, S. M., Kobayashi, T., and Westhorpe, D. P. (2010). Responses of estuarine bacterioplankton, phytoplankton and zooplankton to dissolved organic carbon (DOC) and inorganic nutrient additions. Estuar. Coast. 33, 78–91. doi: 10.1007/s12237-009-9229-x
Hummel, H., Sokolowski, A., Bogaards, R., and Wolowicz, M. (2000). Ecophysiological and genetic traits of the Baltic clam Macoma balthica in the Baltic: differences between populations in the Gdansk Bay due to acclimatization or genetic adaptation. Int. Rev. Hydrobiol. 85, 621–637. doi: 10.1002/1522-2632(200011)85:5/6<621::AID-IROH621<3.0.CO;2-F
Hyland, J., Balthis, L., Karakassis, I., Magni, P., Petrov, A., Shine, J., et al. (2005). Organic carbon content of sediments as an indicator of stress in the marine benthos. Mar. Ecol. Prog. Ser. 295, 91–103. doi: 10.3354/meps295091
Josefson, A. B., and Widbom, B. (1988). Differential response of benthic macrofauna and meiofauna to hypoxia in the Gullmar Fjord basin. Mar. Biol. 100, 31–40. doi: 10.1007/BF00392952
Kemp, W. M., Boynton, W. R., Adolf, J. E., Boesch, D. F., Boicourt, W. C., Brush, G., et al. (2005). Eutrophication of Chesapeake Bay: historical trends and ecological interactions. Mar. Ecol. Prog. Ser. 303, 1–29. doi: 10.3354/meps303001
Korpanty, C. A., and Kelley, P. H. (2014). Molluscan live–dead agreement in anthropogenically stressed seagrass habitats: siliciclastic versus carbonate environments. Palaeogeogr. Palaeoclimatol. Palaeoecol. 410, 113–125. doi: 10.1016/j.palaeo.2014.05.014
Levin, L. A., Talley, T. S., and Hewitt, J. (1998). Macrobenthos of Spartina foliosa (Pacific Cordgrass) salt marshes in Southern California: community structure and comparison to a Pacific mudflat and a Spartina alterniflora (Atlantic smooth cordgrass) marsh. Estuaries 21, 129–144. doi: 10.2307/1352552
Lin, H., Kun, L., Wang, J., Huang, Y., Zhong, L., Junhui, L., et al. (2018). Spatial pattern of macrobenthic communities along a shelf-slope-basin transect across the Bering Sea. Acta Oceanol. Sini. 37, 72–81. doi: 10.1007/s13131-018-1192-6
Long, W. C., and Seitz, R. D. (2009). Hypoxia in Chesapeake Bay tributaries: worsening effects on macrobenthic community structure in the York River. Estuaries Coast. 32, 287–297. doi: 10.1007/s12237-009-9132-5
Lu, X., Zhou, F., Chen, F., Lao, Q., Zhu, Q., Meng, Y., et al. (2020). Spatial and seasonal variations of sedimentary organic matter in a subtropical bay: implication for human interventions. Int. J. Environ. Res. Public Health 17:1362. doi: 10.3390/ijerph17041362
Mangum, C. P., Santos, S. L., and Rhodes, W. R. (1968). Distribution and feeding in the onuphid polychaete, Diopatra cuprea (Bosc). Mar. Biol. 2, 33–40. doi: 10.1007/BF00351635
Mikkelsen, P. M., Mikkelsen, P. S., and Karlen, D. J. (1995). Molluscan biodiversity in the Indian River lagoon, Florida. Bull. Mar. Sci. 57, 94–127.
Morris, L. J., and Virnstein, R. W. (2004). The demise and recovery of seagrass in the northern Indian River Lagoon, Florida. Estuaries 27, 915–922. doi: 10.1007/BF02803418
National Research Council (1989). Contaminated Marine Sediments: Assessment and Remediation. Washington, DC: The National Academies Press.
Norkko, A., Rosenberg, R., Thrush, S. F., and Whitlatch, R. B. (2006). Scale-and intensity-dependent disturbance determines the magnitude of opportunistic response. J. Exp. Mar. Biol. Ecol. 330, 195–207. doi: 10.1016/j.jembe.2005.12.027
Paerl, H. W. (2006). Assessing and managing nutrient-enhanced eutrophication in estuarine and coastal waters: interactive effects of human and climatic perturbations. Ecol. Eng. 26, 40–54. doi: 10.1016/j.ecoleng.2005.09.006
Pearson, T. H., and Rosenberg, R. (1978). Macrobenthic succession in relation to organic enrichment and pollution of the marine environment. Oceanogr. Mar. Biol. Ann. Rev. 16, 229–311.
Peltzer, E. T., Zhang, X., Walz, P. M., Luna, M., and Brewer, P. G. (2016). In situ Raman measurement of HS– and H2S in sediment pore waters and use of the HS–:H2S ratio as an indicator of pH. Mar. Chem. 184, 32–42.
Phlips, E. J., Badylak, S., Lasi, M. A., Chamberlain, R., Green, W. C., Hall, L. M., et al. (2015). From red tides to green and brown tides: bloom dynamics in a restricted subtropical lagoon under shifting climatic conditions. Estuar. Coasts 38, 886–904. doi: 10.1007/s12237-014-9874-6
Rhyther, J. H., and Dunstan, W. M. (1971). Nitrogen, phosphorus, and eutrophication in the coastal marine environment. Science 171, 1008–1013. doi: 10.1126/science.171.3975.1008
Santos, S. L., and Simon, J. L. (1980). Response of soft-bottom benthos to annual catastrophic disturbance in a south Florida estuary. Mar. Ecol. Prog. Ser. 3, 347–355. doi: 10.3354/meps003347
Schultz, P., and Urban, N. R. (2008). Effects of bacterial dynamics on organic matter decomposition and nutrient release from sediments: a modeling study. Ecol. Modell. 210, 1–14. doi: 10.1016/j.ecolmodel.2007.06.026
Schulz, H. N., Strotmann, B., Gallardo, V. A., and Jørgensen, B. B. (2000). Population study of the filamentous sulfur bacteria Thioploca spp. off the Bay of Concepcion, Chile. Mar. Ecol. Prog. Ser. 200, 117–126. doi: 10.3354/meps200117
Seitz, R. D., Lipcius, R. N., Hines, A. H., and Eggleston, D. B. (2001). Density-dependent predation, habitat variation, and the persistence of marine bivalve prey. Ecology 82, 2435–2451.
Shin, P. K., Lam, N. W. Y., Wu, R. S. S., Qian, P. Y., and Cheung, S. G. (2008). Spatio-temporal changes of marine macrobenthic community in sub-tropical waters upon recovery from eutrophication. I. Sediment quality and community structure. Mar. Pollut. Bull. 56, 282–296. doi: 10.1016/j.marpolbul.2007.10.023
Shumway, S. E., Scott, T. M., and Shick, J. M. (1983). The effects of anoxia and hydrogen sulphide on survival, activity and metabolic rate in the coot clam, Mulinia lateralis. J. Exp. Mar. Biol. Ecol. 71, 135–146. doi: 10.1016/0022-0981(93)90069-Z
Smith, N. P. (2007). Inter-annual variability in net outflow from Indian River Lagoon. Florida Sci. 70, 332–343.
Southwell, M. W., Kieber, R. J., Mead, R. N., Avery, G. B., and Skrabal, S. A. (2010). Effects of sunlight on the production of dissolved organic and inorganic nutrients from resuspended sediments. Biogeochemistry 98, 115–126. doi: 10.1007/s10533-009-9380-2
Stoner, A. W. (1980). The role of seagrass biomass in the organization of benthic macrofaunal assemblages. Bull. Mar. Sci. 30, 537–551.
Tetra Tech Inc and Closewaters LLC (2021). Save Our Indian River Lagoon Project Plan Update 2021. Melbourne, FL: Brevard County Natural Resources Management.
Thompson, B., and Lowe, S. (2004). Assessment of macrobenthos response to sediment contamination in the San Francisco estuary, California, USA. Environ. Toxicol. Chem. 23, 2178–2187. doi: 10.1897/03-233
Thomsen, M. S., and McGlathery, K. (2005). Facilitation of macroalgae by the sedimentary tube forming polychaete Diopatra cuprea. Estuar. Coast Shelf Sci. 62, 63–73. doi: 10.1016/j.ecss.2004.08.007
Trefry, J. H., Metz, S., Trocine, R. P., Iricanin, N., Burnside, D., Chen, N., et al. (1990). Design and Operation of a Muck Sediment Survey. Special Report SJ90-SP3. Palatka, FL: Saint Johns River Water Management District.
Trefry, J. H., and Trocine, R. P. (2011). Metals in sediments and clams from the Indian River Lagoon, Florida: 2006–7 versus 1992. Florida Sci. 74, 43–62.
United States Army Corps of Engineers (2018). Final Integrated Feasibility Report and Environmental Assessment: Lake Worth Lagoon, Continuing Authorities Program (CAP) Section 1135 Project. Jacksonville, FL: United States Army Corps of Engineers.
United States Environmental Protection Agency [USEPA] (1989). Ambient Water Quality Criteria for Ammonia (Saltwater) EPA 440/5-88-004. Washington, DC: United States Environmental Protection Agency.
Unsworth, R. K., Ambo-Rappe, R., Jones, B. L., La Nafie, Y. A., Irawan, A., Hernawan, U. E., et al. (2018). Indonesia’s globally significant seagrass meadows are under widespread threat. Sci. Total Environ. 634, 279–286. doi: 10.1016/j.scitotenv.2018.03.315
Virnstein, R., Mikkelsen, P., Cairns, K., and Capone, M. (1983). Seagrass beds versus sand bottoms: the trophic importance of their associated benthic invertebrates. Florida Sci. 46, 363–381.
Virnstein, R. W., and Howard, R. K. (1987). Motile epifauna of marine macrophytes in the Indian River Lagoon, Florida. II. Comparisons between drift algae and three species of seagrasses. Bull. Mar. Sci. 41, 13–26.
Warwick, R. M., and Clarke, K. R. (1995). New ‘biodiversity’ measures reveal a decrease in taxonomic distinctness with increasing stress. Mar. Ecol. Prog. Ser. 129, 301–305. doi: 10.3354/meps129301
Keywords: Indian River Lagoon, benthic infauna, sediment management, total organic carbon (TOC), anoxic sediments, sediment remediation, eutrophication
Citation: Fuller KM, Fox AL, Jacoby CA and Trefry JH (2021) Biological Abundance and Diversity in Organic-Rich Sediments From a Florida Barrier Island Lagoon. Front. Mar. Sci. 8:768083. doi: 10.3389/fmars.2021.768083
Received: 01 September 2021; Accepted: 29 October 2021;
Published: 22 November 2021.
Edited by:
Edward J. Phlips, University of Florida, United StatesReviewed by:
David J. Karlen, Environmental Protection Commission of Hillsborough County, United StatesJan Vanaverbeke, Royal Belgian Institute of Natural Sciences, Belgium
Copyright © 2021 Fuller, Fox, Jacoby and Trefry. This is an open-access article distributed under the terms of the Creative Commons Attribution License (CC BY). The use, distribution or reproduction in other forums is permitted, provided the original author(s) and the copyright owner(s) are credited and that the original publication in this journal is cited, in accordance with accepted academic practice. No use, distribution or reproduction is permitted which does not comply with these terms.
*Correspondence: Kate M. Fuller, a2JlY2tldHQyMDEzQGdtYWlsLmNvbQ==