- 1Department of Ecology and Evolutionary Biology, College of Agriculture and Life Sciences, Cornell University, Ithaca, NY, United States
- 2Seagrove Kelp Co., Ketchikan, AK, United States
- 3Department of Biology, Southeast Missouri State University, Cape Girardeau, MO, United States
- 4Department of Natural Resources, College of Agriculture and Life Sciences, Cornell University, Ithaca, NY, United States
- 5Department of Computer Science, College of Arts and Sciences, Cornell University, Ithaca, NY, United States
Seagrass meadows provide valuable ecosystem benefits but are at risk from disease. Eelgrass (Zostera marina) is a temperate species threatened by seagrass wasting disease (SWD), caused by the protist Labyrinthula zosterae. The pathogen is sensitive to warming ocean temperatures, prompting a need for greater understanding of the impacts on host health under climate change. Previous work demonstrates pathogen cultures grow faster under warmer laboratory conditions and documents positive correlations between warmer ocean temperatures and disease levels in nature. However, the consequences of disease outbreaks on eelgrass growth remain poorly understood. Here, we examined the effect of disease on eelgrass productivity in the field. We coupled in situ shoot marking with high-resolution imagery of eelgrass blades and used an artificial intelligence application to determine disease prevalence and severity from digital images. Comparisons of eelgrass growth and disease metrics showed that SWD impaired eelgrass growth and accumulation of non-structural carbon in the field. Blades with more severe disease had reduced growth rates, indicating that disease severity can limit plant growth. Disease severity and rhizome sugar content were also inversely related, suggesting that disease reduced belowground carbon accumulation. Finally, repeated measurements of diseased blades indicated that lesions can grow faster than healthy tissue in situ. This is the first study to demonstrate the negative impact of wasting disease on eelgrass health in a natural meadow. These results emphasize the importance of considering disease alongside other stressors to better predict the health and functioning of seagrass meadows in the Anthropocene.
Introduction
Rapid environmental changes significantly impact and reshape our oceans. Elevated temperatures can alter marine host-pathogen dynamics by increasing host stress and pathogen virulence, expanding pathogen ranges, and altering host ranges, thus triggering increased occurrence and severity of disease outbreaks (Harvell et al., 2002; Burge et al., 2014; Cohen et al., 2018; Burge and Hershberger, 2020). Climate-driven disease outbreaks can be especially devastating when they target foundation species—like seagrass and corals—that structure nearshore communities and play vital roles in ecosystem function (Harvell and Lamb, 2020). This paper examines the impacts of seagrass wasting disease (SWD) on eelgrass (Zostera marina) health and productivity.
Seagrass wasting disease historically shaped eelgrass meadows and continues to persist today. In the 1930s, SWD outbreaks decimated up to 90% of eelgrass meadows throughout the North Atlantic (Renn, 1936; Short et al., 1987; Muehlstein, 1989), reducing waterfowl, shrimp, scallop, and fish populations (Renn, 1936; Stauffer, 1937; Moffitt and Cottam, 1941; Milne and Milne, 1951) and compromising eelgrass ecosystem services (Orth et al., 2006). These and subsequent die-offs were traced to Labyrinthula zosterae (Muehlstein et al., 1988), which is now recognized as a virulent pathogen in eelgrass (Groner et al., 2014, 2016; Martin et al., 2016) and other seagrasses worldwide (reviewed in Sullivan et al., 2018). Given that eelgrass creates nursery and feeding grounds (Orth et al., 1984), filters and oxygenates seawater (Costanza et al., 1997; Hasegawa et al., 2008), stabilizes sediments (Fonseca et al., 1983), efficiently stores carbon (Duarte et al., 2005; Röhr et al., 2018), and plays important roles in nutrient cycling in nearshore communities (reviewed in Moore and Short, 2006), SWD can compromise the health of eelgrass individuals, populations, and entire coastal communities.
Understanding the impact of SWD on eelgrass health and productivity under field conditions is particularly pertinent, given the synergistic relationship between warming temperatures L. zosterae (Rasmussen, 1977; Muehlstein, 1992; Bull et al., 2012; Bockelmann et al., 2013; Dawkins et al., 2018; Groner et al., in press; Aoki et al., in review). Laboratory studies indicate the pathogen grows faster in vitro under elevated temperatures (Dawkins et al., 2018), and warmer temperatures coincided with historic (Rasmussen, 1977) and more recent disease outbreaks (Bull et al., 2012; Bockelmann et al., 2013). More recently, field surveys of natural eelgrass meadows in the San Juan Islands, Washington, United States indicate that elevated levels of SWD and declines in meadow density were significantly correlated with warmer winter (Groner et al., in press) and summer temperatures (Aoki et al., in review).
Despite the growing body of literature on L. zosterae biology and ecology and continued monitoring of SWD in natural eelgrass meadows (Bockelmann et al., 2012; Groner et al., 2014, 2016, in press; Jakobsson-Thor et al., 2018; Aoki et al., in review), little is known about the impacts of SWD on eelgrass under natural conditions. Laboratory studies have shown that L. zosterae attacks and consumes plant chloroplasts (Muehlstein, 1992), reduces photosynthetic capabilities of tissue (Ralph and Short, 2002), and creates large areas of necrotic tissue (Pokorny, 1967; Short et al., 1986). SWD therefore likely compromise eelgrass growth rates and ability to accumulate non-structural carbon in rhizomes, which contain high concentrations of sugar. Normally, this sugar accumulates during the summer growing season and fuels regrowth in spring (Burke et al., 1996). However, since SWD prevalence peaks during warm summer months (Bockelmann et al., 2013), disease could reduce these valuable sugar reserves and impair eelgrass growth the subsequent year. Here, we coupled ecological surveys and image analysis using artificial intelligence to determine the effect of SWD on eelgrass growth, health, and rhizome sugar in the field.
Eelgrass meadows are considered bioindicators of health within the Salish Sea, an inland sea spanning the United States-Canada border in the northeastern Pacific Ocean (Dennison et al., 1993; McManus et al., 2020), and have experienced high levels of SWD in recent years (Groner et al., 2014, 2016, in press; Aoki et al., in review), making this region an important location for understanding the ecological impacts of SWD. The aim of this study was to examine the effect of SWD on aboveground eelgrass growth and productivity and belowground sugar. We measured blade-level disease status (healthy or diseased), site-level disease prevalence (proportion of shoots with SWD), severity (proportion of tissue damaged by wasting disease lesions), and specific productivity (percentage of new blade area produced per day). Given the significant historical role of L. zosterae in the extirpation of many eelgrass meadows globally, we expected SWD to compromise eelgrass growth. Specifically, we expected plants with elevated levels of disease to have reduced blade growth rates and rhizome sugar concentrations compared to eelgrass with lower disease levels.
Materials and Methods
Field Study 1: Eelgrass Growth
To determine temporal differences and interactions between eelgrass growth, productivity, and SWD, we conducted field trials in June and July 2019 at Fourth of July Beach, San Juan Island, WA (48°28′01.4″ N, 123°00′00.4″ W). We selected this site because its intertidal eelgrass has had consistently high levels of SWD since monitoring began in 2017 (Aoki et al., in review) and remains exposed for several hours during extreme low tides, providing ample time for fieldwork. Each month, we deployed three, 30-m transects spaced approximately 5 m apart in the shallow, intertidal eelgrass meadow at low tide, using a compass to maintain consistent headings and depth gradients for each. We targeted eelgrass in the interior of the meadow to avoid any potential edge effects, since eelgrass growth can vary with nutrients, hydrodynamics, and sediment dynamics within a meadow (Bell et al., 2007). Using neon flagging tape, we tagged individual eelgrass shoots at 1-m intervals along each transect (n = 90 shoots/month, Supplementary Figure 1) to minimize heavily impacting any one area (ex: tagging many shoots in a quadrat), since all shoots were ultimately destructively sampled. We marked each tagged shoot for shoot-specific growth rate measurements using the needle punch method (Short and Duarte, 2001), a standard approach for measuring direct, short-term growth rates in seagrasses. At 5-m intervals along each transect, we recorded eelgrass shoot densities. We also scanned a subset of tagged shoots from each transect for baseline disease measurements (n = 4 in June, n = 25 in July) and to track lesion development following 6 days of growth in the field. To do so, we removed epiphytes from the third-rank blade of the shoot and carefully scanned it at high resolution (600 dpi) using a photocopy scanner (Canon CanoScan LiDE 220) whilst the shoot was still rooted in the sediment. We also deployed 2 HOBO Pendant loggers in the eelgrass meadow to capture in situ temperatures.
After 6 days, we collected all tagged shoots (n = 90 in each month). In lab, we removed epiphytes from all shoots and separated the blades from each shoot by age; each shoot contained 3–7 blades. The needle-punch method enabled us to easily separate old growth—the blade area above the needle scar—from the new growth—the blade area below the scar (Supplementary Figure 1). We scanned all blades (n = 434 in June, n = 398 in July) to measure disease and blade areas (Supplementary Figure 1). We also measured canopy height, number of blades, and sheath length for each shoot. We calculated blade growth rates (specific productivity) as the percentage of daily new blade area (mm2d–1).
Field Study 2: Non-structural Carbon Analyses
We collected belowground rhizomes to determine the effect of SWD on sugar reserves in July 2019. We targeted three sites in the San Juan Islands—Fourth of July Beach, Indian Cove (48°33′47.6″ N, 122°56′11.2″ W), False Bay (48°28′57.9″ N, 123°04′25.0″ W)—known for having variable levels of SWD (Groner et al., 2016, in press; Aoki et al., in review). At each site, we deployed three, 30-m transects at the same tidal height in the intertidal, where we measured shoot densities and collected five shoots with intact rhizomes at 0, 15, and 30 m (n = 45 shoots/site, n = 135 total). We targeted rhizomes at least 15 cm long to ensure sufficient sample biomass for subsequent non-structural carbon analyses. We scanned the third-rank (third youngest, Short and Duarte, 2001) blade of each shoot for disease analyses. To analyze belowground sugar content (non-structural carbon), we dried rhizomes at 60°C, homogenized with a mortar and pestle, and extracted sugars using the hot ethanol extraction method with 2% phenol and concentrated sulfuric acid. We determined total sugar concentrations based on the absorbance at 490 nm using a glucose-fructose-galactose standard curve according to published protocols (Chow and Landhausser, 2004).
Disease Analyses
A state-of-the-art computer learning algorithm, the Eelgrass Lesion Image Segmentation Application (EeLISA), identified and measured the total area of wasting disease lesions and the total area of healthy tissue on all scanned eelgrass images. We used these to determine blade-level disease status (healthy or diseased), and to calculate SWD prevalence (proportion of shoots with SWD lesions), and blade- and site-level severity (proportion of blade area covered in SWD lesions). Details on EeLISA development and training are provided elsewhere (Rappazzo et al., 2021; Aoki et al., in review). EeLISA was trained on over 789 expert-labeled scanned eelgrass images that distinguished SWD lesions from other forms of damage (Rappazzo et al., 2021). Other forms of damage, including desiccation stress and herbivore grazing scars, are distinctive and readily distinguished from SWD lesions, which have been well characterized (Short et al., 1986, 1987; Muehlstein et al., 1988; Burdick et al., 1993). Using ImageJ, we measured the areas of new and old growth for each blade by hand (Supplementary Figure 1).
We compared lesion growth rates and blade growth rates on individual blades using the 29 blades scanned for disease in the field at the start of the marking period and in the lab at the end of the marking period. Using the output from EeLISA for initial and final lesion area, we determined lesion growth rates (mm2d–1) and compared them to the blade growth rates (mm2d–1) calculated from the old and new blade areas at the end of the marking period.
We validated pathogen presence using qPCR to detect L. zosterae DNA in samples of diseased eelgrass tissue. In July 2019, we collected lesion tissue samples from the three field sites and processed the samples using established extraction and qPCR methods (Bockelmann et al., 2013; Groner et al., 2018).
Statistical Analyses
We performed all statistical analyses in R (v. 1.4.1106). To compare the growth rates between all blades from the eelgrass growth study, we ran a generalized linear mixed model (GLMM) with a gamma distribution and log link using the “glmmTMB” package (Brooks et al., 2017), with specific productivity as the response variable. We included blades of all ages (youngest to oldest) in this model to capture the variation in growth rates with respect to disease. Since we measured multiple blades from the same individual shoot, we included shoot identifier as a random effect; fixed effects were blade rank (i.e., age), disease status (healthy or diseased), blade area, total blades per shoot, shoot density (measured at the transect level), and month. We also included an interaction between disease status and blade rank; we compared the full model to simpler models (excluding the interaction and individual terms) using AIC and removed model terms based on ΔAIC > 2. The best-performing model by AIC did not include the interaction term but did include all individual fixed effects. We used a gamma distribution, since there was high variation in growth rates and included a dispersion term, as young blades had a greater spread in growth rates. Blades with growth rates of zero were excluded from these analyses, leaving 530 blades in the model.
To determine the impacts of severity on blade growth, we standardized our measurements by targeting the third-rank (third youngest) blades from each shoot (n = 176). These blades provided useful disease estimates because third-rank blades contained both healthy and diseased tissue and grew at a measurable rate, in contrast to the oldest blades which were often deteriorating and the youngest blades which rarely had any visually apparent lesions. Third-rank blades are also recognized as the best indicators of recent environmental conditions (Sand-Jensen, 1975), including disease (Aoki et al., in review). We used a quantile regression model using the package “quantreg” (Koenker, 2021) to assess the relationship between third-rank blade growth rate and severity. For the third-rank blades (n = 29) for which we had initial and final disease and blade area measurements, we used a paired t-test to compare the lesion and blade growth rates.
To assess disease impacts on belowground non-structural carbon, we ran a linear model with rhizome sugar content as the response variable and disease severity, blade area, shoot density, site, and an interaction between site and severity as fixed effects; site was treated as a fixed effect due to the low number of sites sampled (n = 3). Data met assumptions for normality and homoscedasticity.
Results
Site Characteristics
Temperature loggers deployed at Fourth of July Beach in the intertidal meadow documented daily temperature ranges of 11–20°C during the June marking period and 11–23°C during the July marking period. Daily mean (12.5°C) and median (11.8°C) temperatures were similar during the two marking periods. Maximum daily temperatures occurred when the meadow was exposed during midday low tides. Across the three meadows, shoot densities were lowest at Fourth of July Beach (60 ± 28 shoots m–2, mean ± SD) and were similar at False Bay (106 ± 28 shoots m–2) and Indian Cove (103 ± 28 shoots m–2). Molecular analysis confirmed the presence of L. zosterae DNA in diseased eelgrass tissue from all three sites, supporting the presence of L. zosterae as an infectious pathogen (Supplementary Table 1).
Disease Prevalence and Severity
Wasting disease was prevalent at Fourth of July Beach, in both June and July 2019. In both months, disease prevalence (number of infected shoots/total number of shoots) was approximately 95%. Shoot-level severity (total lesion area of all blades on a shoot/total blade area for the shoot) was also similar between the two months, ranging from 0–36% in June and 0–39% in July and averaging 9% in June and 10% in July. Overall, these disease metrics indicated a substantial presence of the pathogen and potential for meadow-wide impacts from disease.
Disease and Blade Growth Rates
Blade-level disease status and blade rank (age), standardized blade area, and the total number of blades per shoot were significant predictors of blade growth rates (Figure 1 and Supplementary Table 2). Diseased and older blades grew significantly slower than healthy and younger blades (glmm, p < 0.001). A diseased, first-rank (youngest) blade grew at approximately 82% of the rate of a healthy, first-rank blade (Supplementary Figure 2). Blade growth rates steadily decreased in both diseased and healthy blades with increasing blade age; second-rank blades grew at 17% the rate of first-rank blades. Total number of leaves per shoot and blade area were also significant in the best performing model; smaller blades and shoots with more leaves had faster relative growth (Supplementary Figure 2). Shoot density and month were not significant predictors of growth rates.
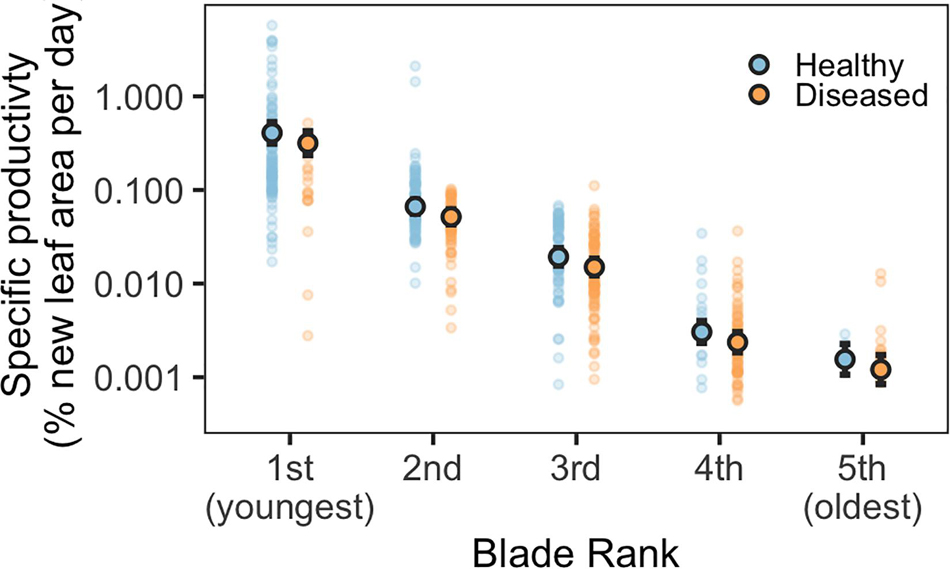
Figure 1. Predicted specific productivity for diseased and healthy eelgrass blades of different ages, modeled as a function of blade rank (age) and disease status (note the log scale). Blade rank refers to blade age and position on each shoot (1st rank = youngest, 5th rank = 5th youngest). Outlined circles indicate mean predicted values, solid circles indicate underlying data. Error bars indicate 95% confidence intervals of the prediction.
Disease severity ranged from 0–70% for third-rank blades across June and July. Blades with more severe SWD (larger proportion of blade tissue damaged) had reduced growth rates compared to blades with less severe SWD, as predicted. Quantile regression showed that this effect was larger for blades with higher disease levels (Figure 2). In higher quantiles with elevated severity, the slopes of the regression lines were steeper than lower quantiles, indicating larger effects. This was also reflected by severity coefficients, which sharply increased in magnitude at higher quantiles (Supplementary Figure 3).
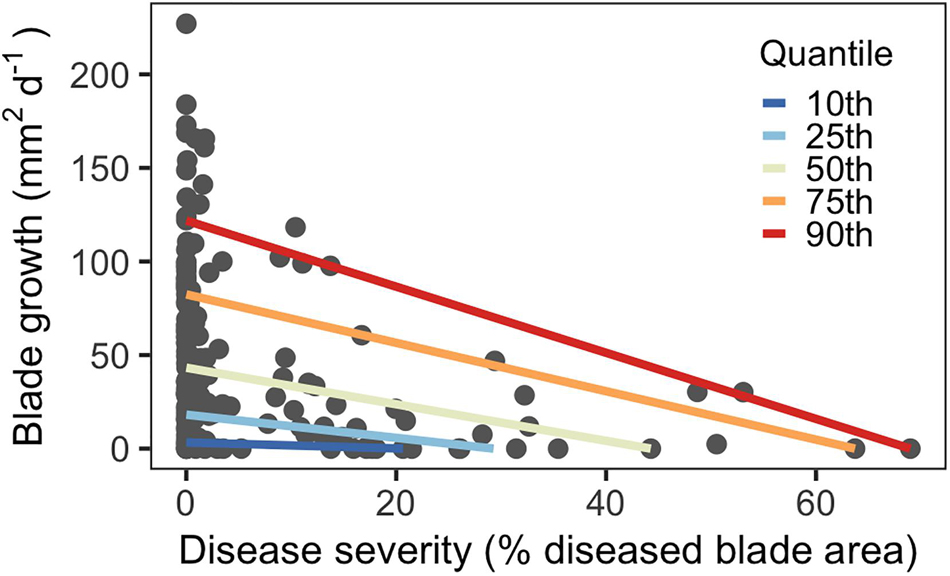
Figure 2. Quantile regression model showing decreasing blade growth in response to disease severity for third-rank blades (n = 176).
Lesion Growth Rates
Repeated measurements of diseased blades indicated that lesion growth rates can significantly exceed blade growth rates in situ for third-rank (third youngest) blades (Figure 3, paired t-test, p < 0.05). Mean lesion growth rates were 51 mm2d–1, with a maximum rate of 371 mm2d–1. In comparison, the mean absolute blade growth rate for this subset of third-rank blades was 16 mm2d–1 and the maximum growth rate was 71 mm2d–1.
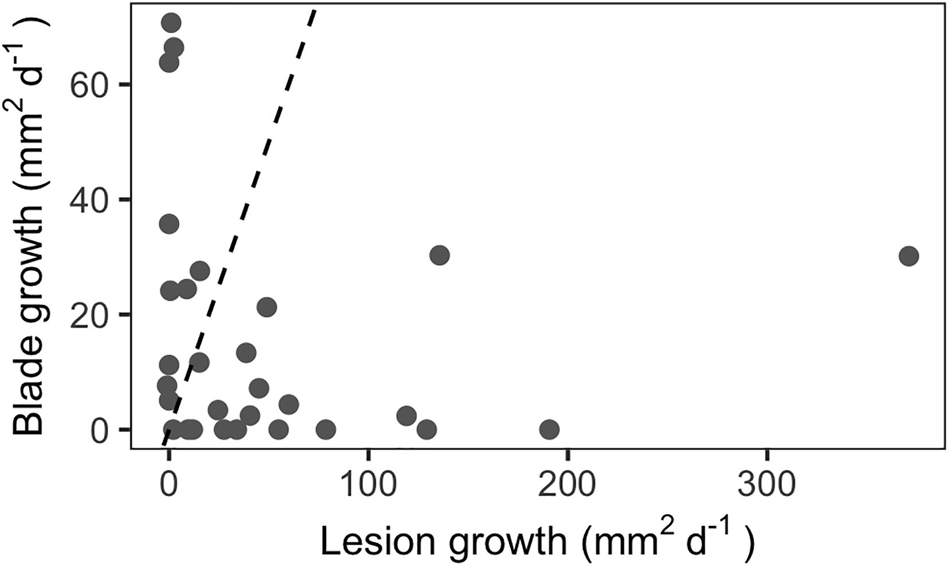
Figure 3. In situ lesion growth rates outpaced blade growth rates among third-rank blades (n = 29). Dashed line indicates the 1:1 line.
Non-structural Carbon Analyses
Across the three meadows, disease severity and rhizome sugar content (mg per g rhizome dry weight) were inversely related (Figure 4). Disease severity ranged from 0–10% at Indian Cove and from 0–20% at Fourth of July Beach and False Bay. Both disease severity and site were significant predictors for rhizome sugar concentrations, together explaining roughly 50% of variation in sugar content (Supplementary Table 3). Shoot density was not a significant predictor of belowground sugar content. Mean (± SD) sugar content was highest at Fourth of July Beach (419 ± 56 mg per g rhizome dry weight) and similar at Indian Cove (311 ± 53 mg per g) and False Bay (356 ± 54 mg per g). Despite the variation between sites, the overall pattern was consistent: at higher disease severities, rhizome sugar concentrations decreased significantly.
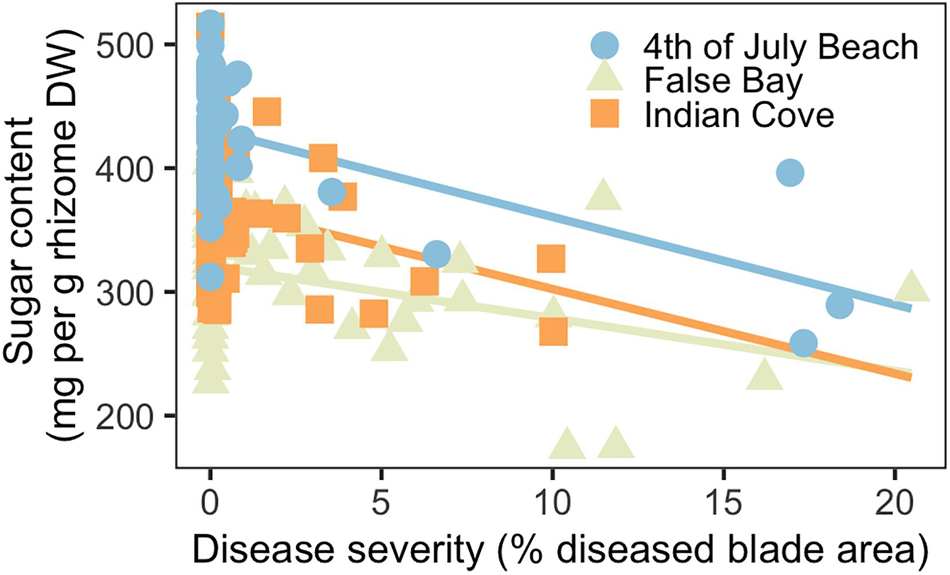
Figure 4. Rhizome sugar content declined as a function of disease severity (n = 135) at three eelgrass meadows.
Discussion
Globally, seagrasses are declining under pressure from coastal development, climate change, and disease (Orth et al., 2006; Waycott et al., 2009; Dunic et al., 2021). To sustain and conserve these crucial habitats and their ecosystem services, we must determine the impacts of disease on eelgrass health. Though laboratory studies have identified the impact of SWD on eelgrass photosynthesis (Ralph and Short, 2002) and necrosis (Pokorny, 1967; Short et al., 1986; Muehlstein, 1992) and field surveys continue to highlight outbreak-levels of disease (Groner et al., 2014, 2016, in press; Jakobsson-Thor et al., 2018; Aoki et al., in review), the direct impact of SWD on eelgrass growth and sugar reserves in natural meadows remains unknown. Here we show that SWD predicts reduced blade growth rates and rhizome sugar concentrations in natural meadows. However, given the observational nature of this field study, reverse causality could account for the reduced blade growth among eelgrass with elevated SWD severity. For example, slower growing eelgrass could be more susceptible to SWD compared to faster-growing eelgrass. The observed reduced blade growth rates also could be due to other confounding factors like blade-level differences in epiphyte loads, which have been associated with higher probabilities of eelgrass having SWD (Groner et al., 2016). Shading due to epiphytes could then impact both SWD severity and eelgrass growth.
Our observed SWD prevalence and severity levels were higher than historical measurements from nearby sites. Previously, intertidal field surveys from 2012–2013 found disease prevalence ranged from 6–79% (Groner et al., 2014, 2016) elsewhere in the San Juan Islands, WA. These disparities may in part be due to different methods for calculating prevalence. Groner et al. (2014) measured prevalence—the proportion of diseased blades relative to total number of blades—based on the longest blade on each shoot, while Groner et al. (2016) used the second-oldest intact blade on each shoot. Our results based on the proportion of diseased shoots relative to the total number of shoots indicated elevated SWD levels, like observed prevalence of 95.8–100% in intertidal Swedish eelgrass (Jakobsson-Thor et al., 2018). While some spatiotemporal variation is expected, the near-100% prevalence we observed at Fourth of July Beach could be in part linked to warmer ocean temperatures in 2019 (Aoki et al., in review; Amaya et al., 2020). In comparison to historical sea surface temperature records, summer 2019 temperatures were warmer locally in the San Juan Islands (Aoki et al., in review) and more broadly for the North Pacific (Amaya et al., 2020). This is important for eelgrass growth and health, since warm temperatures are associated with reduced production of phenolic compounds (Vergeer et al., 1995), which are used in microbial defense. Among eelgrass, thermal stress during warmer summer months can also increase respiration compared to photosynthesis, altering photosynthetic and growth rates (Phillips and Menez, 1988; Lee et al., 2007). Taxed by thermal stress and compromised growth, eelgrass could be more susceptible to SWD in certain conditions. Indeed, warming temperatures have recently been suggested to contribute to SWD outbreaks (Groner et al., 2014, in press; Martin et al., 2016; Sullivan et al., 2018; Aoki et al., in review).
While outbreaks of SWD have been implicated in meadow decline and collapse, understanding how disease impacts at individual blade- and shoot-levels scale across eelgrass populations and ecosystems remains unclear. Our results suggest that consequences of disease may have ecosystem-wide effects under natural conditions. Diseased, first-rank blades grew nearly 25% slower than healthy, first-rank blades. Because blade growth is concentrated in the youngest two blades (Sand-Jensen, 1975), our findings suggest SWD in natural meadows may limit shoot size at maturity by compromising the growth of the young, fast-growing blades. Disease on mature blades may also reduce total shoot growth. Although third-rank blades have lower specific productivity rates (blade elongation as a percent of existing blade area per day), absolute blade growth rates could exceed 200 mm2d–1 for third-rank blades. These growth rates declined significantly with more severe SWD, suggesting that greater disease severity could limit plant growth. Rapidly spreading SWD may further influence total shoot physiology by interrupting transport of oxygen, photosynthesis products, and/or nutrients (Ralph and Short, 2002). The reduced rhizome sugar concentrations in severely diseased plants indicate SWD likely affected belowground carbon accumulation. Indeed, reduced non-structural carbon reserves were likely related to the observed, compromised blade growth, as diseased eelgrass has reduced photosynthetic capacity and may dedicate more resources to immune response (Vergeer et al., 1995). Ultimately, the combination of compromised growth and diminished sugar stores may reduce shoot survival, and in meadows such as this study site, where almost every plant had SWD, the impacts could accumulate at the ecosystem scale.
Reduced blade growth and rhizome sugars associated with severe SWD suggest that the disease can limit the persistence of entire eelgrass meadows over time. Some eelgrass meadows experience increased thermal and pathogen stress during warmer summer months (Young, 1943; Bockelmann et al., 2013; Aoki et al., in review). Taken together, this could reduce not only immediate eelgrass growth, which normally peaks in summer (Sand-Jensen, 1975), but also alter seasonal patterns in eelgrass productivity. Heavily diseased eelgrass with compromised growth and rhizome sugars may be vulnerable to environmental stressors over winter or early spring when eelgrass draws upon critical carbohydrate reserves from the previous summer (Burke et al., 1996). Less robust plants may be further vulnerable to SWD the following summer, creating a reinforcing feedback loop that could drive meadow-wide declines. Prior studies have noted that while SWD outbreaks can cause large-scale meadow die-offs, other meadows can support widespread disease without collapsing (Short et al., 1987; Jakobsson-Thor et al., 2018). The results from this study show how SWD may negatively impact the health of a meadow without immediately causing an ecosystem collapse. A precipitating disturbance or change in environmental conditions may then trigger widespread die-offs of vulnerable eelgrass. The global losses of eelgrass in the 1930s likely resulted from some combination of SWD and environmental stressors (Sullivan et al., 2013). However, studies testing the combined effects of SWD and stressors such as light availability, salinity, and temperature tend to occur in short-term laboratory mesocosms experiments (e.g., Brakel et al., 2019; Jakobsson-Thor et al., 2020). While these allow for carefully controlled testing of multiple stressors, they do not fully capture the long-term effects of SWD and environmental conditions in natural meadows. Additional field-based studies are needed to better understand these dynamic interactions over time.
In addition to making meadows more vulnerable to collapse, SWD may also impair meadow recovery following disturbance. At the meadow scale, reduced growth rates and carbohydrate reserves may limit regrowth of diseased shoots compared to healthy shoots. Rapid regrowth following disturbance is key to maintaining seagrass ecosystem services, such as carbon sequestration and biodiversity (Nowicki et al., 2017; Aoki et al., 2021); slower recovery rates may also increase meadow vulnerability to repeated disturbance, such as successive marine heat waves. By both increasing vulnerability and impairing recovery, SWD reduces the ecological resilience of eelgrass meadows and may impede conservation of existing meadows. Given the significant role of eelgrass in structuring coastal ecosystems and driving biogeochemical processes (reviewed in Moore and Short, 2006), reduced meadow resilience will have negative consequences for habitat provisioning, nutrient cycling, and other ecosystem services. Better understanding of the impact of chronic SWD on meadow trajectories through time is needed to optimize seagrass conservation and restoration efforts.
Further work is needed to understand the complex interactions between climate change, SWD, and eelgrass meadow resilience. The effects of environmental stressors on eelgrass, including changes in sea surface temperatures, salinity, ocean acidification, and altered light availability due to sea level rise, are not well understood (Short et al., 2016). Recent field surveys indicate positive associations between temperature and SWD (Groner et al., in press; Aoki et al., in review), while others suggest the relationship may be more bell-shaped, given the optimal thermal ranges for L. zosterae (Young, 1943; Olsen et al., 2015). Furthermore, the interactions between SWD and other environmental stressors remains unclear. Some reports indicate future conditions may reduce the effects of disease (Brakel et al., 2019), while others determined SWD is correlated with salinity (Jakobsson-Thor et al., 2018) and may be sensitive to pH (Groner et al., 2018). Certainly, interactions between temperature and other aspects of climate change may influence SWD but necessitate further research to understand these complex relationships and determine drivers of eelgrass resilience.
This study emphasizes the importance of considering disease alongside other climate change stressors to better predict the health and functioning of seagrass meadows in the Anthropocene. To the best of our knowledge, this is the first project to demonstrate that L. zosterae can significantly compromise eelgrass growth and belowground, non-structural carbohydrates in natural meadows. This work broadens our understanding of the effects of SWD on eelgrass productivity and is particularly relevant given mounting environmental stressors. Of course, these analyses were limited to eelgrass in one region over one summer growing season. Furthermore, at a site there can be multiple L. zosterae strains, which can vary in virulence (Martin et al., 2016) and produce significant differences in disease severity (Dawkins et al., 2018). Other groups of seagrass pathogens, including oomycetes and Phytomyxea (reviewed in Sullivan et al., 2018), should also be considered in the context of eelgrass health and productivity. As such, more studies are needed to examine these plant-pathogen relationships across the range of environmental conditions of existing eelgrass meadows. Eelgrass is a foundation species of critical coastal habitat across the northern hemisphere. Understanding the vulnerability of these meadows to disease is essential to their conservation across the species’ range and in a changing climate.
Data Availability Statement
The datasets presented in this study can be found in online repositories. The names of the repository/repositories and accession number(s) can be found below: The datasets analyzed for this study can be found in the Zenodo repository (https://doi.org/10.5281/zenodo.5339331).
Author Contributions
OG, TS, and CH conceived of this study. OG, LA, and SD developed the methods. OG, LA, TS, and JS collected and processed samples in the laboratory. BR and CG developed EeLISA. BR processed images. LA analyzed the data. OG and LA wrote the manuscript. CG and CH provided resources and supervision. CH helped in the field. All authors contributed to editing this manuscript.
Funding
SD and JS received support from the National Science Foundation’s Research Experience for Undergraduates (NSF-REU) program. The Friday Harbor Labs Research Fellowship Endowment provided support for OG. LA was supported by NSF award OCE-1829921.
Conflict of Interest
TS is employed by Seagrove Kelp Co.
The remaining authors declare that the research was conducted in the absence of any commercial or financial relationships that could be construed as a potential conflict of interest.
Publisher’s Note
All claims expressed in this article are solely those of the authors and do not necessarily represent those of their affiliated organizations, or those of the publisher, the editors and the reviewers. Any product that may be evaluated in this article, or claim that may be made by its manufacturer, is not guaranteed or endorsed by the publisher.
Acknowledgments
We would like to thank Jack Novak for help with collecting and processing samples, and to the University of Washington’s Friday Harbor Laboratories community, which supports collaborative research like this! We would also like to thank three reviewers for their thoughtful, constructive feedback.
Supplementary Material
The Supplementary Material for this article can be found online at: https://www.frontiersin.org/articles/10.3389/fmars.2021.768668/full#supplementary-material
References
Amaya, D. J., Miller, A. J., Xie, S.-P., and Kosaka, Y. (2020). Physical drivers of the summer 2019 North Pacific marine heatwave. Nat. Commun. 11:1903. doi: 10.1038/s41467-020-15820-w
Aoki, L. R., McGlathery, K. J., Wiberg, P. L., Oreska, M. P. J., Berger, A. C., Berg, P., et al. (2021). Seagrass recovery following marine heat wave influences sediment carbon stocks. Front. Mar. Sci. 7:576784. doi: 10.3389/fmars.2020.576784
Aoki, L., Rappazzo, B., Beatty, D., Domke, L., Eckert, G., Eisenlord, M., et al. (in review). Continental scale disease surveillance by artificial intelligence links seagrass wasting disease to ocean warming. (In review at Limnology and Oceanography).
Bell, S., Fonesca, M., and Stafford, N. (2007). “Seagrass Ecology: New Contributions from a Landscape Perspective,” in Seagrasses: Biology, Ecology, and Conservation, eds A. Larkum, R. Orth, and C. Duarte (Netherland: Springer), 625–645. doi: 10.1007/1-4020-2983-7_26
Bockelmann, A. C., Beining, K., and Reusch, T. (2012). Widespread occurrence of endophytic Labyrinthula spp. in northern European eelgrass Zostera marina beds. Mar. Ecol. Prog. Ser. 445, 109–116. doi: 10.3354/meps09398
Bockelmann, A. C., Tams, V., Ploog, J., Schubert, P. R., and Reusch, T. B. H. (2013). Quantitative PCR reveals strong spatial and temporal variation of the wasting disease pathogen, Labyrinthula zosterae in Northern European eelgrass (Zostera marina) beds. PLoS One 8:e62169. doi: 10.1371/journal.pone.0062169
Brakel, J., Jakobsson-Thor, S., Bockelmann, A. C., and Reusch, T. B. H. (2019). Modulation of the eelgrass – Labyrinthula zosterae interaction under predicted ocean warming, salinity change and light limitation. Front. Mar. Sci. 6:268. doi: 10.3389/fmars.2019.00268
Brooks, M. E., Kristensen, K., van Benthem, K. J., Magnusson, A., Berg, C. W., Nielsen, A., et al. (2017). glmmTMB balances speed and flexibility among packages for zero-inflated generalized linear mixed modeling. R. J. 9, 378–400. doi: 10.32614/rj-2017-066
Bull, J. C., Kenyon, E. J., and Cook, K. J. (2012). Wasting disease regulates long-term population dynamics in a threatened seagrass. Oecologia 169, 135–142. doi: 10.1007/s00442-011-2187-6
Burdick, D. M., Short, F. T., and Wolf, J. (1993). An index to assess and monitor the progression of wasting disease in eelgrass Zostera marina. Mar. Ecol. Prog. Series. 94, 83–90.
Burge, C. A., Eakin, M. C., Friedman, C. S., Froelich, B., Hershberger, P. K., Hofmann, E. E., et al. (2014). Climate change influences on marine infectious diseases: Implications for management and society. Ann. Rev. Mar. Sci. 6, 249–277. doi: 10.1146/annurev-marine-010213-135029
Burge, C. A., and Hershberger, P. K. (2020). “Climate change can drive marine diseases,” in Marine Disease Ecology, eds D. C. Behringer, B. R. Silliman, and K. D. Lafferty (Oxford: Oxford University Press), 83–94.
Burke, M., Dennison, W., and Moore, K. (1996). Non-structural carbohydrate reserves of eelgrass Zostera marina. Mar. Ecol. Prog. Ser. 137, 195–201. doi: 10.3354/meps137195
Chow, P. S., and Landhausser, S. M. (2004). A method for routine measurements of total sugar and starch content in woody plant tissues. Tree Phys. 24, 1129–1136. doi: 10.1093/treephys/24.10.1129
Cohen, R., James, C., Lee, A., Martinelli, M., Muraoka, W., Ortega, M., et al. (2018). Marine host-pathogen dynamics: Influences of global climate change. Oceanography 31:201. doi: 10.5670/oceanog.2018.201
Costanza, R., d’Arge, R., de Groot, R., Farber, S., Grasso, M., Hannon, B., et al. (1997). The value of the world’s ecosystem services and natural capital. Ecol. Eco. 25, 3–15. doi: 10.1016/S0921-8009(98)00020-2
Dawkins, P., Eisenlord, M., Yoshioka, R., Fiorenza, E., Fruchter, S., Giammona, F., et al. (2018). Environment, dosage, and pathogen isolate moderate virulence in eelgrass wasting disease. Dis. Aquat. Org. 130, 51–63. doi: 10.3354/dao03263
Dennison, W. C., Orth, R. J., Moore, K. A., Stevenson, J. C., Carter, V., Kollar, S., et al. (1993). Assessing water quality with submersed aquatic vegetation. Biosci 43, 86–94.
Duarte, C. M., Middelburg, J. J., and Caraco, N. (2005). Major role of marine vegetation on the oceanic carbon cycle. Biogeosciences 2, 1–8. doi: 10.1371/journal.pone.0052932
Dunic, J. C., Brown, C. J., Connolly, R. M., Turschwell, M. P., and Côté, I. M. (2021). Long−term declines and recovery of meadow area across the world’s seagrass bioregions. Glob. Change Biol. 27, 4096–4109. doi: 10.1111/gcb.15684
Fonseca, M. S., Zieman, J. C., Thayer, G. W., and Fisher, J. S. (1983). The role of current velocity in structuring eelgrass (Zostera marina L.) meadows. Estuar. Coast. Shelf Sci. 17, 367–380. doi: 10.1016/0272-7714(83)90123-3
Groner, M., Burge, C. A., Couch, C., Kim, C., Siegmund, G., Singhal, S., et al. (2014). Host demography influences the prevalence and severity of eelgrass wasting disease. Dis. Aquat. Org. 108, 165–175. doi: 10.3354/dao02709
Groner, M., Burge, C. A., Kim, C., Rees, E., Van Alstyne, K., Yang, S., et al. (2016). Plant characteristics associated with widespread variation in eelgrass wasting disease. Dis. Aquat. Org. 118, 159–168. doi: 10.3354/dao02962
Groner, M., Eisenlord, M., Yoshioka, R., Fiorenza, E., Dawkins, P., Graham, O., et al. (in press). Warming sea surface temperatures fuel summer epidemics of eelgrass wasting disease. Mar. Ecol. Prog. Ser. doi: 10.3354/meps13902
Groner, M. L., Burge, C. A., Cox, R., Rivlin, N. D., Turner, M., Van Alstyne, K. L., et al. (2018). Oysters and eelgrass: potential partners in a high PCO2 ocean. Ecology 99, 1802–1814. doi: 10.1002/ecy.2393
Harvell, C. D., and Lamb, J. B. (2020). “Disease outbreaks can threaten marine biodiversity,” in Marine Disease Ecology. Oxford: Oxford University Press, 141–158.
Harvell, C. D., Mitchell, C., Ward, J., Altizer, S., Dobson, A., Ostfeld, R., et al. (2002). Climate warming and disease risks for terrestrial and marine biota. Science 296, 2158–2162. doi: 10.1126/science.1063699
Hasegawa, N., Hori, M., and Mukai, H. (2008). Seasonal changes in eelgrass functions: current velocity reduction, prevention of sediment resuspension, and control of sediment–water column nutrient flux in relation to eelgrass dynamics. Hydrobiologia 596, 387–399. doi: 10.1007/s10750-007-9111-4
Jakobsson-Thor, S., Brakel, J., Toth, G. B., and Pavia, H. (2020). Complex interactions of temperature, light and tissue damage on seagrass wasting disease in Zostera marina. Front. Mar. Sci. 7:575183. doi: 10.3389/fmars.2020.575183
Jakobsson-Thor, S., Toth, G., Brakel, J., Bockelmann, A., and Pavia, H. (2018). Seagrass wasting disease varies with salinity and depth in natural Zostera marina populations. Mar. Ecol. Prog. Ser. 587, 105–115. doi: 10.3354/meps12406
Koenker, R. (2021). quantreg: Quantile Regression. R package version 5.85. https://CRAN.R-project.org/package=quantreg.
Lee, K.-S., Park, S. R., and Kim, Y. K. (2007). Effects of irradiance, temperature, and nutrients on growth dynamics of seagrasses: a review. J. Exp. Mar. Biol. Ecol. 350, 144–175. doi: 10.1016/j.jembe.2007.06.016
Martin, D. L., Chiari, Y., Boone, E., Sherman, T. D., Ross, C., Wyllie-Echeverria, S., et al. (2016). Functional, phylogenetic, and host-geographic signatures of Labyrinthula spp. provide for putative species delimitation and a global-scale view of seagrass wasting disease. Estuar. Coast. 39, 1403–1421. doi: 10.1007/s12237-016-0087-z
McManus, E., Durance, K., and Khan, S. (2020). Revisions to Puget Sound vital signs and indicators. Seattle: Ross Strategic.
Milne, L., and Milne, M. (1951). The eelgrass catastrophe. Sci. Am. 184, 52–55. doi: 10.1038/scientificamerican0151-52
Moffitt, J., and Cottam, C. (1941). Eelgrass depletion on the Pacific coast and its effect upon the Black Brant. Virginia: US Department of Interior Fish and Wildlife Service.
Moore, K. A., and Short, F. T. (2006). “Zostera: Biology, ecology, and management,” in Seagrasses: Biology, Ecology, and Conservation, ed. A. W. D. Larkum (Netherlands: Springer), 361–386. doi: 10.1007/1-4020-2983-7_16
Muehlstein, L. K. (1989). Perspectives on the wasting disease of eelgrass Zostera marina. Dis. Aquat. Org. 7, 211–221. doi: 10.3354/dao007211
Muehlstein, L. K. (1992). The host – pathogen interaction in the wasting disease of eelgrass. Zostera marina. Can. J. Bot. 70, 2081–2088. doi: 10.1139/b92-258
Muehlstein, L. K., Porter, D., and Short, F. T. (1988). Labyrinthula sp., a marine slime mold producing the symptoms of wasting disease in eelgrass, Zostera marina. Mar. Biol. 99, 465–472. doi: 10.1007/bf00392553
Nowicki, R., Thomson, J., Burkholder, D., Fourqurean, J., and Heithaus, M. (2017). Predicting seagrass recovery times and their implications following an extreme climate event. Mar. Ecol. Prog. Ser. 567, 79–93. doi: 10.3354/meps12029
Olsen, Y. S., Potouroglou, M., Garcias-Bonet, N., and Duarte, C. M. (2015). Warming reduces pathogen pressure on a climate-vulnerable seagrass species. Estuar. Coast. 38, 659–667. doi: 10.1007/s12237-014-9847-9
Orth, R. J., Carruthers, T. J. B., Dennison, W. C., Duarte, C. M., Fourqurean, J. W., Heck, K. L., et al. (2006). A global crisis for seagrass ecosystems. BioScience 56:987. doi: 10.1641/0006-3568
Orth, R. J., Heck, K. L., and van Montfrans, J. (1984). Faunal communities in seagrass beds: A review of the influence of plant structure and prey characteristics on predator: prey relationships. Estuaries 7:339. doi: 10.2307/1351618
Pokorny, K. S. (1967). Labyrinthula. J. Protozool. 14, 697–708. doi: 10.1111/j.1550-7408.1967.tb02065.x
Ralph, P., and Short, F. (2002). Impact of the wasting disease pathogen, Labyrinthula zosterae, on the photobiology of eelgrass Zostera marina. Mar. Ecol. Prog. Ser. 226, 265–271. doi: 10.3354/meps226265
Rappazzo, B. H., Eisenlord, M. E., Graham, O. J., Aoki, L. R., Dawkins, P. D., Harvell, C. D., et al. (2021). EeLISA: Combating global warming through the rapid analysis of eelgrass wasting disease. Proc. AAAI Confer. Artif. Intel. 35, 15156–15165.
Rasmussen, E. (1977). “The wasting disease of eelgrass (Zostera marina) and its effects on environmental factors and fauna,” in Seagrass Ecosystems: A Scientific Perspective, eds C. P. McRoy and C. Helfferich (New York: Marcel Dekker), 1–52.
Renn, C. (1936). The wasting disease of Zostera marina: A phytological investigation of the diseased plant. Biol. Bull. 70, 148–158. doi: 10.2307/1537320
Röhr, M. E., Holmer, M., Baum, J. K., Björk, M., Boyer, K., Chin, D., et al. (2018). Blue carbon storage capacity of temperate eelgrass (Zostera marina) meadows. Glob. Biogeochem. Cycles 32, 1457–1475. doi: 10.1029/2018GB005941
Sand-Jensen, K. (1975). Biomass, net production, and growth dynamics in an eelgrass (Zostera marina L.) population in Vellerup Vig, Denmark. Ophelia 14, 185–201. doi: 10.1080/00785236.1975.10422501
Short, F. T., and Duarte, C. M. (2001). “Methods for the measurement of seagrass growth and production in Global Seagrass Research Methods. Amsterdam: Elsevier, 155–182. doi: 10.1016/B978-044450891-1/50009-8
Short, F. T., Kosten, S., Morgan, P. A., Malone, S., and Moore, G. E. (2016). Impacts of climate change on submerged and emergent wetland plants. Aqua. Bot. 135, 3–17. doi: 10.1016/j.aquabot.2016.06.006
Short, F. T., Mathieson, A., and Nelson, J. (1986). Recurrence of the eelgrass wasting disease at the border of New Hampshire and Maine. USA Mar. Ecol. Prog. Ser. 29, 89–92. doi: 10.3354/meps029089
Short, F. T., Muehlstein, L. K., and Porter, D. (1987). Eelgrass wasting disease: Cause and recurrence of a marine epidemic. Biol. Bull. 173, 557–562. doi: 10.2307/1541701
Stauffer, R. C. (1937). Changes in the invertebrate community of a lagoon after disappearance of the eel grass. Ecology 18, 427–431. doi: 10.2307/1931212
Sullivan, B. K., Sherman, T. D., Damare, V. S., Lilje, O., and Gleason, F. H. (2013). Potential roles of Labyrinthula spp. in global seagrass population declines. Fung. Ecol. 6, 328–338. doi: 10.1016/j.funeco.2013.06.004
Sullivan, B. K., Trevathan-Tackett, S. M., Neuhauser, S., and Govers, L. L. (2018). Review: Host-pathogen dynamics of seagrass diseases under future global change. Mar. Poll. Bull. 134, 75–88. doi: 10.1016/j.marpolbul.2017.09.030
Vergeer, L. H. T., Aarts, T. L., and de Groot, J. D. (1995). The ‘wasting disease’ and the effect of abiotic factors (light intensity, temperature, salinity) and infection with Labyrinthula zosterae on the phenolic content of Zostera marina shoots. Aquat. Bot. 52, 35–44. doi: 10.1016/0304-3770(95)00480-N
Waycott, M., Duarte, C. M., Carruthers, T. J. B., Orth, R. J., Dennison, W. C., Olyarnik, S., et al. (2009). Accelerating loss of seagrasses across the globe threatens coastal ecosystems. Proc. Nat. Acad. Sci. 106, 12377–12381. doi: 10.1073/pnas.0905620106
Keywords: Zostera marina, Labyrinthula zosterae, specific productivity, non-structural carbon, climate change
Citation: Graham OJ, Aoki LR, Stephens T, Stokes J, Dayal S, Rappazzo B, Gomes CP and Harvell CD (2021) Effects of Seagrass Wasting Disease on Eelgrass Growth and Belowground Sugar in Natural Meadows. Front. Mar. Sci. 8:768668. doi: 10.3389/fmars.2021.768668
Received: 01 September 2021; Accepted: 21 October 2021;
Published: 10 November 2021.
Edited by:
Stacey Marie Trevathan-Tackett, Deakin University, AustraliaReviewed by:
Janina Brakel, Scottish Association for Marine Science, United KingdomLuísa Magalhães, University of Aveiro, Portugal
Forest Schenck, Massachusetts Division of Marine Fisheries, United States
Copyright © 2021 Graham, Aoki, Stephens, Stokes, Dayal, Rappazzo, Gomes and Harvell. This is an open-access article distributed under the terms of the Creative Commons Attribution License (CC BY). The use, distribution or reproduction in other forums is permitted, provided the original author(s) and the copyright owner(s) are credited and that the original publication in this journal is cited, in accordance with accepted academic practice. No use, distribution or reproduction is permitted which does not comply with these terms.
*Correspondence: Olivia J. Graham, b2pnNUBjb3JuZWxsLmVkdQ==
†These authors have contributed equally to this work and share first authorship