- Department of Biological and Marine Sciences, University of Hull, Hull, United Kingdom
Studies on pH stress in marine animals typically focus on direct or species-specific aspects. We here test the hypothesis that a drop to pH = 7.6 indirectly affects the intra- and interspecific interactions of benthic invertebrates by means of chemical communication. We recorded fitness-relevant behaviours of small hermit crabs Diogenes pugilator, green shore crabs Carcinus maenas, and harbour ragworms Hediste diversicolor in response to short-term pH drop, and to putative stress metabolites released by conspecifics or gilt-head sea bream Sparus aurata during 30 min of acute pH drop. Not only did acute pH drop itself impair time to find a food cue in small hermit crabs and burrowing in harbour ragworms, but similar effects were observed under exposure to pH drop-induced stress metabolites. Stress metabolites from S. aurata, but not its regular control metabolites, also induced avoidance responses in all recipient species. Here, we confirm that a short-term abrupt pH drop, an abiotic stressor, has the capacity to trigger the release of metabolites which induce behavioural responses in conspecific and heterospecific individuals, which can be interpreted as a behavioural cost. Our findings that stress responses can be indirectly propagated through means of chemical communication warrant further research to confirm the effect size of the behavioural impairments caused by stress metabolites and to characterise their chemical nature.
Introduction
Compared to the open ocean, coastal areas, and particularly intertidal zones are highly variable environments characterised by abrupt changes in water parameters. This includes fluctuations in pH beyond 0.3 units, the levels predicted for average change related to the process of ocean acidification toward the end of the century (Caldeira and Wickett, 2003; Sabine et al., 2004; IPCC, 2019). While this may mean that intertidal species are more resilient to climate change due to their acquired tolerances of pH fluctuations, it also forces them to live more frequently near their physiological tolerance limits (Truchot and Duhamel-Jouve, 1980; Hofmann et al., 2011; Sokolova, 2018; Wolfe et al., 2020). Superimposing ocean acidification on natural pH fluctuations may further increase the variability of organisms’ responses (Eriander et al., 2015). Therefore, organisms inhabiting intertidal areas are interesting models to study the effects of short-term pH fluctuations within the IPCC predicted range. A low environmental pH can directly alter animal behaviour through several pathways, which include (i) deviation of energy budgets toward the stress response (Pörtner, 2008), (ii) fleeing to avoid the sources of stress (Pörtner and Peck, 2010; Abreu et al., 2016), (iii) disrupted information detection and processing leading to impaired decision-making (Briffa et al., 2012; Porteus et al., 2018; Thomas et al., 2020; Cothran et al., 2021), and (iv) alteration of the chemical signals themselves impacting the sensory environment and the transfer of information (Wyatt et al., 2014; Roggatz et al., 2016, 2019). Behavioural effects triggered by lowered pH are known to occur in different taxonomic groups such as crustaceans (de la Haye et al., 2011), marine ragworms (Bond, 2018), and fish (Munday et al., 2009), although recent research debates both their ubiquitousness and effect size (Clark et al., 2020a; Clements et al., 2020a,b, but see Clark et al., 2020b; Munday et al., 2020; Williamson et al., 2021 for the importance of which comparisons are made). In addition, it should be mentioned that although there is little literature disentangling the behavioural effects of pH drop vs. high CO2, an increasing body of recent research evidenced the role of pH-dependent altered chemical communication (Roggatz et al., 2016; Schirrmacher et al., 2020; Porteus et al., 2021; Velez et al., 2021).
In aquatic environments, where visibility can be limited, infochemicals and chemosensory functions, such as pheromones used for mating (Karlson and Lüscher, 1959) are crucial for communication (Hardege, 1999; Jordão and Volpato, 2000; Ashur et al., 2017; Saha et al., 2019). However, infochemicals are also a mechanism to signal stress—but stress communication has mostly been researched within the context of biotic stressors. For example, alarm cues released following mechanical damage from fish epidermal club cells can trigger panic reactions in conspecifics and heterospecifics (Toa et al., 2004; Júnior et al., 2010). Such mechanisms of chemical communication also occur in invertebrates, as evidenced by the reduced out-of-burrow activity of the marine polychaete Alitta virens exposed to damaged conspecifics (Watson et al., 2005; Ende et al., 2017). Disturbance cues refer to chemicals that may be stored in gill epithelium or urine, and are released voluntarily or involuntarily by disturbed or stressed prey, to induce early antipredator responses in recipients to anticipate potential threats (Bairos-Novak et al., 2017; Goldman et al., 2020a). The central role of chemical communication in the aquatic environment and the recent evidence of its impairment by ocean acidification pinpoints the need for a deeper understanding of such potential environmental modulation of chemical signalling (Chivers et al., 2013).
Overall, though, it is not well understood yet whether abiotic stressors such as pH drop can also induce the release of chemical cues and whether these can propagate the stress response to other individuals. For such cues, we here introduce the term “stress metabolites” as defining such compounds released, voluntarily or not, by an organism in response to abiotic stressors. These can be detected and processed by conspecifics and/or heterospecifics, leading to the induction of a behavioural stress response (Hazlett, 1985). Of note, Draper and Weissburg (2019) defined the “direct effects” of elevated CO2 on predator-prey interactions as the effects modifying the sensory pathways (cue production and emission by the donor and cue reception and processing by the receiver). Here, we term the effects of acute pH drop as “direct” when the animal is exposed to the stressor itself and as “indirect” when the animal is exposed to the stress metabolites released upon pH stress. Both direct and indirect effects may be guided by sensory pathways as well as metabolism in senders and receivers (as defined in Draper and Weissburg, 2019). We term “stress propagation” the signalling of stress responses within or between species by means of stress metabolites (Figure 1A). Detecting stress metabolites may allow other individuals to modify their behaviour to avoid a change in the abiotic environment, and communities to coordinate or potentiate their response to ensure survival (Mothersill et al., 2007; Giacomini et al., 2015; Abreu et al., 2016). However, to investigate the function of any pH drop-induced chemical cues, the original stressor (pH drop) must first be removed from the experimental design.
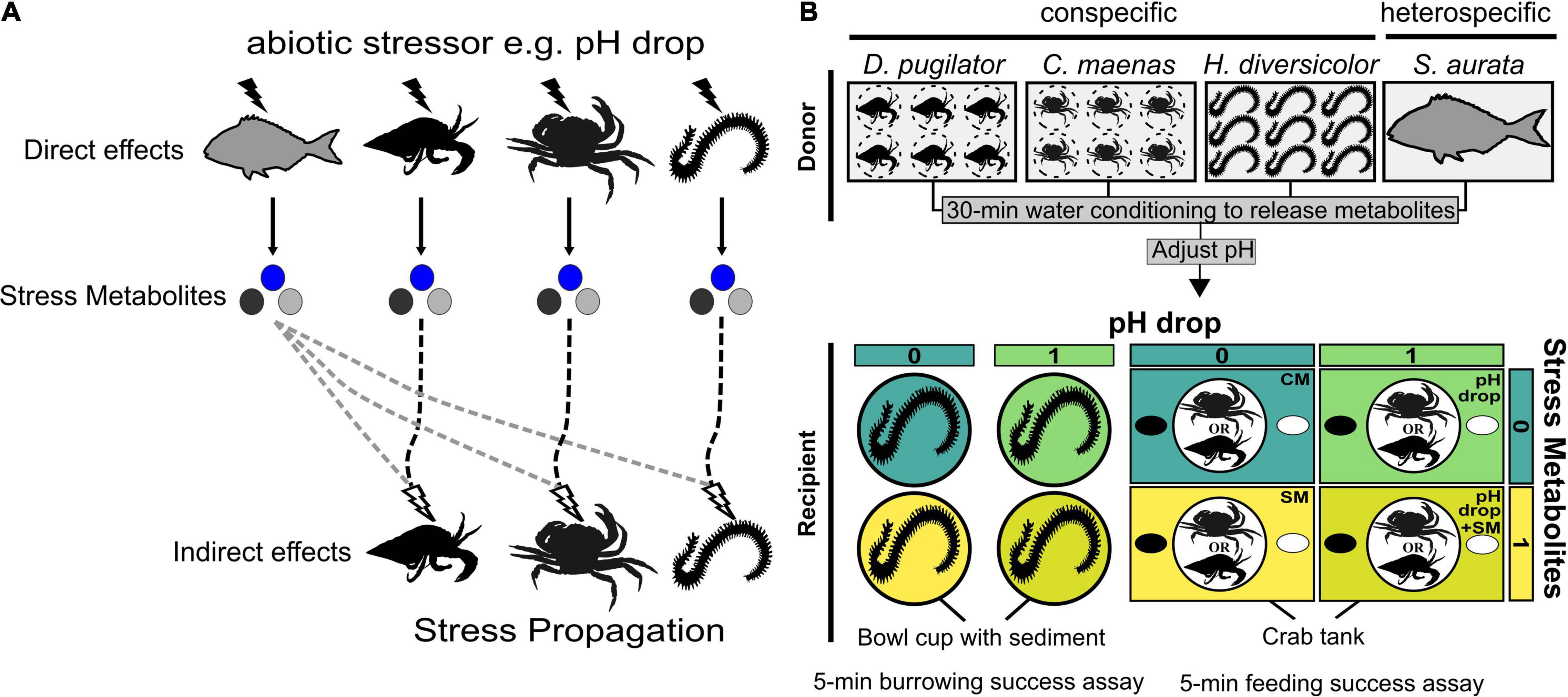
Figure 1. (A) Concept of stress propagation from pH-stressed donors to naive receivers, themselves stressed by metabolites released by the donors. Solid lines and black flash symbols represent direct pH drop effects. Dashed lines and white flash symbols represent indirect pH drop effects mediated by stress metabolites (circles) released by conspecifics (black lines) or heterospecifics (grey lines). (B) Schematic experimental design showing the presence vs. absence of predictors (pH drop, stress metabolites, donor). Behavioural effects of short-term acute pH drop and stress metabolites it induced were tested in three species: Diogenes pugilator, Carcinus maenas, and Hediste diversicolor. Metabolites were obtained by conditioning donors in either control pH = 8.1 (releasing putative control metabolites), or pH drop = 7.6 (releasing putative stress metabolites), followed by pH adjustments for factorial design yielding four experimental conditions CM, pH drop, SM, and pH drop + SM. Predictors are binary coded as 0 (control metabolites, control pH) and 1 (stress metabolites, pH drop). Metabolites originated from conspecifics or the heterospecific Sparus aurata. Behaviour assays for crabs consisted in locating a feeding cue in 300 s. Specimens of H. diversicolor were placed on top of sediment and burrowing behaviour was recorded for 300 s. A range of avoidance behaviours were also recorded. Experimental conditions were CM, control metabolites in control pH; SM, control metabolites in control pH; pH drop, control metabolites in pH drop; pH drop + SM, stress metabolites in pH drop. Animal drawings by A. Murcia and KCWV.
In this study, we investigate, within and among species inhabiting the intertidal zone, the indirect effects of acute pH drop through stress metabolites induced by it on fitness-relevant behaviours. Using a full factorial design, we test the hypothesis that an acute pH drop to end-of-century level (7.6) will both directly (i.e., when the animal is exposed to the abiotic stressor) and indirectly (i.e., when the animal is exposed to the stress metabolites) affect behaviour through the induction of stress metabolites. We observe three marine invertebrates (small hermit crab Diogenes pugilator, green shore crab Carcinus maenas, and harbour ragworm Hediste diversicolor) exposed to control pH and pH drop in combination with conditioned water from both conspecifics and their potential vertebrate predator, the gilt-head sea bream S. aurata.
Materials and Methods
Experimental Design
Small hermit crabs Diogenes pugilator and green shore crabs Carcinus maenas were collected in autumn at low tide in the Ria Formosa lagoon (Portugal). Harbour ragworms (Hediste diversicolor) were sourced via a local supplier (Valbaits) from the Setubal lagoon (Portugal). Animals were acclimated for 1 week at pH 8.1 (pH is reported using National Bureau of Standards scale) in large flow-through open-circuit communal tanks (continuous flow of 0.05 L/min) mimicking natural habitats at the Ramalhete Marine Station (CCMAR, Faro, Portugal) in a direct CO2-controlled system (described in Sordo et al., 2016, 2018; Gregório et al., 2019) with pCO2 constantly measured and adjusted. Two system seawater conditions were used, either control pH of 8.1 ± 0.015 or pH drop of 7.6 ± 0.008. For a measured total alkalinity of 2,500 μmol/kg SW, the pCO2 was calculated as 537 and 1,922 μatm in the control and pH drop system water, respectively, according to the CO2calc software (v1.2.0, Robbins et al., 2010). pCO2 was not estimated in any treatment containing reef buffer, HCl, or dilutions, as total alkalinity was not measured in those, and pCO2 could therefore have differed from that in the system water (see Supplementary Information for details). Seawater parameters were measured daily at 2.30 pm (mean temperature: 20.16°C ± 1.05°C, mean salinity: 35.67 PSU ± 0.26 PSU, mean dissolved oxygen: 7.55 mg/L ± 0.16 mg/L). A total pool of approx. 100–120 D. pugilator, 150–200 C. maenas, and over 150 H. diversicolor was used during the experimental period, yielding a total of 921 observations. Because no individual marking could be achieved, we aimed to space out the reuse of animals in successive behavioural assays by circulating animals through recovery tanks afterward, before returning them to their stock population tanks once a day. Animal reuse was randomised across treatments and treatments randomised per day to prevent any confounding effects on the measured behaviours. All experiments were conducted in October 2019, except one independent set of observations (n = 40) in C. maenas conducted in September 2018, which was analysed together with the 2019 data with the year treated as a covariate.
A three-way factorial design of pH drop × stress metabolites × donor species was generated to study the effects of acute pH drop, stress metabolites induced by it, and their combination vs. a control containing regular metabolites (control metabolites) (Figure 1B). Metabolite donor species were D. pugilator, C. maenas, H. diversicolor (called conspecific donors), and the potential predator Sparus aurata (called heterospecific donor). Metabolite donors were conditioned for 30 min in seawater at regular pH (pH 8.1, 538 μatm CO2, putatively inducing control metabolite release), or pH drop (pH 7.6, 1,922 μatm CO2, putatively inducing stress metabolite release). Recipient species were D. pugilator, C. maenas, and H. diversicolor which received water containing either conspecific or heterospecific metabolites. To achieve the full factorial design, recipient species received either control or stress metabolites and were tested in either control pH (8.1) or pH drop (7.6), by re-adjusting the pH of the conditioned water before each bioassay (with the pH verified with an accuracy of 0.02 units using a portable pH metre). Overall, four different experimental treatments were obtained: (i) putative control metabolites at pH 8.1 (CM), (ii) putative control metabolites at pH 7.6 (pH drop), (iii) putative stress metabolites at pH 8.1 (SM), (iv) and putative stress metabolites at pH 7.6 (pH drop + SM). Testing conspecific and heterospecific metabolites with three recipient species amounted to a total of 12 treatments. Prior to use in recipient species, conditioned seawater from H. diversicolor and S. aurata (0.0067 fish/L) was 10-fold diluted in fresh system seawater at the desired pH. To explore the possibility that stress metabolites are equivalent to highly concentrated control metabolites, we additionally tested the effects of undiluted S. aurata control metabolites at pH 8.1 in H. diversicolor and D. pugilator. See Supplementary Information for further details on treatments (Supplementary Table 1) and water conditioning (Supplementary Methods).
Behavioural Bioassays
For each of the four conditions, we measured the time to find a feeding cue (1/10 diluted mussel juice) vs. a mock (seawater) cue in D. pugilator and C. maenas, or to bury the head entirely in the sand in H. diversicolor, and dubbed this variable “time-to-success.” Behavioural assays were terminated once the animal successfully grabbed the ballasted sponge containing the feeding cue with their pincers (D. pugilator and C. maenas) or buried its entire head (H. diversicolor), or at a maximum time of 300 s. Both feeding and burrowing behaviours are tested response variables in crabs (de la Haye et al., 2012; Wang et al., 2018) and ragworm (Bhuiyan et al., 2021) exposed to pH drop. Additionally, avoidance behaviour was binary coded. In D. pugilator and C. maenas, these avoidance behaviours included freezing (suddenly retreating into the shell or attempting to burrow, sudden and lasting arrest in locomotion for at least 120 consecutive seconds or more than 150 s in total), and escaping (walking along the walls or retreating into a corner of the tank for at least 120 consecutive seconds or more than 150 s in total). Such freeze and escape behaviours indicate danger in crustaceans (Katz and Rittschof, 1993; Perrot-Minnot et al., 2017; Tomsic et al., 2017). In H. diversicolor, avoidance behaviours consisted of freezing, which might be accompanied by spread of jaws, sideways-undulating behaviour, and formation of a noticeable slime cap whilst outside the sediment. These behaviours indicate extreme stress in marine polychaetes (Mouneyrac et al., 2003; Burlinson and Lawrence, 2007; McBriarty et al., 2018). The feeding cues were randomly assigned left or right to the crabs. See Supplementary Methods for further details on behavioural assays. All data is given as Supplementary Dataset.
Statistical Analysis
Avoidance behaviours were analysed using generalised linear models for logit regression with binomial distribution using the stats R package (R Core Team, 2020). Time-to-success was modelled for the time to reach a feeding cue in D. pugilator and C. maenas or to burrow the head in H. diversicolor depending on the three predictors: pH drop, stress metabolites, and donor. Time-to-success data was analysed with a time-to-event analysis (also called survival analysis) using Cox proportional hazard models from the survival R package (Therneau and Grambsch, 2000; Therneau, 2021). The event being the success of food cue location (in crabs) or burrowing the head in sediment (in worms), the analysis was hereafter referred to as “time-to-success analysis.” The exponentiated estimates (hazard ratios) from the Cox proportional hazard models were expressed as “success ratio.” Success ratios were visualised using the plot_model function from the sjPlot R package (Lüdecke, 2021). The “success probability” (the probability of the feeding or burrowing event occurring at any time over 300 s) over time was represented by Kaplan-Meier curves drawn using the survminer R package (Kassambara et al., 2020). Animals not reaching the feeding cue or not burying their head were censored and assigned the maximum time of “300 +”.
All statistical models always included the main effects and interaction terms of the binary predictors: pH drop (pH = 8.1 vs. pH = 7.6), stress metabolites (stress metabolites vs. control metabolites), and donor (conspecific vs. heterospecific). Covariates (year, number of water uses, crab size, where relevant; see section 2.2 in Supplementary Information) were excluded from models when deemed insignificant compared to the null model by at least two model selection methods among the following: an ANOVA Chi-square test from the stats R package (R Core Team, 2020), the Akaike Information Criterion (ΔAIC), and Bayesian Information Criterion (ΔBIC) from the AICcmodavg R package (Mazerolle, 2020). Overall model fit p-values were retrieved from the ANOVA Chi-squared test or the Likelihood ratio test for generalised linear models and Cox-proportional models, respectively. Pairwise comparisons between treatments involved in the interaction term between the three predictors were obtained from statistical models using the emmeans R package (Lenth, 2021), wherein false discovery rate p-value adjustments were applied for post hoc term-wide multiple testing.
To investigate the possibility that stress metabolites are equivalent to highly concentrated control metabolites (which were present in CM and pH drop treatments but in 10-fold dilution), additional tests compared the behavioural response of D. pugilator and H. diversicolor to 10-fold diluted stress metabolites and control metabolites to that of undiluted control metabolites from S. aurata (see Supplementary Information, section 2.4). The full dataset may include animals tested at most twice over the course of the experimental period. We therefore performed random bootstrapping of 50% of the dataset (Supplementary Information, section 2.5). All statistical analyses were conducted in RStudio (R Studio Team, 2020) with a significance threshold of P ≤ 0.05. See R script and Supplementary Information for further details.
Results
Response of Small Hermit Crab Diogenes pugilator to pH, Stress Metabolite, and Donor Predictors
The time-to-success analysis was not conclusive for the stress metabolite predictor in D. pugilator (Figure 2A and Table 1, Z = −0.66, P = 0.5073). On the other hand, the Cox proportional hazard model (overall Likelihood ratio test model fit: P = 0.06) found that both pH drop (feeding success ratio = 0.47, Z = −2.14, P = 0.0325) and metabolites from S. aurata (feeding success ratio = 0.35, Z = −2.76, P = 0.0058) had significant negative effects on the feeding success ratio of D. pugilator. In addition, there was a significant interaction between pH drop and metabolite donor terms on the feeding success of D. pugilator (Z = 2.19, P = 0.0289). Overall, pH drop induced a significantly lower success (risk) score in the conspecific treatment (Figure 2B). Post hoc analyses evidenced that all treatments (CM, pH drop, SM, and pH drop + SM) induced similar feeding time responses in both the conspecific and heterospecific donor groups (Supplementary Table 2).
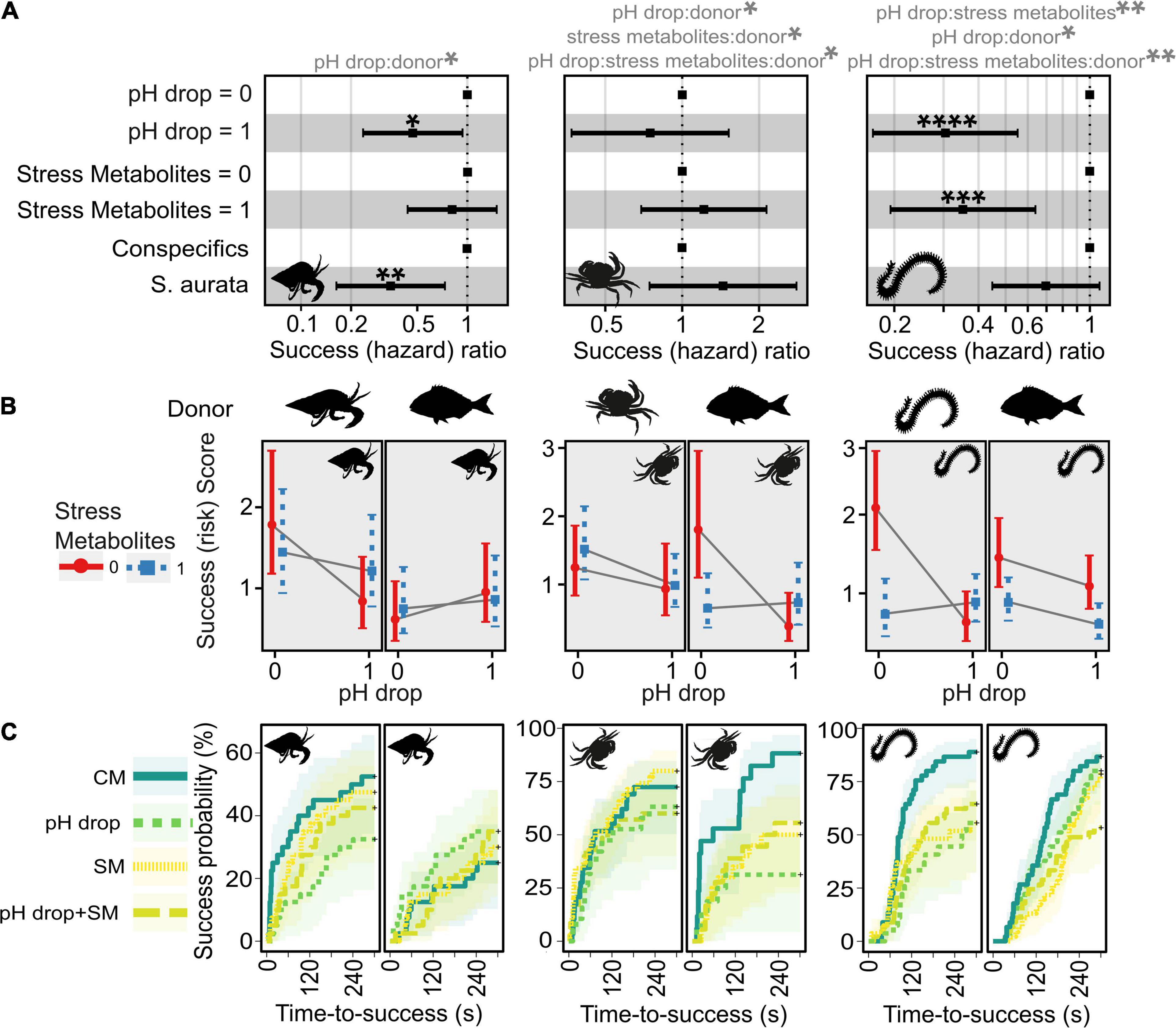
Figure 2. Effects of predictors (pH drop, stress metabolites, donor) on time-to-success in Diogenes pugilator, Carcinus maenas, and Hediste diversicolor. (A) Effects of predictors on success ratio (aka hazard ratio with success as event, arbitrary units). Likelihood ratio tests for overall model fit were: (D. pugilator: P = 0.06; C. maenas: P = 0.009; H. diversicolor: P < 0.0001). Significant predictors from Cox proportional hazard models are shown with asterisks, and significant interaction terms are shown in grey above the plots. (B) Interaction term plot of marginal effects showing predicted success score (aka risk score with success as event, arbitrary units ± confidence interval) split by donor and recipient species. Crossed solid grey lines represent an interacting effect of pH and metabolites. (C) Kaplan-Meier curves to visualise success probability (cumulative event) for each experimental condition over time. *P ≤ 0.05, **P < 0.01, ***P < 0.001, ****P < 0.0001. Experimental treatments were: CM, control metabolites in control pH; pH drop, control metabolites in pH drop; SM, stress metabolites in control pH; pH drop + SM, stress metabolites in pH drop.
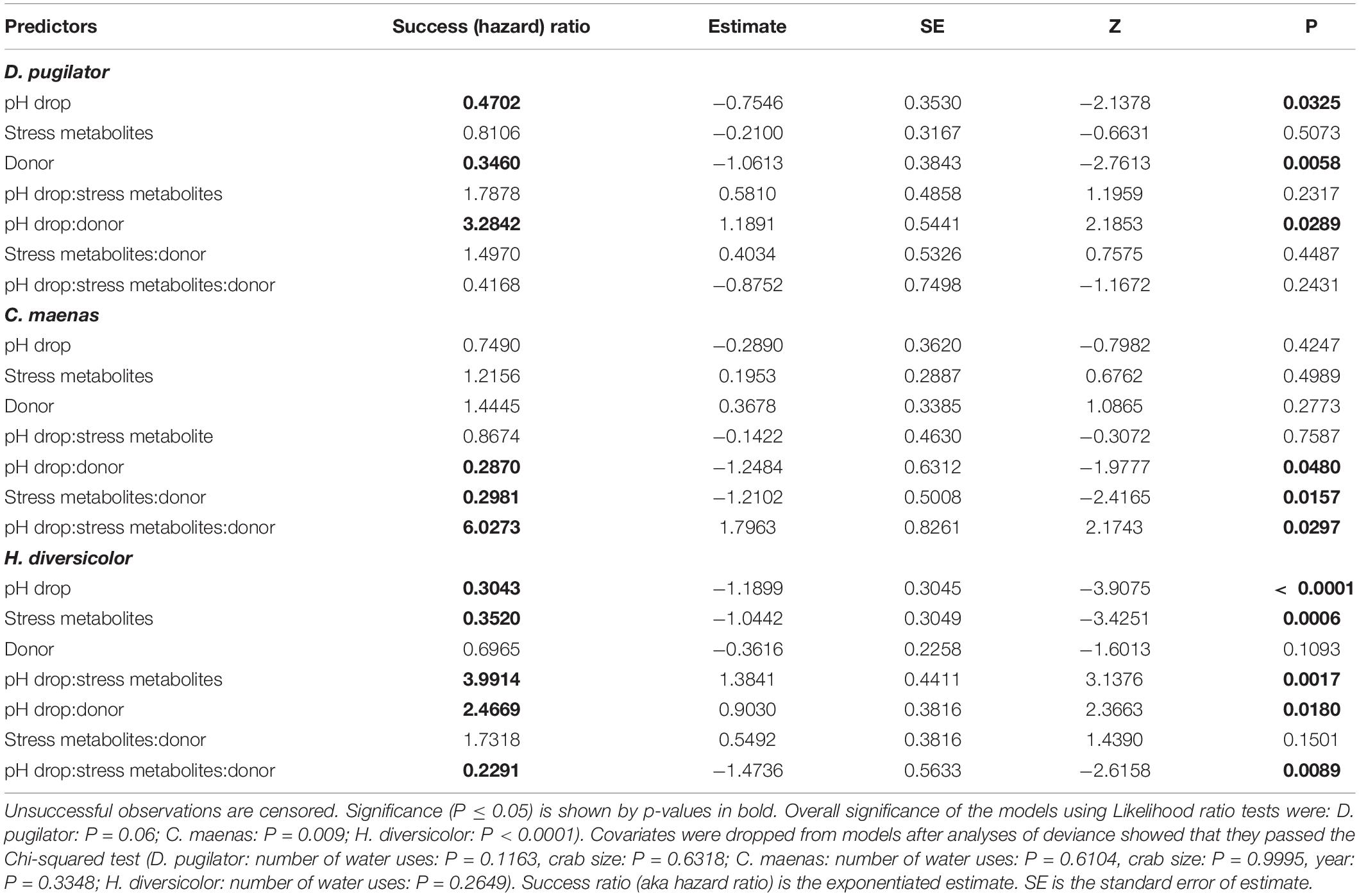
Table 1. Results of Cox proportional hazard model for the main effects of predictors (pH drop, stress metabolites, donor) on the time-to-success analysis in small hermit crab (Diogenes pugilator, n = 320 observations with 120 events of finding a food cue, degrees of freedom = 312), green shore crab (Carcinus maenas, n = 189 observations with 122 events of finding a food cue, degrees of freedom = 181), and harbour ragworm (Hediste diversicolor, n = 325 observations with 234 events of burrowing head in sediment, degrees of freedom = 317).
Avoidance responses of D. pugilator did not depend on pH drop (Z = 0.25, P = 0.8049) nor on stress metabolites (Z = −0.25, P = 0.7995, overall Chi-square test model fit: P < 0.0001, Table 2), but were significantly more pronounced when metabolites originated from S. aurata (69%) instead of conspecifics (26%, Z = 2.46, P = 0.0139, Figure 3 and Table 2). After splitting the display of avoidance behaviour by donor, pairwise comparisons failed to find differences between the four treatments (CM, pH drop, SM, and pH drop + SM), but confirmed that the donor effect existed across all four treatments (Supplementary Table 3 and Figure 3). However, partitioning the avoidance behaviour of D. pugilator into freezing and escaping responses evidenced that escaping significantly increased with S. aurata control metabolites only when tested in pH drop (Supplementary Figures 1, 2 and Supplementary Tables 4–7, see Supplementary Results).
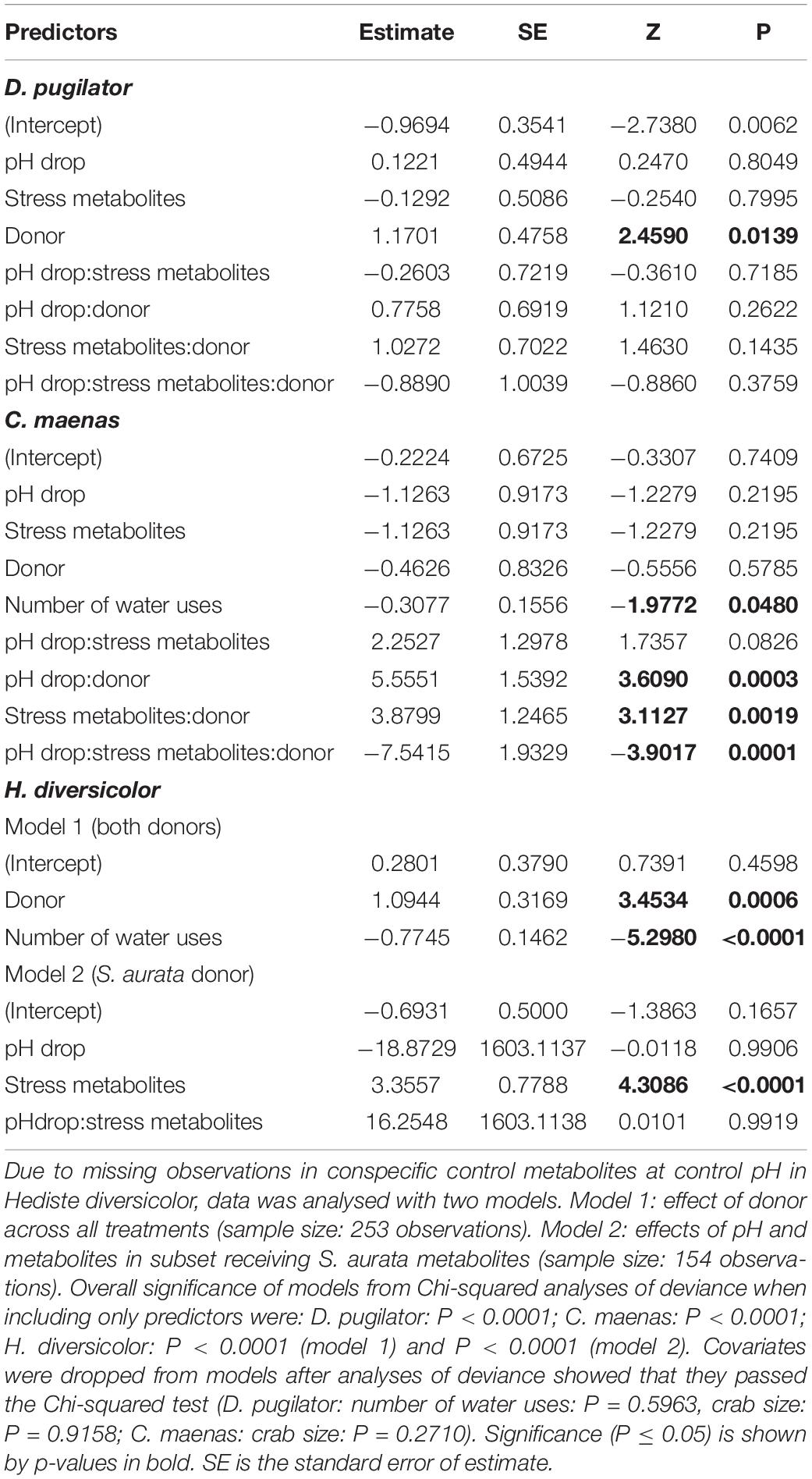
Table 2. Results of the binomial generalised linear model for the main effects of predictors (pH drop, stress metabolites, donor) on the avoidance behaviour in small hermit crab (Diogenes pugilator, n = 320 observations of finding a food cue, degrees of freedom = 312), green shore crab (Carcinus maenas, n = 151 observations of finding a food cue, degrees of freedom = 142), and harbour ragworm (Hediste diversicolor, n = 253 observations of burrowing head in sediment, degrees of freedom = 251).
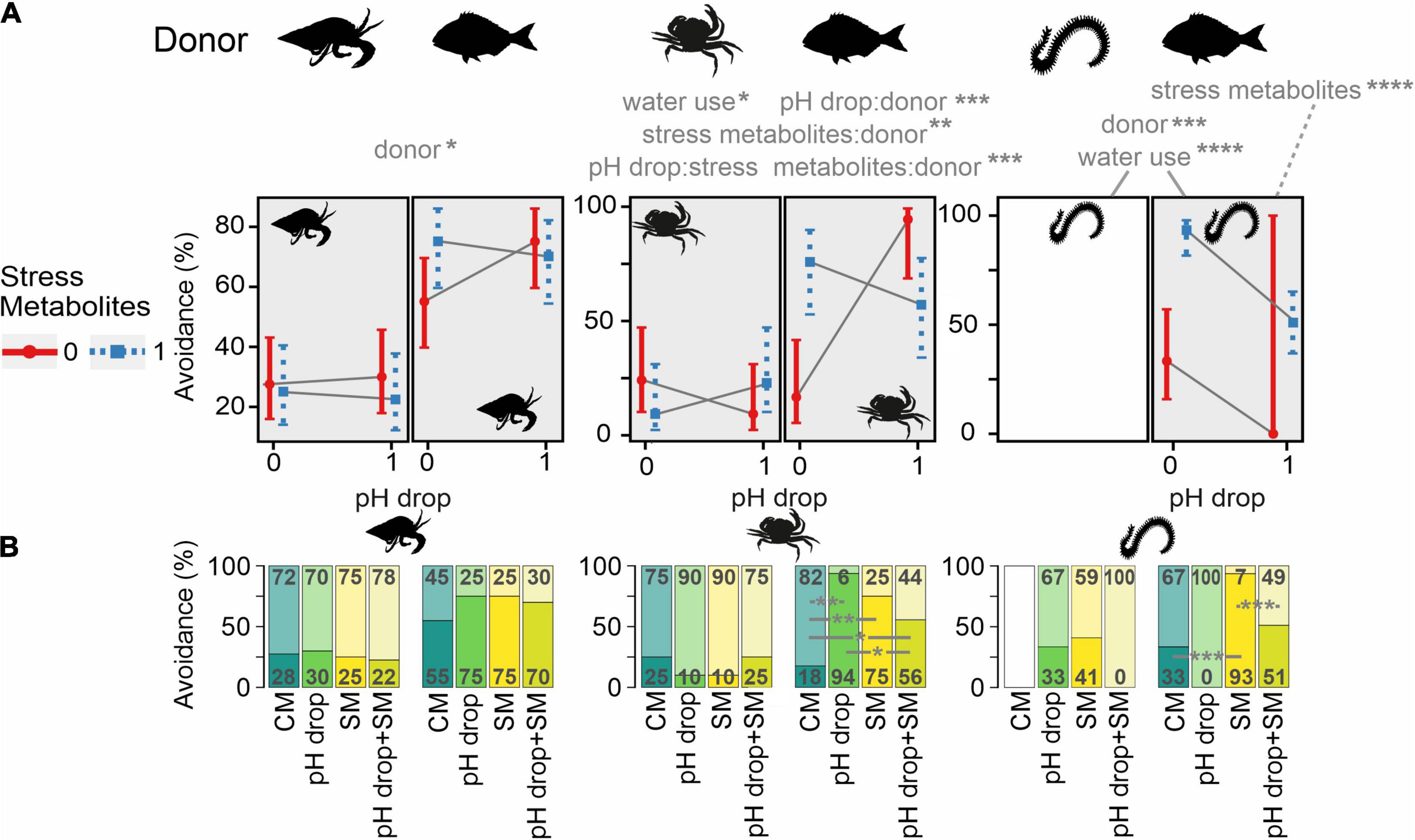
Figure 3. Effects of predictors (pH drop, stress metabolites, donor) on the percentage of avoidance behaviour in Diogenes pugilator, Carcinus maenas, and Hediste diversicolor. Avoidance behaviour included freezing and escaping (D. pugilator and C. maenas), or freezing, curling, flipping, and slime secretion (H. diversicolor). (A) Split bars represent the presence (dark area) or absence (light area) of avoidance behaviours. Significant main predictors (and their interaction terms) and covariates are shown above plots. Significant pairwise comparisons between treatments in each donor/recipient are shown as horizontal grey lines. *P ≤ 0.05, **P < 0.01, ***P < 0.001, ****P < 0.0001. Experimental treatments were: CM, control metabolites in control pH; pH drop, control metabolites in pH drop; SM, stress metabolites in control pH; pH drop + SM, stress metabolites in pH drop. (B) Interaction plot showing the marginal effects on the predicted avoidance percentages (±confidence interval) of stress metabolites and pH drop within the conspecific and heterospecific groups for each species. Crossing solid grey lines represent an interacting effect of pH and metabolites for each donor. The interactive effects of predictors for H. diversicolor/H. diversicolor are not represented due to the missing CM treatment. Overall effects of donor and water use across the response of H. diversicolor (for either donor) are shown with solid lines, whereas the effects of stress metabolites are for S. aurata/H. diversicolor only (grey dashed line).
Response of Green Shore Crab Carcinus maenas to pH, Stress Metabolite, and Donor Predictors
The Cox proportional hazard model (overall Likelihood ratio test model fit: P = 0.009) showed that the feeding success ratio of C. maenas did not significantly vary with pH (Z = −0.80 P = 0.4247), metabolites (Z = 0.68, P = 0.4989), nor donor (Z = 1.09, P = 0.2773) terms (Figure 2 and Table 1). Nevertheless, the interaction terms were significant. Post hoc tests revealed that time-to-success responses were similar across treatments CM, pH drop, SM, and pH drop + SM when C. maenas received conspecific metabolites (Supplementary Table 8). This pattern changed when S. aurata was the metabolite donor as evidenced by significantly lower feeding success scores in C. maenas exposed to pH drop (Z = 2.97, P = 0.0179), SM (Z = 2.48, P = 0.0394), and the trend for pH drop + SM (Z = 2.20, P = 0.0563), compared to the control CM.
In C. maenas, the predictors pH drop (Z = −1.23, P = 0.2195), stress metabolites (Z = −1.23, P = 0.2195), and donor (Z = −0.56, P = 0.5785) did not alter avoidance responses but their interaction terms were significant (overall Chi-square test model fit: P < 0.0001, Table 2 and Figure 3). Pairwise comparisons showed no differences between the four treatments (CM, pH drop, SM, pH drop + SM) in avoidance patterns of C. maenas receiving conspecific metabolites. Conversely, C. maenas facing S. aurata metabolites while experiencing treatments of pH drop (94%, Z = −3.60, P = 0.0020), SM (75%, Z = −3.28, P = 0.0031), and pH drop + SM (56%, Z = −2.33, P = 0.04) significantly increased their avoidance display compared to control CM (18%). Moreover, pH drop combined with stress metabolites (pH drop + SM treatment) instead of control metabolites (pH drop treatment) significantly lowered avoidance behaviours (Z = 2.21, P = 0.0405, Supplementary Table 9 and Figure 3). Partitioning the avoidance behaviour of C. maenas into freezing and escaping responses evidenced that escaping explained the avoidance response whereas no clear patterns were observed in the freezing behaviour (Supplementary Figures 1, 2 and Supplementary Tables 10–13, see Supplementary Results).
Response of Harbour Ragworm Hediste diversicolor to pH, Stress Metabolite, and Donor Predictors
Burrowing success responses of H. diversicolor did not depend on the donor species (P = 0.1093, Figure 2 and Table 1). However, the Cox proportional hazard model (overall Likelihood ratio test model fit: P < 0.0001) evidenced that both pH drop (burrowing success ratio = 0.30, Z = −3.91, P < 0.0001) and stress metabolites (burrowing success ratio = 0.35, Z = −3.42, P = 0.0006) terms significantly altered burrowing time responses of H. diversicolor. Significant interactions between the main predictors required to decipher the involvement of within-donor treatment effects (Supplementary Table 14). Post hoc tests revealed that pH drop (Z = 3.91, P = 0.0006), SM (Z = 3.43, P = 0.0012), and pH drop + SM (Z = 3.47, P = 0.0012) treatments significantly lowered burrowing success scores compared to the control CM in H. diversicolor exposed to conspecific metabolites. Moreover, H. diversicolor facing S. aurata metabolites had lower burrowing success scores in the pH drop + SM treatment compared to the control CM (Z = 3.35, P = 0.0049).
H. diversicolor displayed more avoidance in response to S. aurata metabolites (47%) compared to conspecific metabolites (20%, Z = 1.09, P = 0.0006, overall Chi-square test model fit: P < 0.0001, Table 2 and Figure 3). H. diversicolor exposed to S. aurata metabolites were not affected by pH drop (Z = −0.01, P = 0.9906) whereas stress metabolites induced significantly more avoidance than control metabolites (Z = 4.31, P < 0.0001, overall Chi-square test model fit: P < 0.0001, Table 2). There were no differences across pH drop, SM, and pH drop + SM treatments in H. diversicolor receiving conspecific metabolites (Supplementary Table 15). Pairwise comparisons revealed that the SM treatment (93%) triggered significantly more avoidance than CM (33%, Z = −4.31, P = 0.0001) and pH drop + SM treatments (51%, Z = 3.92, P = 0.0003). Specimens of H. diversicolor exposed to S. aurata metabolites displayed different avoidance responses in the control treatment CM (Supplementary Video 1) compared to pH drop (slowed-down burrowing response, Supplementary Video 2), SM (sideways-undulating behaviour with body flips, Supplementary Video 3), and pH drop + SM (raised head and spread mouth parts with freezing behaviour in Supplementary Video 4 and slowed-down movement with sideways undulating behaviour in Supplementary Video 5).
Importantly, the behavioural responses of H. diversicolor and D. pugilator remained unchanged even when we used undiluted control metabolites (see Supplementary Information, section 2.4, Supplementary Figure 3 and Supplementary Tables 16, 17). Of note, the avoidance response of H. diversicolor and D. pugilator faded as water was reused (Supplementary Information, section 2.2, Supplementary Figure 4).
Furthermore, random bootstrapping of 50% of the dataset (Supplementary Information, section 2.5, Supplementary Figure 5 and Supplementary Table 20) did not change the outcome of time-to-event analysis for any species.
Discussion
Direct Effects of pH Drop and Donor Type on Assay Success and Avoidance Behaviours
In this study, we performed over 900 behavioural observations, and our results show species-specific responses, donor-dependent effects, as well as sometimes large within-treatment variability. Sparus aurata-conditioned water, whether it contained control metabolites or pH stress-induced metabolites, elicited avoidance in all recipient species which can be attributed to antipredator behaviours. Despite the donor effect, however, much of the behavioural variance was explained by the effects of pH drop and stress metabolites, their interaction, or their interaction with the donor term (Figure 2).
We found that pH drop lowered success ratios in feeding and burrowing in Diogenes pugilator and Hediste diversicolor, respectively. Such low pH-induced impairments of fitness-relevant behaviours were previously found in hermit crabs Pagurus bernhardus and P. tanneri as well as in the Japanese stone crab Charybdis japonica and may indicate impaired decision-making (de la Haye et al., 2011, 2012; Kim et al., 2015; Wu et al., 2017). Past literature also reported delayed burrowing responses in the harbour ragworm H. diversicolor exposed for 28 days to pH drop (Bhuiyan et al., 2021), and an inverse relationship between burrowing and pH in the king ragworm Alitta virens (Batten and Bamber, 1996). These behavioural alterations may reflect the cost of acid-base regulation to counteract hypercapnia (Pörtner, 2008), as marine ragworms overexpress the acid-base regulator Carbonic Anhydrase (CA) at the expense of energy reserves in response to acidification (Freitas et al., 2016; Wage et al., 2016). In the longer term, behavioural costs of an altered burrowing activity may extend to crucial ecological functions as shown by the diminished bioturbation activity of H. diversicolor in similar conditions (Bond, 2018). In contrast, Carcinus maenas did not delay its feeding response to sudden pH drop in presence of conspecific metabolites, which is in line with meta-analyses showing that some aquatic arthropods are more robust to ocean acidification conditions (Kroeker et al., 2013; Wittmann and Pörtner, 2013; Clements and Darrow, 2018). The resilience to pH stress in C. maenas may be due to their pH regulatory capacities allowing them to maintain feeding during short-term assays, but they may show altered responses in longer-term assays—for instance after 10 weeks—as their energetic requirements increase (Appelhans et al., 2012; Clements and Darrow, 2018). Alternatively, the fact that C. maenas did not respond to pH drop may arise from differences in the type of cues that are presented or that not all behaviours are similarly affected by pH drop (Richardson et al., 2021). Short-term hypercapnia does not seem to be a strong stressor in C. maenas, due to its pH-variable intertidal habitat (Fehsenfeld et al., 2011). Other than reduced success ratios, pH drop itself did not induce any avoidance behaviours indicative of stress.
Besides the direct effect of pH drop on behaviour, previous studies on ocean acidification also found contrasting effects of pH drop on predator-prey interactions (Draper and Weissburg, 2019). Here, we observed an increased escaping tendency of C. maenas in response to S. aurata control metabolites in pH drop but not in control pH. Although this was not the main focus of our study, it could mean that pH drop renders predator odour more potent for its prey, for example through pH-dependent changes in odour molecular structure, receptor binding, or information processing (Munday et al., 2009; Roggatz et al., 2016, 2019; Schirrmacher et al., 2020). Our results contrast with earlier reports of decreased predator avoidance (e.g., in snails; see Jellison et al., 2016; Froehlich and Lord, 2020). Overall, our data suggests that a short-term acute pH drop has the ability to impair decision-making and predator-prey interactions, and in turn alter fitness-relevant behaviours. Nevertheless, future studies should aim to disentangle the effects of pH drop from that of pCO2, which ideally also would test short, medium, and long-term responses.
Indirect Effects of pH Drop on Recipients’ Behaviour Through Stress Chemical Communication
Despite the above-mentioned species-specific direct effects of pH drop on behaviour success ratios, we could show here that pH drop also has indirect effects on behaviour by altering chemical communication. We found that stress metabolites released by animals exposed to pH drop altered feeding and burrowing, and increased avoidance behaviours, but that the response was donor and recipient species-specific. Hermit crabs D. pugilator did not react to any stress metabolites. Significant responses in the other two recipient species, however, were directionally equivalent to those induced by pH drop itself since it took longer to find a food cue or to burrow the head in the presence of stress metabolites. Although behavioural stress response propagation through chemical communication is well documented for alarm substances and disturbance cues upon predation stress (Giacomini et al., 2015; Abreu et al., 2016; Mathuru, 2016), our results show here that chemical communication induced by abiotic stressors such as pH drop can trigger the release of waterborne chemicals that in turn trigger a behavioural stress response in its recipients. Stress metabolites conditioned by the potential predator S. aurata induced more avoidance behaviours and delayed feeding in C. maenas and burrowing in H. diversicolor, compared to S. aurata control metabolites. Similarly, the presence of conspecific stress metabolites significantly lowered H. diversicolor burrowing success ratio compared to conspecific control metabolites.
On the other hand, C. maenas did not react to conspecific stress metabolites. The different behavioural effect of conspecific stress metabolites between crabs and H. diversicolor has several possible explanations ranging from different defence strategies related to stress risk perception and evaluation (Hazlett, 1985; Bairos-Novak et al., 2017, 2018; Goldman et al., 2020b) to different types, concentrations, or ratios of the released chemicals that serve as risk cues (Júnior et al., 2010; Morishita and Barreto, 2011). As we have shown here a pH drop to 7.6 is not a strong stressor in crabs (Fehsenfeld et al., 2011) which may explain that we failed to observe responses to water from pH drop-conditioned conspecific green shore crabs. However, the presence of stress metabolites conditioned by S. aurata caused a marked drop in the success ratio of C. maenas at low (but not control) pH, evidenced by significant interaction terms.
Whilst we did not investigate their chemical structure, our experiment allows us to characterise novel properties of stress metabolites. Stress metabolites, like disturbance cues, potentially consist of regularly excreted metabolites such as urea and ammonia (Bairos-Novak et al., 2017; Shrivastava et al., 2019). However, the fact that behavioural responses of H. diversicolor and D. pugilator remained unchanged even when we used undiluted control metabolites might mean that stress metabolites are not just up-concentrated control metabolites (at least in the tested range of 10-fold control metabolites), or that the cocktail of chemicals contains much higher ratios of stress cues relative to control cues. We also observed that avoidance behaviours by both C. maenas and H. diversicolor became somewhat less pronounced with subsequent water uses, suggesting that the recipients may be able to discriminate degraded vs. fresh metabolites and react accordingly to the degree of threat they may indicate (Fuselier et al., 2009; Bairos-Novak et al., 2018). This shows that stress metabolites induced by pH drop are either not identical with metabolites released in normal pH, and/or are at least more than 10-fold higher concentrated than control metabolites.
The avoidance behaviours we recorded in this study are known indicators of stress. S. aurata stress metabolites elicited atypical freezing, eversion of the proboscis, mucus secretion, flipping and sideways-undulating behaviours in H. diversicolor which were previously described as indicators for a physiological stress response following exposure to copper sulphate (Burlinson and Lawrence, 2007), antiparasitic drugs (McBriarty et al., 2018), and trace metals (Mouneyrac et al., 2003). We also observed increased freeze and escape responses in crabs, which indicate a stress response in crustaceans (Katz and Rittschof, 1993; Perrot-Minnot et al., 2017; Tomsic et al., 2017). The finding that S. aurata stress metabolites induced such responses in potential prey species suggests that responses to disturbance cues are do not depend on the “audience,” similar as what has been observed in tadpoles (Bairos-Novak et al., 2020).
A directionally similar response to pH drop and to the stress metabolites it induces shows that pH drop can have indirect effects through stress propagation (Figure 1A), by which stressed animals directly experiencing environmental stressors negatively influence fitness-relevant behaviours of community members. The stress propagation toward naive conspecific individuals might constitute a “positive stress feedback loop” (Feugere et al., 2021). This was first observed by Hazlett (1985) after freshwater crayfish exposed to water conditioned with heat-stressed conspecifics displayed increased alertness, after returning the conditioned water to normal temperature. Similar indirect effects through chemical signalling from stressed donors toward unstressed, naive recipients exposed to the water conditioned by the directly stressed donors but not to the stressor itself, were also shown in response to different types of biotic stressors such as mock predator chase (Toa et al., 2004; Giacomini et al., 2015), handling (Barcellos et al., 2011), and acute fasting (Abreu et al., 2016), and to physical injury including irradiation (Mothersill et al., 2007) and predation (Frisch, 1938; Mathuru et al., 2012; Oliveira et al., 2013).
Conclusion and Perspectives
In this study, we could show that short-term pH drop of a similar magnitude of that experienced within the intertidal zone, but also aligned to end-of-century predicted average values (Chavez et al., 2017; Landschützer et al., 2018), had negative consequences on fitness-relevant behaviours in harbour ragworm H. diversicolor and small hermit crab D. pugilator. Additionally, we confirm that pH drop events also impede the same behaviours in the same way indirectly via chemical communication, albeit these effects depended on donor and recipient species. Sea bream S. aurata and harbour ragworm H. diversicolor stressed by pH drop released stress metabolites which possibly differ from control metabolites and negatively affect fitness-relevant behaviours in metabolite recipients. Stress metabolites induced similar avoidance behaviours as those exhibited under physiological stress, meaning that a stress response was propagated from donor to recipient. These negative indirect behavioural effects of pH drop warrant further study, especially as our results were inconclusive with regards to the combined treatments of low pH and stress metabolites. Short-term pH drops thus involve behavioural additionally to metabolic trade-offs, which is also of interest for better predicting the response of natural and aquaculture systems under ocean acidification combined with tidal pH fluctuations. One potential limitation of our experimental design was that we could not achieve individual marking of animals. Despite circulating animals to recovery tanks to limit animal reuse, this may have led to a small degree of animal reuse wherein the full dataset may include animals tested at most twice over the course of the experimental period. Nevertheless, we show through random bootstrapping of 50% of the dataset, that the outcomes of the time-to-event analysis remain unchanged even if only half of the observations were analysed, which confirms the validity of our experimental data. Whether or not pseudoreplication is present in ecological studies has been subject to debate (Davies and Gray, 2015), but owing to our large number of observations we can show here that animal reuse had no effect on the model outcomes and effect size was not overestimated. Given the potential high ecological significance of indirect negative effects of pH fluctuations on population fitness, studies aiming to replicate our results should aim to further optimise our experimental design with better technical equipment as that available to us (Baker, 2016).
Data Availability Statement
The original contributions presented in the study are included in the article/Supplementary Material, further inquiries can be directed to the corresponding author/s.
Ethics Statement
The animal study was reviewed and approved by the University of Hull Ethics Committee under the approvals U020 and FEC_2019_81.
Author Contributions
KW designed the study. LF, SS, LA, JF, RN, KR, and KW performed the experiments. LF analysed the data and wrote the first manuscript draft with KW, JH, and HB-H. All authors contributed to the final manuscript.
Funding
JH and KW acknowledge funding by Natural Environment Research Council (NERC grant #NE/T001577/1), and KW, JH, and LF acknowledge funding by the University of Hull toward the MolStressH2O research cluster.
Conflict of Interest
The authors declare that the research was conducted in the absence of any commercial or financial relationships that could be construed as a potential conflict of interest.
Publisher’s Note
All claims expressed in this article are solely those of the authors and do not necessarily represent those of their affiliated organizations, or those of the publisher, the editors and the reviewers. Any product that may be evaluated in this article, or claim that may be made by its manufacturer, is not guaranteed or endorsed by the publisher.
Acknowledgments
We wish to acknowledge J. Pena dos Reis, P. Hubbard, Z. Velez, and all staff members of the CCMAR-Ramalhete Marine Station. We thank Andrea Murcia for the drawings of Diogenes pugilator, Sparus aurata, and Hediste diversicolor.
Supplementary Material
The Supplementary Material for this article can be found online at: https://www.frontiersin.org/articles/10.3389/fmars.2021.773870/full#supplementary-material
Abbreviations
CM, Control Metabolites; SM, Stress Metabolites.
References
Abreu, M. S., Giacomini, A. C. V., Gusso, D., Koakoski, G., Oliveira, T. A., Marqueze, A., et al. (2016). Behavioral responses of zebrafish depend on the type of threatening chemical cues. J. Comp. Physiol. A Neuroethol. Sens. Neural Behav. Physiol. 202, 895–901. doi: 10.1007/s00359-016-1129-5
Appelhans, Y. S., Thomsen, J., Pansch, C., Melzner, F., and Wahl, M. (2012). Sour times: seawater acidification effects on growth, feeding behaviour and acid–base status of Asterias rubens and Carcinus maenas. Mar. Ecol. Prog. Ser. 459, 85–98. doi: 10.3354/meps09697
Ashur, M. M., Johnston, N. K., and Dixson, D. L. (2017). Impacts of ocean acidification on sensory function in marine organisms. Integr. Comp. Biol. 57, 63–80. doi: 10.1093/icb/icx010
Bairos-Novak, K. R., Crane, A. L., Achtymichuk, G. H., Hsin, J., Rivera-Hernández, I. A. E., Simko, O. M., et al. (2020). Forget the audience: tadpoles release similar disturbance cues regardless of kinship or familiarity. Behav. Ecol. Sociobiol. 74, 1–10. doi: 10.1007/s00265-020-02936-8
Bairos-Novak, K. R., Crane, A. L., Chivers, D. P., and Ferrari, M. C. O. (2018). Better the devil you know? How familiarity and kinship affect prey responses to disturbance cues. Behav. Ecol. 30, 446–454. doi: 10.1093/beheco/ary184
Bairos-Novak, K. R., Mitchell, M. D., Crane, A. L., Chivers, D. P., and Ferrari, M. C. O. (2017). Trust thy neighbour in times of trouble: background risk alters how tadpoles release and respond to disturbance cues. Proc. Biol. Sci. 284:20171465. doi: 10.1098/rspb.2017.1465
Baker, M. (2016). 1,500 scientists lift the lid on reproducibility. Nature 533, 452–454. doi: 10.1038/533452a
Barcellos, L. J. G., Volpato, G. L., Barreto, R. E., Coldebella, I., and Ferreira, D. (2011). Chemical communication of handling stress in fish. Physiol. Behav. 103, 372–375. doi: 10.1016/j.physbeh.2011.03.009
Batten, S. D., and Bamber, R. N. (1996). The effects of acidified seawater on the polychaete Nereis virens Sars, 1835. Mar. Pollut. Bull. 32, 283–287. doi: 10.1016/0025-326X(95)00163-H
Bhuiyan, K. A., Rodríguez, B. M., Pires, A., Riba, I., Dellvals, Á, Freitas, R., et al. (2021). Experimental evidence of uncertain future of the keystone ragworm Hediste diversicolor (O.F. Müller, 1776) under climate change conditions. Sci. Total Environ. 750:142031. doi: 10.1016/j.scitotenv.2020.142031
Bond, D. (2018). Ocean Acidification Threat? How pH affects Burrowing Behaviour of Nereis Diversicolor and Its Bearing on Mass Extinction Scenarios. Munich: EGU.
Briffa, M., de la Haye, K., and Munday, P. L. (2012). High CO2 and marine animal behaviour: potential mechanisms and ecological consequences. Mar. Pollut. Bull. 64, 1519–1528. doi: 10.1016/j.marpolbul.2012.05.032
Burlinson, F. C., and Lawrence, A. J. (2007). Development and validation of a behavioural assay to measure the tolerance of Hediste diversicolor to copper. Environ. Pollut. 145, 274–278. doi: 10.1016/j.envpol.2005.03.014
Caldeira, K., and Wickett, M. E. (2003). Oceanography: anthropogenic carbon and ocean pH. Nature 425:365. doi: 10.1038/425365a
Chavez, F. P., Pennington, J. T., Michisaki, R. P., Blum, M., Chavez, G. M., Friederich, J., et al. (2017). Climate variability and change: response of a coastal ocean ecosystem. Oceanography 30, 128–145.
Chivers, D. P., Dixson, D. L., White, J. R., McCormick, M. I., and Ferrari, M. C. O. (2013). Degradation of chemical alarm cues and assessment of risk throughout the day. Ecol. Evol. 3, 3925–3934. doi: 10.1002/ece3.760
Clark, T. D., Raby, G. D., Roche, D. G., Binning, S. A., Speers-Roesch, B., Jutfelt, F., et al. (2020a). Ocean acidification does not impair the behaviour of coral reef fishes. Nature 577, 370–375. doi: 10.1038/s41586-019-1903-y
Clark, T. D., Raby, G. D., Roche, D. G., Binning, S. A., Speers-Roesch, B., Jutfelt, F., et al. (2020b). Reply to: methods matter in repeating ocean acidification studies. Nature 586, E25–E27. doi: 10.1038/s41586-020-2804-9
Clements, J. C., and Darrow, E. S. (2018). Eating in an acidifying ocean: a quantitative review of elevated CO2 effects on the feeding rates of calcifying marine invertebrates. Hydrobiologia 820, 1–21. doi: 10.1007/s10750-018-3665-1
Clements, J. C., Poirier, L. A., Pérez, F. F., Comeau, L. A., and Babarro, J. M. F. (2020a). Behavioural responses to predators in Mediterranean mussels (Mytilus galloprovincialis) are unaffected by elevated pCO2. Mar. Environ. Res. 161:105148. doi: 10.1016/j.marenvres.2020.105148
Clements, J. C., Sundin, J., Clark, T. D., and Jutfelt, F. (2020b). An extreme decline effect in ocean acidification ecology. EcoEvoRxiv [Preprint]. doi: 10.32942/osf.io/k9dby
Cothran, R. D., Monahan, P. J., and Relyea, R. A. (2021). Antipredator behaviour affected by prey condition, food availability and pH-mediated info-disruption. Anim. Behav. 171, 111–118. doi: 10.1016/j.anbehav.2020.11.007
Davies, G. M., and Gray, A. (2015). Don’t let spurious accusations of pseudoreplication limit our ability to learn from natural experiments (and other messy kinds of ecological monitoring). Ecol. Evol. 5, 5295–5304. doi: 10.1002/ece3.1782
de la Haye, K. L., Spicer, J. I, Widdicombe, S., and Briffa, M. (2011). Reduced sea water pH disrupts resource assessment and decision making in the hermit crab Pagurus bernhardus. Anim. Behav. 82, 495–501. doi: 10.1016/j.anbehav.2011.05.030
de la Haye, K. L., Spicer, J. I, Widdicombe, S., and Briffa, M. (2012). Reduced pH sea water disrupts chemo-responsive behaviour in an intertidal crustacean. J. Exp. Mar. Bio. Ecol. 412, 134–140. doi: 10.1016/j.jembe.2011.11.013
Draper, A. M., and Weissburg, M. J. (2019). Impacts of Global Warming and Elevated CO2 on sensory behavior in predator-prey interactions: a review and synthesis. Front. Ecol. Evol. 7:307. doi: 10.3389/fevo.2019.00072
Ende, S. S. W., Capelle, J., Kals, J., Schrama, J. W., and Verreth, J. A. J. (2017). A matter of perception: the influence of waterborne signals from fish and conspecifics on the feeding related behavior of Alitta virens (Sars). J. Exp. Mar. Bio. Ecol. 496, 91–96. doi: 10.1016/j.jembe.2017.08.004
Eriander, L., Wrange, A.-L., and Havenhand, J. N. (2015). Simulated diurnal pH fluctuations radically increase variance in—but not the mean of—growth in the barnacle Balanus improvisus. ICES J. Mar. Sci. 73, 596–603. doi: 10.1093/icesjms/fsv214
Fehsenfeld, S., Kiko, R., Appelhans, Y., Towle, D. W., Zimmer, M., and Melzner, F. (2011). Effects of elevated seawater pCO(2) on gene expression patterns in the gills of the green crab, Carcinus maenas. BMC Genomics 12:488. doi: 10.1186/1471-2164-12-488
Feugere, L., Scott, V. F., Rodriguez-Barucg, Q., Beltran-Alvarez, P., and Wollenberg Valero, K. C. (2021). Thermal stress induces a positive phenotypic and molecular feedback loop in zebrafish embryos. J. Therm. Biol. 102:103114. doi: 10.1016/j.jtherbio.2021.103114
Freitas, R., Pires, A., Moreira, A., Wrona, F. J., Figueira, E., and Amadeu, M. V. (2016). Biochemical alterations induced in Hediste diversicolor under seawater acidification conditions. Mar. Environ. Res. 117, 75–84. doi: 10.1016/j.marenvres.2016.04.003
Frisch, K. (1938). Zur Psychologie des Fisch-Schwarmes. Naturwissenschaften 26, 601–606. doi: 10.1007/BF01590598
Froehlich, K. R., and Lord, J. P. (2020). Can ocean acidification interfere with the ability of mud snails (Tritia obsoleta) to sense predators? J. Exp. Mar. Bio. Ecol. 526:151355. doi: 10.1016/j.jembe.2020.151355
Fuselier, L., Rugg, M., Korpi, N., and Wisenden, B. (2009). Lab and field estimates of active time of chemical alarm cues of a cyprinid fish and an amphipod crustacean. Behaviour 146, 1423–1442. doi: 10.1163/156853909X440998
Giacomini, A. C., de Abreu, M. S., Koakoski, G., Idalêncio, R., Kalichak, F., Oliveira, T. A., et al. (2015). My stress, our stress: blunted cortisol response to stress in isolated housed zebrafish. Physiol. Behav. 139, 182–187. doi: 10.1016/j.physbeh.2014.11.035
Goldman, J. A., Désormeaux, I. S., and Brown, G. E. (2020a). Disturbance cues as a source of risk assessment information under natural conditions. Freshw. Biol. 65, 981–986. doi: 10.1111/fwb.13484
Goldman, J. A., Feyten, L. E. A., Ramnarine, I. W., and Brown, G. E. (2020b). Sender and receiver experience alters the response of fish to disturbance cues. Curr. Zool. 66, 255–261. doi: 10.1093/cz/zoz050
Gregório, S. F., Ruiz-Jarabo, I., Carvalho, E. M., and Fuentes, J. (2019). Increased intestinal carbonate precipitate abundance in the sea bream (Sparus aurata L.) in response to ocean acidification. PLoS One 14:e0218473. doi: 10.1371/journal.pone.0218473
Hardege, J. D. (1999). Nereidid polychaetes as model organisms for marine chemical ecology. Hydrobiologia 402, 145–161. doi: 10.1023/A:1003740509104
Hazlett, B. A. (1985). Disturbance pheromones in the crayfishOrconectes virilis. J. Chem. Ecol. 11, 1695–1711. doi: 10.1007/BF01012121
Hofmann, G. E., Smith, J. E., Johnson, K. S., Send, U., Levin, L. A., Micheli, F., et al. (2011). High-frequency dynamics of ocean pH: a multi-ecosystem comparison. PLoS One 6:e28983. doi: 10.1371/journal.pone.0028983
IPCC (2019). IPCC Special Report on the Ocean and Cryosphere in a Changing Climate. Geneva: Intergovernmental Panel on Climate Change.
Jellison, B. M., Ninokawa, A. T., Hill, T. M., Sanford, E., and Gaylord, B. (2016). Ocean acidification alters the response of intertidal snails to a key sea star predator. Proc. Biol. Sci. 283:20160890. doi: 10.1098/rspb.2016.0890
Jordão, L. C., and Volpato, G. L. (2000). Chemical Transfer of Warning Information in Non-Injured Fish. Behaviour 137, 681–690. doi: 10.1163/156853900502286
Júnior, A. B., Magalhães, E. J., Hoffmann, A., and Ide, L. M. (2010). Conspecific and heterospecific alarm substance induces behavioral responses in piau fish Leporinus piau. Acta Ethol. 13, 119–126. doi: 10.1007/s10211-010-0081-6
Karlson, P., and Lüscher, M. (1959). Pheromone. Naturwissenschaften 46, 63–64. doi: 10.1007/BF00599084
Kassambara, A., Kosinski, M., and Biecek, P. (2020). Drawing Survival Curves using “ggplot2” [R package survminer version 0.4.8]. Available Online at: https://CRAN.R-project.org/package=survminer (accessed November 2021).
Katz, J. N., and Rittschof, D. (1993). Alarm/investigation responses of hermit crabs as related to shell fit and crab size. Mar. Behav. Physiol. 22, 171–182. doi: 10.1080/10236249309378845
Kim, T. W., Taylor, J., Lovera, C., and Barry, J. P. (2015). CO2-driven decrease in pH disrupts olfactory behaviour and increases individual variation in deep-sea hermit crabs. ICES J. Mar. Sci. 73, 613–619. doi: 10.1093/icesjms/fsv019
Kroeker, K. J., Kordas, R. L., Crim, R., Hendriks, I. E., Ramajo, L., Singh, G. S., et al. (2013). Impacts of ocean acidification on marine organisms: quantifying sensitivities and interaction with warming. Glob. Chang. Biol. 19, 1884–1896. doi: 10.1111/gcb.12179
Landschützer, P., Gruber, N., Bakker, D. C. E., Stemmler, I., and Six, K. D. (2018). Strengthening seasonal marine CO2 variations due to increasing atmospheric CO2. Nat. Clim. Change 8, 146–150. doi: 10.1038/s41558-017-0057-x
Lenth, R. (2021). emmeans: Estimated Marginal Means, aka Least-squares Means. R package version 1.5.5-1. Available Online at: https://cran.r-project.org/web/packages/emmeans (accessed November 2021).
Lüdecke, D. (2021). Data Visualization for Statistics in Social Science. R package sjPlot version 2.8.7. Available Online at: https://CRAN.R-project.org/package=sjPlot (accessed November 2021).
Mathuru, A. S. (2016). Conspecific injury raises an alarm in medaka. Sci. Rep. 6:36615. doi: 10.1038/srep36615
Mathuru, A. S., Kibat, C., Cheong, W. F., Shui, G., Wenk, M. R., Friedrich, R. W., et al. (2012). Chondroitin fragments are odorants that trigger fear behavior in fish. Curr. Biol. 22, 538–544. doi: 10.1016/j.cub.2012.01.061
Mazerolle, M. J. (2020). Model selection and multimodel inference using the AICcmodavg package. Available Online at: https://mirror.marwan.ma/cran/web/packages/AICcmodavg/vignettes/AICcmodavg.pdf [accessed Oct 4, 2021].
McBriarty, G. J., Kidd, K. A., and Burridge, L. E. (2018). Short-Term Effects of the Anti-sea Lice Therapeutant Emamectin Benzoate on Clam Worms (Nereis virens). Arch. Environ. Contam. Toxicol. 74, 539–545. doi: 10.1007/s00244-017-0461-2
Morishita, V. R., and Barreto, R. E. (2011). Black sea urchins evaluate predation risk using chemical signals from a predator and injured con- and heterospecific prey. Mar. Ecol. Prog. Ser. 435, 173–181. doi: 10.3354/meps09253
Mothersill, C., Smith, R. W., Agnihotri, N., and Seymour, C. B. (2007). Characterization of a radiation-induced stress response communicated in vivo between zebrafish. Environ. Sci. Technol. 41, 3382–3387. doi: 10.1021/es062978n
Mouneyrac, C., Mastain, O., Amiard, J. C., Amiard-Triquet, C., Beaunier, P., Jeantet, A.-Y., et al. (2003). Trace-metal detoxification and tolerance of the estuarine worm Hediste diversicolor chronically exposed in their environment. Mar. Biol. 143, 731–744. doi: 10.1007/s00227-003-1124-6
Munday, P. L., Dixson, D. L., Donelson, J. M., Jones, G. P., Pratchett, M. S., Devitsina, G. V., et al. (2009). Ocean acidification impairs olfactory discrimination and homing ability of a marine fish. Proc. Natl. Acad. Sci. U. S. A. 106, 1848–1852. doi: 10.1073/pnas.0809996106
Munday, P. L., Dixson, D. L., Welch, M. J., Chivers, D. P., Domenici, P., Grosell, M., et al. (2020). Methods matter in repeating ocean acidification studies. Nature 586, E20–E24. doi: 10.1038/s41586-020-2803-x
Oliveira, T. A., Koakoski, G., Kreutz, L. C., Ferreira, D., da Rosa, J. G. S., de Abreu, M. S., et al. (2013). Alcohol impairs predation risk response and communication in zebrafish. PLoS One 8:e75780. doi: 10.1371/journal.pone.0075780
Perrot-Minnot, M.-J., Banchetry, L., and Cézilly, F. (2017). Anxiety-like behaviour increases safety from fish predation in an amphipod crustacea. R. Soc. Open Sci. 4:171558. doi: 10.1098/rsos.171558
Porteus, C. S., Hubbard, P. C., Uren Webster, T. M., van Aerle, R., Canário, A. V. M., Santos, E. M., et al. (2018). Near-future CO 2 levels impair the olfactory system of a marine fish. Nat. Clim. Chang. 8, 737–743. doi: 10.1038/s41558-018-0224-8
Porteus, C. S., Roggatz, C. C., Velez, Z., Hardege, J. D., and Hubbard, P. C. (2021). Acidification can directly affect olfaction in marine organisms. J. Exp. Biol. 224:jeb237941 doi: 10.1242/jeb.237941
Pörtner, H. (2008). Ecosystem effects of ocean acidification in times of ocean warming: a physiologist’s view. Mar. Ecol. Prog. Ser. 373, 203–217. doi: 10.3354/meps07768
Pörtner, H. O., and Peck, M. A. (2010). Climate change effects on fishes and fisheries: towards a cause-and-effect understanding. J. Fish Biol. 77, 1745–1779. doi: 10.1111/j.1095-8649.2010.02783.x
R Core Team (2020). R: A Language and Environment for Statistical Computing. Vienna: R Foundation for Statistical Computing.
Richardson, B., Martin, H., Bartels-Hardege, H., Fletcher, N., and Hardege, J. D. (2021). The role of changing pH on olfactory success of predator–prey interactions in green shore crabs, Carcinus maenas. Aquat. Ecol. doi: 10.1007/s10452-021-09913-x [Epub ahead of print].
Robbins, L., Hansen, M. E., Kleypas, J. A., and Meylan, S. C. (2010). CO2calc: A User-Friendly Seawater Carbon Calculator for Windows, Mac OS X, and iOS (iPhone). Reston: US Geological Survey.
Roggatz, C. C., Fletcher, N., Benoit, D. M., Algar, A. C., Doroff, A., Wright, B., et al. (2019). Saxitoxin and tetrodotoxin bioavailability increases in future oceans. Nat. Clim. Chang. 9, 840–844. doi: 10.1038/s41558-019-0589-3
Roggatz, C. C., Lorch, M., Hardege, J. D., and Benoit, D. M. (2016). Ocean acidification affects marine chemical communication by changing structure and function of peptide signalling molecules. Glob. Chang. Biol. 22, 3914–3926. doi: 10.1111/gcb.13354
RStudio Team (2020). RStudio: Integrated Development Environment for R. Available Online at: http://www.rstudio.com/ (accessed November 2021).
Sabine, C. L., Feely, R. A., Gruber, N., Key, R. M., Lee, K., Bullister, J. L., et al. (2004). The oceanic sink for anthropogenic CO2. Science 305, 367–371. doi: 10.1126/science.1097403
Saha, M., Berdalet, E., Carotenuto, Y., Fink, P., Harder, T., John, U., et al. (2019). Using chemical language to shape future marine health. Front. Ecol. Environ. 17, 530–537. doi: 10.1002/fee.2113
Schirrmacher, P., Roggatz, C. C., Benoit, D. M., and Hardege, J. D. (2020). Ocean acidification amplifies the Olfactory Response to 2-Phenylethylamine: altered cue reception as a mechanistic pathway? bioRxiv [Preprint]. doi: 10.1101/2020.10.08.329870
Shrivastava, J., Ndugwa, M., Caneos, W., and De Boeck, G. (2019). Physiological trade-offs, acid-base balance and ion-osmoregulatory plasticity in European sea bass (Dicentrarchus labrax) juveniles under complex scenarios of salinity variation, ocean acidification and high ammonia challenge. Aquat. Toxicol. 212, 54–69. doi: 10.1016/j.aquatox.2019.04.024
Sokolova, I. (2018). Mitochondrial adaptations to variable environments and their role in animals’ stress tolerance. Integr. Comp. Biol. 58, 519–531. doi: 10.1093/icb/icy017
Sordo, L., Santos, R., Barrote, I., and Silva, J. (2018). High CO2 decreases the long-term resilience of the free-living coralline algae Phymatolithon lusitanicum. Ecol. Evol. 8, 4781–4792. doi: 10.1002/ece3.4020
Sordo, L., Santos, R., Reis, J., Shulika, A., and Silva, J. (2016). A direct CO2 control system for ocean acidification experiments: testing effects on the coralline red algae Phymatolithon lusitanicum. PeerJ 4:e2503. doi: 10.7717/peerj.2503
Therneau, T. M. (2021). Survival Analysis [R package survival version 3.2-10]. Available Online at: https://CRAN.R-project.org/package=survival (accessed November 2021).
Therneau, T. M., and Grambsch, P. M. (2000). Modeling Survival Data: Extending the Cox Model. Statistics for Biology and Health. Berlin: Springer Science & Business Media. doi: 10.1007/978-1-4757-3294-8
Thomas, J. T., Munday, P. L., and Watson, S.-A. (2020). Toward a Mechanistic Understanding of Marine Invertebrate Behavior at Elevated CO2. Front. Mar. Sci. 7:345. doi: 10.3389/fmars.2020.00345
Toa, D. G., Afonso, L. O. B., and Iwama, G. K. (2004). Stress response of juvenile rainbow trout (Oncorhynchus mykiss)to chemical cues released from stressed conspecifics. Fish Physiol. Biochem. 30, 103–108. doi: 10.1007/s10695-005-0266-5
Tomsic, D., Sztarker, J., Berón de Astrada, M., Oliva, D., and Lanza, E. (2017). The predator and prey behaviors of crabs: from ecology to neural adaptations. J. Exp. Biol. 220, 2318–2327. doi: 10.1242/jeb.143222
Truchot, J. P., and Duhamel-Jouve, A. (1980). Oxygen and carbon dioxide in the marine intertidal environment: diurnal and tidal changes in rockpools. Respir. Physiol. 39, 241–254. doi: 10.1016/0034-5687(80)90056-0
Velez, Z., Costa, R. A., Wang, W., and Hubbard, P. C. (2021). Independent effects of seawater pH and high PCO2 on olfactory sensitivity in fish: possible role of carbonic anhydrase. J. Exp. Biol. 224:jeb238485. doi: 10.1242/jeb.238485
Wage, J., Rohr, S., Hardege, J. D., and Rotchell, J. M. (2016). Short-term effects of CO2-induced low pH exposure on target gene expression in Platynereis dumerilii. J. Mar. Biol. Oceanogr. 5:2. doi: 10.4172/2324-8661.1000155
Wang, Y., Hu, M., Wu, F., Storch, D., and Pörtner, H.-O. (2018). Elevated pCO2 affects feeding behavior and acute physiological response of the brown crab Cancer pagurus. Front. Physiol. 9:1164. doi: 10.3389/fphys.2018.01164
Watson, G. J., Hamilton, K. M., and Tuffnail, W. E. (2005). Chemical alarm signalling in the polychaete Nereis (Neanthes) virens (Sars) (Annelida: polychaeta). Anim. Behav. 70, 1125–1132. doi: 10.1016/j.anbehav.2005.03.011
Williamson, P., Pörtner, H.-O., Widdicombe, S., and Gattuso, J.-P. (2021). Ideas and perspectives: when ocean acidification experiments are not the same, repeatability is not tested. Biogeosciences 18, 1787–1792. doi: 10.5194/bg-18-1787-2021
Wittmann, A. C., and Pörtner, H.-O. (2013). Sensitivities of extant animal taxa to ocean acidification. Nat. Clim. Chang. 3, 995–1001. doi: 10.1038/nclimate1982
Wolfe, K., Nguyen, H. D., Davey, M., and Byrne, M. (2020). Characterizing biogeochemical fluctuations in a world of extremes: a synthesis for temperate intertidal habitats in the face of global change. Glob. Chang. Biol. 26, 3858–3879. doi: 10.1111/gcb.15103
Wu, F., Wang, T., Cui, S., Xie, Z., Dupont, S., Zeng, J., et al. (2017). Effects of seawater pH and temperature on foraging behavior of the Japanese stone crab Charybdis japonica. Mar. Pollut. Bull. 120, 99–108. doi: 10.1016/j.marpolbul.2017.04.053
Keywords: behavioural cost, stress propagation, stress metabolites, chemical communication, ocean acidification
Citation: Feugere L, Angell L, Fagents J, Nightingale R, Rowland K, Skinner S, Hardege J, Bartels-Hardege H and Wollenberg Valero KC (2021) Behavioural Stress Propagation in Benthic Invertebrates Caused by Acute pH Drop-Induced Metabolites. Front. Mar. Sci. 8:773870. doi: 10.3389/fmars.2021.773870
Received: 10 September 2021; Accepted: 09 November 2021;
Published: 30 November 2021.
Edited by:
Paul E. Renaud, Akvaplan Niva, NorwayReviewed by:
Marc Weissburg, Georgia Institute of Technology, United StatesPhilip Munday, ARC Centre of Excellence for Coral Reef Studies, Australia
Copyright © 2021 Feugere, Angell, Fagents, Nightingale, Rowland, Skinner, Hardege, Bartels-Hardege and Wollenberg Valero. This is an open-access article distributed under the terms of the Creative Commons Attribution License (CC BY). The use, distribution or reproduction in other forums is permitted, provided the original author(s) and the copyright owner(s) are credited and that the original publication in this journal is cited, in accordance with accepted academic practice. No use, distribution or reproduction is permitted which does not comply with these terms.
*Correspondence: Katharina C. Wollenberg Valero, ay53b2xsZW5iZXJnLXZhbGVyb0BodWxsLmFjLnVr; orcid.org/0000-0001-8858-1804