- 1Antarctic Ecosystem Research Division, Southwest Fisheries Science Center, NOAA Fisheries, La Jolla, CA, United States
- 2Marine and Environmental Science Department, Hampton University, Hampton, VA, United States
Antarctic fur seals (AFS) are an ecologically important predator and a focal indicator species for ecosystem-based Antarctic fisheries management. This species suffered intensive anthropogenic exploitation until the early 1900s, but recolonized most of its former distribution, including the southern-most colony at Cape Shirreff, South Shetland Islands (SSI). The IUCN describes a single, global AFS population of least concern; however, extensive genetic analyses clearly identify four distinct breeding stocks, including one in the SSI. To update the population status of SSI AFS, we analyzed 20 years of field-based data including population counts, body size and condition, natality, recruitment, foraging behaviors, return rates, and pup mortality at the largest SSI colony. Our findings show a precipitous decline in AFS abundance (86% decrease since 2007), likely driven by leopard seal predation (increasing since 2001, p << 0.001) and potentially worsening summer foraging conditions. We estimated that leopard seals consumed an average of 69.3% (range: 50.3–80.9%) of all AFS pups born each year since 2010. AFS foraging-trip durations, an index of their foraging habitat quality, were consistent with decreasing krill and fish availability. Significant improvement in the age-specific over-winter body condition of AFS indicates that observed population declines are driven by processes local to the northern Antarctic Peninsula. The loss of SSI AFS would substantially reduce the genetic diversity of the species, and decrease its resilience to climate change. There is an urgent need to reevaluate the conservation status of Antarctic fur seals, particularly for the rapidly declining SSI population.
Introduction
Rapid changes in the abundance of, or prey selection by, apex predators can fundamentally alter ecosystems through dynamic predator-prey interactions (Hairston et al., 1960; Paine, 1966; Dayton, 1971; Estes et al., 2011). Marine mammalian apex predators, in particular, may drive rapid declines in coastal prey populations because of their large body sizes and proportionally high energetic demands (Williams et al., 2004; Pagano et al., 2018). Despite this potential, regional-scale examples of such effects have been rare (Estes et al., 1998; Springer et al., 2003), and sometimes controversial (DeMaster et al., 2006; Wade et al., 2009); in part, because direct observations of predation are difficult to obtain in remote marine environments (Estes et al., 1998).
Long-term monitoring of marine predators is fundamental to ecosystem-based management of marine ecosystems (Boyd et al., 2006; Moore, 2008). The population success of central place foragers, like some pinnipeds, depends upon predictable prey availability. Therefore, estimates of pinniped abundance (e.g., Southwell et al., 2012; Trukhanova et al., 2013; Lowry et al., 2014), and characterizations of their foraging behavior (Trillmich and Dellinger, 1991; Melin et al., 2008) and body condition (Costa et al., 1989) are used to inform conservation and fisheries management (Weise and Harvey, 2008; Melin et al., 2010). The Antarctic fur seal (AFS, Arctocephalus gazella) is a key indicator species for the ecosystem-based management of the Antarctic krill (Euphausia superba) fishery (Boyd and Murray, 2001).
Rapid climatic warming and the associated loss of sea ice (Meredith and King, 2005; Vaughan, 2006; Turner et al., 2014) in the western Antarctic Peninsula (WAP) are expected to alter pelagic communities and shift their geographical distributions, including those of Antarctic krill and their manifold dependent predators (Massom and Stammerjohn, 2010; Ducklow et al., 2013; Klein et al., 2018). As expected, ice-dependent, and ice-associated penguins are in decline throughout the Peninsula region (Hinke et al., 2007; Trivelpiece et al., 2011; Lynch et al., 2012). Ice seals, like crabeater (Lobodon carcinophagus) and leopard seals (Hydrurga leptonyx), have also likely experienced substantial changes in abundance and distribution (Forcada et al., 2012; Hückstädt et al., 2020). Conversely, at the high-latitude extreme of their thermal tolerance (Costa et al., 1989; Reid and Croxall, 2001), populations of AFS were expected to flourish in a warmer climate due to increased habitat (Siniff et al., 2008; Costa et al., 2010).
Extensive harvesting of AFS between the late 1700s and early 1900s extirpated the species from much of its range, and reduced the global population to near extinction (Bonner, 1968; Weddell, 1970; McCann and Doidge, 1987). By the late 1990s the global population of AFS was estimated to have recovered to near historical levels (Hofmeyr, 2016; Foley and Lynch, 2020). The current conservation paradigm for AFS hypothesizes that a single population survived near the subantarctic island of South Georgia, expanded through the mid-1900s and then recolonized other subantarctic island groups as well as the only Antarctic breeding population of AFS in the South Shetland Islands (SSI; Laws, 1973; Payne, 1977; Shaughnessy and Goldsworthy, 1990), where the only Antarctic breeding population of AFS exists. This hypothesis was consistent with the lack of population structure detected using mitochondrial DNA (mtDNA) markers (Wynen et al., 2000). Therefore, based on the best available science in 2014, the International Union for Conservation of Nature (IUCN) determined that AFS is a species of “least concern” and “neither this species as a whole, nor any separate colonies, are likely to become extinct in the near future” (Hofmeyr, 2016).
A suite of recent studies, however, suggest that the existing conservation paradigm may be inaccurate on two key points. First, genetic analyses using large sample sizes and nuclear markers demonstrate that rather than a single genetic stock, there are at least four genetically distinct subpopulations from South Georgia, Bouvetøya, SSI, and the remaining subantarctic islands (Bonin et al., 2013; Humble et al., 2018; Cleary et al., 2019; Paijmans et al., 2020). Second, three of these four subpopulations have declined in abundance. In addition to reductions (2.8–5.6% per annum) in the number of breeding females at South Georgia and Bouvetøya since the early 2000s (Forcada and Hoffman, 2014; Hofmeyr, 2016), recent reports suggest a rapid, potentially catastrophic decline in the SSI population (Krause et al., 2020; Krause and Hinke, 2021).
Initial concern for AFS related to climate change focused on population and habitat effects due to redistribution of their prey resources (Siniff et al., 2008; Kovacs et al., 2012). Indeed, the use of AFS as indicator species for fisheries management (Boyd and Murray, 2001) and the bulk of population dynamics research to date both assumed and demonstrated bottom-up population control (Boyd, 1993; Reid and Croxall, 2001; Forcada et al., 2005; Forcada and Hoffman, 2014). While predation of AFS pups by leopard seals was reported at South Georgia as early as the 1980s, analyses indicated this top-down forcing had no effect on AFS population dynamics (McCann and Doidge, 1987; Forcada et al., 2009).
As the only breeding population of AFS south of the Antarctic Convergence and the highest-latitude otariid population on Earth, the SSI colonies face a unique array of environmental and ecological challenges. Adaptation to colder air and water temperatures, for example, are likely drivers for the shorter provisioning trip durations and larger body sizes observed for SSI AFS compared with their subantarctic counterparts (this study). Further, AFS from SSI colonies are closer to the core habitat of leopard seals (Forcada et al., 2012). Top-down control of a small SSI AFS colony by leopard seals was modeled in the late 1990s (Boveng et al., 1998), however, broad population recovery continued across the archipelago at that time (Goebel et al., 2003). The recent population crash at Cape Shirreff may be driven by leopard seal predation, and the magnitude could be substantially larger than previously reported (Krause et al., 2015, 2020).
AFS colonies on and around Cape Shirreff, Livingston Island produce 60–85% of all pups born in the SSI (Bengtson et al., 1990; Krause and Hinke, 2021). Further, since the late 1990s the National Oceanic and Atmospheric Administration has annually operated a comprehensive, individual-based monitoring program tracking foraging behavior, body condition, pup survival, observations of predation, and population dynamics of AFS at Cape Shirreff. Here our objectives are to: (1) evaluate the current evidence of AFS population structure, particularly the assignment of the SSI AFS as a genetically distinct subpopulation, (2) summarize the population trends of AFS in the SSI over the last 20 years, and, (3) examine standardized behavioral and demographic parameters of SSI AFS to explain observed trends and inform future research.
Materials and Methods
Study Site
Research was conducted at Cape Shirreff (62.47° S, 60.77° W) on the north shore of Livingston Island, in the South Shetland Islands archipelago (Figure 1). Historically, the southern boundary of the southern Antarctic Circumpolar Current front overlaps with the continental shelf break approximately 50 km north of the Cape (Orsi et al., 1995). That association enhances the availability of Antarctic krill near the Cape (Atkinson et al., 2009), and results in a persistent foraging hotspot for AFS and other Antarctic predators (Santora and Veit, 2013). Since 1996–1997 (hereafter austral summers are referred to by the second year, e.g., 1997), the U.S. Antarctic Marine Living Resources (U.S. AMLR) Program has conducted long-term ecological monitoring of Antarctic pinnipeds, including AFS, as indicator species to inform management of the regional krill fishery (Agnew, 1997; Goebel et al., 2008). All methods reported here were implemented each summer between 2001 and 2020 unless otherwise indicated.
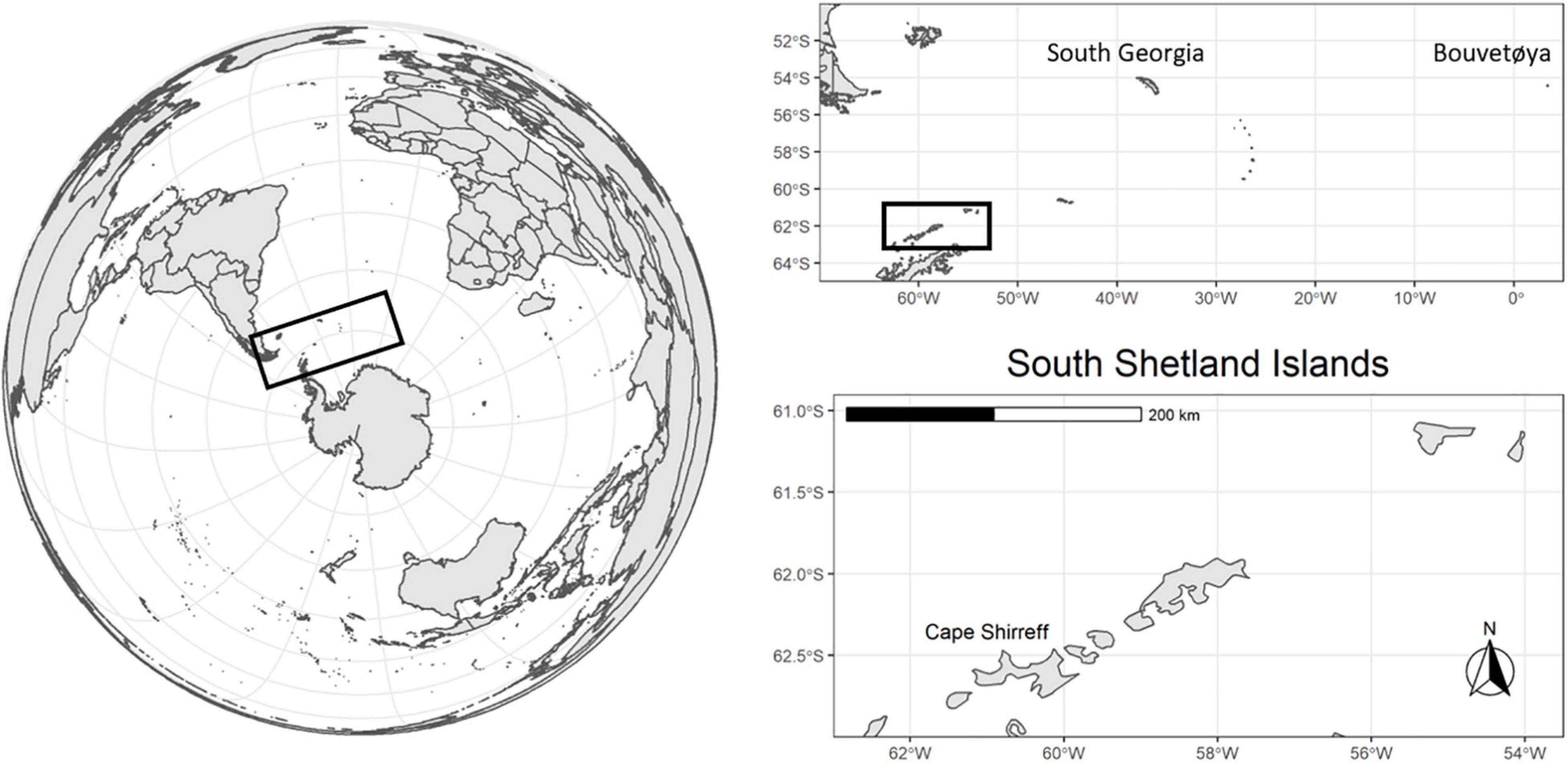
Figure 1. Locations of the western Antarctic fur seal breeding centers including: South Georgia, Bouvetøya, and the South Shetland Islands.
All animal research described herein was conducted in accordance with Marine Mammal Protection Act Permit Nos. 1024, 774-1649, 774-1847, 16472, and 20599, Antarctic Conservation Act Permit Nos. 97-016, 2002-007, 2007-003, 2012-005 and 2017-012, and NMFS-SWFSC Institutional Animal Care and Use Committee Permit Nos. IACUC SWPI2011-02, SWPI 2014-03, and SWPI 2017-03.
Antarctic Fur Seal Breeding Ecology and Dispersal
AFS are polygynous breeders in which males establish territories on breeding beaches (October-November) and accumulate harems of females as the latter arrive to the colonies. Pregnant females give birth within 1–2 days of arrival and most pupping occurs in late November to early December (Bonner, 1968). After suckling their pups for 5–7 days they begin a cyclical series of foraging trips to sea interspersed with onshore pup-provisioning visits (Payne, 1977). During the austral summer, AFS are dependent upon Antarctic krill as their main food source, with myctophid fishes and squid as alternates (Polito and Goebel, 2010). While provisioning pups, female AFS foraging trips vary in duration depending upon the availability of prey, but typically last 4–5 days near South Georgia (Costa et al., 1989; Boyd, 1999). Pups that survive through the summer are weaned in late March to early April. After weaning, females from Cape Shirreff disperse widely and forage in pelagic habitats, including off the coasts of Chile and Argentina (Arthur et al., 2017; Hinke et al., 2017). Conversely, weaned pups depart from Cape Shirreff, but typically remain in the South Shetland Islands and northern Antarctic Peninsula during their first year. Subadult and adult males move south along the Antarctic Peninsula in the fall and winter.
Antarctic Fur Seals Identification Marking and Daily Observations
We deployed bilateral identification (ID) flipper tags on every study animal captured since 1998 (NID–tagged adults = 613). The individually numbered Dalton cattle tags (40 mm × 20 mm × 2 mm, 10 g) were deployed in the cartilaginous tissue at the proximal trailing edge of the fore flippers to maximize visibility and reduce hydrodynamic drag. As part of our mark re-capture study we also deployed ∼500 ID flipper tags per annum on AFS pups between 1998 and 2011. Thereafter, we decreased the tagging rate to target 10% of the pups born each year through 2020 (NID–tagged pups = 8,776).
Attendance Behavior
In addition to daily observations of behavior, each year we captured and closely monitored approximately 30 ( = 28.9, range: 16–34) adult female-pup pairs to study inter- and intra-annual changes in female body condition, pup attendance and foraging behavior. We captured visibly healthy females with hoop nets (Animal Equipment by Stoney, LLC) during the perinatal period (1–2 days postpartum during late November—early December) following procedures described by Gentry and Holt (1982) and Majluf and Goebel (1992). Some animals were restrained on capture boards, however, the majority (>82%) were anesthetized using isoflurane gas (Gales, 1989; Gales and Mattlin, 1998) and midazolam (dosage: 0.1–0.25 mg/kg, Haulena, 2014). Pups were manually restrained, and reunited with their mother post-anesthetic recovery. We measured adult females and pups for standard length and axillary girth to the nearest 0.5 cm (Scheffer, 1967); we also weighed them using suspension scales (± 0.1 kg). We marked pups with unique bleach patterns in their fur, and applied identification tags to all adult females that were not previously tagged.
We attached marine VHF radio transmitters (164 Mhz, 55 mm × 23 mm × 10 mm, 23 g, Advanced Telemetry Systems Inc.) to the dorsal pelage of all females included in the attendance study using standard 5-min marine epoxy. VHF receivers integrated with automated data loggers recorded the presence or absence of each female every 30 min throughout the breeding season. Each absence longer than 7 h of a female who later returned to a living pup was classified as a foraging trip. We corroborated VHF presence data with alternate VHF receivers, visual observations, and animal-borne data loggers when available. We calculated mean trip durations and standard errors for the first six foraging trips per season (Agnew, 1997). As a metric for describing unusually good or poor foraging conditions, we defined seasons with average trip lengths above or below one standard deviation of the mean for the entire study period as extreme.
Annual Pup Census
Due to regular absences by foraging females throughout the breeding season, and the irregular haul out patterns of males and subadults, the most informative measure of fur seal population size is to annually count pups (Payne, 1979; Bengtson et al., 1990). The U.S. AMLR Program conducted a synoptic census of AFS pups born at Cape Shirreff each year between 2008 and 2020 (for methods used in other years see references listed in Supplementary Table 1). Annual pup censuses were done in late December when over 99% of pups were born, before pups explore widely beyond their birth beach, and before seasonal rates of pup predation increased. In each season at least three field biologists surveyed every breeding beach independently using hand-held binoculars. Counts of pups (live and dead) were independently reviewed, and recounts were conducted as necessary to ensure that all beach-specific counts were within 5% across all observers.
Female Return and Natality Rates
Population dynamics are essentially controlled by the balance between birth and death rates; however, in polygynous otariid populations such rates are disproportionately affected by key factors such as adult female survival, and natality rates (Boyd et al., 1995). Long-term pinniped monitoring programs rely on regular observations of a subset of individuals from their respective breeding populations to provide indices of these key demographic parameters (Boyd, 1993; Melin et al., 2012; DeLong et al., 2017).
Each season every previously ID-tagged adult female was observed daily from arrival through early March. We recorded arrival date and location for all returning females, and parturition status. Because rates of philopatry are exceptionally high for AFS females (Payne, 1977; Hoffman and Forcada, 2012), we considered return rate a proxy for adult female survival and calculated natality rates as the annual proportions of returned adult females that bore pups.
Pup Mortality
A key bottleneck to recruitment for Antarctic predators is survival through the first year (Payne, 1977; Hinke et al., 2020). Unfortunately, it is challenging to assess pup survival for the entire population due to the likelihood that carcasses will be missed, washed away, or double-counted (Hofmeyr et al., 2005). Therefore, to estimate rates of survival to weaning, we tracked the daily health, nutritive status, and survival of all pups born to ID-tagged females at Cape Shirreff. We categorized pup mortality as: (1) neonate mortality (pups that died within 21 days of birth, still birth, being injured by adults, or starvation), (2) assumed leopard seal predation (pups observed being killed by a leopard seal, pup carcasses found with characteristic indicators of leopard seal predation, e.g., crushed or missing skull, inverted body cavity, or healthy pups that disappeared suddenly with no other explanation), or (3) other (all other known causes of death, e.g., starvation beyond 21 days after birth, predation by skuas or giant petrels, potential disease, or other injuries).
Age Determination
Many demographic and health parameters are affected by age. For example, body size and natality rates are significantly lower for young females (Payne, 1979), and pregnancy rates decline in females over the age of 17 at Cape Shirreff (Goebel and Reiss, 2014). Therefore, in addition to all known-age animals tagged as pups, we derived the age of some adult females by analyzing one of their teeth. Characteristic growth layers in the cementum can accurately reflect the age of pinnipeds (Laws, 1952; Arnbom et al., 1992; Childerhouse et al., 2004). For a subset of our anesthetized attendance study females, we extracted a lower post-canine tooth (N = 345). We stored teeth in 90% ethanol. We decalcified the teeth, cut a longitudinal section (0.4 mm) using a diamond-edged saw, and mounted each section to a glass microscope slide (Childerhouse et al., 2004). A tooth-aging laboratory (Matson’s Lab, Missoula, MT) provided independent counts of dentition growth layers from each slide by multiple observers.
Based on a multi-year analysis of known-age females at Cape Shirreff, we defined females in our study between the ages of 7 and 17 as prime breeders, due to their substantially higher natality and pup growth rates (Goebel and Reiss, 2014).
Data Analysis
We conducted all analyses using R (R-Core-Team, 2021). All values are listed as mean () ± standard deviation, and all inferences were based on a significance level of p ≤ 0.05 unless otherwise indicated. We tested for predictable trends in measurements over the study period using ordinary least squares regressions, verified test assumptions were met, and plotted all figures using ggplot2 (Wickham, 2009).
Inter-Regional Body Size Comparison
In addition to reviewing literature on genetic comparisons between known AFS populations, we compared standard lengths and masses between published measurements from the South Georgia population collected during 1973 and 1974 (Payne, 1979), and the Cape Shirreff population collected between 2001 and 2020. We matched the range of capture dates (late January—early March), and selected only breeding age females (≥ 3 years). We tested for differences using a Welch’s t-test.
Female Arrival Body Condition
The relative proportion of fat stores to body size, or body condition, of otariids is related to quality of the foraging environment before measurement (Boyd and McCann, 1989; Costa et al., 1989). Further, body condition is positively related to natality rate (Guinet et al., 1998). For each season we calculated a body condition index (BCI) for all females included in our attendance study by linearly regressing body mass against standard length and using the residuals from the fitted line as BCI following Guinet et al. (1998). We controlled for the variable weight of fetus and placenta in females just after arrival by only selecting females who were 1–2 days postpartum.
Results
Antarctic Fur Seal Population Structure
Between 2001 and 2020 we captured, measured, and weighed adult female AFS during the second half of the breeding season. Mean standard length was 129.98 ± 6.0 cm (N = 376), and mean mass was 42.12 ± 5.7 kg (N = 377). Based on captures at South Georgia during the 1973 and 1974 summer seasons, Payne (1979) reported standard lengths from 190 ( = 126.30 ± 6.4 cm), and masses from 166 ( = 32.31 ± 5.7 kg) adult female AFS. Adult females from Cape Shirreff were both longer (t = 6.6176, df = 357.01, p << 0.001, Figure 2A), and more massive (t = 18.392, df = 293.93, p << 0.001, Figure 2B) than those from South Georgia.
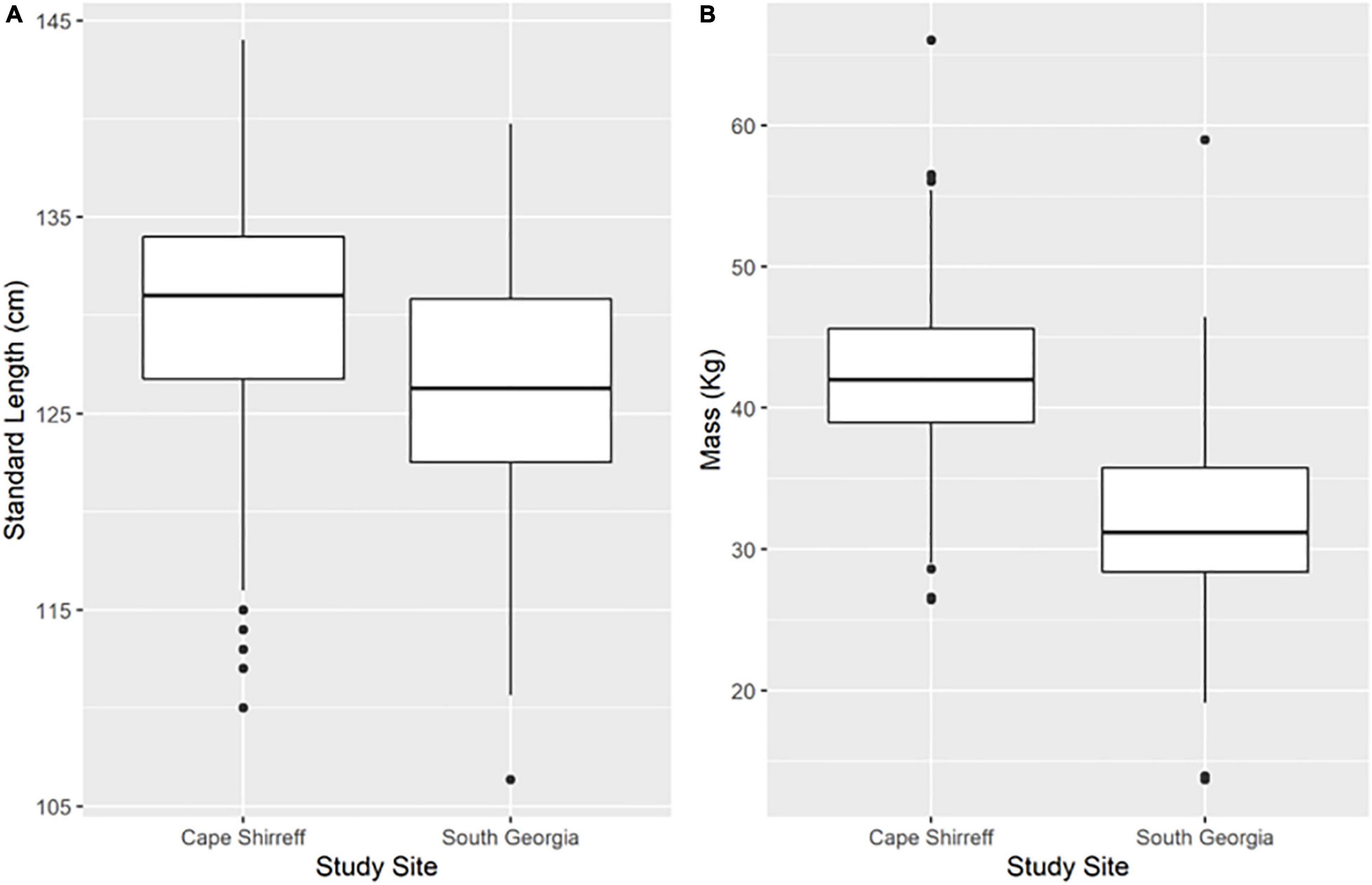
Figure 2. The (A) standard length and (B) mass values from adult Antarctic fur seals measured at South Georgia (NStandard length = 190, Nmass = 166) and Cape Shirreff (NStandard length = 376, Nmass = 377) between 1973–74 and 2000–2020, respectively. Boxes indicate upper and lower quartiles, horizontal lines indicate median values, whiskers represent 10th and 90th percentiles, and dots represent outliers.
South Shetland Islands Population Trends
All known, synoptic counts of AFS born in the South Shetland Islands archipelago, including from Cape Shirreff and the San Telmo Islets, are listed in Supplementary Table 1 (Figure 3). From pup counts, we estimated the total population of all ages using the ratio 1:4.1 (Payne, 1979). The highest pup production for the entire South Shetlands population was measured in 2002 at 10,057 pups born, or 41,233 total AFS. The most recent count from the San Telmo Islets (333 pups in 2019) represents a 90% reduction in pup production since a peak in 1997 (Krause and Hinke, 2021). Similarly, pup production at Cape Shirreff has decreased by 86% since 2007 (Table 1).
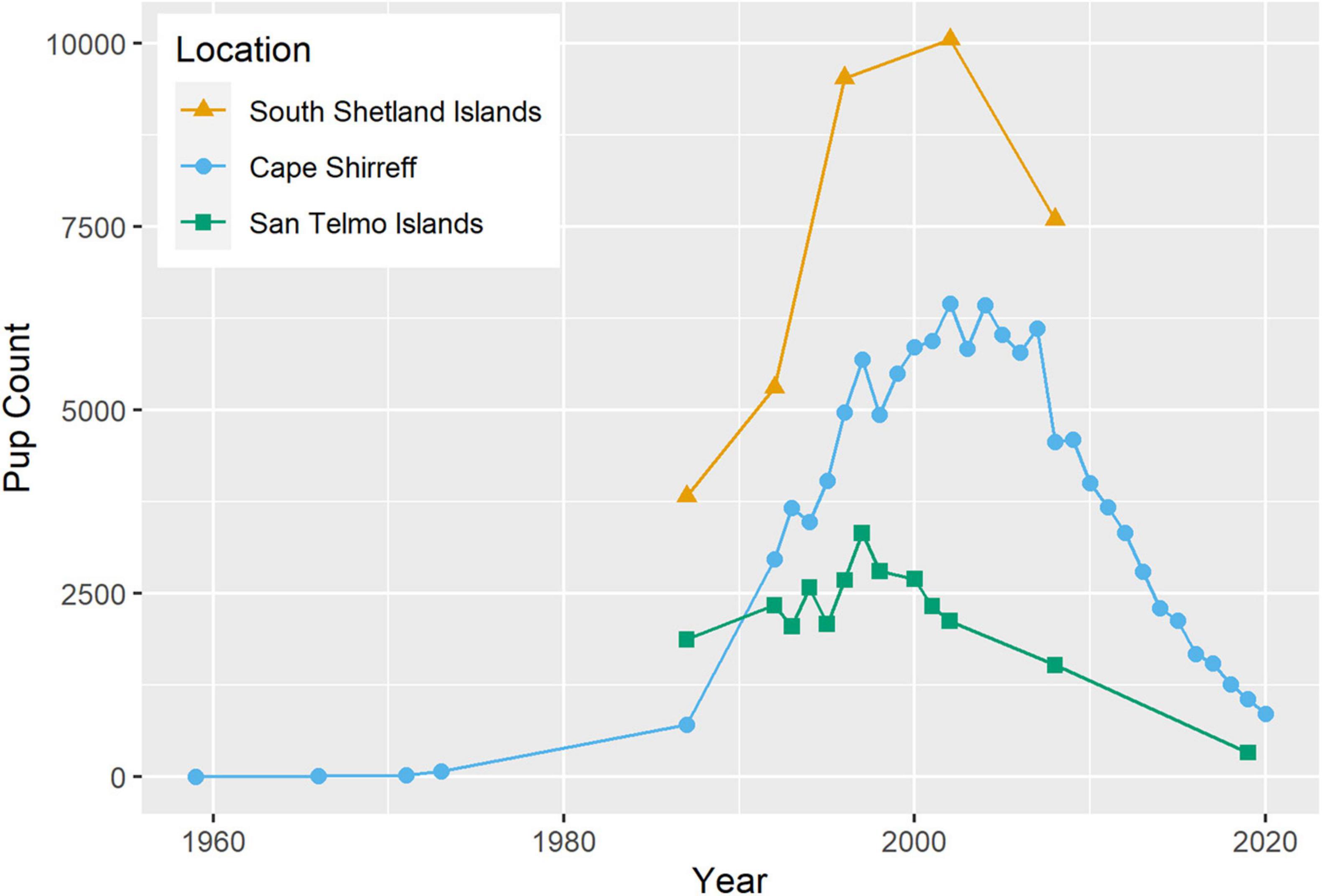
Figure 3. Total synoptic pup counts (live and dead) from the South Shetland Islands archipelago (gold triangles), and from the subset populations at Cape Shirreff (blue circles) and the San Telmo Islets (green squares), between the 1959 and the 2020 Antarctic seasons. Raw data and associated citations listed in Supplementary Table 1.
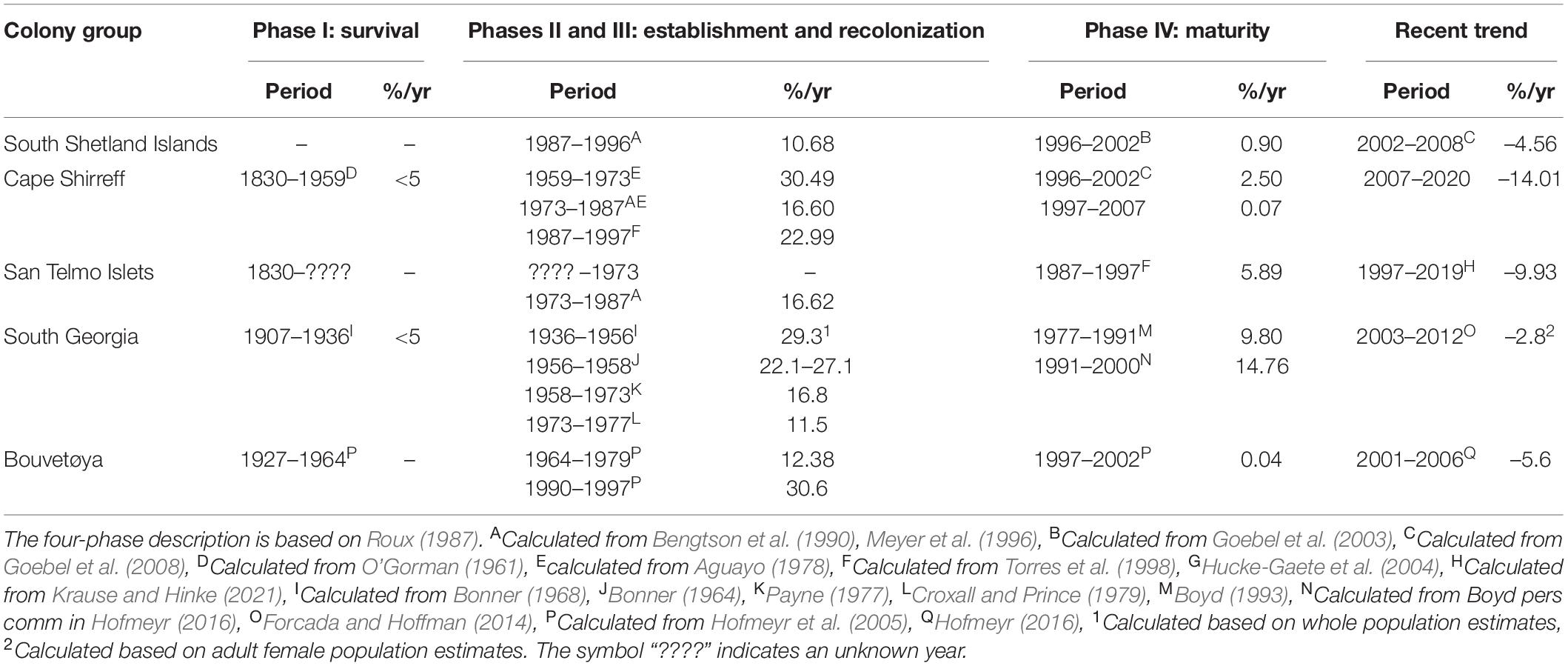
Table 1. The time periods and associated rates of population change (% per annum), based on annual pup production unless otherwise indicated, for AFS in several population centers.
Drivers of Population Change
Age-Specific Adult Female Return Rates
The rates at which adult females at Cape Shirreff survived the winter and successfully returned to the breeding colony decreased between 2001 and 2020. This was the case for the entire population (N = 3,149, slope = −0.66%/year, p = 0.003) and for prime-age females only (N = 2,154, slope = −0.75%/year, p = 0.004, Figure 4A).
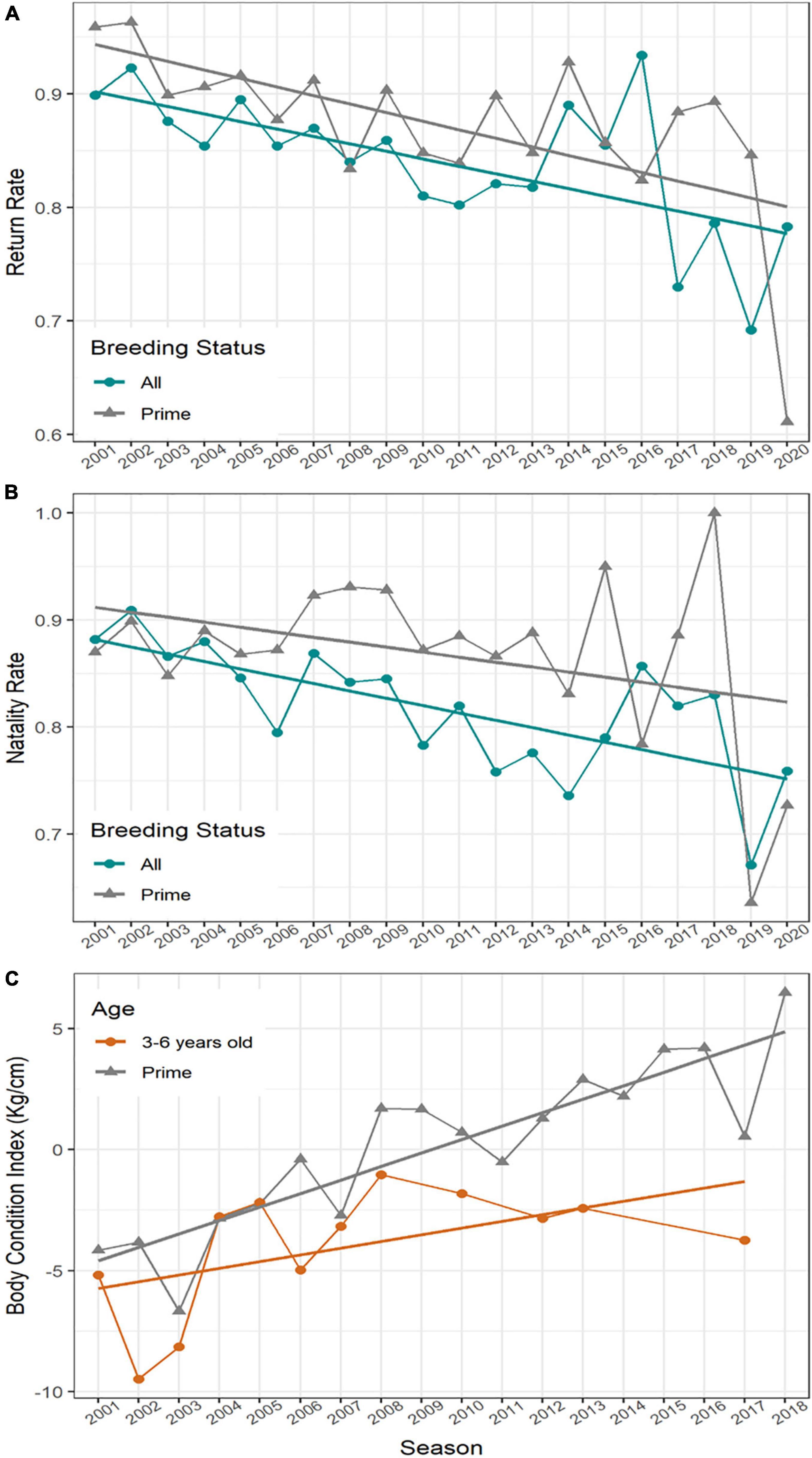
Figure 4. Proportions of (A) all ID-tagged adult female Antarctic fur seals that departed the breeding colony at Cape Shirreff the previous season that returned per annum, (B) all ID-tagged adult females present that pupped in a given season, between 2001 and 2020 at Cape Shirreff, (C) Mean Body Condition Index at colony arrival for adult female Antarctic fur seals at Cape Shirreff during each season between 2001 and 2018. Prime age females (gray triangles) are between 7 and 17 years old; non-prime age females (orange circles) are between 3 and 6 years old.
Age-Specific Natality Rates
The proportion of adult females that returned to Cape Shirreff and pupped in a given season significantly decreased over the study period (NFemales observed = 3,060, slope = −0.69%/year, p < 0.001). However, when only prime-age females were considered, there was no significant trend over the study period (N = 1,917, slope −0.47%/year, p = 0.133, Figure 4B). The mean natality rates between 2001 and 2020 were = 81.7 ± 5.8%, and = 86.7 ± 7.9% for all females, and only prime-age females, respectively.
Body Condition Index
The regression of AFS body mass (M) on standard length (sl) used to calculate BCI, M = −33.02 + 0.627 × sl, was highly significant (N = 624, p << 0.001), and the arrival BCI of females included in our attendance study increased over the study period. This trend was significant for both prime-aged (N = 419, slope = 0.55 BCI/year, p << 0.001), and females aged 3–6 years (N = 46, slope = 0.36 BCI/year, p = 0.01, Figure 4C). None of the females captured and measured during the 2019 and 2020 seasons were of known-age and therefore were not included in calculations of BCI.
Foraging Trip Lengths
Mean foraging trip lengths from 2003 ( = 170.1 ± 4.5 h) were anomalously long (>5.5 standard deviations above the long term mean), and were unique during the 20-year study period. As such, 2003 trip lengths are an overly influential outlier in the regression analysis (Cook’s D >> 1). Therefore, we present our analysis with and without data from 2003 included. The mean foraging trip length over the study period was = 84.79 ± 15.31 h (Nfemales = 484, NTrips = 2,904) and ranged from 54.99 to 112.03 h without data from 2003, and = 89.05 ± 16.72 h with data from 2003 (N2003females = 15, N2003Trips = 90, range2003 = 56.64–333.06 h). With 2003 excluded, mean trip length per season increased between 2001 and 2020 (slope = 1.38 h/year, p = 0.022, Figure 5A), however there was no linear trend when data from 2003 were included (p = 0.761). The incidence of seasons with extremely short foraging trips decreased from 50% in the first half of the study ( = 75.54 ± 14.01 h) to 0% in the second half ( = 95.16 ± 8.61 h, Figure 5B).
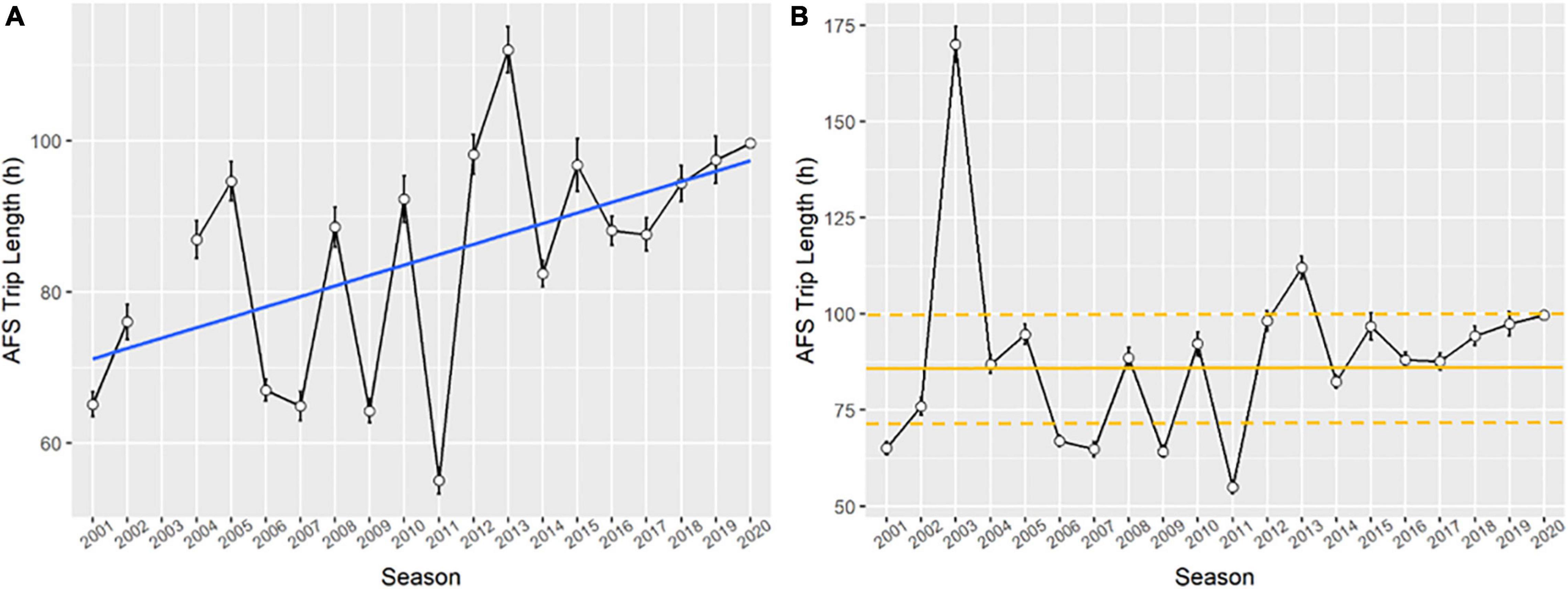
Figure 5. Mean foraging trip lengths from the first 6 trips in a given season by attendance study AFS females from Cape Shirreff (A) excluding 2003 data (Nfemales = 484, NTrips = 2,904), the blue line is a significant (P = 0.022) regression, trip length = -2692 + 1.38 season; and (B) including the anomalously long foraging trip lengths from 2003, the solid orange line marks the mean trip length and dashed orange lines mark standard deviation lines for the entire study period.
Population Age Structure
The mean age of all adult females present within a season increased steadily throughout the study period (N = 3,044, Adjusted R2 = 0.932, p << 0.001, Figure 6A). The overall average age across the study period was = 13.39 ± 1.79 years, increasing from 10.48 ± 3.63 years in 2001 to 17.03 ± 4.86 years in 2018.
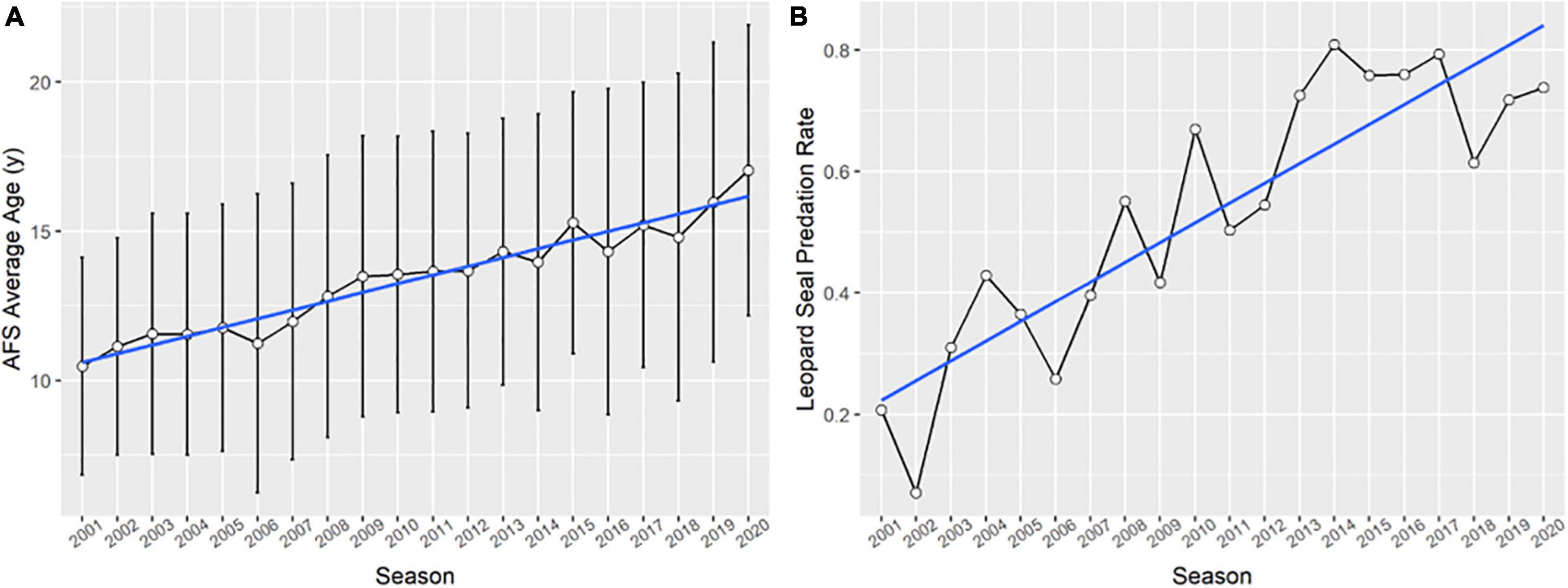
Figure 6. (A) The mean age, with standard deviation bars, of all adult Antarctic fur seal females present at Cape Shirreff in a given season. (B) The proportion of individually-monitored Antarctic fur seal pups born in a given year at Cape Shirreff that were consumed by leopard seals. All panels cover the period between 2001 and 2020.
Pup Mortality
We individually tracked the within-season fates of 2,454 (annual = 127.7 ± 54.1, range: 28–197) AFS pups over the study period. On average relatively few pups were lost to neonate mortality per annum ( = 4.7 ± 3.2%, range: 0.03–11.0%), while most were likely consumed by leopard seals ( = 53.2 ± 21.8%, range: 7.1–80.9%). The percentage of pups dying from all other sources (e.g., starvation after 21 days postpartum, potential disease, or seabird predation) averaged 0.05%, and was never higher than 2.3%. Pup mortality due to leopard seal predation increased over the study period (slope = 3.2%/year, p << 0.001, Figure 6B) and since 2010 pups were more likely to be eaten by leopard seals than not ( = 69.3%, range: 50.3–80.9%). The rate of predation rapidly increased in the early 2000s, passing 40% during the 2004 season; 4 years later, or the typical length of time for pups born in 2004 to recruit into the population, both recruitment and the number of pups born drastically decreased (Figure 7 and Supplementary Table 2).
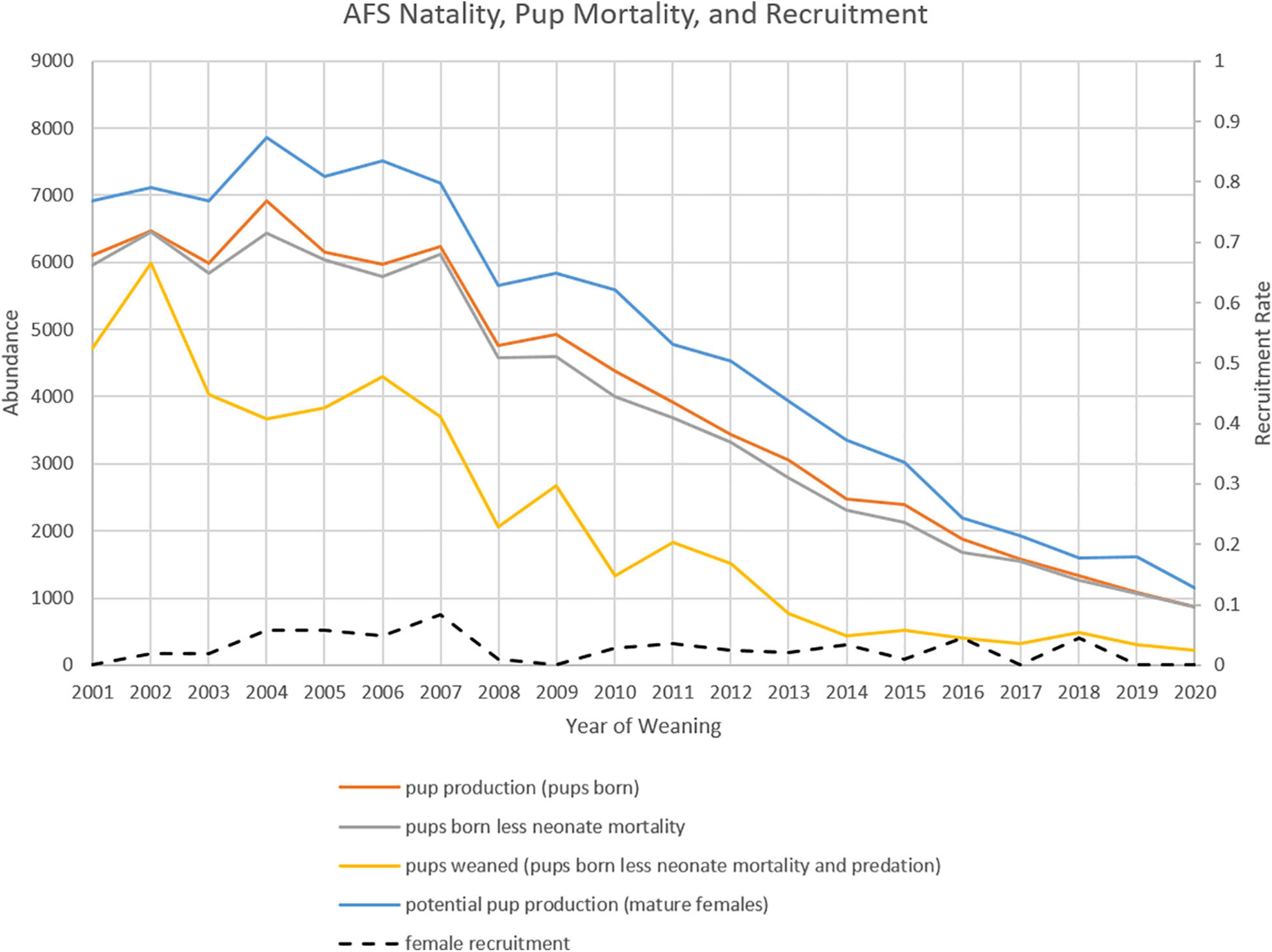
Figure 7. The number of adult Antarctic fur seal females (blue line), pups born (orange line), pups surviving through late December (gray line), pups surviving until weaning (yellow line), and proportion of primiparous females recruited into the population, estimated from the annual Cape Shirreff synoptic pup census and the natality, neonate mortality, and predation mortality rates from the study population. Raw data in Supplementary Table 2.
Discussion
Antarctic fur seals are key indicator species within and surrounding the Antarctic ecosystem. Therefore, monitoring population trends of this species and understanding past and current patterns of genetic variation are essential for Antarctic ecosystem management. Here we review the results of previously published studies indicating that the AFS population at SSI is distinct, and potentially vulnerable to a dramatic decline in abundance. We then provide a summary of morphometric, life history, and population trend data collected over two decades in the SSI, which reinforce the unique evolutionary trajectory of the SSI population and indicate its imminent collapse after a post-sealing recovery. We emphasize that the loss of the SSI AFS population would have a disproportionately large negative impact on the genetic diversity of the species, and reduce the species’ resilience to climate change.
Antarctic Fur Seal Population Structure
The current conservation paradigm for AFS postulates that an expanding population from South Georgia (SG) was the main source of pioneers that recolonized other subantarctic and Antarctic islands (Laws, 1973; Payne, 1977; Hucke-Gaete et al., 2004; Hofmeyr, 2016). However, recent genetic studies largely reject this hypothesis. Instead, a genetic break between eastern and western stocks, first postulated by Wynen et al. (2000), was unequivocally confirmed by subsequent studies utilizing nuclear DNA markers (Humble et al., 2018; Cleary et al., 2019, 2021) showing high levels of differentiation (Paijmans et al., 2020). Distinct clusters correspond to populations in the west and the east (Kerguelen, Heard, and Macquarie Islands; Humble et al., 2018; Paijmans et al., 2020). Additionally, a further sub-division of the “west stock” into three genetically distinct populations breeding at SG, Bouvetøya, and SSI was verified by studies utilizing a combination of mtDNA and microsatellites (Bonin et al., 2013) or microsatellites and genome-wide SNPs (Humble et al., 2018; Cleary et al., 2019; Paijmans et al., 2020). Within the west, 97% of pups from SG and SSI could be correctly assigned to their birth location without a priori knowledge, and assignment probabilities were typically >80% (Bonin et al., 2013). In contrast to the current single source hypothesis, Paijmans et al. (2020) explained these patterns of genetic differentiation by suggesting that several relict populations in the east and west survived sealing, including at the San Telmo Islets in the SSI. While some immigration occurred between regions, the recoveries and current patterns of genetic diversity were driven by these local, relict populations.
Analyses of population structure for AFS at finer scales based on FST estimates from pre-weaned pups, a traditional measure of population differentiation, demonstrate moderate levels of genetic differentiation between SG (n = 266) and SSI (n = 119) (nuclear FST = 0.014, 95%, CI 0.010–0018; mtDNA ΦST = 0.048, p = 0.00098; Bonin et al., 2013). These differentiation estimates are modest relative to other otariid species, but it is important to interpret such estimates within either an evolutionary or a conservation context (Waples and Gaggiotti, 2006). Steller sea lions (Eumetopias jubatus) are managed as distinct east and west stocks based on higher differentiation estimates (FST = 0.06–0.14, Φst = 0.18–0.26; O’Corry-Crowe et al., 2014), but on an evolutionary time scale these stocks have diverged enough to be considered separate sub-species (Phillips et al., 2009). In contrast, mid-range FST values can be biologically meaningful for conservation when combined with demographic (Lang et al., 2021) and observational data (Lowe and Allendorf, 2010). For instance, California sea lions in Mexico (Zalophus californianus) are managed as three stocks: Upper Gulf of California, Southern Baja Peninsula, and Upper Pacific Coast (FST = 0.023 95% CI 0.018–0.029), a decision largely driven by observations of reductions to the Gulf population (González-Suárez et al., 2009). Given the divergent population trajectories and drivers between the AFS breeding populations considered here, we suggest that the four populations should similarly be managed as distinct subpopulations.
Inter-Regional Body Size Comparison
As the highest latitude breeding population of otariids on the planet, SSI AFS face elevated thermal stress due to their extremely cold environment. These fur seals face the coldest sea and air temperatures experienced by any population. Comparisons of their foraging behavior with close relatives suggest that AFS exist near their physiological limits (Costa et al., 1989). Common strategies for marine mammals adapting to colder conditions are to consume more (Reid and Croxall, 2001) or higher quality food (Pagano et al., 2018) over short time scales, or to increase body size over evolutionary time scales (Berta et al., 2018). We tested for differences in the mass and standard length of adult female AFS in late summer between the SSI and SG populations and found females from SSI were both more massive and longer than the SG animals. Given the substantial differences in colony sizes, environmental factors, and resource competition between these sites, size differences should be interpreted with caution. In large populations, like SG, density-dependent forces can reduce body size (Eberhardt, 1977), or competition for food can lead to lower mean mass. However, in the mid-1970s the SG population was expanding rapidly, therefore such density-dependent and local competition effects were unlikely. Further, standard length provides an effective and simple metric to differentiate between genetically distinct breeding stocks within a species (Sweeney et al., 2015). Further comparisons with larger sample sizes and other measurements should be conducted in the future. However, the morphometric differences described here indicate an adaptation to colder conditions since population divergence (Cleary et al., 2021) and support the robust genetic evidence that SSI AFS are genetically distinct from those at SG.
Reevaluating the Antarctic Fur Seal Recolonization Hypothesis
In addition to various population-growth models, a variety of concepts have historically been applied to describe and predict post-exploitation recolonization processes by pinnipeds (Bonin, 2021). Roux (1987) proposed a phased system for fur seals. Phase I, or survival, describes the period when individuals exist in a given region, but at low densities that prevent effective population growth (<5% increase per annum). Phase II, or establishment, is characterized by the formation of observable breeding colonies; however, densities and therefore population increase, remain relatively low (<10% per annum). Phase III, or recolonization, describes a positive density-dependent mechanism where core colonies reach maximum density and drive “spill over” from highly populated colonies to nearby, unoccupied sites (Gaggiotti et al., 2002; Bonin, 2021). This process allows young breeders to escape harassment and increase their chance of successful mating (Grandi et al., 2008). Rates of population increase in the recolonization phase are nominally highest because substrate and other resources are not limiting (15–25% per annum). Finally, Phase IV, or maturity, describes the point at which resources (e.g., substrate, prey) become limiting, thereby reducing the rate of increase.
The phased system of recolonization provides a useful categorization for commonly observed demographic processes in an expanding population (Roux, 1987); however, over-interpretation of population data, in the absence of phase-specific genetic analyses, can be problematic. In particular, the low numbers of individuals and often less reliable data from early phases can easily be misinterpreted. For example, AFS surveys from Cape Shirreff before 1980 indicated rates of population increase beyond what might be biologically possible without immigration (>25% per annum). Previous researchers thus inferred that the SSI AFS population was receiving immigrants from the rapidly recovering population at SG (Laws, 1973; Aguayo, 1978), especially between 1966 and 1973 (Hucke-Gaete et al., 2004). However, pre-1980 estimates of population growth rates (Table 1) were based on very few individuals (12–83 pups, Supplementary Table 1), and the surveys conducted during that period did not include the San Telmo Islets, which are within 1 km, and were later shown to produce more pups than Cape Shirreff until the 1990s (Croll et al., 1992). Although AFS adult females are highly philopatric, dispersal from high-density breeding beaches to adjacent, low-density beaches is common (Roux, 1987; Boyd, 1993; Bonin, 2021). Further, movement of breeding females between the San Telmo Islets and Cape Shirreff has been observed (Hucke-Gaete et al., 2004), suggesting that the earliest estimates of population growth may have reflected movement within the SSI rather than from SG, over 1,500 km away. Density-dependent population growth from within the SSI is consistent with available genetic evidence.
While some gene flow exists between SG and SSI, more individuals likely survived sealing in the SSI than were previously estimated. In addition to genetic evidence, this hypothesis is supported by historical observations. Over 50% of SSI fur seals possess mtDNA haplotypes not found in individuals sampled at South Georgia (Bonin et al., 2013), which is also reflected in neutral variation for nuclear DNA (Bonin et al., 2013; Humble et al., 2018; Paijmans et al., 2020). Additionally, historical reports describe AFS populations surviving post-sealing in at least three geographically distinct areas across the southwest Atlantic sector of the Southern Ocean. Following several waves of hunting between 1790 and 1907 at SG viable breeding populations survived at Bird and Willis Islands (Bonner, 1968). A small population also survived near Bouvetøya which almost certainly did not immigrate from SG (Hofmeyr et al., 2005 and references therein). Finally, a viable population likely also persisted in the SSI over the last 200 years. Sealers discovered the SSI AFS population in 1819 and exploited it rapidly thereafter. At least 47 vessels sealed the area in 1821 and the exploitation rate was so high that many of the 44 vessels which returned in 1822 left empty (Bonner, 1968). During those two seasons an estimated 320,000 fur seals were killed in the SSI, and at least 100,000 pups starved (Weddell, 1970). An expedition conducted a few years later reported no AFS present (Webster, 1834). However, the SSI population did survive in “a few secluded and inhospitable places,” and 18,000 furs were harvested in the SSI between 1871 and 1873 (Williams, 1888). Again, this early “recovery” was almost certainly not driven by immigration from SG, where five sealing vessels managed to secure only 600 pelts in 1871 (Bonner, 1968). We hypothesize that the same inaccessible, and possibly cryptic, AFS colonies in the SSI that seeded population recovery in the 1870s did so again in the 1950s.
Extreme Genetic Diversity at the Edge
The existence of one or more refugia in the SSI not only fueled recolonization, but also preserved high levels of genetic diversity within the species as a whole (Paijmans et al., 2020; Bonin, 2021). In general, “edge” populations are crucial for stabilizing population growth and stability (Rehm et al., 2015). In the face of climate change, a species’ fate may be largely determined by the responses of populations like SSI AFS, which can be described as “long-term stores of diversity” (Hampe and Petit, 2005). A model of genetic variation (Stoffel et al., 2018) indicated that the SSI AFS only recovered from sealing without a severe genetic bottleneck because sealing was not prolonged (i.e., >20 generations) and was less extreme (i.e., leaving an effective population size <50) than previously thought (Paijmans et al., 2020). While sealing was opportunistic and ceased when no longer cost-effective, climate change will be prolonged and potentially irreversible. Indices of genetic diversity are comparable between SG and SSI, despite the fact that the SSI population is two orders of magnitude smaller than SG (Bonin et al., 2013). Therefore, as a population, SSI AFS harbor exceptional genetic diversity for the species. Collectively, these studies indicate that a collapse of the SSI population would cause a disastrous loss of genetic diversity. Such diversity is worth conserving if decision makers and stakeholders wish to promote resilience and future recolonization potential for this species.
South Shetland Islands Population Trends
Following decades of recovery, the two largest AFS populations, at SG and Bouvetøya, have been in moderate decline since the early 2000’s (Table 1). Several smaller breeding populations in East Antarctica are believed to be stable, but have not been assessed recently (Shaughnessy and Goldsworthy, 1990; Hofmeyr et al., 2006; Goldsworthy et al., 2009; Wege et al., 2016). After its survival and establishment phases (Table 1), the SSI AFS population recovered rapidly reaching an estimated total population of 41,233 individuals in 2002. Although the abundance of SSI AFS was an order of magnitude lower than its pre-exploitation level, recovery of the population slowed in the late 1990s, and is now in steep decline (Figure 3 and Table 1), having decreased by 86% since 2007.
Marine top predators are vital to ecosystem function. Many of these predators are highly mobile consumers that connect marine food webs in different regions, and the populations of these predators are responding to climate change in complex ways (Bestley et al., 2020). For example, the decline of AFS in the southwest Atlantic differed from expectations of stable or increasing populations due to a reduction in thermal stress and expanded habitat caused by regional warming (Siniff et al., 2008; Costa et al., 2010).
Drivers of Population Change
Regional declines in the abundances of large marine predators may arise for several reasons, including emigration, increased mortality, or reduced reproduction. AFS are highly philopatric (Payne, 1977; Hoffman and Forcada, 2012), therefore it is unlikely that the rapid decline in pup production in the SSI is due to emigration of adult females. However, with rapid changes in the regional climate, increases in competition for and the redistribution of krill may control pinniped reproduction rates (Costa et al., 2010); multiple lines of evidence suggest such bottom-up dynamics are limiting the SSI AFS population.
Bottom-Up
The population declines in recent decades at SG are likely being driven by reduced krill availability due to climate change (Forcada et al., 2005; Forcada and Hoffman, 2014). Similar food-limitation dynamics may be occurring in the SSI. Population models based on data collected at Cape Shirreff before 2006 suggested resource limitation in the late 1990s (Hucke-Gaete et al., 2004), and climate-induced reduction of adult vital rates in the early 2000s (Schwarz et al., 2013). While trends in available krill biomass within the SSI region are debated (Kinzey et al., 2015, 2019; Cox et al., 2018; Hill et al., 2019), it is clear that climate change is affecting the availability of krill to predators (Atkinson et al., 2019). Krill is a crucial diet component for both AFS and leopard seals (Krause et al., 2020), however, as central place foragers, breeding AFS females have limited ability to adjust their feeding grounds. Changes that shift krill away from breeding colonies are harmful and may continue (Klein et al., 2018).
Observations of AFS behavior and diet indicate a reduction in food availability, during the breeding season, since the early- to mid-2000s. The foraging trip lengths of adult female AFS reflect prey availability (McCafferty et al., 1998), where longer trips result in lower female body condition, slower pup growth, and lower pup survival (Boyd et al., 1994; Vargas et al., 2009; Hiruki-Raring et al., 2012). The anomalously long foraging trip lengths during 2003 provided an opportunity to assess long-term trends and learn how AFS respond to decadally poor conditions. When trip lengths from 2003 were excluded, trip lengths from Cape Shirreff increased over the last 20 years (Figure 5A). Irrespective of that trend, the historically common extremely short foraging trip length years (i.e., very good prey availability) have disappeared entirely (Figure 5A). Further, observations from the 2003 AFS diets emphasize other reductions in AFS prey availability.
Although AFS are dependent on krill as their primary food source, fish are an important alternative (Polito and Goebel, 2010). During 2003, female AFS compensated for low krill availability by switching to myctophid fishes. In fact, analysis of AFS scats collected during February 2003 showed a higher frequency of fish than krill for the only instance in over 20 years (Goebel et al., 2003). A recent analysis of otoliths from scat samples collected between 2000 and 2015 showed a decline in the frequency of occurrence, and of the mean age, of myctophid fishes in AFS diets, indicating a reduction in the availability of this alternative prey source as well (Klemmedson et al., 2020).
The population dynamics of AFS appear more sensitive to climate variation than other Antarctic seabirds and mammals at SG in recent decades (Forcada et al., 2008). Mark-recapture analysis of AFS that breed at Cape Shirreff in the early 2000s indicated that adult female survival and natality were weakly related to climatic variability (Schwarz et al., 2013). Indeed, over the last 20 years the return rates (i.e., survival) of adult female AFS have consistently decreased (Figure 4A). Natality rates at Cape Shirreff also declined over the study period, but when we only considered prime-aged females there was no trend (Figure 4B). The mean age of AFS females at Cape Shirreff increased over the study period, and was always substantially higher than values reported from growing populations elsewhere (e.g., 7.41 years, at SG during the mid-1970s, Payne, 1977). Therefore, the decrease in natality rates observed at Cape Shirreff was likely due to senescence in the aging population (Figure 6A), driven by poor female recruitment (Figure 7; York, 1987). Finally, neonate mortality rates have been low at Cape Shirreff (Hucke-Gaete et al., 2004, this study), indicating that the colony-density effects seen at SG (Doidge et al., 1984) and Bouvetøya (Hofmeyr et al., 2005) have not affected the population dynamics of SSI AFS during the recovery phase.
The over-winter survival and natality rates of adult female AFS are also heavily influenced by the physical conditions and food availability in their winter foraging grounds (Guinet et al., 1998). While SSI AFS forage locally during the summer breeding season, adult females travel thousands of kilometers and forage off the east and west coasts of South America during the winter (Arthur et al., 2017; Hinke et al., 2017). Winter food availability determines if a female can simultaneously compensate for the nutritional stress of the previous breeding season and support her developing fetus. Winter food availability is reflected in female body condition upon arrival to the breeding colony (Lunn and Boyd, 1993; Hiruki-Raring et al., 2012). Age plays a role in body condition as well. As AFS age, they both grow and gain more experience, and their size and body condition increase in kind. Females over 6 years are consistently larger and in better condition than their younger conspecifics (Lunn and Boyd, 1993). The body condition index (BCI) of AFS females arriving to Cape Shirreff significantly improved over the study period (Figure 4C), even for young, non-prime (3–6 years old) females. Some of the positive BCI trend over time may reflect the increased autumn fat stores of females who lost their pups to leopard seal predation during the previous summer. However, the upward trend of young females, including primiparous females that could not have lost pups, suggests that overwinter foraging conditions have been improving. The positive trend in BCI also lends weight to the hypothesis that the rapid population decrease in SSI AFS is being driven by local conditions around the northern Antarctic Peninsula.
Increased competition for a common resource can induce bottom-up population control when the environment limits prey availability while consumer demands increase. Catches taken by the commercial krill fishery have increased (Nicol et al., 2012), and fishing activities overlap in time and space with foraging SSI AFS (Hinke et al., 2017). This fishery is concentrating effort in smaller geographical areas over shorter time periods, which may limit the performance and population growth of krill-dependent predators via competition (Watters et al., 2020; Krüger et al., 2021).
Additionally, the widespread removal of baleen whales throughout the southwest Atlantic by whaling during the early to mid-twentieth century substantially reduced competition for food among krill-dependent seabirds and seals (Laws, 1977; Hodgson and Johnston, 1997; Surma et al., 2014). Conversely, it is likely that strong recoveries by some Antarctic whale populations (Branch, 2011; Pallin et al., 2018; Zerbini et al., 2019) in recent decades are increasing resource competition with other krill-dependent predators (Ballance et al., 2006; Trivelpiece et al., 2011). While little is known about the population sizes and distribution of krill-dependent ice seals in the region, it is likely that crabeater seals are shifting southward along the Antarctic Peninsula away from SSI AFS colonies (Hückstädt et al., 2020). However, the number of krill-dependent, seasonally resident leopard seals rapidly increased in the SSI between the late 1990’s and early 2010s (Goebel et al., 2014; Krause et al., 2015, 2020).
Top-Down
As environmental perturbations propagate through food webs, understanding the drivers of a species’ population dynamics requires an understanding of both bottom-up and top-down influences, particularly changing levels of predation (Fretwell, 1987). Due to their large size, foraging efficiency, and high energetic needs, even a small number of apex predators can substantially affect the population dynamics of other marine carnivores (Williams et al., 2004; Reisinger et al., 2011); particularly when predators target the early life stages of their prey (Munch et al., 2005; Barber-Meyer et al., 2008).
To date the majority of AFS population dynamics research has focused on bottom-up explanations for observed patterns (Payne, 1977; Shaughnessy and Goldsworthy, 1990; Boyd, 1993; Wickens and York, 1997; Hucke-Gaete et al., 2004; Hofmeyr et al., 2005; Ainley et al., 2007; Goldsworthy et al., 2009; Wege et al., 2016), or concluded that top-down forcing was negligible (Forcada et al., 2008, 2009). Although Goldsworthy et al. (2009) concluded that predation of AFS pups by New Zealand sea lions (Phocarctos hookeri) was limiting AFS population growth at Macquarie Island.
However, recent changes in the summer foraging locations and prey choices of some leopard seals around the northern Antarctic Peninsula have created dynamics not seen elsewhere. A growing body of evidence indicates that predation of AFS pups by leopard seals is decreasing the SSI population from the top-down. Perhaps as a result of drastic reductions in their preferred ice habitat (Forcada et al., 2012), the numbers of seasonally resident leopard seals have substantially increased at SSI AFS colonies in recent decades (Krause et al., 2015). While no more than two leopard seals were seen foraging concurrently at Cape Shirreff before 1996 (Weddell, 1970; Boveng et al., 1998), their numbers rose rapidly between 1998 and 2011 (Vera et al., 2005; Goebel et al., 2014). Between 2011 and 2020 the maximum number of leopard seals observed foraging concurrently at Cape Shirreff averaged 20 (range = 11–41). Fur seal pups appear to be preferentially targeted by large, adult female leopard seals which use specialized hunting tactics to achieve high rates (>92%) of prey capture success (Hiruki et al., 1999; Krause et al., 2015). Between 2013 and 2017 AFS pups alone contributed an estimated 21.3–37.6% of female leopard seal summer diets (Krause et al., 2020). High leopard seal density, focused feeding on AFS pups, and the associated intraspecific competition (Krause et al., 2016), including kleptoparasitism (i.e., prey stealing) and food caching behavior (Krause and Rogers, 2019), have significantly elevated rates of pup mortality at Cape Shirreff.
We have little doubt that top-down controls are currently dominating the population dynamics of AFS at Cape Shirreff. Demographic and population modeling of two SSI AFS colonies demonstrated that mean annual predation rates on pups of 34.4% at Seal Island (Boveng et al., 1998), and 35.8% at Cape Shirreff (Schwarz et al., 2013) were the primary drivers of negative population growth. By comparison, between 2002 and 2020 the rate of pup mortality due to leopard seal predation at Cape Shirreff rose from 7.1 to 73.8%, and has averaged 69.3% (range: 50.3–80.9%) per annum since 2010.
Conclusion
Top-down effects on the recruitment of juveniles into long-lived breeding populations are likely to be greatest during early life stages, while bottom-up effects are detected over longer periods of time (Munch et al., 2005; Hinke et al., 2020). Drawn from a long-term data set of age-specific, and individually tracked behavioral and demographic metrics, multiple lines of evidence indicate both types of control are compounding to force a rapid population crash of the SSI AFS. Further, improved body condition at arrival to the colony, prior to breeding, strongly suggests that the sharp population decline observed at Cape Shirreff is being driven by effects originating around the northern Antarctic Peninsula.
The successful recovery of the AFS species during the twentieth century was supported by the genetic diversity of four relict populations, rather than a putative single stock.
SSI AFS harbor vital genetic diversity for the species, therefore, we assert that its imminent collapse will disproportionally reduce the species’ adaptive capacity to deal with sustained environmental change and growing resource competition. We emphasize that the loss of the SSI AFS population would have a disproportionately large negative effect on the genetic diversity of the species, and reduce the species’ resilience to climate change. In addition to more detailed studies of shifting AFS demographics and prey availability, there is an urgent need to reevaluate the conservation status of Antarctic fur seals, particularly the subpopulation status of the vulnerable and genetically important SSI population.
Data Availability Statement
The original contributions presented in the study are included in the article/Supplementary Material, further inquiries can be directed to the corresponding author/s.
Ethics Statement
All research at Cape Shirreff described herein was conducted in accordance with Marine Mammal Protection Act Permit Nos. 1024, 774-1649, 774-1847, 16472, and 20599, Antarctic Conservation Act Permit Nos. 97-016, 2002-007, 2007-003, 2012-005 and 2017-012, and NMFS-SWFSC Institutional Animal Care and Use Committee Permit Nos. IACUC SWPI2011-02, SWPI 2014-03, and SWPI 2017-03.
Author Contributions
MG designed the study with support from CB and DK. DK analyzed the data with advice from GW, CR, and MG. DK, CB, and GW wrote the manuscript with input from MG and CR. MG, DK, and CB was completed field work. All authors read and approved the final manuscript.
Funding
Principle funding for this research was provided by the NOAA U.S. AMLR Program, CB was supported by NSF HRD 2000211 and NOAA-LMRCSC-FY2016 Award # NA16SEC4810007.
Conflict of Interest
The authors declare that the research was conducted in the absence of any commercial or financial relationships that could be construed as a potential conflict of interest.
Publisher’s Note
All claims expressed in this article are solely those of the authors and do not necessarily represent those of their affiliated organizations, or those of the publisher, the editors and the reviewers. Any product that may be evaluated in this article, or claim that may be made by its manufacturer, is not guaranteed or endorsed by the publisher.
Acknowledgments
We send a heartfelt thank you to the dozens of dedicated U.S. AMLR biologists, technicians, and support staff, and our colleagues at the Instituto Antártico Chileano (INACH) without whom this work would not be possible. Voluminous assistance from S. Woodman with data cleaning and organization is deeply appreciated. This manuscript was improved by comments from A. Lang and S. Woodman. We also thank D. Losey for extensive support with library research.
Supplementary Material
The Supplementary Material for this article can be found online at: https://www.frontiersin.org/articles/10.3389/fmars.2021.796488/full#supplementary-material
References
Agnew, D. J. (1997). Review: the CCAMLR ecosystem monitoring programme. Antarct. Sci. 9, 235–242. doi: 10.1017/S095410209700031X
Aguayo, L. A. (1978). The present status of the Antarctic Fur Seal Arctocephalus gazella at South Shetland Islands. Polar Rec. 19, 167–173. doi: 10.1017/S003224740000190X
Ainley, D., Ballard, G., Ackley, S., Blight, L., Eastman, J., Emslie, S., et al. (2007). Paradigm lost, or is top-down forcing no longer significant in the Antarctic marine ecosystem? Antarct. Sci. 19, 283–290. doi: 10.1017/s095410200700051x
Arnbom, T. A., Lunn, N. J., Boyd, I. L., and Barton, T. (1992). Aging live Antarctic fur seals and southern elephant seals. Mar. Mamm. Sci. 8, 37–43. doi: 10.1111/j.1748-7692.1992.tb00123.x
Arthur, B., Hindell, M., Bester, M., De Bruyn, P. J. N., Trathan, P., Goebel, M., et al. (2017). Winter habitat predictions of a key Southern Ocean predator, the Antarctic fur seal (Arctocephalus gazella). Deep Sea Res. II Top. Stud. Oceanogr. 140, 171–181. doi: 10.1016/j.dsr2.2016.10.009
Atkinson, A., Hill, S. L., Pakhomov, E. A., Siegel, V., Reiss, C. S., Loeb, V. J., et al. (2019). Krill (Euphausia superba) distribution contracts southward during rapid regional warming. Nat. Clim. Change 9, 142–147. doi: 10.1038/s41558-018-0370-z
Atkinson, A., Siegel, V., Pakhomov, E. A., Jessopp, M. J., and Loeb, V. (2009). A re-appraisal of the total biomass and annual production of Antarctic krill. Deep Sea Res. I Oceanogr. Res. Pap. 56, 727–740. doi: 10.1016/j.dsr.2008.12.007
Ballance, L. T., Pitman, R. L., Hewitt, R. P., Siniff, D. B., Trivelpiece, W. Z., Clapham, P., et al. (2006). “The removal of large whales from the southern ocean: evidence for long-term ecosytem effects?,” in Whales, Whaling, and Ocean Ecosystems, eds D. P. D. James, A. Estes, D. F. Doak, T. M. Williams, and R. L. Brownell (California, IL: University of California Press, Ltd), 215–230.
Barber-Meyer, S. M., Mech, L. D., and White, P. J. (2008). Elk calf survival and mortality following wolf restoration to yellowstone national park. Wildlife Monogr. 169, 1–30. doi: 10.2193/2008-004
Bengtson, J. L., Ferm, L. M., Härkönen, T. J., and Stewart, B. S. (1990). “Abundance of antarctic fur seals in the South Shetland Islands, Antarctica, During the 1986/87 Austral Summer,” in Antarctic Ecosystems, ed. K. R. Kerry (Berlin: Springer), 265–270. doi: 10.1007/978-3-642-84074-6_30
Berta, A., Churchill, M., and Boessenecker, R. W. (2018). The origin and evolutionary biology of pinnipeds: seals, sea lions, and walruses. Annu. Rev. Earth Planet. Sci. 46, 203–228. doi: 10.1146/annurev-earth-082517-010009
Bestley, S., Ropert-Coudert, Y., Bengtson Nash, S., Brooks, C. M., Cotté, C., Dewar, M., et al. (2020). Marine ecosystem assessment for the Southern Ocean: birds and marine mammals in a changing climate. Front. Ecol. Evol. 8:566936. doi: 10.3389/fevo.2020.566936
Bonin, C. A. (2021). “Genetic consequences of dispersal, philopatry and reproductive behaviors,” in Ethology and Behavioral Ecology of Otariids and the Odobenid, eds C. Campagna and R. Harcourt (Berlin: Springer International Publishing), 223–241. doi: 10.1007/978-3-030-59184-7_11
Bonin, C. A., Goebel, M. E., Forcada, J., Burton, R. S., and Hoffman, J. I. (2013). Unexpected genetic differentiation between recently recolonized populations of a long-lived and highly vagile marine mammal. Ecol. Evol. 3, 3701–3712. doi: 10.1002/ece3.732
Bonner, W. N. (1964). “Population increase in the fur seal, Arctocephalus tropicalis gazella, at South Georgia,” in Biologie antarctique: Proceedings of the first symposium organised by SCAR 1962, Hermann. doi: 10.1046/j.1365-294x.2000.00856.x
Boveng, P., Hiruki, L., Schwartz, M., and Bengtson, J. (1998). Population growth of Antarctic fur seals: limitation by a top predator, the leopard seal? Ecology 79, 2863–2877. doi: 10.1890/0012-9658(1998)079[2863:pgoafs]2.0.co;2
Boyd, I. (1993). Pup production and distribution of breeding Antarctic fur seals (Arctocephalus gazella) at South Georgia. Antarct. Sci. 5, 17–24. doi: 10.1017/s0954102093000045
Boyd, I. L. (1999). Foraging and provisioning in Antarctic fur seals: interannual variability in time-energy budgets. Behav. Ecol. 10, 198–208. doi: 10.1093/beheco/10.2.198
Boyd, I. L., Arnould, J. P. Y., Barton, T., and Croxall, J. P. (1994). Foraging behaviour of antarctic fur seals during periods of contrasting prey abundance. J. Anim. Ecol. 63, 703–713. doi: 10.2307/5235
Boyd, I. L., Croxall, J. P., Lunn, N. J., and Reid, K. (1995). Population demography of antarctic fur seals: the costs of reproduction and implications for life-histories. J. Anim. Ecol. 64, 505–518. doi: 10.2307/5653
Boyd, I. L., and McCann, T. S. (1989). Pre-natal investment in reproduction by female Antarctic fur seals. Behav. Ecol. Sociobiol. 24, 377–385. doi: 10.1007/BF00293265
Boyd, I. L., and Murray, A. W. A. (2001). Monitoring a marine ecosystem using responses of upper trophic level predators. J. Anim. Ecol. 70, 747–760. doi: 10.1046/j.0021-8790.2001.00534.x
Boyd, I. L., Wanless, S., and Camphuysen, C. J. (2006). Top Predators in Marine Ecosystems: Their Role in Monitoring and Management. Cambridge, MA: Cambridge University Press.
Branch, T. A. (2011). Humpback whale abundance south of 60°S from three complete circumpolar sets of surveys. J. Cetacean Res. Manag. 3, 53–69. doi: 10.47536/jcrm.vi.305
Childerhouse, S., Dickie, G., and Hessel, G. (2004). Ageing live New Zealand sea lions (Phocarctos hookeri) using the first post-canine tooth. Wildlife Res. 31, 177–181. doi: 10.1071/WR03006
Cleary, A., Hoffman, J., Forcada, J., Lydersen, C., Lowther, A., and Kovacs, K. (2021). 50,000 years of ice and seals: impacts of the last glacial maximum on antarctic fur seals. Ecol. Evol. [Epub ahead of print]. doi: 10.1002/ece3.8104
Cleary, A. C., Bester, M., Forcada, J., Goebel, M., Goldsworthy, S. D., Guinet, C., et al. (2019). Prey differences drive local genetic adaptation in Antarctic fur seals. Mar. Ecol. Prog. Ser. 628, 195–209. doi: 10.3354/meps13108
Costa, D. P., Croxall, J. P., and Duck, C. D. (1989). Foraging energetics of Antartic fur seals in relation to changes in prey availability. Ecology 70, 596–606. doi: 10.2307/1940211
Costa, D. P., Huckstadt, L. A., Crocker, D. E., McDonald, B. I., Goebel, M. E., and Fedak, M. A. (2010). Approaches to studying climatic change and its role on the habitat selection of antarctic pinnipeds. Integr. Comp. Biol. 50, 1018–1030. doi: 10.1093/icb/icq054
Cox, M. J., Candy, S., de la Mare, W. K., Nicol, S., Kawaguchi, S., and Gales, N. (2018). No evidence for a decline in the density of Antarctic krill Euphausia superba Dana, 1850, in the Southwest Atlantic sector between 1976 and 2016. J. Crustacean Biol. 38, 656–661. doi: 10.1093/jcbiol/ruy072
Croll, D., Bengtson, J., Holt, R. S., and Torres, D. (1992). AMLR 1991/92 Field Season Report. Census of Antarctic fur seal colonies of the South Shetland Islands, 1991-92. U.S. Department of Commerce, NOAA Administrative Report LJ-92-17. Washington, DC: NOAA, 82–85.
Croxall, J. P., and Prince, P. A. (1979). Antarctic seabird and seal monitoring studies. Polar Rec. 19, 573–595. doi: 10.1017/S0032247400002680
Dayton, P. K. (1971). Competition, disturbance, and community organization: the provision and subsequent utilization of space in a rocky intertidal community. Ecol. Monogr. 41, 351–389. doi: 10.2307/1948498
DeLong, R. L., Melin, S. R., Laake, J. L., Morris, P., Orr, A. J., and Harris, J. D. (2017). Age- and sex-specific survival of California sea lions (Zalophus californianus) at San Miguel Island, California. Mar. Mamm. Sci. 33, 1097–1125. doi: 10.1111/mms.12427
DeMaster, D., Trites, A., Clapham, P., Mizroch, S., Wade, P., Small, R., et al. (2006). The sequential megafaunal collapse hypothesis: testing with existing data. Prog. Oceanogr. 68, 329–342. doi: 10.1016/j.pocean.2006.02.007
Doidge, D. W., Croxall, J. P., and Baker, J. R. (1984). Density-dependent pup mortality in the Antarctic fur seal Arctocephalus gazella at South Georgia. J. Zool. 202, 449–460. doi: 10.1111/j.1469-7998.1984.tb05095.x
Ducklow, H. W., Fraser, W. R., Meredith, M. P., Stammerjohn, S. E., Doney, S. C., Martinson, D. G., et al. (2013). West Antarctic Peninsula: an ice-dependent coastal marine ecosystem in transition. Oceanography 26, 190–203. doi: 10.5670/oceanog.2013.62
Eberhardt, L. L. (1977). Optimal policies for conservation of large mammals, with special reference to marine ecosystems. Environ. Conserv. 4, 205–212. doi: 10.1017/S0376892900025819
Estes, J., Tinker, M., Williams, T., and Doak, D. (1998). Killer whale predation on sea otters linking oceanic and nearshore ecosystems. Science 282, 473–476. doi: 10.1126/science.282.5388.473
Estes, J. A., Terborgh, J., Brashares, J. S., Power, M. E., Berger, J., Bond, W. J., et al. (2011). Trophic downgrading of planet earth. Science 333, 301–306. doi: 10.1126/science.1205106
Foley, C. M., and Lynch, H. J. (2020). A method to estimate pre-exploitation population size. Conserv. Biol. 34, 256–265. doi: 10.1111/cobi.13416
Forcada, J., and Hoffman, J. I. (2014). Climate change selects for heterozygosity in a declining fur seal population. Nature 511, 462–465. doi: 10.1038/nature13542
Forcada, J., Malone, D., Royle, J., and Staniland, I. (2009). Modelling predation by transient leopard seals for an ecosystem-based management of Southern Ocean fisheries. Ecol. Model. 220, 1513–1521. doi: 10.1016/j.ecolmodel.2009.03.020
Forcada, J., Trathan, P. N., Boveng, P. L., Boyd, I. L., Burns, J. M., Costa, D. P., et al. (2012). Responses of Antarctic pack-ice seals to environmental change and increasing krill fishing. Biol. Conserv. 149, 40–50. doi: 10.1016/j.biocon.2012.02.002
Forcada, J., Trathan, P. N., and Murphy, E. J. (2008). Life history buffering in Antarctic mammals and birds against changing patterns of climate and environmental variation. Glob. Change Biol. 14, 2473–2488. doi: 10.1111/j.1365-2486.2008.01678.x
Forcada, J., Trathan, P. N., Reid, K., and Murphy, E. J. (2005). The effects of global climate variability in pup production of Antarctic fur seals. Ecology 86, 2408–2417. doi: 10.1890/04-1153
Fretwell, S. D. (1987). Food chain dynamics: the central theory of ecology? Oikos 50, 291–301. doi: 10.2307/3565489
Gaggiotti, O. E., Jones, F., Lee, W. M., Amos, W., Harwood, J., and Nichols, R. A. (2002). Patterns of colonization in a metapopulation of grey seals. Nature 416, 424–427. doi: 10.1038/416424a
Gales, N. J. (1989). Chemical restraint and anesthesia of pinnipeds: a review. Mar. Mamm. Sci. 5, 228–256. doi: 10.1111/j.1748-7692.1989.tb00338.x
Gales, N. J., and Mattlin, R. H. (1998). Fast, safe, field-portable gas anesthesia for otariids. Mar. Mamm. Sci. 14, 355–361. doi: 10.1111/j.1748-7692.1998.tb00727.x
Gentry, R., and Holt, J. R. (1982). Equipment and Techniques for Handling Northern Fur Seals. NOAA Technical Report, NMFS SSRF-758. Washington, DC: NOAA.
Goebel, M., McDonald, B., Freeman, S., Haner, R., Spear, N., and Sexton, S. (2008). Pinniped Research at Cape Shirreff, Livingston Island, Antarctica. AMLR 2007/08 Field Season Report. NOAA-TM-NMFS-SWFSC-427. Washington, DC: NOAA.
Goebel, M. E., Pussini, N., Buchheit, R., Pietrzak, K. W., Krause, D. J., Van Cise, A. M., et al. (2014). Pinniped Research at Cape Shirreff, Livingston Island, Antarctica. AMLR 2010/11 Field Season Report. NMFS-SWFSC-524. Washington, DC: NOAA, 50–57.
Goebel, M. E., and Reiss, C. S. (2014). “Squeezed from both ends: decline in Antarctic fur seals in the South Shetland Islands driven by both top-down and bottom-up processes,” in Proceedings of the CCAMLR Working Group on Ecosystem Monitoring and Management, Punta Arenas.
Goebel, M. E., Vallejos, V. I., Trivelpiece, W. Z., Holt, R. S., and Acevedo, J. (2003). Antarctic Fur Seal Pup Production. AMLR 2001/02 Field Season Report. NOAA Technical Memo-NMFS-SWFSC-350. Washington, DC: NOAA, 139–152.
Goldsworthy, S. D., McKenzie, J., Page, B., Lancaster, M. L., Shaughnessy, P. D., Wynen, L. P., et al. (2009). Fur seals at Macquarie Island: post-sealing colonisation, trends in abundance and hybridisation of three species. Polar Biol. 32, 1473–1486. doi: 10.1007/s00300-009-0645-y
González-Suárez, M., Flatz, R., Aurioles-gamboa, D., Hedrick, P. W., and Gerber, L. R. (2009). Isolation by distance among California sea lion populations in Mexico: redefining management stocks. Mol. Ecol. 18, 1088–1099. doi: 10.1111/j.1365-294X.2009.04093.x
Grandi, M. F., Dans, S. L., and Crespo, E. A. (2008). Social composition and spatial distribution of colonies in an expanding population of south american sea lions. J. Mammal. 89, 1218–1228. doi: 10.1644/08-mamm-a-088.1
Guinet, C., Roux, J. P., Bonnet, M., and Mison, V. (1998). Effect of body size, body mass, and body condition on reproduction of female South African fur seals (Arctocephalus pusillus) in Namibia. Can. J. Zool. 76, 1418–1424. doi: 10.1139/z98-082
Hairston, N. G., Smith, F. E., and Slobodkin, L. B. (1960). Community structure, population control, and competition. Am. Nat. 94, 421–425. doi: 10.1086/282146
Hampe, A., and Petit, R. J. (2005). Conserving biodiversity under climate change: the rear edge matters. Ecol. Lett. 8, 461–467. doi: 10.1111/j.1461-0248.2005.00739.x
Haulena, M. (2014). “Otariid seals,” in Zoo Animal and Wildlife Immobilization and Anesthesia, eds G. West, D. Heard, and N. Caulkett (Hoboken, NJ: Blackwell Publishing), 661–672. doi: 10.1002/9781118792919.ch47
Hill, S. L., Atkinson, A., Pakhomov, E. A., and Siegel, V. (2019). Evidence for a decline in the population density of Antarctic krill Euphausia superba Dana, 1850 still stands. J. Crustacean Biol. 39, 316–322. doi: 10.1093/jcbiol/ruz004
Hinke, J. T., Cossio, A. M., Goebel, M. E., Reiss, C. S., Trivelpiece, W. Z., and Watters, G. M. (2017). Identifying risk: concurrent overlap of the antarctic krill fishery with krill-dependent predators in the scotia sea. PLoS One 12:e0170132. doi: 10.1371/journal.pone.0170132
Hinke, J. T., Salwicka, K., Trivelpiece, S. G., Watters, G. M., and Trivelpiece, W. Z. (2007). Divergent responses of Pygoscelis penguins reveal a common environmental driver. Oecologia 153:853. doi: 10.1007/s00442-007-0781-4
Hinke, J. T., Watters, G. M., Reiss, C. S., Santora, J. A., and Santos, M. M. (2020). Acute bottlenecks to the survival of juvenile Pygoscelis penguins occur immediately after fledging. Biol. Lett. 16:20200645. doi: 10.1098/rsbl.2020.0645
Hiruki, L., Schwartz, M., and Boveng, P. (1999). Hunting and social behaviour of leopard seals (Hydrurga leptonyx) at Seal Island, South Shetland Islands, Antarctica. J. Zool. 249, 97–109. doi: 10.1111/j.1469-7998.1999
Hiruki-Raring, L. M., Hoef, J. M. V., Boveng, P. L., and Bengtson, J. L. (2012). A Bayesian hierarchical model of Antarctic fur seal foraging and pup growth related to sea ice and prey abundance. Ecol. Appl. 22, 668–684. doi: 10.1890/11-0102.1
Hodgson, D. A., and Johnston, N. M. (1997). Inferring seal populations from lake sediments. Nature 387, 30–31. doi: 10.1038/387030a0
Hoffman, J. I., and Forcada, J. (2012). Extreme natal philopatry in female Antarctic fur seals (Arctocephalus gazella). Mamm. Biol. 77, 71–73. doi: 10.1016/j.mambio.2011.09.002
Hofmeyr, G. J. G., Bester, M. N., Makhado, A. B., and Pistorius, P. A. (2006). Population changes in Subantarctic and Antarctic fur seals at Marion Island : research article. S. Afr. J. Wildlife Res. 36, 55–68.
Hofmeyr, G. J. G., Krafft, B. A., Kirkman, S. P., Bester, M. N., Lydersen, C., and Kovacs, K. M. (2005). Population changes of Antarctic fur seals at Nyrøysa, Bouvetøya. Polar Biol. 28, 725–731. doi: 10.1007/s00300-005-0732-7
Hucke-Gaete, R., Osman, L. P., Moreno, C. A., and Torres, D. (2004). Examining natural population growth from near extinction: the case of the Antarctic fur seal at the South Shetlands, Antarctica. Polar Biol. 27, 304–311. doi: 10.1007/s00300-003-0587-8
Hückstädt, L. A., Piñones, A., Palacios, D. M., McDonald, B. I., Dinniman, M. S., Hofmann, E. E., et al. (2020). Projected shifts in the foraging habitat of crabeater seals along the Antarctic Peninsula. Nat. Clim. Change 10, 472–477. doi: 10.1038/s41558-020-0745-9
Humble, E., Dasmahapatra, K. K., Martinez-Barrio, A., Gregório, I., Forcada, J., Polikeit, A.-C., et al. (2018). RAD sequencing and a hybrid antarctic fur seal genome assembly reveal rapidly decaying linkage disequilibrium, global population structure and evidence for inbreeding. G3 Genes Genomes Genet. 8, 2709–2722. doi: 10.1534/g3.118.200171
Kinzey, D., Watters, G. M., and Reiss, C. S. (2015). Selectivity and two biomass measures in an age-based assessment of Antarctic krill (Euphausia superba). Fish. Res. 168, 72–84. doi: 10.1016/j.fishres.2015.03.023
Kinzey, D., Watters, G. M., and Reiss, C. S. (2019). Estimating recruitment variability and productivity in Antarctic krill. Fish. Res. 217, 98–107. doi: 10.1016/j.fishres.2018.09.027
Klein, E. S., Hill, S. L., Hinke, J. T., Phillips, T., and Watters, G. M. (2018). Impacts of rising sea temperature on krill increase risks for predators in the Scotia Sea. PLoS One 13:e0191011. doi: 10.1371/journal.pone.0191011
Klemmedson, A. D., Reiss, C. S., Goebel, M. E., Kaufmann, R. S., Dorval, E., Linkowski, T. B., et al. (2020). Variability in age of a Southern Ocean myctophid (Gymnoscopelus nicholsi) derived from scat-recovered otoliths. Mar. Ecol. Prog. Ser. 633, 55–69. doi: 10.3354/meps13176
Kovacs, K. M., Aguilar, A., Aurioles, D., Burkanov, V., Campagna, C., Gales, N., et al. (2012). Global threats to pinnipeds. Mar. Mamm. Sci. 28, 414–436. doi: 10.1111/j.1748-7692.2011.00479.x
Krause, D. J., Goebel, M. E., and Kurle, C. M. (2020). Leopard seal diets in a rapidly warming polar region vary by year, season, sex, and body size. BMC Ecol. 20:32. doi: 10.1186/s12898-020-00300-y
Krause, D. J., Goebel, M. E., Marshall, G. J., and Abernathy, K. (2015). Novel foraging strategies observed in a growing leopard seal (Hydrurga leptonyx) population at Livingston Island, Antarctic Peninsula. Anim. Biotelemetry 3:24. doi: 10.1186/s40317-015-0059-2
Krause, D. J., Goebel, M. E., Marshall, G. J., and Abernathy, K. (2016). Summer diving and haul-out behavior of leopard seals (Hydrurga leptonyx) near mesopredator breeding colonies at Livingston Island, Antarctic Peninsula. Mar. Mamm. Sci. 32, 839–867. doi: 10.1111/mms.12309
Krause, D. J., and Hinke, J. T. (2021). Finally within reach: a drone census of an important, but practically inaccessible, Antarctic fur seal colony. Aquat. Mamm. 47, 349–354. doi: 10.1578/AM.47.4.2021.349
Krause, D. J., and Rogers, T. L. (2019). Food caching by a marine apex predator, the leopard seal (Hydrurga leptonyx). Can. J. Zool. 97, 573–578. doi: 10.1139/cjz-2018-0203
Krüger, L., Huerta, M. F., Santa Cruz, F., and Cárdenas, C. A. (2021). Antarctic krill fishery effects over penguin populations under adverse climate conditions: implications for the management of fishing practices. Ambio 50, 560–571. doi: 10.1007/s13280-020-01386-w
Lang, A. R., Boveng, P., Quakenbush, L., Robertson, K., Lauf, M., Rode, K. D., et al. (2021). Re-examination of population structure in Arctic ringed seals using DArTseq genotyping. Endang. Spec. Res. 44, 11–31. doi: 10.3354/esr01087
Laws, R. M. (1952). A new method of age determination for mammals. Nature 169, 972–973. doi: 10.1038/169972b0
Laws, R. M. (1973). Population increase of Fur Seals at South Georgia. Polar Rec. 16, 856–858. doi: 10.1017/S003224740006397X
Laws, R. M. (1977). Seals and whales of the Southern Ocean. Philos. Trans. R. Soc. Lond. B Biol. Sci. 279, 81–96. doi: 10.1098/rstb.1977.0073
Lowe, W., and Allendorf, F. (2010). What can genetics tell us about population connectivity? Mol. Ecol. 19, 3038–3051. doi: 10.1111/j.1365-294X.2010.04688.x
Lowry, M. S., Condit, R., Hatfield, B., Allen, S. G., Berger, R., Morris, P. A., et al. (2014). Abundance, distribution, and population growth of the northern elephant seal (Mirounga angustirostris) in the United States from 1991 to 2010. Aquat. Mamm. 40, 20–31.
Lunn, N. J., and Boyd, I. L. (1993). Effects of maternal age and condition on parturition and the perinatal period of Antarctic fur seals. J. Zool. 229, 55–67. doi: 10.1111/j.1469-7998.1993.tb02620.x
Lynch, H. J., Naveen, R., Trathan, P. N., and Fagan, W. F. (2012). Spatially integrated assessment reveals widespread changes in penguin populations on the Antarctic Peninsula. Ecology 93, 1367–1377. doi: 10.1890/11-1588.1
Majluf, P., and Goebel, M. E. (1992). The capture and handling of female South American fur seals and their pups. Mar. Mamm. Sci. 8, 187–190. doi: 10.1111/j.1748-7692.1992.tb00382.x
Massom, R. A., and Stammerjohn, S. E. (2010). Antarctic sea ice change and variability – Physical and ecological implications. Polar Sci. 4, 149–186. doi: 10.1016/j.polar.2010.05.001
McCafferty, D. J., Boyd, I. L., Walker, T., and Taylor, R. I. (1998). Foraging responses of Antarctic fur seals to changes in the marine environment. Mar. Ecol. Prog. Ser. 166, 285–299. doi: 10.3354/meps166285
McCann, T. S., and Doidge, D. W. (1987). “Antarctic fur seal (Arctocephalus gazella),” in Status, Biology and ecology of Fur Seals, eds J. P. Croxall and R. L. Gentry (Cambridge, MA: National Marine Fisheries Service).
Melin, S. R., DeLong, R. L., and Siniff, D. B. (2008). The effects of El Niño on the foraging behavior of lactating California sea lions (Zalophus californianus) during the nonbreeding season. Can. J. Zool. 86, 192–206. doi: 10.1139/z07-132
Melin, S. R., Laake, J. L., DeLong, R. L., and Siniff, D. B. (2012). Age-specific recruitment and natality of California sea lions at San Miguel Island, California. Mar. Mamm. Sci. 28, 751–776. doi: 10.1111/j.1748-7692.2011.00538.x
Melin, S. R., Orr, A. J., Harris, J. D., Laake, J., DeLong, R. L., Gulland, F. M. D., et al. (2010). Unprecedented mortality of California sea lion pups associated with anomalous oceanographic conditions along the central California coast in 2009. CalCOFI Rep. 51, 182–194.
Meredith, M. P., and King, J. C. (2005). Rapid climate change in the ocean west of the Antarctic Peninsula during the second half of the 20th century. Geophys. Res. Lett. 32:L19604. doi: 10.1029/2005GL024042
Meyer, W., Walker, B., and Holt, R. (1996). Antarctic Fur Seal Abundance and Distribution in the South Shetland Islands, 1996. AMLR 1995/96 Field Season Report.NOAA-AR-LJ 96-15. Antarctic Ecosystem Research Group, SWFSC/NMFS/NOAA. Washington, DC: NOAA.
Moore, S. E. (2008). Marine mammals as ecosystem sentinels. J. Mammal. 89, 534–540. doi: 10.1644/07-mamm-s-312r1.1
Munch, S. B., Snover, M. L., Watters, G. M., and Mangel, M. (2005). A unified treatment of top-down and bottom-up control of reproduction in populations. Ecol. Lett. 8, 691–695. doi: 10.1111/j.1461-0248.2005.00766.x
Nicol, S., Foster, J., and Kawaguchi, S. (2012). The fishery for Antarctic krill – recent developments. Fish Fish. 13, 30–40. doi: 10.1111/j.1467-2979.2011.00406.x
O’Corry-Crowe, G., Gelatt, T., Rea, L., Bonin, C., and Rehberg, M. (2014). Crossing to safety: dispersal, colonization and mate choice in evolutionarily distinct populations of Steller sea lions, Eumetopias jubatus. Mol. Ecol. 23, 5415–5434. doi: 10.1111/mec.12944
O’Gorman, F. (1961). Fur seals breeding in the Falkland Islands Dependencies. Nature 192, 914–916. doi: 10.1038/192914a0
Orsi, A. H., Whitworth, T. III, and Nowlin, W. D. Jr. (1995). On the meridional extent and fronts of the antarctic circumpolar current. Deep Sea Res. I Oceanogr. Res. Pap. 42, 641–673. doi: 10.1016/0967-0637(95)00021-W
Pagano, A. M., Durner, G. M., Rode, K. D., Atwood, T. C., Atkinson, S. N., Peacock, E., et al. (2018). High-energy, high-fat lifestyle challenges an Arctic apex predator, the polar bear. Science 359, 568–572. doi: 10.1126/science.aan8677
Paijmans, A. J., Stoffel, M. A., Bester, M. N., Cleary, A. C., De Bruyn, P. J. N., Forcada, J., et al. (2020). The genetic legacy of extreme exploitation in a polar vertebrate. Sci. Rep. 10:5089. doi: 10.1038/s41598-020-61560-8
Paine, R. T. (1966). Food web complexity and species diversity. Am. Nat. 100, 65–75. doi: 10.1086/282400
Pallin, L. J., Baker, C. S., Steel, D., Kellar, N. M., Robbins, J., Johnston, D. W., et al. (2018). High pregnancy rates in humpback whales (Megaptera novaeangliae) around the Western Antarctic Peninsula, evidence of a rapidly growing population. R. Soc. Open Sci. 5:180017. doi: 10.1098/rsos.180017
Payne, M. (1977). Growth of a fur seal population. Philos. Trans. R. Soc. Lond. B Biol. Sci. 279, 67–79. doi: 10.1098/rstb.1977.0072
Payne, M. (1979). Growth in the Antarctic fur seal Arctocephalus gazella. J. Zool. 187, 1–20. doi: 10.1111/j.1469-7998.1979.tb07709.x
Phillips, C., Bickham, J., Patton, J., and Gelatt, T. (2009). Systematics of Steller sea lions (Eumetopias jubatus): subspecies recognition based on concordance of genetics and morphometrics. Occasion. Pap. Museum Texas Tech. Univ. 283, 1–15.
Polito, M. J., and Goebel, M. E. (2010). Investigating the use of stable isotope analysis of milk to infer seasonal trends in the diets and foraging habitats of female Antarctic fur seals. J. Exp. Mar. Biol. Ecol. 395, 1–9. doi: 10.1016/j.jembe.2010.08.015
R-Core-Team (2021). R: A Language and Environment for Statistical Computing. R Foundation for Statistical Computing, 4.0.2. Vienna: R Foundation for Statistical Computing.
Rehm, E. M., Olivas, P., Stroud, J., and Feeley, K. J. (2015). Losing your edge: climate change and the conservation value of range-edge populations. Ecol. Evol. 5, 4315–4326. doi: 10.1002/ece3.1645
Reid, K., and Croxall, J. P. (2001). Environmental response of upper trophic-level predators reveals a system change in an Antarctic marine ecosystem. Proc. R. Soc. Lond. B Biol. Sci. 268, 377–384. doi: 10.1098/rspb.2000.1371
Reisinger, R. R., de Bruyn, P. J. N., and Bester, M. N. (2011). Predatory impact of killer whales on pinniped and penguin populations at the Subantarctic Prince Edward Islands: fact and fiction. J. Zool. 285, 1–10. doi: 10.1111/j.1469-7998.2011.00815.x
Roux, J. P. (1987). “Recolonization process in the sub-Antarctic fur seal, Arctocephalus tropicalis, on Amsterdam Island,” in Status, Biology and Ecology of Fur Seals, NOAA Technical Report, Vol. 51, eds J. P. Croxall and R. L. Gentry (Cambridge, MA: National Marine Fisheries Service).
Santora, J., and Veit, R. (2013). Spatio-temporal persistence of top predator hotspots near the Antarctic Peninsula. Mar. Ecol. Prog. Ser. 487, 287–304. doi: 10.3354/meps10350
Scheffer, V. B. (1967). Standard measurements of seals. J. Mammal. 48, 459–462. doi: 10.2307/1377778
Schwarz, L. K., Goebel, M. E., Costa, D. P., and Kilpatrick, A. M. (2013). Top-down and bottom-up influences on demographic rates of Antarctic fur seals Arctocephalus gazella. J. Anim. Ecol. 82, 903–911. doi: 10.1111/1365-2656.12059
Shaughnessy, P. D., and Goldsworthy, S. D. (1990). Population size and breeding season of the Antarctic fur seal Arctocephalus gazella at Heard Island—1987/88. Mar. Mamm. Sci. 6, 292–304. doi: 10.1111/j.1748-7692.1990.tb00359.x
Siniff, D. B., Garrott, R. A., Rotella, J. J., Fraser, W. R., and Ainley, D. G. (2008). Opinion: projecting the effects of environmental change on Antarctic seals. Antarct. Sci. 20, 425–435. doi: 10.1017/S0954102008001351
Southwell, C., Bengtson, J., Bester, M., Blix, A. S., Bornemann, H., Boveng, P., et al. (2012). A review of data on abundance, trends in abundance, habitat use and diet of ice-breeding seals in the Southern Ocean. CCAMLR Sci. 19, 49–74.
Springer, A., Estes, J., Van Vliet, G., Williams, T., Doak, D., Danner, E., et al. (2003). Sequential megafaunal collapse in the North Pacific Ocean: an ongoing legacy of industrial whaling? Proc. Natl. Acad. Sci. U.S.A. 100:12223. doi: 10.1073/pnas.1635156100
Stoffel, M. A., Humble, E., Paijmans, A. J., Acevedo-Whitehouse, K., Chilvers, B. L., Dickerson, B., et al. (2018). Demographic histories and genetic diversity across pinnipeds are shaped by human exploitation, ecology and life-history. Nat. Commun. 9:4836. doi: 10.1038/s41467-018-06695-z
Surma, S., Pakhomov, E. A., and Pitcher, T. J. (2014). Effects of whaling on the structure of the southern ocean food web: insights on the “krill surplus” from ecosystem modelling. PLoS One 9:e114978. doi: 10.1371/journal.pone.0114978
Sweeney, K. L., Shertzer, K. W., Fritz, L. W., and Read, A. J. (2015). A novel approach to compare pinniped populations across a broad geographic range. Can. J. Fish. Aquat. Sci. 72, 175–185. doi: 10.1139/cjfas-2014-0070
Torres, D., Vallejos, V., Acevedo, J., Hucke-Gaete, R., and Zarate, S. (1998). Registros biológicos atípicos en cabo Shirreff, isla Livingston, Antártica. Bol. Antarct. Chileno 17, 17–19.
Trillmich, F., and Dellinger, T. (1991). “The effects of El Niño on Galapagos pinnipeds,” in Pinnipeds and El Niño: Responses to Environmental Stress, Vol. 88, eds F. Trillmich and K. Ono (Berlin: Springer), 66–74.
Trivelpiece, W. Z., Hinke, J. T., Miller, A. K., Reiss, C. S., Trivelpiece, S. G., and Watters, G. M. (2011). Variability in krill biomass links harvesting and climate warming to penguin population changes in Antarctica. Proc. Natl. Acad. Sci. U.S.A. 108, 7625–7628. doi: 10.1073/pnas.1016560108
Trukhanova, I. S., Gurarie, E., and Sagitov, R. A. (2013). Distribution of hauled-out ladoga ringed seals (Pusa hispida ladogensis) in Spring 2012. Arctic 66, 417–428.
Turner, J., Barrand, N. E., Bracegirdle, T. J., Convey, P., Hodgson, D. A., Jarvis, M., et al. (2014). Antarctic climate change and the environment: an update. Polar Rec. 50, 237–259. doi: 10.1017/S0032247413000296
Vargas, R., Osman, L. P., and Torres, D. (2009). Inter-sexual differences in Antarctic fur seal pup growth rates: evidence of environmental regulation? Polar Biol. 32, 1177–1186. doi: 10.1007/s00300-009-0615-4
Vaughan, D. (2006). Recent trends in melting conditions on the Antarctic Peninsula and their implications for ice-sheet mass balance and sea level. Arctic Antarctic Alpine Res. 38, 147–152. doi: 10.1657/1523-0430(2006)038[0147:rtimco]2.0.co;2
Vera, C., Vargas, R., and Torres, D. N. (2005). Estrategias depredatorias del la foca leopardo sobre cachorros de lobo fino Antarctico. Bol. Antarct. Chileno 24, 12–17.
Wade, P., Ver Hoef, J., and DeMaster, D. (2009). Mammal eating killer whales and their prey—trend data for pinnipeds and sea otters in the North Pacific Ocean do not support the sequential megafaunal collapse hypothesis. Mar. Mamm. Sci. 25, 737–747. doi: 10.1111/j.1748-7692.2009.00282.x
Waples, R., and Gaggiotti, O. (2006). Invited review: what is a population? An empirical evaluation of some genetic methods for identifying the number of gene pools and their degree of connectivity. Mol. Ecol. 15, 1419–1439. doi: 10.1111/j.1365-294X.2006.02890.x
Watters, G. M., Hinke, J. T., and Reiss, C. S. (2020). Long-term observations from Antarctica demonstrate that mismatched scales of fisheries management and predator-prey interaction lead to erroneous conclusions about precaution. Sci. Rep. 10:2314. doi: 10.1038/s41598-020-59223-9
Webster, W. H. B. (1834). “Narrative of a voyage to the southern Atlantic Ocean in the years 1828,29,30 performed,” in Chanticleer, Under the Command of the late Captain Henry Foster, ed. H. M. Sloop (Cambridge, MA: Cambridge University Press).
Weddell, J. (1970). A Voyage Towards the South Pole, Performed in the Years 1822-24 : Containing an Examination of the Antarctic Sea (1827), 2nd Edn. Maryland: United States Naval Institue.
Wege, M., Etienne, M.-P., Chris Oosthuizen, W., Reisinger, R. R., Bester, M. N., and de Bruyn, P. J. N. (2016). Trend changes in sympatric Subantarctic and Antarctic fur seal pup populations at Marion Island, Southern Ocean. Mar. Mamm. Sci. 32, 960–982. doi: 10.1111/mms.12306
Weise, M. J., and Harvey, J. T. (2008). Temporal variability in ocean climate and California sea lion diet and biomass consumption: implications for fisheries management. Mar. Ecol. Prog. Ser. 373, 157–172. doi: 10.3354/meps07737
Wickens, P., and York, A. E. (1997). Comparative population dynamics of fur seals. Mar. Mamm. Sci. 13, 241–292. doi: 10.1111/j.1748-7692.1997.tb00631.x
Williams, C. A. (1888). Report to the Committee of Congress on Merchant and Marine Fisheries. Washington, DC: Government Printing Office.
Williams, T., Estes, J., Doak, D., and Springer, A. (2004). Killer appetites: assessing the role of predators in ecological communities. Ecology 85, 3373–3384. doi: 10.1890/03-0696
Wynen, L. P., Goldsworthy, S. D., Guinet, C., Bester, M. N., Boyd, I. L., Gjertz, I., et al. (2000). Postsealing genetic variation and population structure of two species of fur seal (Arctocephalus gazella and A. tropicalis). Mol. Ecol. 9, 299–314. doi: 10.1046/j.1365-294x.2000.00856.x
York, A. E. (1987). “On comparing the population dynamics of fur seals,” in Status, Biology and Ecology of Fur Seals, NOAA Technical Report, Vol. 51, eds J. P. Croxall and R. L. Gentry (Cambridge, MA: National Marine Fisheries Service).
Keywords: Antarctic fur seal, conservation status, South Shetland Islands, top-down and bottom-up control, edge population, Arctocephalus gazella, population dynamics (ecology)
Citation: Krause DJ, Bonin CA, Goebel ME, Reiss CS and Watters GM (2022) The Rapid Population Collapse of a Key Marine Predator in the Northern Antarctic Peninsula Endangers Genetic Diversity and Resilience to Climate Change. Front. Mar. Sci. 8:796488. doi: 10.3389/fmars.2021.796488
Received: 16 October 2021; Accepted: 22 November 2021;
Published: 03 January 2022.
Edited by:
Romuald Lipcius, William and Mary’s Virginia Institute of Marine Science, United StatesReviewed by:
Birgitte I. McDonald, Moss Landing Marine Laboratories, United StatesRebecca Ruth McIntosh, Phillip Island Nature Parks, Australia
Copyright © 2022 Krause, Bonin, Goebel, Reiss and Watters. This is an open-access article distributed under the terms of the Creative Commons Attribution License (CC BY). The use, distribution or reproduction in other forums is permitted, provided the original author(s) and the copyright owner(s) are credited and that the original publication in this journal is cited, in accordance with accepted academic practice. No use, distribution or reproduction is permitted which does not comply with these terms.
*Correspondence: Douglas J. Krause, ZG91Z2xhcy5rcmF1c2VAbm9hYS5nb3Y=; orcid.org/0000-0002-2517-3106