- 1Cedar Coast Field Station, Vargas Island, BC, Canada
- 2Institute of Ocean Sciences, Fisheries and Oceans Canada, Sidney, BC, Canada
- 3Hakai Institute, Victoria, BC, Canada
- 4Department of Earth, Ocean and Atmospheric Sciences, University of British Columbia, Vancouver, BC, Canada
- 5Sciences and Mathematics, Division of School of Interdisciplinary Arts and Sciences, University of Washington Tacoma, Tacoma, WA, United States
- 6Uu-a-thluk Fisheries, Nuu-chah-nulth Tribal Council, Ahousaht, BC, Canada
While fjords often have low oxygen concentrations in their deep waters, this research identified seasonal, near-surface hypoxia (≤ 2 mL L-1 or 2.9 mg L-1) through a year-long monthly time series in Clayoquot Sound, British Columbia. Temperature, salinity, and oxygen data were collected monthly in the upper 50 m at three stations in Herbert Inlet from June 2020 to July 2021, marking the first time series of its kind in a Clayoquot Sound fjord. Hypoxic conditions were shallowest (minimum depth of 12 m) and most intensified in summer; near-surface hypoxia was recorded at one or more stations in all months except in winter. Considering that many local marine species, including wild Pacific salmon, experience adverse effects at oxygen concentrations much higher than the hypoxic threshold, we note that 50 to 100% of the upper 50 m of Herbert Inlet consistently presented low oxygen concentrations (defined here as a guideline as ≤ 4.9 mL L-1 or 6.9 mg L-1) during the 14-month study period. Previous observations collected sporadically since May 1959 confirmed the presence of hypoxic conditions in the past. These findings suggest that long-term, multidisciplinary studies are needed to understand and predict the impact of hypoxia and deoxygenation on wild salmon stocks as climate changes.
1 Introduction
The global inventory of oceanic oxygen is decreasing (Schmidtko et al., 2017) and subsurface dissolved oxygen (DO) concentrations are declining faster in coastal waters than in the open ocean (Gilbert et al., 2010). Coastal systems experience the combined effects of global temperature-driven deoxygenation, anthropogenic nutrient loading, and eutrophication (Diaz and Rosenberg, 2008; Genovesi et al., 2011; Breitburg et al., 2018), as well as enhanced remineralization (Johannessen et al., 2014). The strength and timing of wind-driven upwelling is also changing (Bakun, 1990; Foreman et al., 2011), which affects the delivery of high-density, low-oxygen water onto continental shelves in eastern boundary upwelling ecosystems (Sydeman et al., 2014; García-Reyes et al., 2015). Exposure to hypoxic upwelled waters in such coastal ecosystems can have deleterious effects and can even lead to massive fish kills, as on the coast of Oregon (Grantham et al., 2004; Bakun et al., 2015).
Fjords are coastal geographic features carved out by glaciers and are often oxygen-depleted at depth (Pickard, 1961; Silva and Vargas, 2014; Björk et al., 2017). A typical along-fjord DO distribution may show higher oxygen at the mouth and lower oxygen near the head (e.g., Pickard, 1961; Pickard, 1963; Silva and Vargas, 2014). Several coastal fjord systems on the British Columbia (BC) mainland coast and the west coast of Vancouver Island (WCVI) have already been identified as hypoxic/anoxic at depth, often due to limited horizontal exchange over shallow sills and river outflow-driven stratification and inhibition of vertical mixing (e.g., Anderson and Devol, 1973; Pawlowicz, 2017; Thomson et al., 2017). Near surface hypoxia has been observed in Clayoquot Sound (CS) in previous microbial and redox chemistry studies (e.g. Diaz et al., 2012; Kusch et al., 2021), but without further oceanographic analysis.
Our research identifies seasonally hypoxic waters near the surface (10 – 50 m deep) in Herbert Inlet. The latter is a coastal fjord within the traditional territory of the Ahousaht First Nation and the CS UNESCO Biosphere Reserve, on the WCVI, BC, Canada (Figure 1). The region hosts many finfish aquaculture farms, including two in Herbert Inlet. Despite CS’s economic, cultural and ecological importance, oceanographic dynamics in Herbert Inlet and in other proximal fjord systems (see Pickard, 1963) are poorly understood. Running ~ 23 km sill to head and ~ 2 km across, Herbert Inlet contains a single deep (140 – 160 m) basin bounded at the mouth by a shallow (~ 5 m) entrance sill and by the Moyeha River (Ahousaht name Muuyaḥi) at the head. Herbert Inlet has two connections to the outer shelf: the entrance sill in Russel Channel to the south and Millar Channel (via Sydney Inlet; sill depth ~ 25 m) to the north. The Moyeha River supports four main wild Pacific salmon runs: Chum (Oncorhynchus keta/hink̓uuʔas), Coho (O. kisutch/cuw̓it), Chinook (O. tshawytscha/sac̓up), and Sockeye (O. nerka/miʕaat hissit - muwač’atḥ). Despite being one of the only CS watersheds unaffected by the commercial logging industry, Moyeha’s salmon populations have dwindled. While many factors could be responsible for the salmon decline, shoaling or expanding hypoxia could contribute to a growing list of stressors faced by wild salmon populations.
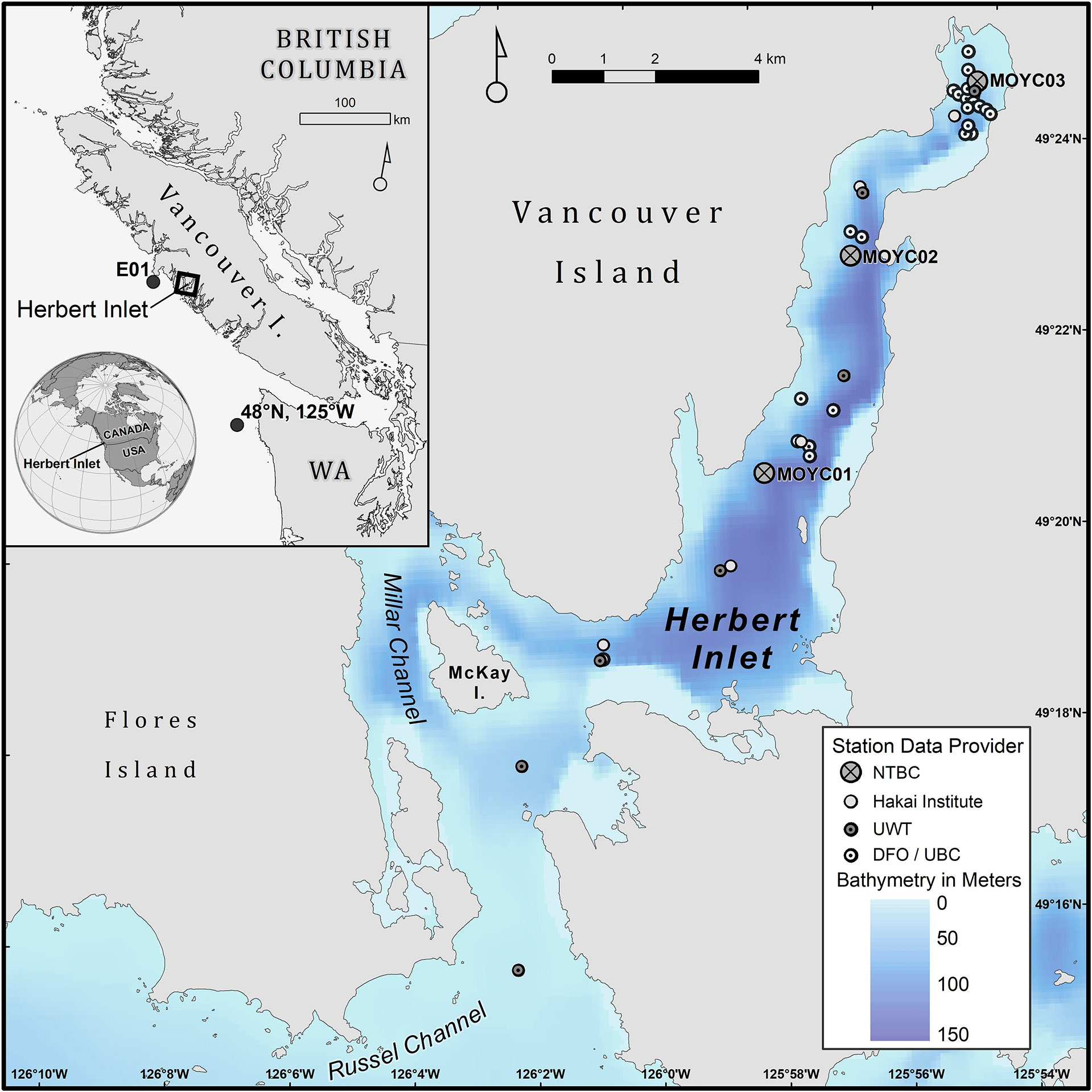
Figure 1 Bathymetric map of Herbert Inlet with all sampling stations shown. Inset shows location of Herbert Inlet relative to the rest of Vancouver Island and North America, DFO’s E01 mooring, and the location at which Bakun daily Cumulative Upwelling Index (CUI) values were recorded (black circles).
Here, we examine oxygen and other water properties in the upper 50 m of Herbert Inlet from June 2020 to July 2021 and report hypoxia at one or more stations in all months except in winter 2021. Additionally, analysis of sporadic observations available since 1959 shows that hypoxic conditions occurred, both near-surface and deeper, in the historic record. In the discussion section, we identify possible mechanisms of near-surface oxygen depletion and reflect on the efforts and research needed to address the effect of deoxygenation on declining wild salmon populations.
2 Methods
2.1 Oceanographic and atmospheric data
Several different oceanographic datasets were used in this research. The first was monthly conductivity, temperature, depth (CTD), and oxygen data that were collected from June 2020 to July 2021 by the Nature Trust of British Columbia (NTBC; https://www.naturetrust.bc.ca) in collaboration with the Hakai Institute, Ahousaht Fisheries, and Uu-a-thluk - Nuu-Chah-Nulth Tribal Council Fisheries. Data were collected in the upper 50 m at three equally spaced stations in Herbert Inlet (MOYC01, MOYC02, and MOYC03; see Figure 1). The NTBC CTD instrument was originally intended to undertake research on estuary resilience to sea-level rise and was consequently calibrated to sample only the upper 50 m. While this depth constraint limits the extent of possible oceanographic analysis (i.e., no full water column information), a monthly timeseries of water property profiles over the course of a year in these fjords is rare, such that exploring the available dataset is still a valuable exercise.
Further available datasets were from occasional sampling of Herbert Inlet conducted since 1959 by the University of Washington at Tacoma (UWT), the Hakai Institute, Cedar Coast Field Station, the University of British Columbia (UBC), and Fisheries and Oceans Canada (DFO; see Tables S1 – S3). We refer to these datasets as ‘historical data’ to distinguish them from the 14-month NTBC dataset. Mid-channel stations deeper than 50 m were selected from historical datasets for comparison to NTBC data (see Figure 1). All CTD and oxygen sensors were calibrated yearly by the manufacturers, with nominal accuracy within 2% of saturation for oxygen. Historical oxygen data analyzed by the Winkler titration method are considered accurate to within ±3 to 5% (Carpenter, 1965). Further information regarding CTD models, firm/software, and sensors is provided in Table S3.
Data from DFO mooring E01 (49° 17.4’ N, 126° 36.31’ W; see Figure 1), located ~ 10 km southwest of Estevan Point (described in more detail by Thomson et al., 2017), were used to examine the connectivity between Herbert Inlet and the shelf. Mooring bottom depth was 101 m, and temperature and salinity sensors were positioned at depths of 35, 75, and 95 m. Oxygen sensors were present at 95 m depth. The 95 m and 35 m sensors recorded from July 2020 – June 2021, while the 75 m sensor stopped recording in February 2021.
The Bakun daily index from 48° N, 125° W (see Figure 1) was retrieved from NOAA’s Environmental Research Division to calculate the Cumulative Upwelling Index (CUI) from June 29, 2020 to July 16, 2021.
2.2 Defining the oxygen minimum
We focus on single-point oxygen minima to track the seasonal variation of depth and properties of low-oxygen water. Hence, we define the minimum oxygen concentration as the lowest DO concentration in the sampled water column, such that Omin50 is the minimum in the top ~ 50 m sampled by the NTBC dataset and Omin is the minimum in the entire water column in historical observations. In two historical datasets, where equally low DO concentrations occurred at multiple depths, the shallowest one was selected. The depth of the Omin50 and its corresponding water properties over the 14-month study period are tracked seasonally and compared to historical observations.
2.3 Calculated oxygen variables and oxygen thresholds
2.3.1 Oxygen saturation and apparent oxygen utilization
Osat is the ratio of measured to saturated oxygen content (SOC; as in Boyer et al., 1999). The SOC is the amount of DO that would be dissolved in a 1 L water parcel if it were in equilibrium with the atmosphere (Boyer et al., 1999). Osat accounts for the effects of temperature and salinity; hence, a horizontal Osat gradient indicates biological consumption or production of oxygen (Jackson et al., 2021b). We calculate Osat from measured salinity, temperature, and DO using the equations recommended by Garcia and Gordon (1992).
AOU is the difference between the SOC and measured oxygen content in a parcel of seawater (Boyer et al., 1999). Practically, AOU is a measure of the oxygen consumption that remains unexplained by changes in SOC; negative AOU values indicate net primary production of oxygen and positive values denote net consumption.
2.3.2 Hypoxic and low-oxygen thresholds
We adopt a hypoxic threshold of DO ≤ 2 mL L-1 (~ 2.9 mg L-1). Conventional hypoxic thresholds usually denote DO levels that will result in fisheries collapse and/or the emergence of “dead zones” (Vaquer-Sunyer and Duarte, 2008) and are hence conservative estimates of lethal oxygen concentration. Negative responses have been observed at much higher concentrations for many species. For instance, Davis (1975) reported 6.9 mg L-1 (~4.9 mL L-1) as a mean incipient oxygen response threshold for marine anadromous salmonids. Other studies report reduced hatching success for Chinook salmon at 5.5 mg L-1 (~3.9 mL L-1; Del Rio et al., 2019), increased respiration for adult Sockeye salmon at 5.0 to 6.0 mg L-1 (~3.5 to 4.2 mL L-1; Birtwell et al., 1994), reduced swim speed of juvenile Coho salmon at oxygen less than 6.0 mg L-1 (~4.2 mL L-1; Davis et al., 1963), avoidance reactions for juvenile Chinook salmon to concentrations under 7.0 – 8.0 mg L-1 (~4.9 – 5.6 mL L-1, Birtwell and Kruzynski, 1989), etc. Here, we use the Davis (1975) as a guideline, since it encompasses several salmonid species, but note that its selection is somewhat arbitrary and harmful or deleterious conditions can be found at higher (or lower) concentrations for different species. Lastly, recognizing the many oxygen units available and the fact that different units are preferred by different disciplines, we report DO values in both mL L-1 and mg L-1 (where 1 mg L-1 ≈ 0.7 mL L-1).
3 Results
3.1 Spatial and seasonal variability of oxygen, temperature, salinity, and density in the upper 50 m
Here, we present monthly DO section plots (Figures 2A–N) and 14-month time series of Bakun daily CUI, salinity, temperature, DO, AOU, and percent hypoxia (Figures 3A–F) in the upper 50 m at the head (MOYC03) of Herbert Inlet. Low-oxygen conditions above 50 m generally intensified towards the head of the inlet and weakened towards the mouth, particularly in spring and summer (Figure 2). Hypoxia in the upper 50 m was present at one or more stations in all months except between January 22nd and March 1st and persisted longest at the head. The lowest oxygen concentrations in the upper 50 m recorded in NTBC data occurred at the head in August 2020 (0.6 mL L-1 or ~ 0.8 mg L-1 at 48.7 m) and in April 2021 (0.3 mL L-1 or ~ 0.4 mg L-1 at 49.7 m; Figures 2C, K; Table S4).
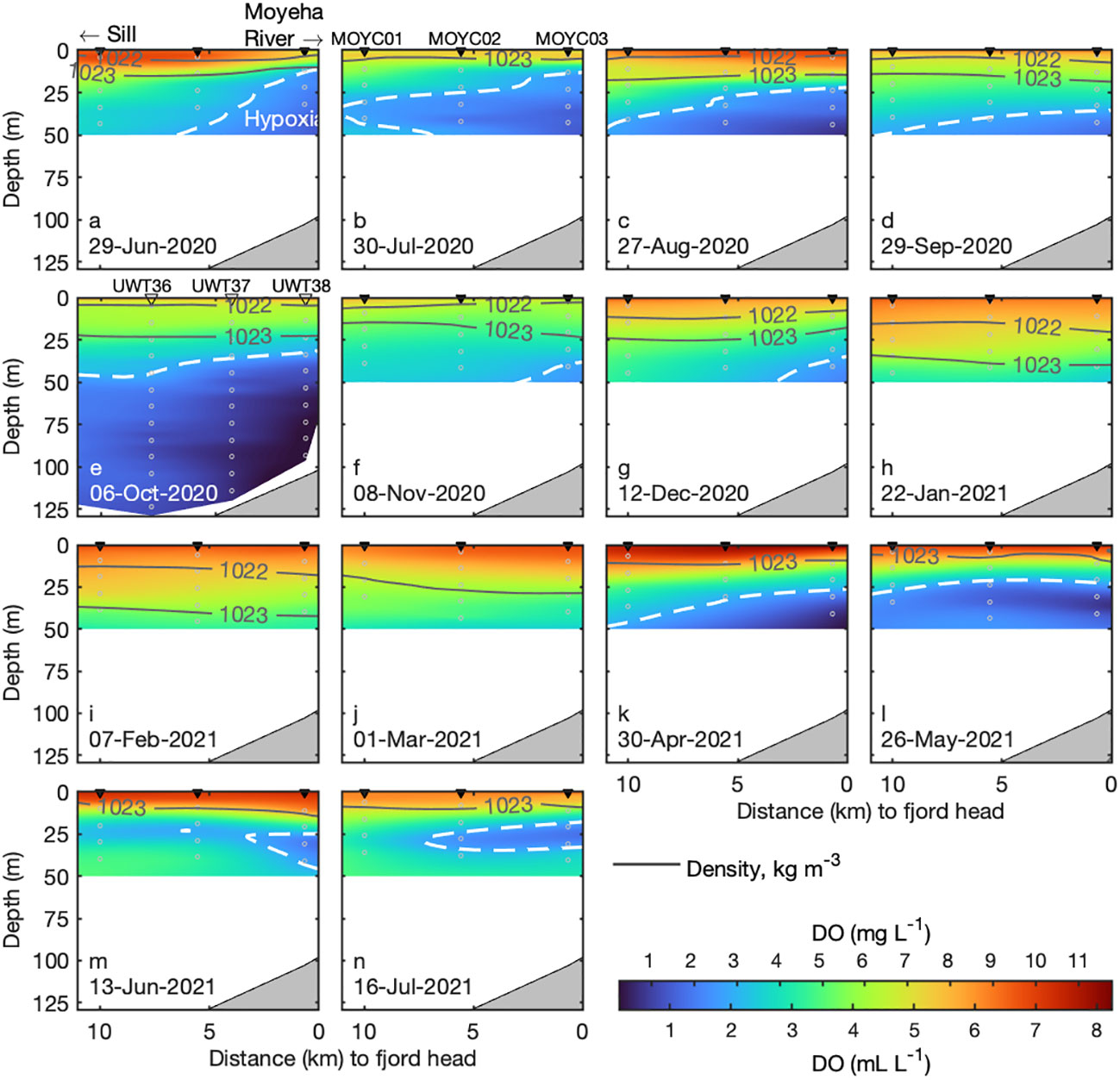
Figure 2 Monthly section plots (June 2020 – July 2021) of dissolved oxygen (DO) in Herbert Inlet. Hypoxic threshold (2 mL L-1 or 2.9 gm L-1) is outlined with white dashes; potential density contours (1022 and 1023 kg m-3) are shown in grey lines. Stations are arranged MOYC01 (mouth) to MOYC03 (head). The October 2020 panel (E) shows UWT station data.
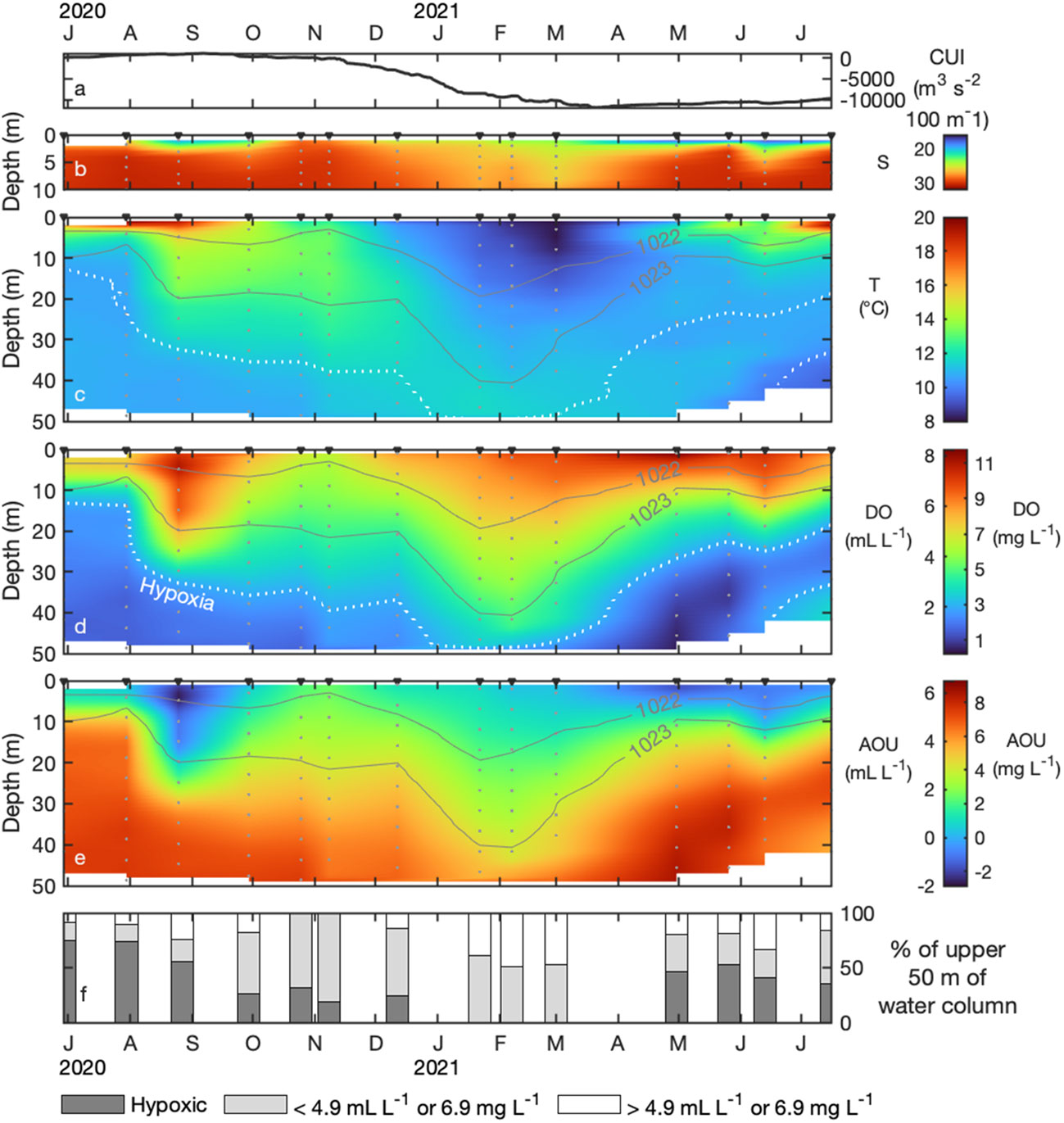
Figure 3 Time series (June 2020 – July 2021) of the Bakun daily CUI at 48° N, 125° W (A) and salinity (≤10 m depth; B), temperature (C), oxygen (D), apparent oxygen utilization (AOU; E), and percent hypoxia (F) in the upper 50 m at the head of Herbert Inlet (MOYC03). White dotted lines (panels C, D) indicate a hypoxic threshold of 2 mL L-1 (~ 2.9 mg L-1). Potential density isopycnals are shown as solid grey lines.
From June to September 2020, the water column was hypoxic at or above 50 m; from June to August, hypoxic waters occupied approximately half of the sampled column at all stations (Figures 2A–C; Figures 3D, F). In June and July 2020, hypoxic waters comprised nearly 75% of the upper 50 m at MOYC03 (Figure 3F); oxygen concentrations exceeded 2 mL L-1 (~ 2.9 mg L-1) only in the upper ~ 10 m of the sampled water column (Figure 3F). From November to March (Figures 2F–J), oxygen increased and the isopycnals deepened (by as much as ~ 30 m from July 2020 values), indicating weaker stratification as well as physical mixing and consequent reoxygenation. Nevertheless, oxygen concentrations under 4.9 mL L-1 (~ 6.9 mg L-1) take up > 50% of the top 50 m during these months (Figure 3F). Hypoxia returned in April 2021 (Figure 2K) and occupied ~ 50% of the upper 50 m through to July (Figure 3F), coinciding with shoaling of the 1022 and 1023 kg m-3 isopycnals to < 20 m depth (Figure 2). From May to July 2021 (Figures 2L–N), the Omin50 was shallower (~ 25 m) than previously observed (Figures 2A–K).
At the head of the inlet (MOYC03), surface salinities were highest and the water column least stratified during the winter and no hypoxia was observed above 50 m (Figure 3B, D). The lowest temperatures at MOYC03 were recorded at the surface in March (Figure 3C). Salinities were low and temperatures elevated at the surface during spring and summer months (Figures 3B, C). Surface AOU was negative (indicating net DO production) at the head in August 2020 and from February to July 2021 (Figure 3E). Downwelling winds at 48° N, 125° W ceased in the 3rd week of March 2021 (Figure 3A); the onset of near-surface hypoxia in Herbert Inlet was observed in the 4th week of April 2021 (Figure 2).
3.2 Comparison of Omin50 and historical Omin properties
Hypoxic conditions were present in all historical datasets (Figure 4A), with observations reaching nearly anoxic levels in the lower water column in some years (May 1959, June 1963, and May 2000). In the upper 50 m of the water column, hypoxia was observed at one or more stations in September 1988, October 2015, July 2019, and August 2019 (Figure 4B), indicating that near-surface hypoxia has been, at very least, a sporadic occurrence in Herbert Inlet in the last few decades.
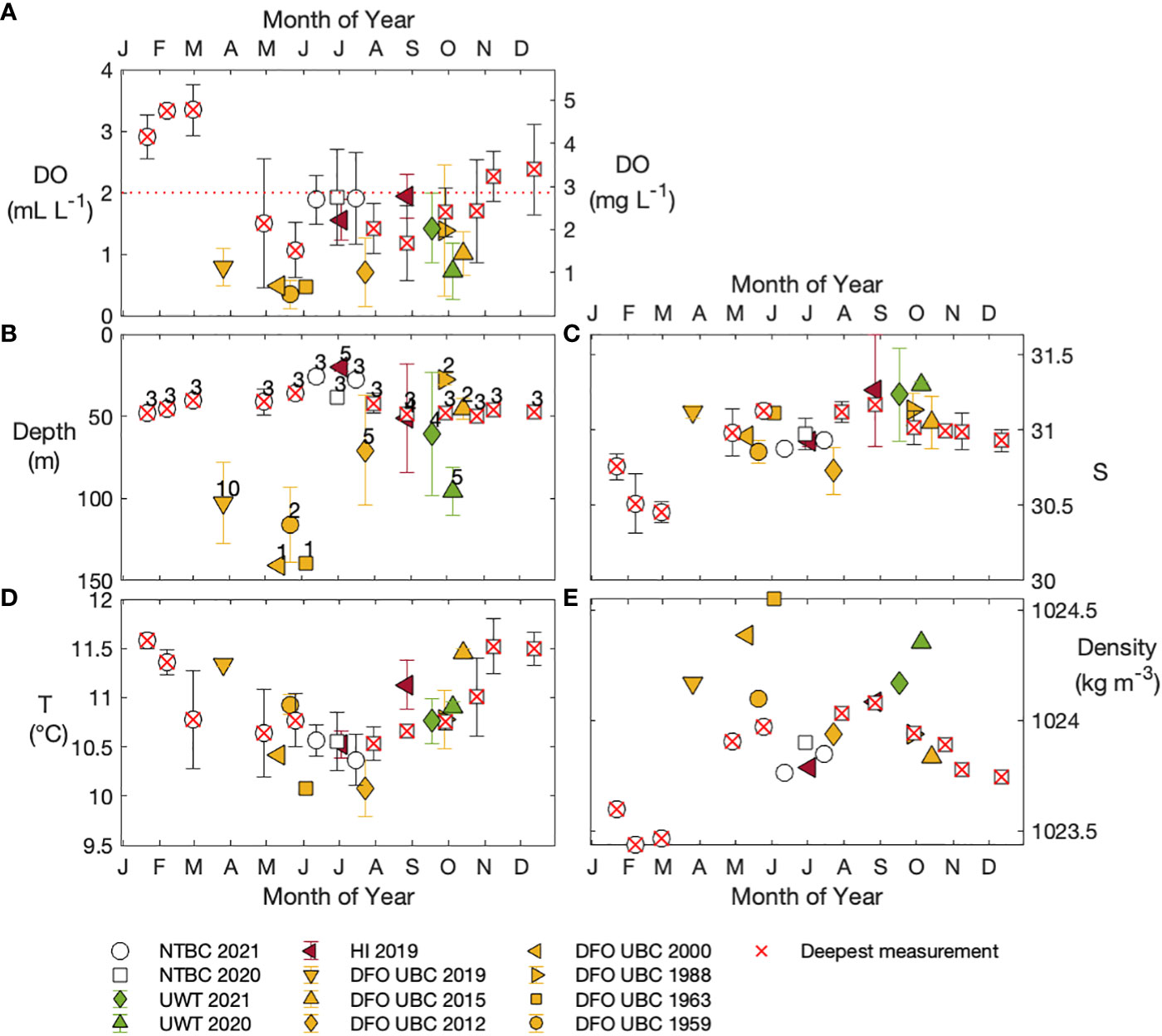
Figure 4 Seasonal comparison of NTBC data (Tables S4–S6; open circles and squares) and historical properties: (A) oxygen concentration of Omin50 and Omin, (B) depth of Omin50 and Omin, and (C–E). salinity, temperature, and density at Omin50 and Omin locations. Red crosses inside NTBC data markers denote months in which Omin50 was the deepest recorded measurement at one or more stations (data occupied top ~50 m only). Historical data span the full water column and are shown in yellow (Fisheries and Oceans Canada/University of British Columbia), red (Hakai Institute), and green (University of Washington - Tacoma); different shapes correspond to different sampling years. Omin and Omin50 properties in all datasets are spatially averaged (error bars show one standard deviation). Numbers above data points in panel (B) specify the number of stations for each legend entry.
Between the months of March and June, spatially-averaged historical Omin were consistently deeper than 50 m (Figure 4B), strongly suggesting that our monthly dataset (limited to the upper 50 m) does not capture the lowest oxygen concentrations in the inlet. From July to October, the historical Omin occurred both above and below 50 m, suggesting high interannual variability in the location of the most oxygen-depleted waters (Figure 4B; Figure S7). No historical data exist for the months of November to February, but evidence of physical mixing and reoxygenation of waters at and slightly above 50 m (Figures 2F–I; Figure 3C) suggests that the lowest oxygen concentration in November – February occurred below the deepest NTBC measurements.
In general, the salinity at historical Omin does not differ from NTBC Omin50 salinities by more than ~ 0.5, regardless of the historical Omin depth (Figure 4C). Historical Omin temperatures were rarely colder than those observed at Omin50 above 50 m between June 2020 – July 2021 (Figure 4D), and no clear pattern exists between either historical Omin temperature or depth in relation to NTBC 2020 – 2021 observations (Figures 4A–D). The historical observations that showed shallow Omin depths comparable to those of the NTBC dataset (i.e., ≤ 50 m; Figure 4B) also had similar Omin densities (~ 1023.8 – 1024.1 kg m-3; Figure 4E). Overall, these results demonstrate that interannual and spatial variability is important in Herbert Inlet and emphasize the need for future sampling to include full water column measurements, year-round.
4 Discussion and conclusions
Monthly observations from June 2020 to July 2021 showed the presence of hypoxic conditions near the surface of Herbert Inlet, a temperate fjord in the British Columbia coast. Hypoxia was as shallow as 12 m deep in summer of 2020, occupying up to 75% of the top 50 m of the water column near the head of the inlet. Some of the historical observations available show similarly shallow hypoxic waters, while others show hypoxia at depth, which is the typically expected location of low oxygen waters in fjords (e.g., Pickard, 1963; Silva and Vargas, 2014; Björk et al., 2017). These results highlight the importance of measuring and studying hypoxia at seasonal, annual, and interannual timescales, emphasizing the need for long-term monitoring efforts to understand this process and how it may be affected by climate change. Below, we discuss potential mechanisms that cause seasonal near-surface hypoxia in the upper waters of Herbert Inlet and reflect on the need for a better understanding of hypoxia dynamics and their connection with the decline of wild salmon populations.
4.1 Causes of seasonal hypoxia in the upper 50 m of Herbert Inlet
Oceanographic circulation in the CS region is complex: Herbert Inlet alone has two connections with the open ocean (Russel and Millar Channels), each with its own sill. Because we lacked year-round full water column data (see section 2.1), the following discussion of physical and biological processes is constrained to the upper 50 m of Herbert Inlet. Future studies should collect year-round full water column data to understand how large-scale atmospheric and oceanographic forcing influence oxygen over the entire water column in Herbert Inlet.
4.1.1 External processes
Annual deep-water renewal, which replaces fjord bottom waters with cold, usually higher-oxygen offshore water, is common in most of BC’s fjords and in other enclosed coastal systems (e.g., Anderson and Devol, 1973; Johannessen et al., 2014). Deep-water renewal is typically driven by upwelling-favourable winds, which dominate from April to October on the BC coast (Thomson et al., 2014). In Rivers Inlet, Jackson et al. (2021b) found that deep-water renewal formed the base of a seasonal Oxygen Minimum Layer (OML); above the upwelled water, the low-oxygen conditions observed at mid-depth were created by increased in situ biological oxygen consumption following the cessation of winter downwelling winds. With the NTBC dataset, we are unable to properly examine deep-water renewal and characterize an OML; however, analyzing wind data and water properties on the outer shelf offers insight into causes of low oxygen in the upper water column in Herbert Inlet.
The Cumulative Upwelling Index showed large-scale upwelling starting in late March 2021 (Figure 3A). Data from mooring E01 were compared to CTD profiles from the mouth of Herbert Inlet before, during, and after the transition period and showed that the densities of shelf waters at 75 m and 95 m did not match waters at the mouth of Herbert Inlet at any depth in any season (Figure S8). This result indicates that either a) water upwelled to 75 – 95 m depth on the outer WCVI shelf is not advected into the upper 50 m in Herbert Inlet, or b) upwelled water is vigorously mixed and consequently modified over the shallow entrance sills. Therefore, even if E01 becomes hypoxic in summer (see Figure S9), it is highly unlikely that those waters are directly responsible for the seasonal hypoxia above 50 m in Herbert Inlet (Figure 2 and 3). Nevertheless, upwelling may have an indirect effect on oxygen, as it may increase nutrient availability in the spring and enhance local biological processes (described in more detail below).
4.1.2In situ processes
In all months where hypoxic conditions were observed above 50 m, low-oxygen conditions intensified towards the head of Herbert Inlet (Figure 2). Identical spatial gradients of low oxygen in other fjord systems have been attributed to limited vertical mixing caused by persistent haloclines/pycnoclines and to the degradation of terrigenous organic matter at the head (Silva and Vargas, 2014), further suggesting that hypoxia in the upper 50 m of Herbert Inlet generated primarily in situ rather than imported from outside the inlet. In Rivers Inlet, a mid-depth Omin was associated with spatial gradients in Osat (that declined toward the head) and AOU (that increased toward the head; Jackson et al., 2021b). Likewise, Osat is depleted and AOU is enhanced at the head relative to the mouth at Omin50 in the upper 50 m of Herbert Inlet year-round (Figure S10), implying that local metabolic DO consumption is at least partially responsible for creating the seasonally low-oxygen conditions presented in Figure 2. Furthermore, local biological processes in Herbert Inlet may be enhanced by larger-scale upwelling. While upwelled waters from the shelf cannot arrive with low DO concentrations due to the reoxygenation expected to occur over the sills, they could still bring nutrients into the inlet. The combination of increased solar radiation in spring and the extra input of nutrients from upwelling could lead to high primary production, which eventually decays and degrades, consuming oxygen. More observational and modelling research is needed to fully understand the role of biology and nutrients in creating near-surface hypoxia in Herbert Inlet.
Another in situ DO sink is the respiration of organic matter associated with nutrient and waste discharges from finfish aquaculture (e.g., Lee et al., 1991; Lee et al., 2003). While the resolution of our dataset does not allow for an in-depth exploration of the extent of this process, we note that hypoxia was recorded in 1959 and 1963 (Figure 4) before the start of salmon farming in BC in the mid-1970s (BC Salmon Farmers Association, https://bcsalmonfarmers.ca/history/). Future analyses should explore whether the frequency and strength of hypoxia were modified after the aquaculture industry started operations in CS.
4.2 Reflections on hypoxia, salmon, and addressing knowledge gaps
As in most other CS rivers, wild salmon runs in the Moyeha River have recently declined (Monks et al., 2022). Chinook salmon returns in CS are below management benchmarks (COSEWIC, 2020) and have triggered a federal inquiry and management action (DFO, 2021). Among many stressors, the presence of open net-pen finfish farms has been identified as a risk factor to wild salmon populations, as they can release pathogens and contaminants to the environment (Cohen, 2012). Understanding and identifying responses of salmon populations to stressors like deoxygenation are key to assessing the role of climate change in the health of ocean ecosystems and the sustainability of livelihoods and communities.
A growing body of research demonstrates that salmon are vulnerable to and influenced by low oxygen conditions, particularly in conjunction with additional stressors such as water temperature (Houde et al., 2019; Akbarzadeh et al., 2020). Shoaled hypoxic waters and elevated sea-surface temperatures cause vertical habitat compression (Bakun et al., 2015), which may increase interaction with predators, parasites, and pathogens, and possibly decrease feeding opportunities (Breitburg et al., 2018). Thus, projected deoxygenation and warming in fjord seawater (Jackson et al., 2021a) will likely pose an increasing threat to salmon populations.
Our results showed low oxygen conditions on most of the upper 50 m of Herbert Inlet, with hypoxia reaching depths of ~ 10 – 20 m in summer and waters with DO less than 4.9 mL L-1 (6.9 mg L-1) occupying 50 to 100% of the water column all year. While these conditions seem unfavourable for salmon, the scarce historical record shows the presence of hypoxic waters at similar depths in the past, so it is not possible to rule out acclimation to low oxygen (Côte et al., 2012; Wellband et al., 2018) in local stocks. Furthermore, other stressors likely contribute to the long term declines (e.g. open net-pen aquaculture; Cohen, 2012). Overall, closer collaboration between multiple disciplines and a ‘two-eyed seeing’ approach that includes Indigenous knowledge (Reid et al., 2021) are needed to understand the causes of wild salmon decline. In particular, a relationship between wild salmon decline and deoxygenation (as with warming, ocean acidification, and climate change) is difficult to establish without sustained long-term environmental and population monitoring. In conclusion, year-round, full depth water property profiling of Clayoquot Sound inlets, in conjunction with salmon monitoring and research are necessary to address these issues. Collaborations between research institutions, non-profit organizations, and First Nations groups, like the partnership that produced the NTBC dataset studied here, are key to achieve the needed long-term monitoring.
Data availability statement
The datasets presented in this study can be found in onlinerepositories. The names of the repository/repositories and accession number(s) can be found below: Dryad Repository, https://datadryad.org/stash/share/bAoqE97kJuF509q09npGzE7dXY18bH0GQALvw1w5yuI.
Author contributions
Conception of the work: JJ; Supervision of the work: LB and JJ; Data collection: JD; Data analysis/interpretation: SR, LB, JJ, AH, CG, RM, and MB; Initial draft: SR supported by LB, JJ, AH, CG, RM, and MB; Submitted draft and revised manuscript: LB supported by JJ, AH, CG, and SR. Figures: AH (except for Figure 1). All authors contributed to the article and approved the submitted version.
Funding
This project was funded by Cedar Coast Field Station Society (SR, MB, and RM), Fisheries and Oceans Canada’s Competitive Science Research Fund (CSRF; SR and LB), the Tula Foundation (JJ and AH), and the University of Washington - Tacoma (CG).
Acknowledgments
We acknowledge with gratitude that this research was conducted within the traditional territories of the Ahousaht First Nation. We thank the Nature Trust of British Columbia, Uu-a-thluk Fisheries, the Hakai Institute, Fisheries and Oceans Canada, Clayoquot Biosphere Trust, the University of Washington-Tacoma, and the University of British Columbia for collecting and processing the data used in this project. Keith Holmes created Figure 1. Danny O’Farrell and the Maaqutusiis Hahoulthee Stewardship Society provided feedback. Three reviewers and editor SS provided valuable comments and feedback.
Conflict of interest
The authors declare that the research was conducted in the absence of any commercial or financial relationships that could be construed as a potential conflict of interest.
Publisher’s note
All claims expressed in this article are solely those of the authors and do not necessarily represent those of their affiliated organizations, or those of the publisher, the editors and the reviewers. Any product that may be evaluated in this article, or claim that may be made by its manufacturer, is not guaranteed or endorsed by the publisher.
Supplementary material
The Supplementary Material for this article can be found online at: https://www.frontiersin.org/articles/10.3389/fmars.2022.1000041/full#supplementary-material
References
Akbarzadeh A., Houde A. L. S., Sutherland B. J., Günther O. P., Miller K. M. (2020). Identification of hypoxia-specific biomarkers in salmonids using RNA-sequencing and validation using high-throughput qPCR. G3.: Genes. Genomes. Genet. 10 (9), 3321–3336. doi: 10.1534/g3.120.401487
Anderson J. J., Devol A. H. (1973). Deep water renewal in saanich inlet, an intermittently anoxic basin. Estuar. Coast. Mar. Sci. 1 (1), 1–10. doi: 10.1016/0302-3524(73)90052-2
Bakun A. (1990). Global climate change and intensification of coastal ocean upwelling. Science 247 (4939), 198–201. doi: 10.1126/science.247.4939.198
Bakun A., Black B. A., Bograd S. J., Garcia-Reyes M., Miller A. J., Rykaczewski R. R., et al. (2015). Anticipated effects of climate change on coastal upwelling ecosystems. Curr. Climate Change Rep. 1 (2), 85–93. doi: 10.1007/s40641-015-0008-4
Birtwell I. K., Hyatt K., Korstrom J. S., Kruzynski G. M., Langton C. M., Piercey G. E., et al. (1994). “Behavior and physiological responses of adult sockeye (Oncorhynchus nerka) salmon to simulated estuarine conditions and salt water hypoxia. in high performance fish,” in Proceedings of an international fish physiology symposium. Ed. Mackinley D. D. (University of British Columbia, Vancouver: American Fisheries Society and Fish Physiology Association), 243–245.
Birtwell I. K., Kruzynski G. M. (1989). In situ and laboratory studies on the behaviour and survival of pacific salmon (genus oncorhynchus). Hydrobiologia 188 (1), 543–560. doi: 10.1007/BF00027822
Björk G., Nordberg K., Arneborg L., Bornmalm L., Harland R., Robijn A., et al. (2017). Seasonal oxygen depletion in a shallow sill fjord on the Swedish west coast. J. Mar. Syst. 175, 1–14. doi: 10.1016/j.jmarsys.2017.06.004
Boyer T., Conkright M. E., Levitus S. (1999). Seasonal variability of dissolved oxygen, percent oxygen saturation, and apparent oxygen utilization in the Atlantic and pacific oceans. Deep. Sea. Res. Part I.: Oceanogr. Res. Papers. 46 (9), 1593–1613. doi: 10.1016/S0967-0637(99)00021-7
Breitburg D., Levin L. A., Oschlies A., Grégoire M., Chavez F. P., Conley D. J., et al. (2018). Declining oxygen in the global ocean and coastal waters. Science 359 (6371), eaam7240. doi: 10.1126/science.aam7240
Carpenter J. H. (1965). The accuracy of the winkler method for dissolved oxygen analysis 1. Limnol. Oceanogr. 10 (1), 135–140. doi: 10.4319/lo.1965.10.1.0135
Cohen B. I. (2012). “The uncertain future of Fraser river sockeye, volume 2: Causes of the decline,” in Commission of inquiry into the decline of sockeye salmon in the Fraser river - final report (Ottawa, Ontario: Bruce I. Cohen, commissioner.
COSEWIC (2020). Committee on the status of endangered wildlife in Canada (COSEWIC) assessment and status report on the Chinook salmon Oncorhynchus tshawytscha, designatable units in southern British Columbia (Part two - designatable units with high levels of artificial releases in the last 12 years), in Canada (Ottawa: Committee on the Status of Endangered Wildlife in Canada), 203.
Côte J., Roussel J. M., Le Cam S., Bal G., Evanno G. (2012). Population differences in response to hypoxic stress in Atlantic salmon. J. Evol. Biol. 25 (12), 2596–2606. doi: 10.1111/jeb.12007
Davis J. C. (1975). Minimal dissolved oxygen requirements of aquatic life with emphasis on Canadian species: a review. J. Fisheries. Board. Canada. 32 (12), 2295–2332. doi: 10.1139/f75-268
Davis G. E., Foster J., Warren C. E., Doudoroff P. (1963). The influence of oxygen concentration on the swimming performance of juvenile pacific salmon at various temperatures. Trans. Am. Fisheries. Soc. 92 (2), 111–124. doi: 10.1577/1548-8659(1963)92[111:TIOOCO]2.0.CO;2
Del Rio A. M., Davis B. E., Fangue N. A., Todgham A. E. (2019). Combined effects of warming and hypoxia on early life stage Chinook salmon physiology and development. Conserv. Physiol. 7 (1), coy078. doi: 10.1093/conphys/coy078
DFO (2021) Wild salmon policy 2018 – 2019 implementation plan. Available at: https://waves-vagues.dfo-mpo.gc.ca/Library/41031209.pdf.
Diaz J. M., Ingall E. D., Snow S. D., Benitez-Nelson C. R., Taillefert M., Brandes J. A. (2012). Potential role of inorganic polyphosphate in the cycling of phosphorus within the hypoxic water column of effingham inlet, British Columbia. Global Biogeochem. Cycles. 26 (2), GB2040. doi: 10.1029/2011GB004226
Diaz R. J., Rosenberg R. (2008). Spreading dead zones and consequences for marine ecosystems. Science 321 (5891), 926–929. doi: 10.1126/science.1156401
Foreman M. G. G., Pal B., Merryfield W. J. (2011). Trends in upwelling and downwelling winds along the British Columbia shelf. J. Geophys. Res.: Oceans. 116 (C10023), 1–11. doi: 10.1029/2011JC006995
Garcia H. E., Gordon L. I. (1992). Oxygen solubility in seawater: Better fitting equations. Limnol. Oceanogr. 37 (6), 1307–1312. doi: 10.4319/lo.1992.37.6.1307
García-Reyes M., Sydeman W. J., Schoeman D. S., Rykaczewski R. R., Black B. A., Smit A. J., et al. (2015). Under pressure: Climate change, upwelling, and eastern boundary upwelling ecosystems. Front. Mar. Sci. 2. doi: 10.3389/fmars.2015.00109
Genovesi L., De Vernal A., Thibodeau B., Hillaire-Marcel C., Mucci A., Gilbert D. (2011). Recent changes in bottom water oxygenation and temperature in the gulf of st. Lawrence: Micropaleontological and geochemical evidence. Limnol. Oceanogr. 56 (4), 1319–1329. doi: 10.4319/lo.2011.56.4.1319
Gilbert D., Rabalais N. N., Diaz R. J., Zhang J. (2010). Evidence for greater oxygen decline rates in the coastal ocean than in the open ocean. Biogeosciences 7 (7), 2283. doi: 10.5194/bg-7-2283-2010
Grantham B. A., Chan F., Nielsen K. J., Fox D. S., Barth J. A., Huyer A., et al. (2004). Upwelling-driven nearshore hypoxia signals ecosystem and oceanographic changes in the northeast pacific. Nature 429 (6993), 749–754. doi: 10.1038/nature02605
Houde A. L. S., Akbarzadeh A., Günther O. P., Li S., Patterson D. A., Farrell A. P., et al. (2019). Salmonid gene expression biomarkers indicative of physiological responses to changes in salinity and temperature, but not dissolved oxygen. J. Exp. Biol. 222 (13), jeb198036. doi: 10.1242/jeb.198036
Jackson J. M., Bianucci L., Hannah C. G., Carmack E. C., Barrette J. (2021a). Deep waters in British Columbia mainland fjords show rapid warming and deoxygenation from 1951 to 2020. Geophys. Res. Lett. 48 (3), e2020GL091094. doi: 10.1029/2020GL091094
Jackson J. M., Johannessen S., Del Bel Belluz J., Hunt B. P., Hannah C. G. (2021b). Identification of a seasonal subsurface oxygen minimum in rivers inlet, British Columbia. Estuaries. Coasts. 45, 1–18. doi: 10.1007/s12237-021-00999-y
Johannessen S. C., Masson D., Macdonald R. W. (2014). Oxygen in the deep strait of Georgia 1951–2009: The roles of mixing, deep-water renewal, and remineralization of organic carbon. Limnol. Oceanogr. 59 (1), 211–222. doi: 10.4319/lo.2014.59.1.0211
Kusch S., Wakeham S. G., Dildar N., Zhu C., Sepúlveda J. (2021). Bacterial and archaeal lipids trace chemo(auto)trophy along the redoxcline in Vancouver island fjords. Geobiology 19, 521–541. doi: 10.1111/gbi.12446
Lee J. H. W., Choi K. W., Arega F. (2003). Environmental management of marine fish culture in Hong Kong. Mar. pollut. Bull. 47, 202–210. doi: 10.1016/S0025-326X(02)00410-1
Lee J. H. W., Wu R. S. S., Chung Y. K. (1991). Forecasting of dissolved oxygen in marine fish culture zone. J. Environ. Eng. 117 (12), 816–833. doi: 10.1061/(ASCE)0733-9372(1991)117:6(816
Monks R., Carson C. M., Tersigni C., Hollyer K., Simmerling J., Bartlett M. (2022). Stream-level population assessment of salmon on the West coast of Vancouver island and clayoquot sound (Vargas Island, BC: A report from the Cedar Coast Field Station Society). Available at: https://www.cedarcoastfieldstation.org.
Pawlowicz R. (2017). Seasonal cycles, hypoxia, and renewal in a coastal fjord (Barkley sound, British Columbia). Atmosphere-Ocean 55 (4-5), 264–283. doi: 10.1080/07055900.2017.1374240
Pickard G. L. (1961). Oceanographic features of inlets in the British Columbia mainland coast. J. Fisheries. Board. Canada. 18 (6), 907–999. doi: 10.1139/f61-062
Pickard G. L. (1963). Oceanographic characteristics of inlets of Vancouver island, British Columbia. J. Fisheries. Board. Canada. 20 (5), 1109–1144. doi: 10.1139/f63-080
Reid A. J., Eckert L. E., Lane J.-F., Young N., Hinch S. G., Dairmont C. T., et al. (2021). “Two-eyed seeing: An indigenous framework to transform fisheries research and management. Fish. Fisheries. 22, 243–261. doi: 10.1111/faf.12516
Schmidtko S., Stramma L., Visbeck M. (2017). Decline in global oceanic oxygen content during the past five decades. Nature 542 (7641), 335–339. doi: 10.1038/nature21399
Silva N., Vargas C. A. (2014). Hypoxia in Chilean Patagonian fjords. Prog. Oceanogr. 129, 62–74. doi: 10.1016/j.pocean.2014.05.016
Sydeman W. J., García-Reyes M., Schoeman D. S., Rykaczewski R. R., Thompson S. A., Black B. A., et al. (2014). Climate change and wind intensification in coastal upwelling ecosystems. Science 345 (6192), 77–80. doi: 10.1126/science.1251635
Thomson R. E., Heesemann M., Davis E. E., Hourston R. A. (2014). Continental microseismic intensity delineates oceanic upwelling timing along the west coast of north America. Geophys. Res. Lett. 41 (19), 6872–6880. doi: 10.1002/2014GL061241
Thomson R. E., Spear D. J., Krassovski M. V., Hourston R. A., Juhász T. A., Mihály S. F. (2017). Buoyancy-driven coastal current blocks ventilation of an anoxic fjord on the pacific coast of Canada. J. Geophys. Res.: Oceans. 122 (4), 2976–2998. doi: 10.1002/2016JC012512
Vaquer-Sunyer R., Duarte C. M. (2008). Thresholds of hypoxia for marine biodiversity. Proc. Natl. Acad. Sci. 105 (40), 15452–15457. doi: 10.1073/pnas.0803833105
Keywords: dissolved oxygen (DO), hypoxia, fjord, Herbert Inlet, Clayoquot Sound, British Columbia (BC)
Citation: Rosen S, Bianucci L, Jackson JM, Hare A, Greengrove C, Monks R, Bartlett M and Dick J (2022) Seasonal near-surface hypoxia in a temperate fjord in Clayoquot Sound, British Columbia. Front. Mar. Sci. 9:1000041. doi: 10.3389/fmars.2022.1000041
Received: 21 July 2022; Accepted: 20 October 2022;
Published: 07 November 2022.
Edited by:
Sabine Schmidt, Centre National de la Recherche Scientifique (CNRS), FranceReviewed by:
Arash Akbarzadeh, University of Hormozgan, IranMichael Price, Simon Fraser University, Canada
Copyright © 2022 Rosen, Bianucci, Jackson, Hare, Greengrove, Monks, Bartlett and Dick. This is an open-access article distributed under the terms of the Creative Commons Attribution License (CC BY). The use, distribution or reproduction in other forums is permitted, provided the original author(s) and the copyright owner(s) are credited and that the original publication in this journal is cited, in accordance with accepted academic practice. No use, distribution or reproduction is permitted which does not comply with these terms.
*Correspondence: Laura Bianucci, TGF1cmEuQmlhbnVjY2lAZGZvLW1wby5nYy5jYQ==