Arginine as the sole nitrogen source for Ostreococcus tauri growth: Insights on nitric oxide synthase enzyme
- 1Molecular and Integrative Physiology Lab, Instituto de Investigaciones Biológicas-CONICET, Universidad Nacional de Mar del Plata, Mar del Plata, Argentina
- 2INBIOTEC and FIBA, Mar del Plata, Argentina
Introduction: Photosynthetic organisms respond to nitrogen (N) deprivation with the slowdown of photosynthesis and electron transport resulting in the balance the carbon (C)/N ratio. Under this extreme condition, organisms trigger complex mechanisms to keep growing using different N sources and recycling N containing molecules. In particular, phytoplankton are able to uptake L-arginine (L-Arg) as an organic N source. L-Arg can be assimilated mainly by the arginase, arginine deimidase, arginine decarboxylase or L-amino oxidase pathways.
Results: We analyzed the effect of different N sources on the growth of the green algae Ostreococcus tauri. N starvation caused an inhibition of culture growth and a decrease in chlorophyll content. The addition of L-Arg to an N-deprived medium promotes a sustained growth rate of O. tauri culture and the increase of chlorophyll levels. The transcript level of genes involved in N uptake and metabolism were increased in N-starved condition while the addition of L-Arg as the sole N source reduced their induction. Since the O. tauri genome lacks the classical pathways to metabolize L-Arg, another enzyme/s may be responsible for L-Arg catabolism. Previously, we characterized the nitric oxide synthase (NOS) enzyme from O. tauri (OtNOS) which oxidizes L-Arg producing nitric oxide (NO) and citrulline. The NOS inhibitor L-NAME blocks the effect promoted by L-Arg on N-deprived O. tauri growth. Besides, NO level increased in O. tauri cells growing in L-Arg containing medium, suggesting the participation of OtNOS enzyme in L-Arg metabolism during N starvation.
Discussion: Our hypothesis suggests that, after NOS-dependent Arg degradation, non-enzymatic oxidation of NO produces N oxides (mainly NO2-) that are re-incorporated to the N primary metabolism. As expected, N deprivation increases the lipid content in Ostreococcus. The addition of L-Arg or NO2- as the sole N sources showed a similar increase in lipid content to N deprivation. In summary, our results demonstrate that L-Arg is able to function as N source in Ostreococcus. The evidences on an alternative pathway of N supply and metabolism in a photosynthetic microorganism are discussed. These results could also allow the development of biotechnological tools for increasing lipid production for industry.
Introduction
All living organisms require constant nitrogen (N) supply for growth and survival. N is an essential macronutrient necessary for the biosynthesis of amino acids, proteins and nucleic acids. The ocean’s N cycle is driven by complex microbial transformations, including N fixation, assimilation, nitrification and denitrification. N can be incorporated in eukaryotic organisms from either organic or inorganic forms. The availability and concentrations of inorganic N sources fluctuate in the environment limiting growth and productivity (Raven et al., 2014). Ammonia (NH4+), nitrite (NO2-) and nitrate (NO3-) with different spatial distributions in oceans are the most frequent inorganic N sources assimilated by photosynthetic organisms (Gruber and Galloway, 2008).
Based on the function they fulfill within the ecosystem; microalgae possess diverse adaptations for an appropriate N uptake and assimilation. Two adaptive strategies are generally considered when N sources are insufficient: i) increase uptake of N sources by inducing high-affinity transporters and/or by changing growth patterns or chemotaxis, known collectively as N scavenging, and ii) mobilize internal N reserves such as storage proteins and N-rich molecules, known collectively as N salvaging (Andersson et al., 2006; Sanz-Luque et al., 2015; Terrado et al., 2015; Shahar et al., 2020; Lage et al., 2021; van Tol and Armbrust, 2021). The expression of genes that participate in N collecting and recovering is inhibited when the primary N sources are available. This response to N source quality in the growing medium is called N catabolite repression and has been extensively studied in the model system Saccharomyces cerevisiae (Zhang et al., 2018). Assimilation of inorganic N sources requires a significant amount of carbon (C) skeletons and reducing equivalents forcing an increase in photosynthetic products (Foyer et al., 2018). Under N-limitation, many photosynthetic organisms change their C storage pattern in favor of carbohydrates and neutral lipids (NLs) mainly in the form of triacylglycerol (TAG) as a protective response (Illman et al., 2000; Rodolfi et al., 2009; Li et al., 2012; Johnson and Alric, 2013; Rodolfi et al., 2017).
Generally, unicellular algae show little significant ability to use amino acids supplied at near-natural concentrations (Flynn, 1990). In this sense, among 18 amino acids tested, only L-Arg was efficiently assimilated in Chlamydomonas reinhardtii and Volvox carteri (Kirk and Kirk, 1978; Muñoz-Blanco et al., 1990). Munz et al. (2020), recently demonstrated that L-Arg-fed cultures of C. reinhardtii activate N catabolic genes together with N starvation-induced responses such as gametogenesis and TAG accumulation while supporting a growth rate comparable to even higher than NH4+-fed cultures. Additionally, L-Arg sustains growth and lipid accumulation in the non-photosynthetic green alga Polytomella parva (Lapina et al., 2022). In photosynthetic eukaryotic organisms, several N-producing ways of L-Arg degradation were described: the arginase pathway which produces ornithine and urea, the arginine decarboxylase (ADC) pathway that converts L-Arg to agmatine and then to N-carbamoyl-putrescine and NH4+ (by agmatine iminohydrolase; AIH) and the L-Arg deiminase (ADI) pathway which produces NH4+, CO2, and ATP in 3 step-enzyme reactions (Vallon and Spalding, 2009). Moreover, the L-amino oxidase (LAO) activity can also provide assimilable N from L-Arg (Calatrava et al., 2019).
Picoplankton is the smallest group of microorganisms of the plankton with a diameter of 0.2-3 μm, and are the most abundant primary producers in the oceans, despite representing less than 1% of the photosynthetic biomass (Falkowski, 1994; Field et al., 1998; Falkowski et al., 2008; Seymour, 2014). Therefore, a change in the phytoplankton population will severely affect the biogeochemical cycles. The picoeukaryote Ostreococcus represents a diversified and widely distributed genus within the algae (Le Bihan et al., 2011). Ostreococcus is one of the smallest picoeukaryote, with a very simple cell structure, including only one chloroplast and mitochondrion and no flagella (Six et al., 2005; Cardol et al., 2008; Demir-Hilton et al., 2011). Specifically, in Ostreococcus tauri, we characterized three calcium-dependent protein kinases (CDPKs) and showed that they are involved in the stress response to N deprivation (Caló et al., 2017). Moreover, it was proposed that CDPK signaling originated early in the green lineage is crucial for nutrient perception in Prasinophytes. In a recent study, we described the TOR kinase and its regulation under nutrient stress conditions in O. tauri, highlighting the importance of this microalgae as a model for studying signaling pathways in photosynthetic organisms (Caló et al., 2022).
Additionally, we described a nitric oxide synthase in O. tauri (OtNOS) that metabolizes L-Arg generating NO and citrulline (Foresi et al., 2010; Weisslocker-Schaetzel et al., 2017). The recombinant OtNOS has a Km for the substrate L-Arg of ∼12 μM and its activity is suppressed by the specific inhibitor L-NAME. NOS activity is 3-fold higher during the exponential growth phase than the stationary phase and is increased under high light, evidencing a link between NO production and the microalgal physiology (Foresi et al., 2010).
The influence of different N conditions on O. tauri cell physiology was investigated in the present study. Results showed that N deficiency triggers growth inhibition and a decrease of chlorophyll content in the first days of treatment. On the other hand, L-Arg supplementation to N-deprived cells allows this culture to grow at similar rates to those of N-complete medium for the first 10 days. Based on in silico analysis, we propose a possible pathway of L-Arg catabolism that may overlook the N physiological status. Our results report a study system where N starvation responses are induced without compromising growth, unraveling the way in which cellular N status in eukaryotic phototrophs is sensed and modified. This evidence would allow the development of biotechnological tools that could increase algae growth and lipids content under N deprivation.
Materials and methods
Reagents
Antibiotics penicillin, neomycin and kanamycin as well as amino acids L-Arginine (L-Arg) and L-Lysine (L-Lys), Nω-Nitro-L-arginine methyl ester hydrochloride (L-NAME), were purchased at Sigma-Aldrich. Sodium nitrite and sodium tungstate were purchased in BDH Chemicals.
Biological material and culture conditions
Ostreococcus tauri OTTH0595 (RCC745) cell cultures were obtained from Roscoff Culture Collection. Cells were grown in Keller medium (MK) (8.82 x 10-4 M NaNO3, 5 x 10-5 M NH4Cl, 1 x 10-5 M Na2 β-glycerophosphate.6H2O, 1 x 10-8 M H2SeO3, 1 x 10-3 M Tris-base (pH 7.2), trace metal solution (1.12 x 10-4 M Na2EDTA.2H2O, 1.17 x 10-5 M FeCl3.6H2O, 9.1 x 10-7 M MnCl2.4H2O, 7.65 x 10-8 M ZnSO4.7H2O, 4.2 x 10-8 M CoCl2.6 H2O, 2.6 x 10-8 M Na2MoO4.2H2O, 3.92 x 10-8 M CuSO4.5H2O), supplemented with f/2 vitamin solution (1 x 10-10 M cyanocobalamin, 1 x 10-9 M biotin, 1 x 10-7 thiamine-HCl) and filtered through 0.22 μm filters before use (Keller et al., 1987). The cultures were grown in the presence of penicillin 25 µg.ml-1, neomycin 20 µg.ml-1 and kanamycin 25 µg.ml-1, and maintained under a 12:12 h light: dark regime in 60 µE white light at 20 ± 1°C. For growth curves, cells were cultured in 15 ml of MK starting at OD 660 nm of 0.2 units and readings were taken each 48 h.
For N starvation, amino acid and inhibitor treatments, 15 ml of cells were centrifuged at 3,500 rpm in conical tubes for 20 min at room temperature. Cell pellets were resuspended depending on treatment in: (MK, control), MK lacking N source [MK (-N)] or MK (-N) supplemented with the different N sources (L-Arg, L-Lys, NO2-) and inhibitors (L-NAME, tungstate). The osmotic balance in MK (-N) was covered by equal NaCl quantities. Cultures were grown for 17-19 d.
Chlorophyll was extracted with 100% ethanol for 15 min in the dark. Ethanolic extracts were centrifuged at 8,000 rpm for 10 min at 4°C and chlorophyll levels were determined by measuring at 665 nm (OD665). Cell count and chlorophyll fluorescence intensity were assessed by flow cytometry in a Partec Cyflow Space cytometer using FloMax software. Data analysis was performed with FlowJo software (https://www.flowjo.com/).
Lipid quantification
Total lipids were determined by the sulfo-phospho-vanillin method using commercial canola oil as lipid standard (2 mg.ml-1). On 20 μl of reaction volume, 400 μl of concentrated sulfuric acid was added and the samples were boiled for 10 min. After that, 1 ml vanillin reagent was added. The samples were incubated for 15 min at 37°C, shaking at 200 rpm. The absorbance was read at 530 nm (Mishra et al., 2014).
RNA isolation, reverse transcription and quantitative RT-PCR
O. tauri cultures (150 ml) of each treatment were harvested at exponential phase and sonicated at 40 W in an ice bath (10 cycles of 10 s, followed by a 10 s pause each). Total RNA was isolated using the RNeasy plant mini kit (Qiagen).
Quantitation of RNA was determined using the Nanodrop spectrophotometer (Thermo Scientific). Treatment with DNase was performed after RNA extraction at 37°C for 1 h using DNase (Promega). Reverse transcription was performed from 3 µg of total RNA, 1 μl of random primers (Macrogen), 1 μl of 10 mM dNTP, 2 μl of 0.1 M DTT and M-MLV reverse transcriptase (Invitrogen) in a final volume of 20 μl. For RT-qPCR, reactions were performed on a Step-one Real-time PCR system (Applied Biosystems, California, USA) with Fast Universal SYBR Green Master Rox (Roche) to monitor double-stranded DNA synthesis. LinRegPCR software v2014.7 (Ruijter et al., 2009) was used to calculate N0 values, which reflects the initial amount of template. Data were normalized against the levels of GAPDH transcript. The primer sequences used are listed in Supplemental Table S1.
NO production in O. tauri cultures
NO content in O. tauri cultures was quantified using the NO-sensitive probe 4-amino-5-methylamino-2’,7’-difluorofluorescein diacetate (DAF-FM DA, Invitrogen). DAF-FM DA (10 μM) was added to the culture medium and incubated in dark 20 min before measurement. NO fluorescence intensity (excitation 495 nm; emission 525 nm) was measured using a fluorescence plate reader (Fluoroskan Ascent; Thermo Electron) over an hour. Data are expressed as a fold increase of arbitrary units (a.u.) per min.
Statistical analysis
Results are expressed as means ± standard error (SE) or standard deviations (SD). Data were analyzed using ANOVA with post-hoc Tukey or Dunnett’s method comparisons as indicated. We have developed a linear mixed-effects model, using the lme function from the nlme library in R software (version 3.1; R Foundation for Statistical Computing). Fixed effect was the nutrient media; experiments were treated as a random effect.
Results
O. tauri can grow in the presence of the amino acid L-Arg as sole N source
To analyze the effect of N deprivation and the utilization of L-Arg as N source, O. tauri was grown on Keller medium (MK), MK without N supply (MK(-N)) or (MK(-N)) supplemented with L-Arg as the sole N source. Growth and cell number were determined by optical density (OD 660 nm) and flow cytometry (Figures 1A, B). As reported, N deprivation drastically reduced O. tauri growth compared to culture with MK. The decrease in OD 660 nm correlated with the cell number quantified (Figure 1B). Interestingly, O. tauri can grow in the presence of the amino acid L-Arg (0.1 mM) up to the first 10 d. This growth was inhibited by the NOS inhibitor L-NAME (Figures 1A, B). In the presence of another basic amino acid, L-Lys, O. tauri growth was not restored, suggesting that this basic amino acid is not used as a N source in O. tauri. The addition of increasing doses of L-Arg (0.1, 0.5, 1 mM) maintains sustained O. tauri growth (Supplemental Figure S1).
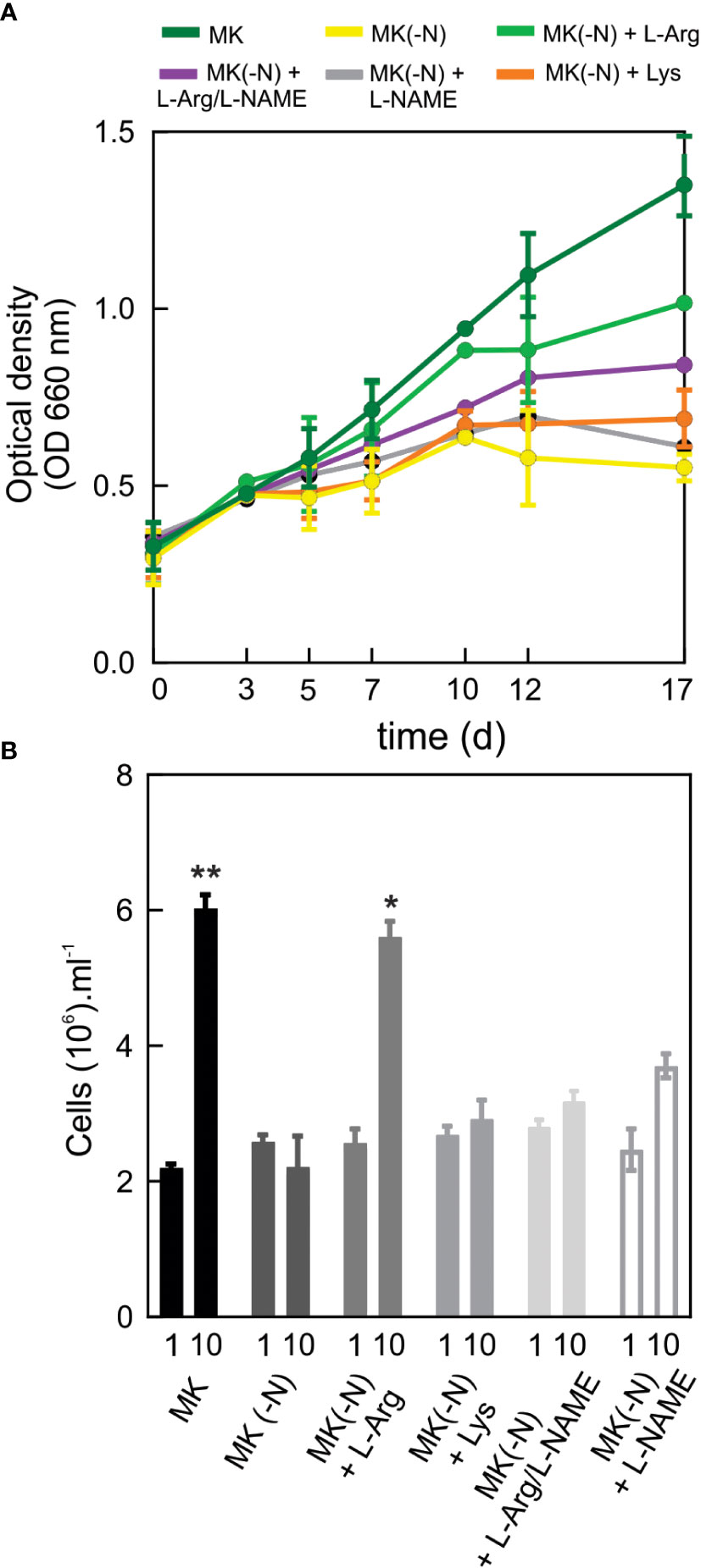
Figure 1 O. tauri can grow in a culture medium containing L-Arg as the sole source of N. O. tauri OTTH0595 (RCC745) culture was obtained from Roscoff Culture Collection. (A) For cell growth curves, 15 ml of cell culture grown at a 12:12 h light: dark regime in 60 µE white light at 20 ± 1°C were centrifuged at room temperature at 3,500 rpm for 20 min. Cell pellets were suspended N-completed K medium (MK), MK without N source [MK(-N)] and MK(-N) supplemented with 0.1 mM L-Arg (MK(-N)+L-Arg) or 0.1 mM L-Lys (MK(-N)+L-Lys) or 0.1 mM of the NOS inhibitor L-NAME (MK(-N)+L-NAME) or 0.1 mM L-Arg plus 0.1 mM L-NAME ((MK(-N)+L-Arg/L-NAME). Optical densities at 660 were taken every 2-3 d over 17 d of cell culture. (B) Cell number was quantified by flow cytometry at day 0 and day 10 of the growth culture. Values are means ± SD (n = 2, for each parameter). Two independent experiments (n =2 and n=3) were performed with similar results. The asterisks indicate statistical difference compared to MK (-N), (ANOVA, post-hoc Dunnett method, **p < 0.01, *p <0.05).
N deficiency is accompanied by chlorophyll loss and a drastic reduction of photosynthetic activity (Saux et al., 1987; Plumley and Schmdt, 1989). Thus, we tested the effect of L-Arg as the sole N source on chlorophyll content in O. tauri cultures. Figure 2A shows that N deprivation significantly reduced total chlorophyll content (Figure 2A) and the mean chlorophyll fluorescence in the cell distribution (Figures 2B, C). Cultures supplemented with L-Arg showed a 20% increase in mean fluorescence intensity of chlorophyll compared to MK (-N) cultures (Figure 2C). Thus, the N incorporation by L-Arg degradation in O. tauri can reverse the N deficiency effect on chlorophyll content. L-NAME is an L-Arg analogue, which inhibits the synthesis of NO by competitive antagonism towards NOS (Scheller et al., 1998). At identical concentration of L-Arg and inhibitor, a 50% reduction of NOS activity is expected. Figures 2A–C shows a decrease of about 50% in growth and chlorophyll fluorescence intensity in the presence of the L-NAME. In concordance with the OD660 results, the use of L-Lys as an N source was not able to restore the chlorophyll content (Figures 2A–C). Furthermore, the addition of 0.1 mM L-Arg in complete MK resulted in an improvement of O. tauri OD and increase of 16% mean chlorophyll content per cell with respect to the MK condition at 10 days (Supplemental Figure S1). These results suggest that chlorophyll reduction is a direct response to N starvation signaling in O. tauri, a physiological process that is partially restored by L-Arg supplementation.
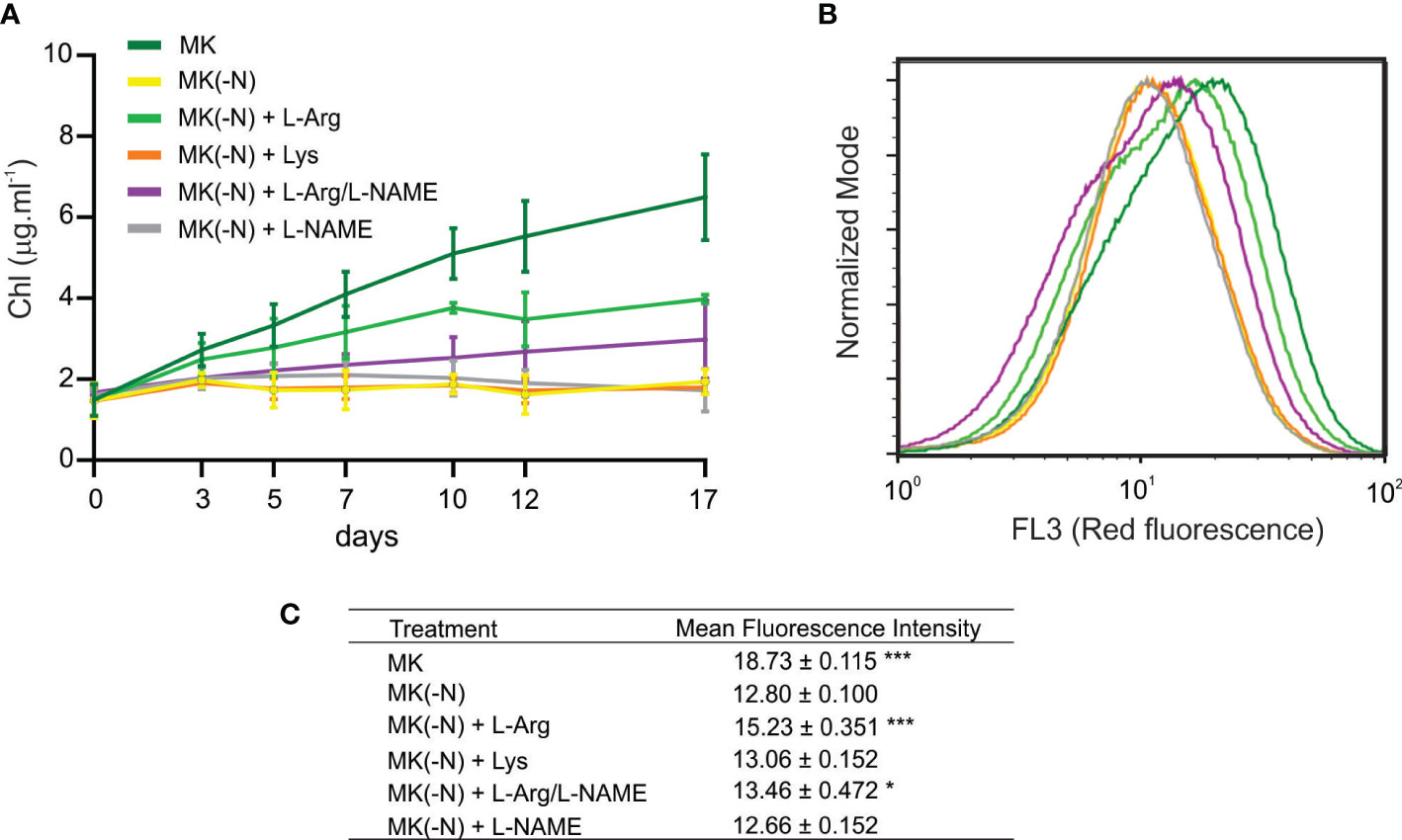
Figure 2 Chlorophyll content in O. tauri growing under different N conditions. O. tauri cell culture (OD 660 ~ 0.3) was grown in the same culture media described for Figure 1. (A) At the indicated times, cells were centrifuged at 8,000 rpm for 10 min and chlorophyll was extracted with 100% ethanol for 15 min in dark. Chlorophyll content was determined by measuring the ethanolic fraction at 665 nm. Values are mean ± SE (n ≥ 5). (B) Histogram shows chlorophyll autofluorescence intensity of O. tauri cells at 10 days of culture measured by flow cytometry with FL3 detector. (C) Mean chlorophyll fluorescence of the cell distribution after 10 days of culture. Values are mean and S.D (n = 3). The asterisks indicate statistical difference compared to MK (-N), (ANOVA, post-hoc Dunnett method, ***p < 0.001, *p <0.05).
Increase of lipid content and transcript level of N-associated genes in L-Arg-fed O. tauri culture
When N deprivation is imposed upon a culture exposed to suitable irradiances, photosynthesis occurs at a reduced rate, and the flow of fixed C is diverted from proteins to either lipids or carbohydrate synthesis (Hu et al., 2008; Rodolfi et al., 2009). In accordance, Figure 3 shows that total lipid content in O. tauri cells subjected to N starvation increased as was previously described (Caló et al., 2022). Interestingly, L-Arg-fed cells also accumulated lipids similar to N-deprivation (Figure 3), even when the growth rate was not equally affected in these conditions. Cells cultured in L-Arg produced higher amounts of total lipids in a short period of treatment compared to control and N-deprived condition at 24 h (10%) and also, they had higher lipid content than control at 10 days (10%) with similar OD values as in the complete medium.
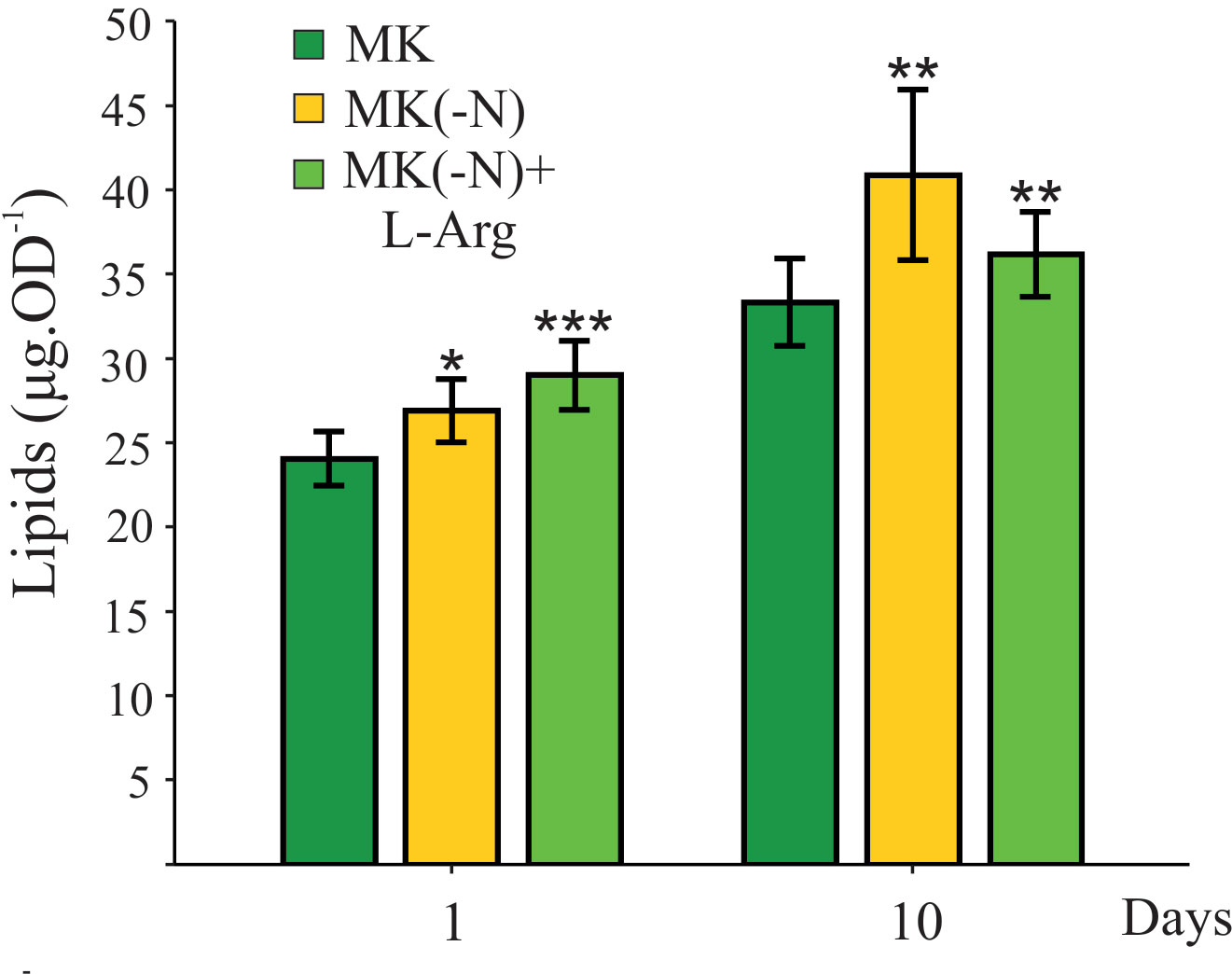
Figure 3 Total lipid content increases in O. tauri cultures grown in the absence of N and the presence of L-Arg as the sole N source. O. tauri cultures grown under phototrophic conditions in MK (OD 660 ~ 0.3) were harvested and incubated in MK (green), MK(-N) (yellow), and MK(-N) + L-Arg (light green) after 1 and 10 days. Total lipid levels were determined by the sulfo-phospho-vanillin method. Values are mean ± SE (n ≥ 5). The asterisk indicates statistical difference compared to MK (ANOVA, post-hoc Dunnett method, ***p < 0.001, **p < 0.01, *p<0.05).
We further investigated whether L-Arg supplemented cultures activate unique molecular responses of N-starved cultures. It was reported that when N concentration in the culture medium is scarce, cells activate the expression of genes involved in N uptake and assimilation (Schmollinger et al., 2014; Munz et al., 2020). We evaluated the mRNA levels of the NO3- transporter (NTR), NO3- reductase (NR) and nitrite reductase (NIR), three N-starvation marker genes involved in NO3- uptake and assimilation. Figure 4 A shows that the three-marker genes were up-regulated in N-deprived cells. In the N-deprived culture supplemented with L-Arg, marker genes were partially induced compared to total N deprivation (Figure 4A). This finding suggests that although L-Arg is metabolized and can partially bypass the N-deficiency status it is still up-regulated N deficiency responses such as genes involved in N uptake and assimilation.
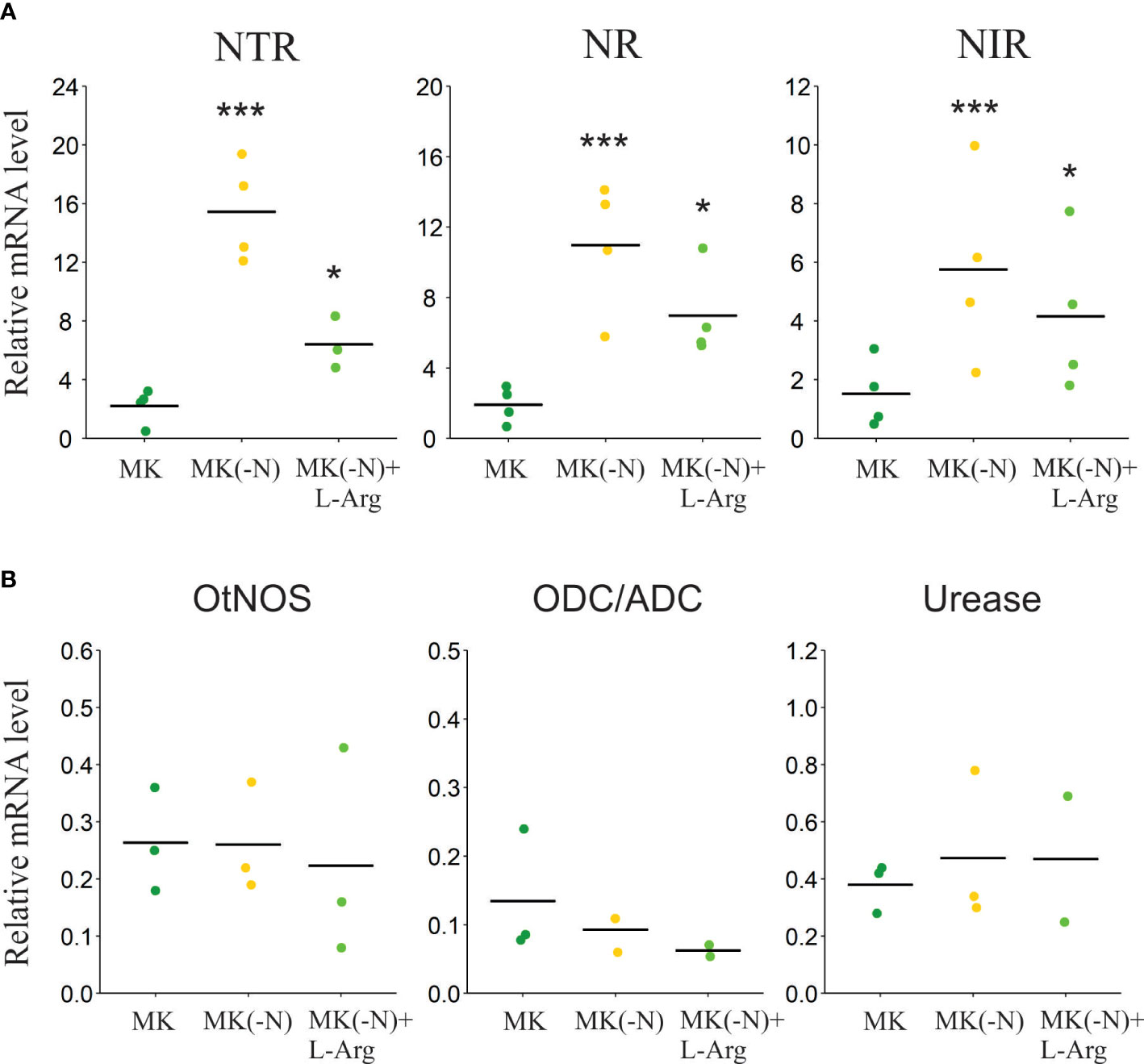
Figure 4 Transcript level of genes associated with N assimilation in O. tauri grown in L-Arg as the sole N source. Cultures grown under phototrophic conditions in MK (OD 660 ~ 0.3) were harvested and incubated in MK (green), MK (-N) (yellow) and MK (-N) + L-Arg (light green) for 24 h. (A) The transcript levels of genes involved in N scavenging (NO3- transport, NTR) and NO3- primary assimilation (NO3- reductase; NR) and (NO2- reductase; NIR) were analyzed by RT-qPCR. Values were normalized to GAPDH mRNA levels. Bars denote means, n ≥ 3. Asterisks indicate statistical differences (ANOVA, post-hoc Dunnett method, *p < 0.05, ***p < 0.001). (B) The transcript levels of the genes coding for nitric oxide synthase (NOS), ornithine/arginine decarboxylase (ODC/ADC), and urease were analyzed by RT-qPCR. Values were normalized to GAPDH mRNA levels. Bars denote means, n ≤ 3. No statistical differences were observed among treatments (ANOVA, p >0.05).
Therefore, we investigated plausible routes of L-Arg catabolism according to those present in the O. tauri genome. No orthologues of arginase, ADI and LAO can be identified in the O. tauri genome, suggesting that three of the four described ways to assimilate N from L-Arg are not available. This leaves a few alternatives for L-Arg catabolism in the O. tauri genome: one begins with a homologous to ornithine/arginine decarboxylase (ODC/ADC) protein and the second with L-Arg-dependent NOS. We evaluated the transcript levels of ODC/ADC and NOS, after 24 h in the cultures without N and with L-Arg as the only source of N. Figure 4B shows that there are no significant differences in the expression of these genes in the tested conditions. The expression of the alpha subunit of urease, a protein involved in the catalysis of urea, was also analyzed. Urease transcript levels also did not increase significantly in -N cultures and with L-Arg as the only N source (Figure 4B).
NO production in O. tauri cell cultures
Numerous investigations suggested that algae from diverse lineages, such as chlorophytes, charophytes, red algae, or diatoms produce NO (Mallick et al., 2002; Sakihama et al., 2002; Tischner et al., 2004; Vardi et al., 2006; Vardi et al., 2008; Foresi et al., 2010). To analyze whether O. tauri produces NO in the different N culture conditions, cell suspensions were incubated with the NO-specific fluorophore (DAF-FM DA), after 24 h in the cultures without N, and with L-Arg as the only source of N. Figure 5 shows that, the addition 0.1 mM L-Arg increased NO production which was blocked by the treatment with the NOS inhibitor L-NAME. We also quantified NO production at different times of culture growth in MK(-N) and MK(-N) supplemented with 0.1 mM L-Arg. NO production was higher in cultures supplemented with L-Arg after growing for 1, 2 and 4 days and a pronounced decrease was detected after 7 days (Supplemental Figure S2). These results suggest that NOS activity is induced in L-Arg-fed cultures. In contrast, the highest concentration of L-Arg tested, 5 mM, increases more than 10-fold the NO content and inhibits the growth of O. tauri, reaching a similar OD660 to N deprivation (Supplemental Figure S3). NO is a free radical, reactive nitrogen species (RNS), that functions as a diffusible gasotransmitter messenger in a dose-dependent manner. It was demonstrated that at high level, NO is toxic and induces cell death (Beligni and Lamattina, 1999; Astuti et al., 2016; Bruand and Meilhoc, 2019). At the same time, here we show that NO at optimum concentration would be re-incorporated and assimilated as a N source in O. tauri.
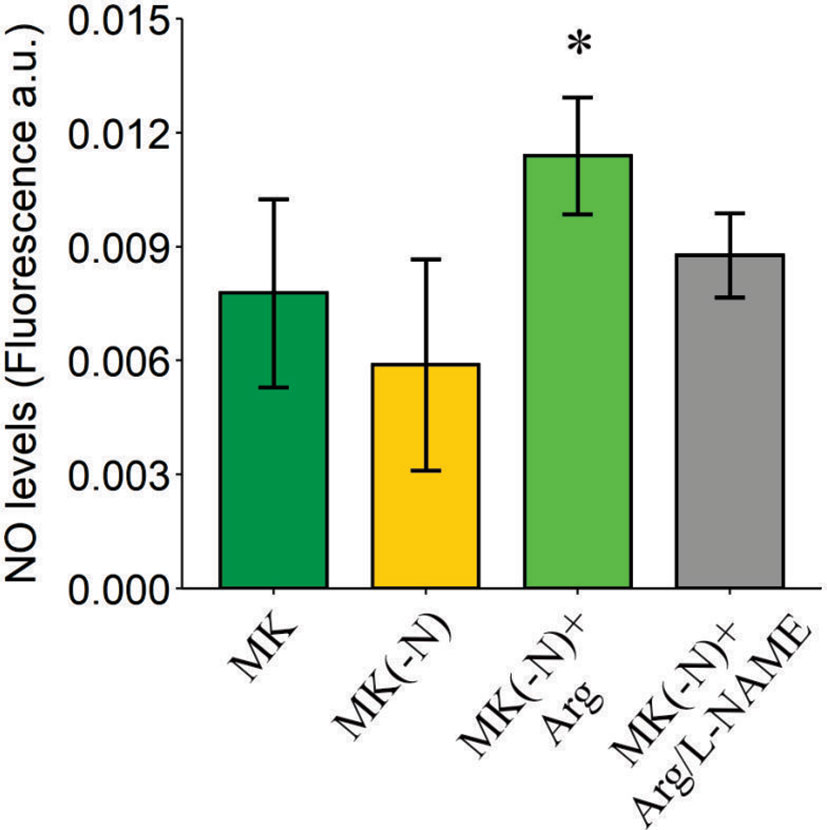
Figure 5 NO generation increases in O. tauri growing in culture medium containing L-Arg as the sole N source. Cultures grown under a phototrophic condition in MK OD 660 ~ 0.3 were harvested and incubated in MK (green), MK(-N) (yellow), MK(-N) + L-Arg (light green), and MK(-N) + L-Arg/L-NAME (gray) for 24 h. NO was determined using the NO sensitive probe DAF-FM DA. NO fluorescence intensity (excitation 495 nm; emission 525 nm) was measured using a fluorescence plate reader (Fluoroskan Ascent; Thermo Electron) over an hour. Data are expressed as a fold increase of arbitrary units (a.u.) per min. Values are mean ± SE (n = 3). The asterisk indicates statistical difference compared to MK (ANOVA, post-hoc Dunnett method, *p < 0.05).
NO3- and NO2- can be produced non-enzymatically from NO oxidation, which then could be assimilated by NR and NIR activity respectively and re-incorporated into the N metabolism. To corroborate this hypothesis, we analyzed whether O. tauri can grow in the presence of NO2- as the only N source. Figure 6A shows that a NO2- dose-dependent growth of O. tauri cell cultures while a decrease of NO2- concentration of the cell-free culture media is observed (Supplemental Figure S4). NO2- dependent growth in O tauri is accompanied with lipid accumulation similar to L-Arg-fed cultures (Figure 6B). In addition, we evaluated the effect of the molybdenum cofactor inhibitor (tungstate, unspecific NR inhibitor) in cultures grown in L-Arg as the only N source. Supplemental Figure S5 shows that the treatment with tungstate could not block the growth in MK(-N) L-Arg medium. These findings allow us to infer that NO2- would be the main product of NO oxidation that is assimilated by the alga and incorporated as a source of N in O. tauri.
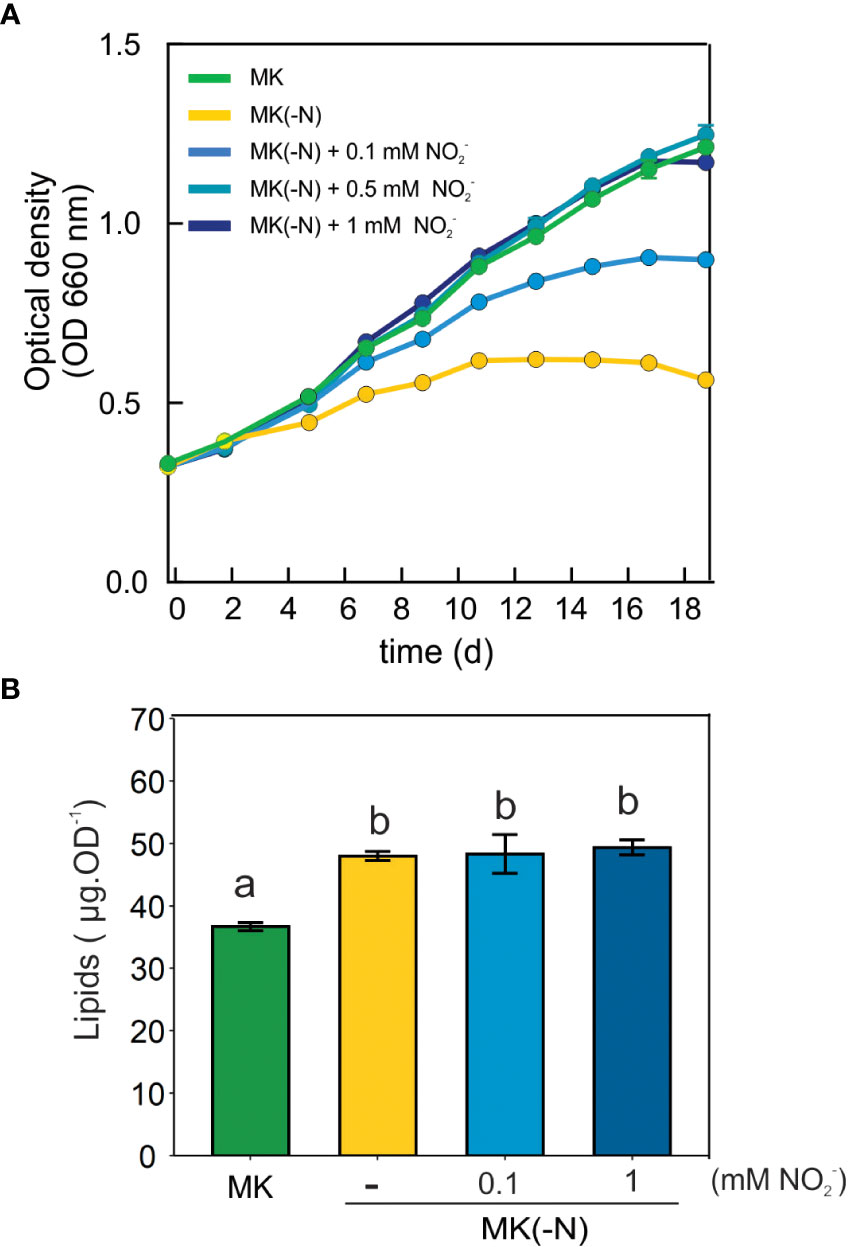
Figure 6 O. tauri can grow and accumulates lipids in a culture medium containing NO2- as the sole N source. (A) For cell growth curves, 15 ml of cell culture grown at a 12:12 h light: dark regime in 60 µE white light at 20 ± 1°C were centrifuged at room temperature at 3,500 rpm for 20 min. Cell pellets were suspended N-completed K medium (MK), MK without N source [MK(-N)] and MK(-N) supplemented with 0.1 mM, 0.5 or 1 mM NO2-. Optical densities at 660 were taken every 2-3 d over 17 d of cell culture. (B) Total lipid levels were determined in MK, MK(-N), MK(-N) + 0.1 or 1 mM NO2 after 10 days by the sulfo-phospho-vanillin method. Different letters indicate significant differences (ANOVA, posthoc Tuckey method, p < 0.05).
Discussion
In this report, we showed that cultures of O. tauri supplemented with L-Arg display a series of N deficiency responses, including the induction of genes involved in N metabolism and uptake, reduced cellular chlorophyll content and accumulation of lipid storage despite robust growth. Thus, L-Arg-supplemented cultures may trigger the same signaling that induces N starvation. The N starvation responses in L-Arg fed cultures were also observed in C. reinhardtii and P. tricornutum, suggesting a common effect of L-Arg supplementation in alga (Flynn and Wright, 1986; Munz et al., 2020). Triggering N limitation responses requires signaling mechanisms that perceive external and internal N availability. In the model alga C. reinhardtii, transcriptional/proteomic changes upon N starvation have been intensely studied to know the routes of TAG accumulation in the microalgae (Wase et al., 2014). N limitation rapidly induces genes including the classical N catabolite genes for N scavenging and N salvaging, indicating the activation of N remobilization from purines and amino acids while searching for other external N sources (Schmollinger et al., 2014; Park et al., 2015). Additionally, low N resources down-regulate the expression of genes associated with the antenna system, decreasing light absorption and electron transport (Huang et al., 2019).
In this study, we describe that O. tauri can grow at the same time that produces lipids in an N-deficient medium supplemented with L-Arg. Previously, Degraeve-Guilbault et al. (2017); Degraeve-Guilbault et al. (2021) provided a comprehensive study of the glycerolipidome and validated this species as a model for related picoeukaryotes. O. tauri lipids showed unique characteristics that combined traits from green and chromalveolate lineages (Degraeve-Guilbault et al., 2017; Degraeve-Guilbault et al., 2021). Ostreococcus presents a single APC-type transporter (Amino acid/polyamine transporter, NCBI Sequence: XP_003083160.2) orthologous to AOC5/6 (plant cationic amino acid transporters). These transporters facilitate the bidirectional transport of cationic amino acids, thereby supporting critical metabolic functions, such as the synthesis of proteins, NO, and polyamines (Hatzoglou et al., 2004). The fact that O. tauri has an APC-type transporter indicates that this alga will show preferential use of L-Arg over other amino acids (Vallon and Spalding, 2009).
There are several pathways for L-Arg degradation that produce reusable N in photosynthetic organisms (Figure 7). Higher plants use L-Arg as an N source whose utilization is primarily dependent on arginase activity followed by urease that catalyzes the formation of NH4+ and CO2 (Winter et al., 2015). These activities primarily occur during the germination process where a rapid N remobilization from N sources is required for plant growth (Goldraij and Polacco, 2000; Todd et al., 2001; Winter et al., 2015). In addition to germination, arginase plays a key role in the recycling of N during plant senescence and grain production in rice (Ma et al., 2013). However, the arginase enzyme is absent in the O. tauri genome. The evidence supporting the ADI pathway is also weak, as no gene/coding sequence was found. The ADI pathway is present in fungi, algal, and cyanobacterial genomes (Novák et al., 2016; Noens and Lolkema, 2017; Flores et al., 2019; Flores, 2020). For example, Chlorella and Chlamydomonas can utilize L-Arg for growth via the ADI pathway (Laliberté and Hellebust, 1990; Zuo et al., 2012). In addition, Chlamydomonas can also deaminate various amino acids including L-Arg in a N-depleted medium by the action of LAO activity (Calatrava et al., 2019). LAO1 is found in the periplasmic space and was proposed to contribute to N assimilation in oceanic environments. Chlamydomonas genome encodes for a putative intracellular LAO3 (Calatrava et al., 2019). The reaction of LAO enzymes produces ammonium, hydrogen peroxide and the corresponding keto acid; then NH4+ can be incorporated and assimilated by algae (Figure 7). In contrast, a homologous sequence coding for this protein is absent in the Ostreococcus genome.
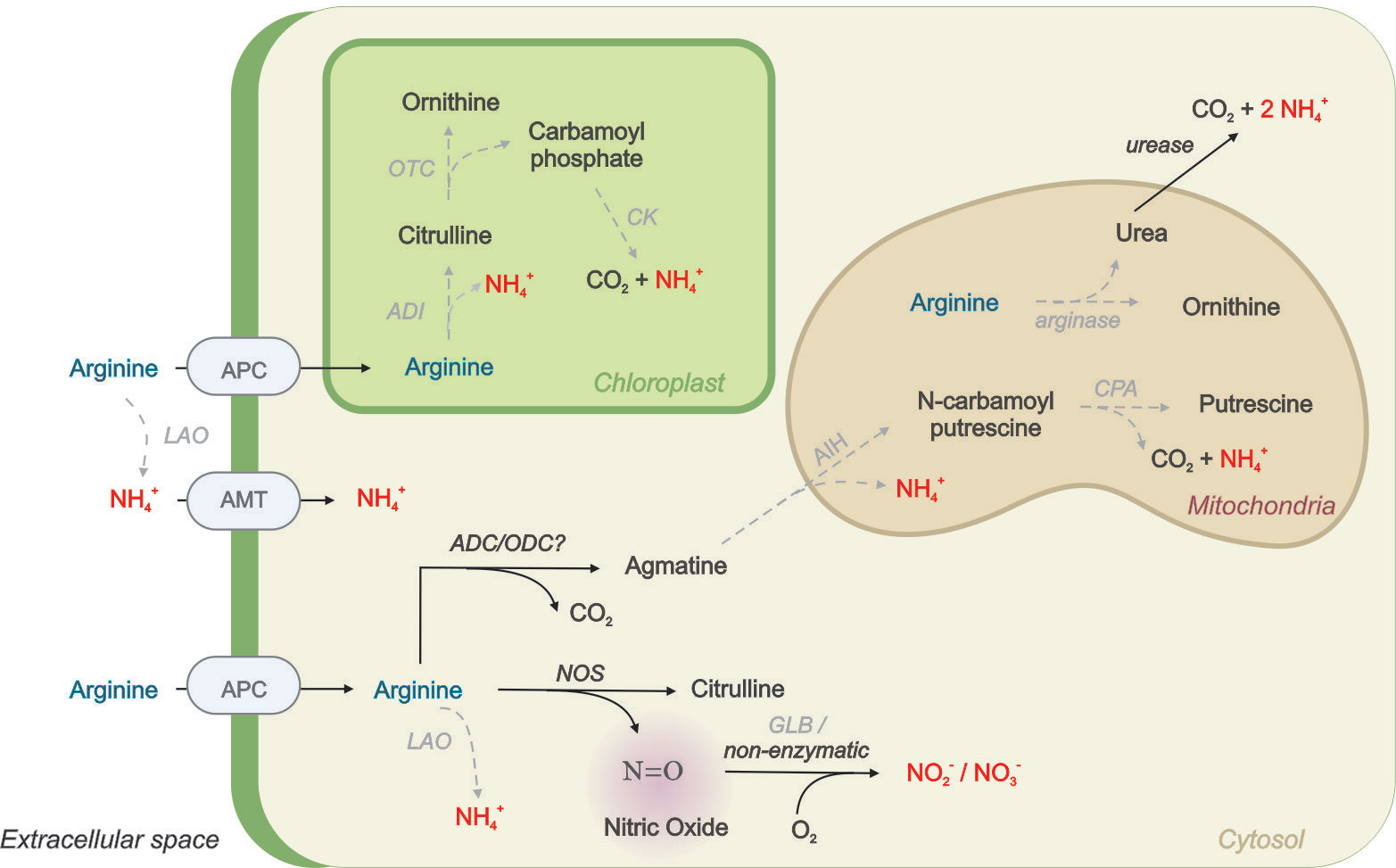
Figure 7 Simplified scheme proposing L-Arg metabolism in photosynthetic cells containing NOS enzyme. Arginine uptake from the medium is carried out by cationic amino acid transporters (APC) or degraded extracellularly by L-amino oxidases (LAO) enzyme to produce NH4+ that then is incorporated by ammonium transporters (AMT). There are also intracellular LAO enzymes described in algae. Intracellular Arginine can be metabolized in the cytoplasm by arginine decarboxylase (ADC) with the formation of agmatine, which then is metabolized in the mitochondria to putrescine and NH4+by the action of agmatine iminohydrolase (AIH) and N-carbamoylputrescine amidohydrolase (CPA). Arginine can also be metabolized by nitric oxide synthases (NOS) to produce citrulline and NO. NO can be rapidly oxidized by cellular globins (GLBs) or non-enzymatically in the presence of O2 to produce N oxides (NOx). Arginine can also be metabolized in chloroplasts by the arginine deiminase (ADI) pathways including the enzymes ornithine carbamoyltransferase (OTC) and carbamate kinase leading to NH4+, CO2 and ATP. Finally, Arginine may also be degraded by arginase to produce urea. Urea can be exported to cytosol and metabolized by ureases to produce NH4 and CO2. Gray dash arrows represent the enzymes that were not found in the O. tauri genome.
The O. tauri genome presents a coding sequence with partial homology (38% similarity) with ADC from Arabidopsis thaliana, with the two classical domains required for activity (PFAM PS00878 and PS00879). ADC cleaves the carboxyl group of the amino acid group releasing agmatine and CO2. ADC homologous have been reported in plants and several Chlorella species (Cohen et al., 1983; Beigbeder et al., 1995; Lin and Lin, 2019). Then, agmatine is converted to N-carbamoylputrescine and NH4+ by agmatine iminohydrolase (AIH) and then to putrescine by N-carbamoylputrescine amidohydrolase (CPA, Figure 7). The agmatine catabolic enzymes, encoded by two AIH1 and AIH2 in Chlamydomonas, are predicted to localize to the mitochondria meaning the ADC pathway would likely be directed to the mitochondria. Both AIH and CPA genes are conserved in Volvox, but not in Ostreococcus (Vallon and Spalding, 2009). Thus, this evidence suggests that there should be another pathway apart from the ones described to get assimilable N from L-Arg in O. tauri.
Our results evince that O. tauri grown in L-Arg produces NO by NOS, which was previously described and characterized in O. tauri (Foresi et al., 2010). NO has a half-life of only a few seconds since it rapidly interacts with O2, producing nitrogen oxides (NOx), such as NO3- and NO2- (Lancaster, 2015). This NO oxidation may be also catalyzed by globins (GLBs), a ubiquitous class of proteins present in all kingdoms (Vinogradov et al., 2006; Figure 7). Curiously, we could not find a sequence coding for GLBs in the O. tauri genome. In this sense, our results suggest that NO2- produced by spontaneous NO oxidation could be assimilated by NIR and incorporated into the N metabolism of the alga. Assimilation of NO2- derived from NO in flue gas influences the growth of the microalgae Nannochloropsis salina and Phaeodactylun tricornutum (Brown, 1996; Matsumoto et al., 1997). Moreover, Botryococcus braunii can grow in NO2- as the sole N source for in a range about 2-4 mM while higher concentrations inhibit algae growth (Yang et al., 2004).
So far, this pathway would be a possible way to re-assimilate N from L-Arg in O. tauri. On the other hand, NOS activity produce citrulline, which may be further processed into ornithine and carbamoyl phosphate by the enzyme ornithine carbamoyltransferase (OTC) and then to CO2 and NH4+ by carbamate kinase (CK) again absent in the O. tauri genome (Vallon and Spalding, 2009). The role of NOS in N assimilation from L-Arg is supported by the use of the L-NAME, which prevents growth and NO production in O. tauri grown in L-Arg. Although transgenic lines of O. tauri have been generated using pOtOXLuc transgenics (Degraeve-Guilbault et al., 2020), no commercial mutant lines of O. tauri are currently available. Therefore, employing a pharmacological approach using specific inhibitors is now extremely useful.
Besides N source, the latest data have described NO as a signal molecule in algae (Foresi et al., 2010; Foresi et al., 2017; Astier et al., 2021; Chatelain et al., 2021). NO acts as a signal in the transcriptional and posttranslational regulation of NR and inorganic N transport in Chlamydomonas (Sanz-Luque et al., 2015). NO participates in stress responses, in cell physiology, and is involved in greenhouse gas emission due to the reduction of NO into N2O in algae (Romano et al., 2011; Kumar et al., 2015; Burlacot et al., 2020). Burlacot et al. (2020) reported that C. reinhardtii produces N2O in the light by NO reduction catalyzed by flavodiiron proteins (FLVs), whereas in unlighted N2O production is catalyzed by cytochrome P450 (CYP55). Through a sequence homology search we identify a flavodiiron protein (XP_003075215.2, 60-52% similarity to Chlamydomonas FLVs) and several cytochrome P450 (40-50% similarity to CYP55) in O. tauri. This is extremely interesting for the study of the relationship between NOS function and N2O production in O. tauri.
This report provides evidence that that directly relates NOS activity with a function in the N metabolism and growth in algae. In a previous work, we analyzed the effect of recombinant OtNOS expressed under IPTG-induced promoter in E. coli, which lacks the gene NOS. The OtNOS expression promotes E. coli growth in a complete nutrient medium and provides a more efficient metabolization of L-Arg as an N source (Correa-Aragunde et al., 2022). Furthermore, the expression of OtNOS under the control of the Cauliflower Mosaic Virus (CaMV) in Nicotiana tabacum enhances growth and seed production, suggesting that OtNOS expression in plants has a straight impact on N metabolism (Nejamkin et al., 2020).
This alga has commercial potential as photoautotrophic cell factories capable of producing high-value biofuels and specialty oils (Degraeve-Guilbault et al., 2017). Advances in bioprocess technology, coupled with strain improvement through traditional methods of mutagenesis or genetic engineering, now offer the potential to improve the economics of oil production. Recently a promising transformation protocol for O. tauri has been reported (Sanchez et al., 2019). The characterization of NOS as an enzyme that metabolizes L-Arg and allows the growth of O. tauri demonstrates a novel and potential biotechnological role of NOS in N metabolism in photosynthetic organisms.
Data availability statement
The raw data supporting the conclusions of this article will be made available by the authors, without undue reservation.
Author contributions
NF, GM-N, LL, GS and NC-A designed research. GC, FC, AN, NC-A and NF perform the experiments. GM-N, NF and AN analyzed data. NF, NC-A, wrote the paper with the input from the other authors. All authors contributed to the article and approved the submitted version.
Funding
This research was supported by Agencia Nacional de Promoción Científica y Tecnológica (PICT 2716/18).
Conflict of interest
The authors declare that the research was conducted in the absence of any commercial or financial relationships that could be construed as a potential conflict of interest.
Publisher’s note
All claims expressed in this article are solely those of the authors and do not necessarily represent those of their affiliated organizations, or those of the publisher, the editors and the reviewers. Any product that may be evaluated in this article, or claim that may be made by its manufacturer, is not guaranteed or endorsed by the publisher.
Supplementary material
The Supplementary Material for this article can be found online at: https://www.frontiersin.org/articles/10.3389/fmars.2022.1064077/full#supplementary-material
References
Andersson M. G., van Rijswijk P., Middelburg J. J. (2006). Uptake of dissolved inorganic nitrogen, urea and amino acids in the Scheldt estuary: Comparison of organic carbon and nitrogen uptake. Aquat. Microbial Ecol. 44 (3), 303–315. doi: 10.3354/ame044303
Astier J., Rossi J., Chatelain P., Klinguer A., Besson-Bard A., Rosnoblet C., et al. (2021). Nitric oxide production and signalling in algae. J. Exp. Bot. 72 (3), 781–792. doi: 10.1093/jxb/eraa421
Astuti R. I., Watanabe D., Takagi H. (2016). Nitric oxide signaling and its role in oxidative stress response in Schizosaccharomyces pombe. Nitric. Oxide 52, 29–40. doi: 10.1016/j.niox.2015.11.001
Beigbeder A., Vavadakis M., Navakoudis E., Kotzabasis K. (1995). Influence of polyamine inhibitors on light-independent and light-dependent chlorophyll biosynthesis and on the photosynthetic rate. J. Photochem. Photobiol. B: Biol. 28 (3), 235–242. doi: 10.1016/1011-1344(95)07113-G
Beligni M. V., Lamattina L. (1999). Is nitric oxide toxic or protective? Trends Plant Sci. 4 (8), 299–300. doi: 10.1016/s1360-1385(99)01451-x
Brown L. M. (1996). Uptake of carbon dioxide from flue gas by microalgae. Energy Conversion Manage. 37 (6-8), 1363–1367. doi: 10.1016/0196-8904(95)00347-9
Bruand C., Meilhoc E. (2019). Nitric oxide in plants: pro-or anti-senescence. J. Exp. Bot. 70 (17), 4419–4427. doi: 10.1093/jxb/erz117
Burlacot A., Richaud P., Gosset A., Li-Beisson Y., Peltier G. (2020). Algal photosynthesis converts nitric oxide into nitrous oxide. Proc. Natl. Acad. Sci. 117 (5), 2704–2709. doi: 10.1073/pnas.191527611
Calatrava V., Hom E. F., Llamas A., Fernandez E., Galvan A. (2019). Nitrogen scavenging from amino acids and peptides in the model alga Chlamydomonas reinhardtii. role extracellular l-amino oxidase. Algal Res. 38, 101395. doi: 10.1016/j.algal.2018.101395
Caló G., De Marco M. A., Salerno G. L., Martínez-Noël G. M. A. (2022). TOR signaling in the green picoalga Ostreococcus tauri. Plant Sci. 323, 111390. doi: 10.1016/j.plantsci.2022.111390
Caló G., Scheidegger D., Martínez-Noël G. M., Salerno G. L. (2017). Ancient signal for nitrogen status sensing in the green lineage: Functional evidence of CDPK repertoire in Ostreococcus tauri. Plant Physiol. Biochem. 118, 377–384. doi: 10.1016/j.plaphy.2017.07.009
Cardol P., Bailleul B., Rappaport F., Derelle E., Béal D., Breyton C., et al. (2008). An original adaptation of photosynthesis in the marine green alga Ostreococcus. Proc. Natl. Acad. Sci. 105 (22), 7881–7886. doi: 10.1073/pnas.0802762105
Chatelain P., Astier J., Wendehenne D., Rosnoblet C., Jeandroz S. (2021). Identification of partner proteins of the algae Klebsormidium nitens NO synthases: Towards a better understanding of NO signaling in eukaryotic photosynthetic organisms. Front. Plant Sci., 3068. doi: 10.3389/fpls.2021.797451
Cohen E., Arad S., Heimer Y. M., Mizrahi Y. (1983). Polyamine biosynthetic enzymes in Chlorella: Characterization of ornithine and arginine decarboxylase. Plant Cell Physiol. 24 (6), 1003–1010. doi: 10.1093/oxfordjournals.pcp.a076601
Correa-Aragunde N., Nejamkin A., Del Castello F., Foresi N., Lamattina L. (2022). Nitric oxide synthases from photosynthetic organisms improve growth and confer nitrosative stress tolerance in E. coli. Insights on the pterin cofactor. Nitric. Oxide 119, 41–49. doi: 10.1016/j.niox.2021.12.005
Degraeve-Guilbault C., Bréhélin C., Haslam R., Sayanova O., Marie-Luce G., et al. (2017). Glycerolipid characterization and nutrient deprivation-associated changes in the green picoalga Ostreococcus tauri. Plant Physiol. 173 (4), 2060–2080. doi: 10.1104/pp.16.01467
Degraeve-Guilbault C., Gomez R. E., Lemoigne C., Pankansem N., Morin S., Tuphile K., et al. (2020). Plastidic Δ6 Fatty-Acid Desaturases with Distinctive Substrate Specificity Regulate the Pool of C18-PUFAs in the Ancestral Picoalga Ostreococcus tauri. Plant Physiol. 184 (1), 82–96. doi: 10.1104/pp.20.00281
Degraeve-Guilbault C., Pankasem N., Gueirrero M., Lemoigne C., Domergue F., Kotajima T., et al. (2021). Temperature acclimation of the picoalga Ostreococcus tauri triggers early fatty-acid variations and involves a plastidial ω3-desaturase. Front. Plant Sci. 12. doi: 10.3389/fpls.2021.639330
Demir-Hilton E., Sudek S., Cuvelier M. L., Gentemann C. L., Zehr J. P., Worden ,. A. Z. (2011). ). Global distribution patterns of distinct clades of the photosynthetic picoeukaryote Ostreococcus. ISME J. 5 (7), 1095–1107. doi: 10.1038/ismej.2010.209
Falkowski P. G. (1994). The role of phytoplankton photosynthesis in global biogeochemical cycles. Photosynthesis Res. 39 (3), 235–258. doi: 10.1007/BF00014586
Falkowski P. G., Fenchel T., Delong E. F. (2008). The microbial engines that drive Earth's biogeochemical cycles. Science 320 (5879), 1034–1039. doi: 10.1126/science.1153213
Field C. B., Behrenfeld M. J., Randerson J. T., Falkowski P. (19985374). Primary production of the biosphere: integrating terrestrial and oceanic components. Science 281, 237–240. doi: 10.1126/science.281.5374.237
Flores E. (2020). Arginine catabolism enzyme AgrE/ArgZ likely involves a cyanobacterial specific factor. J. Biol. Chem. 295 (10), 2915. doi: 10.1074/jbc.L120.012850
Flores E., Arévalo S., Burnat M. (2019). Cyanophycin and arginine metabolism in cyanobacteria. Algal Res. 42, 101577. doi: 10.1016/j.algal.2019.101577
Flynn K. J. (1990). Composition of intracellular and extracellular pools of amino acids, and amino acid utilization of microalgae of different sizes. J. Exp. Mar. Biol. Ecol. 139 (3), 151–166. doi: 10.1016/0022-0981(90)90143-Z
Flynn K. J., Wright C. R. N. (1986). The simultaneous assimilation of ammonium and L-arginine by the marine diatom Phaeodactylum tricornutum Bohlin. J. Exp. Mar. Biol. Ecol. 95 (3), 257–269. doi: 10.1016/0022-0981(86)90258-3
Foresi N., Correa-Aragunde N., Lamattina L. (2017). “Synthesis, actions, and perspectives of nitric oxide in photosynthetic organisms,” in Nitric Oxide (Academic Press: New York, USA.), 125–136. doi: 10.1016/B978-0-12-804273-1.00010-7
Foresi N., Correa-Aragunde N., Parisi G., Calo G., Salerno G., Lamattina L. (2010). Characterization of a nitric oxide synthase from the plant kingdom: NO generation from the green alga Ostreococcus tauri is light irradiance and growth phase dependent. Plant Cell 22 (11), 3816–3830. doi: 10.1105/tpc.109.073510
Foyer C. H., Noctor G., Verrier P. (2018). Photosynthetic carbon–nitrogen interactions: modelling inter-pathway control and signalling. Annu. Plant Rev. Online, 325–347. doi: 10.1002/9781119312994.apr0227
Goldraij A., Polacco J. C. (2000). Arginine degradation by arginase in mitochondria of soybean seedling cotyledons. Planta 210 (4), 652–658. doi: 10.1007/s004250050056
Gruber N., Galloway J. N. (2008). An Earth-system perspective of the global nitrogen cycle. Nature 451 (7176), 293–296. doi: 10.1038/nature06592
Hatzoglou M., Fernandez J., Yaman I., Closs E. (2004). Regulation of cationic amino acid transport: the story of the CAT-1 transporter. Annu. Rev. Nutr. 24, 377–399. doi: 10.1146/annurev.nutr.23.011702.073120
Huang L., Gao B., Wu M., Wang F., Zhang C. (2019). Comparative transcriptome analysis of a long-time span two-step culture process reveals a potential mechanism for astaxanthin and biomass hyper-accumulation in Haematococcus pluvialis JNU35. Biotechnol. Biofuels 12 (1), 1–20. doi: 10.1186/s13068-019-1355-5
Hu Q., Sommerfeld M., Jarvis E., Ghirardi M., Posewitz M., Seibert M., et al. (2008). Microalgal triacylglycerols as feedstocks for biofuel production: perspectives and advances. Plant J. 54 (4), 621–639. doi: 10.1111/j.1365-313X.2008.03492.x
Illman A. M., Scragg A. H., Shales ,. S. W. (2000). Increase in Chlorella strains calorific values when grown in low nitrogen medium. Enzyme microbial Technol. 27 (8), 631–635. doi: 10.1016/S0141-0229(00)00266-0
Johnson X., Alric J. (2013). Central carbon metabolism and electron transport in Chlamydomonas reinhardtii: metabolic constraints for carbon partitioning between oil and starch. Eukaryotic Cell 12 (6), 776–793. doi: 10.1128/EC.00318-12
Keller M. D., Selvin R. C., Claus W., Guillard R. R. (1987). Media for the culture of oceanic ultraphytoplankton, Vol. 1. 2. doi: 10.1111/j.1529-8817.1987.tb04217.x
Kirk M. M., Kirk D. L. (1978). Carrier-mediated uptake of arginine and urea by Volvox carteri f. nagariensis. Plant Physiol. 61 (4), 549–555. doi: 10.1104/pp.61.4.556
Kumar A., Castellano I., Patti F. P., Palumbo A., Buia M. C. (2015). Nitric oxide in marine photosynthetic organisms. Nitric. Oxide 47, 34–39. doi: 10.1016/j.niox.2015.03.001
Lage S., Toffolo A., Gentili F. G. (2021). Microalgal growth, nitrogen uptake and storage, and dissolved oxygen production in a polyculture based-open pond fed with municipal wastewater in northern Sweden. Chemosphere 276, 130122. doi: 10.1016/j.chemosphere.2021.130122
Laliberté G., Hellebust J. A. (1990). Arginine utilization by Chlorella autotrophica and Chlorella saccharophila. Physiologia Plantarum 79 (1), 57–64. doi: 10.1111/j.1399-3054.1990.tb05866.x
Lancaster J. J.R. (2015). Nitric oxide: a brief overview of chemical and physical properties relevant to therapeutic applications. Future Sci. OA 1 (1). doi: 10.4155/fso.15.59
Lapina T., Statinov V., Puzanskiy R., Ermilova E. (2022). Arginine-Dependent Nitric Oxide Generation and S-Nitrosation in the Non-Photosynthetic Unicellular Alga Polytomella parva. Antioxidants 11 (5), 949. doi: 10.3390/antiox11050949
Le Bihan T., Martin S. F., Chirnside E. S., van Ooijen G., Barrios-LLerena M. E., O'Neill J. S., et al. (2011). Shotgun proteomic analysis of the unicellular alga Ostreococcus tauri. J. Proteomics 74 (10), 2060–2070. doi: 10.1016/j.jprot.2011.05.028
Li X., Moellering E. R., Liu B., Johnny C., Fedewa M., Sears B. B., et al. (2012). A galactoglycerolipid lipase is required for triacylglycerol accumulation and survival following nitrogen deprivation in Chlamydomonas reinhardtii. Plant Cell 24 (11), 4670–4686. doi: 10.1105/tpc.112.105106
Lin H. Y., Lin H. J. (2018). Polyamines in microalgae: something borrowed, something new. Mar. Drugs 17 (1), 1. doi: 10.3390/md17010001
Ma X., Cheng Z., Qin R., Qiu Y., Heng Y., Yang H., et al. (2013). OsARG encodes an arginase that plays critical roles in panicle development and grain production in rice. Plant J. 73 (2), 190–200. doi: 10.1111/j.1365-313x.2012.05122.x
Mallick N., Mohn F. H., Soeder C. J., Grobbelaar J. U. (2002). Ameliorative role of nitric oxide on H2O2 toxicity to a chlorophycean alga Scenedesmus obliquus. J. Gen. Appl. Microbiol. 48 (1), 1–7. doi: 10.2323/jgam.48.1
Matsumoto H., Hamasaki A., Sioji N., Ikuta Y. (1997). Influence of CO2, SO2 and NO in flue gas on microalgae productivity. J. Chem. Eng. Japan 30 (4), 620–624. doi: 10.1252/jcej.30.620
Mishra S. K., Suh W. I., Farooq W., Moon M., Shrivastav A., Park M. S., et al. (2014). Rapid quantification of microalgal lipids in aqueous medium by a simple colorimetric method. Bioresource Technol. 155, 330–333. doi: 10.1016/j.biortech.2013.12.077
Muñoz-Blanco J., Hidalgo-Martinez J., Cárdenas J. (1990). Extracellular deamination of L-amino acids by Chlamydomonas reinhardtii cells. Planta 182 (2), 194–198. doi: 10.1007/BF00197110
Munz J., Xiong Y., Kim J. Y. H., Sung Y. J., Seo S., Hong R. H., et al. (2020). Arginine-fed cultures generates triacylglycerol by triggering nitrogen starvation responses during robust growth in Chlamydomonas. Algal Res. 46, 101782. doi: 10.1016/j.algal.2019.101782
Nejamkin A., Foresi N., Mayta M. L., Lodeyro A. F., Castello F. D., Correa-Aragunde, et al. (2020). Nitrogen depletion blocks growth stimulation driven by the expression of nitric oxide synthase in tobacco. Front. Plant Sci. 11. doi: 10.3389/fpls.2020.00312
Noens E. E., Lolkema J. S. (2017). ). Convergent evolution of the arginine deiminase pathway: the ArcD and ArcE arginine/ornithine exchangers. Microbiologyopen 6 (1), e00412. doi: 10.1002/mbo3.412
Novák L., Zubáčová Z., Karnkowska A., Kolisko M., Hroudová M., Stairs C. W., et al. (2016). Arginine deiminase pathway enzymes: evolutionary history in metamonads and other eukaryotes. BMC evolutionary Biol. 16 (1), 1–14. doi: 10.1186/s12862-016-0771-4
Park J. J., Wang H., Gargouri M., Deshpande R. R., Skepper J. N., Holguin F. O., et al. (2015). The response of Chlamydomonas reinhardtii to nitrogen deprivation: a systems biology analysis. Plant J. 81 (4), 611–624. doi: 10.1111/tpj.12747
Plumley F. G., Schmidt G. W. (1989). Nitrogen-dependent regulation of photosynthetic gene expression. Proc. Natl. Acad. Sci. 86 (8), 2678–2682. doi: 10.1073/pnas.86.8.2678
Raven J. A., Beardall J., Giordano M. (2014). Energy costs of carbon dioxide concentrating mechanisms in aquatic organisms. Photosynthesis Res. 121 (2), 111–124. doi: 10.1007/s11120-013-9962-7
Rodolfi L., Biondi N., Guccione A., Bassi N., D'Ottavio M., Arganaraz G., et al. (2017). Oil and eicosapentaenoic acid production by the diatom Phaeodactylum tricornutum cultivated outdoors in Green Wall Panel (GWP®) reactors. Biotechnol. bioengineering 114 (10), 2204–2210. doi: 10.1002/bit.26353
Rodolfi L., Chini Zittelli G., Bassi N., Padovani G., Biondi N., Bonini G., et al. (2009). Microalgae for oil: Strain selection, induction of lipid synthesis and outdoor mass cultivation in a low-cost photobioreactor. Biotechnol. bioengineering 102 (1), 100–112. doi: 10.1002/bit.22033
Romano G., Costantini M., Buttino I., Ianora A., Palumbo A. (2011). Nitric oxide mediates the stress response induced by diatom aldehydes in the sea urchin Paracentrotus lividus. PloS One 6 (10), e25980. doi: 10.1371/journal.pone.0025980
Ruijter J. M., Ramakers C., Hoogaars W. M., Karlen Y., Bakker O., van den Hoff M. J., et al. (2009). Amplification efficiency: linking baseline and bias in the analysis of quantitative PCR data. Nucleic Acids Res. 37, e45. doi: 10.1093/nar/gkp045
Sakihama Y., Nakamura S., Yamasaki H. (2002). Nitric oxide production mediated by nitrate reductase in the green alga Chlamydomonas reinhardtii: an alternative NO production pathway in photosynthetic organisms. Plant Cell Physiol. 43 (3), 290–297. doi: 10.1093/pcp/pcf034
Sanchez F., Geffroy S., Norest M., Yau S., Moreau H., Grimsley N. (2019). Simplified transformation of Ostreococcus tauri using polyethylene glycol. Genes 10 (5), 399. doi: 10.3390/genes10050399
Sanz-Luque E., Chamizo-Ampudia A., Llamas A., Galvan A., Fernandez E. (2015). Understanding nitrate assimilation and its regulation in microalgae. Front. Plant Sci. 6, 899. doi: 10.3389/fpls.2015.00899
Saux C., Lemoine Y., Marion-Poll A., Valadier M. H., Deng M., Morot-Gaudry J. F. (1987). Consequence of absence of nitrate reductase activity on photosynthesis in Nicotiana plumbaginifolia plants. Plant Physiol. 84 (1), 67–72. doi: 10.1104/pp.84.1.67
Scheller M., Blobner M., Von Loewenich C., Schneck H., Stadler J., Franke C., et al. (1998). The NO synthase inhibitors L-NAME and L-NMMA, but not L-arginine, block the mammalian nicotinic acetylcholine receptor channel. Toxicol. Lett. 100, 109–113. doi: 10.1016/S0378-4274(98)00173-8
Schmollinger S., Mühlhaus T., Boyle N. R., Blaby I. K., Casero D., Mettler T., et al. (2014). Nitrogen-sparing mechanisms in Chlamydomonas affect the transcriptome, the proteome, and photosynthetic metabolism. Plant Cell 26 (4), 1410–1435. doi: 10.1105/tpc.113.122523
Seymour J. R. (2014). A sea of microbes: the diversity and activity of marine microorganisms. Microbiol. Aust. 35 (4), 183–187. doi: 10.1071/ma14060
Shahar B., Shpigel M., Barkan R., Masasa M., Neori A., Chernov H., et al. (2020). Changes in metabolism, growth and nutrient uptake of Ulva fasciata (Chlorophyta) in response to nitrogen source. Algal Res. 46, 101781. doi: 10.1016/j.algal.2019.101781
Six C., Worden A. Z., Rodríguez F., Moreau H., Partensky F. (2005). New insights into the nature and phylogeny of prasinophyte antenna proteins: Ostreococcus tauri, a case study. Mol. Biol. Evol. 22 (11), 2217–2230. doi: 10.1093/molbev/msi220
Terrado R., Monier A., Edgar R., Lovejoy C. (2015). Diversity of nitrogen assimilation pathways among microbial photosynthetic eukaryotes. J. Phycology 51 (3), 490–506. doi: 10.1111/jpy.12292
Tischner R., Planchet E., Kaiser W. M. (2004). Mitochondrial electron transport as a source for nitric oxide in the unicellular green alga Chlorella sorokiniana. FEBS Lett. 576 (1-2), 151–155. doi: 10.1016/j.febslet.2004.09.004
Todd C. D., Cooke J. E., Mullen R. T., Gifford D. J. (2001). Regulation of loblolly pine (Pinus taeda L.) arginase in developing seedling tissue during germination and post-germinative growth. Plant Mol. Biol. 45 (5), 555–565. doi: 10.1023/A:1010645616920
Vallon O., Spalding M. H. (2009). “Amino acid metabolism,” in The Chlamydomonas Sourcebook (Academic Press), 115–158. doi: 10.1016/B978-0-12-370873-1.00012-5
van Tol H. M., Armbrust E. V. (2021). Genome-scale metabolic model of the diatom Thalassiosira pseudonana highlights the importance of nitrogen and sulfur metabolism in redox balance. PloS One 16 (3), e0241960. doi: 10.1371/journal.pone.0241960
Vardi A. (2008). Cell signaling in marine diatoms. Communicative Integr. Biol. 1 (2), 134–136. doi: 10.4161/cib.1.2.6867
Vardi A., Formiggini F., Casotti R., de Martino A., Ribalet F., Miralto A., et al (2006). A stress surveillance system based on calcium and nitric oxide in marine diatoms. PLos Biol. 4 (3), e60. doi: 10.1371/journal.pbio.0040060
Vinogradov S. N., Hoogewijs D., Bailly X., Arredondo-Peter R., Gough J., Dewilde S., et al. (2006). A phylogenomic profile of globins. BMC Evolutionary Biol. 6 (1), 1–17. doi: 10.1186/1471-2148-6-31
Wase N., Black P. N., Stanley B. A., DiRusso C. C. (2014). Integrated quantitative analysis of nitrogen stress response in Chlamydomonas reinhardtii using metabolite and protein profiling. J. Proteome Res. 13 (3), 1373–1396. doi: 10.1021/pr400952z
Weisslocker-Schaetzel M., André F., Touazi N., Foresi N., Lembrouk M. Dorlet., et al. The NOS-like protein from the microalgae Ostreococcus tauri is a genuine and ultrafast NO-producing enzyme. Plant Sci. 265, 100–111. doi: 10.1016/j.plantsci.2017.09.019
Winter G., Todd C. D., Trovato M., Forlani G., Funck D. (2015). Physiological implications of arginine metabolism in plants. Front. Plant Sci. 6. doi: 10.3389/fpls.2015.00534
Yang S., Wang J., Cong W., Cai Z., Ouyang F. (2004). Utilization of nitrite as a nitrogen source by Botryococcus braunii. Biotechnol. Lett. 26 (3), 239–243. doi: 10.1023/B:BILE.0000013722.45527.18
Zhang W., Du G., Zhou J., Chen J. (2018). Regulation of sensing, transportation, and catabolism of nitrogen sources in Saccharomyces cerevisiae. Microbiol. Mol. Biol. Rev. 82 (1), e00040-17. doi: 10.1128/mmbr.00040-17
Keywords: Ostreococcus tauri, arginine, nitric oxide, NO synthase, nitrogen, lipids, growth
Citation: Foresi N, Caló G, Del Castello F, Nejamkin A, Salerno G, Lamattina L, Martínez-Noël G and Correa-Aragunde N (2022) Arginine as the sole nitrogen source for Ostreococcus tauri growth: Insights on nitric oxide synthase enzyme. Front. Mar. Sci. 9:1064077. doi: 10.3389/fmars.2022.1064077
Received: 07 October 2022; Accepted: 24 November 2022;
Published: 19 December 2022.
Edited by:
Sophia Letsiou, University of West Attica, GreeceReviewed by:
Angel Llamas, University of Cordoba, SpainHongli Cui, Shanxi Agricultural University, China
Copyright © 2022 Foresi, Caló, Del Castello, Nejamkin, Salerno, Lamattina, Martínez-Noël and Correa-Aragunde. This is an open-access article distributed under the terms of the Creative Commons Attribution License (CC BY). The use, distribution or reproduction in other forums is permitted, provided the original author(s) and the copyright owner(s) are credited and that the original publication in this journal is cited, in accordance with accepted academic practice. No use, distribution or reproduction is permitted which does not comply with these terms.
*Correspondence: Noelia Foresi, npforesi@mdp.edu.ar; Natalia Correa-Aragunde, mncorrea@mdp.edu.ar; Giselle Martínez-Noël, giselleastrid@gmail.com