Vocalizing humpback whales (Megaptera novaeangliae) migrating from Antarctic feeding grounds arrive earlier and earlier in the Perth Canyon, Western Australia
- 1Centre for Marine Science and Technology, Curtin University, Perth, WA, Australia
- 2School of Molecular and Life Sciences, Curtin University, Perth, WA, Australia
Migratory species undertake seasonal, long-distance travel between feeding and breeding grounds, and time their arrivals with high-quality resources. The Breeding Stock D population of humpback whales (Megaptera novaeangliae) migrates from Antarctic to Western Australian waters every austral winter. Based on 16 years (2002-2017) of passive acoustic recordings in the Perth Canyon, Western Australia, the hourly presence/absence of humpback whale vocalizations was used as an indicator of inter-annual changes in migration timing. A trend of earlier arrivals in the Perth Canyon by 1.4 days/year during the northward migration and possibly earlier departures from the Perth Canyon during the southward migration was observed. A distance-based linear model and a generalized linear model (GLM) both identified sea surface temperature (SST) as the most significant predictor for acoustic presence in the Perth Canyon. A 1 °C increase in SST corresponded to a decrease in humpback whale acoustic presence by 4.4 hours/day. Mean SST at the peak of the humpback whale season in the Perth Canyon was 19 °C. Exploratory analysis of the metocean environment of the Antarctic feeding grounds suggested that whales were leaving the Antarctic at the end of the austral summer, as sea ice concentration (SIC) increased and SST decreased. Further research should investigate whether changes in the metocean conditions on Australian breeding grounds correspond to changing departures from the Perth Canyon during the southward migration. If environmental conditions on breeding and feeding grounds change out-of-sync, migrating whales might be unable to arrive at either ground during optimal conditions.
1 Introduction
Migration is an evolved survival mechanism prevalent across a multitude of taxa around the world (Dingle and Drake, 2007). It is defined as a long-range, mass movement of a population towards high-quality habitat or resources (Dingle, 2014). Long-distance travel such as this is energy costly, and the decision to disperse is a trade-off between prey availability, suitability of habitat, and opportunities to breed (Alerstam et al., 2003; Bauer et al., 2011). To ensure synchronicity between seasonal resources, migration timing is triggered by both extrinsic and intrinsic factors (Dingle and Drake, 2007). Some examples of migration drivers include environmental factors, photoperiod, and internal cues (Bauer et al., 2011). Species that rely on such fine-scale changes to initiate migration may experience discrepancy between optimum habitat and presence. A common example is the movement of migrant birds between breeding and feeding grounds (Mayor et al., 2017). Different rates of environmental change between the two habitats create a mis-match. For example, migration arrivals no longer match up with peak insect production, generating a negative fitness consequence for migratory birds (Dobson et al., 2017; Mayor et al., 2017). Long-term monitoring is essential to understand trends in migration arrivals and departures, along with the environmental factors that influence presence. Many terrestrial migrants are well studied and show vulnerability in the face of climate change; yet, comparatively little research has been dedicated towards susceptibility of migrants in a changing marine environment (Balbontín et al., 2009; Mayor et al., 2017; Rickbeil et al., 2019).
The humpback whale (Megaptera novaeangliae) annually migrates to access quality habitats with optimum environmental conditions. Specifically, the animals migrate from polar, higher-latitude feeding grounds in the summer months to lower-latitude, temperate/tropical breeding grounds in the winter months (Fossette et al., 2014; Andrews-Goff et al., 2018). In the Southern Hemisphere, populations of humpback whales have been divided into different stocks, dependent upon their genetic structure and feeding grounds in Antarctic waters (IWC, 2007). The population migrating through Western Australia (WA) belongs to the Southern Hemisphere population known as Breeding Stock D (BSD) (IWC, 2007). These animals spend November to May in Antarctica feeding on Antarctic krill (Euphausia superba) and taking advantage of the highly productive upwellings (Jenner et al., 2001; Bestley et al., 2019). Historic whaling records and satellite tags show that this population resides in the Antarctic International Whaling Commission (IWC) Management Area IV during this time (Jenner et al., 2001; Matsuoka et al., 2011; Bestley et al., 2019). Amongst others, the Southern Kerguelen Plateau is a hotspot for phytoplankton blooms, which consequently attracts high densities of humpback whales during the feeding season (Schallenberg et al., 2018; Bestley et al., 2019). Once this resource is depleted, the population disperses north and is found from June to November in Australian waters (Jenner et al., 2001). But not all humpback whales migrate simultaneously.
The humpback whale migration is staggered into a progression of cohorts. This behavior is known as “temporal segregation” and is recognized in humpback whale populations around the world (Dawbin, 1997; Recalde-Salas et al., 2020). Migration starts with the resting females (not lactating or with year-old calves) travelling from Antarctic waters to South-West Australia (Dawbin, 1997). Sequentially, the following cohorts are the male and female juveniles, females that are not pregnant or lactating, then males, and the last to arrive in Australia are the heavily pregnant females (Dawbin, 1997). The population travels north along the WA coast to reach the high-latitude breeding grounds in the Kimberley region in North-West Australian waters (Jenner et al., 2001). The 6000 km structured migration is reversed when the population begins the journey back to Antarctic waters (Jenner et al., 2001). Resting females are followed by juveniles and mature males (Dawbin, 1997). Females with newborn calves are last to leave the continent (Dawbin, 1997). In some instances, male humpback whales will linger to travel with mothers and act as an “escort” for breeding advantages or protection against killer whales (Smith et al., 2008).
Aside from predators, humpback whales face many threats throughout their migration including pollution (e.g., noise or plastic), entanglement, and ship collisions (Prideaux, 2003). Lesser known are the impacts of environmental change on the fitness of humpback whales. Sea surface temperature (SST), particulate organic carbon (POC), chlorophyll a (Chl-a), and sea ice concentration (SIC) have seasonal fluxes that impact the distribution and abundance of whales (Doney et al., 2012; Cheung et al., 2013; Melbourne-Thomas et al., 2016; Chavez-Rosales et al., 2019; Szesciorka et al., 2020). Some population studies have found long-term trends of humpback whales arriving earlier to breeding grounds, with strong influences from environmental variables (Avila et al., 2020; Davis et al., 2020; Szesciorka et al., 2020). Temporal shifts in arrival and departure dates have not been studied in the BSD population. Migration timing discrepancies between optimum Antarctic feeding grounds and Kimberley breeding grounds may pose an additional threat to the population. However, collecting long-term data on whale migration can be expensive and prohibitive (Nowacek et al., 2016). Therefore, we used passive acoustic detections as a proxy for whale presence to investigate migration timing.
Passive acoustic monitoring has identified humpback whale presence since the 1950s, when musical tones in Hawaiian waters were attributed to their seasonal migration (Schreiber, 1952). The humpback whale vocal repertoire is exhaustive and can be broken down into recognizable components that distinguish them from other vocal marine species (Payne and McVay, 1971). Humpback whales produce song and non-song sounds (Payne and McVay, 1971; Recalde-Salas et al., 2020). The complexities of songs can be dissected into smaller components. Discrete sounds are referred to as a “unit”, units make up a “phrase”, and repeating phrases compose a “theme”. Humpback whale songs are sequenced themes that can last from minutes to days (Payne and McVay, 1971). Song is heard in migratory pathways as well as feeding and breeding grounds (Payne and McVay, 1971; Winn and Winn, 1978; Tyack, 1981; Smith et al., 2008; Schall et al., 2021). Humpback whale song is exclusive to males and suggested as being a sexually selected trait (Payne and McVay, 1971). Discrete units may also be uttered as unstructured, stand-alone non-song sounds (Dunlop et al., 2008; Recalde-Salas et al., 2020). All cohorts produce non-song sounds and the function of these discrete units is proposed as social communication or a navigational tool (Payne and McVay, 1971; Winn and Winn, 1978; Tyack, 1981; Mercado and Perazio, 2022). The complex and hierarchical qualities of vocalizations can potentially distinguish males from females, populations from neighboring groups, and humpback whales from any other species in a marine soundscape (Payne and McVay, 1971; Tyack, 1981; Winn et al., 1981)
Based on 16 years of marine soundscape recording in the Perth Canyon, the goals of this study were to: (i) determine past migration arrival and departure dates in the Perth Canyon; (ii) identify any long-term trends or differences in humpback whale presence between years; (iii) evaluate the frequency of occurrence (FO) for humpback whale vocalizations per day and model against environmental variables (SST, Chl-a, and POC); and (iv) conduct an exploratory analysis of Antarctic environmental variables against the call FO in the Perth Canyon with a time lag.
2 Methods
2.1 Site description
The Perth Canyon is located on the south-western continental shelf, approximately 50 km west from Perth; it is 120 km long (Trotter et al., 2019). The passive acoustic recorders were deployed on the eastern plateau of the Perth Canyon, in depths between 400 and 490 m (Figure 1). The canyon intrudes on the continental shelf and extends 4000 m in depth. It is home to a variety of deep-sea fauna including deep-sea cnidaria, echinoderms, fish, and cetaceans (Erbe et al., 2015; Trotter et al., 2019). The Leeuwin current runs over the Perth Canyon and contributes to the seasonal upwellings of nutrients. Long-distance migrants take advantage of the seasonally increased primary production (Feng et al., 2009; Rennie et al., 2009). Pygmy blue whales (Balaenoptera musculus brevicauda), Antarctic blue whales (Balaenoptera musculus intermedia), fin whales (Balaenoptera physalus), Antarctic minke whales (Balaenoptera bonaerensis), and humpback whales have been identified acoustically while migrating through the Perth Canyon (Erbe et al., 2015). Specifically, the Perth Canyon is a migratory corridor for the BSD population of humpback whales on their northward and southward routes (Jenner et al., 2001).
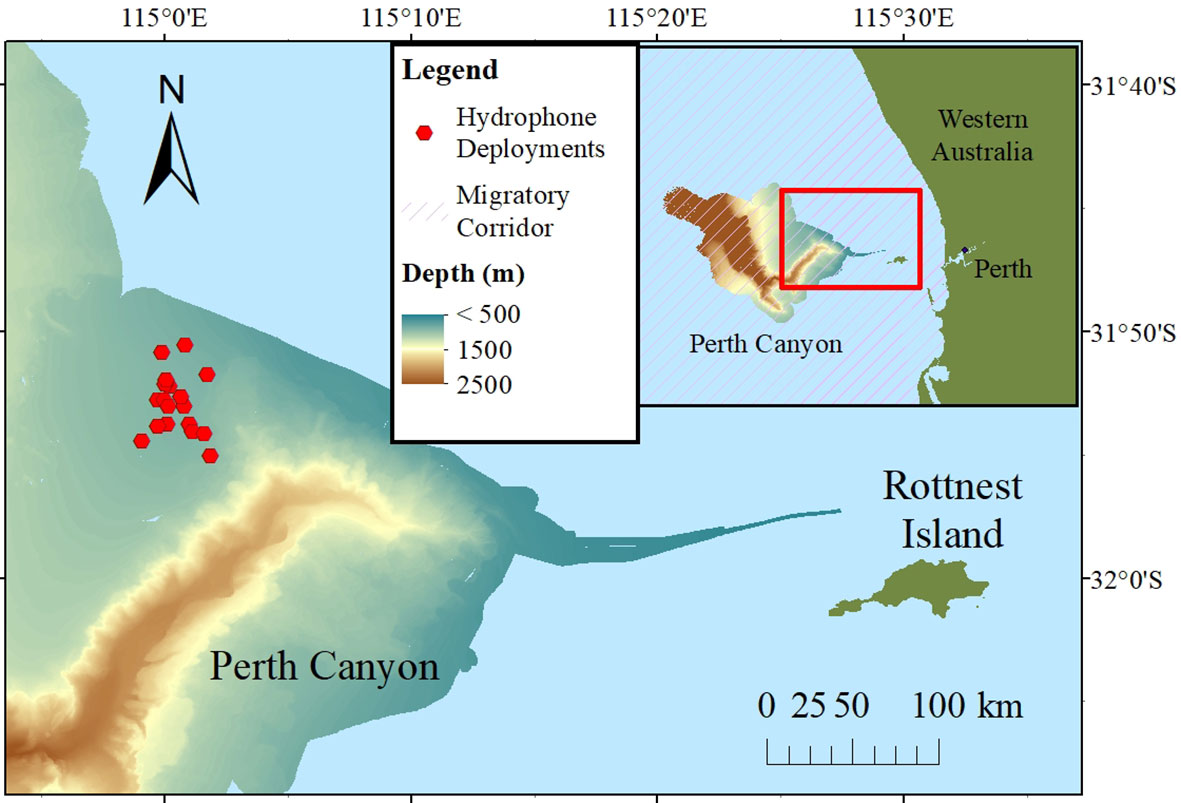
Figure 1 Map of the Perth Canyon study site showing the locations of the underwater acoustic recorders (red hexagons). The Perth Canyon bathymetry data was extracted from the Marine Geoscience Data system (MGDS; www.marine-geo.org/tools/datasets/22035; McCulloch, 2016). The migratory corridor shapefile was provided by the International Union for the Conservation of Nature (IUCN) Marine Mammal Protected Areas Task Force (IUCN MMPATF, 2021). Map was created using ArcGIS (Version 10.8.1, Esri, Redlands, CA, USA).
2.2 Data source
This study investigated humpback whale acoustic presence in the Perth Canyon using underwater acoustic recordings collected by the Centre for Marine Science and Technology (CMST), Curtin University. All recordings were collected with a CMST-built Underwater Sound Recorder (USR; McCauley et al., 2017), using HighTech Inc. HTI-90-U hydrophones (sensitivity –186 dB re 1 V/µPa, 2 Hz – 20 kHz). The recorders were calibrated with input white noise prior to deployment. Deployments were temporally staggered between 2002 and 2017. Recordings from 2009 onwards were collected as part of the Australian Integrated Marine Observing System (IMOS). Refer to Table 1 for deployment set numbers, start and end times, coordinates, sampling rates, and duty cycles.
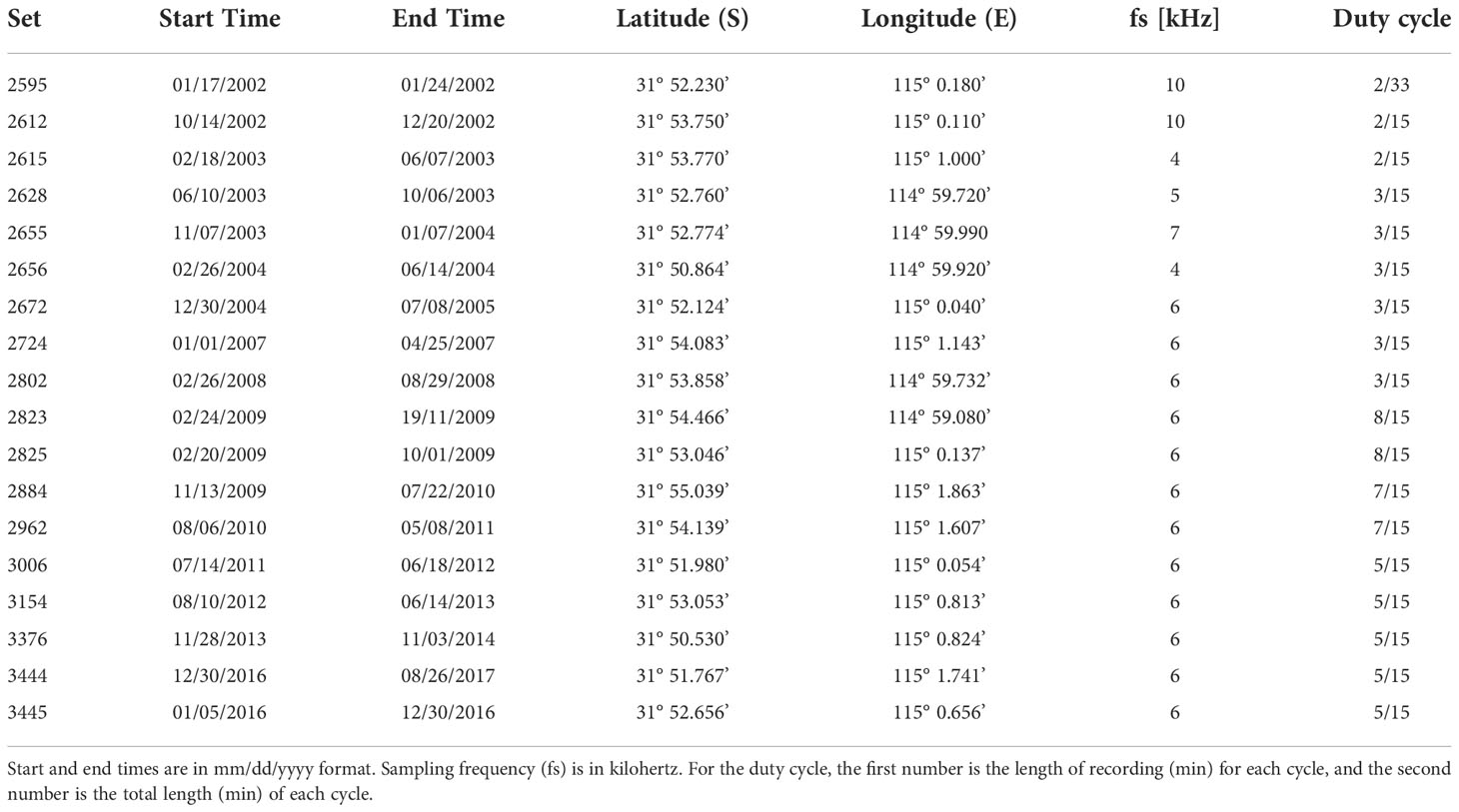
Table 1 Recorder deployment details for the sets (n = 18) used to detect humpback whale vocalizations in the Perth Canyon.
Daily environmental data for the Perth Canyon (SST, Chl-a, and POC) and Antarctic Area IV (SST, Chl-a, POC, and SIC) were sourced from 2000 to 2018. A daily mean was calculated for each region utilizing the boundaries of the Perth Canyon Marine Park (Australian Government, 2013) and the Antarctic Area IV waters, south of 60° (IWC, 2007). SST was extracted from a single-sensor multi-satellite system using observations from Advanced Very-High-Resolution Radiometer (AVHRR) instruments on National Oceanic and Atmospheric Administration (NOAA) polar-orbiting satellites with a 0.02° × 0.02° grid cell size. Bias between satellite and buoy matchups is included for every cell. The SST dataset was summarized to only include cells with the level 5 highest quality. Data were obtained using the Australian Ocean Data Network (https://portal.aodn.org.au/ , accessed 6th October 2021). Chl-a and POC data were provided by NOAA and obtained from the Environmental Research Division’s Data Access Program (ERDDAP; https://coastwatch.pfeg.noaa.gov/erddap/ , accessed 6th October 2021). Data from 2003 onwards were collected in a 4 km grid on the National Aeronautics and Space Administration (NASA) Aqua Spacecraft using a Moderate-Resolution Imaging Spectroradiometer (MODIS). There was Chl-a availability prior to 2003 onboard separate satellites and using different equipment. A cross-correlation was used between two satellites for a comparable year, and data prior to 2003 were utilized if R2 > 0.7. Therefore, Antarctic Chl-a data from 2000 to 2002 were collected in a 4 km grid on the NASA Orbview-2 satellite using a Sea-viewing Wide Field-of-view Sensor (SeaWiFS). Only Science Quality level 3 data were extracted. Daily Antarctic SIC was obtained from the National Snow Ice and Data Centre (NSIDC; https://nsidc.org/ , accessed 25th March 2022; Fetterer et al., 2017). Data were collected in a 25 km grid using a Scanning Multichannel Microwave Radiometer (SMMR) onboard the NASA Nimbus-7 satellite. Concentration values less than 15% were removed from the SIC mean calculation due to data uncertainty from the passive microwave instruments.
Humpback whale population size for every year of acoustic data was computed based on the 13% annual growth estimate by Salgado Kent et al. (2012b), who undertook aerial surveys between 2000 and 2008.
2.3 Call detections
Humpback whale vocalizations were identified based on repertoire descriptions of song and non-song catalogues in the literature (Payne and McVay, 1971; Recalde-Salas et al., 2020) and co-authors’ prior experience with this population. Humpback whale acoustic presence was visually and aurally determined every hour using spectrograms in Raven Pro (Version 1.6, The Cornell Lab of Ornithology, Ithaca, NY, USA), which were created with 2048-point discrete Fourier transforms, Hann windows, and 50% overlap. Spectrograms were scanned in hourly increments for humpback whale vocalizations between 10 Hz and 2 kHz. Presence per hour was determined by selections of non-song or song within each increment. Raven selection tables were exported to xls format.
2.4 Data pre-processing
Hourly presence/absence of humpback whale vocalizations was calculated in MATLAB (Version R2020b, The MathWorks Inc., Natick, MA, USA). Raven selection tables were read into MATLAB and the frequency of occurrence (FO) of humpback vocalizations was calculated as the number of hours that humpback whales were acoustically present per 24 h day. The FOs for the start and end days of deployment were computed as the number of hours with humpback whale vocalizations divided by the number of hours the recorder was in the water on those days.
The environmental variables in the Perth Canyon and Antarctica were averaged to get a daily SST, POC, Chl-a, and SIC value. The datasets were all resourced from satellite imagery, and therefore cloud cover resulted in missing data points.
2.5 Data analyses
2.5.1 Migration arrivals and departures
The date of arrival (A25) of BSD humpback whales in the Perth Canyon was determined as the first day of the year when the call FO exceeded 6/24 hours (25%). This is when the population is ascertained to have begun their northward migration through the Perth Canyon. The departure date (D25) was determined as the last day of the year when the call FO exceeded 6/24 hours. Thus, the departure date is when the BSD population has finished their southward migration through the Perth Canyon. This criterion was arbitrarily selected as a consistent parameter to measure the limits of the BSD’s migration, thus removing the early/late outliers of individual whales in the population. We then performed a linear regression to identify trends in A25 and D25 over the years. We required a 5-day recording buffer before A25 and after D25; if fewer than 5 days of recordings were available prior to A25 or after D25, then we removed the year from our regression.
2.5.2 Differences in frequency of occurrence
The PERMANOVA+ add-on (Anderson et al., 2015) in PRIMER v7 (Clarke and Gorley, 2015) was chosen to analyze the univariate frequency data. A two-factor PERMANOVA (McArdle and Anderson, 2001) with “Year” and “Week of year” as fixed factors was used to test statistically significant differences in inter-annual and weekly variation in humpback whale FO. The test was based on a Euclidean distance resemblance matrix with Type III partial sums of squares and unrestricted permutation of raw data totaling 9999 permutations. For post-hoc pairwise comparisons of significant terms, Monte Carlo p-values were computed when the number of permutations was <100 (Anderson et al., 2015).
2.5.3 Influence of Perth Canyon environmental variables
The mean and standard deviation (SD) of the environmental variables in the Perth Canyon (SST, SST bias, Chl-a, and POC) were tested for multicollinearity in Draftsman’s plots, produced in PRIMER v7. Week of year was correlated with environmental variables in Excel (Version 2019, Microsoft, Redmond, WA, USA) to determine seasonal correlation in the data. Variables with R2 correlation values >0.7 were removed from the analyses (Ratner, 2009). The correlation tests ensure that the predictor variables will create a parsimonious model. The deployment days with missing environmental values and outliers were removed from the dataset. The predictor variables were input into a DISTance-based Linear Model (DISTLM) (Anderson, 2005) to find the best predictors of variability in humpback whale FO (Euclidean distance resemblance matrix; Type III partial sums of squares; fixed effects sum to zero for mixed terms; unrestricted permutation of raw data; 9999 permutations). The percentage of variation and significance were output for each variable from the marginal tests. The overall best model of the groups was determined by the lowest small-sampled, corrected, Akaike Information Criterion (AICc), which was selected to reduce bias in the smaller sample size (Hurvich and Tsai, 1991). The final model provided the variables that best determined the FO, as well as an R2 value that described the percentage of variation that the model explained.
A generalized linear model (GLM) was trialed as it provides additional flexibility in exploring non-linear relationships between the predictors and response through the link function. The eight time series of the environmental variables (i.e., means and SDs of SST, SST bias, Chl-a, and POC) as well as FO were smoothed with a 7-day moving-average filter to reduce day-to-day variability and rather focus on weekly trends. We linearly interpolated over missing values in any of the variables up to a maximum of 7 days. If more than 7 days of data were missing, we kept the gap in that variable (as NaN). Next, the time series were normalized by subtracting the mean and dividing by the root-mean-square. The GLM was fitted using the stepwiseglm function in MATLAB, which performs a stepwise regression, starting with a constant model, adding and subtracting one term at a time, keeping only the relevant terms, until the model cannot be improved further. The terms trialed were the linear terms for each predictor, and all 2-predictor products (i.e., all possible combinations of any two predictors). With predictors and response being of type real, stepwiseglm was run with the identity link function.
2.5.4 Exploration of Antarctic environmental variables
As there remained substantial outliers in the Antarctic environmental data, the time series were further pre-processed by removing values deviating more than 3 SDs in a 20-day moving-average window. We linearly interpolated across gaps in the data (from cloud cover and from outlier removal) and smoothed the time series as above.
To determine the BSD northward travel speed from Antarctic Area IV to the Perth Canyon, a literature review was conducted. Modest et al. (2021) tagged humpback whales migrating from Antarctica to South America, across the open ocean and then along the coast. Firstly, the average speed from Antarctica to Cape Leeuwin was derived from the mean speed of the Breeding Stock G (BSG) population travelling across the open ocean from the Western Antarctic Peninsula to Cape Horn. Secondly, the average speed from Cape Leeuwin to the Perth Canyon was estimated using the mean speed of humpback whales travelling along the South American coast from Cape Horn to Peninsula de Paracas. The distance travelled between Antarctica and the Perth Canyon was estimated using the BSD southward migration routes to the Antarctic feeding grounds as determined by tag data from Bestley et al. (2019). The distance-to-speed ratios for the two lags (open ocean and coast) were subtracted from the A25 in the Perth Canyon to estimate the environmental conditions in Antarctica when the BSD whales presumably initiated their northward migration. With d representing the travel distance and v the speed, from Antarctica to WA (Ant) and along the WA coast (WA), the travel duration T was computed as:
We then investigated what the features of the Antarctic environment were at the time of approximate departure.
3 Results
3.1 Perth Canyon migration arrivals and departures
The FO in the Perth Canyon is visualized in Figure 2. Across the years, whales were acoustically present in the months of May to December. While this period captured two migrations (first northward, then southward), there was no gap in FO in between, so that the migration direction could not be separated at this recording site.
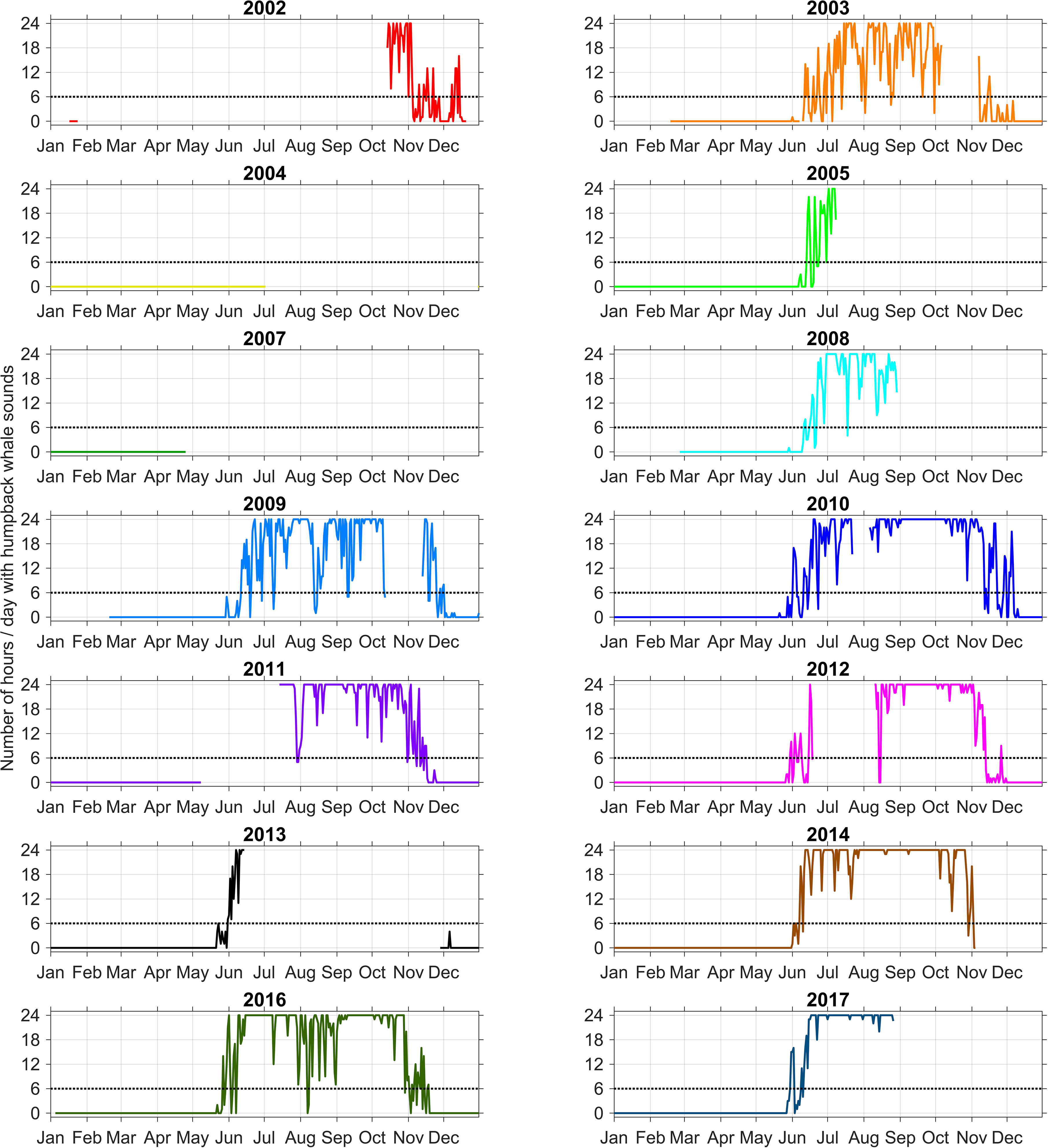
Figure 2 Yearly line graphs of humpback whale acoustic FO. Date ticks correspond to the first day of each month. The arrival and departure boundary are indicated by the black dotted line at a FO of 6h/24h = 0.25 (n = 3358).
Between 2003 and 2009, migration A25 were mid-June, whereas the most recent years (2010-2017) had humpback whale A25 in late-May to early-June (Figure 3). The earliest A25 was the 23rd May in 2013 and the latest A25 was the 13th June in 2005. The rate of change of A25 was -1.4 days/year.
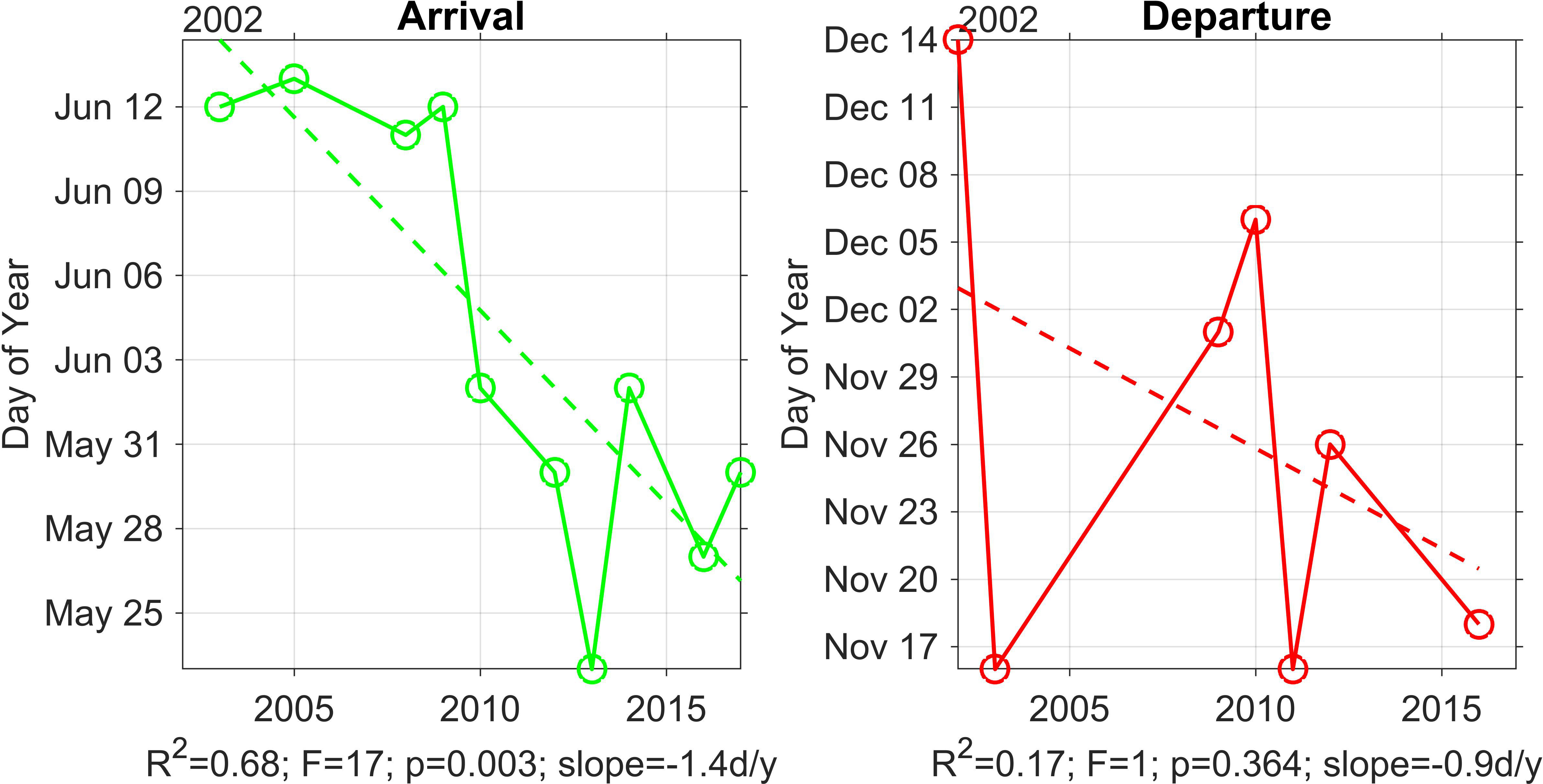
Figure 3 Scatter plots of humpback whale arrival (n = 10) and departure (n = 7) dates for the years of available passive acoustic monitoring data in the Perth Canyon. A linear trendline has been fitted; regression statistics (R2, F, and p-value) are noted beneath each plot.
The D25 were more variable across a longer temporal scale. BSD humpback whales departed the Perth Canyon in early-December to late-November in the early years (2002-2010), except for 2003, which had an unusually early D25. The departures of the population in the later deployment years (2011-2017) were from early to mid-November. The earliest D25 was the 16th November in 2003 and 2011 and the latest D25 was the 14th December in 2002. The rate of change of D25 was insignificant (Figure 3).
3.2 Perth Canyon FO PERMANOVA
The difference of FO between Year, Week of Year, and the interaction between these variables was significant by permutation (p-value ≤ 0.001; Table 2). A post-hoc pairwise analysis showed that the weeks of year in which humpback whales arrived differed significantly between years (PERMANOVA: p-value< 0.05). One of the earlier weeks of arrival (week 23) was in early-June and there were significant differences in humpback whale FO between the early years in the study (2003, 2004, 2005, 2008) and the later years in the study (2012, 2013, 2014, 2016, 2017). The last week of departure from the Perth Canyon was in week 50 and FO was significantly different between the earliest year of data (2002) and all other years (2003, 2009, 2010, 2011, 2012, 2013, 2016). There was variable significance in FO between years in the preceding weeks of departures.

Table 2 Results of PERMANOVAs examining the effects of year, week of year, and the interaction between these factors on humpback whale FO (n = 3358).
3.3 Perth Canyon DISTLM
The Draftsman’s plot found positive, approximately linear correlations between Population size and Year (Figure S1), as well as between Mean and SD of POC and Chl-a (Figures S2, S3). There was also a sinusoidal correlation between Week of Year and SST (Figure S4). The variables POC Mean, POC SD, Population size, and Week of Year were removed from the DISTLM dataset to create the most parsimonious model.
The DISTLM results showed that the highest proportion of the variation in humpback whale acoustic FO was explained by SST Mean, SST SD, and Year (Table 3). These groups were also statistically significant (p ≤ 0.001). The DISTLM model of best AICc fit utilized all selections, and these predictor variables explained 63.2% of the variation in the humpback whales’ vocalizations FO in the Perth Canyon (RSS = 120.65).
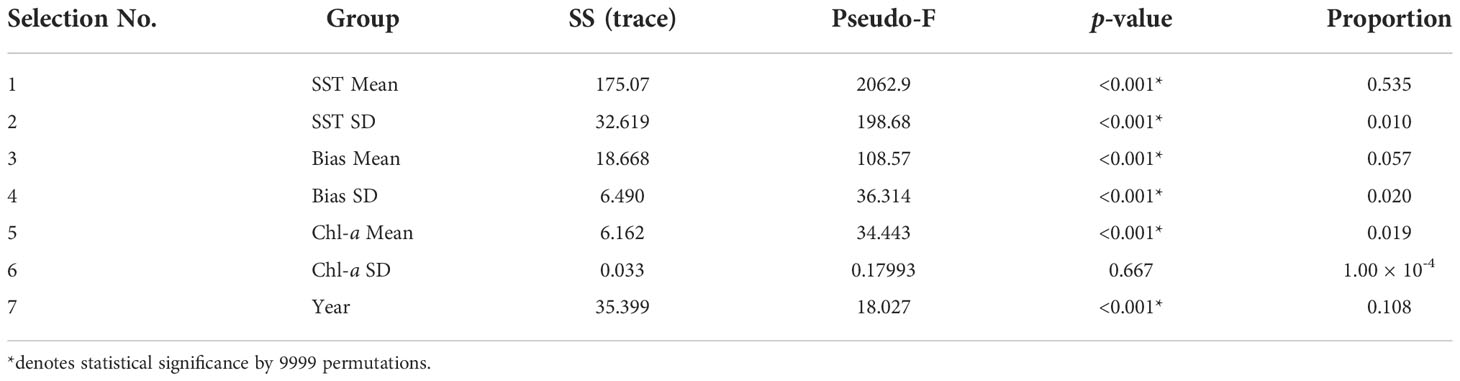
Table 3 DISTLM marginal test results between group and humpback whale FO showing the proportion of the variation explained by the predictor variable (n = 1798).
3.4 Perth Canyon GLM
Out of the predictors, the GLM identified POC Mean as more significant than Chl-a Mean, and so Chl-a was dropped from the model. Year was a stronger predictor than Population size, and so the latter was dropped from the model. The effect of SST bias was insignificant, as was the effect of POC SD. The final model retained the variables SST Mean, SST SD, POC Mean, and Year, and the pair products listed in Table 4. The normal probability plot of residuals is shown in Figure S5. In order of decreasing deviance, the model identified SST Mean as the strongest predictor, followed by POC Mean, then the product of SST Mean and POC Mean, then Year (Table 5). Given the predictor variables were normalized to standard Gaussians, the estimated coefficients were comparable; and so, the influence of SST Mean on humpback whale FO was more than four times stronger than that of the other environmental variables. A 1 °C increase in SST corresponded to a 4.4 h/d decrease in humpback whale acoustic presence—according to the model. At the peak of the humpback whale season in the Perth Canyon (when humpback whale sounds were detected 24 h/d), the mean SST was 19 °C.
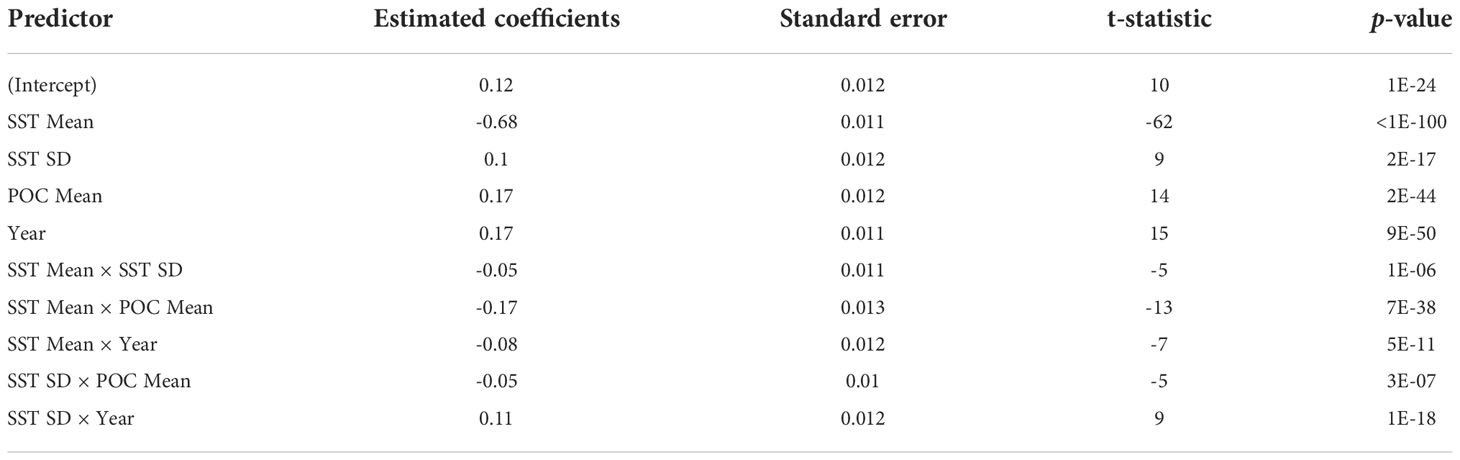
Table 4 GLM specifications estimating the effects of Perth Canyon environmental variables on humpback whale acoustic presence: Predictor variables and their combinations, estimated coefficients, standard errors, t-statistics and p-values.
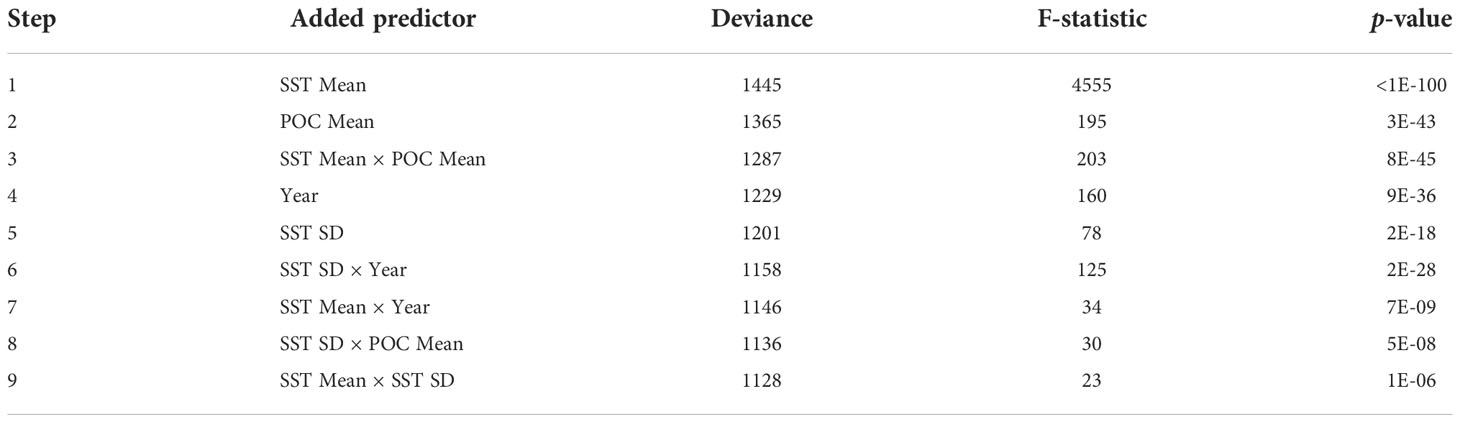
Table 5 Stepwise GLM estimating the effects of Perth Canyon environmental variables on humpback whale acoustic presence: Order of predictors added to the model in decreasing deviance, showing F-statistic and p-value for each step.
3.5 Antarctic environmental correlations
Based on Eq. 1, with dAnt=5100 km, dWA=300 km, vAnt=5.48 km/h, and vWA=6.48 km/h, the estimated travel time from Antarctica to the Perth Canyon was ~40 days. Figure 4 shows the five time series and the values of the Antarctic environmental variables at the estimated departure dates from Antarctica. SST, Chl-a, and POC dropped past their peak in the early months of the year, months prior to the peak of humpback whale vocalizations being detected in the Perth Canyon. Sea ice concentration was lowest when the other environmental variables were at their peak. Humpback whales seemed to depart when SST was, on average, -0.33 °C (higher in recent than earlier years), Chl-a was 0.24 mg/m3, POC 75.0 mg/m3, and SIC 29.6%.
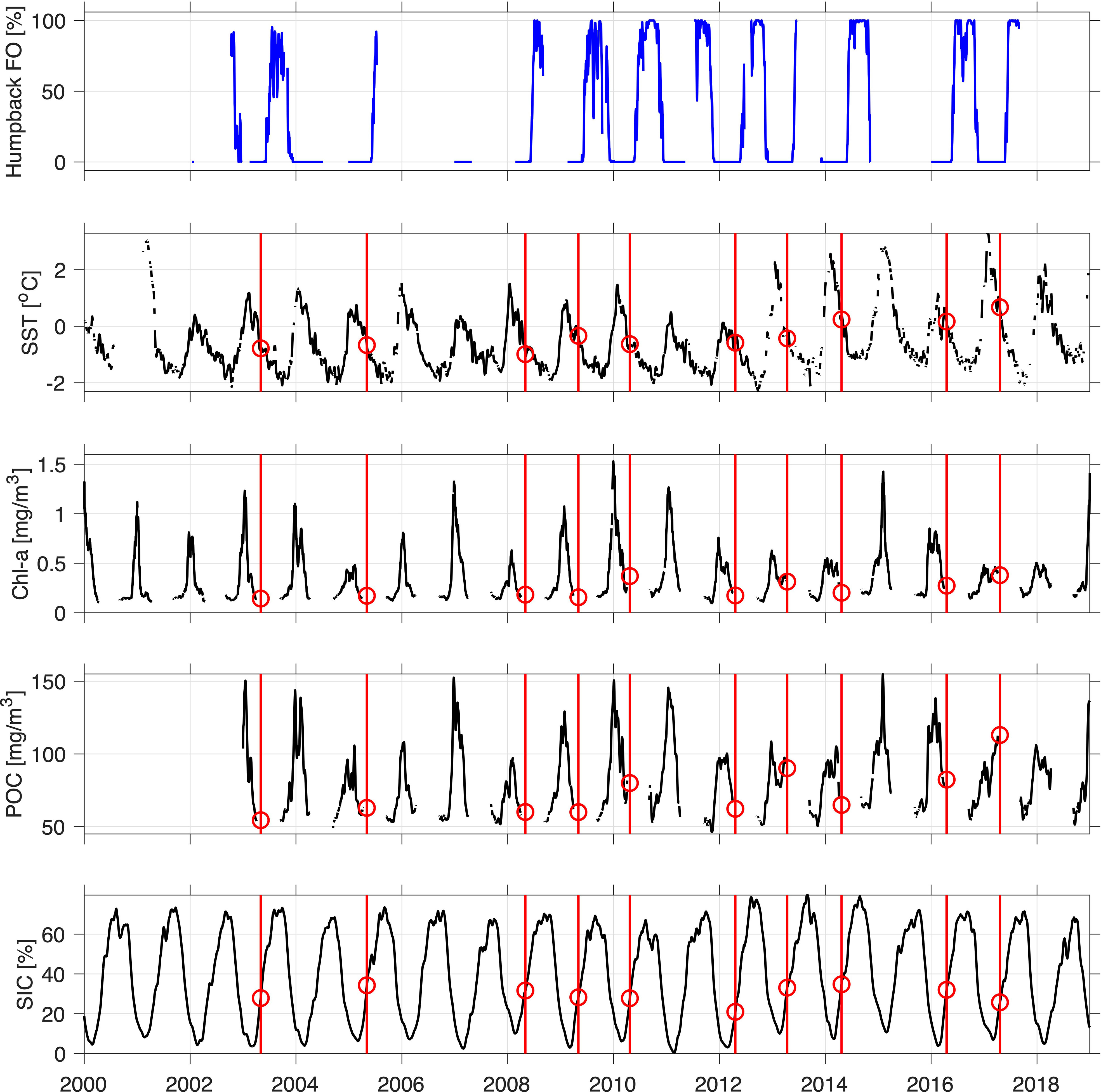
Figure 4 Time series of environmental variables in Antarctic Area IV between 2000 and 2019 with the humpback whale acoustic presence in the Perth Canyon. Red vertical lines indicate dates 40 days before humpback whale arrival in the Perth Canyon. Red circles indicate the environmental conditions when humpback whales possibly departed Antarctica.
4 Discussion
The long-term acoustic examination of the humpback whale BSD population while travelling through the Perth Canyon provided insight into their migration timing. The study revealed thresholds of the north- and southward migrations, determined shifts in migration timing between years, provided environmental predictors of humpback whale acoustic FO in the Perth Canyon, and explored Antarctic environmental conditions at the time when the population likely departed their feeding grounds, heading for WA.
4.1 Temporal shifts in migration
This study showed a gradual change in humpback whale acoustic arrival dates in the Perth Canyon over the years 2002-2017. Both arrival and departure dates in the later years of the study were weeks premature compared to those in the earlier years. However, departure dates exhibited great variability and there were fewer years with acoustic recordings at the time of departure, so that the shift in departure dates was statistically insignificant. These results, which are entirely based on passive acoustic monitoring, are consistent with visual observations in Geographe Bay, WA, finding an earlier shift of the humpback whale season by approximately four weeks over the last 15 years (C. Burton, personal communication, 21st October 2021). The shift of humpback whale presence in the Perth Canyon alters the migration periods described in Jenner et al. (2001): Original estimations of northbound and southbound migrations were mid-late June and mid-October, respectively. The later years in our study estimate that the boundaries of the migration are now late-May to early-June for the arrivals and possibly early to mid-November for the last departures (pending further data collection to increase statistical power). These migration shifts are concurrent with the literature of humpback whale populations elsewhere, with varying rates of change or suggested influences (Ramp et al., 2015; Avila et al., 2020).
4.2 Predictors of Humpback Whale presence
Extrinsic factors are posed as potential triggers for these shifts in migration. Different predictors for humpback whale presence have been found for different populations and geographic regions. At a summer feeding ground in the Gulf of St Lawrence (Canada), earlier arrivals related to sea ice extent and SST (Ramp et al., 2015). Avila et al. (2020) found no correlation to SST but instead suggested ice sheet mass as a predictor for an earlier migration arrival at breeding grounds in Gorgona National Park (Colombia). Possible predictors of humpback whale presence are not consistent in the literature and may be population or site specific.
Our model showed humpback whale acoustic FO in the Perth Canyon was largely driven by Year and SST. Call FO was temporally predicted by Year in the DISTLM, meaning the acoustic presence of humpback whales was not consistent between years. This could be influenced by population densities or behavior. The population growth was estimated as 13% per year by Salgado Kent et al. (2012b) based on population counts between 2000 and 2008, and this may be reflected in the species’ earlier (and ultimately longer) acoustic presence over the years. Others have speculated that a growing population shows earlier migration arrivals (Beazley, 2022). Alternatively, fluctuations in acoustic FO might reflect variability in the proportion of humpback whales migrating from Antarctica each year. Van Opzeeland et al. (2013) found pervasive acoustic detection of humpback whales in Antarctic waters year-round, suggesting that not all individuals complete a full migration each year, and the proportion of the population that undertakes long-distance travel may fluctuate between years based on environmental factors on their feeding grounds (van Opzeeland et al., 2013; Ramp et al., 2015; Avila et al., 2020). A high percentage of the migrating humpback whale population are males, implying that females might circumvent departure in particular years if they cannot afford the energy cost of long-distance travel (Brown et al., 1995; Alerstam et al., 2003). Humpback whales rely on high volumes of krill during the feeding season to build energy reserves for migration (Riekkola et al., 2020). Krill abundance is heavily influenced by SST, thus, environmental variability between years may affect their fitness for migration (Alerstam et al., 2003; Kawaguchi et al., 2013; Melbourne-Thomas et al., 2016; Riekkola et al., 2020).
SST was the strongest environmental predictor for humpback whale presence in the Perth Canyon and this finding aligns with observations elsewhere (Ramp et al., 2015; Chavez-Rosales et al., 2019). For example, along the eastern coast of Australia, humpback whales departed weeks earlier than normal during years of higher temperatures (Meynecke et al., 2017). Consequently, the parameters around migration timing and the presence of the BSD population in the Perth Canyon would be affected by future trends in SST and, on a larger scale, climate change. The southwest Australian waters have experienced an increase in SST over the last few decades (Foster et al., 2014). Coupled with our study results, the rising SST will likely impact the BSD migration in the future. The shifting arrival dates may already be an adaptation to climate change as hypothesised by Ramp et al. (2015). Looking at other taxa, pied flycatchers showed flexibility in migration timing, possibly to keep up with climate change (Both, 2010). Specifically, the birds shifted their migration to more closely align with a shifting food peak. Similarly, in our study, the shifting arrival dates of humpback whales in the Perth Canyon may be a behavioral response to triggering environmental variables on their Antarctic feeding grounds.
4.3 Environmental variables on Antarctic feeding grounds
Assuming a constant (over the years) migration duration from Antarctica to WA, humpback whales would have left Antarctica when SST, Chl-a, and POC were well past their peak and SIC had started to increase again. We hypothesize that shifts in arrival in the Perth Canyon (during northward migration) may be linked to environmental conditions on the feeding grounds. Tsujii et al., (2021) found that the annual migration timing of bowhead whales was linked to both SIC and SST in the Arctic. Antarctic SST may be slowly increasing on the feeding grounds and acting as a trigger for the population to leave earlier (Mintenbeck, 2017).
Once again, the increasing population may create a natural temporal expansion of the migration parameters and pressure more individuals to initiate migration earlier. Conversely, a theorized earlier departure from Antarctica may be in relation to temporal shifts in the peak availability of food. Krill abundance may not thoroughly nourish the entire population sufficiently; an exhaustion of the food supply may encourage earlier departures from the polar waters. Antarctic krill populations are predicted to be negatively impacted by climate change in the future and this could lead to a negative fitness consequence for humpback whales undertaking their long-distance migration (Tulloch et al., 2019). Grey whales (Eschrichtius robustus) on their feeding grounds exhibited overall decreased body condition over a three-year period between the beginning and end of the study (Soledade Lemos et al., 2020). An earlier departure from Antarctica may therefore be indicative of diminishing food availability on the Antarctic feeding grounds.
4.4 Drawbacks of the study
This study was strictly focused on the acoustic presence and trends of the BSD population in a migratory corridor. While it seems plausible that changes in environmental conditions of the Antarctic feeding grounds send them on their way northward (and similarly, changes in environmental conditions of their breeding grounds send them on their way southward), no concurrent observations were available of humpback whale presence (arrival and departure) on feeding or breeding grounds. Acoustic recordings on the feeding grounds could substantiate theories of earlier migration shifts beginning in Antarctica. Otherwise, the humpback whale population could still be spending the same amount of time in each region but travelling at different speeds each year on their journey between the two (Zerbini et al., 2006; Andrews-Goff et al., 2018). For instance, humpback whales stagger their migration between travel and rest in the Australian coastal waters (Jenner et al., 2001; Bejder et al., 2019)—possibly differently in different years, leading to changes in overall migration duration. From this study, it is uncertain if the humpback whales’ feeding and breeding grounds are facing a similar temporal shift in arrivals and departures as the Perth Canyon migratory corridor. An acoustic comparison with Antarctica and northwest Australia would determine whether inter-annual shifts in arrival and departure also occur on feeding and breeding grounds and ultimately enable an investigation of the distribution of the BSD population throughout the entire migratory cycle.
The long-term nature of this study had shortcomings in the early acoustic datasets. Technology constraints at the beginning of the study condensed the available recordings to be much shorter in length and temporally sporadic. For the total 15 years of passive acoustics, there were only 10 years of arrival data and seven years of departure data. Additionally, only five years had both arrival and departure data. A more complete dataset with arrival and departure recordings every year would legitimize how migration patterns may develop (Laake et al., 2012). Hence, more long-term passive acoustic data for this region can better project future shifts in migration.
In addition, monitoring at a different location within the migratory corridor, where the northward and southward migrations are temporally separated, would be beneficial and provide both arrival and departure dates for both migration lags. In the Perth Canyon, the two lags were not separable acoustically; meaning that some vocalizing humpback whales were still migrating north when others were already on their return journey, migrating south. So we missed the departures of the northward migration from the Perth Canyon, and the arrivals of the southward migration. At a different location, such as Cape Leeuwin (the whales’ first contact with Australia on their northward migration and last contact with Australia on their southward migration), we expect the two migration lags to be temporally separated, but acoustic recordings with a sampling frequency appropriate for humpback whale sounds are unavailable.
During migration, all humpback whales can vocalize non-song sounds, but males also produce song (Recalde-Salas et al., 2020). Songs are much easier to detect because they run for longer periods of time and create recognizable patterns (Payne and McVay, 1971). We may have missed individuals in the population if they were not vocalizing when travelling within range of the recorder. Moreover, some cohorts (i.e., the mother-and-calf cohort) vocalize very quietly and far less frequently than the males (Félix and Haase, 2005; Salgado Kent et al., 2012a). These particular cohorts would likely be largely missed by the Perth Canyon recorders.
4.5 Ecosystem impacts
Migration timing is important to maximize presence in suitable habitat and needs to match up with environmental variables that inform desirability (Dingle, 2014). There is evidence of some migrant species adapting their behavior with climate change to maintain temporal and spatial synchronicity (Both, 2010; Sanders and Mennill, 2014; Mayor et al., 2017). Under increasing environmental pressure, short-distance migrants are more successful at balancing opportune habitats than long-distance migrants (Jenni and Kéry, 2003; Both et al., 2006). Long-distance migrants might struggle to keep arriving at their functionally different habitats at the optimum time (i.e., when habitat features are optimum). Furthermore, if the different habitats change out-of-sync, it might become impossible to be present in each at the optimum time. Some long-distance migrant birds are compensating for such environmental mis-match by leaving breeding grounds sooner to align with the phenology of insects on their feeding grounds (Jenni and Kéry, 2003). Similarly, a shift in humpback whale migration presence in Australian waters may be an indicator of shifting parameters elsewhere. Marine mammal migrants are less studied than their terrestrial counterparts and it remains undetermined whether a shift in humpback whale migration is detrimental to the population’s survivability. However, larger shifts increase the likelihood of habitat mis-match and may negatively affect the survivability, as witnessed with other long-range migrants (Both et al., 2006; Sanders and Mennill, 2014; Mayor et al., 2017).
Studies with similar shifts in migration arrivals and environmental predictors are also finding that the rates of change in migration are not the same between migrant species that occupy the same habitat (Ramp et al., 2015). For example, the increasingly earlier humpback whale northbound migration may temporally and spatially overlap migrant species that do not usually co-exist (Ramp et al., 2015). Another migrant whale species in the Perth Canyon is the pygmy blue whale (McCauley and Jenner, 2010). Although this species is listed as data deficient on the IUCN Red List of Threatened Species, other studies on a range of marine mammals have shown that shifting migration arrivals is not limited to humpback whales (Szesciorka et al., 2020; Huang et al., 2022). Therefore, the pygmy blue whales that utilise the Perth Canyon for feeding during summer could be affected by interspecific competition, should the humpback whale presence increasingly overlap their own. The humpback whale BSD population has recovered significantly over the last few decades; however, resource competition may add another threat to the growing number of concerns for the species.
This study was able to determine the arrival and departure parameters of the BSD population, identify a significant temporal shift of humpback whale migration, and provide supporting evidence of SST predicting their presence in the Perth Canyon. This research is novel for the BSD population in determining long-term trends of their presence. Future research should further investigate the Antarctic Area IV to determine the population’s geographic distribution and the contributing extrinsic factors in initiating their migration. Similar trends on the feeding grounds may pose additional threats to the BSD population in the face of climate change.
Data availability statement
The original contributions presented in the study are included in the article/Supplementary Material. Further inquiries can be directed to the corresponding author.
Ethics statement
Ethical review and approval was not required for the animal study because the study relied entirely on archival data, which were collected passively and remotely.
Author contributions
CG, CE, and EH contributed to the design of the study. RM collected the acoustic data. The environmental data was sourced by CG and MLF. The data was analyzed by CG, CE, EH, and MLF. CG wrote the first draft of the manuscript. CE contributed to sections of the manuscript. All authors contributed to manuscript edits, read, and approved the submitted version.
Acknowledgments
Data was sourced from Australia’s Integrated Marine Observing System (IMOS), Passive Acoustic Observatories; https://imos.org.au/facilities/nationalmooringnetwork/acousticobservatories. – IMOS is enabled by the National Collaborative Research Infrastructure Strategy (NCRIS). It is operated by a consortium of institutions as an unincorporated joint venture, with the University of Tasmania as Lead Agent.
Conflict of interest
The authors declare that the research was conducted in the absence of any commercial or financial relationships that could be construed as a potential conflict of interest.
Publisher’s note
All claims expressed in this article are solely those of the authors and do not necessarily represent those of their affiliated organizations, or those of the publisher, the editors and the reviewers. Any product that may be evaluated in this article, or claim that may be made by its manufacturer, is not guaranteed or endorsed by the publisher.
Supplementary material
The Supplementary Material for this article can be found online at: https://www.frontiersin.org/articles/10.3389/fmars.2022.1086763/full#supplementary-material
References
Alerstam T., Hedenström A., Åkesson S. (2003). Long-distance migration: Evolution and determinants. Oikos 103, 247–260. doi: 10.1034/j.1600-0706.2003.12559.x
Anderson M. (2005). DISTLM v.5: a FORTRAN computer program to calculate a distance-based multivariate analysis for a linear model. 1–10 (New Zealand: Department of Statistics, University of Auckland).
Anderson M. J., Gorley R. N., Clarke R. K. (2015). PERMANOVA+ primer V7: User manual. Primer-E Ltd. Plymouth UK, 1–214. Available at: http://updates.primer-e.com/primer7/manuals/PERMANOVA+_manual.pdf.
Andrews-Goff V., Bestley S., Gales N. J., Laverick S. M., Paton D., Polanowski A. M., et al. (2018). Humpback whale migrations to Antarctic summer foraging grounds through the southwest Pacific Ocean. Sci. Rep. 8, 1–14. doi: 10.1038/s41598-018-30748-4
Avila I. C., Dormann C. F., García C., Payán L. F., Zorrilla M. X. (2020). Humpback whales extend their stay in a breeding ground in the Tropical Eastern Pacific. ICES J. Mar. Sci. 77, 109–118. doi: 10.1093/icesjms/fsz251
Balbontín J., Møller A. P., Hermosell I. G., Marzal A., Reviriego M., De Lope F. (2009). Individual responses in spring arrival date to ecological conditions during winter and migration in a migratory bird. J. Anim. Ecol. 78, 981–989. doi: 10.1111/j.1365-2656.2009.01573.x
Bauer S., Nolet B. A., Giske J., Chapman J. W., Åkesson S., Hedenström A., et al. (2011). “Cues and decision rules in animal migration,” in Milner-Gulland E. J., Fryxell J. M., Sinclair A. R. E.Animal migration: a synthesis (Oxford: Oxford University Press), 87. doi: 10.1093/acprof:oso/9780199568994.003.0006
Beazley J. (2022)Whale watching season starts early as humpback population bounces back. In: The guardian. Available at: https://www.theguardian.com/environment/2022/jun/05/whale-watching-season-starts-early-as-humpback-population-bounces-back?CMP=share_btn_tw (Accessed July 11, 2022).
Bejder L., Videsen S., Hermannsen L., Simon M., Hanf D., Madsen P. T. (2019). Low energy expenditure and resting behavior of humpback whale mother-calf pairs highlights conservation importance of sheltered breeding areas. Sci. Rep. 9, 1–11. doi: 10.1038/s41598-018-36870-7
Bestley S., Andrews-Goff V., van Wijk E., Rintoul S. R., Double M. C., How J. (2019). New insights into prime southern Ocean forage grounds for thriving Western Australian humpback whales. Sci. Rep. 9, 1–12. doi: 10.1038/s41598-019-50497-2
Both C. (2010). Flexibility of timing of avian migration to climate change masked by environmental constraints en route. Curr. Biol. 20, 243–248. doi: 10.1016/j.cub.2009.11.074
Both C., Bouwhuis S., Lessells C. M., Visser M. E. (2006). Climate change and population declines in a long-distance migratory bird. Nature 441, 81–83. doi: 10.1038/nature04539
Brown M. R., Corkeron P. J., Hale P. T., Schultz K. W., Bryden M. M. (1995). Evidence for a sex-segregated migration in the humpback whale (Megaptera novaeangliae). Proc. R. Soc B 259, 229–234. doi: 10.1098/rspb.1995.0034
Chavez-Rosales S., Palka D. L., Garrison L. P., Josephson E. A. (2019). Environmental predictors of habitat suitability and occurrence of cetaceans in the western North Atlantic Ocean. Sci. Rep. 9, 5833. doi: 10.1038/s41598-019-42288-6
Cheung W. W. L., Watson R., Pauly D. (2013). Signature of Ocean warming in global fisheries catch. Nature 497, 365–368. doi: 10.1038/nature12156
Clarke K., Gorley R. (2015). PRIMER version 7: User manual/tutorial. Primer-E Ltd. Plymouth UK, 1–296. Available at: http://updates.primer-e.com/primer7/manuals/User_manual_v7a.pdf.
Davis G. E., Baumgartner M. F., Corkeron P. J., Bell J., Berchok C., Bonnell J. M., et al. (2020). Exploring movement patterns and changing distributions of baleen whales in the western North Atlantic using a decade of passive acoustic data. Glob. Change Biol. 26, 4812–4840. doi: 10.1111/gcb.15191
Dawbin W. H. (1997). Temporal segregation of humpback whales during migration in southern hemisphere waters. Oceanogr. Lit. Rev. 1, 125–126.
Dingle H. (2014). “Seasonal migration,” in Migration: The biology of life on the move (USA: Oxford University Press), 243.
Dobson S., Becker P. H., Arnaud C. M., Bouwhuis S., Charmantier A. (2017). Plasticity results in delayed breeding in a long-distant migrant seabird. Ecol. Evol. 7, 3100–3109. doi: 10.1002/ece3.2777
Doney S. C., Ruckelshaus M., Emmett Duffy J., Barry J. P., Chan F., English C. A., et al. (2012). Climate change impacts on marine ecosystems. Ann. Rev. Mar. Sci. 4, 11–37. doi: 10.1146/annurev-marine-041911-111611
Dunlop R. A., Cato D. H., Noad M. J. (2008). Non-song acoustic communication in migrating humpback whales (Megaptera novaeangliae). Mar. Mamm. Sci. 24, 613–629. doi: 10.1111/j.1748-7692.2008.00208.x
Erbe C., Verma A., McCauley R., Gavrilov A., Parnum I. (2015). The marine soundscape of the Perth Canyon. Prog. Oceanogr. 137, 38–51. doi: 10.1016/j.pocean.2015.05.015
Félix F., Haase B. (2005). Distribution of humpback whales along the coast of Ecuador and management implications. J. Cetacean Res. Manage. 7, 21–31. Available at: https://www.researchgate.net/publication/281286526_Distribution_of_humpback_whales_along_the_coast_of_Ecuador_and_management_implications
Feng M., Waite A. M., Thompson P. A. (2009). Climate variability and Ocean production in the Leeuwin current system off the west coast of Western Australia. J. R. Soc West. Aust. 92, 67–81. Available at: https://www.rswa.org.au/publications/Journal/92(2)/ROY%20SOC%2092.2%20LEEUWIN%2067-81.pdf
Fetterer F., Knowles K., Meier W. N., Savoie M., Windnagel. A. K. (2017). Data from: Sea ice index (Boulder, Colorado USA: National Snow and Ice Data Center). doi: 10.7265/N5K072F8
Fossette S., Heide-Jørgensen M.-P., Jensen M. V., Kiszka J., Bérubé M., Bertrand N., et al. (2014). Humpback whale (Megaptera novaeangliae) post breeding dispersal and southward migration in the western Indian Ocean. J. Exp. Mar. Biol. Ecol. 450, 6–14. doi: 10.1016/j.jembe.2013.10.014
Foster S., Griffin D., Dunstan P. (2014). Twenty years of high-resolution Sea surface temperature imagery around Australia: Inter-annual and annual variability. PloS One 9, e100762. doi: 10.1371/journal.pone.0100762
Huang J. L., Leroy E. C., Truong G., Rogers T. L. (2022). Changes of Oceanic conditions drive chagos whale migration patterns in the central Indian Ocean. Front. Mar. Sci. 9. doi: 10.3389/fmars.2022.843875
Hurvich C. M., Tsai C.-L. (1991). Bias of the corrected AIC criterion for underfitted regression and time series models. Biometrika 78, 499–509. doi: 10.1093/biomet/78.3.499
IUCN-MMPATF (2021) Global dataset of important marine mammal areas (IUCN-IMMA). Available at: http://www.marinemammalhabitat.org/imma-eatlas/.
IWC (2007). Annex H Report of the Sub-committee on other southern hemisphere whale stocks. J. Cetacean Res. Manage. 9, 196–221.
Jenner K. C., Jenner M. M., McCabe K. (2001). Geographical and temporal movements of humpback whales in Western Australian waters. APPEA J. 41, 749. doi: 10.1071/aj00044
Jenni L., Kéry M. (2003). Timing of autumn bird migration under climate change: advances in long-distance mi- grants, delays in short-distance migrants. Proc. R. Soc. Lond. B. Biol. Sci. 270, 1467–1471. doi: 10.1098/rspb.2003.2394
Kawaguchi S., Ishida A., King R., Raymond B., Waller N., Constable A., et al. (2013). Risk maps for Antarctic krill under projected southern Ocean acidification. Nat. Clim. Change 3, 843–847. doi: 10.1038/nclimate1937
Laake J. L., Punt A. E., Hobbs R., Ferguson M., Rugh D., Breiwick J. (2012). Gray Whale southbound migration surveys 1967-2006: An integrated re-analysis. J. Cetacean Res. Manage. 12, 287–306. Available at: https://www.researchgate.net/publication/242650888_Gray_whale_southbound_migration_surveys_1967-2006_An_integrated_re-analysis
Matsuoka K., Hakamada T., Kiwada H., Murase H., Nishiwaki S. (2011). Abundance estimates and trends for humpback whales (Megaptera novaeangliae) in Antarctic areas IV and V based on JARPA sightings data. J. Cetacean Res. Manage., 75–94. doi: 10.47536/jcrm.vi.301
Mayor S. J., Guralnick R. P., Tingley M. W., Otegui J., Withey J. C., Elmendorf S. C., et al. (2017). Increasing phenological asynchrony between spring green-up and arrival of migratory birds. Sci. Rep. 7, 1–10. doi: 10.1038/s41598-017-02045-z
McArdle B., Anderson M. (2001). Fitting multivariate models to community data: A comment on distance-based redundancy analysis. Ecology 82, 290–297. doi: 10.2307/2680104
McCauley R., Jenner K. (2010). Migratory patterns and estimated population size of pygmy blue whales (Balaenoptera musculus brevicauda) traversing the Western Australian coast based on passive acoustics. Agadir, Morocco: Paper SC/62/SH26 presented to the IWC Scientific Committee, 9pp. Available at: https://espace.curtin.edu.au/bitstream/handle/20.500.11937/80310/80372.pdf?sequence=2&isAllowed=y.
McCauley R. D., Thomas F., Parsons M. J. G., Erbe C., Cato D. H., Duncan A. J., et al. (2017). Developing an underwater sound recorder: The long and short (Time) of it. Acoust. Aust. 45, 301–311. doi: 10.1007/s40857-017-0113-8
McCulloch M. (2016). Data from: Processed gridded ship-based bathymetry data (EM302 and EM710) from the Perth Canyon acquired during the Falkor expedition FK150301 (2015) (IEDA). doi: 10.1594/IEDA/322035
Melbourne-Thomas J., Corney S. P., Trebilco R., Meiners K. M., Stevens R. P., Kawaguchi S., et al. (2016). Under ice habitats for Antarctic krill larvae: Could less mean more under climate warming? Geophys. Res. Lett. 43, 10–322. doi: 10.1002/2016GL070846.Received
Mercado E., Perazio C. E. (2022). All units are equal in humpback whale songs, but some are more equal than others. Anim. Cogn. 25, 149–177. doi: 10.1007/s10071-021-01539-8
Meynecke J.-O., Richards R., Sahin O. (2017). Whale watch or no watch: the Australian whale watching tourism industry and climate change. Reg. Environ. Change 17, 477–488. doi: 10.1007/s10113-016-1034-z
Mintenbeck K. (2017). “Impacts of climate change on the Southern Ocean,” in Climate change impacts on fisheries and aquaculture (Hoboken, New Jersey: John Wiley & Sons, Ltd), 663–701. doi: 10.1002/9781119154051.ch20
Modest M., Irvine L., Andrews-Goff V., Gough W., Johnston D., Nowacek D., et al. (2021). First description of migratory behavior of humpback whales from an Antarctic feeding ground to a tropical calving ground. Anim. Biotelemetry 9, 1–16. doi: 10.1186/s40317-021-00266-8
Nowacek D. P., Christiansen F., Bejder L., Goldbogen J. A., Friedlaender A. S. (2016). Studying cetacean behavior: new technological approaches and conservation applications. Anim. Behav. 120, 235–244. doi: 10.1016/j.anbehav.2016.07.019
Payne R. S., McVay S. (1971). Songs of humpback whales. Science 173, 585–597. doi: 10.1126/science.173.3997.58
Prideaux M. (2003). Conserving cetaceans: the convention on migratory species and its relevant agreements for cetacean conservation (Munich, Germany: WDCS).
Ramp C., Delarue J., Palsbøll P. J., Sears R., Hammond P. S. (2015). Adapting to a warmer Ocean - seasonal shift of baleen whale movements over three decades. PloS One 10, 1–15. doi: 10.1371/journal.pone.0121374
Ratner B. (2009). The correlation coefficient: Its values range between +1/–1, or do they? J. Targeting Meas. Anal. Mark. 17, 139–142. doi: 10.1057/jt.2009.5
Recalde-Salas A., Erbe C., Salgado Kent C., Parsons M. (2020). Non-song vocalizations of humpback whales in Western Australia. Front. Mar. Sci. 7. doi: 10.3389/fmars.2020.00141
Rennie S., Hanson C. E., McCauley R. D., Pattiaratchi C., Burton C., Bannister J., et al. (2009). Physical properties and processes in the Perth Canyon, Western Australia: Links to water column production and seasonal pygmy blue whale abundance. J. Mar. Syst. 77, 21–44. doi: 10.1016/j.jmarsys.2008.11.008
Rickbeil G. J. M., Merkle J. A., Anderson G., Atwood M. P., Beckmann J. P., Cole E. K., et al. (2019). Plasticity in elk migration timing is a response to changing environmental conditions. Glob. Change Biol. 25, 2368–2381. doi: 10.1111/gcb.14629
Riekkola L., Andrews-Goff V., Friedlaender A., Zerbini A. N., Constantine R. (2020). Longer migration not necessarily the costliest strategy for migrating humpback whales. Aquat. Conserv. 30, 937–948. doi: 10.1002/aqc.3295
Salgado Kent C. P., Gavrilov A. N., Recalde-Salas A., Burton C. L. K., McCauley R. D. (2012a). “Passive acoustic monitoring of baleen whales in Geographe Bay, Western Australia,” In Acoustics 2012, Fremantle, Australian Acoustical Society Available at: https://espace.curtin.edu.au/bitstream/handle/20.500.11937/43018/42998.pdf?sequence=2.
Salgado Kent C., Jenner C., Jenner M., Bouchet P., Rexstad E. (2012b). Southern hemisphere breeding stock D humpback whale population estimates from North West Cape, Western Australia. J. Cetacean Res. Manage. 12, 29–38.
Sanders C. E., Mennill D. J. (2014). Acoustic monitoring of nocturnally migrating birds accurately assesses the timing and magnitude of migration through the great lakes. Ornithol. Appl. 116, 371–383. doi: 10.1650/CONDOR-13-098.1
Schallenberg C., Bestley S., Klocker A., Trull T. W., Davies D. M., Gault-Ringold M., et al. (2018). Sustained upwelling of subsurface iron supplies seasonally persistent phytoplankton blooms around the Southern Kerguelen Plateau, Southern Ocean. J. Geophys. Res. Oceans 123, 5986–6003. doi: 10.1029/2018JC013932
Schall E., Thomisch K., Boebel O., Gerlach G., Mangia Woods S., T. Roca I., et al. (2021). Humpback whale song recordings suggest common feeding ground occupation by multiple populations. Sci. Rep. 11, 18806. doi: 10.1038/s41598-021-98295-z
Schreiber O. W. (1952). Some sounds from marine life in the Hawaiian area. J. Acoust. Soc Am. 24, 116–116. doi: 10.1121/1.1917427
Smith J. N., Goldizen A. W., Dunlop R. A., Noad M. J. (2008). Songs of male humpback whales, Megaptera novaeangliae, are involved in intersexual interactions. Anim. Behav. 76, 467–477. doi: 10.1016/j.anbehav.2008.02.013
Soledade Lemos L., Burnett J. D., Chandler T. E., Sumich J. L., Torres L. G. (2020). Intra- and inter-annual variation in gray whale body condition on a foraging ground. Ecosphere 11, e03094. doi: 10.1002/ecs2.3094
Szesciorka A. R., Ballance L. T., Širović A., Rice A., Ohman M. D., Hildebrand J. A., et al. (2020). Timing is everything: Drivers of interannual variability in blue whale migration. Sci. Rep. 10, 1–9. doi: 10.1038/s41598-020-64855-y
Trotter J. A., Pattiaratchi C., Montagna P., Taviani M., Falter J., Thresher R., et al. (2019). First ROV exploration of the Perth Canyon: Canyon setting, faunal observations, and anthropogenic impacts. Front. Mar. Sci. 6. doi: 10.3389/fmars.2019.00173
Tsujii K., Otsuki M., Akamatsu T., Amakasu K., Kitamura M., Kikuchi T., et al. (2021). Annual variation of Oceanographic conditions changed migration timing of bowhead whales Balaena mysticetus in the southern Chukchi Sea. Polar Biol. 44, 2289–2298. doi: 10.1007/s00300-021-02960-y
Tulloch V. J. D., Plagányi ÉE., Brown C., Richardson A. J., Matear R. (2019). Future recovery of baleen whales is imperiled by climate change. Glob. Change Biol. 25, 1263–1281. doi: 10.1111/gcb.14573
Tyack P. (1981). Interactions between singing Hawaiian humpback whales and conspecifics nearby. Behav. Ecol. Sociobiol. 8, 105–116. doi: 10.1007/BF00300822
van Opzeeland I., van Parijs S., Kindermann L., Burkhardt E., Boebel O. (2013). Calling in the cold: Pervasive acoustic presence of humpback whales (Megaptera novaeangliae) in Antarctic coastal waters. PloS One 8, 1–7. doi: 10.1371/journal.pone.0073007
Winn H. E., Thompson T. J., Cummings W. C., Hain J., Hudnall J., Hays H., et al. (1981). Song of the humpback whale — population comparisons. Behav. Ecol. Sociobiol. 8, 41–46. doi: 10.1007/BF00302842
Winn H. E., Winn L. K. (1978). The song of the humpback whale Megaptera novaeangliae in the West indies. Mar. Biol. 47, 97–114. doi: 10.1007/BF00395631
Keywords: humpback whale, migration, arrival, acoustics, Australia, Antarctica
Citation: Gosby C, Erbe C, Harvey SE, Figueroa Landero MM and McCauley RD (2022) Vocalizing humpback whales (Megaptera novaeangliae) migrating from Antarctic feeding grounds arrive earlier and earlier in the Perth Canyon, Western Australia. Front. Mar. Sci. 9:1086763. doi: 10.3389/fmars.2022.1086763
Received: 01 November 2022; Accepted: 30 November 2022;
Published: 19 December 2022.
Edited by:
Jan-Olaf Meynecke, Griffith University, AustraliaReviewed by:
Peter Corkeron, New England Aquarium, United StatesDavid Ainley, H.T. Harvey & Associates, United States
Copyright © 2022 Gosby, Erbe, Harvey, Figueroa Landero and McCauley. This is an open-access article distributed under the terms of the Creative Commons Attribution License (CC BY). The use, distribution or reproduction in other forums is permitted, provided the original author(s) and the copyright owner(s) are credited and that the original publication in this journal is cited, in accordance with accepted academic practice. No use, distribution or reproduction is permitted which does not comply with these terms.
*Correspondence: Corinna Gosby, gosby.corinna@gmail.com