Seagrass Connectivity on the West Coast of Africa Supports the Hypothesis of Grazer-Mediated Seed Dispersal
- 1Center of Marine Sciences (CCMAR-CIMAR), University of Algarve, Faro, Portugal
- 2MARE – Marine and Environmental Sciences Centre, ISPA – Instituto Universitário, Lisbon, Portugal
- 3Centre for Ecology and Conservation, University of Exeter, Penryn, United Kingdom
- 4Dragões do Mar, Nova Estrela, Ilha do Príncipe, São Tomé e Príncipe
- 5Caisse de Depots et Development, Nouakchott, Mauritania & Parc Nationale du Banc d’Arguin, Chami, Mauritania
- 6Universidade Eduardo Mondlane, Department of Biological Sciences, Maputo, Mozambique
- 7Instituto da Biodiversidade e Áreas Protegidas (IBAP), Bissau, Guinea-Bissau
- 8Project ResilienSea, Grid-Arendal, ResilienSea Regional Coordination, Dakar, Senegal
- 9Instituto Nacional de Investigação das Pescas (INIP), Luanda, Angola
- 10Universidade de Cabo Verde, Faculdade de Ciências e Tecnologia, Praia, Cape Verde
- 11CIBIO-InBIO, Centro de Investigação em Biodiversidade e Recursos Genéticos, Vairão, Portugal
Population connectivity influences the distribution of genetic diversity and divergence along a species range, as the likelihood of extinction or differentiation increases in isolated populations. However, there is still poor understanding of the processes mediating inter-population dispersal in marine species that are sessile and lack planktonic life stages. One such case is the seagrass species Halodule wrightii, which produces basal seeds, although detached plants can drift. Along the tropical western coast of Africa, this species occurs in distant discontinuous habitats, raising the question of how interpopulation dispersal is mediated. The species is a key source of ecosystem functions including feeding large migratory grazers. This study aims to infer whether genetic differentiation of the seagrass H. wrightii along the western coast of Africa supports a hypothesis of predominant transportation of rafting seagrass by ocean currents, versus the hypothesis of biotic vectors of dispersal. Additional hypotheses were addressed concerning range-wide clonality and genetic diversity, assessed with microsatellite markers on populations of the west coast of Africa from Mauritania to Angola. Population genetic diversity and structure were compared with predictions from biophysical models of dispersal by oceanographic currents. The genetic data revealed low divergence among most populations, in strong contrast with predictions of very low probability of connectivity mediated by currents along the western African coastline. Moderate to high genotypic diversity showed important seed recruitment, but genetic and genotypic diversities were lower at range edges. Populations north and south of the equator were differentiated, and remarkably, so were neighboring equatorial populations despite their proximity. These results reveal independent sources of colonization of meadows in these islands, which are major habitat for migratory grazing green turtles, also supporting the hypothesis of biotically mediated seed transport. The importance of seagrass for conservation of endangered macrofauna has been widely reported; here we report evidence supporting the reciprocal role, that macrofauna protection can also plays a role in long-term survival and reproductive success of seagrass.
Introduction
Connectivity among populations plays a fundamental role in their ecology and evolution, mediating population dynamics and gene flow in metapopulation systems, and consequently the resilience of populations to environmental changes (Cowen et al., 2006; Cowen et al., 2007; Olds et al., 2016). Low connectivity causes smaller population sizes, increasing the likelihood of local extinctions but also of genetic differentiation and even speciation. In the oceans, the natural connectivity potential of marine populations at evolutionary time scales is often mediated by the oceanic transport of planktonic stages such as propagules, larvae, juveniles and fragments, but also by the active movement of adults (Palumbi, 2003; Cowen and Sponaugle, 2009). However, rafting and anthropogenic activities may also influence the dispersal of organisms over long distances (Thiel and Haye, 2006; Crook et al., 2015). Such dispersal processes are a major determinant of the ecological and evolutionary history of marine species. The marine environment appears to lack obvious obstacles for active or passive migration, suggesting that long distance dispersal is possible for many marine species. Yet, marine gene flow can be restricted by currents, thermal barriers, geographic features, and distance/time relative to the viability of dispersive stages (Goldberg and Lande, 2007). As a result, many species may exhibit genetic variability among populations at several spatial scales, revealing patterns and scales of dispersal that influence population dynamics and distributions (Kinlan and Gaines, 2003; Iacchei et al., 2013).
Dispersal distances in the marine environment may vary by orders of magnitude among or within taxa even for species that occupy similar ecological niches, such as seagrasses. Some seagrass species release their seeds in the sediment with no pelagic life stage while others produce floating propagules (Orth et al., 2006a) and consequently, genetic connectivity can differ greatly among populations (Waycott et al., 2006). There is also insufficient understanding of connectivity processes for species with very limited dispersal capacity, especially when they occupy distant habitats that they are very unlikely to reach without active transportation. Notably, there is almost no empirical evidence to understand the colonization and gene flow of oceanographically discontinuous habitats by seagrass species lacking efficient self-dispersal means, particularly for those that produce basal and negatively buoyant seeds in the genera Cymodocea and Halodule (McMahon et al., 2014). It can be hypothesized that these can only be transported by remaining attached to drifting detached seagrass shoots or by active transportation by animals, but the relative contribution of these potential dispersal mechanisms is poorly understood.
Seagrasses are marine flowering plants, most of which are distributed along shallow and wave-protected, temperate and tropical coastal waters around the world (Green et al., 2003). They provide important ecosystem services such as nursery and habitat for other organisms (e.g. fish and invertebrates), water quality improvement, sediment stabilization, productivity for food webs and carbon sequestration (Nordlund et al., 2016; Nordlund et al., 2018). However, despite their ecological importance, seagrasses are among some of the most threatened coastal ecosystems around the world (Waycott et al., 2009; Short et al., 2016). The increasing decline of seagrass meadows around the world raises the need to understand their specific reproductive and dispersal strategies that allow the connection between populations, to further implement effective conservation and restoration strategies (Orth et al., 2006b).
Seagrasses have the ability to reproduce both sexually, when genetically distinct individuals (genets) result from seeds, and asexually by clonal growth of plants that produce units capable of independent life (ramets, a plant fragment containing leaves, rhizome and roots, that derive from the breakage of the mother plant into physically independent individuals). Although seagrass species differ in producing seeds with contrasting dispersive properties (negative, neutral or positively buoyant) (Orth et al., 2007), seagrass fragments containing viable shoots can become dettached and drift away, transported by currents. These can contribute to recruitment if they eventually reattach in the sediment elsewhere (a process that is more likely in intertidal populations than in subtidal ones), or if the drifting fragments carry fruits and seeds that can disperse over suitable habitat (see Kendrick et al., 2017 for a review). Although seagrass propagules have been reported to be transported by ocean currents, tidal currents, wind, and sediment movement (Ruiz-Montoya et al., 2012; Ruiz-Montoya et al., 2015), their subsequent arrival in a suitable habitat and effective recruitment after transportation, although possible (Berković et al., 2014), is insufficiently understood. Another hypothesis for the dispersal of seagrasses is transportation by biotic vectors. Seagrass propagules can be eaten by herbivores such as green sea turtles and dugongs (e.g. Tol et al., 2017) or redhead ducks, which during winter feed exclusively on Halodule wrightii (Michot et al., 2008). Seagrass propagules can also attach to aquatic birds (e.g. Figuerola and Green, 2002), which by moving between similar suitable seagrass habitats may increase recruitment rates. Ultimately, such biophysical interactions determine connectivity pathways of seagrass populations across a wide range of spatial scales, depending on the transport mechanisms that are present, and the features of the propagule being dispersed (see McMahon et al., 2014 for a review).
Halodule wrightii Ascherson, 1868, is dioecious (i.e., separate males and females), perennial, and tolerates a wide range of environmental conditions (Bercovich et al., 2019). This species typically occupies areas associated with wave-protected coastal features isolated by unsuitable exposed habitat, across the tropical and subtropical eastern and western Atlantic coastal shores (Den Hartog and Kuo, 2007). However, it is unclear whether this distribution reflects the capacity for frequent long-distance dispersal or whether populations are fragmented within their range because their seed biology is not favourable for dispersal H. wrightii produces very persistent basal seeds that can remain dormant for years (McMillan, 1981; Orth et al., 2007) and grow at the base of shoots that are neutrally or negatively buoyant, suggesting highly restricted seed dispersal. While several studies have estimated dispersal rates for other seagrasses (Orth et al., 1994; Harwell and Orth, 2002; Verduin et al., 2002; Van Tussenbroek et al., 2009; Berković et al., 2014), to date few studies have focused on H. wrightii for estimates of seed (Darnell et al., 2015) or fragment dispersal (Hall et al., 2006). The reproductive biology of H. wrightii, which has very restricted dispersal abilities due to basal and non-buoyant seed production (Darnell et al., 2015), raises the hypothesis that population genetic isolation may be prevalent and may contribute to the rare occurrence of this seagrass along the coastlines of tropical Atlantic Africa. Among the seagrass species found in the eastern and western tropical Atlantic, H. wrightii is the only one that has colonized the eastern Atlantic (western coast of Africa) (Spalding et al., 2003), although there is insufficient phylogenetic data on whether it is indeed the same species on both sides. The absence of other tropical seagrasses of the western Atlantic on the eastern side suggest that they have not been able to disperse across the Atlantic via abiotic or biotic means. This shows that despite its apparently non-dispersive seeds, H. wrightii has an effective means to achieve long-range dispersal, even if rarely. Possible mechanisms for this could be drifting seagrass fragments carrying attached seeds, resettling fragments without seeds or animal-mediated dispersal.
The west coast of Africa, one of the world’s largest coastal systems, is an important center of marine biodiversity and marine food production, ranked among the world’s most productive coastal and offshore waters (Roberts et al., 2002), Ukwe et al., 2003). It comprises very distinct coastal zones influenced by different main currents dominated by major wind-driven ocean gyres, the North Equatorial, the Equatorial, and the South Equatorial gyres (Philander, 2001). A semi-arid coast (Mauritania and Senegal) dominated by the cold equatorward coastal current and the Canary Current off northwest Africa associated with intense coastal upwelling; Guinea-Bissau is the transition to a shallow ocean off a humid tropical coast from Guinea to Gabon dominated by a prevailing offshore current, the Guinea Current, where coastal upwelling occurs seasonally; and a subtropical coast southwards to Angola, where it encounters the temperate Benguela Current from southwest Africa and its important coastal upwelling areas (Philander, 2001; Ukwe et al., 2006; Hutchings et al., 2009).
Despite being a tropical region with high biodiversity, the western coast of Africa is one of the least studied regions for seagrasses in the world (Duarte et al., 2008). To date, there are few reports of populations of H. wrightii on the western coast of Africa: it finds its northern limit of distribution at Banc d´Arguin in Mauritania and Senegal (Cunha and Araújo, 2009), was reported in Cape Verde (Creed et al., 2016), São Tomé and Príncipe (Alexandre et al., 2017) and has its southern limit in Angola (Santos, 2007). Other seagrasses that co-occur on this coast have broader distributions reaching colder temperate regions northwards (Cymodocea nodosa and Zostera noltei) and southwards (Zostera capensis in Atlantic South Africa). Although species of Ruppia have rarely been reported in Africa (but see Martínez-Garrido et al., 2017) these are expected to occur more widely given their propensity for seed dispersal by migratory aquatic birds (e.g. Figuerola et al., 2002), so the small number of occurrence records might reflect insufficient published observations rather than real absences. The location of seagrasses on coastal ecosystems, and their vulnerability to environmental conditions, exposes seagrasses to different types of human-induced disturbances, frequently leading to habitat loss (Waycott et al., 2009). Quantifying population connectivity within coastal ecosystems is a key component of the management and conservation of seagrass populations and its ecosystem services.
In this study, we aim to estimate the structure of genetic connectivity of the tropical seagrass species H. wrightii along its eastern Atlantic distributional range on the western coast of Africa, to assess the support for the hypothesis (i) that hydrodynamic connectivity mirrors genetic structure, as expected if ocean currents are the main dispersal mechanism, versus the alternative hypothesis of biotic seed transportation dependent on large-scale migratory patterns of megaherbivores. Additionally, we also address the hypotheses that (ii) partial clonality occurs throughout this coastline, and that clonal propagation occurs at similar levels among all sampled populations and that (iii) genetic diversity is greater in the center of the species range and lower towards the range edges.
This species model is interesting due to its predicted restricted propensity for self-dispersal, and the west coast of Africa is selected because this species has few rare and distant known occurrences reported, making it important to understand to what extent they may be connected. This study fills in a major gap in the understanding of metapopulation connectivity of seagrasses along the west coast of Africa, a useful resource for conservation and management planning.
Materials and Methods
Study Area
The present study focused on the distribution range of H. wrightii along the east Atlantic coastline, where it occurs at a few scattered locations from Mauritania to Angola, including the Cape Verde and São Tomé and Príncipe Islands. This coastline coincides mostly with the biogeographic province Gulf of Guinea and its 5 eco-regions (sensu Spalding et al., 2007), of the Tropical Atlantic biogeographic realm, but also crosses a major biogeographic transition zone located around Guinea-Bissau into the Temperate Northern Atlantic realm at its Lusitanian biogeographic province where the distribution of H. wrightii extends into the Saharan upwelling ecoregion (Spalding et al., 2007; Hemingson and Bellwood, 2018). Across this distributional range, we obtained samples from eleven localities (Figure 1B) where, at each location, a variable number of shoots (spatially separated individual plants) were collected (Table 1). All sampled locations were subtidal but very shallow (up to 5 m depth). Samples collected prior to 2018 were mainly obtained from previously collected herbarium samples (Table 1) thereby resulting in distinct sample sizes (i.e., different number of individuals per location). After 2018, directed sampling of ≥20 shoots (depending on depth, visibility, cover area) was conducted haphazardly, keeping a minimum distance of approximately 1 m between each sampling unit. All plants were stored individually on silica gel drying crystals in the field or dried in herbarium sheets.
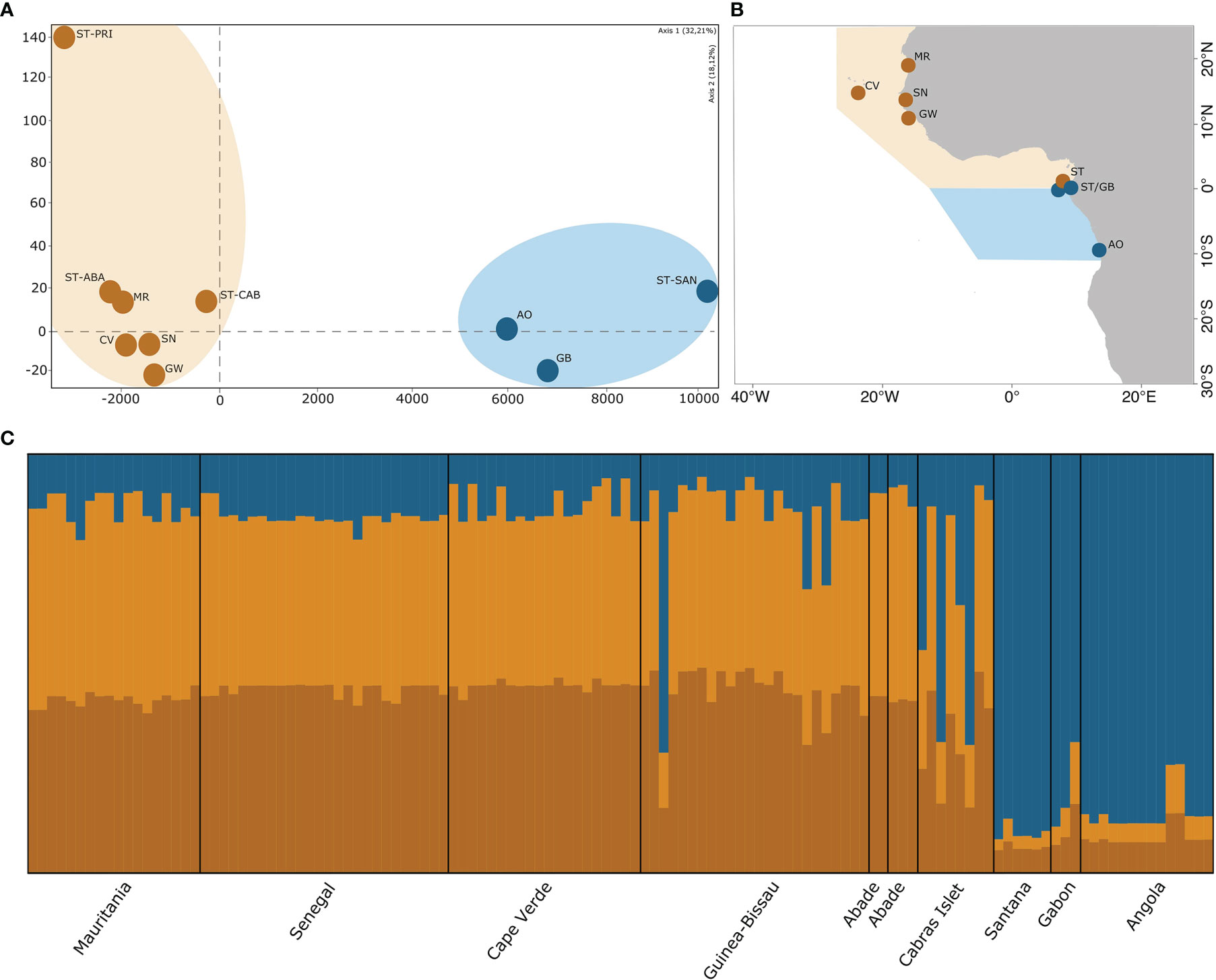
Figure 1 (A) Genetic differentiation of Halodule wrightii illustrated by factorial correspondence analysis (FCA); (B) Sampling sites of Halodule wriightii along West Africa; (C) Assignment of individuals to genetic groups that minimize Hardy-Weinberg and linkage disequilibria, estimated by STRUCTURE, K = 3.
DNA Extraction and Genotyping
Microsatellite development and primer sequences for the eight markers used can be found in Larkin et al. (2012) for loci Hw180, Hw188, Hw190, Hw196, Hw200 and Hw212; and Larkin et al. (2017) for loci Hw190b and Hw228. Genomic DNA was extracted using the NucleoSpin Plant II Kit (Macherey-Nagel, Duren, Germany) following the protocol from the supplier. Samples were genotyped for all the variable microsatellite loci divided into two triplex (HW190b; HW196; HW228) and (HW180; HW200; HW188) and one duplex (HW190; HW212) reactions using a GeneAmp ABI2720 Thermal Cycler (Applied Biosystems, Waltham, MA, USA). Final amplification volume was 15 μL, containing 1x Colorless GoTaq Flexi Buffer (Promega, Madison, WI, USA), 2 mM MgCl2 (2.5 mM), 10 μM forward and reverse primers, 0.2 mM dNTP’s, 1U GoTaq” G2 Flexi DNA Polymerase (Promega, USA) and 5 μL of template DNA (several dilutions for different populations). PCR conditions included an initial denaturation step at 95°C for 5 min, followed by 30 cycles of 95°C for 30 s, Ta for 30s, extension at 72°C for 30 s and a final extension at 72°C for 10 min. Amplified fragments were run on an ABI3130 XL automated DNA sequencer (Applied Biosystems, Waltham, MA, USA) at CCMAR, with 0.25 μL GeneScanTM 500” LIZ Size Standard (Applied Biosystems, UK) plus 9.75 μL of Hi-Di formamide, after denaturation at 95°C for 5 min. Microsatellite alleles were manually scored using STRAND (Veterinary Genetics Laboratory, University of California, Davis; http://www.vgl.ucdavis.edu/STRand), 500 LIZ as size standard (Applied Biosystems) and binned using the R package ‘MsatAllele’ (Alberto, 2009). A detailed and thorough manual revision of microsatellite amplification and scoring was conducted including positive and negative controls, PCR replications to eliminate all ambiguities, and controls testing allele amplification in more diverse (non-African) samples. The final genotyping scores were obtained for each sample after a double-check reading process, to reduce scoring errors and ensure that the peaks were accurately interpreted.
Data Analysis
To perform the analysis, populations were evaluated according to genetic clusters and close geographical areas to maximize the number of individuals per population due to low sample size in some locations. Because seagrass species can propagate asexually and the number of shoots sampled at each location might represent duplicate genotypes collected from the same clone, the package ‘RClone’ (Bailleul et al., 2016) on R 3.6.2 was used to assess clonality for all individuals at all sites. Ramets (i.e., sampling units with identical alleles) were assigned as identical multi-locus genotypes (MLGs, or genets). To check if individuals with the same MLG were truly clones, the probability of finding identical MLGs resulting from distinct sexual reproductive events (Psex) was calculated for each population (Arnaud-Haond et al., 2007). When Psex > 0.01, individuals were considered to belong to the distinct clones and included in analyses of genetic diversity. Based on number of individuals (N) and genets (G) sampled, clonal diversity in each population was calculated using the clonal richness (R) index, (R = (G − 1)/(N − 1), ranging from 0 (one single clone) to 1 (when all samples analyzed correspond to a different genet). Only unique genets per population were considered for genetic diversity analysis to avoid biasing allelic frequencies.
The software Micro-checker 2.2.3 was used to check for stuttering and null alleles (Van Oosterhout et al., 2004). Microsatellite genetic diversity per sampled site was quantified by estimating standardized mean allelic richness (Â) standardized number of private alleles (PÂ), adjusted for the sample size of 8 samples, Nei’s gene diversity (HE), using GENETIX 4.05 (Belkhir et al., 1996). Levels of population differentiation were calculated using FST and Jost’s D using an analysis of molecular variance (AMOVA) based on allele frequency under 999 permutations, using GENODIVE (Meirmans and Van Tienderen, 2004).
To estimate the distribution of genets among genetic groups that minimize Hardy-Weinberg and linkage disequilibrium, a Bayesian assignment of genotypes to K groups was made using the program STRUCTURE (Pritchard et al., 2000). The value K was estimated from the mean log-likelihood for each K value and the ΔK statistic (Earl, 2012) to identify the optimal number of groups. This was analyzed for K ranging from one to eleven with ten replicates per value and a 50,000 burn-in followed by 500,000 MCMC replicates per iteration. All jobs were run in parallel on multiple cores using the R package ‘ParallelStructure’ (Besnier and Glover, 2013). Genotypic structure was assessed with a factorial correspondence analysis (FCA) implemented with GENETIX 4.05 (Belkhir et al., 1996).
Modelling
Connectivity potential of H. wrightii was further inferred with biophysical modelling based on propagule dispersal simulations and network analyses (i.e., graph theory). The model used data from the Hybrid Coordinate Ocean Model (HYCOM), a hindcast of high-resolution three-dimensional ocean velocity fields (regular 1/12-degree horizontal grid with 40 depth layers) able to resolve key oceanographic processes (e.g., filaments, oceanic eddies, fronts and meandering currents) because it integrates effects of precipitation, wind stress, wind speed and heat flux on the final end-products about ocean currents. The hindcast data on the direction and intensity of ocean currents, when integrated with biological traits of planktonic viable time in biophysical modelling, allows to estimate connectivity. This was previously validated with demographic and generic data for macroalgae, seagrasses, limpets, mussels, fish, echinoderms and crustaceans (e.g., Assis et al., 2015; Buonomo et al., 2017; Nicastro et al., 2020).
Individual virtual particles were released on a daily basis (matching HYCOM temporal resolution) throughout a complete year from source/sink sites located 1 km apart (i.e., the spatial resolution of the simulation) where the species is known to occur. The individual particles simulate rafts of H. wrightii that strictly float on the ocean surface, and do not consider any particular shape or density of rafting fragments, as in other studies also conducted at large spatial scales (e.g., Assis et al., 2015; Buonomo et al., 2017; Nicastro et al., 2020). Occurrence records describing the distribution of the species were collated from the available literature and from biodiversity information facilities (OBIS and GBIF). The simulation included the west coast of Africa, from Angola to Mauritania (~6250 km of coastlines from -10.0° to 21.5° latitude; model domain of 15,616,841km2). A high-resolution polygon was used to define landmasses (Haklay and Weber, 2008). The model determined the position of all drifting rafts at every hour of simulation, while fitting a bilinear interpolation estimate over the velocity fields to smooth ongoing trajectories. Rafts were allowed to drift for up to 60 days (extreme propagule duration estimate). Rafts arriving at a shoreline or getting lost in the open ocean (beyond the model domain) were removed from the simulation. Trajectories were aggregated to build asymmetric matrices of pairwise probability of connectivity between source/sink sites, by dividing the number of virtual rafts released from site i that reached site j, by the total number of rafts released from site i. Interannual variability was considered by performing individual simulations per year, for the 10-year period 2008-2017. Over the entire 10-year period, the simulation released 3,653 virtual rafts per site (62,101 rafts in total).
Graph-theory was used to produce networks to visualize connectivity patterns. The connectivity matrix averaging the 10 years of individual simulations structured the graph nodes (individual source/sink sites) and the strength of edges (probability of connectivity). Stepping-stone probability estimates were determined using the graph, by using a product function over the probabilities of connectivity along the shortest paths between all pairs of sites, as found with Floyd–Warshall’s algorithm, which minimises the sum of log‐transformed probabilities. This approach aimed to capture all multigenerational potential connectivity across the study region, beyond the time frame considered in the biophysical simulations (e.g. Assis et al., 2015; Buonomo et al., 2017; Nicastro et al., 2020; Ntuli et al., 2020).
Modelling was performed in R (R Development Core Team, 2021), using the packages bigmemory, doParallel, ncdf4, gstat, gdistance and raster for the biophysical modelling and the package igraph for the network analyses.
Results
A total of 163 sampling units (seagrass shoots) were successfully genotyped with 8 microsatellite loci for 10 populations from the sampled sites along the western coast of Africa. No evidence of null alleles or linkage disequilibrium was found between the eight microsatellite loci. Genetic diversity estimates (Table 1) were low overall, but all diversity metrics were higher in the islands of São Tomé and Principe, including standardized allelic richness, gene diversity (mean expected heterozygosity, HE) and number of private alleles (i.e., endemic genetic diversity represented by alleles that are not present in any other sites sampled along the study region). When Psex values were lower than 0.01, the estimated probabilities of identical MLG were considered derived from independent reproductive events, leading to the detection of a total of 66 individual genets. Clonal richness (R), the proportion of the sampled shoots that were distinct clones arising from sexual reproduction, varied considerably among populations, with an overall value indicating a balanced contribution of seeds and clonal growth (R=0.46). The highest values of clonal richness were found in São Tomé and Príncipe and Gabon (Table 1), indicating that seed propagation is dominating in these regions. The inbreeding coefficient (FIS) was significantly different from zero in six populations (Table 1), and all values were negative (heterozygote excess), suggestive of selection favoring heterozygotes.
All population differentiation analyses revealed a separation between the southern and northern hemisphere sites, with genetically distinct populations in São Tomé and Principe (latitude zero) (Figure 1). The Structure analysis revealed three main clusters (K = 3), which were shared (although in distinct proportions) between all populations, suggesting incipient population divergence, which can be caused by either admixture or recent colonization. The proportions of genotype assignments were distinct between the southern (Angola, Gabon and Santana from São Tomé and Príncipe) versus the northern (all others) hemisphere sites (Figure 1C). The factorial correspondence analysis (FCA) also supported this result (Figure 1A) but revealed further differentiation between population sites. Similar patterns were revealed by the values of genetic differentiation indices (FST and Jost’s D) among the eleven populations, with their lack of significance likely being strongly influenced by the low sample sizes (Table S1; Supplementary Material).
The data collation from literature (Table S2; Supplementary Material) and biodiversity databases resulted in 29 locations where the species is known to occur. This translated in 17 unique source/sink sites aggregated to 1 km distance (i.e., 12 sites within distances < 1km), which were included in biophysical modelling. The aggregated trajectories produced a network showing potential connectivity only at regional scales (average distance of connectivity events: 29.124 ± 54.539km; maximum: 176.811km) (Figure 2), with low probabilities of connectivity (average probability: 0.037 ± 0.081) resulting from a low degree of connectivity events between paired sites (average number of events: 13.577± 36.628) (see Supplementary Information). In particular, the simulations indicated that the sites within São Tomé and Príncipe, Angola and Mauritania were expected to be connected by oceanographic current transportation (Figure 2). The remaining sites had no probability of connectivity mediated by ocean currents (i.e., there were no events of connectivity from/to these sites, in the 10 years of simulation).
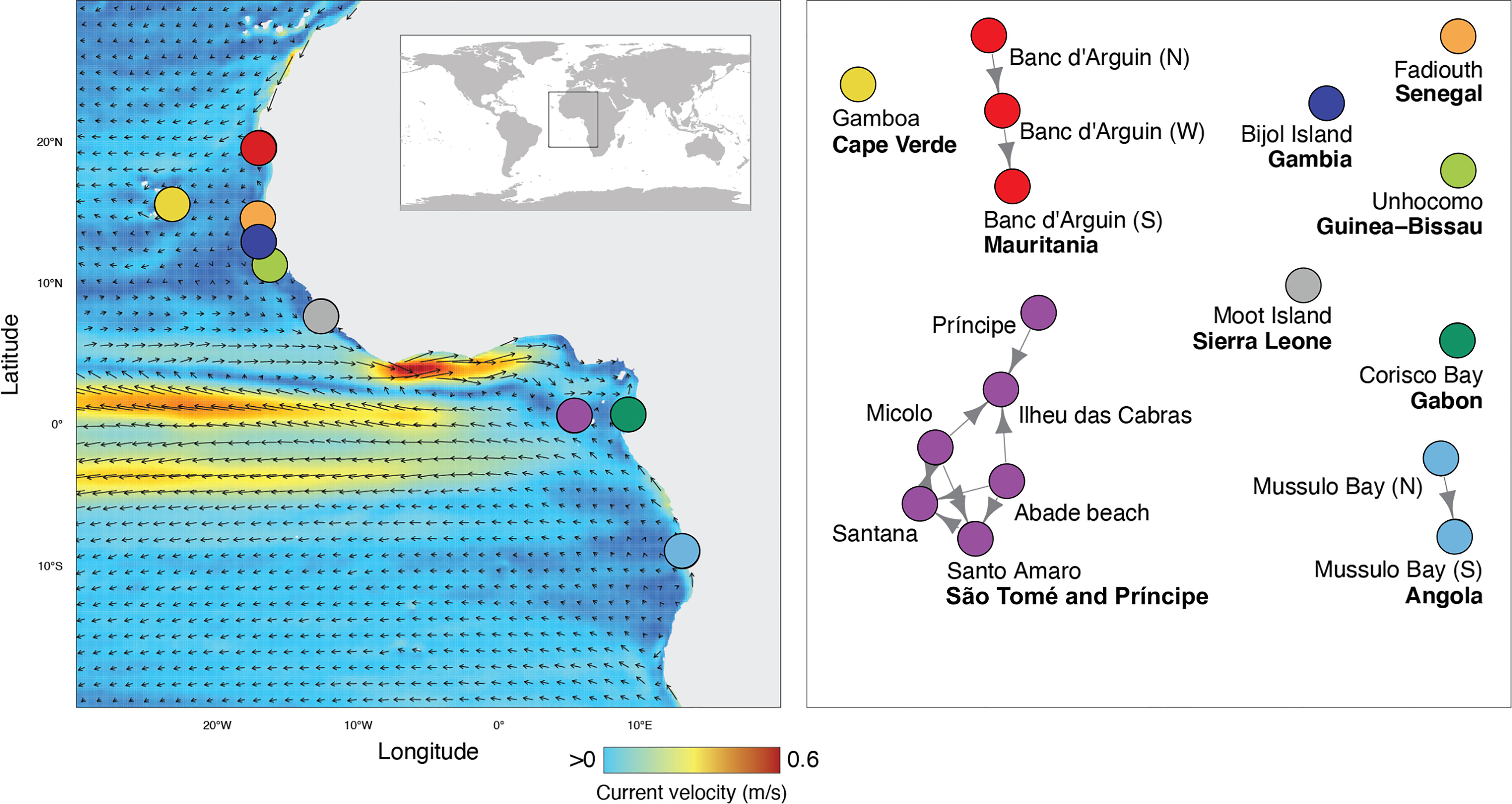
Figure 2 Potential connectivity of Halodule wrightii populations in west Africa, estimated from simulations of transport by ocean currents data (10 years). Circles in the left panel depict all regions where the species is known to occur. Colors of circles in the right panel represent distinct clusters, i.e., sites that are potentially connected by ocean currents. For estimates of probability of connectivity between regions see Supplementary Information, Table S3.
Discussion
The spatial patterns of genetic structure here found for the seagrass H. wrightii in its Tropical Eastern Atlantic range (~6250 km), along the western coast of Africa, did not support our main hypothesis (i) of ocean currents as main dispersal vectors mediating population connectivity. Simulated transportation of drifting seagrass fragments by ocean currents revealed extremely low or null probability of gene flow between all populations along this coast, with a few exceptions for nearby sites. The genetic results, in strong contrast, showed no significant population differentiation besides a broad genetic separation north and south of the equator, while on the equator line (São Tomé and Príncipe) populations were genetically distinct despite close proximity, indicating they originated from independent colonization events by seeds from genetically differentiated sources. This mismatch between the observed genetic structure and the predicted connectivity patterns expected if dispersal was based on ocean currents, suggests that biotic transportation (e.g., seed transport by megaherbivores) may play a larger role in the dispersal and establishment of the species rather than ocean currents, although further studies are needed to investigate this hypothesis.
The moderate or high genotypic (clonal) diversity here discovered across all populations (hypothesis ii) demonstrates successful sexual (seed-based) recruitment, rather than simple clonal propagation, in all populations. However, genotypic (clonal) and genetic (allelic) diversity were not similar along the range (hypothesis iii). Sexual (seed) recruitment and genetic diversity were maximum in the equator (São Tomé and Principe), whereas clonal propagation was more prevalent at both the northern and especially at the southern range of the species, which may reflect either lower seed production and dispersal or lower seed germination and seedling survival, at the range edges. The low genetic (allelic) diversity found across all populations, is compatible with a hypotheses of recent founder events/demographic bottlenecks.
This study also compiled all the distribution records and added new records to fill in the gaps regarding the distribution of H. wrightii species in this region and underscores the need to understand the metapopulation dynamics to further implement conservation measures.
Genetic Structure and Oceanographic Connectivity
Population genetic differentiation was low and non-significant across all populations, suggesting high inter-population connectivity, yet H. wrightii is thought to have low dispersal capability due to basal non-buoyant seeds. Long-distance migration by this species can be mediated either by floating drifting fragments or by animal-mediated transport (entangled or in the digestive tract). Our results do not support the hypothesis that connectivity is predominantly mediated by oceanographic currents, as genetic and hydrodynamic connectivity show very distinct patterns. Although H. wrightii fragments can survive floating in the water column for up to four weeks (Hall et al., 2006), our results indicate that on average, such dispersal by drifting fragments, would not travel far from their source location (Figure 2). Although our oceanographic simulations predict that H. wrightii fragments might still be able to disperse successfully over short distances between nearby populations, this was contradicted by the genetic differentiation of nearby populations in the island of São Tomé.
We cannot rule out the hypothesis of oceanographic transportation playing a role in dispersal given the extremely low variability of the markers in this geographic region, that results in very low power, only capable of detecting genetic differentiation of high magnitude. Besides, stepping-stone connectivity could be higher than predicted if unknown seagrass meadows might exist in between the assessed sites. However, the poor connectivity potential revealed by passive transport simulations among H. wrightii populations at such large scales of distribution indicates that additional factors, such as grazer transportation, might be involved in mediating migratory movements in this species. Remarkably, the islands of São Tomé and Principe contain populations located nearby but assigned to distinct northern and southern groups, further suggesting that stochastic rare colonization events might be at the origin of the colonization of these seagrass populations, as these bear little or no correlation with geographical distance or oceanographic distance (i.e., current transport). Multiple independent colonization events on islands were previously reported for coral species: in São Tomé and Principe from Brazil and from the Caribbean (Teschima et al., 2021), and numerous colonization events to the Canary Islands and Cape Verde Islands (de Souza et al., 2017), although such corals have planktonic life stages. The bio-physical modelling considered key ecological traits (e.g., rafting duration; Assis et al., 2021) and oceanographic processes (e.g., eddies and fronts; Manel et al., 2019) to realistically estimate connectivity, but one cannot neglect the effect of additional drivers like distinct raft morphology changing the effect of wind drag (‘windage’) on their exposed surface (Ruiz-Montoya et al., 2015); however, such incorporation into modelling is challenging.
Multiple factors may interact to drive seagrass migrations. Other seagrass species were reported to rely on multiple means of transportation such as water and sediment movements (Kendrick et al., 2012; Darnell et al., 2015), drifting (Berković et al., 2014) or biotic vectors such as herbivores. Dugongs, manatees, sea turtles, birds or fishes that feed and live among seagrass meadows, can transport seeds through their consumption or transport fragments attached to them (see Heck and Valentine, 2006 for a review). Previous studies have pinpointed the importance of biotic vectors in the dispersal of seagrasses. Zostera marina seeds can be dispersed by up to 1.5 km by sea turtles and 19.5 km by birds (Sumoski and Orth, 2012). Other studies have found viable seagrass seeds in megaherbivores feces, suggesting the potential for green sea turtles Chelonia mydas (277 – 652 km) and dugongs Dugong dugon (173 – 234 km) to act as seed migration agents (Tol et al., 2017). Adding to the potential dispersal distance, it is also important to consider the digestion retention time for these herbivores, with reports for dugongs ranging between 6 – 7 days (Lanyon and Marsh, 1995; Tol et al., 2017) and one to two weeks for green sea turtles (Brand et al., 1999; Mannina N. B., personal communication). Satellite tracking data from post-breeding green sea turtles in our study region indicate that these animals can travel ~ 400 km between H. wrightii sites in about 10 days (8 – 11 days, n=4, Patrício A. R. & Catry P., unpublished data). This vector could therefore facilitate the dispersal of viable seeds over such wide distances. Our results support the hypothesis that the broad distribution of H. wrightii along the western coast of Africa could be mediated by biotic vectors, although to date, no study has addressed this subject for this species. Overall, animals that feed on seagrass meadows on the west coast of Africa, such as dugongs or green sea turtles (Marshall et al., 2003; Godley et al., 2010; Hancock et al., 2019) seem to be the most likely candidates for seagrass seed dispersal, although wading birds could also play an important role, and indeed might be able to transport seeds rapidly over greater distances. On the western side of the Atlantic, one particular species of duck (Aythya americana) actually feeds on H. wrightii exclusively for a large part of the year (Michot et al., 2008), but no equivalent trophic behavior has been reported for any bird species on the African coastline. In addition to the hypothesis of biotic transport as main means for seed propagation by H. wrightii, we also hypothesize that the very large population of green turtles that migrate along this coast (Catry et al., 2002), one of the largest in the world, might be the main dispersal vectors for H. wrightii. Our study raises interesting questions that still require further research to assess the true potential of seagrass seed dispersal via animal vectors along the western coast of Africa.
Genotypic Diversity
Clonal reproduction is common among seagrass species, allowing long-term persistence of individual genotypes (e.g. Arnaud-Haond et al., 2012; Arnaud-Haond et al., 2020). In dioecious species like H. wrightii maintaining genotypically diverse populations is also important to increase the probability of mating encounters, for successful sexual reproduction to occur. Monoclonal dioecious seagrass populations do exist and may persist for millennia (e.g. Alberto et al., 2008), but evidently only by clonal propagation, as a single male or single female cannot form sexual seeds. The proportion of distinct sexually originated (seed-derived) clones in a population (i.e., genotypic richness) is a measure of the long-term balance between sexual and asexual reproduction. Genotypic richness, in addition to genetic diversity, can also play a major role of seagrass ecosystems resilience to maintain vital functions (Massa et al., 2013). Clonal richness of H. wrightii along the western coast of Africa was generally high (mean R=0.46), particularly higher than on the western side of the Atlantic for the same species (mean R=0.34; Larkin et al., 2017), revealing a high contribution of seed recruitment for population maintenance along the west coast of Africa. Our results also revealed that clonal richness was variable among sites, indicating that sexual recruitment from seeds was less relevant and/or successful in some populations, particularly in Angola (R= 0.08, Fis= -0.69), where the lowest R value was observed, and Senegal (R= 0.24, Fis = -0.69), two populations located near the southern and northern range edges of the species. Overall, these results reject our initial hypothesizes of similar observed clonality across the species range, raising the hypothesis of geographical parthenogenesis, i.e., higher clonal propagation in range-edge populations as these occupy marginal habitats (Eckert, 2002; Tilquin and Kokko, 2016). Furthermore, clonal diversity was higher in central populations, showing that sexual reproduction is favored. However, due to the small number of individual samples for some of the central populations, these results should be considered carefully (Table 1). Future studies with more shoot sampling per population could indicate whether sexual reproduction in central populations is indeed higher than in peripheral populations. Seagrasses in general have very variable levels of clonal richness across distinct populations of the same species (e.g. Arnaud-Haond et al., 2007; Alberto et al., 2008; Boyé et al., 2021), and this is therefore not a species-specific trait. However, depending on the levels of genotypic diversity and the number of disturbance events within a population, seagrass recovery can occur through either sexual or asexual reproduction when habitats are disturbed (Becheler et al., 2010; Jarvis et al., 2012). When seed recruitment is frequent, disturbance is predicted to increase and maintain high genotypic variety (Becheler et al., 2010; Becheler et al., 2014; Paulo et al., 2019; Arnaud-Haond et al., 2020), because disturbance increases space available thereby reducing competition, which favors seedling recruitment. In contrast, populations with low genotypic diversity are more likely to recover from disturbances through asexual reproduction of established genotypes. This reproductive plasticity may enhance the resistance and resilience of seagrass species to habitat and environmental changes. Future research for H. wrightii in West Africa should consider a better sampling design, particularly in central populations such as São Tomé and Principe, to increase the number of sampling units to test the hypothesis of whether clonal or sexual reproduction might vary over different geographical scales and whether asexual reproduction is more prevalent towards to the species range edge.
Genetic Diversity
The low genetic diversity found for populations of H. wrightii in western Africa (mean Â=1.59) is distinct from H. wrightii on the western Atlantic (4.08 in Larkin et al., 2017; 3.26 in Reynolds et al., 2019), and thus it is not a species-specific trait. This could be caused by diversity loss to population bottlenecks or to founder events, as might be expected if colonization of these regions was recent. Although the overall low allelic richness of H. wrightii on the west coast of Africa was comparable to mean levels observed in many other seagrasses around the world (1.44 in Jahnke et al., 2019; 1.56 in Nguyen et al., 2014; 1.62 in Tanaka et al., 2011), these levels rank among the lowest in seagrasses (2.16 in Arriesgado et al., 2015; 4.78 in Becheler et al., 2010; 3.25 in Olsen et al., 2004; 5.60 in van Dijk et al., 2018).This pattern supports the hypothesis of a recent colonization history in the eastern side of the Atlantic with founder effects, a scenario that is supported by the observation that this is the only taxon that occurs on both sides of the tropical Atlantic. Most Atlantic tropical seagrass species occur exclusively on the western side of the Atlantic, suggesting that this oceanic migration route must be a very rare event for seagrasses. The lower allelic richness in near marginal populations such as Senegal or Angola was consistent with our hypothesis of lower genetic diversity towards the range edges, as shown for other species (e.g., Arnaud-Haond et al., 2006; Ridgway et al., 2008; Arriesgado et al., 2015).
The results of this study have important conservation implications, particularly as extremely productive and biologically diverse coastal ecosystems of the west coast of Africa have been under increasing stress due to natural and human-induced pressures (Polidoro et al., 2017). The low genetic variation in H. wrightii populations, together with the small number of known populations and their restricted habitats, raises concerns for their conservation and ability to deal with environmental and human mediated impacts, since genetic diversity is the basis for adaptation to future environments (Lande and Shannon, 1996). Seagrasses provide essential habitat for other species and ecosystem services, and their loss is magnified into a loss of multiple ecological functions. Protecting existing seagrass meadows in this region and restoring them where suitable habitats exist, may be vital to prevent loss of existing genetic diversity (Duval-Diop et al., 2014). However, our study suggests that this diversity might also depend on protecting the herbivores that potentially mediate their successful gene flow and colonization of new habitats. The results of this study support the hypothesis that the very large population of green turtles that migrate along this coast might be a main dispersal vector. It is widely accepted that seagrasses must be protected for the survival and persistence of their associated macrofauna; our results suggest that conversely, macrofauna such as green turtles, may also play an important role in long-term seagrass persistence and reproductive success along the tropical western coast of Africa.
Data Availability Statement
The datasets presented in this study can be found in online repositories. The names of the repository/repositories and accession number(s) can be found below: https://figshare.com/, doi.org/10.6084/m9.figshare.14664930.v2.
Author Contributions
The study was conceived and designed by ES, AT, JA, and GP. Laboratory genotyping work was carried out by AT. The genetic data were analyzed by MAT and the biophysical modelling was performed by JA. The manuscript was written by MAT, ES, and JA, with all authors contributing to drafts. All authors contributed to the article and approved the submitted version.
Funding
This work was funded by Portuguese National Funds from FCT - Foundation for Science and Technology, through UIDB/04326/2020, UIDP/04326/2020, LA/P/0101/2020, PTDC/BIA-CBI/6515/2020, and fellowships SFRH/BD/138182/2018, SFRH/BSAB/150485/2019, the transitional norm - DL57/2016/CP1361/CT0035, project MARAFRICA: AGA-KHAN/540316524/2019, and by a Pew Marine Fellowship to EAS. Samples were collected under license and logistic support of the local authorities at each country. The sampling collections were supported by projects LuandaWaterFront AGKN -FCT-333191101, by the MAVA Foundation projects ResilienSEA, "Consolidation of sea turtle conservation at the Bijagós, Guinea-Bissau" granted to the Instituto da Biodiversidade e das Áreas Protegidas da Guiné-Bissau (IBAP), "PNBA-site emblématique pour les tortues" granted to the National Park of the Banc d'Arguin, project "Survie des Tortues Marines” PRCM/STM POOOA4/OA9 by PRCM (Partenariat Régional pour la Conservation de la zone côtière et Marine en Afrique de l'Ouest) , EU-H20202 854248 (Tropibio), EU-BiodivERsA program, Programa de Pós-Graduação em Ciência para o Desenvolvimento (Fundação Calouste Gulbenkian) and by the Scientific Council of the National Park of the Banc d'Arguin (funded by BACOMAB).
Conflict of Interest
The authors declare that the research was conducted in the absence of any commercial or financial relationships that could be construed as a potential conflict of interest.
Publisher’s Note
All claims expressed in this article are solely those of the authors and do not necessarily represent those of their affiliated organizations, or those of the publisher, the editors and the reviewers. Any product that may be evaluated in this article, or claim that may be made by its manufacturer, is not guaranteed or endorsed by the publisher.
Acknowledgments
We thank Paulo Catry for originating this initiative to study population genetics of Halodule along the western coast of Africa. We are grateful to all who helped collect samples in the field such as Matthijs Van Der Geest, Guillem Roca, Aschwin Engelen, Paulo Catry, Ana Alexandre, João Silva and Peter Wirtz.
Supplementary Material
The Supplementary Material for this article can be found online at: https://www.frontiersin.org/articles/10.3389/fmars.2022.809721/full#supplementary-material
References
Alberto F. (2009). MsatAllele_1. 0: An R Package to Visualize the Binning of Microsatellite Alleles. J. Hered. 100, 394–397. doi: 10.1093/jhered/esn110
Alberto F., Massa S., Manent P., Diaz-Almela E., Arnaud-Haond S., Duarte C. M., et al. (2008). Genetic Differentiation and Secondary Contact Zone in the Seagrass Cymodocea Nodosa Across the Mediterranean–Atlantic Transition Region. J. Biogeogr. 35, 1279–1294. doi: 10.1111/j.1365-2699.2007.01876.x
Alexandre A., Silva J., Ferreira R., Paulo D., Serrão E. A., Santos R. (2017). First Description of Seagrass Distribution and Abundance in São Tomé and Príncipe. Aquat. Bot. 142, 48–52. doi: 10.1016/j.aquabot.2017.06.008
Arnaud-Haond S., Duarte C. M., Alberto F., Serrao E. A. (2007). Standardizing Methods to Address Clonality in Population Studies. Mol. Ecol. 16, 5115–5139. doi: 10.1111/j.1365-294X.2007.03535
Arnaud-Haond S., Duarte C. M., Diaz-Almela E., Marbà N., Sintes T., Serrão E. A. (2012). Implications of Extreme Life Span in Clonal Organisms: Millenary Clones in Meadows of the Threatened Seagrass Posidonia Oceanica. PloS One 7, e30454. doi: 10.1371/journal.pone.0030454
Arnaud-Haond S., Stoeckel S., Bailleul D. (2020). New Insights Into the Population Genetics of Partially Clonal Organisms: When Seagrass Data Meet Theoretical Expectations. Mol. Ecol. 29 (17), 3248–3260. doi: 10.1111/mec.15532
Arnaud-Haond S., Teixeira S., Massa S. I., Billot C., Saenger P., Coupland G., et al. (2006). Genetic Structure at Range Edge: Low Diversity and High Inbreeding in Southeast Asian Mangrove (Avicennia Marina) Populations. Mol. Ecol. 15, 3515–3525. doi: 10.1111/j.1365-294X.2006.02997.x
Arriesgado D. M., Kurokochi H., Nakajima Y., Matsuki Y., Uy W. H., Fortes M. D., et al. (2015). Genetic Diversity and Structure of the Tropical Seagrass Cymodocea Serrulata Spanning Its Central Diversity Hotspot and Range Edge. Aquat. Ecol. 49, 357–372. doi: 10.1007/s10452-015-9529-0
Assis J., Fragkopoulou E., Serrão E. A., e Costa B. H., Gandra M., Abecasis D. (2021). Weak Biodiversity Connectivity in the European Network of No-Take Marine Protected Areas. Sci. Total Environ. 773, 145664. doi: 10.1016/j.scitotenv.2021.145664
Assis J., Zupan M., Nicastro K. R., Zardi G. I., McQuaid C. D., Serrao E. A. (2015). Oceanographic Conditions Limit the Spread of a Marine Invader Along Southern African Shores. PloS One 10 (6), e0128124. doi: 10.1371/journal.pone.0128124
Bailleul D., Stoeckel S., Arnaud-Haond S. (2016). RClone: A Package to Identify MultiLocus Clonal Lineages and Handle Clonal Data Sets in R. Methods Ecol. Evol. 7, 966–970. doi: 10.1111/2041-210X.12550
Becheler R., Benkara E., Moalic Y., Hily C., Arnaud-Haond S. (2014). Scaling of Processes Shaping the Clonal Dynamics and Genetic Mosaic of Seagrasses Through Temporal Genetic Monitoring. Heredity 112 (2), 114–121. doi: 10.1038/hdy.2013.82
Becheler R., Diekmann O., Hily C., Moalic Y., Arnaud-Haond S. (2010). The Concept of Population in Clonal Organisms: Mosaics of Temporally Colonized Patches are Forming Highly Diverse Meadows of Zostera Marina in Brittany. Mol. Ecol. 19 (12), 2394–2407. doi: 10.1111/j.1365-294X.2010.04649.x
Belkhir K., Borsa P., Chikhi L., Raufaste N., Bonhomme F. (1996). GENETIX 4.05, Logiciel Sous Windows TM Pour La Génétique Des Populations.Laboratoire Génome, Populations, Interactions, CNRS UMR 5171 (Montpellier (France): Université de Montpellier II).
Bercovich V. M., Schubert N., Almeida Saá A. C., Silva J., Horta P. A. (2019). Multi-Level Phenotypic Plasticity and the Persistence of Seagrasses Along Environmental Gradients in a Subtropical Lagoon. Aquat. Bot. 157, 24–32. doi: 10.1016/j.aquabot.2019.06.003
Berković B., Cabaco S., Barrio J. M., Santos R., Serrão E. A., Alberto F. (2014). Extending the Life History of a Clonal Aquatic Plant: Dispersal Potential of Sexual and Asexual Propagules of Zostera Noltii. Aquat. Bot. 113, 123–129. doi: 10.1016/j.aquabot.2013.10.007
Besnier F., Glover K. A. (2013). ParallelStructure: A R Package to Distribute Parallel Runs of the Population Genetics Program STRUCTURE on Multi-Core Computers. PloS One 8 (7), e70651. doi: 10.1371/journal.pone.0070651
Boyé A., Gauthier O., Becheler R., Le Garrec V., Hily C., Maguer M., et al. (2021). Drivers and Limits of Phenotypic Responses in Vulnerable Seagrass Populations: Zostera Marina in the Intertidal. J. Ecol. 110, 144–161. doi: 10.1111/1365-2745.1379
Brand S. J., Lanyon J. M., Limpus C. J. (1999). Digesta Composition and Retention Times in Wild Immature Green Turtles, Chelonia Mydas: A Preliminary Investigation. Mar. Freshw. Res. 50, 145–147. doi: 10.1071/MF98033
Buonomo R., Assis J., Fernandes F., Engelen A. H., Airoldi L., Serrão E. A. (2017). Habitat Continuity and Stepping-Stone Oceanographic Distances Explain Population Genetic Connectivity of the Brown Alga Cystoseira Amentacea. Mol. Ecol. 26, 766–780. doi: 10.1111/mec.13960
Catry P., Barbosa C., Indjai B., Almeida A., Godley B. J., Vie J.-C. (2002). First Census of the Green Turtle at Poilão, Bijagós Archipelago, Guinea-Bissau: The Most Important Nesting Colony on the Atlantic Coast of Africa. Oryx 36, 400–403. doi: 10.1017/S0030605302000765
Cowen R. K., Gawarkiewicz G., Pineda J., Thorrold S. R., Werner F. E. (2007). Population Connectivity in Marine Systems an Overview. Oceanography 20, 14–21. doi: 10.5670/oceanog.2007.26
Cowen R. K., Paris C. B., Srinivasan A. (2006). Scaling of Connectivity in Marine Populations. Science 311, 522–527. doi: 10.1126/science.1122039
Cowen R. K., Sponaugle S. (2009). Larval Dispersal and Marine Population Connectivity. Ann. Rev. Mar. Sci. 1, 443–466. doi: 10.1146/annurev.marine.010908.163757
Creed J. C., Engelen A. H., Bandeira S., Serrão E. A. (2016). First Record of Seagrass in Cape Verde, Eastern Atlantic. Mar. Biodivers. Rec. 9, 57. doi: 10.1186/s41200-016-0067-9
Crook D. A., Lowe W. H., Allendorf F. W., Erős T., Finn D. S., Gillanders B. M., et al. (2015). Human Effects on Ecological Connectivity in Aquatic Ecosystems: Integrating Scientific Approaches to Support Management and Mitigation. Sci. Total Environ. 534, 52–64. doi: 10.1016/j.scitotenv.2015.04.034
Cunha A. H., Araújo A. (2009). New Distribution Limits of Seagrass Beds in West Africa. J. Biogeogr. 36, 1621–1622. doi: 10.1111/j.1365-2699.2009.02135.x
Darnell K. M., Booth D. M., Koch E. W., Dunton K. H. (2015). And Dunton, KThe Interactive Effects of Water Flow and Reproductive Strategies on Seed and Seedling Dispersal Along the Substrate in Two Sub-Tropical Seagrass Species. J. Exp. Mar. Bio. Ecol. 471, 30–40. doi: 10.1016/j.jembe.2015.05.006
Den Hartog C., Kuo J. (2007). “Taxonomy and Biogeography of Seagrasses,” in Seagrasses: Biology, Ecologyand Conservation (Amsterdam: Springer), 1–24. doi: 10.1007/978-1-4020-2983-7_1
de Souza J. N., Nunes F. L. D., Zilberberg C., Sanchez J. A., Migotto A. E., Hoeksema B. W., et al. (2017). Contrasting Patterns of Connectivity Among Endemic and Widespread Fire Coral Species (Millepora Spp.) in the Tropical Southwestern Atlantic. Coral Reefs 36, 701–716. doi: 10.1007/s00338-017-1562-0
Duarte C. M., Borum J., Short F. T., Walker D. I. (2008). Seagrass Ecosystems: Their Global Status and Prospects. Aquat. Ecosyst. Trends Glob. Prospect., 281–294. doi: 10.1017/CBO9780511751790.025
Duval-Diop D., Senhoury A., Campredon P. (2014). “Governing Through Networks: Working Toward a Sustainable Management of West Africa’s Coastal Mangrove Ecosystems,” in The Land/Ocean Interactions in the Coastal Zone of West and Central Africa (Cham, Switzerland: Springer), 191–206. doi: 10.1007/978-3-319-06388-1_16
Earl D. A. (2012). STRUCTURE HARVESTER: A Website and Program for Visualizing STRUCTURE Output and Implementing the Evanno Method. Conserv. Genet. Resour. 4, 359–361. doi: 10.1007/s12686-011-9548-7
Eckert C. G. (2002). “The Loss of Sex in Clonal Plants,” in Ecology and Evolutionary Biology of Clonal Plants (Dordrecht: Springer), 279–298. doi: 10.1007/978-94-017-1345-0_15
Figuerola J., Green A. J. (2002). Dispersal of Aquatic Organisms by Waterbirds: A Review of Past Research and Priorities for Future Studies. Freshw. Biol. 47, 483–494. doi: 10.1046/j.1365-2427.2002.00829.x
Figuerola J., Green A. J., Santamaría L. (2002). Comparative Dispersal Effectiveness of Wigeongrass Seeds by Waterfowl Wintering in South-West Spain: Quantitative and Qualitative Aspects. J. Ecol. 90, 989–1001. doi: 10.1046/j.1365-2745.2002.00734.x
Godley B. J., Barbosa C., Bruford M., Broderick A. C., Catry P., Coyne M. S., et al. (2010). Unravelling Migratory Connectivity in Marine Turtles Using Multiple Methods. J. Appl. Ecol. 47, 769–778. doi: 10.1111/j.1365-2664.2010.01817.x
Goldberg E. E., Lande R. (2007). Species’ Borders and Dispersal Barriers. Am. Nat. 170, 297–304. doi: 10.1086/518946
Green E. P., Short F. T., Frederick T. (2003). World Atlas of Seagrasses (Berkeley: Univ of California Press).
Haklay M., Weber P. (2008). Openstreetmap: User-Generated Street Maps. IEEE Pervasive Comput. 7, 12–18. doi: 10.1109/MPRV.2008.80
Hall L. M., Hanisak M. D., Virnstein R. W. (2006). Fragments of the Seagrasses Halodule Wrightii and Halophila Johnsonii as Potential Recruits in Indian River Lagoon, Florida. Mar. Ecol. Prog. Ser. 310, 109–117. doi: 10.3354/meps310109
Hancock J. M., Vieira S., Taraveira L., Santos A., Schmitt V., Semedo A., et al. (2019). Genetic Characterization of Green Turtles (Chelonia Mydas) From São Tomé and Príncipe: Insights on Species Recruitment and Dispersal in the Gulf of Guinea. J. Exp. Mar. Bio. Ecol. 518, 151181. doi: 10.1016/j.jembe.2019.151181
Harwell M. C., Orth R. J. (2002). Long-Distance Dispersal Potential in a Marine Macrophyte. Ecology 83, 3319–3330. doi: 10.1890/0012-9658(2002)083[3319:LDDPIA]2.0.CO;2
Heck K. L., Valentine J. F. (2006). Plant-Herbivore Interactions in Seagrass Meadows. J. Exp. Mar. Bio. Ecol. 330, 420–436. doi: 10.1016/j.jembe.2005.12.044
Hemingson C. R., Bellwood D. R. (2018). Biogeographic Patterns in Major Marine Realms: Function Not Taxonomy Unites Fish Assemblages in Reef, Seagrass and Mangrove Systems. Ecography (Cop.). 41, 174–182. doi: 10.1111/ecog.03010
Hutchings L., van der Lingen C. D., Shannon L. J., Crawford R. J. M., Verheye H. M. S., Bartholomae C. H., et al. (2009). The Benguela Current: An Ecosystem of Four Components. Prog. Oceanogr. 83, 15–32. doi: 10.1016/j.pocean.2009.07.046
Iacchei M., Ben-Horin T., Selkoe K. A., Bird C. E., García-Rodríguez F. J., Toonen R. J. (2013). Combined Analyses of Kinship and FST Suggest Potential Drivers of Chaotic Genetic Patchiness in High Gene-Flow Populations. Mol. Ecol. 22, 3476–3494. doi: 10.1111/mec.12341
Jahnke M., Gullström M., Larsson J., Asplund M. E., Mgeleka S., Silas M. O., et al. (2019). Population Genetic Structure and Connectivity of the Seagrass Thalassia Hemprichii in the Western Indian Ocean is Influenced by Predominant Ocean Currents. Ecol. Evol. 9, 8953–8964. doi: 10.5061/dryad.0hn97r5
Jarvis J. C., Moore K. A., Kenworthy W. J. (2012). Characterization and Ecological Implication of Eelgrass Life History Strategies Near the Species’ Southern Limit in the Western North Atlantic. Mar. Ecol. Prog. Ser. 444, 43–56. doi: 10.3354/meps09428
Kendrick G. A., Orth R. J., Statton J., Hovey R., Montoya L. R., Lowe R. J., et al. (2017). Demographic and Genetic Connectivity: The Role and Consequences of Reproduction, Dispersal and Recruitment in Seagrasses. Biol. Rev. 92, 921–938. doi: 10.1111/brv.12261
Kendrick G. A., Waycott M., Carruthers T. J. B., Cambridge M. L., Hovey R., Krauss S. L., et al. (2012). The Central Role of Dispersal in the Maintenance and Persistence of Seagrass Populations. Bioscience 62, 56–65. doi: 10.1525/bio.2012.62.1.10
Kinlan B. P., Gaines S. D. (2003). Propagule Dispersal in Marine and Terrestrial Environments: A Community Perspective. Ecology 84, 2007–2020. doi: 10.1890/01-0622
Lande R., Shannon S. (1996). The Role of Genetic Variation in Adaptation and Population Persistence in a Changing Environment. Evolution 50 (NY), 434–437. doi: 10.2307/2410812
Lanyon J. M., Marsh H. (1995). Digesta Passage Times in the Dugong. Aust. J. Zool. 43, 119–127. doi: 10.1071/ZO9950119
Larkin P. D., Maloney T. J., Rubiano-Rincon S., Barrett M. M. (2017). A Map-Based Approach to Assessing Genetic Diversity, Structure, and Connectivity in the Seagrass Halodule Wrightii. Mar. Ecol. Prog. Ser. 567, 95–107. doi: 10.3354/meps12037
Larkin P., Schonacher T., Barrett M., Paturzzio M. (2012). Development and Characterization of Microsatellite Markers for the Seagrass Halodule Wrightii. Conserv. Genet. Resour. 4, 511–513. doi: 10.1007/s12686-011-9587-0
Manel S., Loiseau N., Andrello M., Fietz K., Goñi R., Forcada A., et al. (2019). Long-Distance Benefits of Marine Reserves: Myth or Reality? Trends Ecol. Evol. 34, 342–354. doi: 10.1016/j.tree.2019.01.002
Marshall C. D., Maeda H., Iwata M., Furuta M., Asano S., Rosas F., et al. (2003). Orofacial Morphology and Feeding Behaviour of the Dugong, Amazonian, West African and Antillean Manatees (Mammalia: Sirenia): Functional Morphology of the Muscular-Vibrissal Complex. J. Zool. 259, 245–260. doi: 10.1017/S0952836902003205
Martínez-Garrido J., Bermejo R., Serrão E. A., Sánchez-Lizaso J., González-Wangüemert M. (2017). Regional Genetic Structure in the Aquatic Macrophyte Ruppia Cirrhosa Suggests Dispersal by Waterbirds. Estuaries Coasts 40, 1705–1716. doi: 10.1007/s12237-017-0247-9
Massa S. I., Paulino C. M., Serrão E. A., Duarte C. M., Arnaud-Haond S. (2013). Entangled Effects of Allelic and Clonal (Genotypic) Richness in the Resistance and Resilience of Experimental Populations of the Seagrass Zostera Noltii to Diatom Invasion. BMC Ecol. 13, 39. doi: 10.1186/1472-6785-13-39
McMahon K., van Dijk K. J., Ruiz-Montoya L., Kendrick G. A., Krauss S. L., Waycott M., et al. (2014). The Movement Ecology of Seagrasses. Proc. R. Soc B Biol. Sci. 281, 20140878–20140879. doi: 10.1098/rspb.2014.0878
McMillan C. (1981). Seed Reserves and Seed Germination for Two Seagrasses, Halodule Wrightii and Syringodium Filiforme, From the Western Atlantic. Aquat. Bot. 11, 279–296. doi: 10.1016/0304-3770(81)90066-8
Meirmans P. G., Van Tienderen P. H. (2004). GENOTYPE and GENODIVE: Two Programs for the Analysis of Genetic Diversity of Asexual Organisms. Mol. Ecol. Notes 4, 792–794. doi: 10.1111/j.1471-8286.2004.00770.x
Michot T. C., Woodin M. C., Nault A. J. (2008). Food Habits of Redheads (Aythya Americana) Wintering in Seagrass Beds of Coastal Louisiana and Texas, USA. Acta Zool. Acad. Sci. Hungaricae 54, 239–250.
Nguyen V. X., Detcharoen M., Tuntiprapas P., Soe-Htun U., Sidik J. B., Harah M. Z., et al. (2014). Genetic Species Identification and Population Structure of Halophila (Hydrocharitaceae) From the Western Pacific to the Eastern Indian Ocean. BMC Evol. Biol. 14, 92. doi: 10.1186/1471-2148-14-92
Nicastro K. R., Assis J., Serrão E. A., Pearson G. A., Neiva J., Valero M., et al. (2020). Congruence Between Fine-Scale Genetic Breaks and Dispersal Potential in an Estuarine Seaweed Across Multiple Transition Zones. ICES J. Mar. Sci. 77, 371–378. doi: 10.1093/icesjms/fsz179
Nordlund L. M., Jackson E. L., Nakaoka M., Samper-Villarreal J., Beca-Carretero P., Creed J. C. (2018). Seagrass Ecosystem Services–What’s Next? Mar. Pollut. Bull. 134, 145–151. doi: 10.1016/j.marpolbul.2017.09.014
Nordlund L. M., Koch E. W., Barbier E. B., Creed J. C. (2016). Seagrass Ecosystem Services and Their Variability Across Genera and Geographical Regions. PloS One 11 (10), e0163091. doi: 10.1371/journal.pone.0163091
Ntuli N. N., Nicastro K. R., Zardi G. I., Assis J., McQuaid C. D., Teske P. R. (2020). Rejection of the Genetic Implications of the “Abundant Centre Hypothesis” in Marine Mussels. Sci. Rep. 10, 1–12. doi: 10.6084/m9.figshare.8910812
Olds A. D., Connolly R. M., Pitt K. A., Pittman S. J., Maxwell P. S., Huijbers C. M., et al. (2016). Quantifying the Conservation Value of Seascape Connectivity: A Global Synthesis. Glob. Ecol. Biogeogr. 25, 3–15. doi: 10.1111/geb.12388
Olsen J. L., Stam W. T., Coyer J. A., Reusch T. B. H., Billingham M., Boström C., et al. (2004). North Atlantic Phylogeography and Large-Scale Population Differentiation of the Seagrass Zostera Marina L. Mol. Ecol. 13, 1923–1941. doi: 10.1111/j.1365-294X.2004.02205.x
Orth R. J., Carruthers T. J.B., Dennison W. C., Duarte C. M., Fourqurean J. W., Heck K. L., et al. (2006a). A Global Crisis for Seagrass Ecosystems. Bioscience 56, 987–996. doi: 10.1641/0006-3568(2006)56
Orth R. J., Harwell M. C., Inglis G. J. (2006b). Ecology of Seagrass Beds Seeds and Dispersal Strategies. Seagrasses Biol. Ecol. Conserv. Eds. Larkum B. D., Orth R., Duarte C. (Dordrecht: Springer Press, Netherlands), 111–133. doi: 10.1007/1-4020-2983-7_5
Orth R. J., Harwell M. C., Inglis G. J. (2007). “Ecology of Seagrass Seeds and Seagrass Dispersal Processes,” in Seagrasses: Biology, Ecologyand Conservation. Eds. Larkum B. D., Orth R., Duarte C. (Dordrecht, Netherlands: Springer Press), 111–133. doi: 10.1007/978-1-4020-2983-7_5
Orth R. J., Luckenbach M., Moore K. A. (1994). Seed Dispersal in a Marine Macrophyte: Implications for Colonization and Restoration. Ecology 75, 1927–1939. doi: 10.2307/1941597
Palumbi S. (2003). Population Genetics, Demographic Connectivity, and the Design of Marine Reserves. Ecol. Appl. 13, 146–158. doi: 10.1890/1051-0761(2003)013[0146:PGDCAT]2.0.CO;2
Paulo D., Diekmann O., Ramos A. A., Alberto F., Serrão E. A. (2019). Sexual Reproduction vs. Clonal Propagation in the Recovery of a Seagrass Meadow After an Extreme Weather Event. Sci. Mar. 83, 357–363. doi: 10.3989/scimar.04843.06A
Philander S. G. (2001). Atlantic Ocean Equatorial Currents. Encycl. Ocean Sci. Book 1, 188–191. doi: 10.1006/rwos.2001.0361
Polidoro B. A., Ralph G. M., Strongin K., Harvey M., Carpenter K. E., Arnold R., et al. (2017). The Status of Marine Biodiversity in the Eastern Central Atlantic (West and Central Africa). Aquat. Conserv. Mar. Freshw. Ecosyst. 27, 1021–1034. doi: 10.1002/aqc.2744
Pritchard J. K., Stephens M., Donnelly P. (2000). Inference of Population Structure Using Multilocus Genotype Data. Genetics 155, 945–959. doi: 10.1093/genetics/155.2.945
R Development Core Team. (2021). R: A Language and Environment for Statistical Computing. R A Lang. Environ. Stat. Comput. R Found. Stat. Comput. Available at: https://www.r-project.org/.
Reynolds L. K., Tiling K. A., Digiantonio G. B., Encomio V. G., Morris L. J. (2019). Genetic Diversity of Halodule Wrightii is Resistant to Large Scale Dieback: A Case Study From the Indian River Lagoon. Conserv. Genet. 20, 1329–1337. doi: 10.1007/s10592-019-01214-z
Ridgway T., Riginos C., Davis J., Hoegh-Guldberg O. (2008). Genetic Connectivity Patterns of Pocillopora Verrucosa in Southern African Marine Protected Areas. Mar. Ecol. Prog. Ser. 354, 161–168. doi: 10.3354/meps07245
Roberts C. M., McClean C. J., Veron J. E. N., Hawkins J. P., Allen G. R., McAllister D. E., et al. (2002). Marine Biodiversity Hotspots and Conservation Priorities for Tropical Reefs. Science 295 (5558), 1280–1284. doi: 10.1126/science.1067728
Ruiz-Montoya L., Lowe R. J., Kendrick G. A. (2015). Contemporary Connectivity is Sustained by Windand Current-Driven Seed Dispersal Among Seagrass Meadows. Mov. Ecol. 3, 1–14. doi: 10.1186/s40462-015-0034-9
Ruiz-Montoya L., Lowe R. J., Niel V., Kendrick G. A. (2012). The Role of Hydrodynamics on Seed Dispersal in Seagrasses. Limnol. Oceanogr. 57, 1257–1265. doi: 10.4319/lo.2012.57.5.1257
Santos C. I. (2007). Comunidades De Macroinvertebrados E Peixes Associadas À Pradaria Marinha De Halodule Wrightii (Ascherson, 1868) Na Laguna do Mussulo, Angola. PhD Dissertation, Departamento de Biologia Animal da Faculdade de Ciências. (Lisboa: Universidade de Lisboa).
Short F. T., Short A., Novak C. A. (2016). Seagrasses. In: The Wetland Book: II: Distribution, Description and Conservation. Eds. Finlayson C. M., Milton G. R., Prentice R. C., Davidson N. C. (Netherlands: Springer Science), 1–19. doi: 10.1007/978-94-007-6173-5_262-1
Spalding M. D., Fox H. E., Allen G. R., Davidson N., Ferdaña Z. A., Finlayson M. A. X., et al. (2007). Marine Ecoregions of the World: A Bioregionalization of Coastal and Shelf Areas. Bioscience 57, 573–583. doi: 10.1641/B570707
Spalding M., Taylor M., Ravilious C., Short F., Green F. (2003). “Global Overview: The Distribution and Status of Seagrasses,” in World Atlas of Seagrasses (University of California Press), 1, 52.
Sumoski S. E., Orth R. J. (2012). Biotic Dispersal in Eelgrass Zostera Marina. Mar. Ecol. Prog. Ser. 471, 1–10. doi: 10.3354/meps10145
Tanaka N., Demise T., Ishii M., Shoji Y., Nakaoka M. (2011). Genetic Structure and Gene Flow of Eelgrass Zostera Marina Populations in Tokyo Bay, Japan: Implications for Their Restoration. Mar. Biol. 158, 871–882. doi: 10.1007/s00227-010-1614-2
Teschima M. M., Zilberberg C., Nunes F. L. D. (2021). Strong Genetic Differentiation Demarks Populations of Favia Across Biogeographic Regions of the Atlantic Ocean. Coral Reefs, 1–12. doi: 10.1007/s00338-021-02203-w
Thiel M., Haye P. A. (2006). The Ecology of Rafting in the Marine Environment. III. Biogeographical and Evolutionary Consequences. Oceanogr. Mar. Biol. 44, 323–429. doi: 10.1201/9780203507810.ch6
Tilquin A., Kokko H. (2016). What Does the Geography of Parthenogenesis Teach Us About Sex? Philos. Trans. R. Soc B Biol. Sci. 371, 20150538. doi: 10.1098/rstb.2015.0538
Tol S. J., Jarvis J. C., York P. H., Grech A., Congdon B. C., Coles R. G. (2017). Long Distance Biotic Dispersal of Tropical Seagrass Seeds by Marine Mega-Herbivores. Sci. Rep. 7, 1–8. doi: 10.1038/s41598-017-04421-1
Ukwe C. N., Ibe C. A., Alo B. I., Yumkella K. K. (2003). Achieving a Paradigm Shift in Environmental and Living Resources Management in the Gulf of Guinea: The Large Marine Ecosystem Approach. Mar. Pollut. Bull. 47, 219–225. doi: 10.1016/S0025-326X(02)00473-3
Ukwe C. N., Ibe C. A., Nwilo P. C., Huidobro P. A. (2006). Contributing to the WSSD Targets on Oceans and Coasts in West and Central Africa : The Guinea Current Large Marine Ecosystem Project. Int. J. Ocean Oceanogr. 1, 21–44.
van Dijk K., Bricker E., van Tussenbroek B. I., Waycott M. (2018). Range-Wide Population Genetic Structure of the Caribbean Marine Angiosperm Thalassia Testudinum. Ecol. Evol. 8, 9478–9490. doi: 10.1002/ece3.4443
Van Oosterhout C., Hutchinson W. F., Wills D. P. M., Shipley P. (2004). MICRO-CHECKER: Software for Identifying and Correcting Genotyping Errors in Microsatellite Data. Mol. Ecol. Notes 4, 535–538. doi: 10.1111/j.1471-8286.2004.00684.x
Van Tussenbroek B. I., Márquez-Guzman J., Wong R. (2009). Phenology of Marine Angiosperms (Seagrasses): Reproductive Synchrony in the Sea. Funct. Divers. Plant Reprod. 17–46.
Verduin J. J., Backhaus J. O., Walker D. I. (2002). Estimates of Pollen Dispersal and Capture Within Amphibolis Antarctica (Labill.) Sonder and Aschers. Ex Aschers. Meadows. Bull. Mar. Sci. 71, 1269–1277.
Waycott M., Duarte C. M., Carruthers T. J. B., Orth R. J., Dennison W. C., Olyarnik S., et al. (2009). Accelerating Loss of Seagrasses Across the Globe Threatens Coastal Ecosystems. Proc. Natl. Acad. Sci. 106, 12377–12381. doi: 10.1073/pnas.0905620106
Keywords: Western Africa, seagrass, Halodule wrightii, population connectivity, dispersal
Citation: Tavares AI, Assis J, Patrício AR, Ferreira R, Cheikh MAS, Bandeira S, Regalla A, Santos I, Potouroglou M, Nicolau S, Teodósio MA, Almada C, Santos R, Pearson GA and Serrao EA (2022) Seagrass Connectivity on the West Coast of Africa Supports the Hypothesis of Grazer-Mediated Seed Dispersal. Front. Mar. Sci. 9:809721. doi: 10.3389/fmars.2022.809721
Received: 05 November 2021; Accepted: 03 May 2022;
Published: 06 June 2022.
Edited by:
Sophie von der Heyden, Stellenbosch University, South AfricaCopyright © 2022 Tavares, Assis, Patrício, Ferreira, Cheikh, Bandeira, Regalla, Santos, Potouroglou, Nicolau, Teodósio, Almada, Santos, Pearson and Serrao. This is an open-access article distributed under the terms of the Creative Commons Attribution License (CC BY). The use, distribution or reproduction in other forums is permitted, provided the original author(s) and the copyright owner(s) are credited and that the original publication in this journal is cited, in accordance with accepted academic practice. No use, distribution or reproduction is permitted which does not comply with these terms.
*Correspondence: Ana I. Tavares, aimtavares@ualg.pt;Ester A. Serrao, eserrao@ualg.pt